- 1RUSA-Center for Advanced Sensor Technology (CAST), Department of Physics, Dr. Babasaheb Ambedkar Marathwada University, Aurangabad, India
- 2Department of Electronics and Telecommunication Engineering, Jawaharlal Nehru Engineering College, Aurangabad, India
Electrochemical recognition of Hg (II) ions utilizing EDTA modified polyaniline (PANI)/Graphene Oxide (GO) composite is reported in the present communication. Graphene Oxide (GO) synthesis was carried out by a modified Hummer’s method. Electrochemical characterizations, namely cyclic voltammetry (CV) and electrochemical impedance spectroscopy (EIS), were performed before and after modification of the composite. The topographies of the PANI/GO composite and EDTA_PANI/GO electrodes were studied using AFM. Roughness parameter values were compared for confirmation of surface modification. Fourier transform infrared spectroscopy (FTIR) was utilized for the compositional analysis of PANI/GO and EDTA_PANI/GO electrodes. The EDTA_PANI/GO composite exhibits sensitivity toward Hg (II) ions when probed using the differential pulse stripping voltammetry (DPSV) technique with a lower detection limit of 2 ppb. EDTA modified SS_PANI/GO composite (PANI/GO composite deposited on a stainless-steel substrate) showed superior sensitivity in the detection of Hg (II) ions. The sensitivity was observed to extend to 1 ppb, which is smaller than the maximum contaminant level (MCL) endorsed by the Environment Protection Agency (EPA, United States).
Introduction
Heavy metals are moderately scarce in the crust of the Earth. These heavy metals are characterized by comparatively high densities and atomic numbers (Tekaya et al., 2013). Heavy metals can harm air, water, and soil quality, and in this manner, cause hazards to human wellbeing and the environment (Bánfalvi, 2011; Turdean, 2011). Among them, some metals like iron, cobalt, and zinc provide crucial nutrients or are relatively innocuous (such as ruthenium, silver, and indium) but yet can be fatal in larger amounts. Some heavy metals like cadmium, mercury, and lead are profoundly poisonous and cancer-causing above trace levels (Nagajyoti et al., 2010; Lansdown, 2013). Of these, mercury has a greater affinity to sulfur and thiol-containing molecules, resulting also in nephrotoxicity and neurotoxicity (Gumpu et al., 2015; Jan et al., 2015). For these reasons, the need arises for sensors that can acquire real-time measurements of heavy metals in water.
Electrochemical detection of heavy metal ion concentration is a versatile tool. Anodic stripping voltammetry (ASV) is the commonly used technique (Bansod et al., 2017; Deshmukh et al., 2018a). However, ASV has weaknesses such as instability, low sensitivity, and limits to detection (Zhao et al., 2014; Patil et al., 2018).
To conquer this problem, many advanced materials are being investigated, such as conducting polymers, carbon nanotubes (CNTs), graphene and its derivatives, and metal oxides (Choi et al., 2011; Oztekin et al., 2011; Strong et al., 2012; Lei et al., 2014). However, these advanced materials have certain limitations, e.g., organic conducting polymers have low selectivity, sensitivity, and a lack of environmental stability (Zhang et al., 2011; Das and Prusty, 2012). One of the shortcomings of carbon nanotubes (CNTs) is the entrapment of molecules at interstitial sites, which decreases reproducibility (Musameh et al., 2011; Herrera-Herrera et al., 2012).
To overcome such limitations, composite or functionalized materials are employed for the effective detection of metal ion concentration (Chen et al., 2012; Deshmukh et al., 2018b). Zhou et al. (2014) have reported on conducting polymers such as polyaniline (PANI), which performs as a p-type semiconductor and has demonstrated incredible potential for application because of its electrical conductivity, solubility, optical activity, easy processing, good sensitivity at room temperature, and good environmental stability. Graphene-based sensors are comparatively novel and can possibly meet the objective of quick in situ estimation of metals in water (Wang et al., 2010; Chang et al., 2014). It was reported that graphene has a huge hypothetical explicit surface area, high intrinsic mobility, and good electrical conductivity (Zhu et al., 2010). Graphene additionally has a honeycomb lattice with two sub-lattices bonded together with a σ-bond. Every carbon atom in the lattice contains a π orbital that offers ascent to a delocalized electron (Charlier et al., 2007; Roth and Carroll, 2015). Aside from these astounding properties, the remarkably low electronic clamor of graphene provides the opportunity to sensitively detect numerous analytes using graphene (Roth and Carroll, 2015).
It was as well reported that Graphene Oxides (GO) have layered, oxygenated graphene sheets that contain oxygen functional groups, for example, epoxides, carboxyls, hydroxyls, and alcohols, on their basal planes and edges. These groups in GO can be utilized as decent sites for surface alteration. As indicated by chemical examination, the carbon to oxygen ratio is 3:1 (Stankovich et al., 2006). GO can be reduced to nearly graphene by various chemical reduction methods; however, some promising applications of GO have recently been reported (Gilje et al., 2007; McAllister et al., 2007; Rao et al., 2009; Chen et al., 2012).
In recent years, compositing or modification of polyaniline (PANI) with carbon-based materials has received much attention. The properties of GO, such as its flat structure, charge transfer ability, incorporation of hydrophilic functional groups, and good dispersion ability in aqueous media, lead to more sites at which PANI can become attached (Dreyer et al., 2010; Vadivel et al., 2016). Also, the interaction of PANI with GO is by Π-Π stacking, electrostatic interaction, and hydrogen bonding (Wang et al., 2010). The Π-Π interaction will support faster signal processing and enhancement of the sensitivity of the sensor. Moreover, in coordination chemistry, EDTA falls within the aminopolycarboxylic acid family of ligands. Typically, EDTA ties to a metal cation through its two amines and four carboxylates. A significant number of the subsequent coordination compounds bear octahedral geometry. This octahedral geometrical structure assists with attaching the metal ion and furthermore expands the level of cross-linking inside the PANI film (Zagal et al., 1996). This implies that a composite of PANI/GO modified by EDTA will have better discrimination toward Hg (II) ion. The differential pulse stripping voltammetry (DPSV) technique is utilized for the identification of heavy metal ions.
In the present investigation, we have amalgamated a composite of polyaniline (PANI) and graphene oxide (GO), which was then modified by ethylenediaminetetraacetic acid (EDTA) for the detection of mercury ions (Hg II) using DPSV.
Over the most recent couple of years, the greater part of the information revealed shows the simultaneous identification of different heavy metal ions, which reflects the issue of selectivity (Gumpu et al., 2017; Ullah et al., 2018; Yi et al., 2019). In the present investigation, this problem is substantially resolved. Moreover, the present investigation also achieves the detection of Hg (II) ions beneath the MCL level, i.e., up to 1 ppb, with a quantifiable yield current.
Materials and Methods
Materials
Aniline of reagent grade, sulfuric acid, and ethylenediaminetetraacetic acid (EDTA) were bought from Fluka (Germany), 1-ethyl-3-(3-dimethylaminopropyl) carbodiimide (EDC) was acquired from Sigma Aldrich (Germany). The phosphate buffer solution (PBS) used was of pH 7. Graphene powder (∼60 mesh size) was procured from Molychem (Mumbai, India), and potassium permanganate was from Kemphasol (India). An acetate buffer solution was set up by regulating 0.1 M sodium acetate (Aldrich) to the desired pH, 4.1. All the progressions were carried out in aqueous media using deionized water (DI).
Preparation of PANI/GO Composite
Graphene Oxide was produced from graphite powder using the Modified Hummer’s Method (Shahriary and Athawale, 2014). Separately, PANI/GO composite was synthesized by an electrochemical method. First, 50 mL of aqueous 0.25 M aniline and 0.5 M H2SO4 suspension were mixed and maintained for 20 min, stirring. Then, 10 ml of GO in the ratio 0.1 mg/ml was mixed with the above electrolyte solution. The mixture was kept for 20 min, stirring, followed by 20 min sonication. The electrochemical synthesis of PANI/GO composite was carried out by the cyclic voltammetry method inside the potential scope of 0 to +1 V at a scan rate of 0.1 V/s for 20 cycles, with a stainless steel (SS) electrode as the working electrode, platinum as the counter electrode, and Ag/AgCl as the reference electrode. It was performed on a CHI 660C electrochemical workstation. After applying the voltage for 20 cycles, a blackish green-colored coating was observed on the working electrode. The deposited film was washed with deionized water to evacuate the concentration of electrolyte at the substrate surface, and the electrode was afterward dried at room temperature. A schematic of the synthesis of PANI/GO composite and the subsequent modification of the composite through the EDTA chelating ligand is represented in Figure 1.
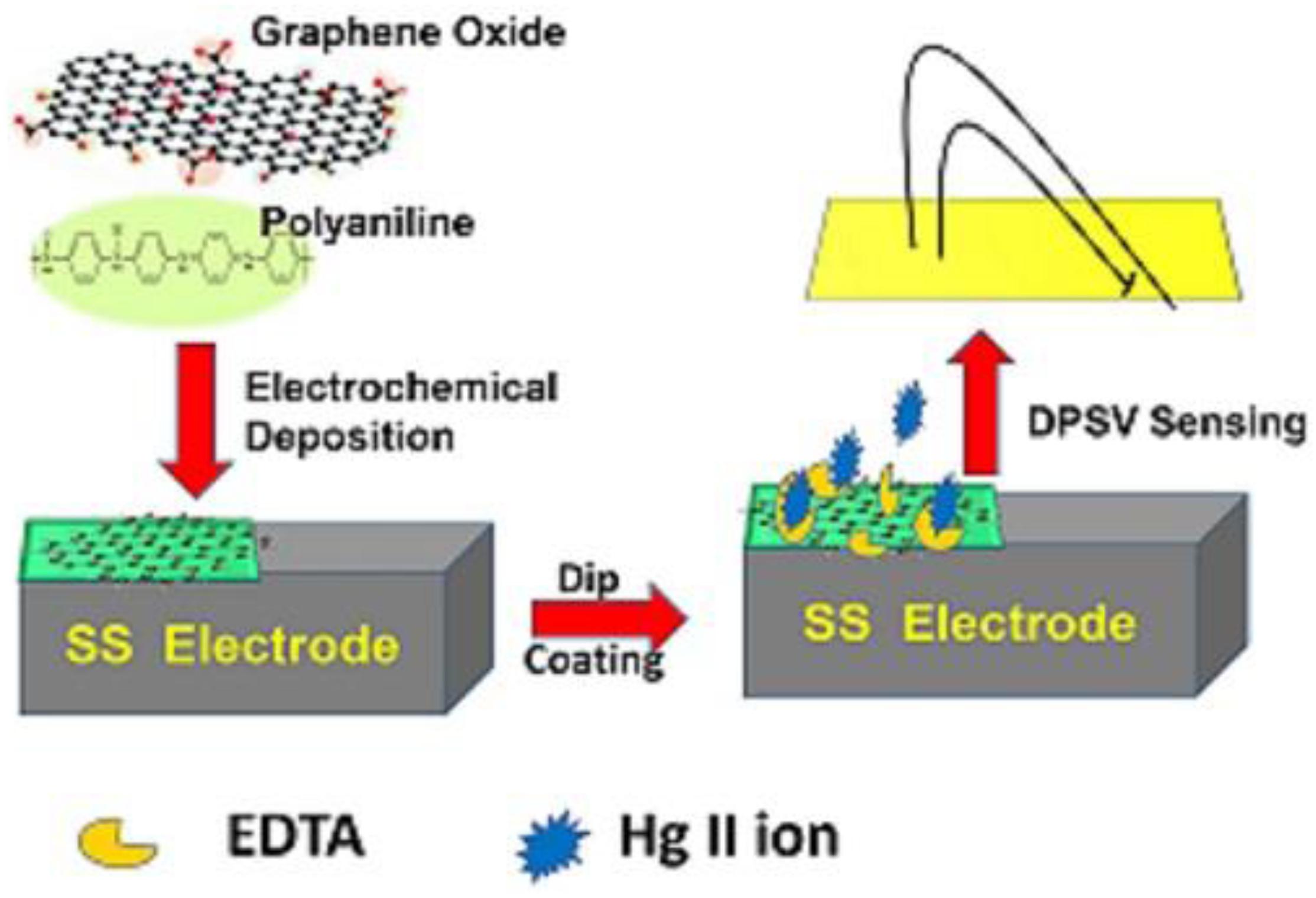
Figure 1. Schematic diagram of the formation of EDTA_PANI/GO modified composite on an SS electrode for the detection of Hg (II) ions by the DPSV technique.
Modification of SS_PANI/GO Composite
The SS_PANI/GO composite electrode (PANI/GO composite synthesized on a stainless steel substrate) was modified by EDTA chelation by a dip-coating method because, after polymerization of a conducting polymer, it is easy to attach another active group covalently. For modification, an EDTA suspension was set up in a 0.2 M phosphate buffer solution (PBS) at pH 7.2, having 0.1 M EDC as a triggering operator and 0.01 M EDTA. The SS PANI/GO electrode was inundated in the readied solution of EDTA and left for 12 h under non-stop stirring at room temperature. This resulted in the formation of covalent bonding of COOH- with the NH2 group of the composite. The electrodes were subsequently cautiously washed with deionized water to expel the gently bound molecules.
Electrochemical Detection of Mercury [Hg (II)] Ions
Electrochemical detection of mercury ions was carried out by differential pulse stripping voltammetry (DPSV) employing a three-terminal arrangement at room temperature. The SS_PANI/GO/EDTA electrode filled in as the working electrode (1 cm2) and Ag/AgCl and platinum as the reference and counter electrodes, respectively. The modified electrode was drenched in acetate buffer solution of pH 4.1 with different concentrations of Hg (II) ion and kept under continuous stirring for 2 min at 600 rpm for accumulation. This 120 s was the deposition time for Hg (II) ions. Since EDTA has the capacity of multidentate ligands to make a complex with many metal ions at various pH values, the pH for Hg (II) ions has been optimized as 4.1, achieved via the acetate buffer solution. After completing accumulation, DPSV scan was applied in the potential range of 0.0 to 0.4 V to oxidize mercury ions from the electrode surface.
Results and Discussion
Electrochemical Synthesis of PANI/GO Composite
Electrochemical synthesis of PANI/GO composite was performed by cyclic voltammetry, as portrayed in Figure 1, between the range 0 to +1 V for 20 cycles on the SS electrode at a 0.1 V/s scan rate. Figure 2 illustrates the relative cyclic voltammogram recorded during the amalgamation of PANI and PANI/GO composite film. During the synthesis of PANI, a greenish-colored film was observed to form, while for, the composite, a blackish green-colored film formed.
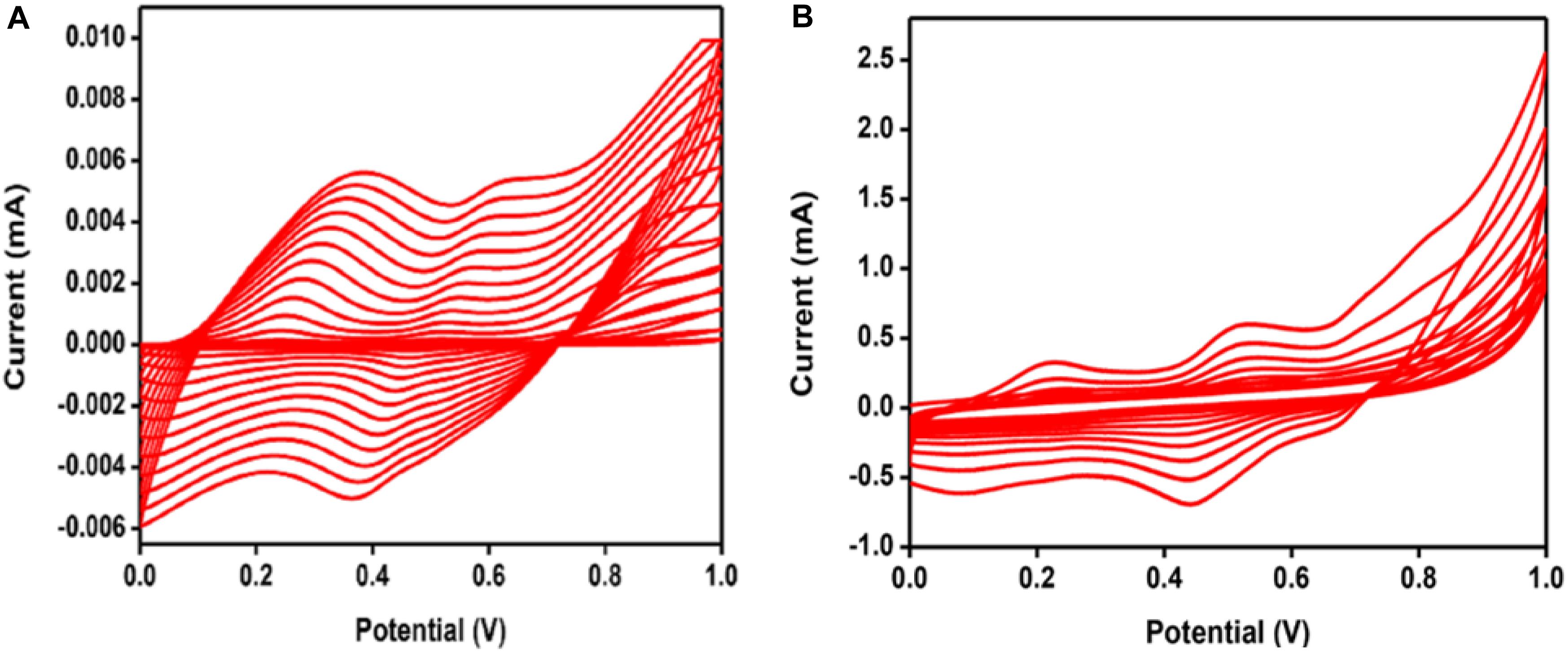
Figure 2. Cyclic voltammogram recorded during the synthesis of (A) PANI and (B) PANI/GO composite for 20 potential cycles.
During synthesis, increase in the current in the PANI/GO composite as compared to PANI is clearly exhibited in Figure 2B, which relates to the development of composite film on the SS electrode. The conductivity of the film increments by expanding the applied potential, which affirms the formation of PANI/GO composite.
Electrochemical Characterization
Figure 3 represents the electrochemical behavior of PANI, PANI/GO Composite, and EDTA modified PANI/GO Composite in 0.5 M H2SO4 solution. The oxidation and reduction peaks of PANI are easily observed, and PANI/GO composite exhibits lower oxidation potential compared to PANI and EDTA modified PANI/GO composite. The PANI/GO composite exhibits an increase in oxidation potential after modification by EDTA due to the electrostatic interaction present on the surfaces of the electrodes.
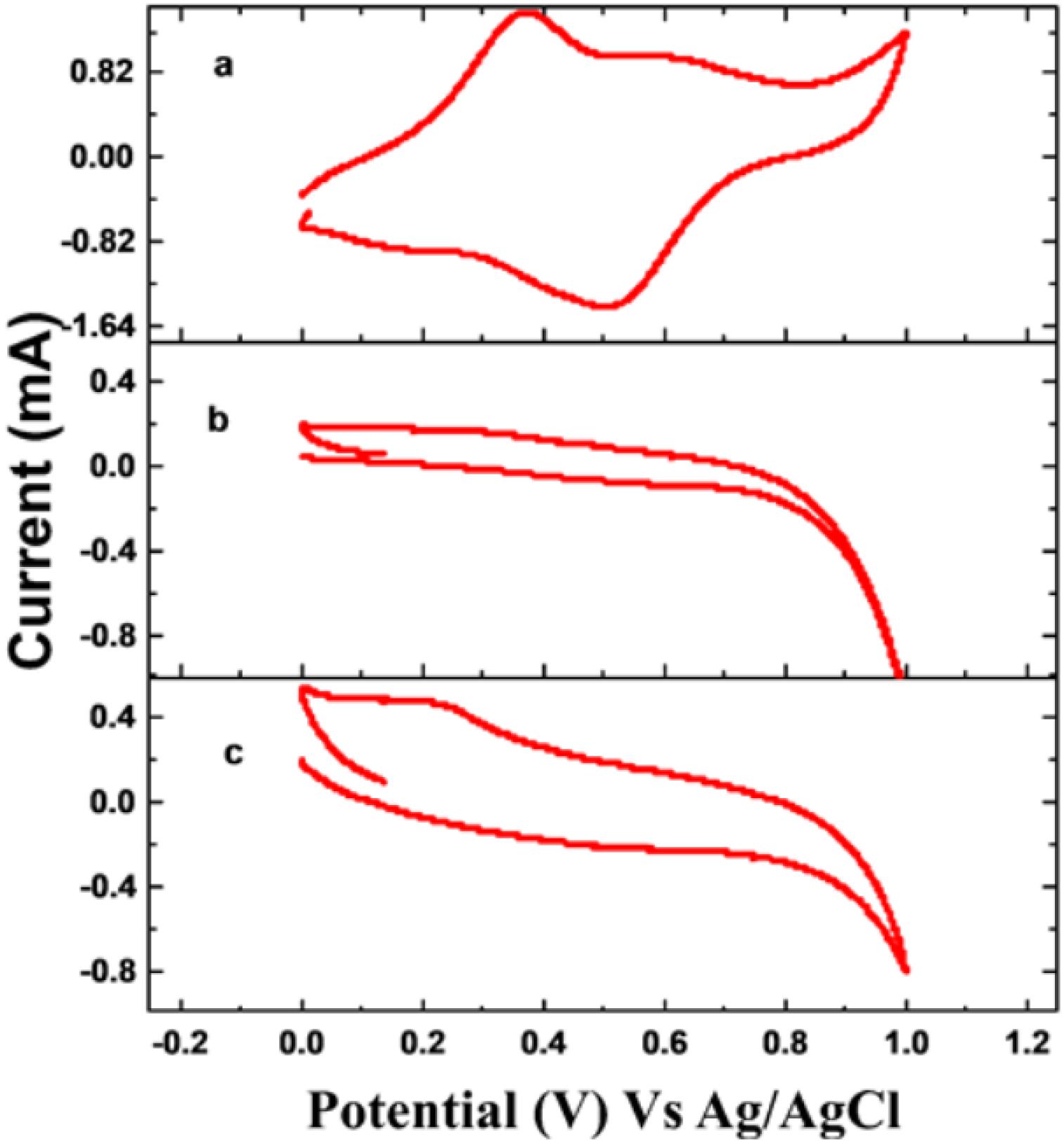
Figure 3. Cyclic voltammogram of (A) PANI, (B) PANI/GO composite, and (C) EDTA modified PANI/GO composite electrodes in 0.5 M H2SO4 electrolyte.
The PANI/GO composite and EDTA modified PANI/GO electrodes were characterized by EIS in the frequency range of 1to 1000 Hz in 0.5 M H2SO4, which is a functioning strategy for evaluation of procedures occurring at electrode surfaces. Figure 4 shows the EIS Nyquist plots of the PANI/GO composite and EDTA modified PANI/GO composite electrodes. The EIS for EDTA modified PANI/GO composite electrodes indicates a moderately large semi-circular province, which exhibits a large interfacial electron transfer resistance between the redox probe and the modified PANI/GO composite electrode. The PANI/GO electrodes show lower interfacial electron resistance in the Nyquist plot, which indicates that the structure of the PANI/GO composite electrode stimulates electron transfer, which demonstrates the superb electrochemical action of the PANI/GO composite electrode.
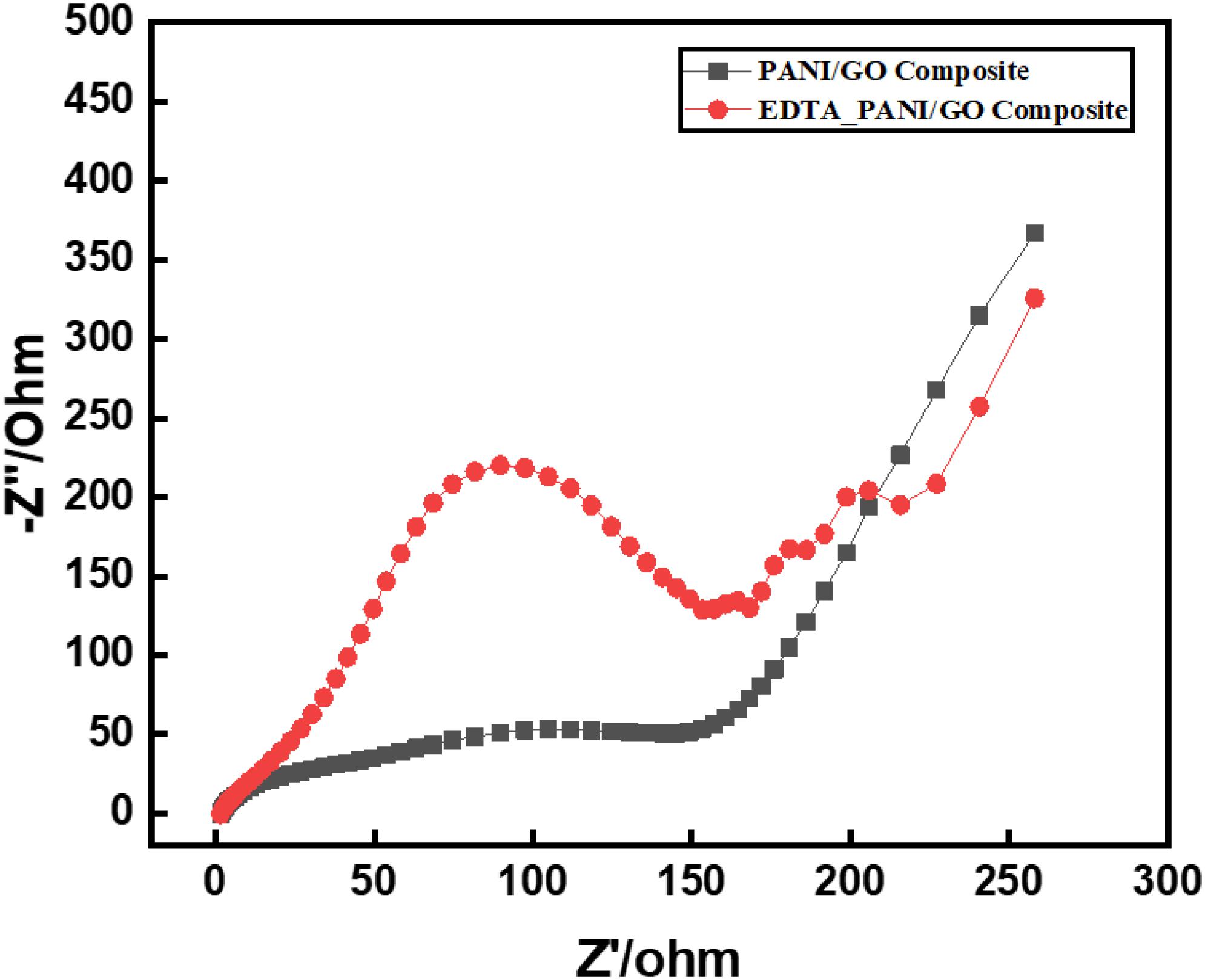
Figure 4. EIS Nyquist plots of PANI/GO composite and EDTA modified PANI/GO composite electrodes in 0.5 M H2SO4 electrolyte.
FTIR Analysis
The PANI/GO composite and EDTA modified PANI/GO composite were further characterized by FTIR spectroscopy, as depicted in Figure 5. Peaks at 1690 cm–1 and 1510 cm–1 in every spectrum confirm the presence of C = O and C-O functional groups. C = N stretching is clearly observed at 1120 cm–1 in the EDTA modified PANI/GO composite. This stretching indicates strong interaction with the EDTA ligand. The peak perceived at 2340 cm–1 relates to the (isocyanate group) N = C = O asymmetric vibrations.
Surface Morphologies of GO, PANI/GO Composite, and EDTA Modified PANI/GO
The surface morphologies of the prepared samples were studied with an atomic force microscope (AFM). Figure 6 represents the surface morphology of (A) bare GO, (B) PANI/GO composite, and (C) EDTA modified PANI/GO composite, which plainly reveals the transformations in surface morphology. The plot in Figure 7 distinguishes the roughness parameters of graphene oxide drop-casted on a glass substrate, PANI/GO composite synthesized by the electrochemical method, and PANI/GO composite modified with EDTA by dip coating.
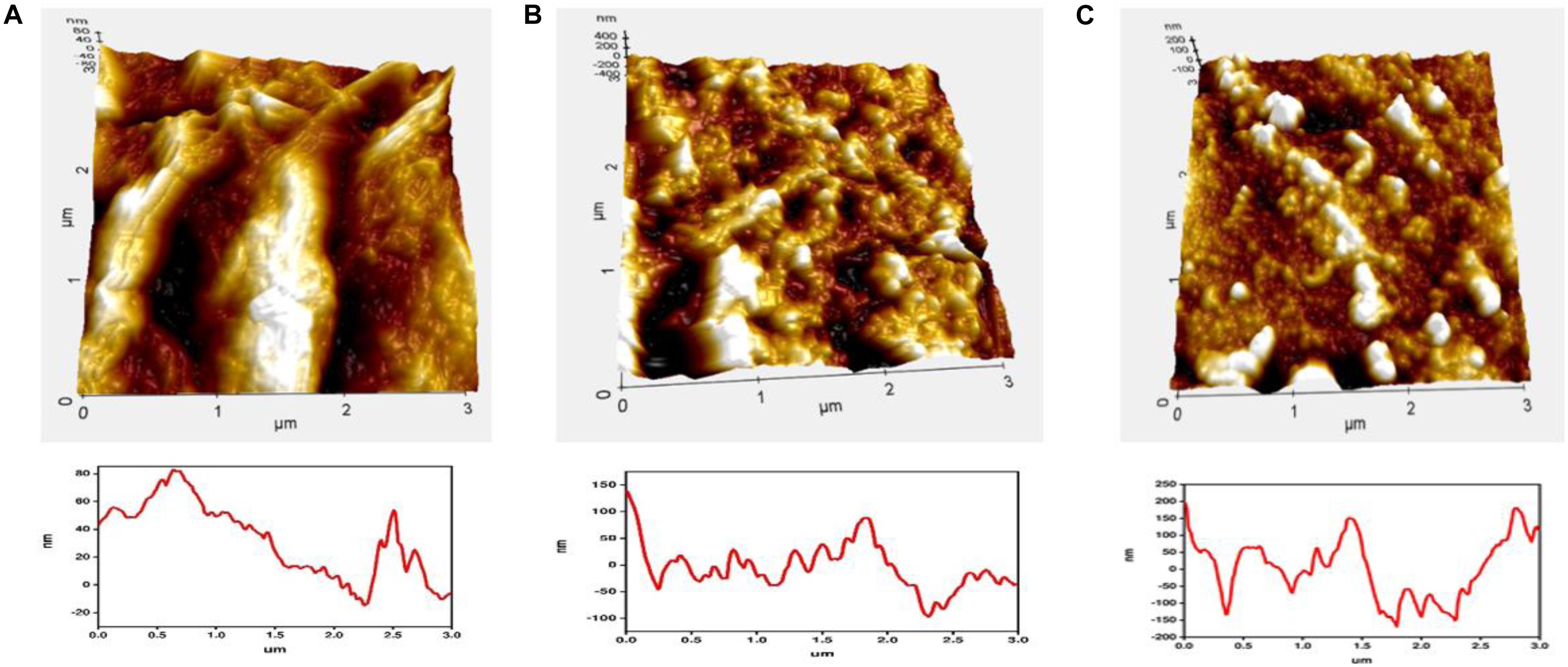
Figure 6. Comparative atomic force microscope (AFM) images with respective line profiles of (A) GO, (B) PANI/GO composite, and (C) EDTA modified PANI/GO composite.
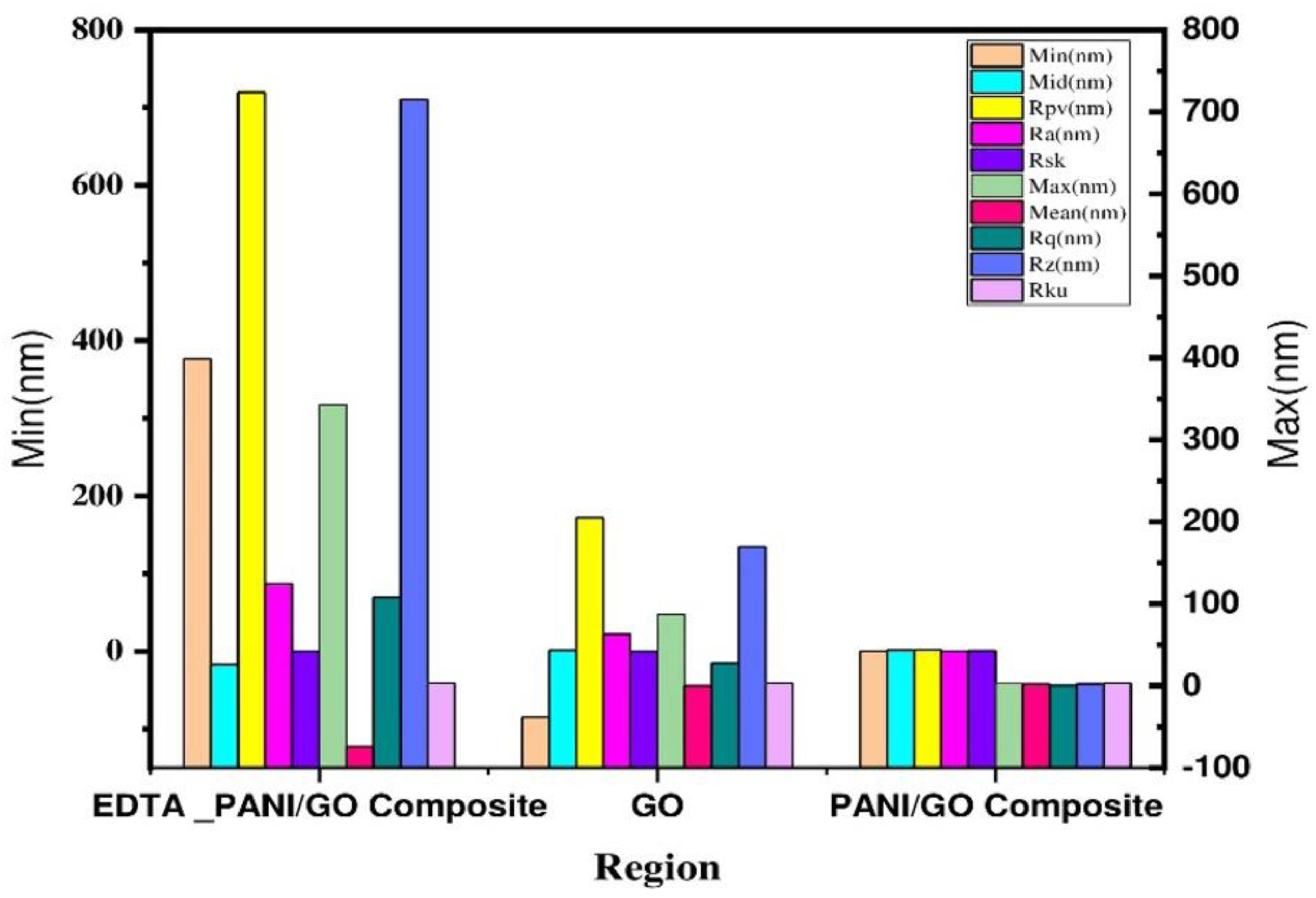
Figure 7. Surface roughness parameters of bare GO, PANI/GO composite, and EDTA modified PANI/GO composite.
Figure 8 shows the height distribution of (A) GO, (B) PANI/GO composite, and (C) EDTA modified PANI/GO composite. Figure 8 evidently shows that there is an increasing distribution height from bare graphene oxide to PANI/GO composite to EDTA modified PANI/GO composite.
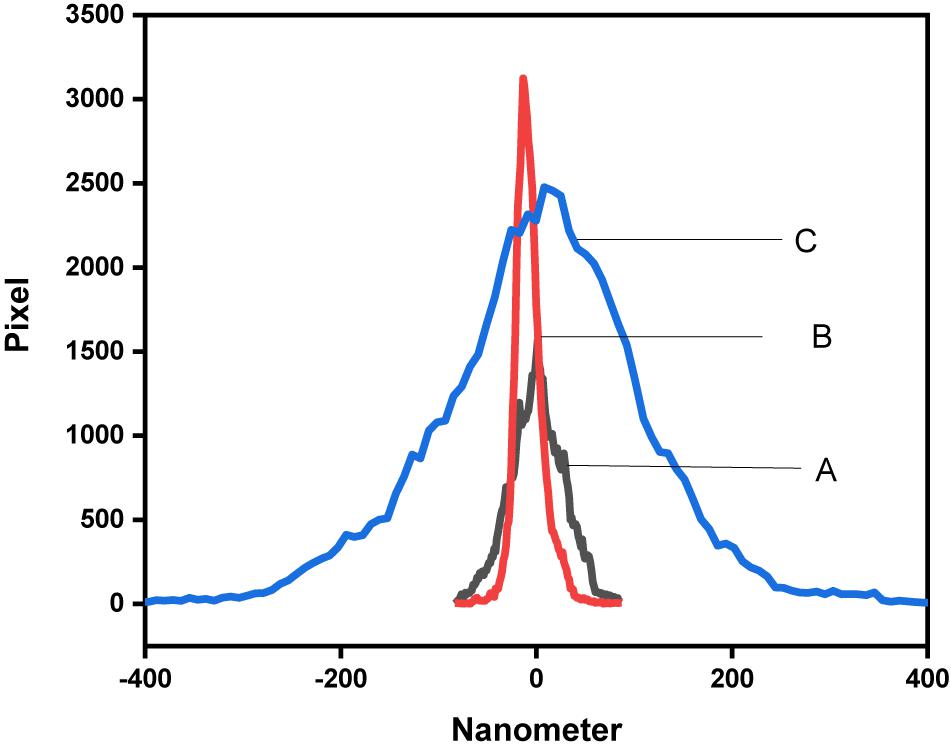
Figure 8. Height distribution of (A) GO, (B) PANI/GO composite, (C) EDTA modified PANI/GO composite.
Comparative and Selective Determination of Hg(II) Ions by the DPSV Method
The best method for the determination of mercury ions was elaborated on in section “Electrochemical Detection of Mercury [Hg (II)] Ions,” Determination was performed by the differential pulse stripping voltammetry technique (DPSV) with EDTA modified PANI/GO composite on an SS electrode. The modified SS electrode was immersed in a mercury ion solution and, by following the methodology mentioned in section “Electrochemical Detection of Mercury [Hg (II)] Ions,” measurement was carried out. Figure 9A, represents the comparative detection of Hg (II) ions at 30 ppb with three different electrodes, that is, (A) PANI/GO composite, (B) only PANI, and (C) EDTA modified PANI/GO composite. From this, it can be easily concluded that EDTA modified PANI/GO composite is more sensitive than the PANI and PANI/GO composite electrode. Thereafter, the determination of Hg (II) ions with the EDTA modified PANI/GO composite electrode was performed at different concentrations down to as low as 1 ppb (Figure 9B). As the concentration increases, the measured value of the current also increases linearly, as depicted in Figure 10A. An estimation of the determination and linearity of the device has been made by way of the calibration plot in Figure 10B. The gadget revealed a linearity factor R2 = 0.91283 for room temperature estimation. The sensitivity of the SS_ EDTA modified PANI/GO composite was 12.42 mA/ppb, and the limit of detection (LOD) was 0.612 ppb.
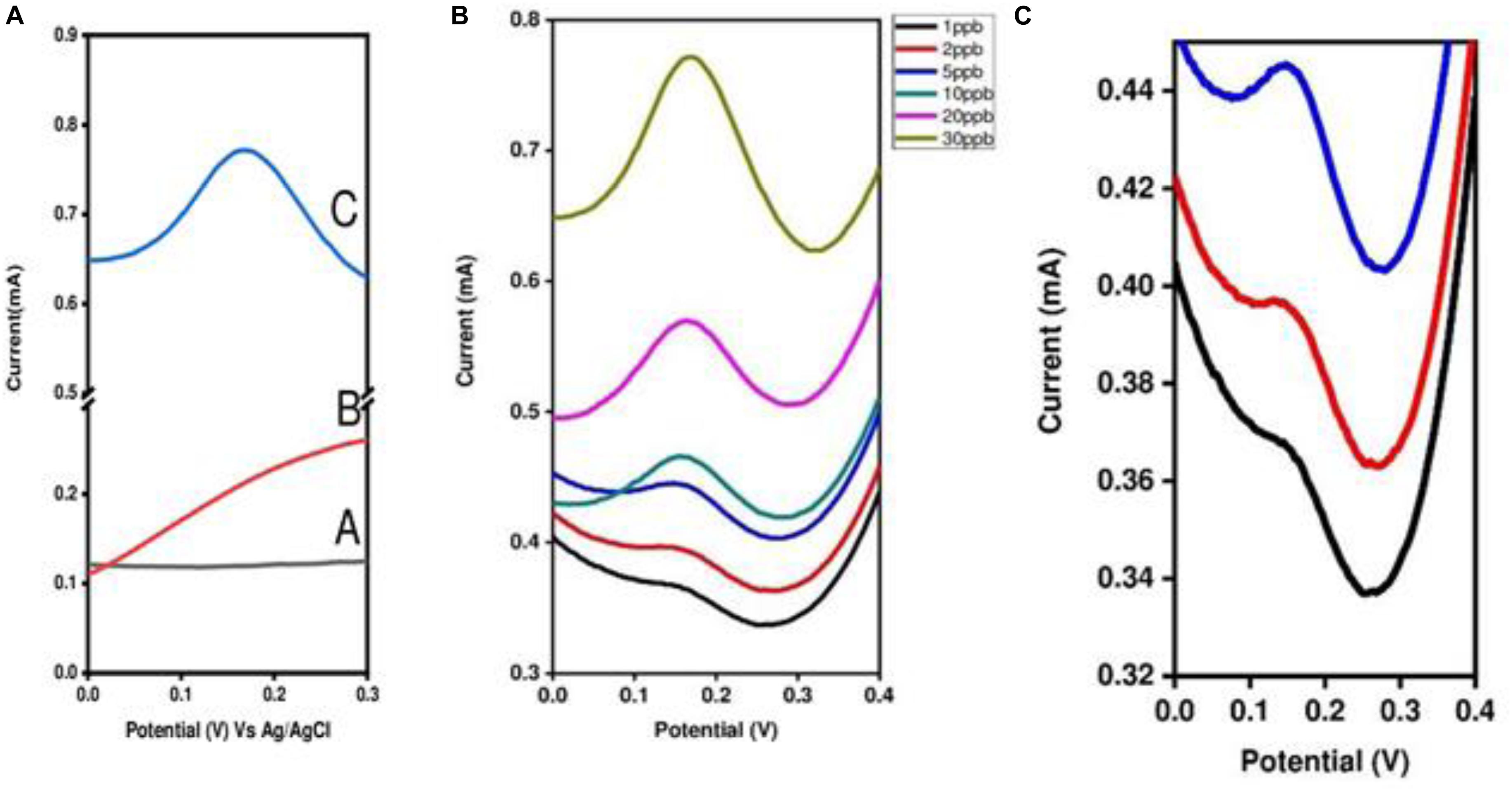
Figure 9. Determination of Hg(II) ions by the DPSV technique (A) with three different electrodes at 30 ppb [(A) PANI/GO composite, (B) only PANI, and (C) EDTA modified PANI/GO composite] and (B) from 1 to 30 ppb with an EDTA modified PANI/GO composite electrode. (C) Enlarged image for 1 to 3 ppb with an EDTA modified PANI/GO composite electrode.
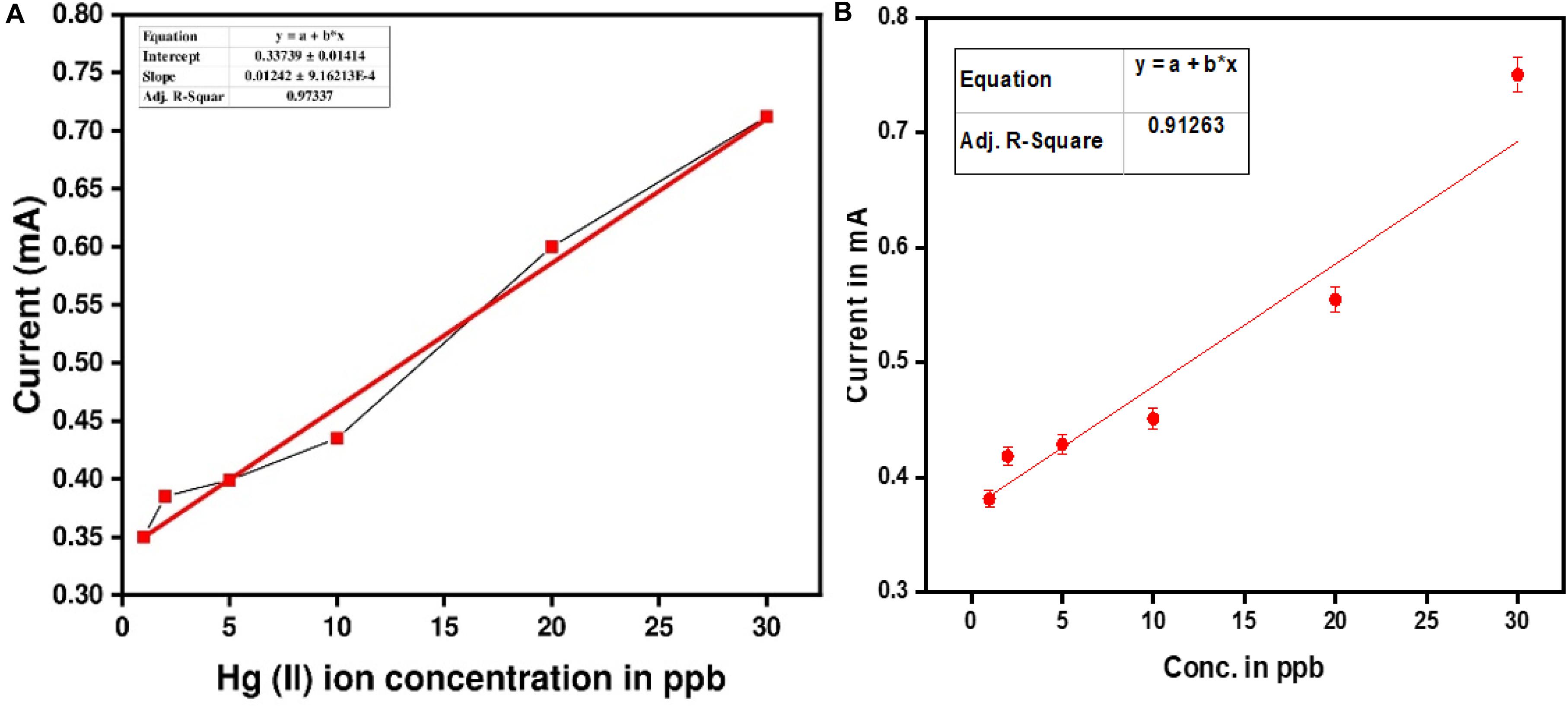
Figure 10. (A) Linear relationship between Hg(II) ion concentration (in ppb) and measured current (mA). (B) Calibration plot of EDTA modified PANI/GO composite.
The limit of detection was determined by utilizing the equation,
Selective determination was carried out from the solution containing Cu, Pb, and Hg ions. For selectivity, the potential (vs. Ag/AgCl) was applied from −0.6 to 0.4 V. The sharp apex is observed at 0.2 V, which clearly indicates the presence of Hg (II) ions (Figure 11). It can be concluded that the EDTA modified PANI/GO composite electrode is selective to Hg (II) ions only.
Conclusion
An EDTA modified PANI/GO composite on stainless steel (SS) electrode for use in the detection of Hg (II) ions was synthesized and characterized effectively. Electron transfer resistance between the redox probe and EDTA modified PANI/GO composite or PANI/GO composite was clearly observed. Modification by the EDTA chelating ligand increases the affinity of PANI/GO composite toward Hg (II) ions. An EDTA modified PANI/GO electrode shows excellent response to Hg (II) ions from 30 to 1 ppb, which is well below MCL (2 ppb). A successful and linear determination of Hg (II) was performed by differential pulse stripping voltammetry (DPSV) down to 1 ppb.
Data Availability Statement
The datasets generated for this study are available on request to the corresponding author.
Author Contributions
MM and MS conceived of the presented idea and developed the theory. MM performed the experiments and computations. HP and GB encouraged the investigation and verified the analytical methods. NI, PS, TA-G, and SS assisted with CV, FTIR, and EIS measurements. All authors discussed the outcomes and added to inscribe the final manuscript. MS contributed to the final version of the manuscript and supervised the project.
Funding
The authors extend their earnest gratitude to the Inter-University Accelerator Center (IUAC), New Delhi, India (UFR no. 62321 and UFR no. 62320), SARTHI-Government of Maharashtra, DST—SERB, New Delhi (Project No. EEQ/2017/000645), UGC—DAE CSR (RRCAT), Indore (Project No. CSR-IC-BL66/CRS-183/2016-17/847), Rashtria Uchachatar Shiksha Abhiyan (RUSA), the UGC-SAP Programme (F.530/16/DRS-I/2016 (SAP-II) Dt.16-04-2016), and DST-FIST (Project No. SR/FST/PSI-210/2016(C) dtd. 16/12/2016) for providing financial support.
Conflict of Interest
The authors declare that the research was conducted in the absence of any commercial or financial relationships that could be construed as a potential conflict of interest.
References
Bánfalvi, G. (2011). “Heavy metals, trace elements and their cellular effects,” in in Cellular Effects of Heavy Metals, ed. G. Banfalvi (Berlin: Springer), 3–28. doi: 10.1007/978-94-007-0428-2_1
Bansod, B., Kumar, T., Thakur, R., Rana, S., and Singh, I. (2017). A review on various electrochemical techniques for heavy metal ions detection with different sensing platforms. Biosens. Bioelectron. 94, 443–455. doi: 10.1016/j.bios.2017.03.031
Chang, J., Zhou, G., Christensen, E. R., Heideman, R., and Chen, J. (2014). Graphene-based sensors for detection of heavy metals in water: a review. Anal. Bioanal. Chem. 406, 3957–3975. doi: 10.1007/s00216-014-7804-x
Charlier, J.-C., Eklund, P., Zhu, J., and Ferrari, A. (2007). “Electron and phonon properties of graphene: their relationship with carbon nanotubes,” in Carbon Nanotubes, eds M. Endo, S. Iijima, and M. S. Dresselhaus (Berlin: Springer), 673–709. doi: 10.1007/978-3-540-72865-8_21
Chen, K., Lu, G., Chang, J., Mao, S., Yu, K., Cui, S., et al. (2012). Hg (II) ion detection using thermally reduced graphene oxide decorated with functionalized gold nanoparticles. Anal. Chem. 84, 4057–4062. doi: 10.1021/ac3000336
Choi, H.-J., Jeon, I.-Y., Kang, S.-W., and Baek, J.-B. (2011). Electrochemical activity of a polyaniline/polyaniline-grafted multiwalled carbon nanotube mixture produced by a simple suspension polymerization. Electroch. Acta 56, 10023–10031. doi: 10.1016/j.electacta.2011.08.103
Das, T. K., and Prusty, S. (2012). Review on conducting polymers and their applications. Polym. Plast. Technol. Eng. 51, 1487–1500.
Deshmukh, M. A., Celiesiute, R., Ramanaviciene, A., Shirsat, M. D., and Ramanavicius, A. (2018a). EDTA_PANI/SWCNTs nanocomposite modified electrode for electrochemical determination of copper (II), lead (II) and mercury (II) ions. Electroch. Acta 259, 930–938. doi: 10.1016/j.electacta.2017.10.131
Deshmukh, M. A., Patil, H. K., Bodkhe, G. A., Yasuzawa, M., Koinkar, P., Ramanaviciene, A., et al. (2018b). EDTA-modified PANI/SWNTs nanocomposite for differential pulse voltammetry based determination of Cu (II) ions. Sens. Actuators B Chem. 260, 331–338. doi: 10.1016/j.snb.2017.12.160
Dreyer, D. R., Park, S., Bielawski, C. W., and Ruoff, R. S. (2010). The chemistry of graphene oxide. Chem. Soc. Rev. 39, 228–240. doi: 10.1039/b917103g
Gilje, S., Han, S., Wang, M., Wang, K. L., and Kaner, R. B. (2007). A chemical route to graphene for device applications. Nano Lett. 7, 3394–3398. doi: 10.1021/nl0717715
Gumpu, M. B., Sethuraman, S., Krishnan, U. M., and Rayappan, J. B. B. (2015). A review on detection of heavy metal ions in water–an electrochemical approach. Sens. Actuators B Chem. 213, 515–533. doi: 10.1016/j.snb.2015.02.122
Gumpu, M. B., Veerapandian, M., Krishnan, U. M., and Rayappan, J. B. B. (2017). Simultaneous electrochemical detection of Cd (II), Pb (II), As (III) and Hg (II) ions using ruthenium (II)-textured graphene oxide nanocomposite. Talanta 162, 574–582. doi: 10.1016/j.talanta.2016.10.076
Herrera-Herrera, A. V., González-Curbelo, M. Á, Hernández-Borges, J., and Rodríguez-Delgado, M. Á (2012). Carbon nanotubes applications in separation science: a review. Anal. Chim. Acta 734, 1–30. doi: 10.1016/j.aca.2012.04.035
Jan, A. T., Azam, M., Siddiqui, K., Ali, A., Choi, I., and Haq, Q. M. (2015). Heavy metals and human health: mechanistic insight into toxicity and counter defense system of antioxidants. Int. J. Mol. Sci. 16, 29592–29630. doi: 10.3390/ijms161226183
Lansdown, A. B. (2013). The Carcinogenicity of Metals: Human Risk Through Occupational and Environmental Exposure. London: Royal Society of Chemistry.
Lei, W., Si, W., Xu, Y., Gu, Z., and Hao, Q. (2014). Conducting polymer composites with graphene for use in chemical sensors and biosensors. Microch. Acta 181, 707–722. doi: 10.1007/s00604-014-1160-6
McAllister, M. J., Li, J.-L., Adamson, D. H., Schniepp, H. C., Abdala, A. A., Liu, J., et al. (2007). Single sheet functionalized graphene by oxidation and thermal expansion of graphite. Chem. Mater. 19, 4396–4404. doi: 10.1021/cm0630800
Musameh, M. M., Hickey, M., and Kyratzis, I. L. (2011). Carbon nanotube-based extraction and electrochemical detection of heavy metals. Res. Chem. Int. 37, 675–689. doi: 10.1007/s11164-011-0307-x
Nagajyoti, P. C., Lee, K. D., and Sreekanth, T. (2010). Heavy metals, occurrence and toxicity for plants: a review. Environ. Chem. Lett. 8, 199–216. doi: 10.1007/s10311-010-0297-8
Oztekin, Y., Yazicigil, Z., Ramanaviciene, A., and Ramanavicius, A. (2011). Polyphenol-modified glassy carbon electrodes for copper detection. Sensors and Actuators B Chemical 152, 37–48. doi: 10.1016/j.snb.2010.09.057
Patil, H. K., Deshmukh, M. A., Bodkhe, G. A., Shirsat, S. M., Asokan, K., and Shirsat, M. D. (2018). Dimethylglyoxime modified swift heavy oxygen ions irradiated polyaniline/single walled carbon nanotubes composite electrode for detection of cobalt ions. Materi. Res. Express 5:065048. doi: 10.1088/2053-1591/aaccb3
Rao, C. E. N. E. R., Sood, A. E. K., Subrahmanyam, K. E. S., and Govindaraj, A. (2009). Graphene: the new two-dimensional nanomaterial. Angew. Chem. Int. Ed. 48, 7752–7777. doi: 10.1080/1061186X.2018.1437920
Roth, S., and Carroll, D. (2015). One-Dimensional Metals: Conjugated Polymers, Organic Crystals, Carbon Nanotubes and Graphene. Hoboken, NJ: John Wiley & Sons.
Shahriary, L., and Athawale, A. A. (2014). Graphene oxide synthesized by using modified hummers approach. Int. J. Renew. Energy Environ. Eng. 2, 58–63.
Stankovich, S., Piner, R. D., Chen, X., Wu, N., Nguyen, S. T., and Ruoff, R. S. (2006). Stable aqueous dispersions of graphitic nanoplatelets via the reduction of exfoliated graphite oxide in the presence of poly (sodium 4-styrenesulfonate). J. Mater. Chem. 16, 155–158. doi: 10.1039/b512799h
Strong, V., Dubin, S., El-Kady, M. F., Lech, A., Wang, Y., Weiller, B. H., et al. (2012). Patterning and electronic tuning of laser scribed graphene for flexible all-carbon devices. ACS Nano 6, 1395–1403. doi: 10.1021/nn204200w
Tekaya, N., Saiapina, O., Ouada, H. B., Lagarde, F., Ouada, H. B., and Jaffrezic-Renault, N. (2013). Ultra-sensitive conductometric detection of pesticides based on inhibition of esterase activity in Arthrospira platensis. Environ. Pollut. 178, 182–188. doi: 10.1016/j.envpol.2013.03.013
Turdean, G. L. (2011). Design and development of biosensors for the detection of heavy metal toxicity. Int. J. Electrochem. 2011:5.
Ullah, N., Mansha, M., Khan, I., and Qurashi, A. (2018). Nanomaterial-based optical chemical sensors for the detection of heavy metals in water: recent advances and challenges. TrAC Trends Anal. Chem. 100, 155–166.
Vadivel, S., Theerthagiri, J., Madhavan, J., and Maruthamani, D. (2016). Synthesis of polyaniline/graphene oxide composite via ultrasonication method for photocatalytic applications. Mater. Focus 5, 393–397. doi: 10.1166/mat.2016.1367
Wang, H., Hao, Q., Yang, X., Lu, L., and Wang, X. (2010). Effect of graphene oxide on the properties of its composite with polyaniline. ACS App. Mater. Interfaces 2, 821–828. doi: 10.1021/am900815k
Yi, W., He, Z., Fei, J., and He, X. (2019). Sensitive electrochemical sensor based on poly (L-glutamic acid)/graphene oxide composite material for simultaneous detection of heavy metal ions. RSC Adv. 9, 17325–17334. doi: 10.1039/c9ra01891c
Zagal, J., Del Rio, R., Retamal, B., and Biaggio, S. (1996). Stability and electrical properties of polyaniline films formed with EDTA and FeEDTA in the electrolyte. J. Appl. Electroch. 26, 95–101. doi: 10.1007/bf00248194
Zhang, Y.-J., Lin, Y.-W., Chang, C.-C., and Wu, T.-M. (2011). Conducting and magnetic behaviors of polyaniline coated multi-walled carbon nanotube composites containing monodispersed magnetite nanoparticles. Synth. Metals 161, 937–942. doi: 10.1016/j.synthmet.2011.02.026
Zhao, D., Guo, X., Wang, T., Alvarez, N., Shanov, V. N., and Heineman, W. R. (2014). Simultaneous detection of heavy metals by anodic stripping voltammetry using carbon nanotube thread. Electroanalysis 26, 488–496. doi: 10.1021/acs.analchem.6b04724
Zhou, Q., Li, Y., Huang, L., Li, C., and Shi, G. (2014). Three-dimensional porous graphene/polyaniline composites for high-rate electrochemical capacitors. J. Mater. Chem. A 2, 17489–17494. doi: 10.1039/c4ta03639e
Keywords: PANI, GO, EDTA_PANI/GO, EIS, DPSV, Hg (II) ion detection
Citation: Mahadik M, Patil H, Bodkhe G, Ingle N, Sayyad P, Al-Gahaouri T, Shirsat SM and Shirsat M (2020) EDTA Modified PANI/GO Composite Based Detection of Hg (II) Ions. Front. Mater. 7:81. doi: 10.3389/fmats.2020.00081
Received: 10 December 2019; Accepted: 19 March 2020;
Published: 17 April 2020.
Edited by:
Ilkwon Oh, Korea Advanced Institute of Science and Technology, South KoreaReviewed by:
Jia Chu, Xi’an University of Science and Technology, ChinaLuwei Zhou, Fudan University, China
Copyright © 2020 Mahadik, Patil, Bodkhe, Ingle, Sayyad, Al-Gahaouri, Shirsat and Shirsat. This is an open-access article distributed under the terms of the Creative Commons Attribution License (CC BY). The use, distribution or reproduction in other forums is permitted, provided the original author(s) and the copyright owner(s) are credited and that the original publication in this journal is cited, in accordance with accepted academic practice. No use, distribution or reproduction is permitted which does not comply with these terms.
*Correspondence: Mahendra Shirsat, bWRzaGlyc2F0LnBoeUBiYW11LmFjLmlu