- 1College of Physics, Guizhou Province Key Laboratory for Photoelectrics Technology and Application, Guizhou University, Guiyang, China
- 2Key Laboratory of Electronic Composites of Guizhou Province, Guizhou University, Guiyang, China
- 3National Laboratory of Solid State Microstructures and Jiangsu Provincial Laboratory for NanoTechnology, Nanjing University, Nanjing, China
In this research, three-dimensional (3D) hierarchical networks of magnetic carbon nanotubes/graphene oxide (CNTs/GO) nanocomposites (NCs) were synthesized by a one-step hydrothermal method with the idea of constructing more interface contacts, a double loss mechanism and synergy effect. The obtained results showed that the content of NCs gradually transitioned from CoCO3 to CoxFe3–xO4. Further, CoCO3-CoxFe3–xO4/CNTs/GO quaternary NCs and CoxFe3–xO4/CNTs/GO ternary NCs could be selectively synthesized when the Co:Fe molar ratio decreased from 1:1 to 1:3. The as-prepared samples displayed excellent microwave absorption properties (MAPs) in terms of the minimum reflection loss (RLmin) and the corresponding absorption bandwidth (FBcor) with reflection loss values lower than −10 dB. The RLmin and large FBcor values for the as-prepared samples were −54.69 dB and 2.36 GHz, −63.99 dB and 2.48 GHz, −63.36 dB and 2.68 GHz, respectively. The designed 3D hierarchical networked structure of magnetic GO/CNTs NCs displayed outstanding comprehensive MAPs, which could deliver a practical and effective strategy to develop high-performance microwave absorbers (MAs), considering their low cost, lightweight, and high stability.
Introduction
In the past decades, microwave absorbers (MAs) have drawn significant attention owing to the expanded electromagnetic (EM) radiation and interference problems (Novoselov et al., 2004; Zhang et al., 2014; Cao et al., 2019). It is well acknowledged that MAs with characteristics such as strong absorption ability, large absorption bandwidth, lightweight, low cost, and remarkable chemical stability are highly desirable because of their promising military and civil application prospects (Zhang et al., 2015; Fang et al., 2016). According to the EM energy conversion principle, the incident EM wave can be attenuated by dielectric and magnetic losses (Wei et al., 2017). Therefore, nanocomposites (NCs) consisting of dielectric loss materials and magnetic nanoparticles (NPs) are considered an effective way to achieve high-performance MAs (Liu et al., 2015, 2018; Quan et al., 2017). For example, CoNi@Air@TiO2 (Liu et al., 2016), Co0.6Fe2.4O4@MoS2 (Long et al., 2019), Ni@SnO2 (Zhao et al., 2016), Co7Fe3@SiO2 (Chen et al., 2017) have been demonstrated to display outstanding microwave absorption properties (MAPs). Generally, owing to their excellent synergistic effect, the development of high-performance MAs mainly focused on core@shell structure magnetic NCs, in which dielectric substances are utilized as a shell and magnetic NPs as a core. Among them, different dielectric substances such as ZnO, TiO2, and BaTiO3 were often used as a shell (Liu et al., 2008; Jiang et al., 2014; Xu et al., 2018). However, the biggest drawback of these NCs is the high density, which hugely restricts their practical application.
In recent years, owing to the lightweight, unique tube structure and excellent dielectric loss, carbon nanotubes (CNTs) were used as substrate to construct the CNTs-based for designing the ideal MAs (Chen et al., 2015; Wang et al., 2018). Examples include Fe/CNTs (Che et al., 2004), ZnFe2O4/CNTs (Khabouri et al., 2015), and Fe/Co/Ni/CNTs (Wen et al., 2011), which were demonstrated to display very outstanding MAPs. Generally, the previously designed core@shell structure CNTs-based NCs were mainly composed of CNTs as a shell and different categories of magnetic NPs as a core (Lv et al., 2008; Weissker et al., 2010). However, the magnetic properties and magnetic loss abilities for these kinds of CNTs-based NCs are hugely limited and cannot be well modulated due to the magnetic NPs acting as the core, and the low encapsulation of magnetic NPs (Pawar et al., 2019; Long et al., 2020). Therefore, constructing the reverse core@shell structure magnetic CNTs-based NCs, in which magnetic NPs serve as the shell and CNTs as the core, is an effective way to overcome these problems (Wang et al., 2012; Li et al., 2017).
Furthermore, the recent results indicated that the outstanding properties of graphene (G) and the designed G-based could provide many more interfaces, which were conducive to improve MAPs (Qu et al., 2016; Wang et al., 2019). Considering these aspects, in this article, we designed and synthesized magnetic CNTs/graphene oxide (GO) NCs by a one-step hydrothermal method. By changing the Co:Fe molar ratio, different compositions of magnetic NPs/CNTs/GO NCs could be selectively produced. The obtained results showed that the as-prepared magnetic CNTs/GO NCs displayed very excellent MAPs.
Experimental
Synthesis of Magnetic CNTs/GO NCs
Similarly to the previously reported experimental method shown in Figure 1 (Wu et al., 2020), 80 mg of GO was prepared by a modified Hummers method (Hummers and Offeman, 1958). The prepared GO was added in 40 mL deionized water and ultrasonicated for 30 min at room temperature (RT). Next, 40 mg of hydroxylated CNTs were ultrasonically dispersed into the GO solution for another 30 min to obtain the GO/CNTs substrate solution. Then, the solution of Co2+ and Fe3+ was obtained by ultrasonic dissolving C4H6CoO4∙4H2O (1 mmol) and FeCl3∙6H2O (1 mmol) into a 30 mL ethylene glycol solution. Subsequently, the resulting Co2+ and Fe3+ solution was dissolved into GO/CNTs substrate solution by stirred for ca. 60 min. Finally, urea (0.9 g) were thoroughly dispersed into the mixed solution for another 5 min. The final solution was transferred into a 100 mL Teflon-lined stainless steel autoclave and heated at 200°C for 24 h and left to cool to RT. The synthesized magnetic CNTs/GO NCs were washed several times and magnetically centrifuged. The obtained sample was left to dry. The experimental conditions were kept the same, with the exception of 2 and 3 mmol of FeCl3∙6H2O, which were used as the Fe source in the synthesis of magnetic NPs/CNTs/GO NCs to control compositions of the sample. The samples were denoted as S1, S2, and S3 for easy description, respectively.
Characterization and Measurement
The phases and microstructures of the as-prepared magnetic CNTs/GO NCs were characterized by an X-ray powder diffractometer (XRD) (model Smart Lab, Rigaku), Raman spectroscopy (Jobin-Yvon Labram HR800), Thermal gravity analysis (TGA) (Netzsch Sta 449F3), transmission electron microscope (TEM) (model Tecnai-G20), and X-ray photoelectron spectroscopy (XPS) (model Escalab 250Xi, Thermo Fisher Scientific). 30 wt% of the obtained samples were mixed with wax and pressed into toroidal shaped samples (Rout: 7.0 mm, Rin: 3.0 mm), respectively, to investigate the EM properties and MAPs. The as-prepared composites were measured by a vector network analyzer (Agilent E8363B) in the frequency region of 2–18 GHz.
Results and Discussion
Figure 2 presents the XRD patterns and Raman spectra of the as-prepared magnetic CNTs/GO NCs. Figure 2A shows that all the as-prepared samples display similar XRD patterns, pointing to the same category of sample. The characteristic peaks at 30.08°, 35.44°, 43.06°, 53.44°, 56.97° and 62.59° corresponding to (220), (311), (400), (422), (511), and (440) crystal planes are consistent with the standard XRD patterns of spinel CoFe2O4 (JCPDS: 22-1086). In comparison, one peak at 32.6° can be observed clearly over S1 and S2, assigned to (104) crystal planes of CoCO3 (JCPDS: 78-0209). However, this peak cannot be seen over sample S3. The comparison results reveal that the obtained samples evolve from CoCO3 to CoxFe3–xO4 when the amount of Fe source increases from 1 mmol to 3 mmol. In confirmation of the results, the experimental conditions were kept the same, with the exception of 5 mmol FeCl3∙6H2O, which was used as the Fe source to produce the sample, S4 for easy description. As shown in Figure 2A, the XRD pattern indicates that the phase of CoCO3 cannot be observed over the as-prepared S4, which is consistent with our previous reported result (Wu et al., 2020). Moreover, consistent with the GO/CNT-Fe3O4 NCs reported elsewhere (Wang et al., 2014), the characteristic diffraction peaks for GO and CNTs cannot be seen, which should be attributed to the uniform dispersion of CoxFe3–xO4 NPs on the surface of CNTs/GO. The existence of carbon materials can be confirmed by Raman spectra. As shown in Figure 2B, the obtained magnetic CNTs/GO NCs show three evident peaks located at 1347 cm–1, 1586 cm–1, and 2694 cm–1, respectively. These peaks can correspond to the D band, G band and 2D band of carbon material, respectively. It is well-known that the D band originates from the sp3 defect/disorder in the graphitic structure, the G band is indicative of a high crystallinity graphitic layer, and the 2D band is excited by a double-resonant Raman process (Ritter et al., 2006; Popov and Lambin, 2013). Based on the obtained results, we can confirm the existence of GO and CNTs in the obtained NCs.
Figure 3 shows the typical TGA curves and XPS spectra of as-prepared samples. As shown in Figure 3A, it can be seen that the obtained S2 and S4 samples present the evident weight loss under air atmosphere in the temperature range of 240–620°C. Based on the XRD results, the mass loss can mainly be ascribed to the thermal decomposition of GO and CNTs, and the residual substances should be assigned to CoxFe3–xO4 NPs. The TGA results indicate that the CoxFe3–xO4 content in the obtained S2 and S4 samples is 18.4% and 30.4%, respectively. To confirm the composition of the obtained samples, Figure 3B provides their XPS spectra. It can be deduced that all the obtained NCs have four peaks located at 284, 530, 712, and 781 eV, respectively. Further, these peaks can be assigned to C 1s, O 1s, Fe 2p, and Co 2p.
To obtain the chemical valence states of Fe and Co, Figure 4A provides the high-resolution XPS spectra of Fe 2p. Four peaks, which are at ca. 711.2, 719.3, 724.6, and 731.4 eV, respectively, can be observed. Among these peaks, 711.2 and 724.6 eV can be assigned to the Fe 2p3/2 and Fe 2p1/2, while the other peaks correspond to the satellite peaks of Fe 2p3/2 and Fe 2p1/2. Similarly to the previous result (Yamashita and Hayes, 2008), the obtained result suggests the presence of Fe3+ in the obtained samples. As indicated in Figure 4B, the Co 2p high-resolution spectra also display four evident peaks. The peaks located at 780.9 and 796.6 eV originate from Co 2p3/2 and Co 2p1/2, and the peaks at 786.8 and 803.3 eV are the satellite peaks of Co 2p3/2 and Co 2p1/2, which indicate the existence of Co2+ ions (Bennet et al., 2016). In general, the obtained XPS results are consistent with their XRD patterns.
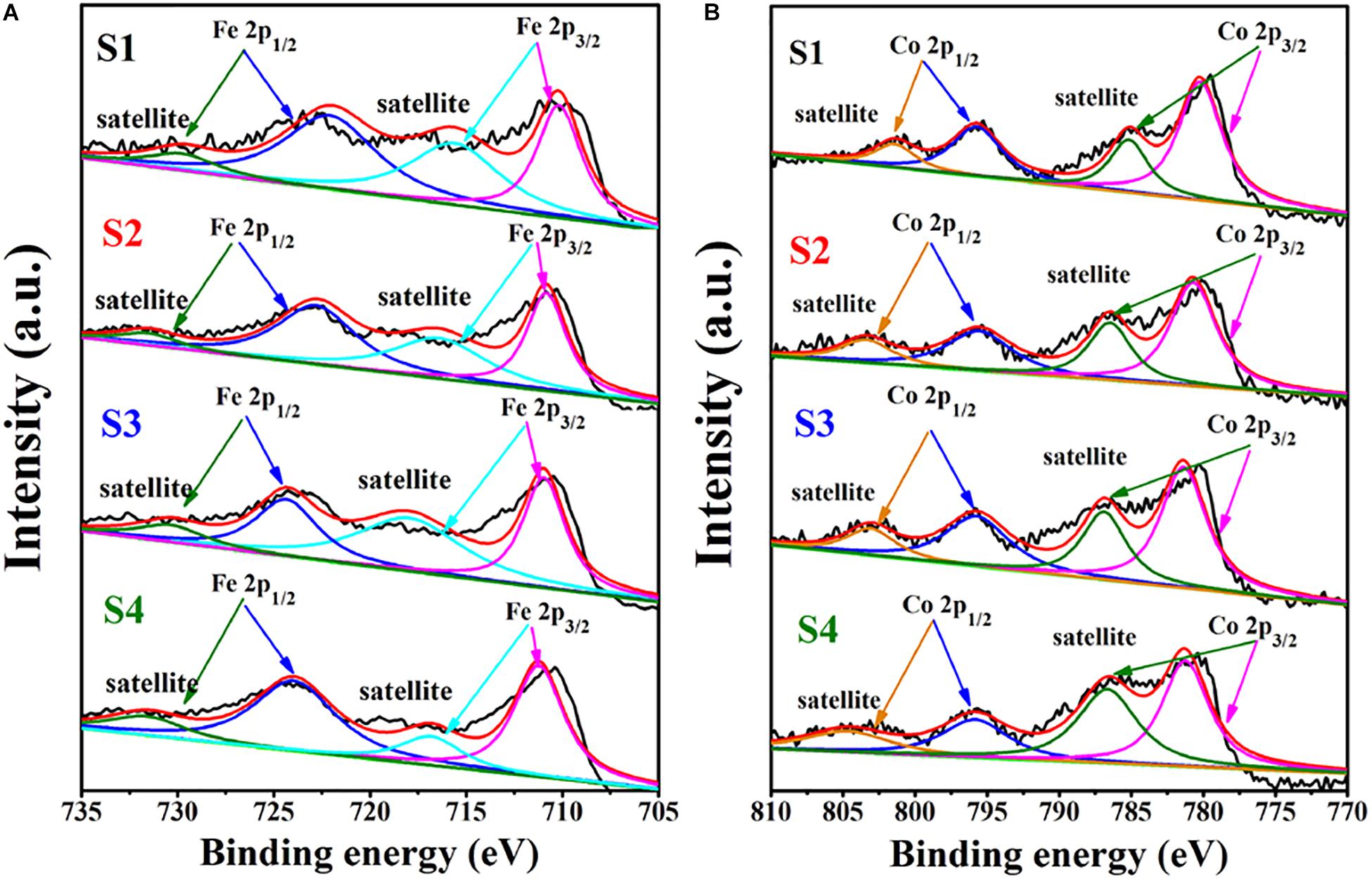
Figure 4. High resolution XPS spectra of (A) Fe 2p, and (B) Co 2p peaks for the as-prepared samples.
Figure 5 provides the morphologies of the as-prepared S1 and S2. As shown in Figure 5a,b it can be observed that larger quantities of CoxFe3–xO4 and CoCO3 NPs attached on the surface of GO and CNTs, similarly to the reported GO/CNT-Fe3O4 NCs (Wang et al., 2014). A closer TEM observation indicates that the NPs, CNTs, and GO nanosheets are well connected with each other to form a good three dimensional (3D) hierarchical networks structure. In the same manner as S1, as displayed in Figures 5c,d, the obtained S2 consists of large numbers of NPs, CNTs and paper-like GO, which presents a typical structure of 3D hierarchical networks. Combined with the XRD and XPS results, the conclusion suggests that the as-prepared S1 and S2 are 3D hierarchical networks structure of CoCO3-CoxFe3–xO4/CNTs/GO quaternary NCs. Equally, the TEM investigation of S3 and S4 (as presented in Figure 6) shows that they are composed of CoxFe3–xO4 NPs with a relatively uniform size, CNTs and GO nanosheets, which bind together to establish the structure of 3D hierarchical networks. Unlike S1 and S2, the XRD, XPS and TEM results demonstrate that the as-prepared S3 and S4 are 3D hierarchical networks structure of CoxFe3–xO4/CNTs/GO ternary NCs. Generally, with the Co:Fe increasing from 1:1 to 1:5, it is discovered that the prepared samples gradually undergo the transition from CoCO3-CoxFe3–xO4/CNTs/GO quaternary NCs to CoxFe3–xO4/CNTs/GO ternary NCs, which is in agreement with our reported results (Wu et al., 2020). Compared to the binary NCs such as CoxFe3–xO4/CNTs and CoxFe3–xO4/GO, it is well recognized that the formed CoCO3-CoxFe3–xO4/CNTs/GO quaternary NCs and CoxFe3–xO4/CNTs/GO ternary NCs can provide many more interface contacts. Further, the generated 3D hierarchical networks structure of our designed magnetic CNTs/GO NCs can provide a double loss mechanism and synergy effect among them, which is conducive to the attenuation of the EM wave. Generally, based on the previously reported papers and our obtained results (Lu et al., 2018; Shi et al., 2018), one can find that CoCO3 will easily form under the condition of the existence of urea and a small quantity of Fe source. With the increasing amounts of Fe source, much more Co2+ will couple with Fe3+ to generate the CoxFe3–xO4. Therefore, the obtained S2 should display a much higher content of CNTs/GO compared to S1. Moreover, with the formation of CoxFe3–xO4 with large quantities, the obtained S3 has a larger CoxFe3–xO4 content compared to S2.
It is well known that the value of minimum reflection loss (RLmin) and its corresponding frequency bandwidth (FBcor), with the reflection loss (RL) values lower than −10 dB (90% EM wave absorption) are the important characteristic for measuring high performance MAs (Ye et al., 2018). Further, it is very desirable that MAs can simultaneously have a low RLmin and large FBcor values. To investigate their MAPs, the values of RL were calculated based on the measured EM parameters and transmission line theory (Yusoff et al., 2002):
Where f is the frequency of the EM wave, d is the thickness of absorber, c is the velocity of light, Z0 is the impedance of air, and Zin is the input impedance of absorber. Figure 7 provides the RL curves of S1, S2, and S3. As shown in Figures 7A,B, the RLmin and large FBcor values for S1 are −54.69 dB at 11.08 GHz with the matching thickness (dm) value of 8.57 mm and 2.36 GHz. The as-prepared S2 presents the RLmin value of −63.99 dB at 14.96 GHz with the dm value of 6.60 mm (Figure 7C), and the FBcor value of 2.48 GHz (Figure 7D), respectively. Equally, as shown in Figures 7E,F, one can find that the RLmin value for S3 is ca. −63.36 dB at 13.12 GHz with the dm value of 7.95 mm, and its FBcor value is 2.68 GHz. Moreover, the corresponding maximum absorption bandwidths (ABmax) with RL < −10 dB for S1, S2, and S3 (as provided in Figure 8) are 2.60 GHz with the dm value of 7.69 mm, 2.72 GHz with the dm value of 6.00 mm, and 3.08 GHz with the dm value of 6.33 mm, respectively. In general, the designed magnetic CNTs/GO NCs display very outstanding MAPs. The as-prepared S3 exhibits the superior comprehensive MAPs compared to S1 and S2. As summarized in Figure 9, the proposed magnetic CNTs/GO NCs present excellent comprehensive MAPs compared to the representative related NCs reported previously (Li et al., 2015; Yang et al., 2016; Zhang et al., 2016; Zhang K.C. et al., 2017; Zhang S. et al., 2017; Ren et al., 2018; Shu et al., 2018a, b; Hu et al., 2019; Lu et al., 2019; Xu et al., 2019).
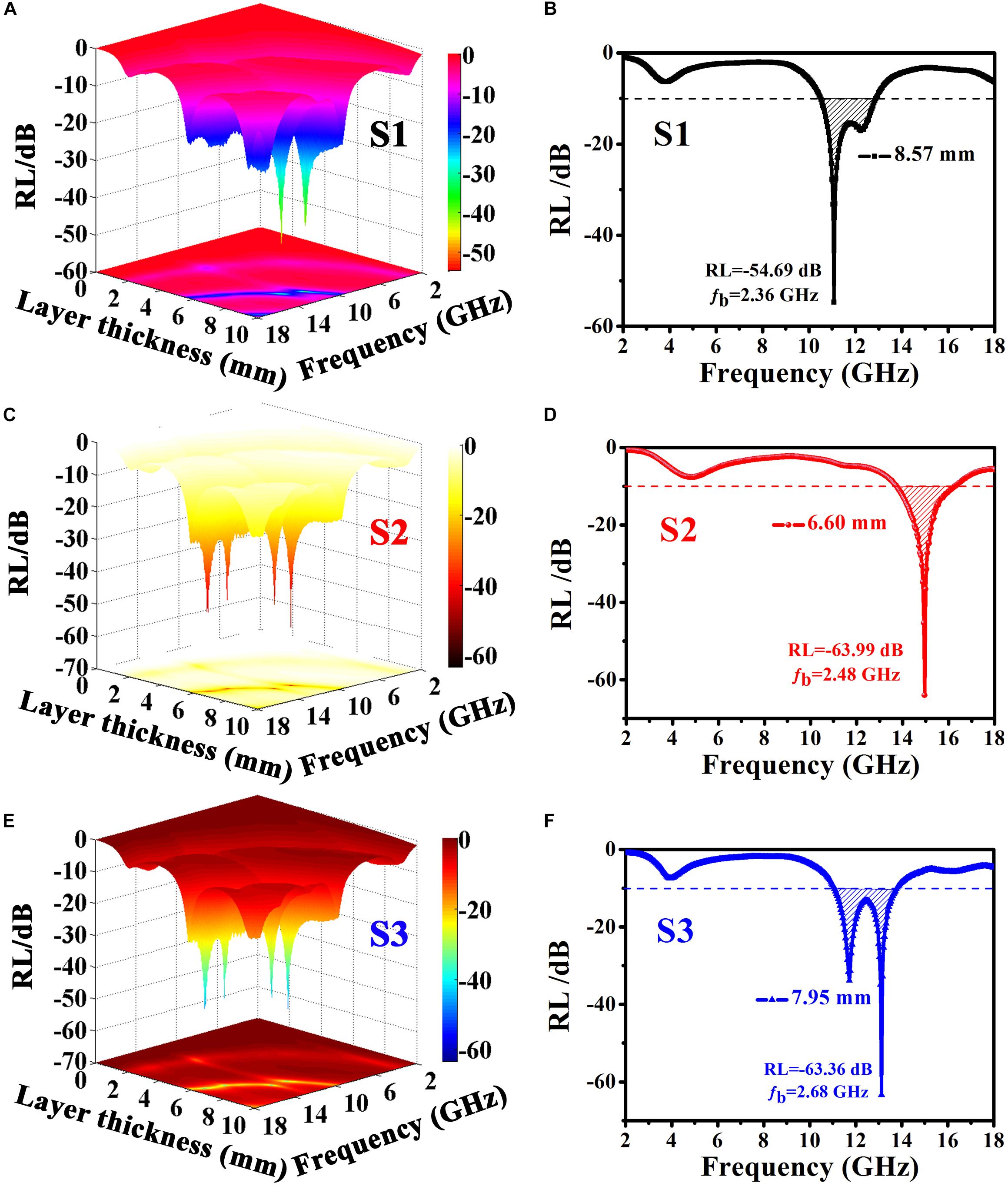
Figure 7. 3D color RL curves and their corresponding RLmin plots of (A,B) S1, (C,D) S2, and (E,F) S3.
To effectively understand their MAPs, Figure 10 gives their complex permittivity (ε = ε′−jε″) and complex permeability (μ = μ′−jμ″). As shown in Figures 10A,B, besides the shift of peak at ca. 13 GHz, the as-prepared samples present the similar ε′ and ε″ variation tendency in the frequency region owing to the same categories of samples. The complex permittivity exhibits several resonant peaks in the whole frequency range, indicating the multiple relaxation processes (Huang et al., 2019). Furthermore, the ε′ and ε″ values of samples present the decreased tendency with the frequency increasing from 2 to 18 GHz, which can be explained by the Debye theory (Wang et al., 2015). Overall, the obtained S2 has larger values of ε′ and ε″ compared to S1 and S3, which can be ascribed to the highest content of CNTs/GO in the as-prepared S2. According to the previous result (Li et al., 2017), the ε′ and ε″ values could be effectively enhanced by increasing the content of CNTs. The obtained S3 presents the lowest values of ε′ and ε″, which should be ascribed to the gradual formation of CoxFe3–xO4 with large quantities in NCs resulting in the decreasing content of CNTs/GO. Figures 10C,D present the μ′ and μ″ values of the obtained samples. Generally, it can be observed that the obtained samples exhibit the gradually growing μ′ and μ″ values with increased addition of Fe sources, which can be related to the enhanced content of CoxFe3–xO4 NPs in the NCs. Figure 11 provides the dielectric loss (tanδE = ε″/ε′) and magnetic capabilities (tanδm = μ″/μ′). Overall, the as-prepared magnetic CNTs/GO NCs display much higher tanδEvalues than those of tanδm, which indicates that the attenuation of wave EM mainly originates from dielectric loss (Feng et al., 2020; Li et al., 2020). Moreover, the obtained S2 displays larger values of tanδE compared to S1 and S3. Further, the large tanδm values of S3 are higher than those of S1 and S2. The difference in dielectric loss and magnetic loss abilities should be related to the different CNTs/GO and magnetic CoxFe3–xO4 NPs contents.
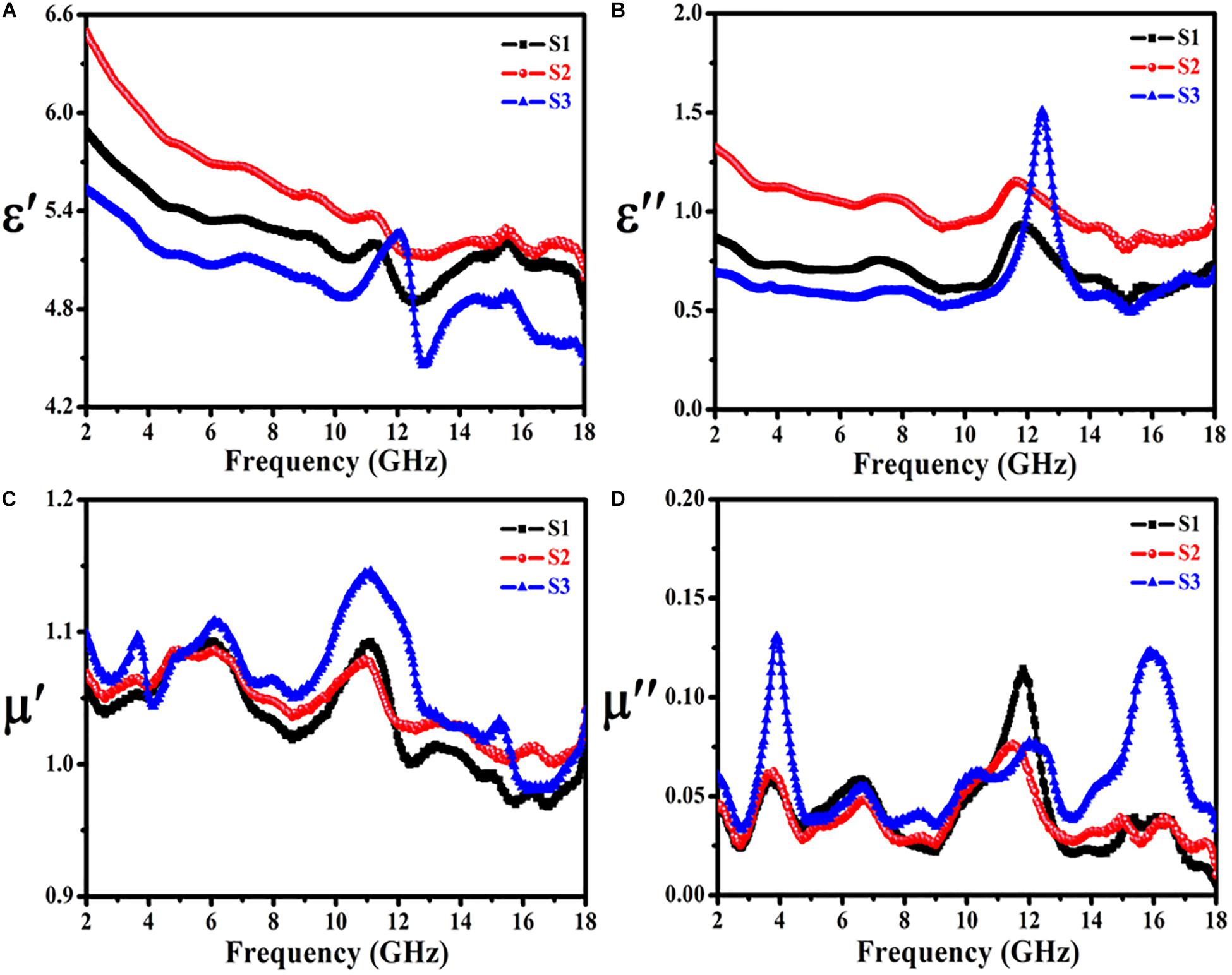
Figure 10. EM parameters for the as-prepared samples: (A,B) complex permittivity, and (C,D) complex permeability.
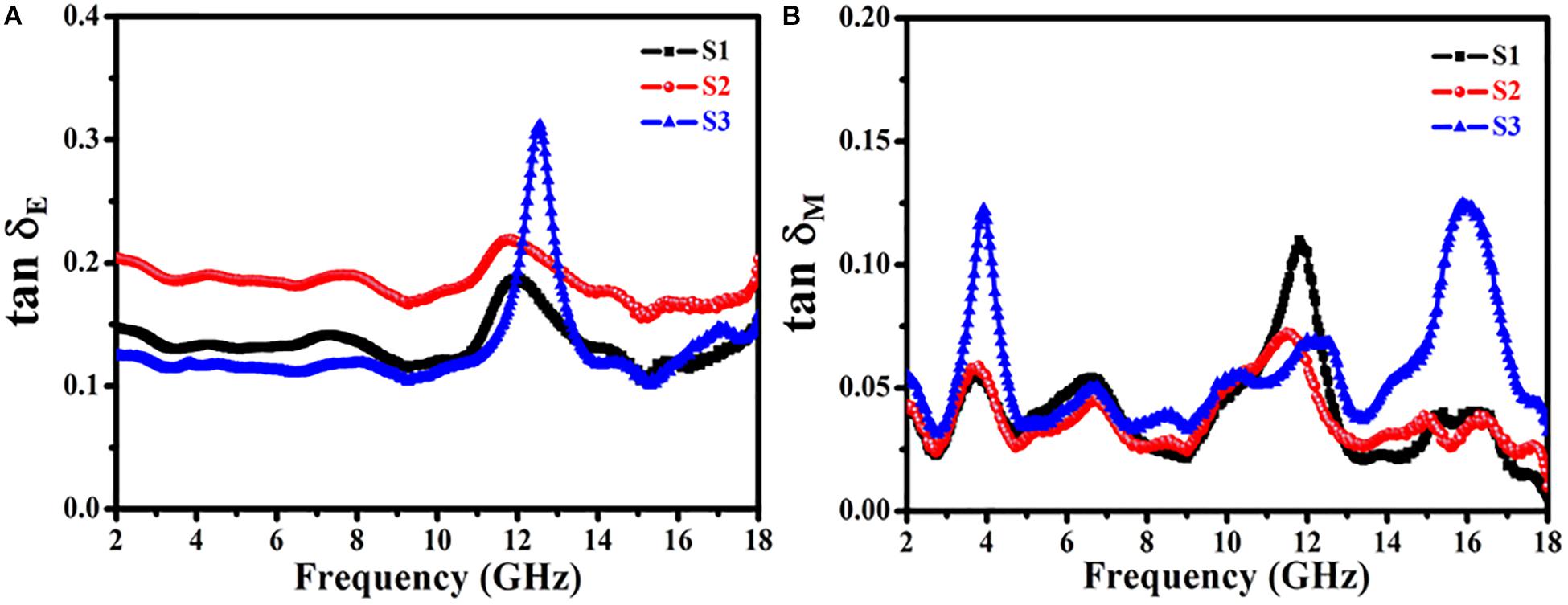
Figure 11. Frequency dependence (A) dielectric loss tangent, and (B) magnetic loss tangent of the as-prepared samples.
Based on the obtained EM parameters, RL curves and the previously reported mechanisms for the interpretation of the good MAPs (Liu et al., 2012, 2020), the excellent MAPs of the as-prepared magnetic CNTs/GO NCs can be explained by the geometrical effect. For this model, the values of dm and peak frequency (fm) should satisfy the 1/4 wavelength equation (Liu et al., 2020):
Figure 12 provides their comparison results between the experimental value of (obtaining directly from their RL curves) and their theoretical curves of [plotting by the formula (3)]. One can find that the scattered dots of land on the theoretical curves of . Consistent with the CoNi@PRM-NC, ZnFe2O4@C, and CNTs@MoS2 reported elsewhere (Gao et al., 2019; Yan et al., 2019; Wang et al., 2020), the agreement between the experimental and theoretical values suggests the cancelation effect of reflected EM waves and a strong EM wave attenuation.
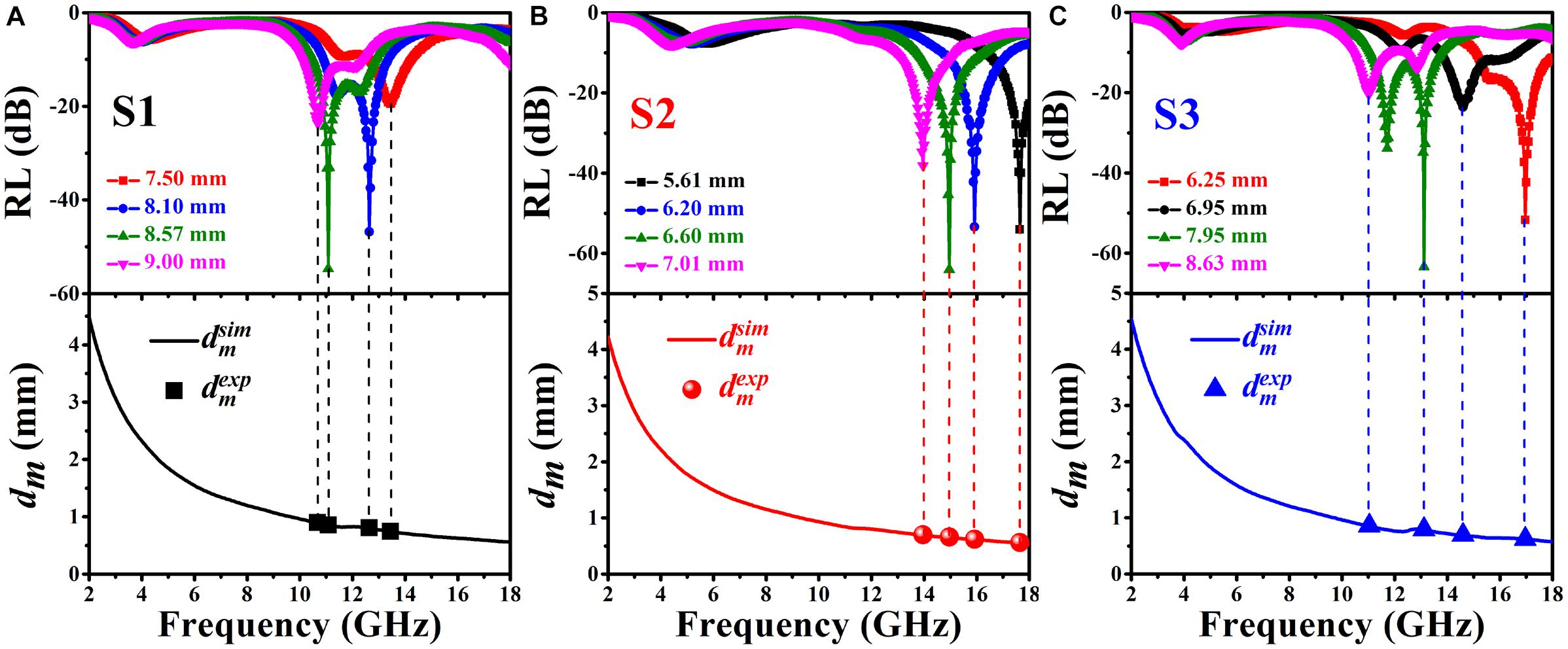
Figure 12. Comparison results between the calculated and experimental matching thicknesses for (A) S1, (B) S2, and (C) S3.
Based on the previous models and our obtained results (Wang et al., 2014; Shu et al., 2018a), as depicted in Figure 13, as for our designed magnetic CNTs/GO NCs, the possible paths for the EM wave attenuation are mainly attributed to the following facts: (i) the 3D hierarchical networks structure results in the multiple reflections and/or scattering of the EM wave (Figure 13A), which plays a significant role in the EM wave attenuation; (ii) many more interface contacts among magnetic NPs, CNTs, and GO nanosheets (Figure 13B) induce the formation of interface polarization with large quantities, which can effectively dissipate the energy of the incident EM wave; (iii) the designed NCs consisted of dielectric substances and magnetic NPs (Figure 13C), which can simultaneously provide the dielectric and magnetic loss mechanism. The excellent synergistic effect between them further improves the attenuation of the EM wave; (iv) the high conductivity of GO/CNT and a large number of defects and functional groups existing on CNTs (Figure 13B) and GO (Figure 13D) can generate massive electrons, defect dipoles and interface polarizations, which are also conducive of the dissipation of the EM wave.
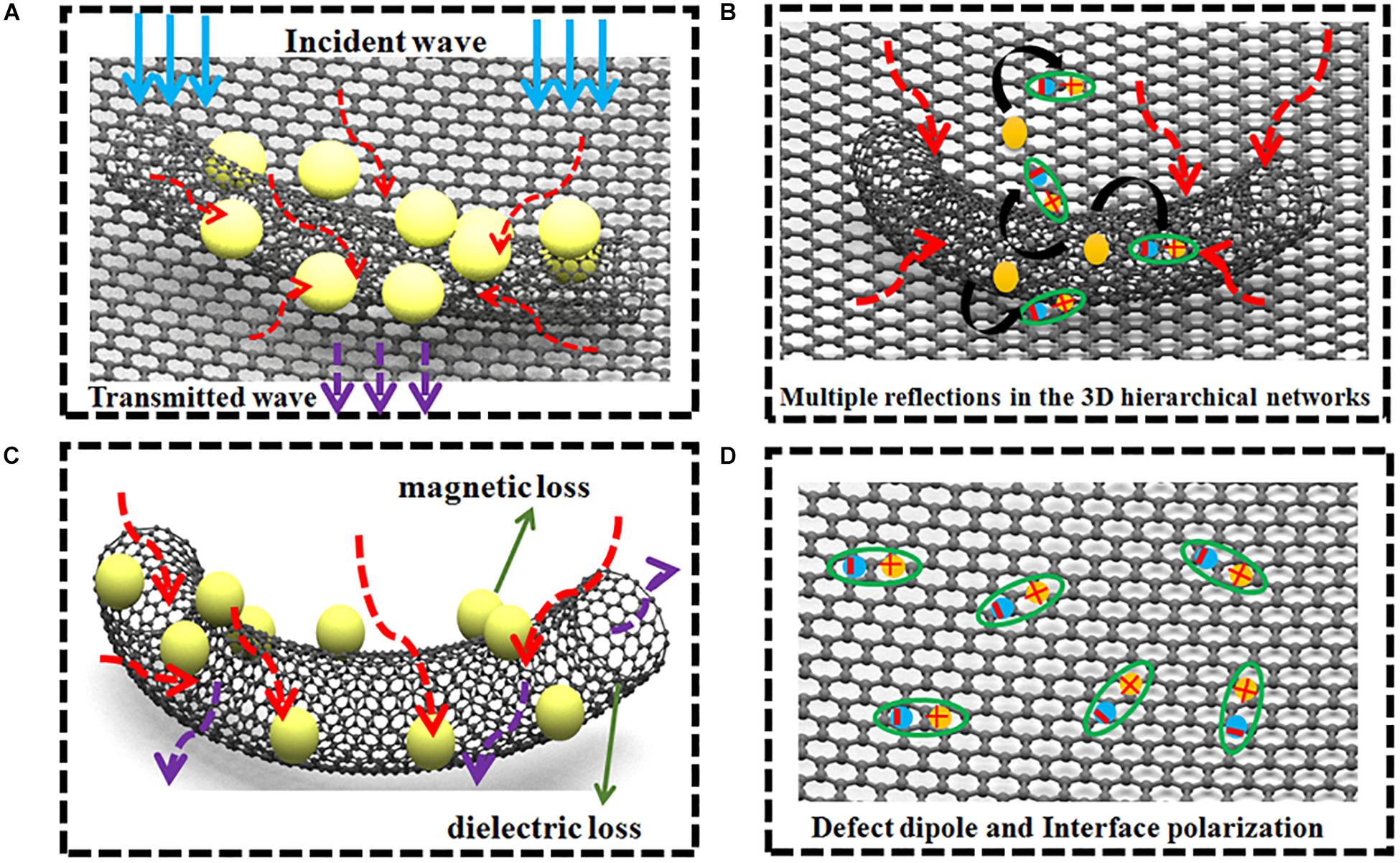
Figure 13. Schematic illustration of possible EM wave absorption avenues of magnetic CNTs/GO NCs. (A,B) Multiple reflections, (C) dielectric and magnetic loss, and (D) defect dipole and interface polarization.
Conclusion
In summary, we proposed a one-step hydrothermal method to selectively synthesize different compositions of magnetic CNTs/GO NCs such as CoCO3-CoxFe3–xO4/CNTs/GO quaternary NCs and CoxFe3–xO4/CNTs/GO ternary NCs. The TEM investigation indicated that the designed NC consisted of CoCO3-CoxFe3–xO4 NPs, CNTs, and GO nanosheets, which were well bound together to form the good 3D hierarchical networks structure. Owing to the construction of many more interface contacts among magnetic NPs, CNTs, and GO nanosheets, an excellent synergistic effect between dielectric and magnetic loss substances, the as-prepared magnetic CNTs/GO NCs presented very extraordinary MAPs. The RLmin and FBcor values for the as-prepared samples were ca. −54.69 dB and 2.36 GHz, −63.99 dB and 2.48 GHz, −63.36 dB and 2.68 GHz, respectively. Moreover, owing to their low cost, lightweight and high stability, the proposed 3D hierarchical networks structures of magnetic GO/CNTs NCs exhibit very outstanding comprehensive MAPs, which may be used as promising candidates for high performance MAs and are very much worth further study.
Data Availability Statement
The raw data supporting the conclusions of this article will be made available by the authors, without undue reservation, to any qualified researcher.
Author Contributions
MW contributed to the conceptualization, methodology, investigation and provided resources. XQ contributed to the conceptualization, methodology and writing of the original draft of the manuscript. RX contributed to the investigation. SQ provided supervision, and contributed to the writing, review and editing of the manuscript. CD provided formal analysis and resources. WZ contributed to the methodology, and the writing, review and editing of the manuscript.
Funding
This work was supported by the Fund of Fok Ying Tung Education Foundation, the Platform of Science and Technology and Talent Team Plan of Guizhou Province (2017-5610 and 2017-5788), the Major Research Project of Innovative Group of Guizhou Province (2018-013), the National Science Foundation of Guizhou Province (2017-1034), and the National Natural Science Foundation of China (Grant Nos. 11474151, 11604060, and 11964006) for financial support.
Conflict of Interest
The authors declare that the research was conducted in the absence of any commercial or financial relationships that could be construed as a potential conflict of interest.
References
Bennet, J., Tholkappiyan, R., Vishista, K., Jaya, N. V., and Hamed, F. (2016). Attestation in self-propagating combustion approach of spinel AFe(2)O(4) (A = Co, Mg and Mn) complexes bearing mixed oxidation states: magnetostructural properties. App. Sur. Sci. 383, 113–125. doi: 10.1016/j.apsusc.2016.04.177
Cao, M. S., Wang, X. X., Zhang, M., Shu, J. C., Cao, W. Q., Yang, H. J., et al. (2019). Electromagnetic response and energy conversion for functions and devices in low-dimensional materials. Adv. Funct. Mater. 25:1807398. doi: 10.1002/adfm.201807398
Che, R. C., Peng, L. M., Duan, X. F., and Chen, Q. (2004). Microwave absorption enhancement and complex permittivity and permeability of Fe encapsulated within carbon nanotubes. Adv. Mater. 16, 401–405. doi: 10.1002/adma.200306460
Chen, N., Jiang, J. T., Xu, C. Y., Yuan, Y., Gong, Y. X., and Zhen, L. (2017). Co7Fe3 and Co7Fe3@SiO2 nanospheres with tunable diameters for high-performance electromagnetic wave absorption. ACS Appl. Mater. Interfaces 9, 21933–21941. doi: 10.1021/acsami.7b03907
Chen, Y. H., Huang, Z. H., Lu, M. M., Cao, W. Q., Yuan, J., Zhang, D. Q., et al. (2015). 3D Fe3O4 nanocrystals decorating carbon nanotubes to tune electromagnetic properties and enhance microwave absorption capacity. J. Mater. Chem. A 3, 12621–12625. doi: 10.1039/C5TA02782A
Fang, J. Y., Liu, T., Chen, Z., Wang, Y., Wei, W., Yue, X. G., et al. (2016). A wormhole-like porous carbon/magnetic particles composite as an efficient broadband electromagnetic wave absorber. Nanoscale 8, 8899–8909. doi: 10.1039/C6NR01863G
Feng, A. L., Hou, T. Q., Jia, Z. R., and Wu, G. L. (2020). Synthesis of a hierarchical carbon fiber@cobalt ferrite@manganese dioxide composite and its application as a microwave absorber. RSC Adv. 10, 10510–10518. doi: 10.1039/C9RA10327A
Gao, Z. G., Xu, B. H., Ma, M. L., Feng, A. L., Zhang, Y., Liu, X. H., et al. (2019). Electrostatic self-assembly synthesis of ZnFe2O4 quantum dots (ZnFe2O4@C) and electromagnetic microwave absorption. Composites B Eng. 179:107417. doi: 10.1016/j.compositesb.2019.107417
Hu, Q. M., Yang, R. L., Mo, Z. C., Lu, D. W., Yang, L. L., He, Z. F., et al. (2019). Nitrogen-doped and Fe-filled CNTs/NiCo2O4 porous sponge with tunable microwave absorption performance. Carbon 153, 737–744. doi: 10.1016/j.carbon.2019.07.077
Huang, L., Li, J. J., Wang, Z. J., Li, Y. B., He, X. D., and Yuan, Y. (2019). Microwave absorption enhancement of porous C@CoFe2O4 nanocomposites derived from eggshell membrane. Carbon 143, 507–516. doi: 10.1016/j.carbon.2018.11.042
Hummers, W. S., and Offeman, R. E. (1958). Preparation of graphitic oxide. J. Am. Chem. Soc. 80:1339. doi: 10.1021/ja01539a017
Jiang, J. J., Li, D., Geng, D. Y., An, J., He, J., Liu, W., et al. (2014). Microwave absorption properties of core double-shell FeCo/C/BaTiO3 nanocomposites. Nanoscale 6, 3967–3971. doi: 10.1039/C3NR04087A
Khabouri, S. A., Harthi, S. A., Maekawa, T., Nagaoka, Y., Hinai, A. A., et al. (2015). Composition, electronic and magnetic investigation of the encapsulated ZnFe2O4 nanoparticles in multiwall carbon nanotubes containing Ni residuals. Nanoscale Res. Lett. 10:262. doi: 10.1186/s11671-015-0971-7
Li, N., Huang, G. W., Li, Y. Q., Xiao, H. M., Feng, Q. P., Hu, N., et al. (2017). Enhanced microwave absorption performance of coated carbon nanotubes by optimizing the Fe3O4 nanocoating structure. ACS Appl. Mater. Interfaces 9, 2973–2983. doi: 10.1021/acsami.6b13142
Li, X., Yu, L. M., Zhao, W. K., Shi, Y. Y., Yu, L. J., Dong, Y. B., et al. (2020). Prism-shaped hollow carbon decorated with polyaniline for microwave absorption. Chem. Eng. J. 379:122393. doi: 10.1016/j.cej.2019.122393
Li, X. H., Feng, J., Du, Y. P., Bai, J. T., Fan, H. M., Zhang, H. L., et al. (2015). One-pot synthesis of CoFe2O4/graphene oxide hybrids and their conversion into FeCo/graphene hybrids for lightweight and highly efficient microwave absorber. J. Mater. Chem. A 3, 5535–5546. doi: 10.1039/C4TA05718J
Liu, J. W., Che, R. C., Chen, H. J., Zhang, F., Xia, F., Wu, Q. S., et al. (2012). Microwave absorption enhancement of multifunctional composite microspheres with spinel Fe3O4 cores and anatase TiO2 shells. Small 8, 1214–1221. doi: 10.1002/smll.201102245
Liu, P. B., Gao, S., Huang, W. H., Ren, J., Yu, D. Y., and He, W. J. (2020). Hybrid zeolite imidazolate framework derived N-implanted carbon polyhedrons with tunable heterogeneous interfaces for strong wideband microwave attenuation. Carbon 159, 83–93. doi: 10.1016/j.carbon.2019.12.021
Liu, Q. H., Cao, Q., Bi, H., Liang, C. Y., Yuan, K. P., She, W., et al. (2016). CoNi@SiO2@TiO2 and CoNi@Air@TiO2 microspheres with strong wideband microwave absorption. Adv. Mater. 28, 486–490. doi: 10.1002/adma.201503149
Liu, X., Chen, Y., Cui, X., Zeng, M., Yu, R., and Wang, G. S. (2015). Flexible nanocomposites with enhanced microwave absorption properties based on Fe3O4/SiO2 nanorods and polyvinylidene fluoride. J. Mater. Chem. A 3, 12197–12204. doi: 10.1039/C5TA01924A
Liu, X. F., Hao, C. C., He, L. H., Yang, C., Chen, Y. B., Jiang, C. B., et al. (2018). Yolk-shell structured Co-C/void/Co9S8 composites with a tunable cavity for ultrabroadband and efficient low-frequency microwave absorption. Nano Res. 11, 4169–4182. doi: 10.1007/s12274-018-2006-z
Liu, X. G., Geng, D. Y., Meng, H., Shang, P. J., and Zhang, Z. D. (2008). Microwave-absorption properties of ZnO coated iron nanocapsules. Appl. Phys. Lett. 92, 64–67. doi: 10.1063/1.2919098
Long, L., Yang, E. Q., Qi, X. S., Xie, R., Bai, Z. C., Qin, S. J., et al. (2019). Core@shell structured flower-like Co0.6Fe2.4O4@MoS2 nanocomposites: a strong absorption and broadband electromagnetic wave absorber. J. Mater. Chem. C 7, 8975–8981. doi: 10.1039/C9TC02140J
Long, L., Yang, E. Q., Qi, X. S., Xie, R., Qin, S. J., Deng, C. Y., et al. (2020). Positive and reverse core/shell structure CoxFe3-xO4/MoS2 and MoS2/CoxFe3-xO4 nanocomposites: selective production and outstanding electromagnetic absorption comprehensive performance. ACS Sustain. Chem. Eng. 8, 613–623. doi: 10.1021/acssuschemeng.9b06205
Lu, M. J., Wang, J., Su, X. G., Wu, Q. L., Yan, T. Y., and Zhang, X. X. (2019). Fabrication, structure, and microwave absorbing properties of plate-like BaFe12O19@ZnFe2O4/MWCNTs nanocomposites. Mater. Lett. 253, 46–49. doi: 10.1016/j.matlet.2019.06.012
Lu, Z. P., Wang, H., Zhou, T., Ma, C., Yin, F., Jiang, X. F., et al. (2018). CoCO3 micrometer particles stabilized by carbon nanofibers networks as composite electrode for enhanced rate and cyclic performance of lithium-ion batteries. Electrochim. Acta 270, 22–29. doi: 10.1016/j.electacta.2018.03.081
Lv, R. T., Kang, F. Y., Gu, J. L., Gui, X. C., Wei, J. Q., Wang, K. L., et al. (2008). Carbon nanotubes filled with ferromagnetic alloy nanowires: lightweight and wide-band microwave absorber. Appl. Phys. Lett. 93:223105. doi: 10.1063/1.3042099
Novoselov, K. S., Geim, A. K., Morozov, S. V., Dubonos, S. V., Zhang, Y., and Jiang, D. (2004). Electric field effect in atomically thin carbon films. Science 306, 666–669. doi: 10.1126/science.1102896
Pawar, S. P., Melo, G., and Sundararaj, U. (2019). Dual functionality of hierarchical hybrid networks of multiwall carbon nanotubes anchored magnetite particles in soft polymer nanocomposites: simultaneous enhancement in charge storage and microwave absorption. Compos. Sci. Technol. 183:107802. doi: 10.1016/j.compscitech.2019.107802
Popov, V. N., and Lambin, P. (2013). Theoretical Raman intensity of the G and 2D bands of strained graphene. Carbon 54, 86–93. doi: 10.1016/j.carbon.2012.11.006
Qu, B., Zhu, C. L., Li, C. Y., Zhang, X. T., and Chen, Y. J. (2016). Coupling hollow Fe3O4-Fe nanoparticles with graphene sheets for high-performance electromagnetic wave absorbing material. ACS Appl. Mater. Interfaces 8, 3730–3735. doi: 10.1021/acsami.5b12789
Quan, B., Liang, X. H., Ji, G. B., Ma, J. N., Ouyang, P. Y., Gong, H., et al. (2017). Strong electromagnetic wave response derived from the construction of dielectric/magnetic media heterostructure and multiple interfaces. ACS Appl. Mater. Interfaces 9, 9964–9974. doi: 10.1021/acsami.6b15788
Ren, F., Guo, Z. Z., Shi, Y. F., Jia, L. C., Qing, Y. C., Ren, P. G., et al. (2018). Lightweight and highly efficient electromagnetic wave-absorbing of 3D CNTs/GNS@CoFe2O4 ternary composite aerogels. J. Alloy Compd. 786, 6–14. doi: 10.1016/j.jallcom.2018.07.209
Ritter, U., Scharff, P., Siegmund, C., Dmytrenko, O. P., Kulish, N. P., Prylutskyy, Y. I., et al. (2006). Radiation damage to multi-walled carbon nanotubes and their Raman vibrational modes. Carbon 44, 2694–2700. doi: 10.1016/j.carbon.2006.04.010
Shi, S. J., Zhang, M., Liu, Y. Y., Hua, X. Y., Guo, H. T., and Yang, G. (2018). Efficient construction of a CoCO3/graphene composite anode material for lithium-ion batteries by stirring solvothermal reaction. Ceram. Int. 44, 3718–3725. doi: 10.1016/j.ceramint.2017.11.152
Shu, R. W., Li, W. J., Zhou, X., Tian, D. D., Zhang, G. Y., Gan, Y., et al. (2018a). Facile preparation and microwave absorption properties of RGO/MWCNTs/ZnFe2O4 hybrid nanocomposites. J. Alloy Compd. 743, 163–174. doi: 10.1016/j.jallcom.2018.02.016
Shu, R. W., Zhang, G. Y., Wang, X., Gao, X., Wang, M., Gan, Y., et al. (2018b). Fabrication of 3D net-like MWCNTs/ZnFe2O4 hybrid composites as high-performance electromagnetic wave absorbers. Chem. Eng. J. 337, 242–255. doi: 10.1016/j.cej.2017.12.106
Wang, C., Murugadoss, V., Kong, J., He, Z. F., Mai, X. M., Shao, Q., et al. (2018). Overview of carbon nanostructures and nanocomposites for electromagnetic wave shielding. Carbon 140, 696–733. doi: 10.1016/j.carbon.2018.09.006
Wang, G. Z., Gao, Z., Tang, S. W., Chen, C. Q., Duan, F. F., Zhao, S. C., et al. (2012). Microwave absorption properties of carbon nanocoils coated with highly controlled magnetic materials by atomic layer deposition. ACS Nano 6, 11009–11017. doi: 10.1021/nn304630h
Wang, L. N., Jia, X. L., Li, Y. F., Yang, F., Zhang, L. Q., Liu, L. P., et al. (2014). Synthesis and microwave absorption property of flexible magnetic film based on graphene oxide/carbon nanotubes and Fe3O4 nanoparticles. J. Mater. Chem. A 2, 14940–14946. doi: 10.1039/C4TA02815E
Wang, R., Yang, E. Q., Qi, X. S., Xie, R., Qin, S. J., Deng, C. Y., et al. (2020). Constructing and optimizing core@shell structure CNTs@MoS2 nanocomposites as outstanding microwave absorbers. Appl. Surf. Sci. 516:146159. doi: 10.1016/j.apsusc.2020.146159
Wang, Y., Gao, X., Wu, X. M., Zhang, W. Z., Luo, C. Y., and Liu, P. B. (2019). Facile design of 3D hierarchical NiFe2O4/N-GN/ZnO composite as a high performance electromagnetic wave absorber. Chem. Eng. J. 375:121942. doi: 10.1016/j.cej.2019.121942
Wang, Y. F., Chen, D. L., Yin, X., Xu, P. F., and He, M. (2015). Hybrid of MoS2 and reduced graphene oxide: a lightweight and broadband electromagnetic wave absorber. ACS Appl. Mater. Interfaces 7, 26226–26234. doi: 10.1021/acsami.5b08410
Wei, S., Wang, X. X., Zhang, B. Q., Yu, M. G., Zheng, Y. W., Wang, Y., et al. (2017). Preparation of hierarchical core-shell C@NiCo2O4@Fe3O4 composites for enhanced microwave absorption performance. Chem. Eng. J. 314, 477–487. doi: 10.1016/j.cej.2016.12.005
Weissker, U., Hampel, S., Leonhardt, A., and Büchner, B. (2010). Carbon nanotubes filled with ferromagnetic materials. Materials 3, 4387–4427. doi: 10.3390/ma3084387
Wen, F. S., Zhang, F., and Liu, Z. Y. (2011). Investigation on microwave absorption properties for multiwalled carbon nanotubes/Fe/Co/Ni nanopowders as lightweight absorbers. J. Phys. Chem. C 115, 14025–14030. doi: 10.1021/jp202078p
Wu, M., Qi, X. S., Xie, R., Bai, Z. C., Qin, S. J., Zhong, W., et al. (2020). Graphene oxide/carbon nanotubes/CoxFe3-xO4 ternary nanocomposites: controllable synthesis and their excellent microwave absorption capabilities. J Alloy Compd. 813:151996. doi: 10.1016/j.jallcom.2019.151996
Xu, J. L., Qi, X. S., Sun, Y., Wang, Z. C., Liu, Y., Luo, C. Z., et al. (2018). Tuning the electromagnetic synergistic effects for enhanced microwave absorption via magnetic nickel core encapsulated in hydrogenated anatase TiO2 shell. ACS Sustain. Chem. Eng. 6, 12046–12054. doi: 10.1021/acssuschemeng.8b02350
Xu, Z., Du, Y. C., Liu, D. W., Wang, Y. H., Ma, W. J., Wang, Y., et al. (2019). Pea-like Fe/Fe3C nanoparticles embedded in nitrogen-doped carbon nanotubes with tunable dielectric/magnetic loss and efficient electromagnetic absorption. ACS Appl. Mater. Interfaces 11, 4268–4277. doi: 10.1021/acsami.8b19201
Yamashita, T., and Hayes, P. (2008). Analysis of XPS spectra of Fe2+ and Fe3+ ions in oxide materials. App. Sur. Sci. 254, 2441–2449. doi: 10.1016/j.apsusc.2007.09.063
Yan, J., Huang, Y., Chen, C., Liu, X. D., and Liu, H. (2019). The 3D CoNi alloy particles embedded N-doped porous carbon foam for high-performance microwave absorber. Carbon 152, 545–555. doi: 10.1016/j.carbon.2019.06.064
Yang, R. B., Reddy, P. M., Chang, C. J., Chen, P. A., Chen, J. K., and Chang, C. C. (2016). Synthesis and characterization of Fe3O4/polypyrrole/carbon nanotube composites with tunable microwave absorption properties: role of carbon nanotube and polypyrrole content. Chem. Eng. J. 285, 497–507. doi: 10.1016/j.cej.2015.10.031
Ye, F., Song, Q., Zhang, Z. C., Li, W., Zhang, S. Y., Yin, X. W., et al. (2018). Direct growth of edge-rich graphene with tunable dielectric properties in porous Si3N4 ceramic for broadband high-performance microwave absorption. Adv. Funct. Mater. 28:1707205. doi: 10.1002/adfm.201707205
Yusoff, A. N., Abdullah, M. H., Ahmad, S. H., Jusoh, S. F., Mansor, A. A., and Hamid, S. A. A. (2002). Electromagnetic and absorption properties of some microwave absorbers. J. Appl. Phys. 92, 876–882. doi: 10.1063/1.1489092
Zhang, H., Hong, M., Chen, P., Xie, A. J., and Shen, Y. H. (2016). 3D and ternary rGO/MCNTs/Fe3O4 composite hydrogels: synthesis, characterization and their electromagnetic wave absorption properties. J. Alloy Compd. 665, 381–387. doi: 10.1016/j.jallcom.2016.01.091
Zhang, K. C., Gao, X. B., Zhang, Q., Li, T. P., Chen, H., and Chen, X. F. (2017). Preparation and microwave absorption properties of asphalt carbon coated reduced graphene oxide/magnetic CoFe2O4 hollow particles modified multi-wall carbon nanotube composites. J. Alloy Compd. 723, 912–921. doi: 10.1016/j.jallcom.2017.06.327
Zhang, S., Qi, Z., Zhao, Y., Jiao, Q., Ni, X., Wang, Y., et al. (2017). Core/shell structured composites of hollow spherical CoFe2O4 and CNTs as absorbing materials. J. Alloy Compd. 694, 309–312.
Zhang, X. J., Wang, G. S., Cao, W. Q., Wei, Y. Z., Liang, J. F., Guo, L., et al. (2014). Enhanced microwave absorption property of reduced graphene oxide (RGO)-MnFe2O4 nanocomposites and polyvinylidene fluoride. ACS Appl. Mater. Interfaces 6, 7471–7478. doi: 10.1021/am500862g
Zhang, Y., Huang, Y., Zhang, T. F., Chang, H. C., Xiao, P. S., Chen, H. H., et al. (2015). Broadband and tunable high-performance microwave absorption of an ultralight and highly compressible graphene foam. Adv. Mater. 27, 2049–2053. doi: 10.1002/adma.201405788
Keywords: magnetic GO/CNTs, 3D hierarchical networks structure, interfacial polarizations, dielectric and magnetic loss mechanisms, microwave absorption properties
Citation: Wu M, Qi X, Xie R, Qin S, Deng C and Zhong W (2020) Magnetic Carbon Nanotubes/Graphene Oxide Nanocomposites: One-Step Controllable Production, and Their Excellent Microwave Absorption Capabilities. Front. Mater. 7:100. doi: 10.3389/fmats.2020.00100
Received: 23 February 2020; Accepted: 31 March 2020;
Published: 08 May 2020.
Edited by:
Biao Zhao, Zhengzhou University of Aeronautics, ChinaReviewed by:
Guanglei Wu, Qingdao University, ChinaYaofeng Zhu, Zhejiang Sci-Tech University, China
Yongxing Zhang, Huaibei Normal University, China
Copyright © 2020 Wu, Qi, Xie, Qin, Deng and Zhong. This is an open-access article distributed under the terms of the Creative Commons Attribution License (CC BY). The use, distribution or reproduction in other forums is permitted, provided the original author(s) and the copyright owner(s) are credited and that the original publication in this journal is cited, in accordance with accepted academic practice. No use, distribution or reproduction is permitted which does not comply with these terms.
*Correspondence: Xiaosi Qi, eHNxaUBnenUuZWR1LmNu
†ORCID: Xiaosi Qi, orcid.org/0000-0003-0987-1622; Wei Zhong, orcid.org/0000-0003-2507-3479