- 1College of Engineering and Technology, Southwest University, Chongqing, China
- 2Electrical and Computer Engineering Department, Wayne State University, Detroit, MI, United States
- 3College of Materials Science and Engineering, Chongqing University, Chongqing, China
In this work, Cr2O3 nanoparticles, and SnO2 nanofibers were fabricated by a sol–gel process and an electrospinning method, respectively. Gas sensitive materials with high sensitivity to C2H2 gas were obtained by coating Cr2O3 nanoparticles on SnO2 nanofibers. The prepared Cr2O3 nanoparticle-coated SnO2 nanofibers (Cr2O3 NPs. coated SnO2 NFs.) were characterized by X-ray diffraction (XRD), scanning electron microscope (SEM), X-ray energy dispersive spectroscopy (EDS), X-ray photoelectron spectroscopy (XPS), and the gas sensing behaviors to C2H2 were studied. The Cr2O3 NPs. coated SnO2 NFs. exhibited low optimal operating temperature, high sensing response, excellent response-recovery time, and long-term stability to C2H2. The optimal operating temperature of the measured material to 20 ppm C2H2 was about 220°C and the C2H2 concentration had a good linear relationship with the response value when the concentration was 60 ppm. In addition, a reasonable gas sensing mechanism was proposed which may enhance the gas sensing performances for the Cr2O3 NPs. coated SnO2 NFs. to C2H2.
Introduction
Metal oxide semiconductors have important practical significance in gas sensing, mainly because of good chemical reliability, real-time monitoring, and easy fabrication (Zhou et al., 2018b; Wei et al., 2019b). The basis of functional materials, including ZnO (Wang et al., 2019; Yoo et al., 2019), TiO2 (Crişan et al., 2018; Meng et al., 2019), In2O3 (Liu et al., 2018; Inyawilert et al., 2019), SnO2 (Zhang et al., 2018; Zhou et al., 2018c; Li et al., 2019a), have been applied in gas sensing in the past for a long time. Among them, SnO2 is one of the earliest metal oxides, due to its wide band gap, excellent physicochemical properties, and low-price for gas sensing (Uddin and Chung, 2015; Thanihaichelvan et al., 2019). It can easily generate oxygen vacancies on the surface, leading to high specific surface area, and excellent sensing properties (Zhang et al., 2017; Ren et al., 2019). For SnO2, various morphologies of nanostructures have been reported, for instance, zero dimensional nanoparticles (Ahmed et al., 2019), one dimensional nanorods (Zhou et al., 2016), nanowires (Tonezzer, 2019), and nanofibers (Mudra et al., 2019), and two dimensional nanosheets (Chang et al., 2019). Among them, one dimensional nanostructures have been rapidly developed due to their surprising properties such as easy control dimension, large surface area to volume ratio, and excellent mechanical performance (Wang et al., 2017b; Bai et al., 2018).
In order to improve the sensitivity of pure SnO2 nanofibers for gas detection more effectively, several approaches have been studied such as the addition of catalysts, doping metals, and metal oxides (Lu et al., 2018; Zhou et al., 2018d; Zheng et al., 2019). Various studies on gas sensing properties of SnO2 nanofibers doped or coated with metal or metal oxide have been reported so far. Qi et al. reported Sm2O3-doped SnO2 showed high sensitivity under various humidity conditions to C2H2 (Qi et al., 2008). Li et al. confirmed the sensor made of La3+ doped SnO2 nanofibers could rapidly correspond to hydrogen, with good selectivity, and long-range linear response (Li et al., 2019b). Chromium is a very interesting dopant because of its unique catalytic properties and several stable valence states. In addition, it can be used to adjust the surface states, energy band gap and carrier transport characteristics of semiconductors. Gönüllü et al. investigated Cr-doped TiO2 as a high-temperature NO2 gas sensor (Gönüllü et al., 2015). Wang et al. demonstrated that the response value of Cr2O3/ZnO nanofibers was 3.6 to 1 ppm ethanol vapor at 300°C (Wang et al., 2010). Park et al. synthesized In2O3 nanorods decorated with Cr2O3-nanoparticles which showed excellent sensing properties to ethanol than other gases (Park et al., 2015). However, there are few reports on Synthesis of Cr2O3 nanoparticle-coated SnO2 nanofibers and its C2H2 sensing properties.
In the paper, we reported the synthesis of Cr2O3 nanoparticle-coated SnO2 nanofibers (Cr2O3 NPs. coated SnO2 NFs.) by a sol–gel method and electrospinning technique. The prepared Cr2O3 NPs. coated SnO2 NFs. were characterized by X-ray diffraction (XRD), scanning electron microscope (SEM), X-ray energy dispersive spectroscopy (EDS), X-ray photoelectron spectroscopy (XPS) and their C2H2 sensing properties were studied. Benefiting from the coating of Cr2O3, the SnO2 nanofibers exhibited good sensitivity to C2H2 gas. In addition, a plausible gas sensing mechanism was proposed which may enhance the gas sensing performances of C2H2.
Experimental Design, Materials, and Methods
Sample Synthesis
Cr2O3 nanoparticles (NPs.) were prepared by a sol-gel method (Puerari et al., 2016). Firstly, 5.0g Cr(NO3)3.9H2O and 1.5 g NaOH were added to 100 ml distilled water for 30 min with stirring to obtain the precursor Cr(OH)3. The Cr(OH)3 was centrifuged at 5,000 rpm and washed several times with distilled water. It was then dried in an oven at 90C for 24 h. 0.05 g of Cr(OH)3 powders were mixed with 10 g of deionized water and heated to 60°C and then stirred for 2 h to obtain a homogeneous sol solution.
SnO2 nanofibers (NFs.) were synthesized by electrospinning method (Yang et al., 2018). Firstly, 6 mL N, N-dimethylformamide (DMF) and 1.2 g SnC2H2O were put into beaker1 and stirred for 2 h with a magnetic stirrer until it became a clarifying solution and set aside. 1 g PVP and 6 mL absolute ethanol were put in beaker2 and stirred with magnetic stirrer at uniform speed until PVP dissolved completely. The solution in beaker2 was added to the solution in beaker1 drop by drop with dropper, and the mixture was obtained as the precursor solution by stirring for 3 h at room temperature. The obtained spinning solution was conveyed to a hypodermic syringe at a constant flow rate, and then 20 kV voltage was applied to electrospinning at the electrode distance of 25 cm. A piece of aluminum foil was used as the cathode, and several sensor substrates were placed on it. Sensor substrates were prepared on SiO2/Si chips by radio frequency sputtering platinum arrays as signal electrodes (Qi et al., 2014). The thickness of the SiO2 layer and the platinum array are about 300 and 100 nm, respectively. The precursor solution was directly electrospun on sensor substrates with arrays of interdigitated platinum electrodes. After 2 h of electrospinning, the substrates were calcined in air at 500°C for 2 h, and then impregnated with the sol solution. The substrates were dried on a gently heated hot plate before the next step after each step. Subsequently, the sensors were calcined in air at 600°C for 1 h, and then annealed in hydrogen atmosphere at 300°C for 10 min. Finally, the Cr2O3 NPs. coated SnO2 NFs. sensors were obtained.
Sample Characterization
In this paper, The X-ray diffractometer (D/Max-1200, Rigaku, Japan) was used to recorded XRD patterns at room temperature. The SEM and EDS images were gained by using Field Emission Electron Microscope (Hitachi S-4800, Marco Polo Shanghai Yongming Automation Equipment Co., Ltd., Shanghai, China) and Oxford INCA 250 EDS detector (JSM-6700F, Japan), respectively. The XPS spectra were determined on an X-ray photoelectron spectrometer (KRATOS XSAM800, Kratos, Kingdom).
The gas sensing properties of the obtained Cr2O3 NPs. coated SnO2 NFs. were performed with the CGS-1TP intelligent gas sensitive analysis system (Beijing Elite Tech Co., Ltd, Beijing, China). The structure of planar gas sensor was shown in Figure 1. The gas sensing experiments were tested under laboratory conditions at the room temperature of 25°C and relative humidity of 50%. The gas response of the sensor (R) is defined as R = Rg/Ra (Du et al., 2018), where Rg and Ra are the resistance values of the sensor in the air and the gas to be measured, respectively. The time required for the sensor resistance to change from Ra to Ra-90% × (Ra-Rg) is defined as the response time when the target gas is introduced into the sensor, and the time from Rg to Rg+90% × (Ra-Rg) is defined as recovery time when the target gas is replaced by air (Choi et al., 2019).
Results
Materials Characterization
Figure 2 demonstrated the XRD patterns of the prepared SnO2 nanofiber sample with Cr2O3. The prominent peaks corresponding to (110), (101), and (211) crystal lattice planes and other smaller peaks showed no difference from the corresponding peaks of the SnO2 rutile structure given in the standard data file (JCPDS File no. 41-1445). The diffraction peaks were observed at 34.7°, 37.8°, 54.2°, where the inconspicuous Cr2O3 peaks were observed, indicating that Cr2O3 successfully coated the SnO2 sample.
The morphologies of the pure SnO2 and Cr2O3 NPs. coated SnO2 NFs. were examined by SEM and the representative images are shown in Figure 3. The prepared samples were composed of a plurality of SnO2 nanofibers as shown in Figure 3A. The SnO2 nanofibers were uniform in size and irregular arranged. After the synthesis process, the Cr2O3 nanoparticles were tightly coated on the surface of SnO2 nanofibers. The surface of the nanofibers was uneven, long and continuous, without adhesion, intertwined into a network. Furthermore, it was observed from the photomicrograph that the prepared fibrous SnO2 samples had a porous structure which was of benefit to subsequent gas sensitivity testing.
XPS is a kind of useful technique for studying the chemical state of the elements and the surface composition in the sample. Figure 4A showed the XPS spectrum of the Cr2O3 NPs. coated SnO2 NFs. gas sensing material, and the chemical states of various elements in the sample were obtained. The C, Sn, Cr, and O elements appeared in the broad spectrum of the sample. The characteristic energy spectrum reflected that the Cr element had been successfully coated on the surface of SnO2, which was consistent with the XRD pattern. At the same time, there was no impurity doping into SnO2. Figure 4B showed the XPS spectrum of Cr 2p. The doublet peaks located at binding energies of 577.5 and 588.4 eV, which is close to the trivalent Cr ion in the standard XPS data, indicating Cr element was in the state of trivalent Cr ions.
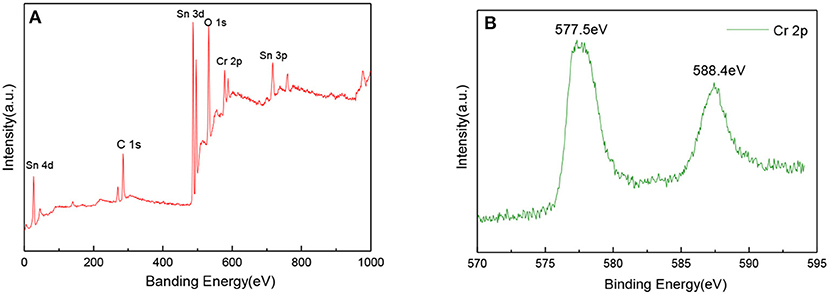
Figure 4. (A) The XPS spectrum of the Cr2O3 NPs. coated SnO2 NFs. sample, and (B) XPS spectrum of Cr 2p.
An EDS measurement was performed to study the component of the sample. Corresponding EDS spectra from the prepared Cr2O3 NPs. coated SnO2 NFs. sample is shown in Figure 5, which confirmed the presence of Sn, Cr, O, in the sample. So, it showed that the sample was composed of Cr2O3 and SnO2 clearly.
Sensing Performances
In order to find out whether the coating of Cr2O3 has a positive effect on the detection of acetylene gas by pure SnO2, the gas sensing properties of pure SnO2 and Cr2O3 NPs. coated SnO2 NFs. for C2H2 gas were tested. As we know, the operating temperature is one of important factors that determine the gas sensitivity of materials. Figure 6A shows the response of pure SnO2 and Cr2O3 NPs. coated SnO2 NFs. to 20 ppm C2H2 at different temperatures to explore the relationship between temperature and gas response as well as the optimal operating temperature in the range of 60–440°C. It found that the response of the samples showed the trend of increasing first and then decreasing. The response increased in the range of 60–250°C and reached the highest point at 250°C then decreased in the range of 250–440°C for pure SnO2. The response increased in the range of 60–220°C and reached the highest point at 220°C then decreased in the range of 220–440°C for Cr2O3 NPs. coated SnO2 NFs. The response values of gas sensor based on pure SnO2 and Cr2O3 NPs. coated SnO2 NFs. for 20 ppm C2H2 gas at optimum operating temperature were 17.12 and 48.54, respectively. Obviously, Cr2O3 NPs. coated SnO2 NFs. exhibited excellent temperature characteristics with the lower optimal operating temperature and higher response value, indicating that the coating of Cr2O3 had a positive effect on the measurement of C2H2, and the optimum operating temperature was effectively reduced.
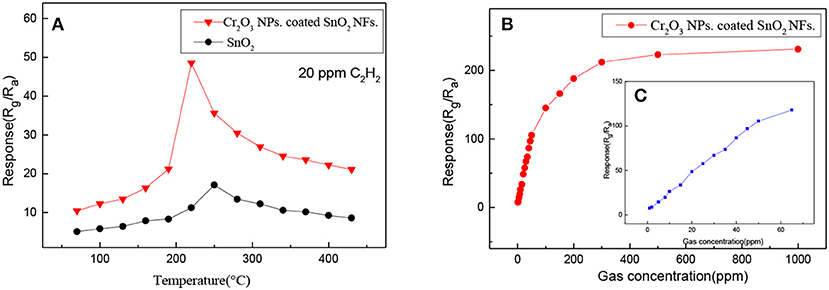
Figure 6. (A) The gas response of the pure SnO2 and Cr2O3 NPs. coated SnO2 NFs. toward 20 ppm of C2H2 at different working temperatures (60–440°C); (B) the gas response of the Cr2O3 NPs. coated SnO2 NFs. to different concentrations (0–1,000 ppm) C2H2 at 220°C and (C) the gas response of the Cr2O3 NPs. coated SnO2 NFs. in the range from 0 to 80 ppm C2H2 at 220°C.
Figure 6B revealed the response of Cr2O3 NPs. coated SnO2 NFs. to the concentrations of C2H2 in the range of 0 to 1,000 ppm at 220°C. The measured results in Figure 6C showed that the gas responses of Cr2O3 NPs. coated SnO2 NFs. increased in a good linear relationship with the concentrations of C2H2 in the range from 0 to 60 ppm. Moreover, when the gas concentrations exceeded 200 ppm, the response increased slowly, indicating that the response gradually became saturated.
Quick response and recovery plays an important role for a gas sensing material. The dynamic response-recovery curve of the Cr2O3 NPs. coated SnO2 NFs. sensors for 1, 5, 10, 15, and 20 ppm C2H2 gas at an optimum operating temperature of 220°C was tested and shown in Figure 7A. When the C2H2 concentration was increased from 1 to 20 ppm, the response time values of the prepared gas sensor were 9, 12, 15, 17, and 20 s, and the recovery time values were 11, 14, 18, 19, and 23 s. The Cr2O3 NPs. coated SnO2 NFs. responded rapidly and could recover to its initial value when they were exposed to the air again. It showed that the sensors had good response recovery characteristics for different concentrations of C2H2 gas, indicating an excellent persistence and stability of C2H2 gas.
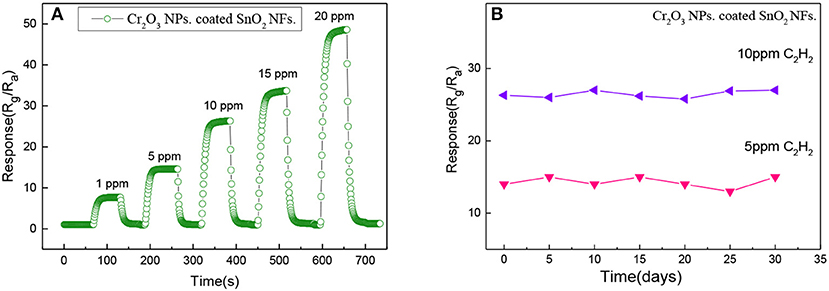
Figure 7. (A) The dynamic response-recovery characteristic of Cr2O3 NPs. coated SnO2 NFs. sample at different concentrations (1, 5, 10, 15, 20 ppm) at optimal operating temperature and (B) the long-term stability of Cr2O3 NPs. coated SnO2 NFs to 5 and 10 ppm C2H2 at 220°C.
For the long-term perspective of practicality, in order to ensure the correctness of the test results, gas sensing materials should keep good stability. Therefore, the long-term stability of Cr2O3 NPs. coated SnO2 NFs. to 5 and 10 ppm C2H2 were tested at 220°C during 30 days to ensure the reliability, as shown in Figure 7B. Even if the response values changed every day, when the gas concentrations were 5 and 10 ppm, the response values only just fluctuated around 14.5 and 26.5, respectively. So, the Cr2O3 NPs. coated SnO2 NFs. had prominent stability.
The gas-sensing characteristics of C2H2 based sensors have been discussed and compared with other metal oxides material gas sensors shown in Table 1. From these reports, gas sensors based on Cr2O3 NPs. coated SnO2 NFs. exhibit lower working temperatures and higher response values compared with most other sensors for C2H2 gas. The obtained results indicate that the Cr2O3 NPs. coated SnO2 NFs sensor is promising for C2H2 gas sensing.
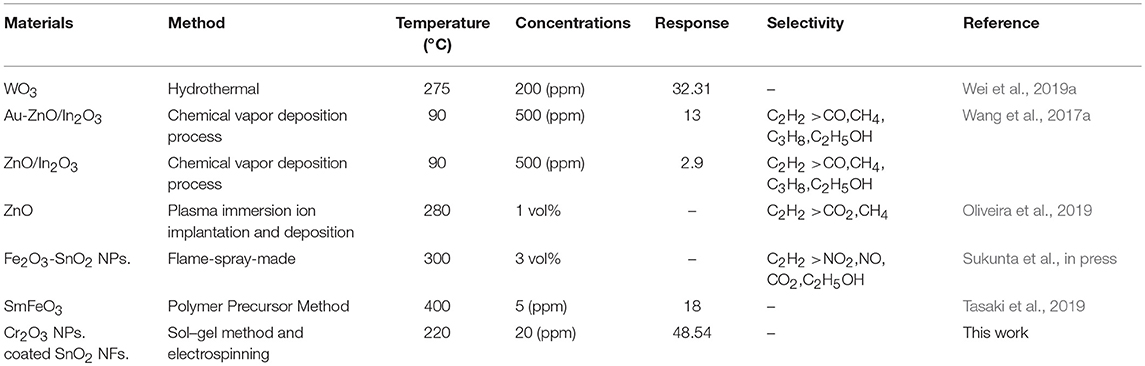
Table 1. The gas-sensing characteristics of C2H2 sensors based on different metal oxides synthesized by various methods.
SnO2 is a wide-bandgap semiconductor whose gas-sensing properties is the change in resistance caused by the adsorption and desorption of surface electrons and gas molecules. The gas sensing mechanism of pure SnO2 NFs. and Cr2O3 NPs. coated SnO2 NFs. was shown in Figure 8. When the sample exposed to the air, the resistant of the sensor was decided by the quantity of chemisorbed oxygen species. In the air, the oxygen would be chemically adsorbed on the surface of SnO2 and electrons were obtained from conduction band of SnO2, leading to the formation of ionic species such as O−, O2−, and . When the SnO2 NFs. was exposed to the atmosphere of C2H2, C2H2 gas had many opportunities for absorption and desorption on the surface. Reactions could be induced after coming into contact with acetylene molecules and then being oxidized with ionic oxygen species to produce H2O and CO2. Thus, the desorbed oxygen species would set free electrons return into the conduction band of SnO2, causing a receded depletion zone, decreasing the resistance, and increasing conductivity shown in Figure 8A.
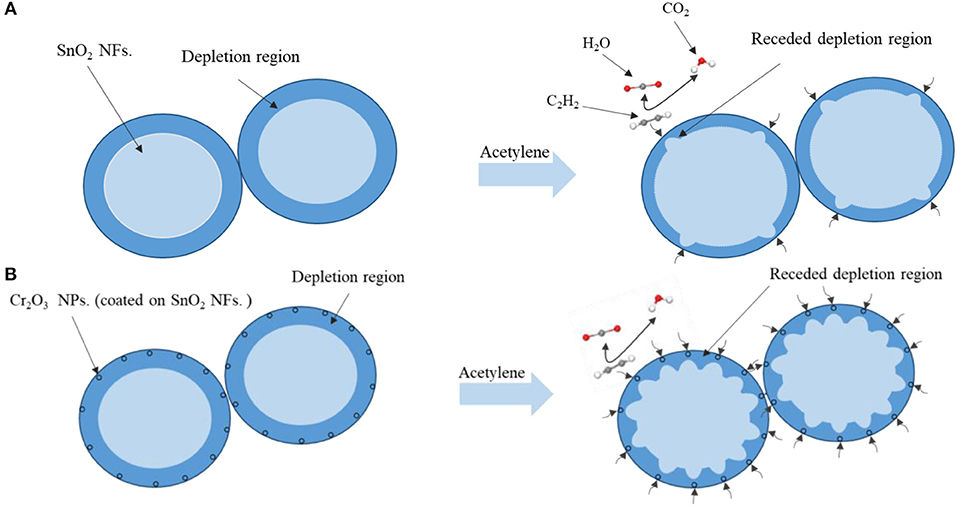
Figure 8. The mechanism diagram of C2H2 sensing process of (A) pure SnO2 and (B) Cr2O3 nanoparticle-coated SnO2 nanofibers.
It is well-known that the ability of chemisorbed oxygen is decided by the specific surface area of the materials and the operating temperature. The surface area of Cr2O3 NPs. coated SnO2 NFs. was large, as shown in Figure 3, which indicated that the adsorption capability of Cr2O3 NPs. coated SnO2 NFs. had been enormously enhanced. Cr2O3 coating provided an effective means with improving the electronic and catalytic properties for gas interaction at the interface. The concentration of oxygen vacancies was greatly increased. The oxygen vacancies could capture ion-adsorbed oxygen in the atmosphere, which facilitated gas sensing reaction. Meanwhile, Cr2O3 coating increased the specific surface area, provided more gas and oxygen adsorption sites, improved the conductivity of SnO2, and contributed to oxygen adsorption (Li et al., 2018; Zhou et al., 2018a) as shown in Figure 8B. Accordingly, the speed at which the reaction occured was accelerated. The adsorption rate of C2H2 to SnO2 increased with Cr2O3 coating, which indicated that Cr2O3 could improve the sensitivity of SnO2 to C2H2 gas.
Conclusions
The Cr2O3 NPs. coated SnO2 NFs. were successfully prepared via a sol-gel process and electrospinning method. The gas detection results showed that the prepared sensor was sensitive to C2H2, and the optimum temperature was about 220°C which was lower than the optimum temperature of pure SnO2 nanofibers. C2H2 gas had a higher response value, and the C2H2 concentration had a good linear relationship with the response value when the concentration was <60 ppm. Moreover, the Cr2O3 NPs. coated SnO2 NFs. had good repeatability and long-term stability. The excellent gas sensing performance of Cr2O3 NPs. coated SnO2 NFs. could be owing to the increase of oxygen vacancies of SnO2 nanofibers by Cr2O3 coating and the large specific surface of the sample. The results certify that the Cr2O3 NPs. coated SnO2 NFs. material is a potential candidate for the detection of acetylene.
Data Availability
All datasets generated for this study are included in the manuscript/supplementary files.
Author Contributions
XG and ZL performed the experiments and analyzed the data with the help from LX and QiZ. XG, QuZ, and WZ wrote and revised the manuscript with input from all authors. All authors read and approved the manuscript.
Conflict of Interest Statement
The authors declare that the research was conducted in the absence of any commercial or financial relationships that could be construed as a potential conflict of interest.
Acknowledgments
This work has been supported in part by the National Natural Science Foundation of China (No. 51507144), Fundamental Research Funds for the Central Universities (No. XDJK2019B021), the artificial intelligence key project of Chongqing (No. cstc2017rgzn-zdyfX0030), the Chongqing Science and Technology Commission (CSTC) (No. cstc2016jcyjA0400), and the project of China Scholarship Council (CSC).
References
Ahmed, A., Siddique, M. N., Ali, T., and Tripathi, P. (2019). Defect assisted improved room temperature ferromagnetism in Ce doped SnO2 nanoparticles. Appl. Surf. Sci. 483, 463–471. doi: 10.1016/j.apsusc.2019.03.209
Bai, S. L., Fu, H., Zhao, Y. Y., Tian, K., and Chen, A. F. (2018). On the construction of hollow nanofibers of ZnO-SnO2 heterojunctions to enhance the NO2 sensing properties. Sens. Actuators B 266, 692–702. doi: 10.1016/j.snb.2018.03.055
Chang, L. M., Yi, Z., Wang, Z. M., Wang, L. M., and Cheng, Y. (2019). Ultrathin SnO2 nanosheets anchored on graphene with improved electrochemical kinetics for reversible lithium and sodium storage. Appl. Surf. Sci. 484, 646–654. doi: 10.1016/j.apsusc.2019.04.144
Choi, M. S., Mirzaei, A., Bang, J. H., Oum, W., Kwon, Y. J., Kim, J.-H., et al. (2019). Selective H2S-sensing performance of Si nanowires through the formation of ZnO shells with Au functionalization. Sens. Actuators B Chem. 289, 1–14. doi: 10.1016/j.snb.2019.03.047
Crişan, M., Mardare, D., Ianculescu, A., Drăgan, N., and Vasile, B. (2018). Iron doped TiO2 films and their photoactivity in nitrobenzene removal from water. Appl. Surf. Sci. 455, 201–215. doi: 10.1016/j.apsusc.2018.05.124
Du, Q., Wang, L., Yang, J., Liu, J. F., Yuan, Y. K., Wang, M. Z., et al. (2018). Enhancing gas sensing performances and sensing mechanism at atomic and molecule level of WO3 nanoparticles by hydrogenation. Sens. Actuators B Chem. 273, 1786–1793. doi: 10.1016/j.snb.2018.07.099
Gönüllü, Y., Haidry, A. A., and Saruhan, B. (2015). Nanotubular Cr-doped TiO2 for use as high-temperature NO2 gas sensor, Sens. Actuators B Chem. 217, 78–87. doi: 10.1016/j.snb.2014.11.065
Inyawilert, K., Wisitsoraat, A., Liewhiran, C., Tuantranont, A., and Phanichphant, S. (2019). H2 gas sensor based on PdOx-doped In2O3 nanoparticles synthesized by flame spray pyrolysis. Appl. Surf. Sci. 475, 191–203. doi: 10.1016/j.apsusc.2018.12.274
Li, F., Ruan, S. P., Zhang, N., Yin, Y. Y., Guo, S. J., Chen, Y., et al. (2018). Synthesis and characterization of Cr-doped WO3 nanofibers for conductometric sensors with high xylene sensitivity. Sens. Actuators B Chem. 265, 355–364. doi: 10.1016/j.snb.2018.03.054
Li, H., Zhang, B., Wang, X., Zhang, J., An, T. H., Ding, Z. Y., et al. (2019a). Heterostructured SnO2-SnS2@C embedded in nitrogen-doped graphene as a robust anode material for lithium-ion batteries. Front. Chem. 7:339. doi: 10.3389/fchem.2019.00339
Li, Z. Y., Yang, Q. B., Wu, Y. P., He, Y., and Wang, J. F. (2019b). La3+ doped SnO2 nanofibers for rapid and selective H2 sensor with long range linearity. Int. J. Hydrogen Energy 44, 8659–8668. doi: 10.1016/j.ijhydene.2019.02.050
Liu, X. J., Tian, X. Y., Jiang, X. M., Jiang, L., and Xu, X. J. (2018). Facile preparation of hierarchical Sb-doped In2O3 microstructures for acetone detection. Sens. Actuators B Chem. 270, 304–311. doi: 10.1016/j.snb.2018.05.046
Lu, Z., Zhou, Q., Wang, C., Wei, Z., Xu, L., and Gui, Y. (2018). Electrospun ZnO–SnO2 composite nanofibers and enhanced sensing properties to SF6 decomposition byproduct H2S. Front. Chem. 6:540. doi: 10.3389/fchem.2018.00540
Meng, L. J., Wang, Z. H., Yang, L., Ren, W. J., and Santos, M. P. (2019). A detailed study on the Fe-doped TiO2 thin films induced by pulsed laser deposition route. Appl. Surf. Sci. 474, 211–217. doi: 10.1016/j.apsusc.2018.03.043
Mudra, E., Shepa, I., Milkovic, O., Dankova, Z., Kovalcikova, A., Annušová, A., et al. (2019). Effect of iron doping on the properties of SnO2 nano/microfibers. Appl. Surf. Sci. 480, 876–881. doi: 10.1016/j.apsusc.2019.03.041
Oliveira, R. M., Vieira, M. S., and Silva, N. F. (2019). Enhancement of acetylene gas sensing properties for a ZnO-based gas sensor produced by plasma immersion ion implantation and deposition. Mater. Sci. Semicond. Process. 93, 339–344. doi: 10.1016/j.mssp.2018.12.031
Park, S., Kim, S., Sun, G.-J., Choi, S., Lee, S., and Lee, C. (2015). Ethanol sensing properties of networked In2O3 nanorods decorated with Cr2O3-nanoparticles. Ceram. Int. 41, 9823–9827. doi: 10.1016/j.ceramint.2015.04.055
Puerari, R. C., Costa, C. H., Vicentini, D. S., Fuzinatto, C. F., Melegari, S. P., Schmidt, É. C., et al. (2016). Synthesis, characterization and toxicological evaluation of Cr2O3 nanoparticles using Daphnia magna and Aliivibrio fischeri. Ecotoxicol. Environ. Saf. 128, 36–43. doi: 10.1016/j.ecoenv.2016.02.011
Qi, Q., Zhang, T., Zheng, X. J., Fan, H. T., and Zeng, Y. (2008). Electrical response of Sm2O3-doped SnO2 to C2H2 and effect of humidity interference. Sens. Actuators B Chem. 134, 36–42. doi: 10.1016/j.snb.2008.04.011
Qi, Q., Zhao, J., Xuan, R. F., Wang, P. P., and Li, G. D. (2014). Sensitive ethanol sensors fabricated from p-type La0.7Sr0.3FeO3 nanoparticles and n-type SnO2 nanofibers. Sens. Actuators B Chem. 191, 659–665. doi: 10.1016/j.snb.2013.10.035
Ren, L., Yao, Y., Wang, K. Y., Li, S. T., Zhu, K. J., and Liu, J. (2019). Novel one-step in situ growth of SnO2 quantum dots on reduced graphene oxide and its application for lithium ion batteries. J. Solid State Chem. 273, 128–131. doi: 10.1016/j.jssc.2019.01.028
Sukunta, J., Wisitsoraat, A., Tuantranont, A., Jaruwongrungsee, K., Phanichphant, S., and Liewhiran, C. (in press). Mechanistic roles of substitutional Fe dopants on catalytic acetylene-sensing process of flame-made SnO2 nanoparticles. Arab. J. Chem. doi: 10.1016/j.arabjc.2018.08.013
Tasaki, T., Takase, S., and Shimizu, Y. (2019). Improvement of sensing performance of impedancemetric C2H2 sensor using SmFeO3 thin-films prepared by a polymer precursor method. Sensors 19:E773. doi: 10.3390/s19040773
Thanihaichelvan, T., Thanihaichelvan, M., Haseeb, M. A. S. M. A, and Akbar, S. A. (2019). Highly sensitive and selective ethanol sensor based on ZnO nanorod on SnO2 thin film fabricated by spray pyrolysis. Front. Chem. 6:122. doi: 10.3389/fmats.2019.00122
Tonezzer, M. (2019). Selective gas sensor based on one single SnO2 nanowire. Sens. Actuators B Chem. 288, 53–59. doi: 10.1016/j.snb.2019.02.096
Uddin, A. S. M. I., and Chung, G. S. (2015). Fabrication and characterization of C2H2 gas sensor based on Ag-loaded vertical ZnO nanowires array. Proced. Eng. 120, 582–585. doi: 10.1016/j.proeng.2015.08.730
Wang, B., Jin, H. T., Zheng, Z. Q., Zhou, Y. H., and Gao, C. (2017a). Low-temperature and highly sensitive C2H2 sensor based on Au decorated ZnO/In2O3 belt-tooth shape nano-heterostructures. Sens. Actuators B Chem. 244, 344–356. doi: 10.1016/j.snb.2016.12.044
Wang, D., Zhang, M. L., Chen, Z. L., Li, H. J., and Yang, J. H. (2017b). Enhanced formaldehyde sensing properties of hollow SnO2 nanofibers by graphene oxide. Sens. Actuators B Chem. 250, 533–542. doi: 10.1016/j.snb.2017.04.164
Wang, J. X., Zhou, Q., and Zeng, W. (2019). Competitive adsorption of SF6 decompositions on Ni-doped ZnO (100) surface: Computational and experimental study. Appl. Surf. Sci. 479, 185–197. doi: 10.1016/j.apsusc.2019.01.255
Wang, W., Li, Z. Y., Zheng, W., Huang, H. M., and Sun, J. H. (2010). Cr2O3-sensitized ZnO electrospun nanofibers based ethanol detectors. Sens. Actuators B Chem. 143, 754–758. doi: 10.1016/j.snb.2009.10.016
Wei, Z. J., Zhou, Q., Lu, Z. R., Xu, L. N., Gui, Y. G., and Tang, C. (2019a). Morphology controllable synthesis of hierarchical WO3 nanostructures and C2H2 sensing properties. Phys. E 109, 253–260. doi: 10.1016/j.physe.2019.01.006
Wei, Z. J., Zhou, Q., Wang, J. X., Gui, Y. G., and Zeng, W. (2019b). A novel porous NiO nanosheet and its H2 sensing performance. Mater. Lett. 245, 166–169. doi: 10.1016/j.matlet.2019.03.013
Yang, Y., Zhang, Z. J., He, Y. L., Wang, Z. H., Zhao, Y. B., and Sun, L. (2018). Fabrication of Ag@TiO2 electrospinning nanofibrous felts as SERS substrate for direct and sensitive bacterial detection. Sens. Actuators B Chem. 273, 600–609. doi: 10.1016/j.snb.2018.05.129
Yoo, R., Güntner, A. T., Park, Y. J., Rim, H. J., Lee, H.-S., and Lee, W. (2019). Sensing of acetone by Al-doped ZnO. Sens. Actuators B Chem. 283, 107–115. doi: 10.1016/j.snb.2018.12.001
Zhang, Q., Zhou, Q., Lu, Z., Wei, Z., Xu, L., and Gui, Y. (2018). Recent advances of SnO2-based sensors for detecting fault characteristic gases extracted from power transformer oil. Front. Chem. 6:364. doi: 10.3389/fchem.2018.00364
Zhang, Q. Y., Zhou, Q., Yin, X. T., Liu, H. C., Xu, L. N., Tan, W. M., et al. (2017). The effect of PMMA pore-forming on hydrogen sensing properties of porous SnO2 thick film sensor. Sci. Adv. Mater. 9, 1350–1355. doi: 10.1166/sam.2017.3111
Zheng, Y. Q., Liu, J. Y., Cheng, B., You, W., Ho, W. K., and Tang, H. (2019). Hierarchical porous Al2O3@ZnO core-shell microfibres with excellent adsorption affinity for Congo red molecule. Appl. Surf. Sci. 473, 251–260. doi: 10.1016/j.apsusc.2018.12.106
Zhou, Q., Chen, W. G., Li, J., Tang, C., and Zhu, S. P. (2016). Synthesis, characterisation and sensing properties of Sm2O3 doped SnO2 nanorods to C2H2 gas extracted from power transformer oil. Mater. Technol. 31, 364–370. doi: 10.1179/1753555715Y.0000000069
Zhou, Q., Chen, W. G., Xu, L. N., Kumarc, R., Gu, Y. G., Zhao, Z. Y., et al. (2018a). Highly sensitive carbon monoxide (CO) gas sensors based on Ni and Zn doped SnO2 nanomaterials. Ceram. Int. 44, 4392–4399. doi: 10.1016/j.ceramint.2017.12.038
Zhou, Q., Lu, Z., Wei, Z., Xu, L., and Gui, Y. (2018b). Hydrothermal synthesis of hierarchical ultrathin NiO nanoflakes for high-performance CH4 sensing. Front. Chem. 6:194. doi: 10.3389/fchem.2018.00194
Zhou, Q., Umar, A., Sodki, E. M., Amine, A., Xu, L. N., Gui, Y. G., et al. (2018c). Fabrication and characterization of highly sensitive and selective sensors based on porous NiO nanodisks. Sens. Actuators B Chem. 259, 604–615. doi: 10.1016/j.snb.2017.12.050
Keywords: Cr2O3 nanoparticles, SnO2 nanofibers, electrospinning, C2H2, sensing properties
Citation: Gao X, Zhou Q, Lu Z, Xu L, Zhang Q and Zeng W (2019) Synthesis of Cr2O3 Nanoparticle-Coated SnO2 Nanofibers and C2H2 Sensing Properties. Front. Mater. 6:163. doi: 10.3389/fmats.2019.00163
Received: 19 May 2019; Accepted: 24 June 2019;
Published: 09 July 2019.
Edited by:
Zhenyu Li, Southwest Petroleum University, ChinaCopyright © 2019 Gao, Zhou, Lu, Xu, Zhang and Zeng. This is an open-access article distributed under the terms of the Creative Commons Attribution License (CC BY). The use, distribution or reproduction in other forums is permitted, provided the original author(s) and the copyright owner(s) are credited and that the original publication in this journal is cited, in accordance with accepted academic practice. No use, distribution or reproduction is permitted which does not comply with these terms.
*Correspondence: Qu Zhou, emhvdXF1JiN4MDAwNDA7c3d1LmVkdS5jbg==