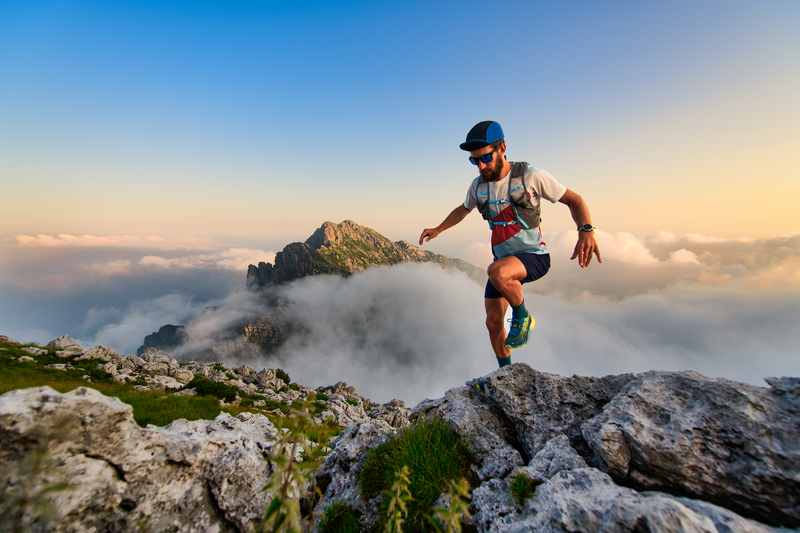
94% of researchers rate our articles as excellent or good
Learn more about the work of our research integrity team to safeguard the quality of each article we publish.
Find out more
ORIGINAL RESEARCH article
Front. Mater. , 18 October 2019
Sec. Ceramics and Glass
Volume 6 - 2019 | https://doi.org/10.3389/fmats.2019.00259
This article is part of the Research Topic Frontiers in Materials: Rising Stars View all 56 articles
Failure of PV modules frequently occurs as a result of degradation of their encapsulation material by destructive UV radiation. Both the life expectancy and efficiency of PV modules can be improved by reducing the transmittance of the destructive UV radiation through the cover glass without compromising the transmittance in the visible wavelength region. In addition, if the absorbed UV photons can be down-shifted to wavelengths that can be more efficiently converted to electrical energy, an additional increase of the PV efficiency could be achieved. In this study we have investigated transparent ZnO and TiO2 thin films deposited by spray pyrolysis on soda lime silicate float glass as functional layers on PV cover glass. The optical bandgap, UV-cutoff, UV-Vis transmittance, reflectivity (total and diffuse) and photoluminescence have been determined. The ZnO coating shifted the optical bandgap to longer wavelengths, resulting in a reduction of the transmittance of destructive UV radiation by up to ~85%. Distinct photoluminescence peaks at 377 nm and at 640 nm were observed for one of the ZnO samples. The TiO2 coated glasses also showed an increased UV cutoff, which resulted in a reduction of transmittance of destructive UV radiation by up to 75%. However, no photoluminescence peaks could be observed from the TiO2 films with 325 nm excitation laser, which can be explained by the fact that only indirect interband transitions are accessible at this excitation wavelength. Deposition of both ZnO and TiO2 coatings resulted in a reduction of the transmitted light convertible by PV modules, by up to 12.3 and 21.8%, respectively. The implication of the results is discussed in terms of lifetime expectancy and efficiency of PV modules.
To stabilize the global temperature and mitigate climate change, the emission of anthropogenic greenhouse gases will have to be greatly reduced. To make it possible, the energy sector will have to transfer from fossil energy to environmentally friendly and carbon neutral sources (IPCC, 2014). Solar energy exists in abundance. In roughly 90 min, the solar energy that reaches the earth equals the consumption of all human societies globally during one year (IEA, 2011). Only a fraction of this energy is captured today, and photovoltaic (PV) modules account for a marginal part of the electricity production worldwide, around 1.8% at the end of 2016. In recent years, however, the sector has been growing exponentially at a rapid rate, which means that the ability to increase efficiency and lifespan of PV modules is interesting from an energy perspective (Masson et al., 2018). PV modules consist of a number of interconnected PV-cells, embedded in an encapsulant and a protective cover glass on the top. One of the issues facing the PV modules available today is the degradation of their encapsulant, which most often consists of EVA (ethylene vinyl acetate). It is damaged by UV radiation with wavelengths below 350 nm. The UV radiation makes the encapsulant degrade and acquire a yellow and eventually brown hue, which reduces the efficiency of the PV modules (Czanderna and Pern, 1996; Oliveira et al., 2018).
Developing the cover glass has become increasingly important as the share of cost for the cover glass is high (Burrows and Fthenakis, 2015). The cover glass (Brow and Schmitt, 2009; Deubener et al., 2009) has several important functionalities, e.g., providing optimal light capture, rigidity, mechanical protection, and chemical protection. Optimal light capture depends on the optical properties of the cover glass such as absorption and reflection. The latter comprises the largest part, about 8% for a typical flat glass, which can be minimized by employing antireflective coatings (Nielsen et al., 2014). Rigidity and mechanical protection are determined by the thickness, the elastic modulus and the strength of glass (Wondraczek et al., 2011), which for PV cover glass is conventionally thermally strengthened (Karlsson and Wondraczek, 2019). The strength of glass is lowered continuously in operation by handling, and it therefore also depends on the glass's scratch and crack resistance (Rouxel et al., 2014; Sundberg et al., 2019). The chemical protection is important and glass provides an excellent chemical protection where in principle the only weaknesses are the lamination (Kuitche et al., 2014) and the potential induced degradation (PID) (Oliveira et al., 2018).
The optical properties of flat glass (Bamford, 1982; Rubin, 1985) are affected by the presence of iron impurities in the glass melt as the iron in the glass increases the absorption of light in the glass in the UV-VIS region of the electromagnetic spectrum. Iron can be used as a colorant of glass, giving the glass a green tint (Volotinen et al., 2008). In some cases, this is a positive feature, e.g., when UV-protection is needed in beer and champagne bottles (Daneo et al., 2009). In other cases, as with PV-modules where transparency is coveted (Goodyear and Lindberg, 1980), the iron in the glass is considered as a contaminant. In these cases, low-iron glass, where measures have been taken to reduce the iron in the glass, is frequently used.
In the case of cover glass for PV modules, the trend has been to use low-iron glass to increase transmitted light (Deubener et al., 2009). A drawback to this type of glass is that a larger amount of high-energy UV radiation is transmitted, which is harmful to the encapsulation material EVA that is used in most PV modules today (Allsopp et al., 2018). When UV radiation below 350 nm reaches the PV module, both the semiconductor material (Osterwald et al., 2003) and the laminate (Kuitche et al., 2014; Oliveira et al., 2018) are degraded. The degradation of the EVA laminate is the major reason for the annual degradation of 0.6–2.5% (Jordan and Kurtz, 2013; Kuitche et al., 2014). As a result of the UV radiation, EVA degrades and loses some of its high transmissivity as it gets a yellow/brown hue and eventually starts to delaminate, letting moisture into the PV modules, which leads to failure of the PV module (Oliveira et al., 2018).
In the current study we have investigated float glass coated with ZnO and TiO2 thin films by spray pyrolysis of organometallic compounds of zinc and titanium. We present a detailed characterization of their optical properties by means of UV-Vis and photoluminescence spectroscopy.
Thin film depositions were made by spray pyrolysis in a hot wall reactor employing the precursor solutions shown in Table 1. The solutions were heated to 70°C for 2 h. The thin films were deposited on the air surface of AGC Planibel Clearview float glass samples (size 50 mm × 50 mm × 3.89 mm), which is a conventional soda-lime silicate float glass. The samples were heated up to 500°C, after which they were sprayed with the metal organic solution using a Preval sprayer (paintbrush equipment driven by the carrier gases dimethyl ether, isobutene, and propane). The furnace hatch was temporarily opened, and the precursor was sprayed onto the glass surface, manually holding the sprayer about a decimeter from the substrate. Between each opening of the furnace hatch, the furnace temperature was set to 500°C, implying that all depositions were made between 485 and 515°C. After deposition, the temperature in the furnace was lowered in a controlled manner at a cooling rate of 0.5°C / min down to 300°C, after which the furnace was turned off and the samples were left to cool down to room temperature. A series of six coated glasses with different deposited quantities were produced. The amount of precursor solution sprayed onto every glass sample is shown in Table 2, and the denotation of each sample according to the numbering. We believe that the deposited coatings are thin (<100 nm), mostly amorphous for both ZnO (Kamata et al., 1994; Hosseinmardi et al., 2012; Villegas et al., 2018) and TiO2 (Okuya et al., 1999; Abou-Helal and Seeber, 2002). This will be discussed in section Change in Transmittance and Reflection and section Effect on Photoluminescence. The surface morphologies of the coatings were measured using a Dimension 3100 (Bruker) Atomic Force Microscopy (AFM) and the root-mean-square (RMS) roughness was determined.
Table 2. Sample series, amount of solution sprayed in grams denoted according to this numbering Zn2, Zn3, … and Ti1, Ti2… etc.
Transmittance, T(λ), and reflectance, R(λ), spectra were recorded in the wavelength range between 325 and 850 nm with a step resolution of 2.5 nm, employing a Lambda 950 double-beam UV/Vis/NIR spectrophotometer equipped with an integrating sphere and a Spectralon reflectance standard. Both total and diffuse reflectance, R(λ), were measured with 8° incidence angle. The absorptance, A(λ) is then obtained as
Instrument corrected values of T(λ) and R(λ) were used in Equation (1) (Roos, 1993). In order to estimate the fraction of blocked UV light and the solar spectrum weighted T% and R% data for given single values throughout the paper, we calculate a Figure of Merit (FoM). The solar spectrum AM 1.5 G-173 from NREL, ϕAM 1.5 G(λ), is used as the solar light intensity distribution function, and the weighted average is between 320 and 350 nm for estimating UV blocking and between 350 and 1200 nm for estimating T% and R% as single values, according to
where λ and λ0 is the wavelength range that is evaluated and P(λ) represents T(λ) or R(λ).
The photoluminescence of the ZnO- and TiO2-coated samples was measured using a Renishaw Invia Raman microscope employing a 40 × objective lens. The samples were irradiated with a He-Cd laser with a wavelength of 325 nm, and photoluminescence of wavelengths between 330 and 720 nm were recorded. The maximum effect of the laser was 6 mW, but 99 and 90% of the laser flux was filtered away for the ZnO and TiO2 samples, respectively.
Energy calibration was performed by Raman spectroscopy on non-coated float glass with the same instrument settings. Prior to the measurements, the instrument was calibrated by measuring the 1,332 cm−1 peak of the diamond.
From the spectrophotometry data it is possible to estimate the optical bandgap, i.e., at which wavelength the coated glass starts to absorb most of the radiation acting as a short wavelength cutoff filter. In this study the cutoff wavelength was defined as the wavelength below which <10% of the incoming light is transmitted, i.e., at a somewhat larger wavelength compared to the optical bandgap.
The optical bandgap, Eg, of the coated glasses was analyzed according to a simplified demarcation point analysis which is very similar to the Tauc analysis (Tauc, 1968). The demarcation point can be graphically extracted from A(λ) by approximating the bandgap region below Eg with a fairly horizontal straight line and approximate the region above Eg (the actual UV-edge) with another straight line. The point where these two straight lines intersect is the demarcation point and is a good approximation of the Eg.
Figure 1 shows the transmittance at normal incidence for the glass samples coated with ZnO and TiO2, respectively. The insets in Figure 1 show optical microscopy images acquired with the Raman instrument, showing that the thin films consist of particulate features with a root-mean-square roughness of about 4–8 nm for the ZnO and 2–10 nm for the TiO2 samples (see Table 3) which is quite common for the spray pyrolysis technique (Perednis and Gauckler, 2005). The ZnO coatings show the expected rough surface, see Figure 2. The highest roughness is for the thinnest layer, Zn2, while the others have comparable roughness values. In comparison, for the TiO2 coated samples, see Figure 3, there are larger structures with peak to valley heights in the range 60–150 nm. Also, most samples show clear pits/openings in the film. The depths of these openings are in the range 10–40 nm. Both ZnO- and TiO2-coated glass show a decrease of the transmittance compared to the uncoated reference sample. The ZnO-coated samples showed larger transmittance in the visible regime than the TiO2-coated. While the sample coated with the largest amount of ZnO (sample Zn6) showed a transmittance of 74.7%, the transmittance for the sample coated with the largest amount of TiO2 (sample Ti6) was 66.7%, see Table 3. Glass with ZnO coatings showed a decreased transmittance in the UV regime <350 nm, exhibiting a plateau between 320 and 370 nm. A small absorption peak can also be observed for the reference samples at 380 which can be denoted to tetrahedral configuration of Fe3+ (Volotinen et al., 2008). Therefore, we attribute the plateau between 320 and 370 nm for the ZnO-coated samples to be caused by Fe3+ but sensitized by the presence of ZnO thin film.
Figure 1. Transmittance of thin film-coated glass samples at normal incidence as well as insets of microscope images of the samples taken from the Raman microscope.
Table 3. Optical properties of coated glass samples, the fraction of the blocked UV light and the transmittance was calculated using (Equation 2).
Figure 4 shows the reflectance in the glass samples coated with ZnO and TiO2. The TiO2 coated glasses show more reflection as expected due to a larger refractive index mismatch between the glass and the coating. The refractive index for TiO2 is 2.65 for rutile-TiO2 (Jellison et al., 1997) or 2.56 anatase-TiO2 (Schröder, 1928) and for ZnO 2.0 (Jellison and Boatner, 1998), all reported at 589.3 nm as reported by Shannon et al. (2002). The peaks at 375 nm for the ZnO samples coincide with their observed absorption edge in Figure 6 as expected for localized transitions, signaling the presence of defect states, possibly with contribution from Fe3+ charge transfer reactions, since the reference sample also exhibits small absorption in this region. It has only been possible to find a zone without that reflection behavior for Zn5. That zone without the mentioned peak corresponded to a place which was far from the internal crack that the sample presented. It has been seen that the samples with that behavior show more spot to spot deviance in reflection measurements. Figure 5 shows the diffuse reflection in the ZnO of the TiO2-coated samples, respectively. The diffuse reflection of the coatings is <4% for both coatings. The low diffuse reflection is likely to be caused by non-uniform deposited thin films. This means that parts of the diffuse transmitted light that reaches the glass back end will be reflected back into the glass instead of being transmitted through it, similar to a conventional light trapping and back-reflecting cover glass structure (Deubener et al., 2009).
Figure 6. Absorptance of ZnO- and TiO2-coated glass samples in the wavelength range near the UV cutoff.
The measured transmittance/reflectance spectra do not exhibit any interference patterns as is expected for thin films with thicknesses >> 100 nm.
Figure 6 shows the change in absorptance near the UV-cutoff in the glass samples coated with ZnO and TiO2. A trend can be observed of a shift of the UV-cutoff to longer wavelength as more ZnO was deposited. This trend is less clear for TiO2. The estimated optical bandgaps, using the demarcation point analysis, are shown in Table 3. In the ZnO spectra, two UV edges can be observed: one at about 330 to 340 nm and another one at 375 to 380 nm. The latter gave optical bandgap values in the range of 3.21–3.22, i.e., very similar to the wurtzite form of ZnO. The optical band gap of ZnO in wurtzite and zinc-blende form is 3.22 and 3.12 eV, respectively (Lee et al., 2002). The TiO2-coated glasses also show a small absorption peak at 380 nm which is caused by the tetrahedral configuration of Fe3+ (Volotinen et al., 2008). TiO2 in rutile, anatase, and brookite form has optical band gaps at 3.00, 3.21, and 3.13 eV, respectively (Dhar et al., 2013). The estimated optical bandgaps in Table 3 are in the range around 3.55 eV, i.e., much influenced by the float glass and its conventional impurity Fe2O3. Fe2O3 has a considerable effect on the UV absorption and probably overshadows some of the effects that would otherwise be seen by our coatings (Volotinen et al., 2008). This is discussed more in section Discussion of the Effect for PV Modules.
Table 3 shows the UV-blocking characteristics as well as the transmittance of light convertible for Si-PV modules. The-ZnO coated samples show larger percentages of blocked UV light with larger transmission in the visible range compared with TiO2 coatings. Compared to the reference float glass, the coatings show a relative increase of the FoM (c.f. Equation 2) up to 54.3 and 36.0% of UV-blocking characteristics and a relative decrease of the transmittance by up to 12.3 and 21.8% for ZnO and TiO2 coatings, respectively.
Figure 7 shows the photoluminescence spectra between 330 and 720 nm of the ZnO- and TiO2-coated glass substrates. All samples as well as the reference shows a distinct peak around 695 nm. This can probably be attributed to the Fe3+ present in the glass since it has shown a peak in photoluminescence at wavelengths around 700 nm when present in silicate glass (Bingham et al., 2007). The reference sample also shows a peak at 390 nm, this could be due to photoluminescence from SiO2 (Nagata et al., 2004).
Figure 7. Photoluminescence spectra of ZnO- and TiO2-coated glass samples. The spectra were normalized to the highest peak. The inset shows the Zn4 photoluminescence spectra subtracted by the reference, clearly revealing the 640 nm emission peak.
All Zn-samples, except Zn2, possess a strong narrow emission peak that can be observed at approximately 377 nm, which is not present in the reference sample. This corresponds well to the photoluminescence observed in ZnO nanorods by Wu et al. (2006) and which can be attributed to a common excitation recombination of ZnO (Studenikin et al., 1998; Kong et al., 2001). The 377 nm PL emission can be an explanation for the sensitization of Fe3+ absorption at 320–380 nm, c.f. Figure 6. Sample Zn4 shows a different pattern compared to the reference and the other coated samples in the wavelength interval 550–650 nm. When the photoluminescence from the reference sample was subtracted from sample 4, a peak at 640 nm could be distinguished; this is shown in the inset of Figure 7. This also corresponds well to the photoluminescence observed for ZnO nanorods (Wu et al., 2006), which was also observed by Studenikin et al. (1998) and has been attributed to oxygen interstitial defects in ZnO, i.e., over-stoichiometric ZnO. The opposite, under-stoichiometric ZnO, is indicated to give a green emission at about 510 nm.
The effect that our deposited ZnO and TiO2 thin films have on the photoluminescence emission makes us confident that these thin films are mostly amorphous. The photoluminescent bands that we interpret as defects and the Fe3+ sensitization emissions for the samples are strong and dominate the photoluminescence spectra, see Figure 7. For the ZnO-coated samples it is despite that similar literature data, but with somewhat different experimental circumstances, refer to the ZnO films as mostly crystalline (Kamata et al., 1994; Hosseinmardi et al., 2012; Villegas et al., 2018).
We have shown that UV blocking can be achieved with the cost of reducing the transmittance. Specifically, there was a 75.7% transmittance and a simultaneous reduction of 83.4% integrated transmittance for the sample Zn4, c.f. Table 3. This opens the possibility for maintaining UV protection and gaining useful energy for the PV by lowering the Fe2O3 content in the glass without compromising the service lifetime of the PV module. The energy balance for transmitted and useful light for PV modules will be possible to model and optimize in future studies based on information as for instance possible limits for Fe2O3 content, cost and efficiency. Furthermore, photon energy down-conversion, i.e., photoluminescence, can be an advantage and a route to utilizing UV light while still not exposing the PV cells to UV light. As for disadvantages, we can list higher reflectivity and scattering. If the surface coating is properly structured, it might not be a serious disadvantage or perhaps even an advantage (Brongersma et al., 2014), as the diffused light contains in fact more photons than the direct light of normal incidence. This is especially valid for façade-applied PV modules where there is in fact very little solar radiation of normal incidence. Another parameter not previously mentioned is the factor of heat. A photon's energy that is not converted into electricity is transformed into heat that in fact lowers the efficiency of the PV module. Beyond the scope of the current paper we would also like to draw the attention to making crystalline ZnO or TiO2 coatings having similar beneficial properties but with the added value of photocatalysis (Gao and Nagai, 2006; He et al., 2012) and hydrophilic behavior with UV exposure (Watanabe et al., 1999; Sun et al., 2001), thereby giving PV-covered glasses reduced maintenance. Doped ZnO also offers another dimension as a transparent conductive coating offering possible IR reflection for wavelengths non-convertible to energy for PV modules (Deubener et al., 2009).
Glass coated with ZnO showed a trend to shift the UV-cutoff to longer wavelength as well as lowering the optical band gap of the coated glass sample. The major reason for this is likely to be caused by tetrahedrally coordinated Fe3+ having an absorption peak at about 380 nm but also being sensitized by the presence of the ZnO coating. Such a trend is less clear for the samples coated with TiO2. Both sample series showed a significant increase in total reflection for the normal incident light due to the higher refractive index of the thin film oxide coatings. However, the increase in diffuse reflection was significantly lower, <4%; this is an advantage for application on the cover glass of PV-modules where most of the incoming light will be of diffuse character.
The coated glass showed a potential improvement in life expectancy of PV modules through a decrease of destructive UV-radiation transmission to the encapsulant up to a relative 36.0% and 54.3% for TiO2 and ZnO, coatings, respectively. Additionally, although the coated samples have shown a relative transmission reduction at the useful spectral region up to 21.8 and 12.3% for TiO2 and ZnO coatings, respectively, the transmissivity degradation of the encapsulant should be effectively prevented. For ZnO it is evident that the Fe3+ content plays an important role for the UV-blocking activity, which would be a tradeoff between limiting the glass's iron content while still having enough UV protection. Furthermore, ZnO-coated glass also showed potential regarding down conversion of UV light to visible wavelength with peaks at 377 and 640 nm. Thus, ZnO is feasible to be investigated for application as coating to cover glasses of PV modules but must be optimized as there is a tradeoff between UV-blocking and transmittance in the useful spectral region for PV modules.
SK perceived the idea of the paper. WJ performed the thin film deposition and photoluminescence measurements with the guidance of SK, BJ, and LÖ. AP performed the UV-VIS spectroscopy measurements with guidance from SA. SA performed the AFM measurements. WJ and AP evaluated the results of the measurements. WJ and SK wrote the draft of the paper. All authors were involved in the discussions and elaborating the final manuscript text.
The authors declare that the research was conducted in the absence of any commercial or financial relationships that could be construed as a potential conflict of interest.
Jakob Thyr at the Ångström Laboratory, Uppsala University was greatly acknowledged for his supervision during the photoluminescence measurements.
Abou-Helal, M. O., and Seeber, W. T. (2002). Preparation of TiO2 thin films by spray pyrolysis to be used as a photocatalyst. Appl. Surf. Sci. 195, 53–62. doi: 10.1016/S0169-4332(02)00533-0
Allsopp, B. L., Christopoulou, G., Brookfield, A., Forder, S. D., and Bingham, P. A. (2018). Optical and structural properties of d0 ion-doped silicate glasses for photovoltaic applications. Phys. Chem. Glasses Euro. J. Glass Sci. Technol. B 59, 193–202. doi: 10.13036/17533562.59.4.003
Bamford, C. R. (1982). Optical properties of flat glass. J. Non Cryst. Solids 47, 1–20. doi: 10.1016/0022-3093(82)90342-8
Bingham, P. A., Parker, J. M., Searle, T. M., and Smith, I. (2007). Local structure and medium range ordering of tetrahedrally coordinated Fe3+ ions in alkali–alkaline earth–silica glasses. J. Non-Crystalline Solids 353, 2479–2494. doi: 10.1016/j.jnoncrysol.2007.03.017
Brongersma, M. L., Cui, Y., and Fan, S. (2014). Light management for photovoltaics using high-index nanostructures. Nat. Mater. 13, 451–460. doi: 10.1038/nmat3921
Brow, R. K., and Schmitt, M. L. (2009). A survey of energy and environmental applications of glass. J. Eur. Ceram. Soc. 29, 1193–1201. doi: 10.1016/j.jeurceramsoc.2008.08.011
Burrows, K., and Fthenakis, V. (2015). Glass needs for a growing photovoltaics industry. Solar Energy Mater. Solar Cells 132, 455–459. doi: 10.1016/j.solmat.2014.09.028
Czanderna, A. W., and Pern, F. J. (1996). Encapsulation of PV modules using ethylene vinyl acetate copolymer as a pottant: a critical review. Solar Energy Mater. Solar Cells 43, 101–181. doi: 10.1016/0927-0248(95)00150-6
Daneo, A. G., Falcone, R., and Hreglich, S. (2009). Effect of the redox state on container glass colour stability. Glass Technol. Euro. J. Glass Sci. Technol. A 50, 147–150. Available online at: https://www.ingentaconnect.com/content/sgt/gta/2009/00000050/00000003/art00004
Deubener, J., Helsch, G., Moiseev, A., and Bornhöft, H. (2009). Glasses for solar energy conversion systems. J. Eur. Ceram. Soc. 29, 1203–1210. doi: 10.1016/j.jeurceramsoc.2008.08.009
Dhar, S., Roy Barman, A., Rusydi, A., Gopinadhan, K., Feng, Y., Breese, M., et al. (2013). “Effect of Ta alloying on the optical, electronic, and magnetic properties of TiO2 thin films,” in Functional Metal Oxides: New Science and Novel Applications, eds S. B. Ogale, T. V. Venkatesan, and M. G. Blamire (Weinheim: Wiley-VCH Verlag GmbH & Co), 133–162. doi: 10.1002/9783527654864.ch4
Gao, Y., and Nagai, M. (2006). Morphology evolution of ZnO thin films from aqueous solutions and their application to solar cells. Langmuir 22, 3936–3940. doi: 10.1021/la053042f
Goodyear, J. K., and Lindberg, V. L. (1980). Low absorption float glass for back surface solar reflectors. Solar Energy Mater. 3, 57–67. doi: 10.1016/0165-1633(80)90049-0
He, H., Liu, C., Dubois, K. D., Jin, T., Louis, M. E., and Li, G. (2012). Enhanced charge separation in nanostructured TiO2 materials for photocatalytic and photovoltaic applications. Ind. Eng. Chem. Res. 51, 11841–11849. doi: 10.1021/ie300510n
Hosseinmardi, A., Shojaee, N., Keyanpour-Rad, M., and Ebadzadeh, T. (2012). A study on the photoluminescence properties of electrospray deposited amorphous and crystalline nanostructured ZnO thin films. Ceramics Int. 38, 1975–1980. doi: 10.1016/j.ceramint.2011.10.031
IPCC (2014). “Summary for policymakers,” in Climate Change 2014: Mitigation of Climate Change. Contribution of Working Group III to the Fifth Assessment Report of the Intergovernmental Panel on Climate Change, eds O. Edenhofer, R. Pichs-Madruga, Y. Sokona, E. Farahani, S. Kadner, K. Seyboth, A. Adler, I. Baum, S. Brunner, P. Eickemeier, B. Kriemann, J. Savolainen, S. Schlömer, C. von Stechow, T. Zwickel, and J. C. Minx (Cambridge, UK; New York, NY: Cambridge University Press).
Jellison, G. E., and Boatner, L. A. (1998). Optical functions of uniaxial ZnO determined by generalized ellipsometry. Phys. Rev. B 58, 3586–3589. doi: 10.1103/PhysRevB.58.3586
Jellison, G. E., Modine, F. A., and Boatner, L. A. (1997). Measurement of the optical functions of uniaxial materials by two-modulator generalized ellipsometry: rutile (TiO2). Opt. Lett. 22, 1808–1810. doi: 10.1364/OL.22.001808
Jordan, D. C., and Kurtz, S. R. (2013). Photovoltaic degradation rates—an analytical review. Prog. Photovoltaics Res. Appl. 21, 12–29. doi: 10.1002/pip.1182
Kamata, K., Nishino, J., Ohshio, S., Maruyama, K., and Ohtuki, M. (1994). Rapid formation of zinc oxide films by an atmospheric-pressure chemical vapor deposition method. J. Am. Ceramic Soc. 77, 505–508. doi: 10.1111/j.1151-2916.1994.tb07021.x
Karlsson, S., and Wondraczek, L. (2019). “Strengthening of Oxide Glasses,” in Encyclopedia for Glass Science, Technology, History and Culture, ed P. Richet (Hoboken, NJ: John Wiley & Sons Inc.).
Kong, Y. C., Yu, D. P., Zhang, B., Fang, W., and Feng, S. Q. (2001). Ultraviolet-emitting ZnO nanowires synthesized by a physical vapor deposition approach. Appl. Phys. Lett. 78, 407–409. doi: 10.1063/1.1342050
Kuitche, J. M., Pan, R., and TamizhMani, G. (2014). Investigation of dominant failure mode(s) for field-aged crystalline silicon PV modules under desert climatic conditions. IEEE J. Photovoltaics 4, 814–826. doi: 10.1109/JPHOTOV.2014.2308720
Lee, G. H., Kawazoe, T., and Ohtsu, M. (2002). Difference in optical bandgap between zinc-blende and wurtzite ZnO structure formed on sapphire (0001) substrate. Solid State Commun. 124, 163–165. doi: 10.1016/S0038-1098(02)00537-9
Masson, G., Kaizuka, I., and Cambiè, C. (2018). IEA Report PVPS: A Snapshot of Global PV (1992-2017). International Energy Agency.
Nagata, S., Yamamoto, S., Toh, K., Tsuchiya, B., Ohtsu, N., Shikama, T., et al. (2004). Luminescence in SiO2 induced by MeV energy proton irradiation. J. Nuclear Mater. 329–333, 1507–1510. doi: 10.1016/j.jnucmat.2004.04.242
Nielsen, K. H., Orzol, D. K., Koynov, S., Carney, S., Hultstein, E., and Wondraczek, L. (2014). Large area, low cost anti-reflective coating for solar glasses. Solar Energy Mater. Solar Cells 128, 283–288. doi: 10.1016/j.solmat.2014.05.034
Okuya, M., Prokudina, N. A., Mushika, K., and Kaneko, S. (1999). TiO2 thin films synthesized by the spray pyrolysis deposition (SPD) technique. J. Eur. Ceram. Soc. 19, 903–906. doi: 10.1016/S0955-2219(98)00341-0
Oliveira, M. C. C. D., Diniz Cardoso, A. S. A., Viana, M. M., and Lins, V. d.F.C. (2018). The causes and effects of degradation of encapsulant ethylene vinyl acetate copolymer (EVA) in crystalline silicon photovoltaic modules: A review. Renew. Sust. Energy Rev. 81, 2299–2317. doi: 10.1016/j.rser.2017.06.039
Osterwald, C. R., Benner, J. P., Pruett, J., Anderberg, A., Rummel, S., and Ottoson, L. (2003). “Degradation in weathered crystalline-silicon PV modules apparently caused by UV radiation,” in 3rd World Conference on Photovoltaic Energy Conversion (Osaka).
Perednis, D., and Gauckler, L. J. (2005). Thin film deposition using spray pyrolysis. J. Electroceramics 14, 103–111. doi: 10.1007/s10832-005-0870-x
Roos, A. (1993). Use of an integrating sphere in solar energy research. Solar Energy Mater. Solar Cells 30, 77–94. doi: 10.1016/0927-0248(93)90033-Y
Rouxel, T., Sellappan, P., Celarie, F., Houizot, P., and Sangleboeuf, J. C. (2014). Toward glasses with better indentation cracking resistance. Cr Mecanique 342, 46–51. doi: 10.1016/j.crme.2013.10.008
Rubin, M. (1985). Optical properties of soda lime silica glasses. Solar Energy Mater. 12, 275–288. doi: 10.1016/0165-1633(85)90052-8
Schröder, A. (1928). XXXV. Beiträge zur Kenntnis des Feinbaues des Brookits und des physikalischen Verhaltens sowie derZustandsänderungen der drei natürlichen Titandioxyde. Z. Kristallograph. 67:485. doi: 10.1524/zkri.1928.67.1.485
Shannon, R. D., Shannon, R. C., Medenbach, O., and Fischer, R. X. (2002). Refractive index and dispersion of fluorides and oxides. J. Phys. Chem. Ref. Data 31, 931–970. doi: 10.1063/1.1497384
Studenikin, S. A., Golego, N., and Cocivera, M. (1998). Fabrication of green and orange photoluminescent, undoped ZnO films using spray pyrolysis. J. Appl. Phys. 84, 2287–2294. doi: 10.1063/1.368295
Sun, R.-D., Nakajima, A., Fujishima, A., Watanabe, T., and Hashimoto, K. (2001). Photoinduced Surface Wettability Conversion of ZnO and TiO2 Thin Films. J. Phys. Chem. B 105, 1984–1990. doi: 10.1021/jp002525j
Sundberg, P., Grund Bäck, L., Orman, R., Booth, J., and Karlsson, S. (2019). Simultaneous chemical vapor deposition and thermal strengthening of glass. Thin Solid Films 669, 487–493. doi: 10.1016/j.tsf.2018.11.028
Tauc, J. (1968). Optical properties and electronic structure of amorphous Ge and Si. Mater. Res. Bull. 3, 37–46. doi: 10.1016/0025-5408(68)90023-8
Villegas, E. A., Aldao, C. M., Savu, R., Ramajo, L. A., and Parra, R. (2018). Effects of grain size on the UV-photoresponse of zinc oxide thin films grown by spray-pyrolysis. Phys. Status Solidi a 215:1800107. doi: 10.1002/pssa.201800107
Volotinen, T. T., Parker, J. M., and Bingham, P. A. (2008). Concentrations and site partitioning of Fe2+ and Fe3+ ions in a soda-lime-silica glass obtained by optical absorbance spectroscopy. Phys. Chem. Glasses Euro. J. Glass Sci. Technol. B 49, 258–270. Available online at: https://www.ingentaconnect.com/content/sgt/ejgst/2008/00000049/00000005/art00004
Watanabe, T., Nakajima, A., Wang, R., Minabe, M., Koizumi, S., Fujishima, A., et al. (1999). Photocatalytic activity and photoinduced hydrophilicity of titanium dioxide coated glass. Thin Solid Films 351, 260–263. doi: 10.1016/S0040-6090(99)00205-9
Wondraczek, L., Mauro, J. C., Eckert, J., Kühn, U., Horbach, J., Deubener, J., et al. (2011). Towards ultrastrong glasses. Adv. Mater. 23, 4578–4586. doi: 10.1002/adma.201102795
Keywords: float glass, thin films, UV protection, photovoltaic modules, cover glass, transparent intelligence, solar energy materials, photoluminescence
Citation: Johansson W, Peralta A, Jonson B, Anand S, Österlund L and Karlsson S (2019) Transparent TiO2 and ZnO Thin Films on Glass for UV Protection of PV Modules. Front. Mater. 6:259. doi: 10.3389/fmats.2019.00259
Received: 30 November 2018; Accepted: 30 September 2019;
Published: 18 October 2019.
Edited by:
Lothar Wondraczek, Friedrich Schiller University Jena, GermanyReviewed by:
Martin Charles Wilding, Sheffield Hallam University, United KingdomCopyright © 2019 Johansson, Peralta, Jonson, Anand, Österlund and Karlsson. This is an open-access article distributed under the terms of the Creative Commons Attribution License (CC BY). The use, distribution or reproduction in other forums is permitted, provided the original author(s) and the copyright owner(s) are credited and that the original publication in this journal is cited, in accordance with accepted academic practice. No use, distribution or reproduction is permitted which does not comply with these terms.
*Correspondence: Stefan Karlsson, c3RlZmFuLmthcmxzc29uQHJpLnNl
Disclaimer: All claims expressed in this article are solely those of the authors and do not necessarily represent those of their affiliated organizations, or those of the publisher, the editors and the reviewers. Any product that may be evaluated in this article or claim that may be made by its manufacturer is not guaranteed or endorsed by the publisher.
Research integrity at Frontiers
Learn more about the work of our research integrity team to safeguard the quality of each article we publish.