- State Key Laboratory of Chemical Resource Engineering, Beijing University of Chemical Technology, Beijing, China
Zn–air batteries (ZABs) have drawn extensive attention for portable and wearable electronic devices owing to their high theoretical specific energy density and low cost. However, due to the dense packing under high mass loading, the mass/charge diffusion is seriously hindered, making their practical performance with high power density, and energy density difficult to sustain. Herein, we reported an efficient bi-functional electrocatalyst of Ni-Co mixed metal oxides incorporated with cobalt/nitrogen-doped carbon with hierarchical hollow nanostructure (H-Co/N-C@NiCo2O4). Benefiting from the advantages of this structure and composition, the H-Co/N-C@NiCo2O4 exhibits superior electrocatalytic activity and long-time durability for both oxygen reduction reaction (ORR) and oxygen evolution reaction (OER). The potential gap between half-wave potential in ORR and overpotential at a current density of 10 mA cm−2 in OER reaches 0.8 V, much smaller than that of most reported bi-functional electrocatalysts. Moreover, H-Co/N-C@NiCo2O4 is constructed as an air electrode for rechargeable ZAB, delivering a high power density and long cycling stability. The good linear relationship between the power density and various mass loading of H-Co/N-C@NiCo2O4 on the electrode demonstrates that the performance has been well-maintained even under high dense packing of catalysts, which offers a new pathway for the practical applications in ZAB.
Introduction
Zinc–air batteries (ZABs) have been investigated tremendously in past decades owing to their promising theoretical specific energy density of 1,086 Wh kg−1 and low cost, which would meet the ever-increasing energy requirements for renewable energy conversion devices (Lee et al., 2011; Li and Dai, 2014; Chen et al., 2018; Meng et al., 2018; Wang H. F. et al., 2018; Wang T. et al., 2018). Despite these advantages, the sluggish kinetics of oxygen reduction reaction (ORR) and oxygen evolution reaction (OER) during discharging and charging at the air-cathode always result in limited energy and power density of the devices in practical applications (Prabu et al., 2014; Park et al., 2017; Jiang H. et al., 2018; Liu et al., 2019; Qiu et al., 2019). To solve the above problems, a large number of electrocatalysts have been developed for high performance of ORR or OER, such as noble metals (Wu et al., 2010; Audichon et al., 2016), transition metals/metal oxides (Fu et al., 2018; Li T. et al., 2018), and metal-free materials (Ferrero et al., 2016; Hang et al., 2018). However, these catalytically active materials generally face issues regarding activities for ORR and OER simultaneously and stabilities (Guo Z. et al., 2018). Thus, exploring highly efficient, stable bi-functional oxygen electrocatalysts is still an urgent task for the ZABs.
Transition metal composites [e.g., oxides (Guan C. et al., 2017; Li Y. et al., 2018; Peng et al., 2018, sulfides (Zhou et al., 2013, 2017b; Ganesan et al., 2015; Zhang et al., 2016), and phosphides (Zhou et al., 2017a; Wu et al., 2018) have been highlighted as attractive candidates for OER electrocatalysts, but they fail to catalyze the ORR process. Recently, heteroatom-doped carbon materials expressed excellent electrocatalytic activity for ORR, where Co-N-C has been regarded as one of the best candidates for ORR and Zn–air batteries (Zhao et al., 2012; Shang et al., 2016; Amiinu et al., 2018; Guo Y. et al., 2018; Jiang R. et al., 2018; Wang T. et al., 2018; Wang Z. et al., 2018; Liang et al., 2019). Being inspired by this, assembling transition metal oxides with heteroatom-doped carbon materials is an effective approach to obtain bi-functional electrocatalysts for both OER and ORR (Guo Z. et al., 2018). However, in practice, the performance of electrocatalysts under high mass loading is difficult to maintain due to the agglomeration of particles that severely inhibits mass/charge diffusion process, which has so far been few considered (Xie et al., 2019). Therefore, it is desirable to construct advanced electrodes with more sophisticated functionalities, so as to boost mass transfer process and more exposed active sites under high catalyst loading.
Herein, we demonstrate a bi-functional electrocatalyst with well-ordered hierarchical hollow nanostructure, which shows high performances for both the ORR and OER even under high mass loading. The bi-functional hollow structured electrocatalyst consisting of cobalt/nitrogen-doped carbon embedded with Ni-Co mixed metal oxides (H-Co/N-C@NiCo2O4) is prepared via a facile self-template approach. Benefiting from the advantages of this structure and composition, the as-synthesized H-Co/N-C@NiCo2O4 exhibits impressive electrocatalytic activity and long-time durability for both ORR and OER. In addition, the advanced H-Co/N-C@NiCo2O4 is constructed as an air cathode for rechargeable ZAB, which delivers a high power density and long cycling stability. The good linear relationship between the power density and various mass loading of H-Co/N-C@NiCo2O4 on the electrode demonstrates that the performance has been well-maintained even under high dense packing of catalysts, which offers a new pathway for the practical applications in ZAB.
Experimental Section
Synthesis of PS@ZIF-67 Microsphere Template
Firstly, the polystyrene (PS) microsphere was synthesized using a method of dispersion polymerization (Lee et al., 2006). Then, the synthesized PS (0.25 g) and Co(NO3)2·6H2O (2.25 g) were dispersed in methanol (150 ml). Then, 150 ml of methanol solution containing 6.225 g of 2-methylimidazole was quickly added into the former dispersion. After stirring at room temperature for 1 h, the products were washed with methanol and dried overnight.
Synthesis of PS@ZIF-67@NiCo-LDH and PS@NiCo-LDH
Firstly, 0.2 g of PS@ZIF-67 was dispersed in 100 ml of ethanol. Then, 25 ml of ethanol solution containing 0.45 g of Ni(NO3)2·6H2O was quickly poured into the former dispersion. After stirring at room temperature for 0.5 h, the products were washed with ethanol and dried overnight. The products were denoted as PS@ZIF-67@NiCo-LDH. Besides, PS@NiCo-LDH was also synthesized by using the above procedures but stirring for 4 h.
Synthesis of H-Co/N-C, H-Co/N-C@NiCo2O4, and H-NiCo2O4
The as-prepared PS@ZIF-67, PS@ZIF-67@NiCo-LDH, and PS@NiCo-LDH were pyrolyzed in N2 at 600°C for 2 h. The products were denoted as H-Co/N-C, H-Co/N-C@NiCo2O4, and H-NiCo2O4, respectively.
Characterization
X-ray diffraction patterns were obtained on a Shimadzu XRD-6000 diffractometer with Cu Kα radiation. Scanning electron microscope (SEM) was obtained on Zeiss Supra 55. Elemental mapping and EDS were recorded using energy-dispersive X-ray spectroscopy (EDS) attached to the SEM. High-resolution transmission electron microscope (HRTEM) was performed on JEOL JEM-2010. X-ray photoelectron spectra (XPS) were collected using a Thermo VG ESCALAB 250. Raman spectra were carried out by HORIBA Jobin Yvon Raman microspectrometer. N2 adsorption/desorption isotherms were investigated using an Micromeritics ASAP 2020 instrument at 77 K.
Electrochemical Measurements
All electrochemical tests were carried out on an electrochemical workstation (CHI 760E, Shanghai Chenhua, China). A glassy carbon (GC) or carbon cloth with catalysts, Pt wire, and Ag/AgCl electrode were used as working electrode, counter electrode, and reference electrode, respectively. The catalyst inks were prepared as follows: 5.89 mg catalyst was ultrasonically dispersed in 770 μl of deionized water, 200 μl of ethanol, and 30 μl of Nafion solution (5 wt%). For the ORR tests, the as-prepared catalyst ink was pipetted onto the rotating disk electrode (RDE, 0.196 cm2) and ring rotating disk electrode (RRDE, carbon disk with a surface area of 0.126 cm2 surrounded by a Pt ring with a surface area of 0.224 cm2) with a loading of 0.3 mg cm−2, and dried under room temperature. Cyclic voltammetry (CV) was tested in O2- and N2-saturated 0.1 M KOH at a scan rate of 100 mV s−1. The following tests were obtained in O2-saturated 0.1 M KOH. RDE tests were carried out at various rotation rates from 400 to 2,025 rpm with a scan rate of 5 mV s−1. RRDE measurements were also conducted in O2-saturated 0.1 M KOH at room temperature. The disk potential was scanned from −1 to 0.2 V at a scan rate of 5 mV s−1 and the ring potential was constant at 0.5 V. The stability tests were measured by i–t amperometric response at 0.16 V vs. RHE. RRDE tests, electrochemical impedance spectra (EIS), and i–t amperometic response were all measured at a rotation rate of 1,600 rpm. The electrical double-layer capacitance (Cdl) of the catalysts was performed using CV at different scan rates from 20 to 120 mV s−1 in non-Faradaic region. For the OER tests, the catalyst ink was pipetted onto carbon cloth with a loading of 1 mg cm−2, and dried under room temperature. Linear sweep voltammetry (LSV) was measured in 1 M KOH at a scan rate of 5 mV s−1 under stirring (90% iR-compensation).
The potential vs. reversible hydrogen electrode (RHE) was calculated via the Nernst equation:
The overpotential (η) was calculated using the following formula:
The electron transfer number (n) was calculated according the Koutecky–Levich equation:
where j, jL, and jK are the measured current density, diffusion-limiting current density, and kinetic current density, respectively; ω is the rate of electrode rotation speed; F is the Faraday constant (96,485 C mol−1); C0 is the bulk concentration of O2 (1.26 × 10−6 mol cm−3); D0 is the diffusion coefficient of O2 in 0.1 M KOH (1.9 × 10−5 cm2 s−1); v is the kinematic viscosity of electrolyte (0.01 cm2 s−1).
For the RRDE measurements, the percentage of hydrogen peroxide yield and the electron transfer number (n) were calculated using the following formula:
where Id and Irare the disk and ring currents, respectively; N is the ring collection efficiency determined to be 0.37.
The difference in current density (Δj = ja – jc) at 0.46 V vs. RHE was plotted against scan rate and fitted to a linear regression for the estimation of double layer capacitances (Cdl).
The Tafel slope was calculated by the following formula:
where η is the overpotential; b is the Tafel slope; j is the current density.
Zn–Air Battery Tests
All Zn–air battery tests were carried out on an electrochemical workstation (CHI 660E, Shanghai Chenhua, China). A carbon cloth loaded with the catalyst inks (1 mg cm−2) served as the air cathode, and a polished Zn foil served as the anode. For the rechargeable Zn–air batteries, 6 M KOH and 0.2 M Zn(CH3COO)2 were chosen as the electrolyte. The polarization curves were measured at a scan rate of 5 mV s−1. The galvanostatic charge and discharge cycling curves were measured at a current density of 5 mA cm−2 (discharge 100 s and charge 100 s). Specifically, discharging polarization curves at a current density of 10 mA cm−2 were measured in 6 M KOH.
The power density, specific capacity, and energy density were calculated by the following formula:
Results and Discussion
Material Characterizations
The synthesis process of hollow Co/N-C@NiCo2O4 microspheres (H-Co/N-C@NiCo2O4) is illustrated in Scheme 1 (Step 2, see the Experimental Section for details). First, PS microspheres with an average size of 2.7 μm were synthesized via a dispersion polymerization process (Figure 1a). Second, zeolitic imidazolate framework (ZIF-67) nanoparticles were compactly assembled on the surface of PS to obtain PS@ZIF-67 microspheres (Figures 1b, 2A). The growth mechanism of ZIF-67 on the surface of PS microsphere is supposed to be the nucleation growth mechanism (Guan B. Y. et al., 2017). Typically, Co ions first coordinate with the carboxylate on the surface of PS microsphere. Then, 2-methylimidazole connects with the Co ions and leads to the in situ nucleation process and the growth of ZIF-67 nanoparticles. Third, the obtained PS@ZIF-67 microspheres were in situ converted into PS@ZIF-67@NiCo-LDH microspheres by reacting with Ni(NO3)2 in ethanol solution for 30 min (Figure 1c). After that, the ZIF-67 nanoparticles with a dodecahedral shape partly dissolved and lots of nanosheets were aligned on the surface of microspheres, which derived a unique architecture of honeycomb with yolk-shelled structure. Last, a thermal treatment was performed to obtain hollow and hierarchical Co/N-C@NiCo2O4 microspheres (Figure 1d). After thermal treatment, the structure of microspheres is maintained while the average size of microspheres is decreased from 3.1 to 2.8 μm due to the contraction of ZIF-67. TEM image reveals that hollow cavities are formed inside H-Co/N-C@NiCo2O4 microspheres as a result of volatilization of PS (Figure 1e), which would facilitate the electrolyte penetration. High-resolution TEM image shows that lots of nanoparticles with an interlayer spacing of 4.7 and 2.9 Å dispersed on the surface of microsphere, corresponding to the (111) and (220) plane of NiCo2O4 (Zhang and Lou, 2013), which are derived from NiCo-LDH (Figure 1f). Other nanoparticles embedded in graphite-like layers with an interlayer spacing of 2.1 Å correspond to the (111) plane of Co metal (Aijaz et al., 2016). Moreover, the EDS elemental mapping of H-Co/N-C@NiCo2O4 displays a well-uniformed distribution of C, N, O, Co, and Ni, confirming the successful introduction of Ni during the etching process (Figure 1g and Figure S5). During the etching process, ZIF-67 could be completely etched away when the etching time is prolonged to 4 h and the product noted is as PS@NiCo-LDH [Scheme 1 (Step 3), Figures S1a, S2]. After thermal treatment, PS@NiCo-LDH is in situ transformed into hollow NiCo2O4 microspheres (denoted as H-NiCo2O4) (Figures S1b,c). In addition, hollow Co, N-doped carbon microspheres (denoted as H-Co/N-C) without NiCo2O4 obtained by direct pyrolysis of PS@ZIF-67 was also synthesized as reference [Scheme 1 (Step 1); Figures S3, S4].
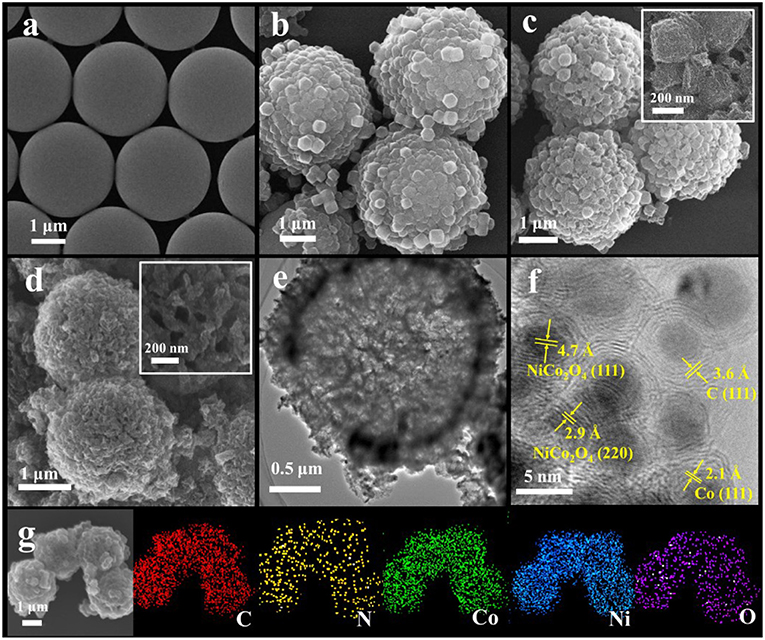
Figure 1. SEM images of (a) PS microspheres, (b) PS@ZIF-67 microspheres, (c) PS@ZIF-67@NiCo-LDH microspheres, and (d) H-Co/N-C@NiCo2O4. (e) TEM and (f) HRTEM image of H-Co/N-C@NiCo2O4. (g) SEM and EDS elemental mapping images of H-Co/N-C@NiCo2O4.
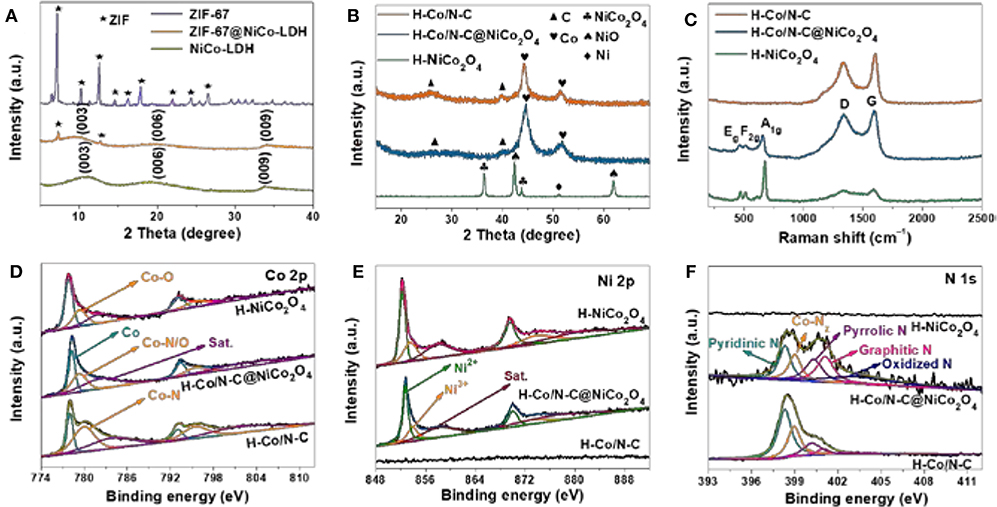
Figure 2. (A) XRD patterns of PS@ZIF-67, PS@ZIF-67@NiCo-LDH, and PS@NiCo-LDH. (B) XRD patterns, (C) Raman spectra, high-resolution XPS spectra of (D) Co 2p, (E) Ni 2p, and (F) N 1s for H-Co/N-C, H-Co/N-C@NiCo2O4 and H-NiCo2O4.
XRD analysis of ZIF-67@NiCo-LDH indicates that ZIF-67 is successfully transformed into NiCo-LDH, from which the peaks of ZIF-67 are transformed into three peaks at ~11°, 20°, and 34°, attributed to the (003), (006), and (009) planes of LDH (Li et al., 2015; Kaneti et al., 2017) (Figure 2A). After thermal treatment, the diffraction patterns of H-Co/N-C@NiCo2O4 and H-Co/N-C show that two well-defined diffraction peaks that emerged at 44.3° and 51.7° correspond to the (111) and (200) planes of face-centered Co (JCPDS15-0806), and the peaks at 26.1° and 44.6° are indexed to the (002) and (111) planes of graphitic carbon (Li J. et al., 2018). The diffraction pattern of H-NiCo2O4 implies the presence of NiCo2O4 (PDF#73-1702), NiO (PDF#89-7390), and Ni (PDF#70-0989). It should be mentioned that the diffraction peak of NiCo2O4 is not observed in H-Co/N-C@NiCo2O4 due to the high peak intensity of Co (Figure 2B). The graphitic feature and defects of synthesized materials were investigated by Raman spectra (Figure 2C). The intensity ratio of the D band (1340 cm−1) to the G band (1590 cm−1) (ID/IG) for H-Co/N-C@NiCo2O4 (0.95) is higher than H-Co/N-C (0.83), indicating that H-Co/N-C@NiCo2O4 contains more defective carbon, which is beneficial to the improvement of electrocatalytic activity (Zhang et al., 2011; Chen et al., 2017; Yang et al., 2017; Asset et al., 2018). Besides, there are three peaks at 463 cm−1 (Eg mode), 507 cm−1 (F2g mode), and 663 cm−1 (A1g mode) in H-Co/N-C@NiCo2O4 and H-NiCo2O4, corresponding to Co-O and Ni-O molecular vibration peaks of NiCo2O4 (Umeshbabu et al., 2015).
The X-ray photoelectron spectroscopy (XPS) spectra confirm the presence of C, N, O, Co, and Ni in H-Co/N-C@NiCo2O4 (Figure S6 and Table S1). The Co 2p spectrum demonstrates the presence of both zero valence state (777.8 and 793.1 eV) and oxidation state (779.3 and 795.6 eV) Co species, which can be assigned to the metallic Co and Co-N/Oχ coordination, respectively (Ni et al., 2017) (Figure 2D). For the Ni 2p spectrum of H-Co/N-C@NiCo2O4 and H-NiCo2O4, the peaks located at 852.2/869.5 and 853.7/873.9 eV correspond to Ni3+ and Ni2+, respectively (Cui et al., 2009) (Figure 2E). The N 1s peak of H-Co/N-C@NiCo2O4 is deconvoluted into five types of N species, namely, pyridinic (398.5 eV), Co-Nχ (399.6 eV), pyrrolic (400.5 eV), graphitic (401.0 eV), and oxidized (403.7 eV) N species (Jia et al., 2017) (Figure 2F). There is no N 1s peak in H-NiCo2O4, indicating that ZIF-67 is completely removed by etching. The surface area and pore size distribution of H-Co/N-C, H-Co/N-C@NiCo2O4, and H-NiCo2O4 are investigated by N2-adsorption/desorption isotherms (Figure S7). The type IV isotherms with H3-type hysteresis loops are observed for all samples, indicating the existence of mesopores (Li et al., 2016; Zhan and Zeng, 2017). Although the specific surface area of H-Co/N-C@NiCo2O4 is the smallest, it exhibits the best electrocatalytic performance in subsequent electrochemical tests, indicating that the synergy between Co/N-C and NiCo2O4 is beneficial to the improvement of electrochemical properties (Table S2).
Oxygen Electrocatalysis Performance
The electrocatalytic activity of H-Co/N-C@NiCo2O4 toward ORR is assessed with a conventional three-electrode cell. To better demonstrate the superior activity of H-Co/N-C@NiCo2O4, the reference samples of H-Co/N-C, H-NiCo2O4, and commercial Pt/C were also evaluated and compared comprehensively. The raised cathodic peak at ~0.75 V vs. RHE in an O2-saturated electrolyte compared to that in an N2-saturated electrolyte indicates effective ORR electrocatalytic activity of synthesized materials (Figure S8). LSV curves (Figure 3A) display more positive onset-potential (0.913 V vs. RHE), half-wave potential (0.796 V vs. RHE), and larger diffusion-limited current density (−4.96 mA cm−2) of H-Co/N-C@NiCo2O4 than that of H-Co/N-C, H-NiCo2O4, and commercial Pt/C (Table S3). The rotating disk electrode (RDE) was carried out to calculate the reaction kinetic parameters via Koutecky–Levich (K–L) equation (Figure 3B and Figure S9). The calculated electron transfer number of H-Co/N-C@NiCo2O4 is 3.99, implying a direct four-electron process for ORR. Rotating ring disk electrode (RRDE) is operated to further quantify the ORR pathway of H-Co/N-C@NiCo2O4 (Figure S10). The results indicate that the yield of H-Co/N-C@NiCo2O4 is the smallest among all synthesized materials. Besides, the average electron transfer number of H-Co/N-C@NiCo2O4 is 3.61, larger than that of H-Co/N-C (3.51) and H-NiCo2O4 (2.40), which conform to the result obtained from K–L plots.
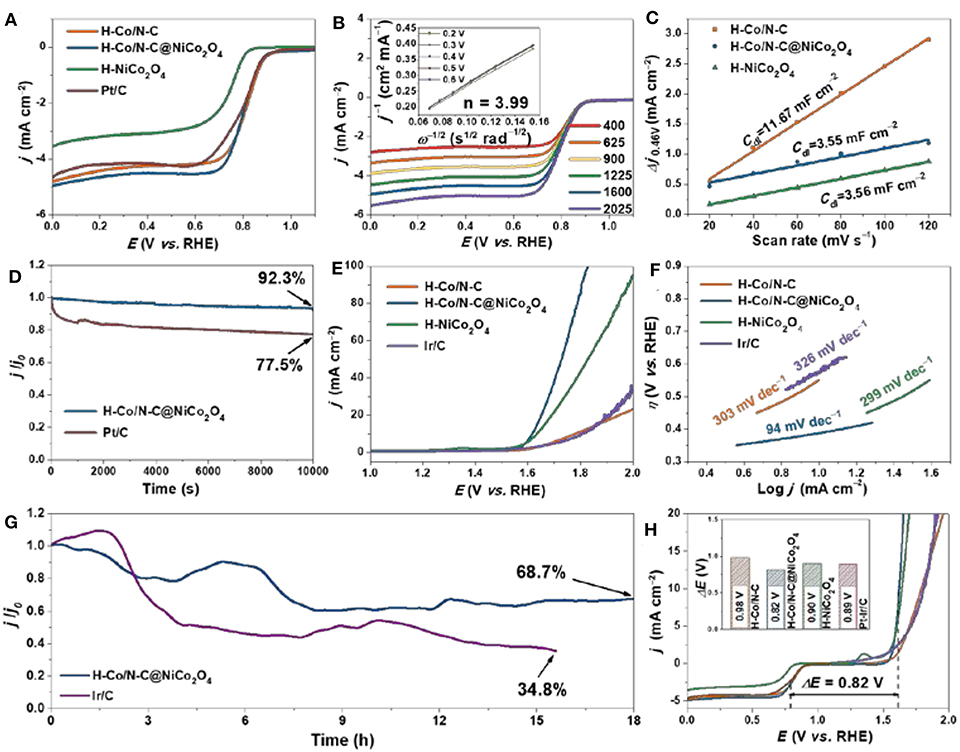
Figure 3. (A) LSV curves of H-Co/N-C, H-Co/N-C@NiCo2O4, H-NiCo2O4, and Pt/C for ORR. (B) LSV curves for H-Co/N-C@NiCo2O4 at various rotation rates. The inset is the corresponding Kouteck–Levich plots for H-Co/N-C@NiCo2O4 at various potentials calculated from RDE data. (C) Cdl of H-Co/N-C, H-Co/N-C@NiCo2O4, and H-NiCo2O4 calculated from current density difference (Δj = ja – jc) plotted vs. scan rate. (D) Amperometric i–t curves of H-Co/N-C@NiCo2O4 and Pt/C for ORR. (E) LSV curves and (F) Tafel slopes of H-Co/N-C, H-Co/N-C@NiCo2O4, H-NiCo2O4, and Ir/C for OER. (G) Amperometric i–t curves of H-Co/N-C@NiCo2O4 and Ir/C for OER. (H) Overall LSV curves of the ORR and OER for H-Co/N-C, H-Co/N-C@NiCo2O4, H-NiCo2O4, Pt/C, and Ir/C. The inset is ΔE for H-Co/N-C, H-Co/N-C@NiCo2O4, H-NiCo2O4, and Pt-Ir/C.
The electrochemical double-layer capacitance (Cdl) was deliberately measured to represent the relative electrochemical surface area (ECSA), which is linearly proportional to the number of active sites (Qiu et al., 2019) (Figure S11). The results in Figure 3C suggest that H-Co/N-C@NiCo2O4 exhibits the smallest Cdl value (3.55 mF cm−2) compared with H-Co/N-C (11.67 mF cm−2) and H-NiCo2O4 (3.56 mF cm−2). This indicates that the largely promoted ORR activity is due to the synergetic effect of Co/N-doped carbon and NiCo2O4. Besides the activity, stability is another important parameter for the practical applications of high-performance ORR electrocatalysts. The H-Co/N-C@NiCo2O4 shows an excellent durability with 92.3% retention after a continuous operation of 10,000 s, much more stable than that of commercial Pt/C catalysts (with only 77.5% retention) (Figure 3D), demonstrating a superb long-term stability of H-Co/N-C@NiCo2O4 toward ORR.
Furthermore, the OER performances are also investigated in KOH solution with 90% iR-compensation (Figure 3E). The H-Co/N-C@NiCo2O4 displays a relatively low onset potential with an overpotential of 386 mV to achieve the current density of 10 mA cm−2, far superior to H-Co/N-C (552 mV), H-NiCo2O4 (405 mV), and commercial Ir/C (567 mV). Besides, H-Co/N-C@NiCo2O4 presents a Tafel slope of 94 mV dec−1, much smaller than that of H-Co/N-C (303 mV dec−1), H-NiCo2O4 (299 mV dec−1), and commercial Ir/C (326 mV dec−1), and reveals much faster reaction kinetics for OER (Figure 3F). Furthermore, the stability of H-Co/N-C@NiCo2O4 for OER was investigated (Figure 3G). Although performance has declined to some extent, which is the result of the dissolution of materials from carbon cloth due to the bubble formation, the H-Co/N-C@NiCo2O4 still retains 68.7% of the initial performance after continuous operation of 18 h, better than that of Ir/C catalysts (with only 34.8% retention after 15 h), demonstrating the promising potential to replace noble metal catalysts for both ORR and OER. Additionally, the robust stability of H-Co/N-C@NiCo2O4 is verified by TEM, HRTEM, and XPS. As can be seen from Figure S12, the structure and composition retain their original state after all electrochemical tests, demonstrating the superior stability of H-Co/N-C@NiCo2O4. XPS measurement of H-Co/N-C@NiCo2O4 after all electrochemical tests exhibits some predictable changes (Figure S13). Namely, the valence state of Co and Ni has increased after all electrochemical tests, which is an unavoidable result after working at a high potential (Cao et al., 2018; Gu et al., 2018).
The overall oxygen electrode activity for both ORR and OER is evaluated by the difference between the overpotential measured at a current density of 10 mA cm−2 for OER and half-wave potential for ORR (ΔE = E j10 – E1/2). Remarkably, the H-Co/N-C@NiCo2O4 displays a ΔE of 0.82 V, much lower than that of H-Co/N-C (0.98 V), H-NiCo2O4(0.90 V), commercial Pt-Ir/C (0.89 V) (Figure 3H), and most reported bi-functional electrocatalysts (Table S4). The electrochemical performance of H-Co/N-C@NiCo2O4 and the control samples is further investigated by electrochemical impedance spectroscopy measurements (Figure S14). From the Nyquist plots, the charge transfer resistance (Rct = ~9.8 Ω) of H-Co/N-C@NiCo2O4 and H-Co/N-C is nearly the same (Rct = ~9.8 Ω) at high frequency due to the excellent conductivity of N-doped carbon. However, the pure H-NiCo2O4 shows relative sluggish electron transfer ability (Rct = ~12.2 Ω) because of the limited conductivity. Moreover, the straight line at low frequency shows significantly increased slope for H-Co/N-C@NiCo2O4 than H-Co/N-C, indicating the faster mass diffusion behavior benefiting from this unique hierarchical structure. Although the mass diffusion ability is not as good as that of H-NiCo2O4, the better ORR performance of H-Co/N-C@NiCo2O4 indicates that the balance between mass diffusion and charge transfer is crucial for the performance of electrocatalysts.
The excellent ORR and OER performance of H-Co/N-C@NiCo2O4 benefits from the advantages of promoted surface reaction, enhanced charge transfer property, and improved mass diffusion process. As shown in Figure S15, the LSV plots of H-Co/N-C@NiCo2O4, H-Co/N-C, and H-NiCo2O4 for ORR were normalized by their ECSA. The current density of H-Co/N-C@NiCo2O4 (−0.274 mA cm2 mF−1) is much higher than that of H-Co/N-C (−0.081 mA cm2 mF−1) and H-NiCo2O4 (−0.195 mA cm2 mF−1), indicating high intrinsic activity for ORR owing to the synergistic effect between the compositions (Figure 3C and Figure S7). Second, the combination of H-Co/N-C and NiCo2O4 renders fast electron transfer between active sites and current collector, which can be verified by the lower resistance of ~9.8 Ω (Figure S14). Last but not least, the unique hierarchical hollow structure also ensures an efficient mass diffusion process, which accelerates the transport and adsorption of reactants to active sites and thus leads to a high reaction kinetics (Tafel slope of 94 mV dec−1), better than that of H-Co/N-C (303 mV dec−1) and H-NiCo2O4 (299 mV dec−1) (Figure 3F and Figure S14).
Encouraged by the superior electrochemical activity under basic medium, the ORR performance of H-Co/N-C@NiCo2O4 was also investigated in acidic medium (Figure S16). The H-Co/N-C@NiCo2O4 delivers acceptable ORR performance with a diffusion-limited current density of −3.06 mA cm−2, better than that of H-NiCo2O4 (−2.47 mA cm−2) and H-Co/N-C (−2.95 mA cm−2). However, due to the instability behavior of NiCo2O4 under acidic conditions, this performance cannot be well-maintained for a long lifespan.
Zn–Air Battery Performance
Motivated by the superior electrocatalytic activity for both ORR and OER, the feasibility of H-Co/N-C@NiCo2O4 as the air cathode in Zn–air battery was further studied. The open-circuit voltage of Zn–air battery based on H-Co/N-C@NiCo2O4 is 1.46 V (Figure 4A), comparable to that of the Zn–air battery based on commercial Pt-Ir/C (1.47 V). The discharge polarization curve of the Zn–air battery based on H-Co/N-C@NiCo2O4 reveals a high peak power density of 34.1 mW cm−2, which is superior to that of the Zn–air battery based on commercial Pt-Ir/C (26.6 mW cm−2) (Figure 4B). Moreover, the Zn–air battery based on H-Co/N-C@NiCo2O4 delivers high specific capacity (713.9 mAh g−1) and energy density (763.8 Wh kg−1) at a current density of 10 mA cm−2, respectively, close to that of Pt-Ir/C (819.7 mAh g−1 and 802.5 Wh kg−1) (Figures 4C,D). A light-emitting diode could be effectively powered by two Zn–air batteries in series (Figure 4E). The discharge curves of Zn–air battery based on H-Co/N-C@NiCo2O4 and commercial Pt-Ir/C are conducted at various current densities from 2 to 20 mA cm−2. Compared with the commercial Pt-Ir/C electrode, the H-Co/N-C@NiCo2O4 electrode shows higher discharge voltages and relatively stable discharge performance, indicating the better ORR activity, and reversible capacity (Figure 4F). Moreover, the H-Co/N-C@NiCo2O4 electrode shows excellent stability as there is only a small change in charge and discharge voltages (voltage gap increases by 4%) over 90 h in the long-term cycling tests, while the commercial Pt-Ir/C electrode shows a large change (voltage gap increases by 30%) after 36 h, which indicates the excellent stability and rechargeability of the Zn–air battery based on H-Co/N-C@NiCo2O4 (Figures 4G,H).
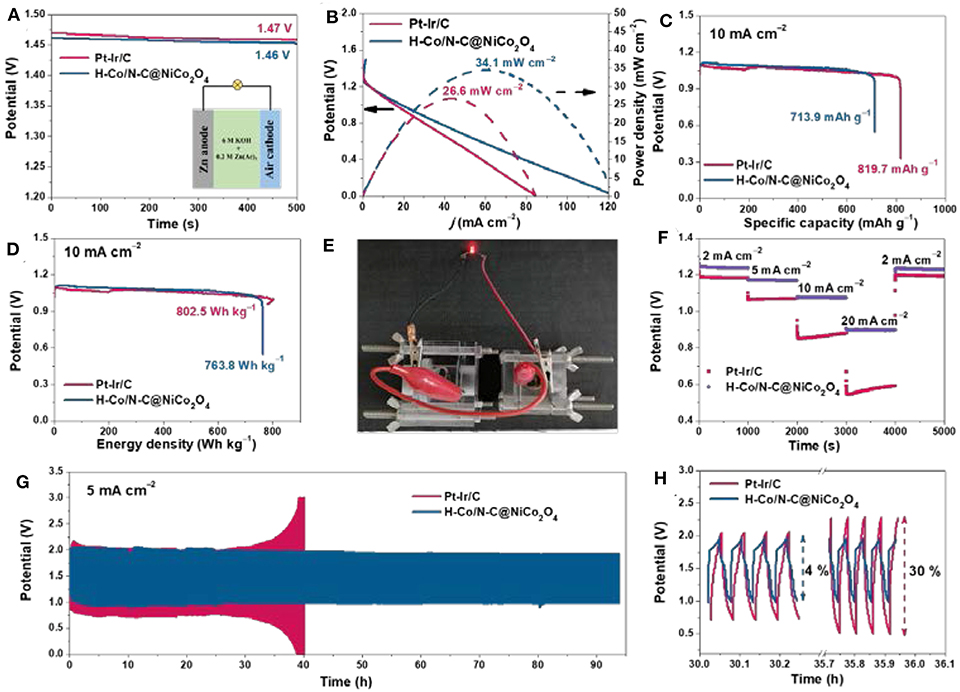
Figure 4. (A) Open-circuit plots (inset picture is the schematic representation of Zn–air battery), (B) polarization curves and corresponding power density plots of the Zn–air battery based on H-Co/N-C@NiCo2O4 and Pt-Ir/C. The calculated (C) specific capacity and (D) energy density of the Zn–air battery based on H-Co/N-C@NiCo2O4 and Pt-Ir/C. (E) Photograph of a red LED powered by two Zn–air battery based on H-Co/N-C@NiCo2O4 in series. (F) Galvanostatic discharge curves, (G,H) galvanostatic discharge–charge cycling curves of Zn–air battery based on H-Co/N-C@NiCo2O4 and Pt-Ir/C.
A most typical strategy to achieve high performance of a device system is to increase mass loading of electrocatalysts (Liu et al., 2018). However, the issues of physical limits to mass loading and plateau effect in performance are all inevitable (Xie et al., 2019). In this work, we further study the battery performance of H-Co/N-C@NiCo2O4 under high mass loading. Obviously, the peak power density of H-Co/N-C@NiCo2O4 gradually increases from 27.2 to 49.7 mW cm−2 with the increasing mass loading from 0.5 to 4 mg cm−2 (Figure 5A and Table S5), which demonstrates that most active sites could participate into the reaction in spite of the electrocatalyst layers becoming thicker (Figure 5B). This high mass-loading-independent performance of H-Co/N-C@NiCo2O4 is largely ascribed to the following hierarchical structure features and compositional merits. First, the synergetic effect of Co/N-C and NiCo2O4 guarantees promising bi-functional performance for both ORR and OER (ΔE = 0.82 V). Second, the hierarchical structure provides large surface area, which enables fast charge transfer and more active sites accessible to electrolyte and O2. Third, the hollow microsphere with sufficient internal void space and abundant mesopores serves as an efficient buffering reservoir and facilitates the fast diffusion of electrolyte. Last, this unique microsphere structure ensures good mechanical stability (more than 90 h) and minimizes particle aggregation, which is important to the practical application in ZAB (Figure 5C).
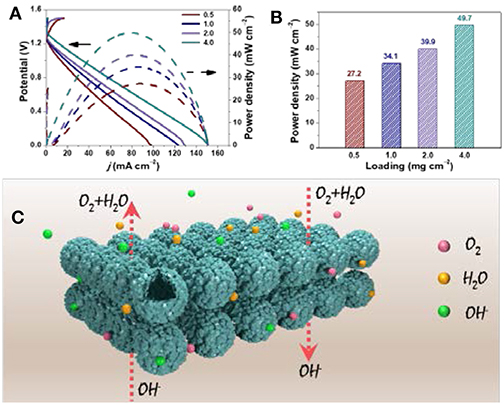
Figure 5. (A) Polarization curves and corresponding power density plots of H-Co/N-C@NiCo2O4 with different mass loading. (B) The histogram of power density vs. mass-loading. (C) Schematic illustration of electrochemical process on H-Co/N-C@NiCo2O4.
Conclusion
In summary, a hierarchical hollow structure H-Co/N-C@NiCo2O4 microsphere with cobalt/nitrogen-doped carbon as core and Ni-Co mixed metal oxides as shell has been successfully synthesized via a facile self-template approach. Benefiting from the advantages of this structure and composition, the H-Co/N-C@NiCo2O4 exhibits superior electrocatalytic activity and long-term stability for both ORR and OER. Furthermore, H-Co/N-C@NiCo2O4 is constructed as an air electrode for rechargeable ZAB, delivering a high power density and long cycling stability. The good linear relationship between the power density and various mass loading of H-Co/N-C@NiCo2O4 on the electrode demonstrates that the performance has been well-maintained even under high dense packing of catalysts, which offers a new pathway for the practical applications in ZAB.
Data Availability Statement
All datasets generated for this study are included in the manuscript/Supplementary Files.
Author Contributions
MS conceived and supervised the project. YS and WX performed the experiment, characterized the materials, and wrote the manuscript supervised by JG and MS. SL performed the SEM characterization. All authors discussed the results and commented on the paper.
Funding
This work was supported by the National Natural Science Foundation of China (21601011 and 21521005) and the Fundamental Research Funds for the Central Universities (XK1802-6).
Conflict of Interest
The authors declare that the research was conducted in the absence of any commercial or financial relationships that could be construed as a potential conflict of interest.
Supplementary Material
The Supplementary Material for this article can be found online at: https://www.frontiersin.org/articles/10.3389/fmats.2019.00261/full#supplementary-material
References
Aijaz, A., Masa, J., Rosler, C., Xia, W., Weide, P., Botz, A. J., et al. (2016). Co@Co3O4 encapsulated in carbon nanotube-grafted nitrogen-doped carbon polyhedra as an advanced bifunctional oxygen electrode. Angew. Chem. Int. Ed. 55, 4087–4091. doi: 10.1002/anie.201509382
Amiinu, I. S., Liu, X., Pu, Z., Li, W., Li, Q., Zhang, J., et al. (2018). From 3D ZIF nanocrystals to Co-Nx/C nanorod array electrocatalysts for ORR, OER, and Zn-air batteries. Adv. Funct. Mater. 28, 1704638–1704646. doi: 10.1002/adfm.201704638
Asset, T., Job, N., Busby, Y., Crisci, A., Martin, V., Stergiopoulos, V., et al. (2018). Porous hollow PtNi/C electrocatalysts: carbon support considerations to meet performance and stability requirements. ACS Catal. 8, 893–903. doi: 10.1021/acscatal.7b03539
Audichon, T., Napporn, T. W., Canaff, C., Morais, C., Comminges, C., and Kokoh, K. B. (2016). IrO2 coated on RuO2 as efficient and stable electroactive nanocatalysts for electrochemical water splitting. J. Phys. Chem. C 120, 2562–2573. doi: 10.1021/acs.jpcc.5b11868
Cao, C., Ma, D., Xu, Q., Wu, X., and Zhu, Q. (2018). Semisacrificial template growth of self-supporting MOF nanocomposite electrode for efficient electrocatalytic water oxidation. Adv. Funct. Mater. 29, 1807418–1807425. doi: 10.1002/adfm.201807418
Chen, T., Zhang, Z., Cheng, B., Chen, R., Hu, Y., Ma, L., et al. (2017). Self-templated formation of interlaced carbon nanotubes threaded hollow Co3S4 nanoboxes for high-rate and heat-resistant lithium-sulfur batteries. J. Am. Chem. Soc. 139, 12710–12715. doi: 10.1021/jacs.7b06973
Chen, X., Zhou, Z., Karahan, H. E., Shao, Q., Wei, L., and Chen, Y. (2018). Recent advances in materials and design of electrochemically rechargeable zinc-air batteries. Small 14, 1801929–1801957. doi: 10.1002/smll.201801929
Cui, B., Lin, H., Liu, Y., Li, J.-B., Sun, P., Zhao, X., et al. (2009). Photophysical and photocatalytic properties of core-ring structured NiCo2O4 nanoplatelets. J. Phys. Chem. C 113, 14083–14087. doi: 10.1021/jp900028t
Ferrero, G. A., Fuertes, A. B., Sevilla, M., and Titirici, M. M. (2016). Efficient metal-free N-doped mesoporous carbon catalysts for ORR by a template-free approach. Carbon N. Y. 106, 179–187. doi: 10.1016/j.carbon.2016.04.080
Fu, Y., Yu, H., Jiang, C., Zhang, T., Zhan, R., Li, X., et al. (2018). NiCo alloy nanoparticles decorated on N-doped carbon nanofibers as highly active and durable oxygen electrocatalyst. Adv. Funct. Mater. 28, 1705094–1705103. doi: 10.1002/adfm.201705094
Ganesan, P., Prabu, M., Sanetuntikul, J., and Shanmugam, S. (2015). Cobalt sulfide nanoparticles grown on nitrogen and sulfur codoped graphene oxide: an efficient electrocatalyst for oxygen reduction and evolution reactions. ACS Catal. 5, 3625–3637. doi: 10.1021/acscatal.5b00154
Gu, C., Hu, S., Zheng, X., Gao, M. R., Zheng, Y. R., Shi, L., et al. (2018). Synthesis of sub-2 nm iron-doped NiSe2 nanowires and their surface-confined oxidation for oxygen evolution catalysis. Angew. Chem. Int. Ed. 57, 4020–4024. doi: 10.1002/anie.201800883
Guan, B. Y., Yu, L., and Lou, X. W. D. (2017). Formation of single-holed cobalt/N-doped carbon hollow particles with enhanced electrocatalytic activity toward oxygen reduction reaction in alkaline media. Adv. Sci. 4, 1700247–1700252. doi: 10.1002/advs.201700247
Guan, C., Liu, X., Ren, W., Li, X., Cheng, C., and Wang, J. (2017). Rational design of metal-organic framework derived hollow NiCo2O4 arrays for flexible supercapacitor and electrocatalysis. Adv. Energy Mater. 7, 1602391–1602398. doi: 10.1002/aenm.201602391
Guo, Y., Yuan, P., Zhang, J., Xia, H., Cheng, F., Zhou, M., et al. (2018). Co2P-CoN double active centers confined in N-doped carbon nanotube: heterostructural engineering for trifunctional catalysis toward HER, ORR, OER, and Zn-air batteries driven water splitting. Adv. Funct. Mater. 28, 1805641–1805649. doi: 10.1002/adfm.201805641
Guo, Z., Wang, F., Xia, Y., Li, J., Tamirat, A. G., Liu, Y., et al. (2018). In situ encapsulation of core-shell-structured Co@Co3O4 into nitrogen-doped carbon polyhedra as a bifunctional catalyst for rechargeable Zn-air batteries. J. Mater. Chem. A 6, 1443–1453. doi: 10.1039/C7TA09958D
Hang, C., Zhang, J., Zhu, J., Li, W., Kou, Z., and Huang, Y. (2018). In situ exfoliating and generating active sites on graphene nanosheets strongly coupled with carbon fiber toward self-standing bifunctional cathode for rechargeable Zn-air batteries. Adv. Energy Mater. 8, 1703539–1703547. doi: 10.1002/aenm.201703539
Jia, G., Zhang, W., Fan, G., Li, Z., Fu, D., Hao, W., et al. (2017). Three-dimensional hierarchical architectures derived from surface-mounted metal-organic framework membranes for enhanced electrocatalysis. Angew. Chem. Int. Ed. 56, 13781–13785. doi: 10.1002/anie.201708385
Jiang, H., Liu, Y., Li, W., and Li, J. (2018). Co nanoparticles confined in 3D nitrogen-doped porous carbon foams as bifunctional electrocatalysts for long-life rechargeable Zn-air batteries. Small 14, 1703739–1703748. doi: 10.1002/smll.201703739
Jiang, R., Li, L., Sheng, T., Hu, G., Chen, Y., and Wang, L. (2018). Edge-site engineering of atomically dispersed Fe-N4 by selective C-N bond cleavage for enhanced oxygen reduction reaction activities. J. Am. Chem. Soc. 140, 11594–11598. doi: 10.1021/jacs.8b07294
Kaneti, Y. V., Dutta, S., Hossain, M. S. A., Shiddiky, M. J. A., Tung, K. L., Shieh, F. K., et al. (2017). Strategies for improving the functionality of zeolitic imidazolate frameworks: tailoring nanoarchitectures for functional applications. Adv. Mater. Weinheim. 29, 1700213–1700243. doi: 10.1002/adma.201700213
Lee, J., Ha, J. U., Choe, S., Lee, C. S., and Shim, S. E. (2006). Synthesis of highly monodisperse polystyrene microspheres via dispersion polymerization using an amphoteric initiator. J. Colloid Interf. Sci. 298, 663–671. doi: 10.1016/j.jcis.2006.01.001
Lee, J. S., Tai Kim, S., Cao, R., Choi, N. S., Liu, M., Lee, K. T., et al. (2011). Metal-air batteries with high energy density: Li-air versus Zn-air. Adv. Energy Mater. 1, 34–50. doi: 10.1002/aenm.201000010
Li, J., Lu, S. Q., Huang, H. L., Liu, D. H., Zhuang, Z. B., and Zhong, C. L. (2018). ZIF-67 as continuous self-sacrifice template derived NiCo2O4/Co,N-CNTs nanocages as efficient bifunctional electrocatalysts for rechargeable Zn-air batteries. ACS Sustainable Chem. Eng. 6, 10021–10029. doi: 10.1021/acssuschemeng.8b01332
Li, T., Lu, Y., Zhao, S., Gao, Z., and Song, Y. (2018). Co3O4-doped Co/CoFe nanoparticles encapsulated in carbon shells as bifunctional electrocatalysts for rechargeable Zn-Air batteries. J. Mater. Chem. A 6, 3730–3737. doi: 10.1039/C7TA11171A
Li, Y., and Dai, H. (2014). Recent advances in zinc-air batteries. Chem. Soc. Rev. 43, 5257–5275. doi: 10.1039/C4CS00015C
Li, Y., Li, F. M., Meng, X. N., Li, S. N., Zeng, J. H., and Chen, Y. (2018). Ultrathin Co3O4 nanomeshes for the oxygen evolution reaction. ACS Catal. 8, 1913–1920. doi: 10.1021/acscatal.7b03949
Li, Z., Shao, M., An, H., Wang, Z., Xu, S., Wei, M., et al. (2015). Fast electrosynthesis of Fe-containing layered double hydroxide arrays toward highly efficient electrocatalytic oxidation reactions. Chem. Sci. 6, 6624–6631. doi: 10.1039/C5SC02417J
Li, Z., Shao, M., Zhou, L., Zhang, R., Zhang, C., Wei, M., et al. (2016). Directed growth of metal-organic frameworks and their derived carbon-based network for efficient electrocatalytic oxygen reduction. Adv. Mater. Weinheim. 28, 2337–2344. doi: 10.1002/adma.201505086
Liang, Q., Jin, H., Wang, Z., Xiong, Y., Yuan, S., Zeng, X., et al. (2019). Metal-organic frameworks derived reverse-encapsulation Co-NC@Mo2C complex for efficient overall water splitting. Nano Energy 57, 746–752. doi: 10.1016/j.nanoen.2018.12.060
Liu, J., Zhu, D., Zheng, Y., Vasileff, A., and Qiao, S. Z. (2018). Self-supported earth-abundant nanoarrays as efficient and robust electrocatalysts for energy-related reactions. ACS Catal. 8, 6707–6732. doi: 10.1021/acscatal.8b01715
Liu, Y., Dong, P., Li, M., Wu, H., Zhang, C., Han, L., et al. (2019). Cobalt nanoparticles encapsulated in nitrogen-doped carbon nanotube as bifunctional-catalyst for rechargeable Zn-air batteries. Front. Mater. 6, 85–101. doi: 10.3389/fmats.2019.00085
Meng, F. L., Liu, K. H., Zhang, Y., Shi, M. M., Zhang, X. B., Yan, J. M., et al. (2018). Recent advances toward the rational design of efficient bifunctional air electrodes for rechargeable Zn-air batteries. Small 14, 1703843–1703862. doi: 10.1002/smll.201703843
Ni, B., Ouyang, C., Xu, X., Zhuang, J., and Wang, X. (2017). Modifying commercial carbon with trace amounts of ZIF to prepare derivatives with superior ORR activities. Adv. Mater. Weinheim. 29, 1701354–1701360. doi: 10.1002/adma.201701354
Park, J., Park, M., Nam, G., Kim, M. G., and Cho, J. (2017). Unveiling the catalytic origin of nanocrystalline yttrium ruthenate pyrochlore as a bifunctional electrocatalyst for Zn-air batteries. Nano Lett. 17, 3974–3981. doi: 10.1021/acs.nanolett.7b01812
Peng, S., Gong, F., Li, L., Yu, D., Ji, D., Zhang, T., et al. (2018). Necklace-like multishelled hollow spinel oxides with oxygen vacancies for efficient water electrolysis. J. Am. Chem. Soc. 140, 13644–13653. doi: 10.1021/jacs.8b05134
Prabu, M., Ketpang, K., and Shanmugam, S. (2014). Hierarchical nanostructured NiCo2O4 as an efficient bifunctional non-precious metal catalyst for rechargeable zinc-air batteries. Nanoscale 6, 3173–3181. doi: 10.1039/c3nr05835b
Qiu, H. J., Du, P., Hu, K., Gao, J., Li, H., Liu, P., et al. (2019). Metal and nonmetal codoped 3D nanoporous graphene for efficient bifunctional electrocatalysis and rechargeable Zn-air batteries. Adv. Mater. Weinheim. 31, 1900843–1900849. doi: 10.1002/adma.201900843
Shang, L., Yu, H., Huang, X., Bian, T., Shi, R., Zhao, Y., et al. (2016). Well-dispersed ZIF-derived Co,N-Co-doped carbon nanoframes through mesoporous-silica-protected calcination as efficient oxygen reduction electrocatalysts. Adv. Mater. Weinheim. 28, 1668–1674. doi: 10.1002/adma.201505045
Umeshbabu, E., Rajeshkhanna, G., Justin, P., and Rao, G. R. (2015). Synthesis of mesoporous NiCo2O4-rGO by a solvothermal method for charge storage applications. RSC Adv. 5, 66657–66666. doi: 10.1039/C5RA11239G
Wang, H. F., Tang, C., and Zhang, Q. (2018). A review of precious-metal-free bifunctional oxygen electrocatalysts: rational design and applications in Zn-air batteries. Adv. Funct. Mater. 28, 1803329–1803350. doi: 10.1002/adfm.201803329
Wang, T., Kou, Z., Mu, S., Liu, J., He, D., Amiinu, I. S., et al. (2018). 2D dual-metal zeolitic-imidazolate-framework-(ZIF)-derived bifunctional air electrodes with ultrahigh electrochemical properties for rechargeable zinc-air batteries. Adv. Funct. Mater. 28, 1705048–1705056. doi: 10.1002/adfm.201705048
Wang, Z., Jin, H., Meng, T., Liao, K., Meng, W., Yang, J., et al. (2018). Fe, Cu-coordinated ZIF-derived carbon framework for efficient oxygen reduction reaction and zinc-air batteries. Adv. Funct. Mater. 28, 1802596–1802603. doi: 10.1002/adfm.201802596
Wu, J., Zhang, J., Peng, Z., Yang, S., Wagner, F. T., and Yang, H. (2010). Truncated octahedral Pt3Ni oxygen reduction reaction electrocatalysts. J. Am. Chem. Soc. 132, 4984–4985. doi: 10.1021/ja100571h
Wu, R., Xiao, B., Gao, Q., Zheng, Y. R., Zheng, X. S., Zhu, J. F., et al. (2018). A janus nickel cobalt phosphide catalyst for high-efficiency neutral-pH water splitting. Angew. Chem. 57, 15445–15449. doi: 10.1002/anie.201808929
Xie, W. F., Li, Z. H., Jiang, S., Li, J. B., Shao, M. F., and Wei, M. (2019). Mass-loading independent electrocatalyst with high performance for oxygen reduction reaction and Zn-air battery based on Co-N-codoped carbon nanotube assembled microspheres. Chem. Eng. J. 373, 734–743. doi: 10.1016/j.cej.2019.04.066
Yang, Q. H., Li, Z. H., Zhang, R. K., Zhou, L., Shao, M. F., and Wei, M. (2017). Carbon modified transition metal oxides/hydroxides nanoarrays toward high-performance flexible all-solid-state supercapacitors. Nano Energy 41, 408–416. doi: 10.1016/j.nanoen.2017.09.049
Zhan, G., and Zeng, H. C. (2017). ZIF-67-derived nanoreactors for controlling product selectivity in CO2 hydrogenation. ACS Catal. 7, 7509–7519. doi: 10.1021/acscatal.7b01827
Zhang, C., Fu, L., Liu, N., Liu, M., Wang, Y., and Liu, Z. (2011). Synthesis of nitrogen-doped graphene using embedded carbon and nitrogen sources. Adv. Mater. Weinheim. 23, 1020–1024. doi: 10.1002/adma.201004110
Zhang, G., and Lou, X. W. (2013). General solution growth of mesoporous NiCo2O4 nanosheets on various conductive substrates as high-performance electrodes for supercapacitors. Adv. Mater. Weinheim. 25, 976–979. doi: 10.1002/adma.201204128
Zhang, J., Wang, T., Pohl, D., Rellinghaus, B., Dong, R., Liu, S., et al. (2016). Interface engineering of MoS2 /Ni3S2 heterostructures for highly enhanced electrochemical overall-water-splitting activity. Angew. Chem. Int. Ed. 55, 6702–6707. doi: 10.1002/anie.201602237
Zhao, D., Shui, J. L., Chen, C., Chen, X., Reprogle, B. M., Wang, D., et al. (2012). Iron imidazolate framework as precursor for electrocatalysts in polymer electrolyte membrane fuel cells. Chem. Sci. 3, 3200–3205. doi: 10.1039/c2sc20657a
Zhou, L., Shao, M., Zhang, C., Zhao, J., He, S., Rao, D., et al. (2017b). Hierarchical CoNi-sulfide nanosheet arrays derived from layered double hydroxides toward efficient hydrazine electrooxidation. Adv. Mater. Weinheim. 29, 1604080–1604087. doi: 10.1002/adma.201604080
Zhou, L., Shao, M. F., Li, J. B., Jiang, S., Wei, M., and Duan, X. (2017a). Two-dimensional ultrathin arrays of CoP: electronic modulation toward high performance overall water splitting. Nano Energy 41, 583–590. doi: 10.1016/j.nanoen.2017.10.009
Keywords: bi-functional electrocatalyst, ORR and OER, synergetic effect, hierarchical hollow nanostructure, zinc–air battery
Citation: Song Y, Xie W, Li S, Guo J and Shao M (2019) Hierarchical Hollow Co/N-C@NiCo2O4 Microsphere as an Efficient Bi-functional Electrocatalyst for Rechargeable Zn–Air Battery. Front. Mater. 6:261. doi: 10.3389/fmats.2019.00261
Received: 18 August 2019; Accepted: 01 October 2019;
Published: 25 October 2019.
Edited by:
Zhenhai Xia, University of North Texas, United StatesReviewed by:
Shichun Mu, Wuhan University of Technology, ChinaBao Yu Xia, Huazhong University of Science and Technology, China
Copyright © 2019 Song, Xie, Li, Guo and Shao. This is an open-access article distributed under the terms of the Creative Commons Attribution License (CC BY). The use, distribution or reproduction in other forums is permitted, provided the original author(s) and the copyright owner(s) are credited and that the original publication in this journal is cited, in accordance with accepted academic practice. No use, distribution or reproduction is permitted which does not comply with these terms.
*Correspondence: Jian Guo, Z3VvamlhbiYjeDAwMDQwO21haWwuYnVjdC5lZHUuY24=; Mingfei Shao, c2hhb21mJiN4MDAwNDA7bWFpbC5idWN0LmVkdS5jbg==
†These authors have contributed equally to this work