- 1Laboratory of Thin Film Techniques and Optical Test, School of Photoelectrical Engineering, Xi'an Technological University, Xi'an, China
- 2Frontier Institute of Science and Technology, Xi'an Jiaotong University, Xi'an, China
Perovskite ferroelectic oxides with simultaneous highly thermal stable dielectric property and piezoelectric response are promising candidate for advanced energy, dielectric, and smart devices. The (1-x)[0.98[(K0.5Na0.5)NbO3]-0.02(BaNi0.5Nb0.5O3)]-xLiNbO3 (abbreviated as (1-x)KNBNNO-xLiNbO3; x = 0.00, 0.02, 0.04, 0.06, 0.08) lead-free multifunction ferroelectric ceramic is synthesized by solid-state reaction method. XRD analysis reveals that the samples exhibit perovskite structure with 0 ≤ x ≤ 0.06, and the second phase K3Li2Nb5O15 appears at x = 0.08. The scanning electron microscopy image show that the grain size of ceramics increases from 0.65 to 3.58 μm with LiNbO3 content increasing. Meanwhile, the Curie temperature (TC) shifts to a higher temperature (~ 427°C for x = 0.06). A high dielectric thermal stability of Δε/ ≤ ±10%, with a high dielectric permittivity (~1,400), is achieved at x = 0.06 over a wide temperature range of ~40–348°C with d33 of ~160 pC·N−1, and a remnant polarization (Pr) of 20.5 μC·cm−2. This work shows that this multifunction material could be applied in sensor to efficiently covert both solar and kinetic energies into electricity over a wide temperature range.
Introduction
Perovskite oxides, with the ABO3 topological structure, have been recognized as the smart platform for the next generation advanced multifunctional energy conversion and/or storage devices (Bai et al., 2017, 2019; Hao et al., 2019). In particular, the subgroup of ferroelectric perovskite materials, with non-centrosymmetric crystallographic structure (Glazer, 1975), are appealing for these multifunctional devices due to their inherent multi-stimuli responsive characteristics. The ferroelectrics are sensitive to external and/or internal stimuli, such as temperature, light, magnetic field, stress, and chemical doping (Bai et al., 2018; Zheng et al., 2018), owing to their highly structural tolerance. Very recently, various types of ferroelectric systems have been used for these promising applications. For example, Pb(Zr0.53Ti0.47)O3 ceramics mediated with Pb(Fe0.5Ta0.5)O3 or PbTiO3-0.35Bi(Ni2/3+xNb1/3−x)O3−δ ceramics (Evans et al., 2013; Liu et al., 2015), (1-x)KNbO3-xBaNi1/2Nb1/2O3−δ (KBNNO) ceramics (Bai et al., 2019), (1–x) Na0.5Bi0.5TiO3-xBa(Ti0.5Ni0.5)O3−δ ceramics (Xiao et al., 2019), and (K0.5Na0.5)NbO3 doped with 2 mol% Ba(Ni0.5Nb0.5)O3−δ ceramics (KNBNNO ceramics) (Bai et al., 2017; Zhong et al., 2019).
However, lead-based ceramics have urgent toxicity for the environment and/or human development due to their intrinsic volatility under extreme conditions (Hong et al., 2016; Zheng et al., 2018). The emerging of (K0.5Na0.5)NbO3 (KNN)-based lead-free system would be a remarkable alternative for these drawbacks due to its high-performance piezoelectric, ferroelectric properties, and high curie temperature (Du et al., 2007b; Ding et al., 2018; Zhao et al., 2019). Furthermore, the piezoelectricity and temperature stability substantially determine the macroscopical performance of ferroelectric materials (Zhao et al., 2007; Sun et al., 2016; Yan et al., 2017, 2018c). According to the structure-activity relationship, chemical modification is an effective method to enhance the temperature stability and piezoelectric properties of KNN-based ceramics, including the formation of solid solutions with other compounds, such as LiNbO3 (Wang and Li, 2010a; Long et al., 2016), LiTaO3 (Chang et al., 2007; Zhao et al., 2007), LiSbO3 (Shi et al., 2014), BaTiO3 (Kim et al., 2016), Bi0.5Na0.5TiO3 (Zuo et al., 2007; Liu et al., 2014, 2017), or a combination of multiple additives (Mazhao et al., 2015). Among them, the lithium niobate (LiNbO3), not composing of the expensive Ta and toxic Sb elements, could simultaneously increase the piezoelectric coefficient (d33) and the Curie temperature (TC) instead of sacrificing TC for a higher d33. Moreover, the Li ion is beneficial to reduce the sintering temperature of ceramics (Wang and Li, 2010a). Unfortunately, few studies have focus to the thermal stability of KNN-based functional ceramics, which is the pivotal parameter for practical device working. Therefore, it is of great interest to design KNN-based multifunction materials with both improved piezoelectricity properties and favored temperature stability. The KNBNNO system is a kind of excellent multifunctional ferroelectric material with narrower bandgap (1.6 eV), large piezoelectric coefficient (100 pC·N−1), large pyroelectric coefficient (130 μC·K−1·m−2) but smaller remanent polarization (11.3 μC·cm−2). The synergetic enhancement of as-mentioned piezoelectricity and thermal stability would make this system to be a paradigm for smart platform applications (Bai et al., 2017).
In this work, (1-x)[0.98[(K0.5Na0.5)NbO3]-0.02(BaNi0.5Nb0.5O3)]-xLiNbO3 (abbreviated as (1-x)KNBNNO-xLiNbO3; x = 0.00, 0.02, 0.04, 0.06, 0.08) ceramics were prepared by solid-state reaction method through A-site substitution by Li+. The results show that both piezoelectricity and thermal stability of KNBNNO-LiNbO3 system can be enhanced simultaneously. The underlying physical picture regarding these properties is the appearing of morphotropic phase boundary (MPB) between orthorhombic and tetragonal phases by LiNbO3 doping. Furthermore, the phase structure, surface morphology, and electric properties (such as dielectric, ferroelectric and piezoelectric properties) were studied. This work would promote the development of lead-free multifunctional ferroelectrics over a broad temperature range.
Materials and Methods
Materials
KNBNNO-LiNbO3 lead-free ceramics were synthesized by solid-state reaction method using the analysis reagent of oxides and carbonates: Na2CO3 (99.8%), K2CO3 (99.0%), Nb2O5 (99.5%), Li2CO3 (98.0%), BaCO3 (99.0%), and NiO (98.0%). The powders were mixed by stoichiometric ratio, then ball-milled in ethanol with stabilized zirconia media for 12 h. After drying at 90°C, the powder was calcined at 850°C for 3 h using alumina crucibles before milled for 12 h. The 6 wt.% PVA was mixed into the powders after drying and sieving, and the pellets were pressed at 200 MPa. Finally, the pressed disks were sintered between 1,040°C and 1,180°C for 2 h. In order to weaken the evaporation of alkalis during sintering, the disks were embedded in precursor powders.
Methods
The bulk densities were obtained by Archimedes' Method. The phase structure of samples was measured using X-ray diffraction spectrometry (XRD, Shimadzu, LabX XRD-600) with Cu-Kα radiation (λ = 1.54056 Å). The morphology and grain size were investigated using scanning electron microscopy (SEM, JEOL, JSM EMP-800 Tokyo). The remaining disks were polished and coated with high temperature sliver electrodes for electrical property analysis. The dielectric property was measured at 10 kHz using an LCR meter (Agilent, Agilent 4980A, Santa Clara) from 40°C to 480°C, while the ferroelectric property was measured using a ferroelectric analyzer (axiACCT, TF-2000, Germany). The piezoelectric coefficient d33 was measured by Berlincourt-type quasi-static meter, before that, the samples were poled at 150°C for 30 min in silicon oil, then keep pressure and cool to room temperature for testing, the poling electric field was 40 kV/cm.
Results and Discussion
The XRD patterns of KNBNNO-xLiNbO3 solid solution with 0 ≤ x ≤ 0.08 at room temperature are shown in Figure 1A. Only pure perovskite structure is formed at 0 ≤ x ≤ 0.06, and the original diffraction peak moves toward lower angles upon the increasing of x. This characteristic could be attributed to the K+ (1.33 Å) and Na+ (0.97 Å) ions are substituted by Li+ (0.90 Å) ions at the A-site of perovskite structure (Wang and Li, 2007). However, the second phase of tetragonal tungsten bronze K3Li2Nb5O15 (ICDD: 52-0157) appears when x = 0.08. This phenomenon results from the limited solid solubility of Li into the A-sites of KNBNNO, and the different space group between KNN (Amm2) and LiNbO3 (R3c) (Yan et al., 2004).
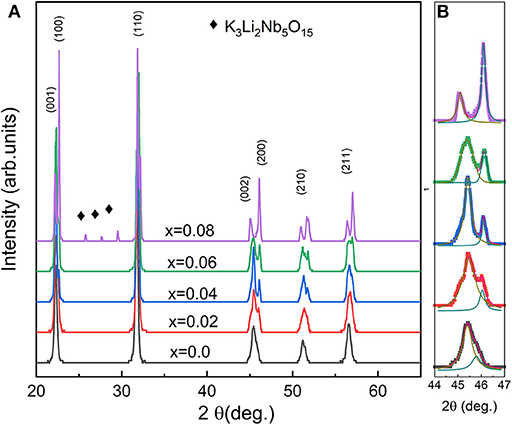
Figure 1. (A) XRD patterns of (1-x)KNBNNO-xLiNbO3 ceramics (x = 0–0.08); (B) enlarged XRD patterns for 2θ = 44–47°.
The relatively integrated intensity of the enlarged XRD patterns between 44° and 47° were fitted using the Lorentz function (see Figure 1B). It deserves to be noted that the theoretical ratio of I(200) / I(002) is 1:2 and 2:1 for an orthorhombic and tetragonal phase, respectively (Liu et al., 2012). Therefore, the pure KNBNNO sample is orthorhombic phase according to the fitting data (Cheng et al., 2012). However, the intensity ratio of I(200) / I(002) gradually raises with the increasing of doping content. It implies that the obtained phase is combined with orthorhombic and tetragonal phases when x = 0.02–0.06 (Liu et al., 2012). This is because the integrated intensity of these two peaks approaches to ~ 1 (Zhang et al., 2006). In this regard, a MPB region has been established in the range of x = 0.02–0.06, which would significantly affect the piezoelectric and dielectric properties (Saito and Takao, 2006). The discrepancy of (002) peaks at x = 0.06 might be due to the mild texturing that formed on the surface of solid solution, as proved in Figure 2C. When x = 0.08, the tetragonal phase is appeared with an intensity ratio of ~ 2:1.
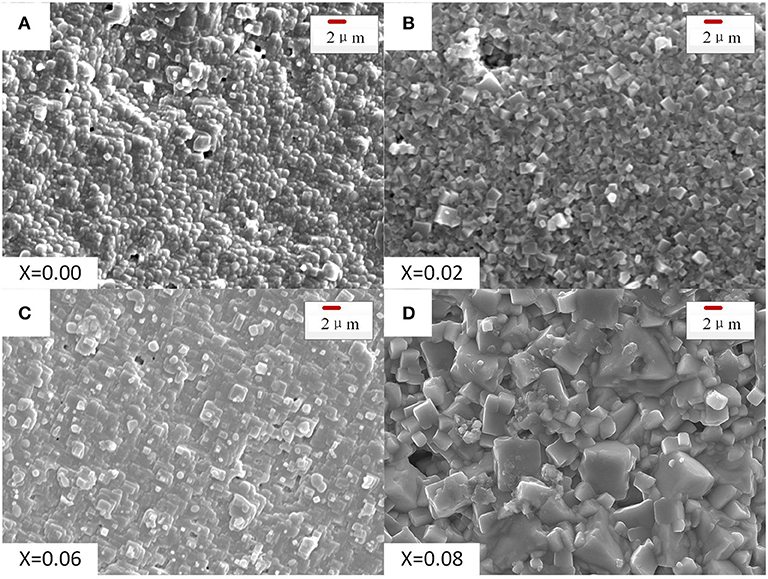
Figure 2. SEM images of (1-x)KNBNNO-xLiNbO3 ceramics for (A) x = 0.00, (B) x = 0.02, (C) x = 0.06, and (D) x = 0.08.
Figure 2 shows the morphology of the (1-x)KNNBNNO-xLiNbO3 solid solution with various x values sintered at their optimized temperatures. It demonstrates most of the regular rectangular grains surrounded by tiny grains. The average grain size increases from ~ 0.65 μm (x = 0.00) to ~ 3.58 μm (x = 0.08) with increasing LiNbO3 content. Besides, the high relative density (>96%) has been obtained. Specifically, the densities of as-synthesized ceramics first increase from 4.35 g·cm−3 (x = 0.00) to 4.46 g·cm−3 (x = 0.06), and then decreased to 4.33 g·cm−3 at x = 0.08. The substitution of Li ions would promote the formation of a transient liquid phase during the sintering process, which could benefit grain growth and compact structure (Zhao et al., 2019). The reason for the decrease in density may be due to the appearance of a low-density second phase K3Li2Nb5O15 (theoretical density: 4.376 g·cm−3).
The effect of temperature on the dielectric properties at 10 kHz for unpoled (1-x)KNBNNO-xLiNbO3 ceramics with 0 ≤ x ≤ 0.08 is shown in Figure 3a. Two dielectric peaks are observed from 40°C to 480°C, corresponding to the phase transition temperatures, from the orthorhombic phase to the tetragonal phase (marked as TO−T) and from the tetragonal phase to the cubic phase (marked as TC), respectively (Zhang et al., 2006; Hao et al., 2015). Meanwhile, the inverse dielectric constant (1/ε) as a function of temperature at 10 kHz has been clearly shown in Figure 3b. The dielectric permittivity above the curie point follows the Curie-Weiss law, as shown in Equation (1).
where T0 is the Curie-Weiss temperature and C is the Curie Weiss constant. And the Curie point TC can be got from the curve (Bokov and Ye, 2012). The TO−T also corresponds with another peak of the curve. The phase transition temperature of pure KNBNNO (x = 0.00) ceramics can be observed at TC = 375.5°C and TO−T = 148.5°C, respectively. Interestingly, the ceramics at x = 0.06 shows the lowest TO−T value, being near to room temperature, and a highest TC, close to 427°C. Overall, the TO−T decreases largely from 148.5°C to 57°C, while the TC increases from 375.5°C to 423°C with the increasing LiNbO3 content (0 ≤ x ≤ 0.06). It is likely due to the smaller radius of the Li ion than that of the K and Na ions, consequentially, the tolerance factor of the perovskite structure decreases upon increasing x. Therefore, the tetragonal distortion of the sample should be enhanced, where TC shifts to a higher temperature, while TO−T moves to a lower temperature, as shown in Figure 3c (Yan et al., 2018a,b). Furthermore, both the high TC of LiNbO3 (~1,200°C) and the large crystal anisotropy could also induce this effect (Acosta et al., 2017).
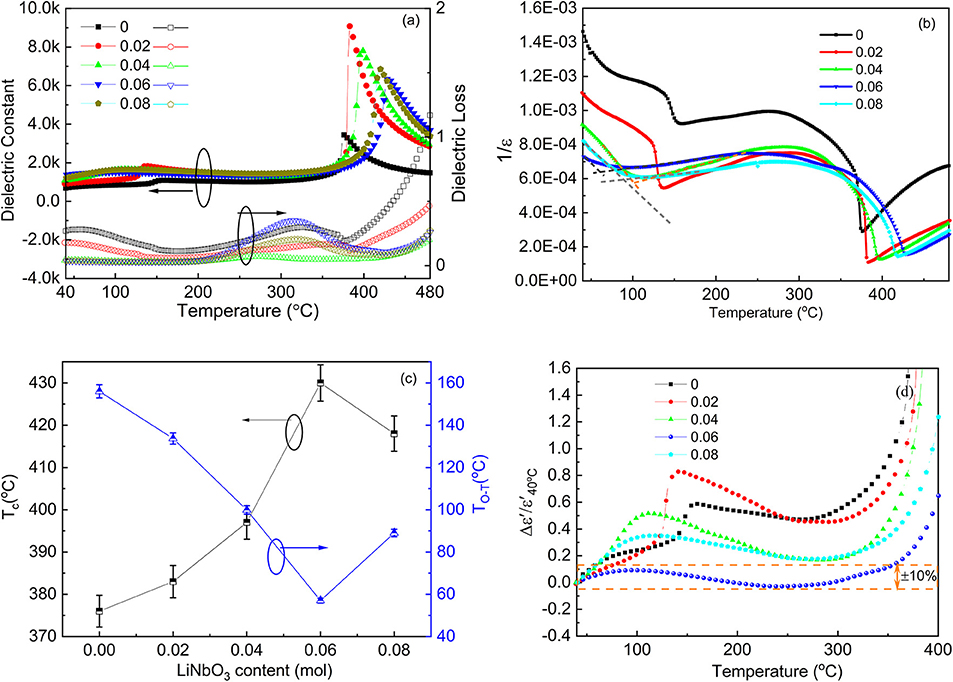
Figure 3. (a) Effect of temperature on the dielectric properties at 10 kHz for KNBNNO-xLiNbO3 ceramics with 0 ≤ x ≤ 0.08; (b) the inverse dielectric constant (1/ε) varies with temperature at 10 kHz; (c)TC and TO−T change with LiNbO3 content; (d) Thermal stability of the relative permittivity (Δε/) change with temperature for the (1-x)KNBNNO-xLiNbO3 (x = 0.00–0.08) ceramics at 10 kHz.
Figure 3d illustrates the thermal stability of the relative permittivity (Δε/ε 40°C) between 40°C and 400°C for the (1-x)KNBNNO-xLiNbO3 (x = 0.00–0.08) ceramics at 10 kHz. The doping of LiNbO3 could reduce the fluctuation, namely, enhancing the thermal stability of the (1-x)KNBNNO-xLiNbO3 solid solution (Δε/ε40°C ≤ ±10%). As a result, the 0.94KNBNNO-0.06LiNbO3 composition exhibits large relative permittivity (~1,400), optimum thermal stability (Δε/ε40°C ≤ ±10%) at 40–348°C, and low dielectric loss (<2.5%). It is well established that this composition will be a promising candidate for advanced smart devices operating at a broad temperature range.
The piezoelectric properties for the (1-x)KNBNNO-xLiNbO3 (x = 0.00–0.08) ceramics are shown in Figure 4. The piezoelectric coefficient of the samples increase to an optimal value (~160 pC·N−1) at x = 0.06, and then it drops to 147 pC·N−1 at x = 0.08. The pure KNBNNO composition also exhibits similar piezoelectric property compared with the existing results (Wang et al., 2013). The piezoelectric properties of the samples could be influenced by the existing of MPB (between orthorhombic and tetragonal). The emerging of more polarization vectors than that of single-phase favors more dipole reorientations, which largely improves the piezoelectric property (Wu et al., 2016; Xu et al., 2016; Acosta et al., 2017; Lv et al., 2019). Moreover, the piezoelectric property might be affected, to some extent, by the dense structure and grain size (Song et al., 2007).
Figure 5A illustrates the polarization-electric field hysteresis loops of the (1-x)KNBNNO-xLiNbO3 (x = 0.00–0.08) ceramics at 10 Hz under room temperature. These samples exhibit typical P-E hysteresis loops. Figure 5B shows that the Pr value first slightly increases during x = 0.00–0.06 and then decreases to 11.18 μC·cm−1 at x = 0.08. The obtained maximum value of 20.5 μC·cm−2 appeared at x = 0.06. The Ec value decreases from 20 kV·cm−1 (x = 0.00) to 15 kV·cm−1 (x = 0.08). That can be ascribed to more polarization states caused by the coexistence of O-T phase at MPB, where domain wall movement would be easier and coercive field would be lower (Du et al., 2007a; Wang and Li, 2010b). On the other hand, when the grain size of samples increases with the improving of LiNbO3 content, the density of grain boundary would decrease. Accordingly, the switching of the domain will much easier, leading to the reduction of the coercive field and increasing of remnant polarization. The piezoelectric and dielectric properties have a similar physical mechanism.
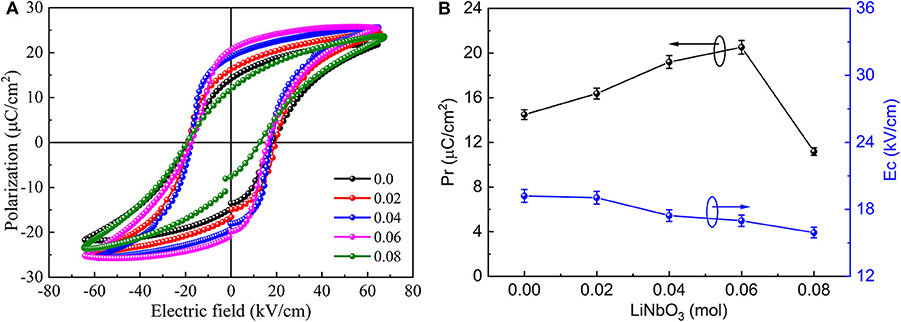
Figure 5. (A) Polarization-electric field hysteresis loops of (1-x)KNBNNO-xLiNbO3 (x = 0.00–0.08) ceramics at 10 Hz under room temperature; (B) Pr and Ec change with LiNbO3 content.
Conclusions
(1-x)KNBNNO-xLiNbO3 ceramics (x = 0–0.08) were successfully synthesized via the traditional solid-state reaction method. The pure perovskite structure was obtained at 0 ≤ x ≤ 0.06. However, the second phase of K3Li2Nb5O15 with tetragonal tungsten bronze structure was formed due to the solubility limit of Li-ions in the A-sites of solid solution (x = 0.08). There exist excellent piezoelectric and dielectric temperature stability properties with a high Curie temperature at 0.02 ≤ x ≤ 0.06, owing to the formation of MPB between the orthorhombic and tetragonal phases. 0.94KNBNNO-0.06LiNbO3 ceramics exhibits excellent electrical performance of εr~1400 (Δε/ε40°C ≤ ±10%), tanδ < 0.02 at 40–348°C, and d33 of ~160 pC N−1, Pr of 20.5 μC cm−2 at room temperature. It is uncovered that this material could be used in energy, sensor, and smart devices, operating over a wide temperature range.
Data Availability Statement
The datasets generated for this study are available on request to the corresponding author.
Author Contributions
HJ and WL conceived and designed the experiments. HJ, HM, and ZC performed the experiments. LX, ZD, LP, and JX analyzed the data. HJ and LX wrote and revised the paper.
Funding
This work was supported by the National Natural Science Foundation of China (Grant No. 61601359), Key Science and Technology Program Funded by Shaanxi Province Science and Technology Bureau (Project No. 16JS038), Natural Science Basic Research Plan in Shaanxi Province of China (Project No. 2019JM-300), and Principal's Foundation from Xi'an Technological University (Project No. XAGDXJJ15003).
Conflict of Interest
The authors declare that the research was conducted in the absence of any commercial or financial relationships that could be construed as a potential conflict of interest.
References
Acosta, M., Novak, N., Rojas, V., Patel, S., Vaish, R., Koruza, J., et al. (2017). BaTiO3-based piezoelectrics: Fundamentals, current status, and perspectives. Appl. Phys. Rev. 4:041305. doi: 10.1063/1.4990046
Bai, Y., Jantunen, H., and Juuti, J. (2018). Energy harvesting research: the road from single source to multisource. Adv. Mater. 30:1707271. doi: 10.1002/adma.201707271
Bai, Y., Tofel, P., Palosaari, J., Jantunen, H., and Juuti, J. (2017). A game changer: a multifunctional perovskite exhibiting giant ferroelectricity and narrow bandgap with potential application in a truly monolithic multienergy harvester or sensor. Adv. Mater. 29:1700767. doi: 10.1002/adma.201700767
Bai, Y., Xiang, H., Jantunen, H., and Juuti, J. (2019). Multi-functional perovskites–an investigation of compositional and processing influence on microstructure, dielectric and ferroelectric properties. Eur. Phys. J-Spec. Top. 228, 1555–1573. doi: 10.1140/epjst/e2019-800132-8
Bokov, A. A., and Ye, Z. G. (2012). Dielectric relaxation in relaxor ferroelectrics. J. Adv. Dielectr. 2:1241010. doi: 10.1142/S2010135X1241010X
Chang, Y., Yang, Z., Hou, Y., Liu, Z., and Wang, Z. (2007). Effects of Li content on the phase structure and electrical properties of lead-free (K0.46−x/2Na0.54−x/2Lix)(Nb0.76Ta0.20Sb0.04)O3 ceramics. Appl. Phys. Lett. 90:232905. doi: 10.1063/1.2746411
Cheng, L. Q., Zhou, J. J., Wang, K., Li, J. F., and Wang, Q. M. (2012). Influence of ball milling on sintering behavior and electrical properties of (Li, Na, K)NbO3 lead-free piezoceramics. J. Mater. Sci. 47, 6908–6914. doi: 10.1007/s10853-012-6635-4
Ding, Y., Zheng, T., Xie, R., Lv, X., Yin, J., and Wu, J. (2018). High-performance potassium sodium niobate-based lead-free materials without antimony. J. Mater. Sci-Mater. El. 29, 14487–14494. doi: 10.1007/s10854-018-9582-2
Du, H., Tang, F., Liu, D., Zhu, D., Zhou, W., and Qu, S. (2007a). The microstructure and ferroelectric properties of (K0.5Na0.5)NbO3-LiNbO3 lead-free piezoelectric ceramics. Mater. Sci. Eng. B, 136, 165–169. doi: 10.1016/j.mseb.2006.09.031
Du, H., Zhou, W., Luo, F., Zhu, D., Qu, S., and Pei, Z. (2007b). An approach to further improve piezoelectric properties of (K0.5Na0.5)NbO3-based lead-free ceramics. Appl. Phys. Lett. 91:202907. doi: 10.1063/1.2815750
Evans, D. M., Schilling, A., Kumar, A., Sanchez, D., Ortega, N., Arredondo, M., et al. (2013). Magnetic switching of ferroelectric domains at room temperature in multiferroic PZTFT. Nat. Commun. 4:1534. doi: 10.1038/ncomms2548
Glazer, A. M. (1975). Simple ways of determining perovskite structures. Acta Crystallograph. Sect. A Cryst. Phys. Diffr. Theoret. Gen. Crystallogr. 31, 756–762. doi: 10.1107/S0567739475001635
Hao, J., Li, W., Zhai, J., and Chen, H. (2019). Progress in high-strain perovskite piezoelectric ceramics. Mater. Sci. Eng. R, 135, 1–57. doi: 10.1016/j.mser.2018.08.001
Hao, J., Xu, Z., Chu, R., Li, W., and Fu, P. (2015). Good temperature stability and fatigue-free behavior in Sm2O3-modified 0.948 (K0.5Na0.5)NbO3-0.052 LiSbO3 lead-free piezoelectric ceramics. Mater. Res. Bull. 65, 94–102. doi: 10.1016/j.materresbull.2015.01.044
Hong, C. H., Kim, H. P., Choi, B. Y., Han, H. S., Son, J. S., Ahn, C. W., et al. (2016). Lead-free piezoceramics–Where to move on? J. Mat. 2, 1–24. doi: 10.1016/j.jmat.2015.12.002
Kim, J., Ryu, J., Jo, S. H., and Lee, H. (2016). Thermal stability and local atomic structure of (Na0.5K0.5)NbO3 doped BaTiO3 ceramics. Ceram. Int. 42, 11739–11742. doi: 10.1016/j.ceramint.2016.04.093
Liu, H., Chen, J., Ren, Y., Zhang, L., Pan, Z., Fan, L., et al. (2015). Large photovoltage and controllable photovoltaic effect in PbTiO3-Bi(Ni2/3+xNb1/3−x) O3−δ ferroelectrics. Adv. Electron. Mater. 1:1400051. doi: 10.1002/aelm.201400051
Liu, L., Huang, Y., Li, Y., Fang, L., Dammak, H., Fan, H., et al. (2012). Orthorhombic to tetragonal structural phase transition in Na0.5K0.5NbO3-based ceramics. Mater. Lett. 68, 300–302. doi: 10.1016/j.matlet.2011.10.103
Liu, L., Knapp, M., Ehrenberg, H., Fang, L., Fan, H., Schmitt, L. A., et al. (2017). Average vs. local structure and composition-property phase diagram of K0.5Na0.5NbO3-Bi½Na½TiO3 system. J. Eur. Ceram. Soc. 37, 1387–1399. doi: 10.1016/j.jeurceramsoc.2016.11.024
Liu, L., Shi, D., Knapp, M., Ehrenberg, H., Fang, L., and Chen, J. (2014). Large strain response based on relaxor-antiferroelectric coherence in Bi0.5Na0.5TiO3-SrTiO3-(K0.5Na0.5)NbO3 solid solutions. J. Appl. Phys. 116:184104. doi: 10.1063/1.4901549
Long, C., Li, T., Fan, H., Wu, Y., Zhou, L., Li, Y., et al. (2016). Li-substituted K0.5Na0.5NbO3-based piezoelectric ceramics: crystal structures and the effect of atmosphere on electrical properties. J. Alloy. Compd. 658, 839–847. doi: 10.1016/j.jallcom.2015.10.245
Lv, X., Wu, J., Zhao, C., Xiao, D., Zhu, J., Zhang, Z., et al. (2019). Enhancing temperature stability in potassium-sodium niobate ceramics through phase boundary and composition design. J. Eur. Ceram. Soc. 39, 305–315. doi: 10.1016/j.jeurceramsoc.2018.08.039
Mazhao, D., Xiao, D., Wu, J., and Zhu, J. (2015). Phase structure and electrical properties of 0.965(K0.45Na0.55)0.95Ag0.05(Nb1−xSbx)O3- 0.035Bi0.5(Na0.5Li0.5)0.5ZrO3 lead-free ceramics. J. Mater. Sci-Mater. El. 26, 7309–7315. doi: 10.1007/s10854-015-3359-7
Saito, Y., and Takao, H. (2006). High performance lead-free piezoelectric ceramics in the (K,Na)NbO3-LiTaO3 solid solution system. Ferroelectrics 338, 17–32. doi: 10.1080/00150190600732512
Shi, D., Liu, L., Huang, Y., Fang, L., and Hu, C. (2014). Structure and electrical properties of LiF doped 0.996(0.95K0.5Na0.5NbO3-0.05LiSbO3)-0.004BiFeO3 piezoelectric ceramics. Ferroelectrics 467, 99–109. doi: 10.1080/00150193.2014.932543
Song, H. C., Cho, K. H., Park, H. Y., Ahn, C. W., Nahm, S., Uchino, K., et al. (2007). Microstructure and piezoelectric properties of (1–x) (Na0.5K0.5)NbO3-xLiNbO3 ceramics. J. Am. Ceram. Soc. 90, 1812–1816. doi: 10.1111/j.1551-2916.2007.01698.x
Sun, X., Deng, J., Liu, L., Liu, S., Shi, D., Fang, L., et al. (2016). Dielectric properties of BiAlO3-modified (Na, K, Li) NbO3 lead-free ceramics. Mater. Res. Bull. 73, 437–445. doi: 10.1016/j.materresbull.2015.10.005
Wang, K., and Li, J. F. (2007). Analysis of crystallographic evolution in (Na,K)NbO3-based lead-free piezoceramics by x-ray diffraction. Appl. Phys. Lett. 91:262902. doi: 10.1063/1.2825280
Wang, K., and Li, J. F. (2010a). Low-temperature sintering of Li-modified (K, Na) NbO3 lead-free ceramics: sintering behavior, microstructure, and electrical properties. J. Am. Ceram. Soc. 93, 1101–1107. doi: 10.1111/j.1551-2916.2009.03532.x
Wang, K., and Li, J. F. (2010b). Domain engineering of lead-free Li-modified (K,Na)NbO3 polycrystals with highly enhanced piezoelectricity. Adv. Funct. Mater. 20, 1924–1929. doi: 10.1002/adfm.201000284
Wang, K., Yao, F. Z., Jo, W., Gobeljic, D., Shvartsman, V. V., Lupascu, D. C., et al. (2013). Temperature-insensitive (K, Na)NbO3-based lead-free piezoactuator ceramics. Adv. Funct. Mater. 23, 4079–4086. doi: 10.1002/adfm.201203754
Wu, B., Wu, H., Wu, J., Xiao, D., Zhu, J., and Pennycook, S. J. (2016). Giant piezoelectricity and high Curie temperature in nanostructured alkali niobate lead-free piezoceramics through phase coexistence. J. Am. Ceram. Soc. 138, 15459–15464. doi: 10.1021/jacs.6b09024
Xiao, H., Dong, W., Guo, Y., Wang, Y., Zhong, H., Li, Q., et al. (2019). Design for highly piezoelectric and visible/near-infrared photoresponsive perovskite oxides. Adv. Mater. 31:1805802. doi: 10.1002/adma.201805802
Xu, K., Li, J., Lv, X., Wu, J., Zhang, X., Xiao, D., et al. (2016). Superior piezoelectric properties in potassium–sodium niobate lead-free ceramics. Adv. Mater. 28, 8519–8523. doi: 10.1002/adma.201601859
Yan, H., Zhang, Z., Zhu, W., He, L., Yu, Y., Li, C., et al. (2004). The effect of (Li, Ce) and (K, Ce) doping in Aurivillius phase material CaBi4Ti4O15. Mater. Res. Bull. 39, 1237–1246. doi: 10.1016/j.materresbull.2004.04.020
Yan, T., Han, F., Ren, S., Deng, J., Ma, X., Ren, L., et al. (2018c). Enhanced temperature-stable dielectric properties in oxygen annealed 0.85(K0.5Na0.5) NbO3-0.15 SrZrO3 ceramic. Mater. Res. Bull. 99, 403–408. doi: 10.1016/j.materresbull.2017.11.040
Yan, T., Han, F., Ren, S., Ma, X., Fang, L., Liu, L., et al. (2018a). Dielectric properties of (K0.5Na0.5)NbO3-(Bi0.5Li0.5)ZrO3 lead-free ceramics as high-temperature ceramic capacitors. Appl. Phys. A 124:338. doi: 10.1007/s00339-018-1757-4
Yan, T., Ren, S., Ma, X., Han, F., Fang, L., Peng, B., et al. (2018b). Dielectric properties of (Bi0.5K0.5)ZrO3 modified (K0.5Na0.5)NbO3 ceramics as high-temperature ceramic capacitors. J. Electron. Mater. 47, 7106–7113. doi: 10.1007/s11664-018-6641-7
Yan, T., Sun, X., Deng, J., Liu, S., Han, F., Liu, X., et al. (2017). Dielectric and conductivity behavior of Mn-doped K0.5Na0.5NbO3 single crystal. Solid State Commun. 264, 1–5. doi: 10.1016/j.ssc.2017.07.009
Zhang, S., Xia, R., Shrout, T. R., Zang, G., and Wang, J. (2006). Piezoelectric properties in perovskite 0.948(K0.5Na0.5)NbO3-0.052LiSbO3 lead-free ceramics. J. Appl. Phys. 100:104108. doi: 10.1063/1.2382348
Zhao, P., Zhang, B. P., and Li, J. F. (2007). Enhancing piezoelectric d33 coefficient in Li/Ta-codoped lead-free (Na,K)NbO3 ceramics by compensating Na and K at a fixed ratio. Appl. Phys. Lett. 91:172901. doi: 10.1063/1.2794405
Zhao, Y., Liu, J., and Yan, D. (2019). Improved piezoelectric and strain performance of Na2B4O7-doped (Li, K, Na) NbO3 lead-free piezoceramics. J. Mater. Sci. 54, 1126–1135. doi: 10.1007/s10853-018-2906-z
Zheng, T., Wu, J., Xiao, D., and Zhu, J. (2018). Recent development in lead-free perovskite piezoelectric bulk materials. Prog. Mater. Sci. 98, 552–624. doi: 10.1016/j.pmatsci.2018.06.002
Zhong, H., Xiao, H., Jiao, N., and Guo, Y. (2019). Boosting piezoelectric response of KNN-based ceramics with strong visible-light absorption. J. Am. Ceram. Soc. 102, 6422–6426. doi: 10.1111/jace.16618
Keywords: piezoelectrics, dielectric temperature stability, morphotropic phase boundary (MPB), lead-free, perovskite, ceramics, oxides
Citation: Ji H, Xin L, Ma H, Liu W, Dai Z, Pang L, Xie J and Chen Z (2020) Simultaneously Enhancing Thermal Stable Dielectric Property and Piezoelectric Response in Lead-Free LiNbO3-Modified (K0.5Na0.5)NbO3- (BaNi0.5Nb0.5O3) System. Front. Mater. 7:13. doi: 10.3389/fmats.2020.00013
Received: 27 October 2019; Accepted: 14 January 2020;
Published: 11 February 2020.
Edited by:
Rajesh Adhikari, Institut National de la Recherche Scientifique (INRS), CanadaReviewed by:
Jigong Hao, Liaocheng University, ChinaLaijun Liu, Guilin University of Technology, China
Copyright © 2020 Ji, Xin, Ma, Liu, Dai, Pang, Xie and Chen. This is an open-access article distributed under the terms of the Creative Commons Attribution License (CC BY). The use, distribution or reproduction in other forums is permitted, provided the original author(s) and the copyright owner(s) are credited and that the original publication in this journal is cited, in accordance with accepted academic practice. No use, distribution or reproduction is permitted which does not comply with these terms.
*Correspondence: Hongfen Ji, jihongfen@126.com; Lipeng Xin, ybsyh3@163.com