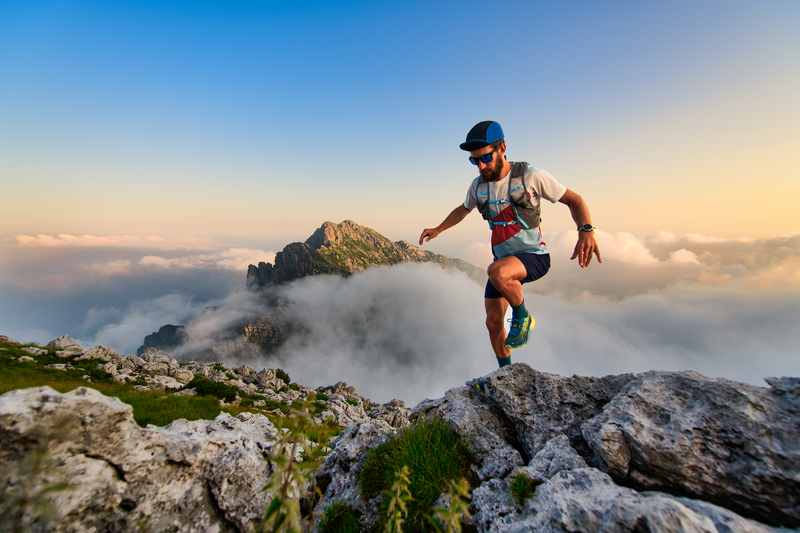
95% of researchers rate our articles as excellent or good
Learn more about the work of our research integrity team to safeguard the quality of each article we publish.
Find out more
REVIEW article
Front. Mater. , 10 June 2019
Sec. Structural Materials
Volume 6 - 2019 | https://doi.org/10.3389/fmats.2019.00126
In this review, we discuss microbiological and molecular concepts of Microbially Induced Calcium Carbonate Precipitation (MICP) and their role in bioconcrete. MICP is a widespread biochemical process in soils, caves, freshwater, marine sediments, and hypersaline habitats. MICP is an outcome of metabolic interactions between diverse microbial communities with organic and/or inorganic compounds present in the environment. Some of the major metabolic processes involved in MICP at different levels are urea hydrolysis, denitrification, dissimilatory sulfate reduction, and photosynthesis. Currently, MICP directed by urea hydrolysis, denitrification, and dissimilatory sulfate reduction has been reported to aid in the development of bioconcrete and has demonstrated an improvement in the mechanical and durability properties of concrete. Bioconcrete is a promising sustainable technology which reduces negative environmental impact caused by CO2 emissions from the construction sector, as well as in terms of economic benefits by way of promoting a self-healing process of concrete structures. Among the metabolic processes mentioned above, urea hydrolysis is the most applied in concrete repair mechanisms. MICP by urea hydrolysis is induced by a series of reactions driven by urease (Ur) and carbonic anhydrase (CA). Catalytic activity of these two enzymes depends on diverse parameters, which are currently being studied under laboratory conditions to better understand the biochemical mechanisms involved and their regulation in microorganisms. It is clearly evident that microbiological and molecular components are essential to improving the process and performance of bioconcrete.
Concrete is the most used construction material due to its resistance, durability and low cost in comparison to other construction materials. Annually, more than 10 billion tons of concrete are used at a global level and experts have predicted that the concrete demand is likely to grow to 16 billion tons in 2050 The current technology employed by the construction industry generates a negative impact on the global environment and economy. The industrial process involved in cement production from lime (precursor of concrete), consumes between 2 and 3% of the global energy demand, generating 0.73–0.99 t CO2/t of cement produced, which accounts for about 8–10% of the global anthropogenic emissions of CO2 and 3.4% of the total CO2 global emissions (Achal et al., 2016; Aprianti, 2017; Miller et al., 2018). Increase in consumption of concrete is a consequence of the susceptibility of infrastructures to physical, chemical and biological factors such as temperature variations, exposure to corrosive and radioactive substances, aggressive gases, natural disasters, and microbial activity (Jroundi et al., 2010; Narayanasamy et al., 2010; Achal et al., 2016; Siddique et al., 2016; Turick and Berry, 2016; Van Tittelboom et al., 2016). These factors cause microcracks formation, which affect mechanical and durability properties of concrete such as compressive strength, flexural strength, and permeability, consequently reducing the useful life of concrete (Achal and Mukherjee, 2015) and increasing the cost of the maintenance and repair of infrastructures. Although the global cost of concrete production ranges between € 60/m3 to € 75/m3, the average cost for crack repair in Europe is about € 130/ m3 (Silva et al., 2015), which reveals the high cost involved in the maintenance and repair of concrete structures. In the last two decades, incorporation of a bacterial metabolic process known as Microbially Induced Calcium Carbonate Precipitation (MICP) has emerged as an alternative method to reduce the cost and environmental impact. Bioconcrete production is based on the addition of bacteria with the ability to induce the formation of minerals (biomineralization) from the cement matrix. This process is facilitated through the ingress of water, CO2 and other chemical substances such as SO4 and , among others (De Muynck et al., 2008; Jonkers et al., 2010; Dhami et al., 2014; Achal and Mukherjee, 2015; Bundur et al., 2015; Verma et al., 2015), aids in self-healing and improves the physical and mechanical properties of the concrete structure. Of late, several reviews have been published on biotechnological aspects of bioconcrete (Zhu and Dittrich, 2016; Joshi et al., 2017; Krajewska, 2018; Jain and Arnepalli, 2019), but microbiological and molecular concepts of MICP and the mechanisms of MICP by different microbial groups have not yet been reported. In this review, types of biomineralization processes, metabolic pathways employed by diverse microbial groups and the genetic factors in relation to urease and carbonic anhydrase activities associated with MICP are discussed.
The biomineralization process consists of the biological synthesis of minerals by microorganisms. In nature, biomineralization processes are widespread in different environments and they involve microorganisms of different taxonomies and with diverse metabolic pathways. Carbonates, phosphates, silicates, sulfates, sulfides, oxides, or hydroxides along with a variety of cations such as Ca2+, Fe, Mg2+, and MnO2 form biominerals through microbial activity. The process also involves organic macromolecules such as proteins, polysaccharides, glycoproteins, and proteoglycans, which function as skeletal support (Fu et al., 2005; Ghosh et al., 2009; Sarayu et al., 2014). Biomineralization is divided into three mechanisms: Biologically Controlled Mineralization (BCM) (Figure 1), Biologically Induced Mineralization (BIM) (Figure 2), and Biologically Mediated Mineralization (BMM) (Figure 3) (Dupraz et al., 2009; Achal et al., 2015). In BCM (Figure 1), the metabolic activity of the microorganism controls nucleation, composition, localization, and the morphology of biominerals. This mechanism could be an extracellular (BCMe) (Figure 1A), intracellular (BCMin) (Figure 1B) and an intercellular (BCMint) process (Figure 1C) with the participation of organic macromolecules exopolysaccharides (EPS) or vesicles. The importance of the regulation of genes on structure, composition, and intrinsic specific morphology of biominerals has also been mentioned previously (Weiner and Dove, 2003).
Figure 1. Schematic representation of Biologically Controlled Mineralization (BCM). (A) BCMe, nucleation of minerals occurs in organic matrix by passive diffusion of cations out of the cell. (B) BCMint, epithelial surfaces of the cells serve as organic substratum and they orient and shape the precipitation of biominerals around the surface (C) BCMin, the biomineral is formed in a specialized vesicle within the cells and subsequently is secreted. Adapted from Weiner and Dove (2003).
Figure 2. Schematic diagram of BIM, precipitation of biominerals is induced due to the interaction between metabolic products of microorganisms and inorganic compounds present in environment. Adapted from Weiner and Dove (2003).
Figure 3. Schematic illustration of BMM. Adapted from Dupraz et al. (2009).
In Biologically Induced Mineralization (BIM), minerals are precipitated indirectly due to the interactions between metabolic byproducts of microorganisms and ions present in the environment (Figure 2). However, the participation of microbial cells in composition, localization, and nucleation of minerals is limited. The minerals generated by BIM are characterized by their wide range in size of particulates, poor crystallinity, and morphology (Weiner and Dove, 2003).
In Biologically Mediated Mineralization (BMM), mineral formation is the result of an interaction between an organic matrix and organic and/or inorganic compounds without the necessity of extracellular or intracellular biological activity (Figure 3). Dupraz et al. (2009) excluded BIM and BMM from the biomineralization process and introduced a term organomineralization process for these mechanisms. The most abundant biominerals and organominerals contain calcium as the principal ion due to its participation in several fundamental processes in the cellular metabolism of organisms. Calcium-bearing minerals comprise 50% of the total of biominerals and organominerals formed and calcium carbonate is one of the most abundant minerals on earth, comprising 4% in weight of the earth's crusts. The precipitation of CaCO3 is a common phenomenon in marine waters, sediments, freshwater, soils and other environments, and this mechanism is known as the microbial precipitation of calcium carbonate (MCP). MCP can occur either actively or passively. BIM (active) known as Microbially Induced CaCO3 Precipitation (MICP) and or passively BMM (passive) occurs through the interaction of the organic matrix (EPS) and calcium ions without the necessity of direct biological activity. In BMM, functional groups such as carboxylic acids (R-COOH), hydroxyl groups (R–OH), amino groups (R–NH2), sulfate- (R–O–SO3H), and sulfhydryl groups (–SH), deprotonate due to an increase in pH, which results in an overall negative charge of extrapolysaccharides produced by the cell which facilitates its binding to metal ions. The role of the organic matrix on the morphology and mineralogy of calcium carbonate crystals (Dupraz et al., 2009; Perito and Mastromei, 2011; Sarayu et al., 2014) is presented in Figure 3.
In MICP, calcium carbonate mineral formation is the result of the interaction among different metabolic byproducts, viz., (HCO3−) and calcium ions present in the microenvironment (Perito and Mastromei, 2011; Achal et al., 2015; Salman et al., 2016). Though, it has been reported that MICP occurs only through extracellular means, several studies showed intracellular precipitation of calcium carbonates in cyanobacteria (Head et al., 2000; Couradeau et al., 2012; Benzerara et al., 2014; Cam et al., 2015). Head et al. (2000) reported that Achromatium oxaliferum precipitates calcite crystals through intracellular means. They observed that more than 70% of the total volume of cells size is occupied by intracellular inclusions of calcium carbonate crystals, surrounded by membranes without vesicles formation. More recently, Xu et al. (2019) reported the calcite and aragonite precipitation through virus induced lysis of cyanobacteria cells and suggested that this new mechanism is expanding the calcium carbonate biomineralization process.
MICP has been reported as part of numerous biotechnological applications such as remediation of soil and water contaminated by heavy metals, metalloids and cations (Ca2+) (Achal et al., 2011; Hamdan et al., 2011; Li et al., 2013; Kang et al., 2014; Chen et al., 2016), in soil bioconsolidation (Whiffin et al., 2007; Cheng and Cord-Ruwisch, 2012), in CO2 bio-sequestration (Yadav et al., 2014; Okyay and Rodrigues, 2015), and in self-healing concrete (bioconcrete) (Achal et al., 2010, 2015; Jonkers et al., 2010; Majumdar et al., 2012; Wang et al., 2014; Bundur et al., 2015; Siddique et al., 2016; Tziviloglou et al., 2016).
Adolphe et al. (1990) are the pioneers of the incorporation of MICP for the restauration of surfaces of ornamental stone. They obtained the patent for the MICP technique known as “bioconcept of calcite or biodeposition,” and this patent was expired in 2010. Nowadays, this biomaterial is known as bioconcrete. Bioconcrete can be defined as the concrete prepared through the addition of bacteria with the capacity for precipitation of calcium carbonate (MICP), and aids in sealing the cracks that appear in it, which is characterized as a self-healing property (Jonkers, 2011). Thus, bioconcrete is considered as one of the most environmentally-friendly and economic technologies since CO2 emissions and the cost of maintenance and repair could be minimized. Bioconcrete has three constituents: microorganisms capable of MICP, nutrients and calcium ions which form cementitious materials (Achal et al., 2015). Bioconcrete is gaining attention due to its self-healing feature and improvement of mechanical and durability properties of concrete structures. Several studies reported self-healing of cracks of (<0.5 mm) by Bacillus cohnii, B. pseudofirmus, B. subtilis, B. alkalinitrilicus, Pseudomonas aeruginosa, and Diaphorobacter nitroreducens (Jonkers et al., 2010; Wang et al., 2012, 2014; Ersan et al., 2015; Khaliq and Ehsan, 2016). Several studies have also been conducted in the restauration of historic monuments such as Thouars church tower (Le Metayer-Levrel et al., 1999; Tiano et al., 1999), Alcázar de Guadalajara (Jroundi et al., 2014), Angera Cathedral (Perito et al., 2014), and Castillo de Chapultepec (Aguilera et al., 2015) using MICP.
In last two decades, there are various studies on the different implementation strategies, to enhance the performance of bioconcrete through the protection of cells such as the addition of nutrient sources and different materials e.g., polyurethane, sol-gel ceramics, calcium sulphoaluminate cement, and magnetic iron oxide nanoparticle (IONS). These methods can benefit the bacteria to survive under harsh concrete matrix conditions such as a high pH (~13.5), limitation of nutrient and water ingress. Bang et al. (2001) incorporated Sporosarcina pasteurii immobilized on polyurethane, which increased compressive strength by 12%. It has also been reported that the addition of lactate in the concrete matrix increases compressive strength in a range of 10–17% over control (Achal et al., 2009; Jonkers and Schlangen, 2009; Mors and Jonkers, 2016). Chen et al. (2016) showed an increment of compressive strength of 56–72% by immobilization of Bacillus mucilaginous L3 and Brewer's yeast JCS 05 C4 on ceramsite with sucrose and yeast extract as the nutrient sources. Wang et al. (2014) employed microcapsules of spores enriched with urea, yeast extract, and calcium nitrate, and observed a 40% decrease in water permeability; however, compressive strength was reduced by 34%. However, (Xu and Wang, 2018) reported that when encapsulating bacteria in low alkali and fast hardening cementitious material, calcium sulphoaluminate cement increased the regain ratio of the compressive strength and water tightness by 84 and 50%, respectively, but the ureolytic activity was found to be lower in encapsulated spores than in the free spores. It can be concluded that the success of a microencapsulation strategy depends on the compatibility of the material used for the capsule as well it's size and distribution through the concrete matrix. Despite the fact that microencapsulation could affect mechanical properties like compressive strength, in some cases it is found to improve water tightness and aids in the activity of bacteria for a long period (120–150 days). Later, Seifan et al. (2018) evaluated the potential of magnetic iron oxide nanoparticles' (IONs) addition in concrete mixtures, on growth and activity of bacterial cells. They observed that while 150 μg/ml of IONs significantly increased the growth of bacteria, 300 μg/ml of IONs achieved the maximum CaCO3 precipitation of 34.54 g/l. More recently, Ruan et al. (2019) reported higher curing of cracks by formation of hydrated magnesium carbonate (>150 μM) in comparison with MICP without addition of IONs. However, the use of these recent strategies involve a high cost, for example €180 for 1 kg of nutrients to generate 13 L of bacterial culture and an equivalent production of 5.4 × 106 units of urease (Achal et al., 2015). Whereas, Silva et al. (2015) reported a total cost of €480 to produce 1 kg of encapsulated bacteria with nutrients.
Earlier, to reduce the costs of nutrients added, Achal et al. (2009, 2010) used industrial waste, such as Lactose Mother Liquor (LML) and Corn Steep Liquor (CSL) and obtained a 17 and 35% increase of compressive strength, respectively. Charpe et al. (2017) observed a 23% increase of compressive strength through the use of lentil seeds as a protein source and sugar as a carbon source and demonstrated a reduction of the cost in comparison with use of peptone as a nutrient source. Later, Fang et al. (2019) obtained 27.8% in compressive strength through the use of Tofu wastewater as a nutrient source. Zhang et al. (2017) demonstrated the potential implementation of activated sludge microbial communities as an alternative source of bacteria and as well as an economic one for self-healing in concrete. They evaluated three microbial consortia under three conditions (aerobic, anaerobic, and facultative anaerobic) and observed that microbial consortia under aerobic conditions showed a more positive effect on inorganic carbon conversion (75.3 ± 3.8%), which was 1.2 ~ 1.8 times more than that of the conditions tested. Adzami et al. (2018) evaluated the large-scale bio-production of calcium carbonate. They reported that agitation speed of 50 rpm and aeration rate of 2.0 vvm recorded the highest specific growth rate (0.141 h−1) of Bacillus sphaericus, which formed CaCO3 crystals with 100% purity.
In the UK, the Resilient Materials for Life project led by Cardiff University in partnership with the universities of Cambridge, Bath and Bradford, have developed four self-healing techniques; the use of microcapsules containing mineral healing agents, bacterial healing, the use of a shape memory polymer based system for crack closure and the delivery of a mineral healing agent through a vascular flow network. They observed that all four techniques showed significant results of accelerated crack healing in the range of 14–28% and the Whole-Life Costing (WLC) analysis indicated a ~12% reduction in the cost of repairs and maintenance (Teall et al., 2016; Al-Tabbaa et al., 2018, 2019; Davies et al., 2018). However, the microencapsulation technique did not show a significant effect on the compressive strength.
Adolphe et al. (1990) were the pioneers in the incorporation of MICP for restauration of surfaces of ornamental stone. They obtained the patent for the MICP technique known as “bioconcept of calcite or biodeposition,” and this patent expired in 2010. Nowadays, this biomaterial is known as bioconcrete. Bioconcrete can be defined as the concrete prepared by addition of bacteria with the capacity for precipitation of calcium carbonate (MICP), which aids in sealing the cracks that form in it, which is characterized as a self-healing property (Jonkers, 2011). Bioconcrete has three constituents: microorganisms capable of MICP, nutrients and calcium ion to form cementitious materials (Achal et al., 2015). Several studies reported autogeneous healing in cracks of (<0.5 mm) by B. cohnii, Bacillus pseudofirmus, Bacillus subtilis, Bacillus alkalinitrilicus, Pseudomonas aeruginosa, and Diaphorobacter nitroreducens (Jonkers et al., 2010; Wang et al., 2012, 2014; Ersan et al., 2015; Khaliq and Ehsan, 2016). In recent years, MICP has gained attention due to its self-healing properties, which is found to benefit the mechanical and durability properties of concrete. Further, it helps in reducing the negative impact on the environment in terms of CO2 emissions by the construction sector and also lowers the cost of repairs of concrete structures in comparison to conventional methods.
Moreover, the potential of a protein known as “Bioremediase,” which promotes biosilicification has been reported. Bioremediase is a silica leaching enzyme, and is 78% similar to bovine carbonic anhydrase II, but does not show carbonic anhydrase activity (Biswas et al., 2010; Majumdar et al., 2012; Sarkar et al., 2014). Biswas et al. (2010) isolated Bioremediase protein from a novel bacterium BKH1 and reported an increase in compressive (>25%) and tensile (>20%) strengths by adding the enzyme powder to the concrete mixture. However, self-healing of bioconcrete is not feasible with the addition of enzyme powder in comparison to the addition of cells which may extend the use and lifetime of bioconcrete by up to 200 years (Jonkers et al., 2010). Bacteria have the ability to resist alkaline conditions by spore formation and reactivate their metabolic activity when water, CO2, SO4, and and others substances ingress, due to the formation of cracks in the structure of concrete (Jonkers et al., 2010; Sarayu et al., 2014). Majumdar et al. (2012) showed 39.4% increment in compressive strength at 28-days cured samples and 42.4% at 120-days with a concentration of 105 cells of Bacterium BKH1. Interestingly, Sarkar et al. (2015) genetically modified a spore forming Bacillus subtilis strain by incorporating the bioremediase like protein gene. They observed that transformed bacterial cells survived for a long time within the cementitious environment and showed a ~16.6% increase in compressive strength and self-healed the cracks by formation of a novel Gehlenite (Ca2Al2SiO7) (~85 nm diameter) along with calcite precipitation inside the mortar matrices. Later, Sarkar et al. (2019) showed an increase of >50% of compressive strength by addition of 104 cells.ml−1 of BKH4, an alkaliphilic bacterium isolated from Bakreshwar hot springs and tolerant to a pH of 12.
A summary of different nutrients used in bioconcrete studies and their effect on bioconcrete characters are presented in Table 1.
Table 1. Nutrients and their effect on compressive strength, flexural strength, water permeability, and self-healing of bioconcrete matrix.
On the other hand, Chaurasia et al. (2019) studied the metabolism of ureolytic Bacillus megaterium, B. pasteurii, and a non-ureolytic Bacillus cohnii in the absence of a substrate, nutrients and their effect on mechanical properties of concrete. They observed that in the absence of nutrient sources, bacteria used an alternate pathway and further, bacteria served as a heterogenous nucleation site, and induced the formation of calcite, and hydrated aluminosilicate phases like beidillite, gismondine. Formation of these crystals increased various mechanical properties of concrete, such as reduction in water absorption (~22%), volume of voids (~24%) and sulfate ion concentration (~26%) at 180 days.
Apart from the advantages discussed above, bioconcrete has also been reported to lower the contribution of carcinogens (one-thirtieth), ecotoxicity (one-tenth), and fossil fuels (six-sevenths) compared to traditional concrete (Gonsalves, 2011). However, the main disadvantages are the generation of ammonia and the possibility of pathogens associated with urea hydrolysis that may harm the health of animals and humans (Burne and Chen, 2000; Backes et al., 2018). It is predicted that real-scale production of bioconcrete based on urea hydrolisis could pollute more than 4.5 × 106 m3 of drinking water and 100 km3 of air. To combat this, Ivanov et al. (2019) propose that MICP technology has to be combined with the proper ventilation of the biogrouting space, proper treatment of MICP effluent, and facilitation for the transformation of the ammonia containing gas to fertilizer. However, this would result in an increase in the cost of bioconcrete. However, alternate metabolic pathways, such as denitrification and sulfate reduction (Alshalif et al., 2016), photosynthesis and methane oxidation (Okwadha and Li, 2011; Ersan et al., 2015; Zhu and Dittrich, 2016) also yielded promising results on MICP, but their environmental effects need to be researched further.
The major microbial metabolic processes involved in MICP are urea hydrolysis, ammonification of amino acids, denitrification, dissimilatory sulfate reduction and photosynthesis (Castanier et al., 1999; Dupraz et al., 2009). Among these metabolic pathways, urea hydrolysis is less complex (Achal et al., 2011) and urea hydrolytic strains showed more higher calcite precipitation (~20–80%) in comparison with other metabolic pathways (Achal et al., 2009, 2010; Wang et al., 2014) and is therefore the most studied MICP process (Achal et al., 2011).
A series of complex reactions in urea hydrolysis are driven by urease (EC 3.5.1.5) and carbonic anhydrase (EC 4.2.1.1) enzymes (Figure 4). One mole of urea is hydrolyzed by urease (UE) to one mole of ammonia and carbamate (Equation 1), and the carbamate is spontaneously hydrolyzed to produce one mole of ammonia and carbonic acid (Equation 2). Carbonic acid is converted to bicarbonate (Equation 3) by carbonic anhydrase (CA) and two moles of ammonium and hydroxide are formed due to ammonia hydrolysis (Equation 4). Consequently, pH is increased around the cell and induces precipitation of calcium carbonate in presence of soluble Ca2+ (Equations 5–7). Under unfavorable conditions, the cell survives by allowing the entry and accumulation of calcium ions, resulting in an excessive expulsion of protons. Subsequently, the cells actively export calcium and compensate the loss of protons. A low concentration of protons and a high concentration of Ca2+ in the microenvironment is required for secretion of carbonate ions while the supersaturation of carbonate induces precipitation of calcium carbonate on the surface of the cell. Exopolymers, biofilms and even inactive spores can provide nucleation sites for the above-mentioned reactions (Van Tittelboom et al., 2010; Kim et al., 2012; Achal et al., 2015; Anbu et al., 2016). Earlier, Park and Hausinger (1995) observed that activities of UE and CA are in synergy due to the incorporation of nickel in the active center of UE, which depends on the regulation of CO2/HCO3, a reaction catalyzed by CA.
With respect to kinetics parameters (Km and Vmax) of UE and CA, a wide range have been reported. Stocks-Fischer et al. (1999) observed that pH influenced UE affinity to the substrate as well as the enzyme activity of Sporosarcina pasteurii. UE activity was found to be decreased at pH 7.0 and with Km and Vmax values of 41.6 mM and 3.55 mM min−1 mg−1 of protein, respectively. However, the affinity improved with pH increase, with a Km of 26.2 mM and Vmax of 1.72 mM min−1 mg−1 at pH of 8.3–9.0. Bachmeier et al. (2002) compared the urease activity of free and immobilized, recombinant Escherichia coli (HB101). Free suspended bacteria recorded a Km and Vmax of 17.3 mM and of 1.57mM min−1 mg−1, respectively. On the other hand, polyurethane immobilized UE showed a Km of 22.9 mM and Vmax of 0.73 mM min−1 mg−1, which indicated that immobilized UE requires higher substrate concentrations than free cells. This suggests that bacteria in bioconcrete conditions will be under stress because of a fault in adequate substrate concentrations for their activity. Similarly, Yadav et al. (2011) reported that CA activity was five times lower in immobilized Single Enzyme Nanoparticles (SENs) than free CA activity. They observed that SENs showed a limitation of substrate transfer due to the presence of a hybrid layer between each carbonic anhydrase molecule and biopolymeric silica network. Achal et al. (2011) characterized UE and CA from Bacillus megaterium, and confirmed the role of CA on UE activity and the importance of factors such as; the concentration of calcium ions, concentration of dissolved inorganic carbon, pH and availability of nucleation sites. Dhami et al. (2014) highlighted the importance of the synergistic role of UE and CA in MICP formation. Apart from the precipitation of calcium carbonate, morphology of the formed crystals also play a significant role in their durability.
Calcite, aragonite, and vaterite are some of the most representative biomineral polymorphs with different types of crystallization such as rhombohedral, orthorhombic, and hexagonal (Sarayu et al., 2014). Earlier, Achal et al. (2011) reported that extracellular polymeric substances, biofilms, and amino acid residues have an impact on polymorphic nature of calcium carbonate biominerals. Sondi and Sondi (2005) demonstrated that interaction between Asp residues and UE molecules induce the formation of vaterite rather than calcite. Ercole et al. (2007) showed that proteins present in organic matter regulate aragonite and vaterite precipitation in Bacillus firmus and Bacillus sphaericus. In the case of Myxococcus sp., vaterite and calcite polyforms (Table 2) are more common precipitations (Rodriguez-Navarro et al., 2003; Chekroun et al., 2004; Jimenez-Lopez et al., 2007).
Table 2. Urease and carbonic anhydrase activity, Km, Vmax, and CaCO3 precipitation of free and immobilized systems.
Another microbial mechanism is ammonification of amino acids, and in this process, microbial activity produces CO2 and ammonia during metabolism of amino acids (Equation 8). Hydrolysis of ammonia produces ammonium and hydroxide ions around the cell (Equation 9), leading to their supersaturation, which consequently favors the precipitation of calcium carbonate (Equations 10, 11) (Zhu and Dittrich, 2016).
Myxococcus xanthus is reported to use this mechanism during its growth in liquid and solid mediums and resulting in different polyforms (Rodriguez-Navarro et al., 2003; Chekroun et al., 2004; Jimenez-Lopez et al., 2007). Furthermore, it has been reported that Myxococcus xanthus precipitates uranium as meta-autunite (Turick and Berry, 2016), which can protect concrete structures exposed to radioactive wastes.
In denitrification, MICP results from the oxidation of organic matter using NO3− as a final electron acceptor. The process produces NO2, CO2, and OH−, (Equation 12) and the bacteria creates an alkaline microenvironment by the consumption of H+ in the presence of soluble calcium ions (Zhu and Dittrich, 2016).
Ersan et al. (2015) incorporated denitrifying and expanded clay particles immobilized Pseudomonas aeruginosa and Diaphorobacter nitroreducens and found that the addition closed microcracks in the range of 200–250 μm size and the permeability was decreased by 42 and 47%, respectively. Ersan et al. (2018) implemented special granules called “activated compact denitrifying core” (ACDC) and observed healing of cracks (>70%) larger than 400 μm (Table 1). However, the denitrification process is inhibited by the accumulation of toxic byproducts generated such as nitrite and nitrous oxide. As mentioned previously, ureolysis showed more efficiency of calcium carbonate precipitation (<0.5 mm) than denitrification.
In organic matter rich anaerobic environments, the presence of calcium induces the indirect formation of calcium carbonate minerals by sulfate-reducing bacteria (SRB) due to dissimilatory sulfate reduction process (Equation 13). It has been demonstrated that Desulfovibrio sp. has the ability to precipitate calcium carbonate through removal of sulfates from gypsum (CaSO4.H2O) by a combination of three mechanisms: dissolution, diffusion and calcium carbonate precipitation. Calcium ions released by gypsum dissolution react with carbon dioxide (CO2) under an alkaline pH microenvironment due to sulfide removal, which leads to calcium carbonate precipitation (Perito and Mastromei, 2011).
Recently Alshalif et al. (2016) reported that the addition of sulfate-reducing bacteria in a concrete matrix increased the compressive strength of concrete by 13% and decreased water permeability by 8.5%. More recently, Tambunan et al. (2019) reported an increase in compressive strength (60.87%) and flexural strength (52.30%) by adding SRB isolated from domestic acidic water (Table 1). However, generation of H2S can cause corrosion of the concrete structure, since H2S reacts with oxygen to form elemental sulfur or a partially oxidized sulfur species, which are considered to be corrosion products of concrete surfaces (O'Connell et al., 2010). Few studies have showed improvement in calcium carbonate precipitation by association of SRB and cyanobacteria. Extracellular polymeric substances produced by cyanobacteria as heterogeneous nucleation sites, influence the diffusion barrier and calcium ions mobility, and improves the kinetics of precipitation by SRB (Hardikar and Matijevic, 2001; Braissant and Verrecchia, 2002; Kim et al., 2005; Dupraz et al., 2009). Thus, microbial consortia of SRB and cyanobacteria appears to be potential candidates for application in bioconcrete technology, but requires more research on combining oxygen producing cyanobacteria with anaerobic SRB.
Apart from the above heterotrophic bacterial metabolic processes, the feasibility of MICP by autotrophic processes, such as photosynthesis and methane oxidation, have also been reported. Cyanobacteria and microalgae are the main photosynthetic microorganisms responsible for MICP in the aquatic environment. Calcium carbonate precipitation by photosynthetic microorganisms occurs due to and exchange (Equations 14, 15); is diffused through the membrane and dissociates in cytosol of the cell into CO2 and OH− and this reaction is catalyzed by carbonic anhydrase (CA), leading to an increase of pH due to OH− generation, which along with calcium ions present in the microenvironment induces MICP (Equation 16) (Dhami et al., 2014; Achal et al., 2015).
It should be mentioned however, that the application of photosynthetic microorganisms as agents of bioconcrete can be achieved only when structures are exposed to CO2 and sunlight, which are principal components for photosynthesis process (Seifan et al., 2016).
In marine and freshwater sediments, the concentration of carbon dioxide is largely driven by methane oxidizing bacteria under both aerobic and anoxic conditions. In aerobic conditions, this process is initiated with conversion of methane to methanol by methane mono-oxygenase activity in the presence of oxygen (Equation 17). In the periplasm of a cell, methanol (carbon source) is converted to formate through several enzymatic processes. Subsequently, when formate is in equilibrium with formic acid, methane mono-oxygenase oxidizes formic acid to CO2 by formate dehydrogenase activity (Equations 18–21). Carbon dioxide produced turns into , and calcium carbonate is precipitated in presence of calcium ions (Equation 22) around the cells (Ersan, 2019).
Anaerobic methanotrophic bacteria too produces bicarbonate as a result of methane anaerobic oxidation, with sulfate as the final electron acceptor and in presence of calcium ions (Equations 23, 24) (Seifan et al., 2016; Zhu and Dittrich, 2016).
Recently, Caesar et al. (2019) showed the potential of MICP in mitigation of methane release into the atmosphere due to anaerobic methane oxidation. However, there are no studies on the use of this mechanism in bioconcrete yet.
As in all bacterial regulatory systems, bacterial UE genes are organized in operons and clusters. Structural subunits of this enzyme are habitually encoded by the genes ureA, ureB, and ureC, which are adjacent to each other and arranged from smallest to largest (Mobley et al., 1995). However, the structure of the UE from Helicobacter pylori is rather unique and is comprised of only two subunits; encoded by ureA and ureB genes (Akada et al., 2000). In addition to the structural genes, various bacterial species, e.g., Proteus mirabilis, Klebsiella aerogenes, Bacillus sp., with exception of Bacillus subtilis, have added accessory genes related to the assembly of the nickel-dependent active site of the enzyme in their operon system. In the case of B. subtilis, organizational structure of the UE operon is comprised of three structural genes; ureABC (Cruz-Ramos et al., 1997). Although no accessory genes adjacent to this operon were found in this study, it has been speculated that they were possibly located in an independent operon elsewhere in the genome. To determine the necessity of accessory genes for Bacillus subtilis UE activity, Kim et al. (2005) expressed recombinant UE of B. subtilis in Escherichia coli along with accessory UE genes from Klebsiella aerogenes and Sporosarcina pasteurii. Insertion of both accessory genes failed to increase UE activity, suggesting that accessory proteins of Klebsiella aerogenes and Sporosarcina pasteurii did not share homology with accessory proteins that could play a role in the activation of B. subtilis urease.
Initially, it was believed that urease as well as other nitrogen metabolism related proteins were regulated by the activity of enzyme glutamine synthetase. However, it was demonstrated that complex transcriptional regulation mechanisms govern only nitrogen related genes. Though regulatory mechanisms of urease are complex too, in most cases except for Sporosarcina pasteurii, its expression in bacteria has shown to be induced by the presence of urea, controlled by nitrogen availability or dependent on pH changes (Figure 5) (Mobley and Hausinger, 1989).
Figure 5. Regulatory mechanisms of urease. (A) Urease constitutive system in Sporosarcina pasteurii. (B) Urease System induced by urea in Proteus mirabilis. (C) Urease system induced by nitrogen availability in Klebsiella aerogenes. (D) Urease system induced by pH in Streptococcus salivarius.
The only regulatory gene of the ure operon identified until now is ureR, which is found in the operon of Proteus mirabilis. The urea from this microorganism is induced by the presence of urea, and it has been demonstrated that ureR is a positive activator that binds to the promoter of ureD (Nicholson et al., 1993; Mobley et al., 1995).
One of the best examples of urease-induced expression by nitrogen availability is the case of K. aerogenes. This bacterium relies on a nitrogen regulatory system (NTR), which consists of two components NTRB an NTRC, for the activation of several genes, glutamine synthetase among them, under nitrogen limiting conditions (Macaluso et al., 1990; Wedel and Kustu, 1995). However, expression of urease is also dependent of the regulator NAC, whose transcription is controlled by the NTR system as well (Macaluso et al., 1990; Goss and Bender, 1995). Therefore, under limiting nitrogen sources, the NTR system activates transcription of NAC, and then NAC induces expression of urease in Klebsiella aerogenes (Figure 5).
Presence of nitrogen-containing compounds was also shown to control the expression of the B. subtilis urease. In this bacterium, three promoters were identified upstream of the ureA gene start codon. The first promoter (P1), the closest one to ureA, was designated as a constitutive one that generates low levels of expression, while the other two promoters (P2 and P3), localized further upstream, were found to be regulated by the protein CodY (Wray et al., 1997). This regulator controls hundreds of genes that are needed for the survival of Bacillus subtilis on adverse growth conditions (Mollem et al., 2003). CodY binds and represses these genes during rapid exponential growth but once bacteria reach the stationary phase, CodY losses affinity and the genes are activated (Sonenshein, 2005). In addition to CodY, it was also determined that promoter P3 was also induced by TnrA under nitrogen limiting growth, but repressed by GlnR when nitrogen sources are available (Schreier et al., 1989;Wray et al., 1997).
Changes in pH were shown to regulate the expression of urease from Streptoccocus salivarius. Huang et al. (2014) proposed a model of transcriptional regulation of urease in which the regulator CodY represses the transcription of the ure operon at neutral pH, but at acidic pH the repression is relieved. The loss of the repression by CodY was attributed to the lower concentrations of branched-side chain aminoacids at pH 5 than at pH 7. However, the authors speculated that other factors like carbohydrate concentration might be involved in urease regulation, as the CodY deficient-mutant still showed dependence on pH values.
Another bacterial urease whose regulation is dependent on pH changes is the one encoded by H. pylori. The urease gene cluster of this microorganism consists of two operons: ureAB, which includes the structural genes, and ureIEFGH, which comprises the accessory genes for the assembling of the active center. Akada et al. (2000) demonstrated that transcription of the whole gene cluster generated three mRNAs of different size: one of each separate operon and another one of the whole gene cluster. The authors concluded that transcripts from ureIEFGH and ureABIEFGH are cleaved into different transcription products, which may be translated or degraded according to the pH of the environment. At acidic pH, all three types of mRNAs showed lower rates of degradation. In contrast, at neutral pH, transcripts from the whole gene cluster and the accessory genes showed higher decay. However, the expression from the ureAB operon remained rather constant in this study. Posterior studies have demonstrated that activation of the urease gene cluster is induced by nickel concentrations and acidic pH, through the regulator protein NikR and the two-component system ArsRS, respectively (Van Vliet et al., 2004; Ernst et al., 2005;Pflock et al., 2005).
Although regulatory mechanisms for urease expression have been extensively investigated in pathogenic bacteria as described above, further research is needed in order to understand the rates of urease expression and the regulatory strategies behind them during calcite precipitation, as little information is available concerning environmental bacteria.
On the other hand, the regulatory mechanisms for CA expression is not understood yet. It is only known that carbonic anhydrase is classified into five different genetically distinct families widely distributed in prokaryotes and eukaryotes; α-, β-, γ-, δ-, and ζ-CAs. In bacteria, genes encoding for enzymes belonging to α-, β-, and ζ-CAs classes, which contain zinc ion (Zn2+) in their active site, coordinated by three histidine residues and a water molecule/hydroxide ion, in β- class by two Cys and one His residues are coupled with a fourth ligand of a water molecule/hydroxide ion (Capasso and Supuran, 2015). Enzymes belonging to ζ-CAs classes present hydrolytic activity in ester groups, and hence P-nitrophenyl acetate hydrolysis to P-nitrophenol acetate is used as a measure of carbonic anhydrase activity. According to Supuran and Capasso (2016), genes encoding for α-CAs are present in Gram negative bacteria whereas genes encoding β- Cas and γ- Cas are found in Gram negative and Gram positive bacteria, with the exception of Helicobacter pylori, which presents encoding genes for three families (α-, β-, and ζ-CAs classes).
It can be observed that a wide variety of bacterial groups play a major role in MICP and has potential in bioconcrete and as well as self-healing agents in concrete repair mechanisms. Most of the studies have focused primarily on mechanical and structural properties of concrete, however, understanding the biological aspect is the most important to select suitable microorganisms and to provide conditions for their survival and activity in concrete structures and to extend their life-time, facilitating a self-healing process. Survival of bacterial cells in bioconcrete depends on the regulation of genetic factors and associated activities of urease and carbonic anhydrase. While great strides have been made on the influence of different factors, such as; type of bacteria, nutrient conditions, enzymes, concentration of calcium, etc., on MICP, there are very few studies on monitoring the survival and activity of the bacterial cells in bioconcrete. Furthermore, studies on the regulatory mechanisms of urease and carbonic anhydrase enzymes, which play a major role in MICP are very limited. Urease expression has been extensively studied in pathogenic bacteria, but not much work has been done on the expression of CA. The importance of understanding the genetic factors related to urease and carbonic anhydrase, and associated regulation mechanisms has been presented in this review. Future work on these aspects will aid in the development of novel bacterial strains by genetic/protein engineering to improve their survival and activity under the harsh conditions of concrete.
MC-A, RN, and NB designed this review based on the perspective from their own work and as well as other published work in this area. MC-A contributed extensively to writing this manuscript. LM-H contributed to the genetic concepts of urease and carbonic anhydrase. MS-M contributed to the microbiological concepts of urease and carbonic anhydrase. MM contributed to the biomineralization. RN contributed to the chemical aspects of calcium carbonate precipitation and bioconcrete and NB contributed to the microbial diversity and their mechanisms. NB along with MC-A and RN revised and structured the manuscript. All authors read and approved the final manuscript.
The authors declare that the research was conducted in the absence of any commercial or financial relationships that could be construed as a potential conflict of interest.
MC-A, LM-H, MS-M, MM, and NB thank Universidad Autónoma de Coahuila for providing digital library support through CONRICyT for consultation of research papers cited in this work. RN also thanks Universidad Juárez del Estado de Durango.
Abo-El-Enein, S. A., El-Sayed, H. A., Ali, A. H., Mohammed, Y. T., Khater, H. M., and Ouda, A. S. (2014). Physico-mechanical properties of high performance concrete using different aggregates in presence of silica fume. HBRC J. 10, 43–48. doi: 10.1016/j.hbrcj.2013.06.002
Achal, V., and Mukherjee, A. (2015). A review of microbial precipitation for sustainable construction. Constr Build Mater. 93, 1224–1235. doi: 10.1016/j.conbuildmat.2015.04.051
Achal, V., Mukherjee, A., Basu, P. C., and Reddy, M. S. (2009). Lactose mother liquor as an alternative nutrient source for microbial concrete production by Sporosarcina pasteurii. J. Ind. Microbiol. Biotechnol. 36, 433–438. doi: 10.1007/s10295-008-0514-7
Achal, V., Mukherjee, A., Kumari, D., and Zhang, Q. (2015). Biomineralization for sustainable construction–a review of processes and applications. Earth. Sci. Rev. 148:1–17. doi: 10.1016/j.earscirev.2015.05.008
Achal, V., Mukherjee, A., and Reddy, M. S. (2010). Biocalcification by Sporosarcina pasteurii using corn steep liquor as the nutrient source. Ind Biotechnol. 6, 170–174. doi: 10.1089/ind.2010.6.170
Achal, V., Mukherjee, A., and Zhang, A. (2016). Unearthing ecological wisdom from natural habitats and its ramifications on development of biocement and sustainable cities. Landsc. Urban. Plan. 155, 61–68. doi: 10.1016/j.landurbplan.2016.04.013
Achal, V., Pan, X., and Özyurt, N. (2011). Improved strength and durability of fly ash-amended concrete by microbial calcite precipitation. Ecol. Eng. 37, 554–559. doi: 10.1016/j.ecoleng.2010.11.009
Adolphe, J. P., Loubière, J. F., Paradas, J., and Soleilhavoup, F. (1990) Procédé Detraitement Biologique d'une Surface Artificielle. París: Francia European Patent 90400G97.0. (after French patent 8903517, 1989).
Adzami, N. S., Ghazali, M. F., Ramli, A. H., Tajarudin, H. A., and Daud, Z. (2018). A new potential of calcium carbonate production induced by Bacillus sphaericus in batch fermentation. Int. J. Integr. Eng. 10, 43–47. doi: 10.30880/ijie.2018.10.09.024
Aguilera, L. A. P., Zapata, J. A. N., and Morales, B. O. O. (2015). La bioprecipitación de carbonato de calcio por la biota nativa como un método de restauración. Nexo Rev. Científ. 28, 25–40. doi: 10.5377/nexo.v28i01.1779
Akada, J. K., Shirai, M., Takeuchi, H., Tsuda, M., and Nakazawa, T. (2000). Identification of the urease operon in Helicobacter pylori and its control by mRNA decay in response to pH. Mol Microbiol. 36, 1071–1084. doi: 10.1046/j.1365-2958.2000.01918.x
Alshalif, A. F., Irwan, J. M., Othman, N., and Anneza, L. H. (2016). Isolation of Sulphate Reduction Bacteria (SRB) to Improve Compress Strength and Water Penetration of Bio-Concrete. MATEC Web Conf. 47:01016. doi: 10.1051/matecconf/20164701016
Al-Tabbaa, A., Lark, B., Paine, K., Jefferson, T., Litina, C., Gardner, D., et al. (2018). Biomimetic cementitious construction materials for next-generation infrastructure. Proc. Inst.Civ. Eng.Smart. Infrastr. Constr. 171, 67–76. doi: 10.1680/jsmic.18.00005
Al-Tabbaa, A., Litina, C., Giannaros, P., Kanellopoulos, A., and Souza, L. (2019). First UK field application and performance of microcapsule-based self-healing concrete. Constr. Build. Mat. 208, 669–685. doi: 10.1016/j.conbuildmat.2019.02.178
Anbu, P., Kang, C. H., Shin, Y. J., and So, J. S. (2016). Formations of calcium carbonate minerals by bacteria and its multiple applications. SpringerPlus 5:250. doi: 10.1186/s40064-016-1869-2
Aprianti, E. (2017). A huge number of artificial waste material can be supplementary cementitious material (SCM) for concrete production–a review part II. J Clean. Prod. 142, 4178–4194. doi: 10.1016/j.jclepro.2015.12.115
Bachmeier, K. L., Williams, A. E., Warmington, J. R., and Bang, S. S. (2002). Urease activity in microbiologically-induced calcite precipitation. J Biotechnol. 93, 171–181. doi: 10.1016/S0168-1656(01)00393-5
Backes, C. W., Anker, H. T., Keessen, A. M., Baaner, L., and Möckel, S. (2018). Comparison of Ammonia Regulation in Germany, The Netherlands and Denmark-Legal Framework. IFRO Report series.
Bang, S. S., Galinat, J. K., and Ramakrishnan, V. (2001). Calcite precipitation induced by polyurethane-immobilized Bacillus pasteurii. Enzyme. Microb. Technol. 28, 404–409. doi: 10.1016/S0141-0229(00)00348-3
Benzerara, K., Skouripanet, F., Li, J., Ferard, C., Gugger, M., Laurent, T., et al. (2014). Intracellular Ca-carbonate biomineralization is widespread in cyanobacteria. Proc. Natl. Acad. Sci. U.S.A. 111, 10933–10938. doi: 10.1073/pnas.1403510111
Biswas, M., Majumdar, S., Chowdhury, T., Chattopadhyay, B., Mandal, S., Halder, U., et al. (2010). Bioremediase a unique protein from a novel bacterium BKH1, ushering a new hope in concrete technology. Enzyme. Microb. Technol. 46, 581–587. doi: 10.1016/j.enzmictec.2010.03.005
Braissant, O., and Verrecchia, E. P. (2002). Microbial biscuits of vaterite in Lake Issyk-Kul (Republic of Kyrgyzstan) – Discussion. J. Sediment. Res. 72, 944–946. doi: 10.1306/041802720944
Bundur, Z. B., Kirisits, M. J., and Ferron, R. D. (2015). Biomineralized cement-based materials: Impact of inoculating vegetative bacterial cells on hydration and strength. Cement Concrete Res. 67, 237–245. doi: 10.1016/j.cemconres.2014.10.002
Burne, R. A., and Chen, Y.-Y. M. (2000). Bacterial ureases in infectious diseases. Microb. Infect. 2, 533–542. doi: 10.1016/S1286-4579(00)00312-9
Caesar, K. H., Kyle, J. R., Lyons, T. W., Tripati, A., and Loyd, S. J. (2019). Carbonate formation in salt dome cap rocks by microbial anaerobic oxidation of methane. Nat. Commun. 10:808. doi: 10.1038/s41467-019-08687-z
Cam, N., Georgelin, T., Jaber, M., Lambert, J. F., and Benzerara, K. (2015). In vitro synthesis of amorphous Mg-, Ca-, Sr- and Ba-carbonates: WHAT do we learn about intracellular calcification by cyanobacteria? Geochim. Cosmochim. Acta 161, 36–49. doi: 10.1016/j.gca.2015.04.003
Capasso, C., and Supuran, C. T. (2015). An overview of the alpha-, beta- and gamma-carbonic anhydrases from Bacteria: can bacterial carbonic anhydrases shed new light on evolution of bacteria?. J. Enzyme Inhib. Med. Chem. 30, 325–332. doi: 10.3109/14756366.2014.910202
Castanier, S., Métayer-Levrel, L. G., and Perthuisot, J. P. (1999) Ca-carbonates precipitation limestone genesis—the microbiogeologist point of view. Sediment. Geol. 126, 9–23. doi: 10.1016/S0037-0738(99)00028-7
Castro-Alonso, M. J., Lopez, C. E., Garcia-Perez, S. O., Narayanasamy, R., Fajardo, G. J., Herrera, H., et al. (2018). Improved strength and durability of concrete through metabolic activity of ureolytic bacteria. Environ. Sci. Pollut. Res. Int. 25, 21451–21458. doi: 10.1007/s11356-017-9347-0
Charpe, A. U., Latkar, M. V., and Chakrabarti, T. (2017). Microbially assisted cementation–a biotechnological approach to improve mechanical properties of cement. Constr. Build. Mater. 135, 472–476. doi: 10.1016/j.conbuildmat.2017.01.017
Chaurasia, L., Bisht, V., Singh, L. P., and Gupta, S. (2019). A novel approach of biomineralization for improving micro and macro-properties of concrete. Constr. Build. Mater. 195, 340–351. doi: 10.1016/j.conbuildmat.2018.11.031
Chekroun, K. B., Rodríguez-Navarro, C., González-Muñoz, M. T., Arias, J. M., Cultrone, G., and Rodríguez-Gallego, M. (2004). Precipitation and growth morphology of calcium carbonate induced by Myxococcus xanthus: implications for recognition of bacterial carbonates. J Sediment Res. 74, 868–876. doi: 10.1306/050504740868
Chen, H., Qian, C., and Huang, H. (2016). Self-healing cementitious materials based on bacteria and nutrients immobilized respectively. Constr. Build. Mater. 126, 297–303. doi: 10.1016/j.conbuildmat.2016.09.023
Cheng, L., and Cord-Ruwisch, R. (2012). In situ soil cementation with ureolytic bacteria by surface percolation. Ecol. Eng. 42:64–72. doi: 10.1016/j.ecoleng.2012.01.013
Couradeau, E., Benzerara, K., Gérard, E., Moreira, D., Bernard, S., Brown, G. E., et al. (2012). An early-branching microbialite cyanobacterium forms intracellular carbonates. Science 336, 459–462. doi: 10.1126/science.1216171
Cruz-Ramos, H., Glaser, P., Wray, L. V., and Fisher, S. H. (1997). The Bacillus subtilis ureABC operon. J. Bacteriol. 179, 3371–3373. doi: 10.1128/jb.179.10.3371-3373.1997
Davies, R., Teall, O., Pilegis, M., Kanellopoulos, A., Sharma, T., Jefferson, A., et al. (2018). Large scale application of self-healing concrete: design, construction, and testing. Front. Mat. 5:51. doi: 10.3389/fmats.2018.00051
De Muynck, W., Cox, K., De Belie, N., and Verstraete, W. (2008). Bacterial carbonate precipitation as an alternative surface treatment for concrete. Constr. Build. Mater. 22, 875–885. doi: 10.1016/j.conbuildmat.2006.12.011
Dhami, N. K., Reddy, M. S., and Mukherjee, A. (2014). Application of calcifying bacteria for remediation of stones and cultural heritages. Front. Microbiol. 5:304. doi: 10.3389/fmicb.2014.00304
Dupraz, C., Reid, R. P., Braissant, O., Decho, A. W., Norman, R. S., and Visscher, P. T. (2009). Processes of carbonate precipitation in modern microbial mats. Earth. Sci. Rev. 96, 141–162. doi: 10.1016/j.earscirev.2008.10.005
Ercole, C., Cacchio, P., Botta, A. L., Centi, V., and Lepidi, A. (2007). Bacterially induced mineralization of calcium carbonate: the role of exopolysaccharides and capsular polysaccharides. Microsc. Microanal. 13, 42–50. doi: 10.1017/S1431927607070122
Ernst, F. D., Kuipers, E. J., Heijens, A., Sarwari, R., Stoof, J., Penn, C. W., et al. (2005). The nickel-responsive regulator NikR controls activation and repression of gene transcription in Helicobacter pylori. Infect. Immun. 73, 7252–7258. doi: 10.1128/IAI.73.11.7252-7258.2005
Ersan, Y., Boon, N., and De Belie, N. (2015). “Microbial self-healing concrete: denitrification as an enhanced and environment-friendly approach,” in 5th International Conference on Self-Healing Materials, ed K. Nicholson (Ghent).
Ersan, Y. C. (2019). “Overlooked strategies in exploitation of microorganisms in the field of building materials,” in Ecological Wisdom Inspired Restoration Engineering, eds. V. Achal and A. Mukherjee (Singapore: Springer; CRC Press), 19–45. doi: 10.1007/978-981-13-0149-0_2
Ersan, Y. C., Boon, N., and De Belie, N. (2018). “Granules with activated compact denitrifying core (ACDC) for self-healing concrete with corrosion protection functionality,” in Microorganisms-Cementitious Materials Interactions: Final Conference of RILEM TC 253-MCI., eds A. Bertron and H. Jonkers (Toulouse: RILEM Publications), 475–484.
Fang, C., He, J., Achal, V., and Plaza, G. (2019). Tofu wastewater as efficient nutritional source in biocementation for improved mechanical strength of cement mortars. Geomicrobiol. J. 36, 515–521. doi: 10.1080/01490451.2019.1576804
Fu, G., Valiyaveettil, S., Wopenka, B., and Morse, D. E. (2005). CaCO3 biomineralization: acidic 8-kDa proteins isolated from aragonitic abalone shell nacre can specifically modify calcite crystal morphology. Biomacromolecules 6, 1289–1298. doi: 10.1021/bm049314v
Ghosh, S., Biswas, M., Chattopadhyay, B. D., and Mandal, S. (2009). Microbial activity on the microstructure of bacteria modified mortar. Cement. Concrete. Comp. 31, 93–98. doi: 10.1016/j.cemconcomp.2009.01.001
Gonsalves, G. M. (2011). Bioconcrete-a Sustainable Substitute for Concrete? (Master's thesis). Universitat Politècnica de Catalunya. Institut Universitari de Recerca en Ciència i Tecnologies de la Sostenibilitat, Barcelone, Spain.
Goss, T. J., and Bender, R. A. (1995). The nitrogen assimilation control protein, NAC, is a DNA binding transcription activator in Klebsiella aerogenes. J. Bacteriol. 177, 3546–3555. doi: 10.1128/jb.177.12.3546-3555.1995
Hamdan, N., Kavazanjian, E. J., and Rittmann, B. E. (2011). “Sequestration of radionuclides and metal contaminants through microbially-induced carbonate precipitation,” in 14th Pan-American Conference on Soil Mechanics and Geotechnical Engineering (Toronto, ON: Canada).
Hardikar, V. V., and Matijevic, E. (2001). Influence of ionic and non ionic dextrans on the formation of calcium hydroxide and calcium carbonate particles. Colloids. Surf. 186, 23–31. doi: 10.1016/S0927-7757(01)00479-4
Head, I. M., Gray, N. D., Babenzien, H. D., and Oliver-Glöckner, F. (2000). Uncultured giant sulfur bacteria of the genus Achromatium. FEMS Microbiol. Ecol. 33, 171–180. doi: 10.1111/j.1574-6941.2000.tb00739.x
Huang, S. C., Burne, R. A., and Chen, Y. Y. (2014). The pH-dependent expression of the urease operon in Streptococcus salivarius is mediated by CodY. Appl. Environ. Microbiol. 80, 5386–93. doi: 10.1128/AEM.00755-14
Ivanov, V., Stabnikov, V., Stabnikova, O., and Kawasaki, S. (2019). Environmental safety and biosafety in construction biotechnology. World J. Microbiol. Biotechnol. 35:26. doi: 10.1007/s11274-019-2598-9
Jain, S., and Arnepalli, D. N. (2019). “Biominerlisation as a remediation technique: a critical review,” in Geotechnical Characterisation and Geoenvironmental Engineering, eds V. Stalin and M. Muttharam (Singapore: Springer), 155–162. doi: 10.1007/978-981-13-0899-4_19
Jimenez-Lopez, C. J., Jroundi, F., Rodríguez-Gallego, M., Arias, J. M., and Gonzalez-Muñoz, M. T. (2007). “Biomineralization induced by Myxobacteria,” in Proceedings of the II International Conference on Environmental, Industrial and Applied Microbiology (BioMicroWorld2007), ed A. Mendez-Vilas (Seville: World Scientific), 143–154. doi: 10.1142/7133
Jonkers, H. M., and Schlangen, E. (2009). “A two component bacteria-based on self-healing concrete,” in Concrete Repair, Rehabilitation, and Retrofitting II: 2nd International Conference on Concrete Repair, Rehabilitation and Retrofitting, eds M. G. Alexander, H. Beushausen, F. Dehn, and P. Moyo (Cape Town: CRC Press). doi: 10.1201/9781439828403.ch27
Jonkers, H. M., Thijssen, A., Muyzer, G., Copuroglu, O., and Schlangen, E. (2010). Application of bacteria as self-healing agent for the development of sustainable concrete. Ecol. Eng. 36, 230–235. doi: 10.1016/j.ecoleng.2008.12.036
Joshi, S., Goyal, S., Mukherjee, A., and Reddy, M. S. (2017). Microbial healing of cracks in concrete: a review. J. Ind. Microbiol. Biotechnol. 44, 1511–1525. doi: 10.1007/s10295-017-1978-0
Jroundi, F., Fernández-Vivas, A., Rodriguez-Navarro, C., Bedmar, E. J., and González-Muñoz, M. T. (2010). Bioconservation of deteriorated monumental calcarenite stone and identification of bacteria with carbonatogenic activity. Microb. Ecol. 60, 39–54. doi: 10.1007/s00248-010-9665-y
Jroundi, F., Gonzalez-Muñoz, M. T., Garcia-Bueno, A., and Rodriguez-Navarro, C. (2014). Consolidation of archaeological gypsum plaster by bacterial biomineralization of calcium carbonate. Acta. biomater. 10, 3844–3854. doi: 10.1016/j.actbio.2014.03.007
Kang, C. H., Choi, J. H., Noh, J., Kwak, D. Y., Han, S. H., and So, J. S. (2014). Microbially induced calcite precipitation-based sequestration of strontium by Sporosarcina pasteurii WJ-2. App. Biochem. Biotechnol. 174, 2482–2491. doi: 10.1007/s12010-014-1196-4
Khaliq, W., and Ehsan, M. B. (2016). Crack healing in concrete using various bio influenced self-healing techniques. Constr. Build. Mater. 102, 349–357. doi: 10.1016/j.conbuildmat.2015.11.006
Kim, I. G., Jo, B. H., Kang, D. G., Kim, C. S., Choi, Y. S., and Cha, H. J. (2012). Biomineralization-based conversion of carbon dioxide to calcium carbonate using recombinant carbonic anhydrase. Chemosphere 87, 1091–1096. doi: 10.1016/j.chemosphere.2012.02.003
Kim, J. K., Mulrooney, S. B., and Hausinger, R. P. (2005). Biosynthesis of active Bacillus subtilis urease in the absence of known urease accessory proteins. J. Bacteriol. 187, 7150–7154. doi: 10.1128/JB.187.20.7150-7154.2005
Krajewska, B. (2018). Urease-aided calcium carbonate mineralization for engineering applications: a review. J. Adv. Res. 13, 59–67. doi: 10.1016/j.jare.2017.10.009
Le Metayer-Levrel, G., Castanier, S., Orial, G., Loubiere, J. F., and Perthuisot, J. P. (1999). Applications of bacterial carbonatogenesis to the protection and regeneration of limestones in buildings and historic patrimony. Sediment. Geol. 126, 25–34. doi: 10.1016/S0037-0738(99)00029-9
Li, M., Cheng, X., and Guo, H. (2013). Heavy metal removal by biomineralization of urease producing bacteria isolated from soil. Int. Biodeterior. Biodegradation. 76, 81–85. doi: 10.1016/j.ibiod.2012.06.016
Macaluso, A., Best, E. A., and Bender, R. A. (1990). Role of the nac gene product in the nitrogen regulation of some NTR-regulated operons of Klebsiella aerogenes. J. Bacteriol. 172, 7249–7255. doi: 10.1128/jb.172.12.7249-7255.1990
Majumdar, S., Sarkar, M., Chowdhury, T., Chattopadhyay, B., and Mandal, S. (2012). Use of bacterial protein powder in commercial fly ash pozzolana cements for high performance construction materials. Open J. Civ. Eng. 2, 218–228. doi: 10.4236/ojce.2012.24029
Miller, S. A., Horvath, A., and Monteiro, P. J. (2018). Impacts of booming concrete production on water resources worldwide. Nat. Sustain. 1:69. doi: 10.1038/s41893-017-0009-5
Mobley, H. L., Garner, R. M., and Bauerfeind, P. (1995). Helicobacter pylori nickel-transport gene nixA: synthesis of catalytically active urease in Escherichia coli independent of growth conditions. Mol. Microbiol. 16, 97–109. doi: 10.1111/j.1365-2958.1995.tb02395.x
Mobley, H. L., and Hausinger, R. P. (1989). Microbial ureases: significance, regulation, and molecular characterization. Microbiol. Rev. 53, 85–108.
Mollem, V., Nakaura, Y., Shivers, R. P., Yamaguchi, H., Losick, R., Fujita, Y., et al. (2003). Additional targets of the Bacillus subtilis global regulator CodY identified by chromatin immunoprecipitation and genome-wide transcript analysis. J. Bacteriol. 185, 1911–1922. doi: 10.1128/JB.185.6.1911-1922.2003
Mors, R. M., and Jonkers, H. M. (2016). Feasibility of lactate derivative based agent as additive for concrete for regain of crack water tightness by bacterial metabolism. Ind. Crop. Prod. 106, 97–104. doi: 10.1016/j.indcrop.2016.10.037
Narayanasamy, R., Alvarado, A., Sanchez Medrano, J., Betancourt Hernandez, J., and Balagurusamy, N. (2013). “Potential of soil bacteria from the comarca lagunera, north-east Mexico for bioconcrete development,” in Proceedings of the 4th International Conference on Self-Healing Materials ICSHM, eds N. De Belie, S. Van der Zwaag, E. Gruyaert, K. Van Tittelboom, and B. Debbaut (Ghent), 601–605.
Narayanasamy, R., Villegas-Flores, N., Betancourt-Silva, F., Betancourt-Hernandez, J., and Balagurusamy, N. (2010). “Application of bacteria in repairing the concrete cracks—a review concrete under severe conditions,” in Sixth International Conference on Concrete Under Severe Conditions, Environment Load, eds. P. Castro-Borges, E. I. Moreno, K. Sakai, O. E. Gjørv, and N. Banthia (Merida Yucatan: CRC Press), 1237–1244. doi: 10.1201/b10552-164
Nicholson, E. B., Concaugh, A. E., Foxall, P. A., Island, M. D., and Mobley, H. L. (1993). Proteus mirabilis urease: transcriptional regulation by UreR. J. Bacteriol. 175, 465–473. doi: 10.1128/jb.175.2.465-473.1993
O'Connell, M., McNally, C., and Richardson, M. G. (2010). Biochemical attack on concrete in wastewater applications: a state of the art review. Cem. Concr. Comp. 32, 479–485. doi: 10.1016/j.cemconcomp.2010.05.001
Okwadha, G. D., and Li, J. (2011). Biocontainment of polychlorinated biphenyls (PCBs) on flat concrete surfaces by microbial carbonate precipitation. J. Environ. Manage. 92, 2860–2864. doi: 10.1016/j.jenvman.2011.05.029
Okyay, T. O., and Rodrigues, D. F. (2015). Biotic and abiotic effects on CO2 sequestration during microbially-induced calcium carbonate precipitation. FEMS Microbiol. Ecol. 91:fiv017. doi: 10.1093/femsec/fiv017
Park, S. I., and Hausinger, R. P. (1995). Requirement of carbon dioxide for in vitro assembly of the urease nickel metallocenter. Science 267, 1156–1158. doi: 10.1126/science.7855593
Perito, B., Marvasi, M., Barabesi, C., Mastromei, G., Bracci, S., Vendrell, M., et al. (2014). A Bacillus subtilis cell fraction (BCF) inducing calcium carbonate precipitation: biotechnological perspectives for monumental stone reinforcement. J. Cult. Herit. 15, 345–351. doi: 10.1016/j.culher.2013.10.001
Perito, B., and Mastromei, G. (2011). “Molecular basis of bacterial calcium carbonate precipitation,” in Molecular Biomineralization: Aquatic Organisms Forming Extraordinary Materials, ed. W. E. G. Müller (Berlin; Heidelberg: Springer), 113–139. doi: 10.1007/978-3-642-21230-7_5
Pflock, M., Kennard, S., Delany, I., Scarlato, V., and Beier, D. (2005). Acid-induced activation of the urease promoters is mediated directly by the ArsRS two-component system of Helicobacter pylori. Infect. Immun. 73, 6437–6445. doi: 10.1128/IAI.73.10.6437-6445.2005
Rodriguez-Navarro, C., Rodriguez-Gallego, M., Chekroun, K. B., and Gonzalez-Muñoz, M. T. (2003). Conservation of ornamental stone by Myxococcus xanthus-induced carbonate biomineralization. Appl. Environ. Microbiol. 69, 2182–2193. doi: 10.1128/AEM.69.4.2182-2193.2003
Ruan, S., Qiu, J., Weng, Y., Yang, Y., Yang, E. H., Chu, J., et al. (2019). The use of microbial induced carbonate precipitation in healing cracks within reactive magnesia cement-based blends. Cem. Concr. Res. 115, 176–188. doi: 10.1016/j.cemconres.2018.10.018
Salman, V., Berben, T., Bowers, R. M., Woyke, T., Teske, A., and Angert, E. R. (2016). Insights into the single cell draft genome of “Candidatus Achromatium palustre”. Stand Genomic. Sci. 11:28. doi: 10.1186/s40793-016-0146-x
Sarayu, K., Iyer, N. R., and Murthy, A. R. (2014). Exploration on the biotechnological aspect of the ureolytic bacteria for the production of the cementitious materials—a review. Appl. Biochem. Biotechnol. 172, 2308–2323. doi: 10.1007/s12010-013-0686-0
Sarkar, A., Chatterjee, A., Mandal, S., and Chattopadhyay, B. (2019). An alkaliphilic bacterium BKH 4 of bakreshwar hot spring pertinent to bio-concrete technology. J. Appl. Microbiol. Biochem. 6, 1742–1750. doi: 10.1111/jam.14236
Sarkar, M., Adak, D., Tamang, A., Chattopadhyay, B., and Mandal, S. (2015). Genetically-enriched microbe-facilitated self-healing concrete–a sustainable material for a new generation of construction technology. RSC Adv. 5, 105363–105371. doi: 10.1039/C5RA20858K
Sarkar, M., Chowdhury, T., Chattopadhyay, B., Gachhui, R., and Mandal, S. (2014). Autonomous bioremediation of a microbial protein (bioremediase) in Pozzolana cementitious composite. J. Mat. Sci. 49, 4461–4468. doi: 10.1007/s10853-014-8143-1
Schreier, H. J., Brown, S. W., Hirschi, K. D., Nomellini, J. F., and Sonenshein, A. L. (1989). Regulation of Bacillus subtilis glutamine synthetase gene expression by the product of the glnR gene. J. Mol. Biol. 210, 51–63. doi: 10.1016/0022-2836(89)90290-8
Seifan, M., Ebrahiminezhad, A., Ghasemi, Y., Samani, A. K., and Berenjian, A. (2018). The role of magnetic iron oxide nanoparticles in the bacterially induced calcium carbonate precipitation. Appl. Microbiol. Biotechnol. 102, 3595–3606. doi: 10.1007/s00253-018-8860-5
Seifan, M., Samani, A. K., and Berenjian, A. (2016). Bioconcrete: next generation of self-healing concrete. Appl Microbiol. Biotechnol. 100, 2591–2602. doi: 10.1007/s00253-016-7316-z
Siddique, R., Singh, K., Singh, M., Corinaldesi, V., and Rajor, A. (2016). Properties of bacterial rice husk ash concrete. Constr. Build. Mater. 121, 112–119. doi: 10.1016/j.conbuildmat.2016.05.146
Silva, F. B., Boon, N., De Belie, N., and Verstraete, W. (2015). Industrial application of biological self-healing concrete: challenges and economical feasibility. J. Commer. Biotechno. 21, 31–38. doi: 10.5912/jcb662
Sondi, I., and Sondi, B. (2005). Influence of the primary structure of enzymes on the formation of CaCO3 polymorphs: a comparison of plant (Canavalia ensiformis) and bacterial (Bacillus pasteurii) ureases. Langmuir 21, 8876–8882. doi: 10.1021/la051129v
Sonenshein, A. L. (2005). CodY, a global regulator of stationary phase and virulence in Gram-positive bacteria. Curr. Opin. Microbiol. 8, 203–207. doi: 10.1016/j.mib.2005.01.001
Stocks-Fischer, A., Galinat, J. K., and Bang, S. S. (1999). Microbiological precipitation of CaCO3. Soil Biol. Biochem. 31, 1563–1571. doi: 10.1016/S0038-0717(99)00082-6
Supuran, C. T., and Capasso, C. (2016). New light on bacterial carbonic anhydrases phylogeny based on the analysis of signal peptide sequences. J. Enzyme. Inhib. Med. Chem. 31, 1254–1260. doi: 10.1080/14756366.2016.1201479
Tambunan, T., Juki, M. I., and Othman, N. (2019). Mechanical properties of sulphate reduction bacteria on the durability of concrete in chloride condition. MATEC Web Conf. EDP Sci. 258:01024 doi: 10.1051/matecconf/201925801024
Teall, O., Davies, R., Pilegis, M., Kanellopoulos, A., Sharma, T., Paine, K., et al. (2016). “Self-healing concrete full-scale site trials,” in Proceedings of the 11th fib International PhD Symposium in Civil Engineering, eds K. Maekawa, A. Kasuga, and J. Yamazaki (Tokyo: Balkema Publishers), 639–646.
Tiano, P., Biagiotti, L., and Mastromei, G. (1999). Bacterial bio-mediated calcite precipitation for monumental stones conservation: methods of evaluation. J. Microbiol. Meth. 36, 139–145. doi: 10.1016/S0167-7012(99)00019-6
Turick, C. E., and Berry, C. J. (2016). Review of concrete biodeterioration in relation to nuclear waste. J. Environ. Radioact. 151, 12–21. doi: 10.1016/j.jenvrad.2015.09.005
Tziviloglou, E., Wiktor, V., Jonkers, H. M., and Schlangen, E. (2016). Bacteria-based self-healing concrete to increase liquid tightness of cracks. Constr. Build. Mat. 122, 118–125. doi: 10.1016/j.conbuildmat.2016.06.080
Van Tittelboom, K., De Belie, N., De Muynck, W., and Verstraete, W. (2010). Use of bacteria to repair cracks in concrete. Cem. Concr. Res. 40, 157–166. doi: 10.1016/j.cemconres.2009.08.025
Van Tittelboom, K., Wang, J., Araújo, M., Snoeck, D., Gruyaert, E., Debbaut, B., et al. (2016). Comparison of different approaches for self-healing concrete in a large-scale lab test. Constr. Build. Mat. 107, 125–137. doi: 10.1016/j.conbuildmat.2015.12.186
Van Vliet, A. H., Ernst, F. D., and Kusters, J. G. (2004). NikR-mediated regulation of Helicobacter pylori acid adaptation. Trends. Microbiol. 12, 489–494. doi: 10.1016/j.tim.2004.09.005
Verma, R. K., Chaurasia, L., Bisht, V., and Thakur, M. (2015). Biomineralization and bacterial carbonate precipitation in mortar and concrete. Biosci. Bioeng. 1, 5–11.
Wang, J., Van Tittelboom, K., De Belie, N., and Verstraete, W. (2012). Use of silica gel or polyurethane immobilized bacteria for self-healing concrete. Constr. Build. Mater. 26, 532–540. doi: 10.1016/j.conbuildmat.2011.06.054
Wang, J. Y., Soens, H., Verstraete, W., and De Belie, N. (2014). Self-healing concrete by use of microencapsulated bacterial spores. Cem. Concr. Res. 56, 139–152. doi: 10.1016/j.cemconres.2013.11.009
Wedel, A., and Kustu, S. (1995). The bacterial enhancer-binding protein NTRC is a molecular machine: ATP hydrolysis is coupled to transcriptional activation. Genes Dev. 9, 2042–2052. doi: 10.1101/gad.9.16.2042
Weiner, S., and Dove, P. M. (2003). An overview of biomineralization processes and the problem of the vital effect. Rev. Mineral. Geochem. 54, 1–29. doi: 10.2113/0540001
Whiffin, V. S., Van Paassen, L. A., and Harkes, M. P. (2007). Microbial carbonate precipitation as a soil improvement technique. Geomicrobiol. J. 24, 417–423. doi: 10.1080/01490450701436505
Wray, L. V., Ferson, A. E., and Fisher, S. H. (1997). Expression of the Bacillus subtilis ureABC operon is controlled by multiple regulatory factors including CodY, GlnR, TnrA, and Spo0H. J. Bacteriol. 179, 5494–5501. doi: 10.1128/jb.179.17.5494-5501.1997
Xu, H., Peng, X., Bai, S., Ta, K., Yang, S., Liu, S., et al. (2019). Precipitation of calcium carbonate mineral induced by viral lysis of cyanobacteria: evidence from laboratory experiments. Biogeosciences 16, 949–960. doi: 10.5194/bg-16-949-2019
Xu, J., and Wang, X. (2018). Self-healing of concrete cracks by use of bacteria-containing low alkali cementitious material. Constr. Build. Mat. 167, 1–14. doi: 10.1016/j.conbuildmat.2018.02.020
Yadav, R., Labhsetwar, N., Kotwal, S., and Rayalu, S. (2011). Single enzyme nanoparticle for biomimetic CO2 sequestration. J. Nanoparticle. Res. 13, 263–271. doi: 10.1007/s11051-010-0026-z
Yadav, R. R., Krishnamurthi, K., Shekh, A. Y., Mudliar, S. N., Devi, S. S., and Chakrabarti, T. (2014) Activity enhancement of carbonic anhydrase in Chlamydomonas sp. for effective CO2 sequestration. Clean. Technol. Environ. Policy. 16, 1827–1833. doi: 10.1007/s10098-014-0734-7
Zhang, J., Zhou, A., Liu, Y., Zhao, B., Luan, Y., Wang, S., et al. (2017). Microbial network of the carbonate precipitation process induced by microbial consortia and the potential application to crack healing in concrete. Sci. Rep. 7:14600. doi: 10.1038/s41598-017-15177-z
Keywords: MICP, urease, carbonic anhydrase, molecular factors, genetic factors, bioconcrete
Citation: Castro-Alonso MJ, Montañez-Hernandez LE, Sanchez-Muñoz MA, Macias Franco MR, Narayanasamy R and Balagurusamy N (2019) Microbially Induced Calcium Carbonate Precipitation (MICP) and Its Potential in Bioconcrete: Microbiological and Molecular Concepts. Front. Mater. 6:126. doi: 10.3389/fmats.2019.00126
Received: 02 March 2019; Accepted: 14 May 2019;
Published: 10 June 2019.
Edited by:
Libo Yan, Technische Universitat Braunschweig, GermanyReviewed by:
Ru Mu, Hebei University of Technology, ChinaCopyright © 2019 Castro-Alonso, Montañez-Hernandez, Sanchez-Muñoz, Macias Franco, Narayanasamy and Balagurusamy. This is an open-access article distributed under the terms of the Creative Commons Attribution License (CC BY). The use, distribution or reproduction in other forums is permitted, provided the original author(s) and the copyright owner(s) are credited and that the original publication in this journal is cited, in accordance with accepted academic practice. No use, distribution or reproduction is permitted which does not comply with these terms.
*Correspondence: Rajeswari Narayanasamy, bmFyYXlhQHVqZWQubXg=; Nagamani Balagurusamy, Ym5hZ2FtYW5pQHVhZGVjLmVkdS5teA==
Disclaimer: All claims expressed in this article are solely those of the authors and do not necessarily represent those of their affiliated organizations, or those of the publisher, the editors and the reviewers. Any product that may be evaluated in this article or claim that may be made by its manufacturer is not guaranteed or endorsed by the publisher.
Research integrity at Frontiers
Learn more about the work of our research integrity team to safeguard the quality of each article we publish.