- GIU 17/061, GI 609, Departamento de Genética, Antropología Física y Fisiología Animal, Facultad de Ciencia y Tecnología, Universidad del País Vasco/Euskal Herriko Unibertsitatea (UPV/EHU), Bilbao, Spain
The aim of this study was to ascertain if the capacity for acute and chronic compensation of thermal effects on physiological rates represents a trait contributing to inter-individual growth rate differences in the mussel Mytilus galloprovincialis. Juvenile mussels (10–11 mm shell length) were collected, transported to the laboratory, and divided into two groups: one group was maintained at 20°C (warm treatment), and the other at 10°C (cold treatment). The mussels were reared at these two temperatures (continuously fed), until clear size differences allowed us to select fast- (F) and slow (S)-growing individuals from both groups (F20/S20 at 20°C and F10/S10 at 10°C). Selected F and S mussels were then exposed to three experimental temperatures (10, 15, and 20°C), and the time-course of their response, in terms of clearance rate (CR: L/h) and routine oxygen consumption (VO2: mLO2/h), was monitored. The overall growth rate of mussels in the warm treatment group was significantly higher than in the cold treatment group. For both treatments, significant differences were found in key physiological parameters between F and S mussels: F mussels had a higher CR and a larger gill surface area than their S counterparts. Although no significant differences in the thermal sensitivity of the clearance or metabolic rates were observed between F20 and S20 mussels reared at 20°C, when exposed to acute temperature changes, experiments with mussels reared at 10°C revealed a different outcome: in response to acute warming (from 10°C to 15 and 20°C), F10 were capable of compensating for the thermal effect on CR and VO2; however, no such compensatory response was observed in S10. We conclude that two significant factors contribute to endogenous differences in the growth rate of mussels: (i) the capacity to exhibit intense filtering activity, which appears to be functionally correlated with the gill surface area and (ii) the capacity to compensate for the effects of temperature on filtration and metabolic rate. The second trait does not appear to make a significant contribution to the inter-individual size-differentiation observed in mussels maintained in warm environments, but explains a significant proportion of inter-individual growth rate differences in cold environments.
Introduction
The physiological mechanisms underlying differences in inter-individual growth rate in bivalves have been thoroughly analyzed in terms of physiological energetics in a wide variety of studies covering a broad range of species and environmental conditions (Toro and Vergara, 1998; Bayne, 1999, 2004; Bayne et al., 1999a,b; Toro et al., 2004; Pace et al., 2006; Pernet et al., 2008; Tamayo et al., 2011, 2015). Those works reported several differences between fast- and slow-growing individuals, including differential feeding rates, metabolic efficiency, and energy allocation patterns. Such heterogeneity indicates that, rather than being associated with innate differences in a single physiological trait, inter-individual differences in growth rate are caused by differences in multiple physiological processes. A series of studies performed in our laboratory showed that the environmental conditions, and particularly trophic conditions, prevailing during the rearing period determine which physiological parameters contribute to differences in the inter-individual growth rates of the mussel Mytilus galloprovincialis (Tamayo et al., 2016; Prieto et al., 2018, 2019). When fed with a constant supply of either high- or low-quality food, fast growers differ from slow growers in their capacity to exhibit significantly higher CR, a feature that appears to be functionally linked to the possession, or development, of larger gill surface area (Prieto et al., 2018, 2019, 2020). However, under severe feeding restrictions, fast growers differ from slow growers in their capacity to develop a lower standard metabolic rate (SMR) during periods of starvation. Such results led Prieto et al. (2018) to define two basic phenotypes for fast-growing bivalves: fast feeders and energy savers.
Together with food availability, water temperature strongly affects growth rate in ectothermic organisms, including bivalves (Newell et al., 1977; Buxton et al., 1981; Kang et al., 2016); however, very few studies have analyzed the possibility that inter-individual differences in capacity for thermal compensation of physiological processes could contribute to differences in inter-individual growth rate. Early studies by Hawkins (1985) and Hawkins et al. (1987) analyzed the protein turnover and energy balance of Mytilus edulis acclimated to 10°C, after warming them to 20°C. These authors found that the individuals that grew faster had slower protein turnover; this resulted in a lower metabolic rate and greater homeostatic ability under conditions that cause a rise in temperature. These authors concluded that (i) the capacity for thermal compensation with regards to metabolic rate has a significant effect on the rate of protein turnover in a given individual, and (ii) faster protein turnover rates constrain the scope for activity, thus resulting in a short thermal range for positive energetic scope for growth in the mussel. In another study, Pernet et al. (2008) analyzed the capacity for thermal adaptation in a genetically distinct group of oyster, Crassostrea virginica, showing clear differences in growth rate. These authors analyzed membrane lipid composition, and energy budget, following acclimation to different water temperatures (4, 12, and 20°C), and found that individuals from fast-growing genetic lines exhibited lower SMRs; this was attributed to the reduced unsaturation indexes of membrane lipids. Tamayo et al. (2013) measured the short and medium-term physiological response of fast (F)- and slow (S)-growing clams (Ruditapes philippinarum) following exposure to cold (10°C) and warm (24°C) water temperatures, and found that the growth rate of the S group was constrained by the significantly higher thermal dependency of the metabolic expenditures which was found to promote a larger increase in the routine metabolic rate (RMR) at warm temperatures.
The aim of the present study was to ascertain if the capacity for acute and chronic compensation for the effects of temperature change on physiological rates could potentially contribute to differences in inter-individual growth rates in the mussel M. galloprovincialis. To this end, we reared juvenile mussels in the laboratory at 20 and 10°C until clear inter-individual size-differentiation allowed us to select fast- (F) and slow-(S) growing individuals from each rearing temperature. Selected F and S individuals were then used in a series of experiments to (i) compare the physiological profiles of F and S mussels under different thermal regimes, and (ii) analyze the acute effects of temperature change on the filtering activity and metabolic rate of F and S individuals.
Materials and Methods
Collection and Selection of Mussel Seeds
Mussel seeds (about 500) of M. galloprovincilis were collected in April 2015 from monolayer mussel beds growing in a rocky intertidal area located in Antzoras (Biscay, Spain, 43°24′29.1″N; 2°40′51.0″W), from a mussel population that has been used in our previous publications (Prieto et al., 2018, 2019, 2020). Once at the laboratory, mussels were maintained immersed in tanks where seawater salinity (33PSU) and temperature (15°C) resembled those measured at the mussel collection point, and were fed Isochrysis galbana during the time (days) needed for checking that mussels were apparently healthy and active, and selecting those to be used in later experiments. During this conditioning period, the shell length of each mussel was measured with electronic calipers and 300 homogenously sized individuals (shell length: 10.65 ± 0.56 mm and live weight: 0.2 ± 0.04 g) were selected to perform the experiments.
Experimental Design
The selected 300 mussels were divided into two groups of 150 individuals that were reared separately in two tanks, under two different maintenance temperatures, 20 and 10°C. We chose those two temperatures for being close to the maximum and minimum seasonal values recorded in their natural environment (Ibarrola et al., 2008; Albaina et al., 2009; Aravena et al., 2009). All other conditions, with the exception of temperature, were identical during the rearing period: seawater (33PSU) was continuously aerated and mussels were fed a high organic content diet (approximately 70%) consisting of cells of our own cultures of I. galbana (T-Iso) mixed with Shellfish Diet® (a commercial mix of four microalgae: Isochrysis, Pavlova, Tetraselmis, and Thalassiosira weissflogii) and pre-sieved particles of natural silt. The diet was continuously pumped to the seawater tanks from concentrated stocks using peristaltic pumps that were set to provide for a stable food concentration of 1.5 mm3/L in the tank. Frequent monitoring of particle concentration in the tanks with a Multisizer 3 Coulter Counter (Beckman Coulter) allowed regulating diet pumping rates. To avoid accumulation of ammonia, the seawater in the tanks was totally renewed twice per week. During the water renewal the tanks were rinsed with running tap water and the mussels were pulled apart from one another by carefully cutting the byssus. Such a procedure avoid mussel clustering, thus, ensuring the total absence of inter-individual competition for food.
Additionally, once per 2–3 weeks, the size of individual mussels was determined by measuring the shell-length using electronic calipers (accurate to 0.05 mm) and the live weight by using a 10–5g precision balance. Mussels were maintained under these constant conditions until clear inter-individual size differences were observed (at 2 and 5 months for mussels maintained at 20 and 10°C, respectively) (Figure 1). After this long-lasting rearing period, we selected in each acclimation temperature the 30 smallest and the 30 biggest mussels that were considered to represent, respectively, the slow (S) and fast (F) growing phenotypes. Accordingly, four experimental groups of mussels were created: fast growers selected at 20°C (F20), slow growers selected at 20°C (S20), fast growers selected at 10°C (F10), and slow growers selected at 10°C (S10). Mussels from these four groups were then used for thermal experiments that were specifically designed to analyze the acute effects of temperature change on physiological performance (Figure 1).
Thermal Experiments With Selected F and S Mussels
The selected F and S mussels from warm (F20 and S20) and cold (F10 and S10) treatments were exposed to three experimental-temperatures (Tex: 10, 15, and 20°C). Mussels were submitted to the experimental temperatures without any conditioning period, this is, the warm treated selected mussels were divided into three groups and were directly immersed at the corresponding experimental temperature: one group at 10°C, another one at 15°C and the last one at 20°C. The same protocol was used for the cold treated selected mussels, that were also split into three experimental groups.
At any Texp, we monitored the time-course of the clearance rate (CR) and RMR until stable values were recorded. As a rule, CR was determined daily (we started monitoring the CR just after the first hour of exposition to any temperature change) and RMR once every 2 days. We could not set in advance the length of the monitoring period, being dependent on the response shown by the experimental mussels, so we extended it until observing constancy; it took between 8 and 22 days for the different experimental conditions. Once feeding and metabolic constant responses were observed at each experimental temperature, food delivery was stopped and the reduction in metabolic rate in starved mussels was analyzed by monitoring metabolic rate until it was observed to reach to a stable minimum value that was considered to represent the SMR.
Physiological Measurements
Clearance Rate
In order to determine clearance rates, we placed five individuals (n = 5) from each mussel group (F20, S20, F10, and S10) on to filtration chambers. These chambers were 150 mL borosilicate glass bottles, with inflow and outflow lines drilled into the plastic lid. Water from a thermostatic feeding tank containing the experimental diet was pumped through the chambers by means of multichannel peristaltic pumps that were regulated to produce flow rates that reduced the particle concentration inside the chambers by 15–30%. A mixture of I. galbana (T-Iso), and silt particles (approximately 2.5:1) was pumped into the feeding tanks (from concentrated stock solutions) using peristaltic pumps at rates that were set to provide a constant concentration of 20,000 particles per mL (approximately 1.5–2 mm3/L). Particle concentration in the feeding tanks was maintained at stable levels by frequently checking the concentration with a particle counter (Coulter Multisizer 3; Beckman Coulter Spain, Barcelona).
Clearance rate (CR; L/h) was measured according to the formula described by Hildreth and Crisp (1976) [CR = F × ((Ci-C0)/Ci)], in which “F” was the flow rate (L/h), “Ci” was the particle concentration in the control outflow, and “C0” was the particle concentration in the experimental chamber outflow. The concentration of particles was measured using a Coulter Counter Z1 (Beckman Coulter Spain, Barcelona). The daily clearance rate recorded for each individual was defined as the mean value of measurements taken every hour for a total period of 11–12 h.
Oxygen Consumption
Both RMR and SMR were determined as rates of oxygen consumption (VO2: mL O2/h). To determine these rates, mussels were removed from the feeding chambers and introduced into 150 mL chambers that had been sealed with luminescent dissolved oxygen (LDO) oxygen probes connected to oximeters (HATCH HQ40d; Hach Lange Spain, Derio). The oxygen consumption values were obtained from the calculation of the rate of decrease of the oxygen dissolved in the water contained in the respirometers over time by using a linear regression. Oxygen concentration was monitored every 5–10 min until values showed a reduction of 20–30% of the initial baseline values. A control chamber was used to determine the stability of oxygen concentration.
The difference between RMR (at the onset of starvation) and SMR (the reduction of metabolic rate during starvation), was defined as the metabolic scope for feeding and growth (MSFG).
Energy Balance
To identify potential differences in the physiological basis underlying differences in inter-individual growth rates between mussels reared at different water temperatures, we determined the complete set of parameters needed to calculate energy balance in selected F and S individuals at their corresponding rearing temperature (i.e., at an exposure temperature of 20°C for F20 and S20 mussels, and at 10°C for F10 and S10 mussels). To this end, we collected samples of water and feces from mussels during the feeding period. Water samples were filtered onto pre-washed/pre-weighed GF/C glass-fibber filters, and subsequently processed to determine the concentrations of total particle matter (TPM) (mg/L), inorganic particulate matter (PIM) (mg/L), and organic particulate matter (POM) (mg/L). Retained salts were rinsed out with a solution of ammonium formate (0.9%). TPM and PIM were then estimated as the increment in dry and ash weight on the filters, respectively. POM was then calculated as the difference between TPM and PIM. The organic content of food (f) was then estimated using a specific formula (f = POM/TPM).
The ingestion rate of organic matter by individual mussels (OIR; mg POM/h) was also calculated using a specific formula (OIR = CR × POM).
Samples of feces were filtered onto pre-washed/pre-weighed GF/C glass-fibber filters and processed in the same way as water samples to determine total matter, inorganic matter, and organic matter in the feces. The organic content of fecal matter (e) was estimated as the ratio of organic/total matter. We then calculated the absorption efficiency (AE) (decimal units) using the Conover (1966) expression (AE = (f − e)/(1 − f) × e), in which “f” and “e” represented the organic content of food and feces, respectively.
Once AE had been determined, we calculated the individual absorption rate (AR) (mg/h) using a specific formula (AR = OIR × AE). The resulting scope for growth (SFG) (J/h) was then determined as the difference between absorbed energy (AR: J/h) and metabolic expenditure (RMR: J/h). AR (mg/h) was transformed into energetic values (J/h) using an energy equivalence of 18.75 J/mg (Whyte, 1987). Oxygen consumption (VO2) was transformed into energy values using an oxycaloric coefficient of 20.08 J/mLO2 (Gnaiger, 1983).
Size Standardization
Physiological rates are expressed in terms of live weight. CR and oxygen consumption were standardized to a common live weight of 1 g, according to a formula described previously (Bayne and Newell, 1983) (YSTD = (1/WEXP)b × YEXP) in which YSTD and YEXP represent standard and experimental physiological rates, respectively, and WEXP represents the experimental weight. The power values used to scale physiological rates to body weight (b) for clearance rate and oxygen consumption were 0.58 (Bayne and Hawkins, 1997) and 0.724 (Bayne et al., 1973).
Thermal Dependency of Physiological Parameters
Thermal dependency of physiological rates was determined according to the Van’t Hoff equation [Q10 = (Y1/Y0) (10/(T1–T0))], in which Y0 and Y1 represented the physiological rate determined at T0 and T1 temperatures, respectively. The resulting Q10 represents the rate of variation of the measured physiological parameter as a consequence of a 10°C increase in the water temperature.
Determination and Standardization of Gill Surface Area (GA, mm2)
After the experiments were completed, 15 individuals per experimental group were dissected and placed on graph paper to allow sizing. A photograph of the internal tissues of each mussel was taken with a digital camera, and the surface area of the gills from each individual was calculated using ImageJ software (National Institutes of Health). All mussels were visually healthy and no injuries were detected. The data given herein correspond to one side of a demibranch. Gill areas were standardized for an equivalent 1 g of mussel live weight according to a specific formula [GASTD = (1/WEXP)b × GAEXP], in which GASTD and GAEXP represent the standardized and experimental gill area, respectively, and WEXP represents the experimental live weight of the mussel. The power function used to scale gill area to live weight was 0.66 (Vahl, 1973; Hawkins and Bayne, 1992; Jones et al., 1992).
Statistical Analysis
Significant differences in the growth rate of mussels during the rearing period at warm (20°C) and cold (10°C) temperatures were identified by testing the slopes of linear regressions (least-squares method) for the mean shell length of mussels (Y) vs. time (X). Slopes and elevations were compared between linear regressions by analysis of covariance (ANCOVA) as described by Zar (2010).
Significant differences in the time-course of thermal adaptations (of CR and VO2) between F and S mussels were analyzed using a repeated measurements two-way analysis of variance (ANOVA). Time was denoted as exposure time when measurements were performed under feeding conditions (CR and VO2R measurements), and as starvation time when VO2 was measured in starved mussels. Prior to statistical analysis, we tested the normality of data using the Shapiro–Wilk test. Data sphericity was tested with the Mauchly test. Accordingly, subsequent analysis was carried out with either a univariate approach (assumed sphericity test), or a multivariate approach (Pillai’s trace test). Multiple comparisons were carried out for all physiological parameters with the least significant difference (LSD) test.
The effect of growth condition (being F or S) and acclimation temperature (being reared at 10 or 20°C) on the surface area of the gills, and the physiological parameters that determine energy budget in mussels held at different rearing temperatures (F20 and S20 at 20°C; F10 and S10 at 10°C) were analyzed by two-way ANOVA. Homogeneity of variance was evaluated with Levene’s test; the Games-Howell, or Tukey test, was applied for multiple comparisons, as appropriate. All statistical analyses were performed using IBM SPSS Statistics for Windows, Version 19.0 (IBM Corp. Released 2010. Armonk, NY: IBM Corp.).
Results
Growth Rates
The growth rates of experimental mussels reared at acclimation temperatures of 20 and 10°C were calculated by adjusting mean shell-length (mm) values to linear regression models. The resulting equations were as follows:
Analysis of covariance results revealed significant differences for both slope and elevation between mussels reared at 20°C and those reared at 10°C (Slope test: t = 14.28, df = 1, 14, p < 0.05; elevation test: t = 10.05, df = 1, 8, p < 0.05). The growth rate of mussels grown at 20°C was almost three-fold higher than that of mussels reared at 10°C. Accordingly, the size differentiation between individuals occurred earlier in the warm treatment (20°C) group than in the cold treatment (10°C) group (60 vs. 150 days, respectively). Table 1 shows the body size (shell length and live weight) and growth rates (g/day and mm/day) of selected F and S individuals from the two different acclimation temperatures (F20 and S20 in the warm treatment; F10 and S10 in the cold treatment). F individuals grew significantly faster, and were approximately 100% heavier and 50% longer, than S individuals; this was the case at both acclimation temperatures.
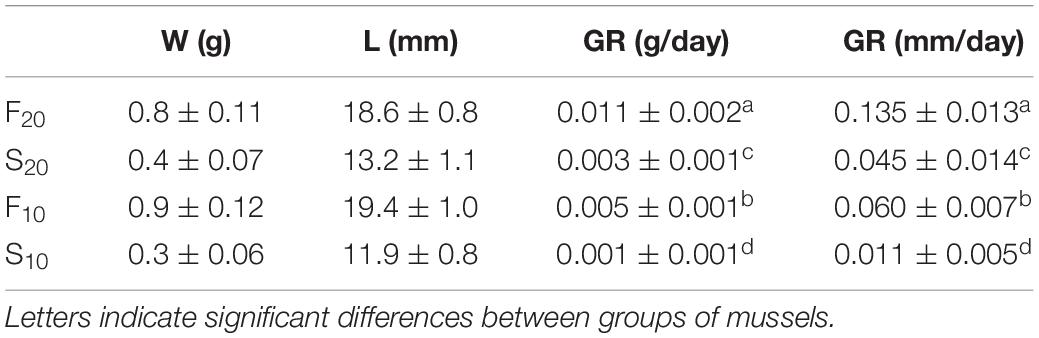
Table 1. Shell-length (L, mm), live weight (W, g), and growth rates (GR, g/day and mm/day) in mussels selected as fast- and slow-growers (n = 15) after 60 and 150 days of maintenance at 20 and 10°C, respectively (mean values ± SD).
Monitoring Changes in the Clearance Rate and Oxygen Consumption of F and S Mussels at Different Exposure Temperatures
Mussels Reared at 20°C
TEXP at 20°C
Figures 2A,B show the CR and oxygen consumption for F20 and S20 at 20°C, respectively. A summary of the results derived from repeated measurements two-way ANOVA with regards to the effects of growth condition (F vs. S), and exposure time, on various physiological parameters is provided in Table 2. F20 mussels had a significantly higher CR than S20 mussels, indicating that growth condition exerted significant effects on CR (Table 2). Exposure time and interaction did not have any significant effects on CR. The mean CR of F20 and S20 mussels was 0.46 ± 0.11 and 0.25 ± 0.07, respectively. VO2R was determined on three occasions (Figure 2B); there were no significant differences between F and S mussels (0.067 ± 0.006 and 0.064 ± 0.002 mLO2/h, respectively). Starvation time had a significant effect on oxygen consumption, inducing a 35% reduction of VO2 in both groups of mussels.
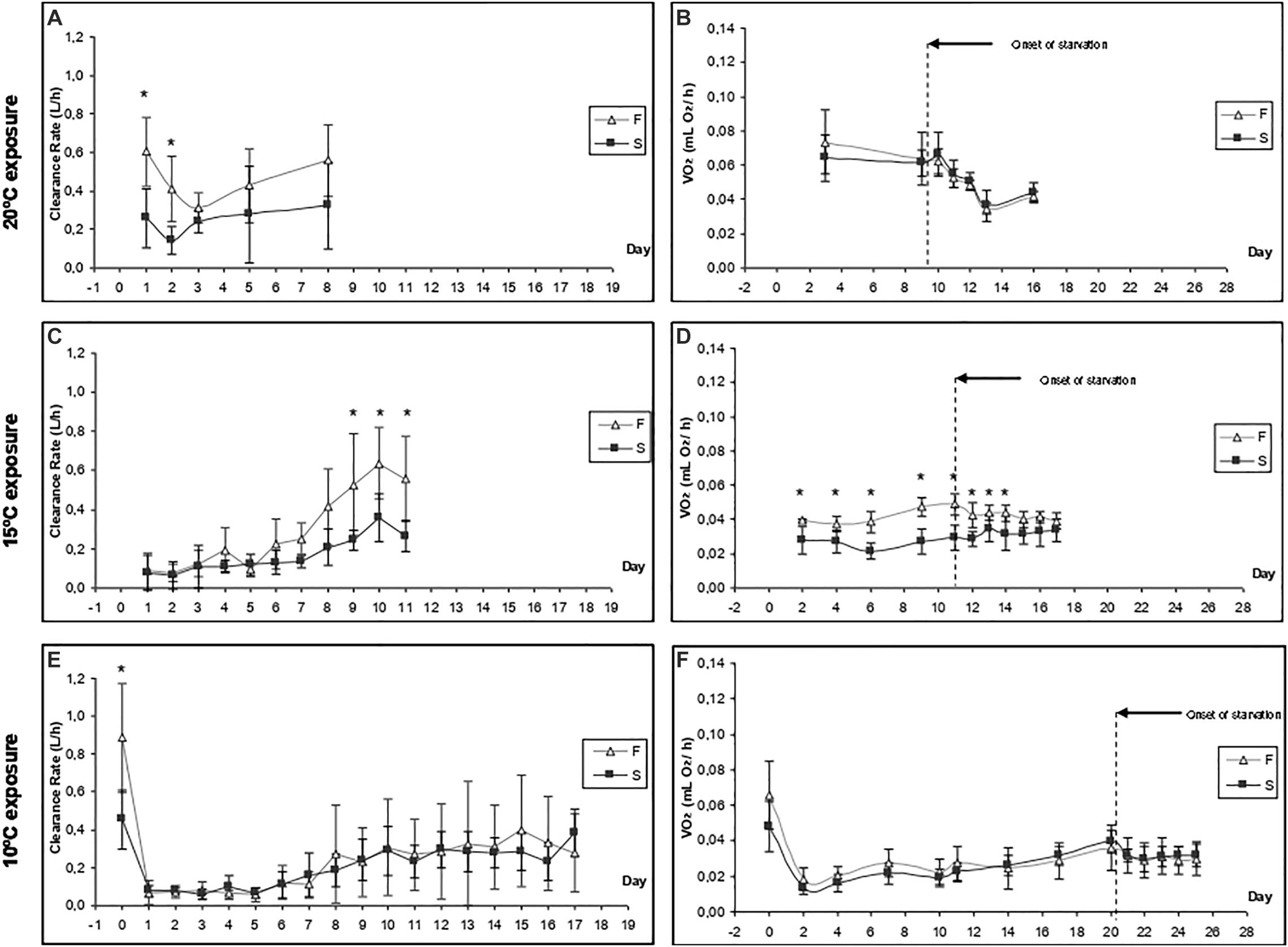
Figure 2. Time-course of the clearance rate (A,C,E) and oxygen consumption (B,D,F) of F20 and S20 mussels at three exposure temperatures (20, 15, and 10°C). Five individuals from each group were monitored in each experimental condition. Asterisks indicate significant differences between fast- and slow-growing individuals (ANOVA, p < 0.05).
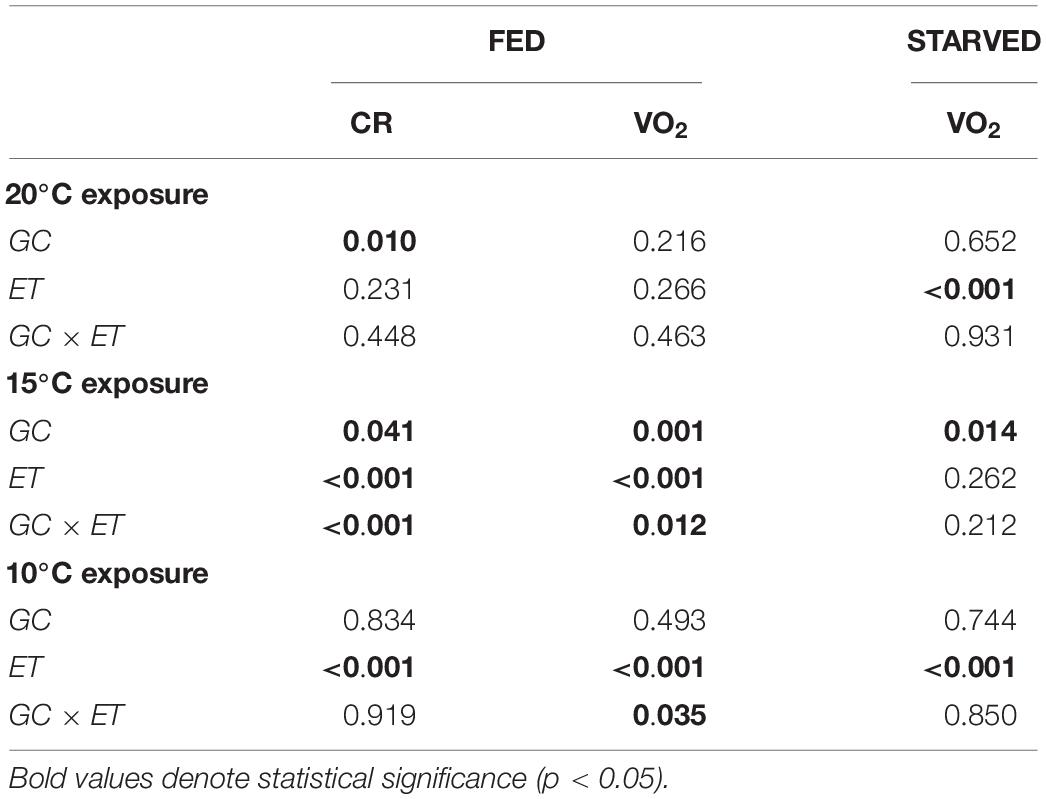
Table 2. Two-way factor ANOVA (repeated measurements) showing the effects of growth condition (GC, F, or S) and exposure time (ET, days) on clearance rate (CR, L/h) and oxygen consumption (VO2, mLO2/h) in fed and starved mussels reared at 20°C.
Response to Cooling at 15°C
When F20 and S20 mussels were exposed to a temperature of 15°C, there was a substantial reduction of CR (Figure 2C) compared with the CR at 20°C. During the first 6 days of exposure to 15°C, there was no significant difference in CR values between the two groups (0.1–0.2 L/h). By day 7, the CR had started to increase in both groups; however, there was a larger increase in the CR of F20 mussels (0.558 ± 0.220 L/h at day 11) than their slow-growing counterparts (0.264 ± 0.081 L/h at day 11); this resulted significant differences between the two groups over the last 3 days of the experimental period (p < 0.05). Accordingly, growth condition, exposure time, and the interaction of these factors exerted significant effect on the CR of mussels (Table 2). Although no initial values were measured on day 0, the CRs of both F20 and S20 mussels on the last few days of the experimental period were similar to the CRs of mussels at 20°C.
Routine metabolic rate was lower at 15°C than at 20°C (Figure 2D); this was the case for both F and S individuals. Throughout the entire exposure period, F20 mussels exhibited a significantly higher RMR than S20 individuals. In other words, growth condition had a significant effect (Table 2). Moreover, F20 and S20 mussels showed differential patterns in terms of VO2R variation: in F individuals, VO2R increased from 0.039 ± 0.001 (day 1) to 0.049 ± 0.006 mL O2/h (day 11), whereas S individuals maintained their RMR at constant values (0.028 ± 0.008 on day 1; 0.029 ± 0.007 mL O2/h on day 11). Exposure time, and interaction, thus exerted a significant effect on the RMR of mussels when transferred from 20 to 15°C (Table 2). Starvation time did not induce a significant reduction in oxygen consumption, and thus, only growth condition exerted a significant effect upon standard VO2.
Response to Cooling at 10°C
A change in temperature to 10°C caused a severe reduction of CR in both groups of mussels (CR fell to approximately 0.07 L/h). CR began to increase between the 7th and 10th days of exposure, reaching approximately 0.3 L/h by the end of the experiment. No significant differences were identified between F20 and S20 mussels. ANOVA identified a significant effect for exposure time, but not for growth condition (Table 2). With regards to VO2, (Figure 2F), exposure to 10°C resulted in a reduction of metabolic rate by more than 70%; this effect occurred irrespective of growth condition. Subsequently, the RMR of both F20 and S20 mussels increased, and following a similar trend to that of CR. Accordingly, ANOVA revealed that exposure time exerted a significant effect on routine VO2. The metabolic rate was slightly higher in S individuals (from 0.013 ± 0.004 to 0.039 ± 0.006 mL O2/h) than in F individuals (from 0.019 ± 0.006 to 0.036 ± 0.013 mL O2/h), and thus, the interaction (exposure time × growth condition) also exerted a significant effect (p = 0.035; Table 3). During the period of starvation, the reduction in SMR was similar in both mussel groups; therefore, only starvation time exerted a significant effect on oxygen consumption.
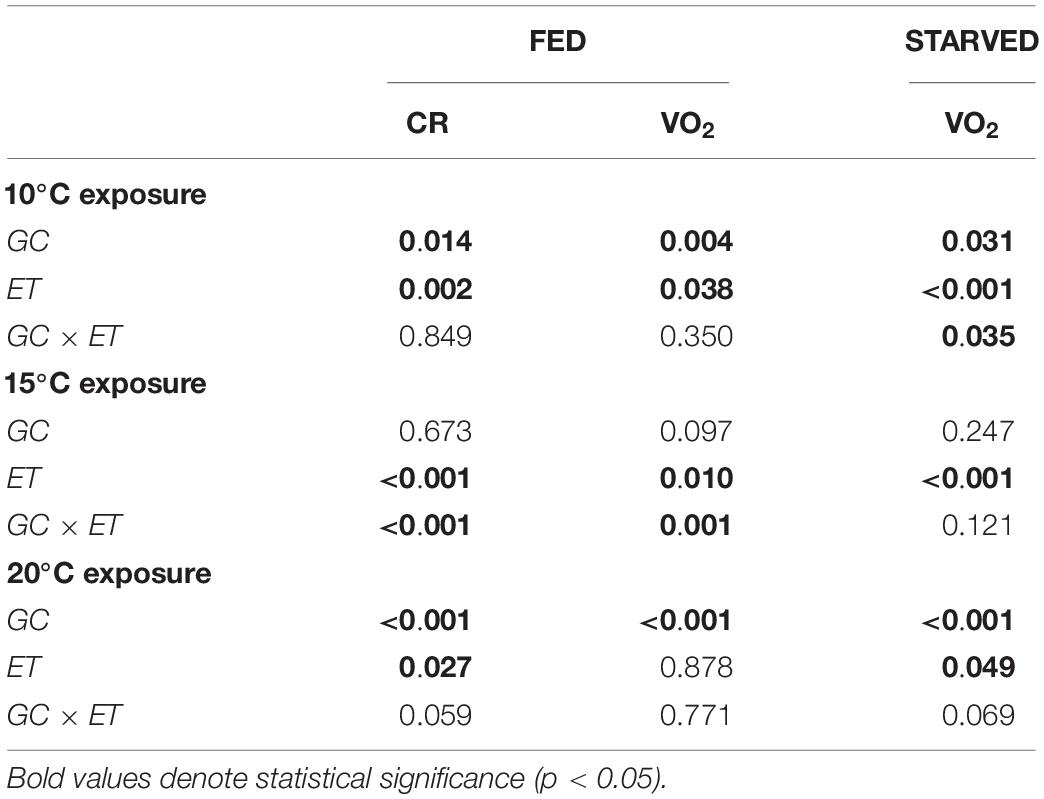
Table 3. Two-way factor ANOVA (repeated measurements) showing the effects of growth condition (GC, F, or S) and exposure time (ET, day) on clearance rate (CR: L/h) and oxygen consumption (VO2: mLO2/h) in fed and starved mussels reared at 10°C.
Mussels Reared at 10°C
TEXP of 10°C
Figures 3A,B show the CR and VO2 for mussels grown at 10°C and maintained at 10°C, respectively. A summary of the results derived from two-way ANOVA with regards to the effects of growth condition (F vs. S) and exposure time on various physiological parameters is provided in Table 3. CR was significantly higher in F10 (Mean CR = 0.384 ± 0.100 L/h) mussels than in S10 mussels (Mean CR = 0.141 ± 0.070 L/h), suggesting that growth condition exerted a significant effect (Table 3). A slight, but significant, temporal change in CR was also observed; in other words, exposure time exerted a significant effect). With regards to RMR, there were no significant temporal changes identified, and F10 mussels had a significantly higher metabolic rate than S10 mussels (Mean RMR = 0.053 ± 0.002 and 0.020 ± 0.001 mL O2/h, respectively). Thus, only growth conditions exerted a significant effect (Table 3). During starvation, F10 mussels exhibited significantly lower oxygen consumption; however, the VO2 of S10 individuals did not change significantly. Consequently, our analysis showed that growth condition and starvation time both had significant effects (Table 3).
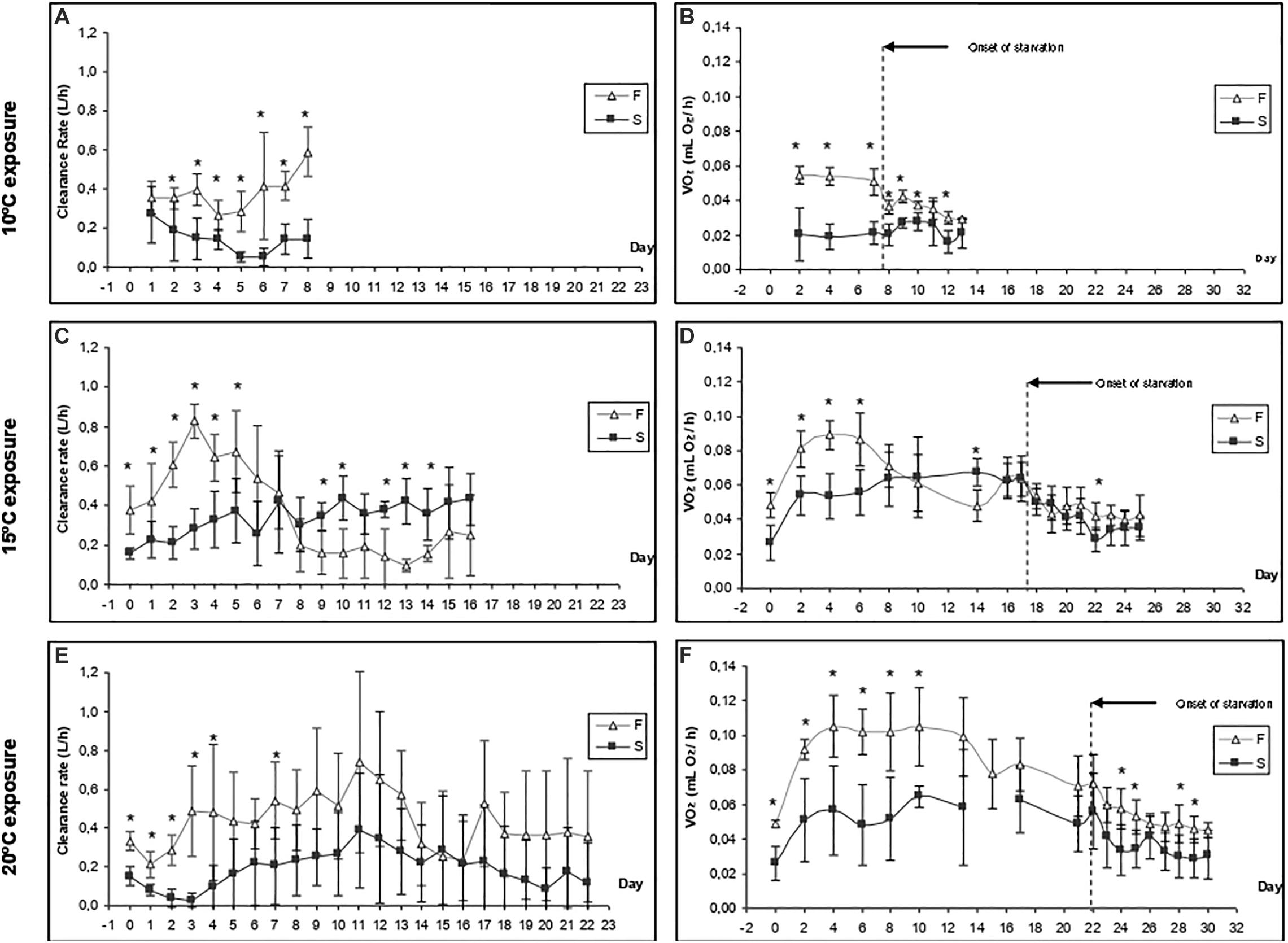
Figure 3. Time-course of the clearance rate (A,C,E) and oxygen consumption (B,D,F) of F10 and S10 mussels at three exposure temperatures (20, 15, and 10°C). Five individuals from each group were monitored in each experimental condition. Asterisks indicate significant differences between fast- and slow-growing individuals (ANOVA, p < 0.05).
Response to Warming at 15°C
The change in CR after increasing the water temperature to 15°C differed between F10 and S10 individuals (Figure 3C). The CR of F10 mussels increased during the first 3 days (from 0.378 ± 0.120 at day 0 to 0.828 ± 0.085 L/h; a Q10 of 4.79), and then a compensatory reduction occurred until day 8 (0.198 ± 0.136). Subsequently, CR was maintained at similar values until the end of the experiment on the 16th day. In contrast, the CR of S10 mussels increased continuously during the feeding period (from 0.157 ± 0.026 to 0.433 ± 0.131 L/h; a Q10 of 7.58). Therefore, F10 mussels had a significantly higher CR than S10 individuals only until the 5th day of experimentation. Then, because of the compensatory reduction of CR in F10 mussels and the increase of CR in S10 mussels, the CR turned to be higher in S10 individuals (significant differences were found at days 9, 10, 12, 13, and 14). Accordingly, exposure time and interaction exerted a significant effect on the CR of mussels; however, growth condition had no effect (Table 3).
Warming to 15°C induced a sudden and intense increase of routine VO2 in both F10 and S10 mussels (Figure 3D). In F10 mussels, RMR increased from 0.048 ± 0.007 to 0.089 ± 0.009 mL O2/h by day 4 (representing a Q10 of 3.43). In contrast, in S10 mussels, the VO2R increased from 0.026 ± 0.010 to 0.053 ± 0.013 mL O2/h by day 4 (representing a Q10 of 4.15). F10 mussels maintained a significantly higher routine VO2 than the S10 mussels until day 6. Thereafter, consistent with the trends for CR, routine VO2 decreased in the fast-growing mussels (Q10 between the last day of feeding and the initial day = 1.77). Because the RMR of S10 mussels was maintained at constant values, significantly higher oxygen consumptions were recorded in S10 individuals at the end of the feeding period. Accordingly, exposure time and interaction between the tested factors exerted significant effects on routine VO2. Starvation promoted a similar reduction in the VO2 of both groups of mussels; consequently, only starvation time had a significant effect on standard VO2.
Response to Warming at 20°C
As an initial response to warming, mussels showed a reduction in CR (Figure 3E). Subsequently, CR increased daily until day 11. Although the CR values of F10 mussels were higher throughout the experiment, the differences between groups were only significant for the first 4 days, and on day 7. Although the intra-group variability in CR increased markedly from day 4, we found that growth condition exerted a significant effect on CR. Exposure time also had a significant effect on CR; however, the interaction term had no significant effect.
In contrast to the trend exhibited by CR, the initial response to warming led to a significant increase in RMR (Figure 3F); this was the case for both F10 and S10 mussels. By day 4, VO2R values were double those measured on day 0; in the F10 group, VO2 increased from 0.049 ± 0.002 to 0.105 ± 0.018 mL O2/h (Q10 = 2.14), while for the S10, VO2 increased from 0.026 ± 0.010 to 0.057 ± 0.026 mL O2/h (Q10 = 2.19). In the F10 mussels, oxygen consumption fell to 0.072 ± 0.017 mL O2/h by the end of the feeding period (Q10 between the last day of feeding and day 1 was 1.77). Both growth condition and exposure time exerted a significant effect on routine VO2. The onset of starvation induced a reduction of VO2 in F10 mussels (from 0.72 to 0.56 mL O2/h) and in S10 mussels (from 0.41 to 0.30 mL O2/h). F10 mussels exhibited a significantly higher SMR throughout the starvation period. Growth condition, starvation time, and their interaction all exerted significant effects on SMR.
Thermal Effects on the Metabolic Scope for Feeding and Growth
Figure 4 shows the mean RMR (recorded immediately before starvation) and mean SMR (recorded at the end of the starvation period) for the four experimental groups of mussels (F20, S20, F10, and S10) at each of the three experimental temperatures (10, 15, and 20°C). The Q10 values for standard and routine oxygen consumption are also indicated in Figure 4. Short-term changes in the exposure temperature promoted differential effects upon both RMR and SMR in fast- and slow-growing mussels, and the resulting MSFG. Figure 4 shows that irrespective of rearing temperature, F mussels displayed higher MSFGs than S mussels in the range of temperatures tested. For both S10 and S20 mussels, the MSFG was almost negligible at low temperatures.
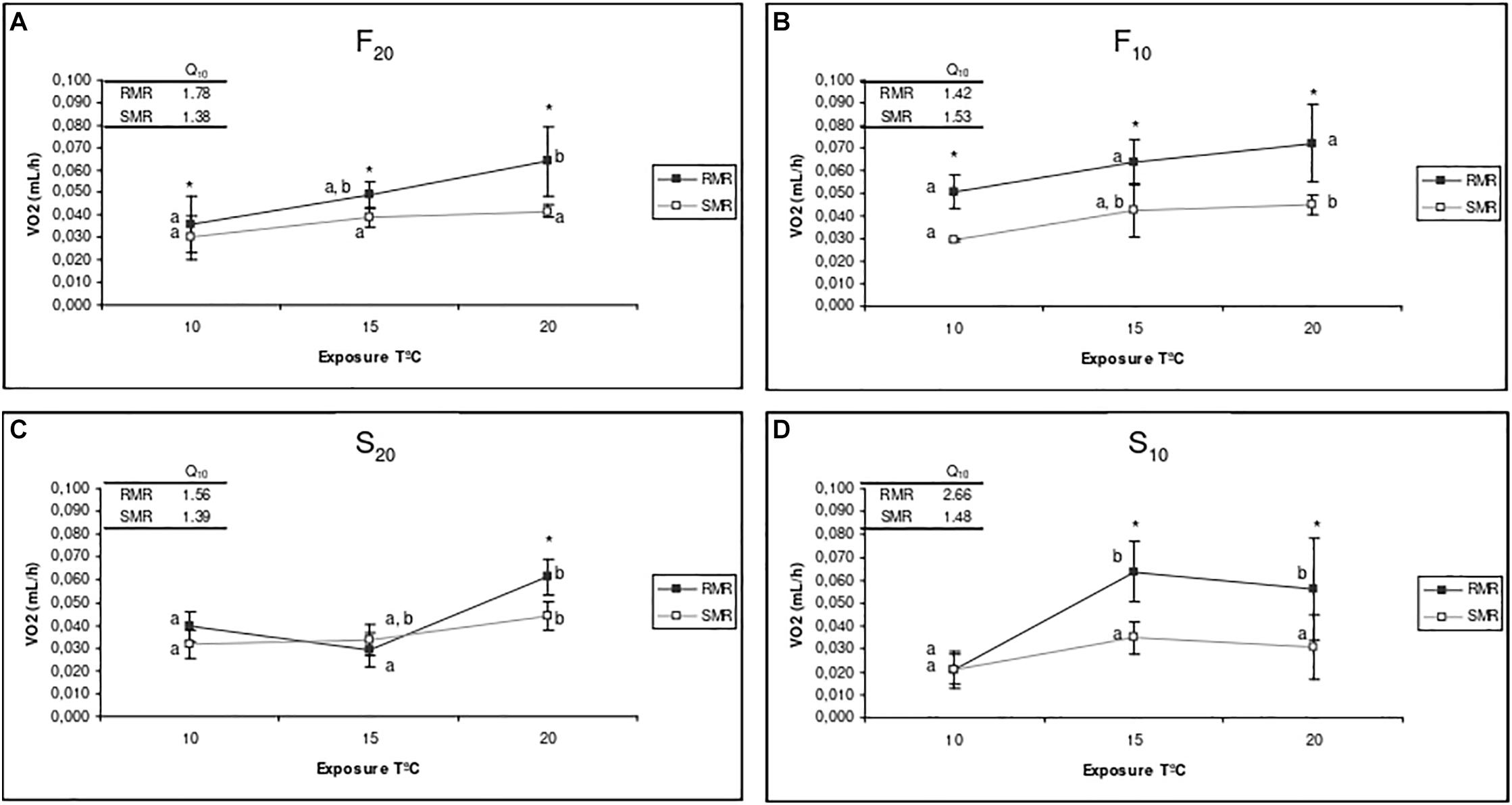
Figure 4. Routine metabolic rate (RMR) and standard metabolic rate (SMR) of F20 (A), F10 (B), S20 (C), and S10 (D) mussels at exposure temperatures of 10, 15, and 20°C. Mean value and standard deviation of five individuals from each group are shown for each experimental condition. The upper side of each graph shows the Q10 values for RMR and SMR in the range 10–20. Asterisks indicate significant differences between RMR and SMR (ANOVA, p < 0.05). Letters indicate significant differences between exposure temperatures for each physiological parameter (p < 0.05).
Energy Balance of Fast- and Slow-Growing Mussels Reared at 20 and 10°C
The physiological components of energy balance for F20, S20, F10, and S10 mussels exposed to their respective rearing temperatures are shown in Table 4, together with the surface areas of gills in 15 individuals from each mussel group. The effects of growth condition and acclimation temperature on the physiological components of energy balance were analyzed by two-way ANOVA (Table 4).
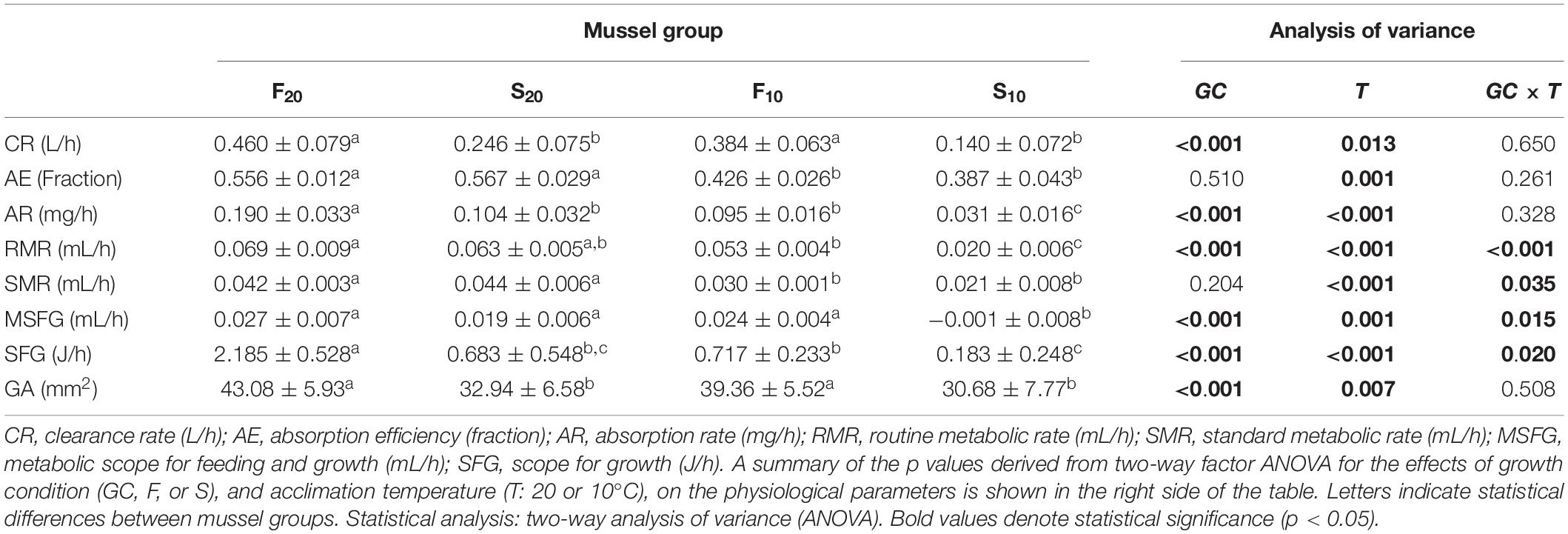
Table 4. Physiological parameters determining the energy budget and gill surface area (GA, mm2) in mussels maintained at 10 and 20°C.
Without exception, all parameters were significantly higher at 20°C than at 10°C; thus, acclimation temperature exerted a positive and significant effect on all parameters. Growth condition exerted a significant effect on all physiological variables except for absorption efficiency and SMR. F mussels had a significantly higher clearance rate, absorption rate, and gill surface area, than their S counterparts; this was the case at both 20 and 10°C acclimation temperatures. Thus, ANOVA detected significant effects for both growth condition and acclimation temperature, but not for their interaction. However, interaction did have a significant effect on metabolic parameters and SFG, thus indicating the existence of distinct patterns of inter-group differences at different acclimation temperatures. Indeed, post hoc analysis indicated that significant differences in RMR between F and S mussels only occurred in mussels reared at 10°C. In other words, the differences in RMR (and hence, in SFG) between F and S individuals were comparatively higher in mussels reared at 10°C. A similar pattern was also observed for SMR (ANOVA identified a significant effect for the interaction term): inter-group differences were higher in mussels reared at 10°C; this was due to the reduced SMR of S10 mussels.
Discussion
The differences in growth rate between mussels reared in the laboratory at 20 and 10°C (0.093 and 0.035 mm/d, respectively) indicate that the growth process in mussels is highly dependent on temperature (Q10 = 2.65). Positive correlations between growth rate and temperature in bivalves have been reported previously, particularly with regards to energy balance (Widdows, 1978; Bayne et al., 1983; MacDonald and Thompson, 1985; Beiras et al., 1995). In the present study, the physiological parameters of mussels reared at 20 and 10°C (Table 4) clearly illustrate the energetic basis for faster growth at higher temperatures: rising temperatures exerted a combined positive effect on energy gain processes (filtering activity and absorption efficiency) that was not overcome by the effect of temperature on their rates of energy expenditure (RMR). More interestingly, the results showed that faster growing individuals reared at 10°C (F10) grew slightly faster than slow-growing individuals reared at 20°C (S20) (Table 1). This indicates that endogenously determined inter-individual differences in growth potential exert an outstanding contribution to the intra-population variability in the size distribution; these effects have also been reported previously (Bayne et al., 1999a,b; Tamayo et al., 2011).
Fast-growing individuals exhibited significantly higher clearance rates than their slow- growing counterparts at both acclimation temperatures in good agreement with previous studies showing that filtration rate is the main physiological parameter underlying fast growing in different bivalves, such as mussels, (Pérez-Camacho et al., 2000; Fernández-Reiriz et al., 2016; Prieto et al., 2018) clams (Holley and Foltz, 1987; Tamayo et al., 2011, 2013) and oysters (Toro and Vergara, 1998; Bayne et al., 1999b; Pace et al., 2006; Tamayo et al., 2014). In good accordance with our previous studies (Tamayo et al., 2011; Prieto et al., 2018, 2020), the current analysis also shows that higher filtration rates in fast-growing mussels were associated with a significantly higher gill surface area than in slow-growers.
The present study also showed that rearing (or acclimation) temperature promotes a differential pattern of inter-individual differences in metabolic performance. At 20°C, there were no significant differences in routine or SMRs, or MSFG, between fast- and slow-growing individuals, thus suggesting that the main physiological features underlying inter-individual differences in growth potential were a higher clearance rate and a larger gill surface area. However, at 10°C, in addition to reduced CR and GA, S mussels also exhibited a lower RMR (the SMR was also reduced, although this was not statistically significant) and MSFG than F mussels. In ectothermic animals, a decrease in temperature induces a reduction in metabolic rate and attenuation of physical activity; these effects, which are due to a reduction in enzymic activity and changes in the structure of membrane phospholipids, lead to membrane dysfunction (Hochachka and Somero, 2002; Pernet et al., 2007). At critically low temperatures, the severe reduction of mitochondrial, ventilatory, and circulatory activities causes a limitation in the respiration that leads to a mismatch between oxygen delivery and oxygen demand (Pörtner, 2001, 2002; Anestis et al., 2010). Oxygen limitation triggers anaerobic metabolism and collapse of physiological function (Sommer et al., 1997; Frederich and Pörtner, 2000; Pörtner, 2001). Bivalves are able to compensate these thermal effects within their temperature-tolerance range by the activation of different tissue-specific homeokinetic mechanisms (Prosser, 1991) such as (i) the modification of the concentration of metabolic enzymes (quantitative changes), (Buckley et al., 2001; Lesser and Kruse, 2004; Fields et al., 2012) (ii) the induction of enzymes with different kinetic properties (qualitative changes), or, more typically, (iii) by remodeling membrane lipids (homeoviscous adaptation) (Pernet et al., 2006, 2007, 2008; Pörtner, 2010; Somero, 2010). The time-course and the degree of compensation for both clearance rate and oxygen consumption in bivalves has been the subject of considerable debate. Early studies by Widdows and Bayne (1971) show that M. edulis could completely counteract the acute thermal effects on both CR and VO2 within 14 days, when maintained within the temperature-tolerance range. Subsequent data have shown that the acclimation of clearance rate can be achieved faster, or that levels of compensation can be higher than those reached by oxygen consumption (Cusson et al., 2005; Dunphy et al., 2006; Resgalla et al., 2007), thus suggesting that the preservation of filtering activity is a critically important feature of thermal adaptation in bivalves.
In the present study, we showed that inter-individual differences in temperature compensation mechanisms contribute significantly to inter-individual variations in growth potential. Slow-growing individuals, selected at low temperature (S10), showed an extremely reduced ability for chronic thermal acclimation. This differential trait of S10 mussels is evident when their behavior is compared with that shown by F20 and S20 mussels in response to cooling to 10°C: both F20 and S20 mussels showed a dramatic reduction in both RMR and CR (to approximately 0.020 mL O2/h and 0.10 L/h, respectively) after being transferred to cold water (10°C). However, after 20 days of acclimation to the new thermal regime, the mussels in both groups exhibited increased oxygen consumption (0.040 mL O2/h), and clearance rate (0.3–0.4 L/h), and achieved considerably higher levels than the S10 mussels (0.021 mL O2/h and 0.14 L/h, see Table 4). In other words, S10 mussels failed to compensate for these thermal effects over a long-term period (5 months of rearing); in contrast, mussels reared at 20°C were able to overcome these thermal effects in just 20 days. Moreover, although the F20 and S20 mussels achieved a positive MSFG after 20 days of acclimation to 10°C, the S10 mussels exhibited only a negligible MSFG, thus suggesting that the capacity to supply O2 to the whole organism remained limited by cold exposure in these mussels. These findings indicate that energy needed for maintenance and/or to meet the cost of feeding must be acquired by alternative pathways, thus restricting the organism’s capacity to acquire food and grow.
Furthermore, the warming experiments performed in the present study with mussels reared at 10°C showed that, in contrast with fast-growers (F10), mussels selected as slow-growers in a cold environment (S10) lack the mechanisms for acute thermal compensation. An elevation of just 5°C (from 10 to 15°C) promoted a short-term increase in CR and RMR in both groups of mussels; fast growers were able to compensate for thermal effects by day 17, a time course that seems to be consistent with a homeoviscous adaptation response. In contrast, slow-growing mussels (S10) had increased CR and VO2 (from 0.2 to 0.4 L/h and from 0.025 to 0.070 mL O2/h by day 16, respectively) and showed no signs of compensation after 16 days of exposure. Similarly, we observed differential behavior between the groups after warming S10 and F10 mussels up to 20°C: in both cases, an initial increase in routine oxygen consumption coincided with a significant reduction in CR, suggesting that thermal stress elevates the metabolic rates to levels that surpass the aerobic capacity of mussels. In F10 mussels, an incremental increase in RMR from 0.049 to 0.092 mL/h by day 4 (Q10 = 1.87) was partially compensated for as RMR fell to 0.07 mL/h. In contrast, no such compensation was observed for the metabolic rate of S10 individuals, in which the initial period of CR inhibition lasted longer than in F mussels.
Thus, our results indicate that two main factors contribute to endogenous inter-individual differences in the growth rates of mussels: (1) the capacity to exhibit intense filtering activity; this appears to be functionally correlated with the gill surface area, and (2) the capacity to compensate for temperature effects on filtration and metabolic rate. The second trait appears not to be associated with the inter-individual size-differentiation observed in mussels that were reared in a warm environment (20°C), but explains a noteworthy proportion of inter-individual growth rate differences in a cold environment (10°C). Moreover, because S10 individuals lie within the 0–20 percentile of the population size distribution, it seems reasonable to conclude that a significant proportion of the individuals in the present mussel population did not possess effective molecular mechanisms to support thermal acclimation.
Pernet et al. (2008) observed that intra-specific variation in physiological and biochemical adaptation to temperature correlates well with inter-individual variation in growth rate differences in the oyster C. virginica. These authors further observed that selected lines of fast-growing individuals were capable of exhibiting reduced unsaturation index of membrane lipids in gill tissue in a more intense manner than observed in slow-growers. In their study, a higher capacity for homeoviscous adaptation in the gills of fast-growing oysters was associated with lower SMRs and higher clearance rates. Thus, Pernet et al. (2008) suggested that the innate capability for the thermal compensation of membrane fluidity represents a key mechanism underlying inter-individual differences in growth rates. We previously reported that that the gill tissue of S mussels, compared with that of F mussels, showed higher expression levels of genes that play roles in the immune system, cellular stress, and anaerobic metabolism, but lower expression of genes involved in ciliary activity, growth processes, extracellular matrix production, and maintenance of aerobic metabolic pathways (Prieto et al., 2019). These transcriptomic differences concur with differences in the clearance rate and gill surface area between F and S mussels, and therefore indicate reduced functional capacity in the gills of slow-growing mussels. In conclusion, two physiological processes underpinning inter-individual differences in growth potential were observed in this study: the ability to exhibit high clearance rates and the capacity for thermal compensation. These findings indicate that the gill is a critical organ for determining growth rate variability in bivalves, as previously suggested by Tamayo et al. (2011) and Prieto et al. (2018, 2019, 2020).
Data Availability Statement
The datasets presented in this study can be found in online repositories. The names of the repository/repositories and accession number(s) can be found below: http://mda.vliz.be/directlink.php?fid=VLIZ_00000813_5e385d280a656.
Author Contributions
DP, EN, MBU, and II designed the experiments. DP, KA, and IU performed the experiments. DP, MBU, and II drafted the manuscript. All authors interpreted the data, revised, and approved the final version of the manuscript.
Funding
DP was funded by an FPI grant from the Basque Government. A part of this study was also funded by the Spanish Ministry of Economy and Competitiveness (Reference: AGL2013-49144-C3-1-R).
Conflict of Interest
The authors declare that the research was conducted in the absence of any commercial or financial relationships that could be construed as a potential conflict of interest.
References
Albaina, A., Villate, F., and Uriarte, I. (2009). Zooplankton communities in two contrasting Basque estuaries (1999-2001): reporting changes associated with ecosystem health. J. Plankton Res. 31, 739–752. doi: 10.1093/plankt/fbp025
Anestis, A., Pörtner, H. O., Karagiannis, D., Angelidis, P., Staikou, A., and Michaelidis, B. (2010). Response of Mytilus galloprovincialis (L.) to increasing seawater temperature and to marteliosis: metabolic and physiological parameters. Compar. Biochem. Physiol. Part A Mol. Integr. Physiol. 156, 57–66. doi: 10.1016/j.cbpa.2009.12.018
Aravena, G., Villate, F., Iriarte, A., Uriarte, I., and Ibáñez, B. (2009). Influence of the North Atlantic oscillation (NAO) on climatic factors and estuarine water temperature on the Basque coast (Bay of Biscay): comparative analysis of three seasonal NAO indices. Contin. Shelf Res. 29, 750–758. doi: 10.1016/j.csr.2008.12.001
Bayne, B. L. (1999). Physiological components of growth differences between individual oysters (Crassostrea gigas) and a comparison with Saccostrea commercialis. Physiol. Biochem. Zool. 72, 705–713. doi: 10.1086/316714
Bayne, B. L. (2004). Phenotypic flexibility and physiological tradeoffs in the feeding and growth of marine bivalve molluscs. Integr. Compar. Biol. 44, 425–432. doi: 10.1093/icb/44.6.425
Bayne, B. L., and Hawkins, A. J. (1997). Protein metabolism, the costs of growth, and genomic heterozygosity: experiments with the mussel Mytilus galloprovincialis Lmk. Physiol. Zool. 70, 391–402. doi: 10.1086/515848
Bayne, B. L., and Newell, R. C. (1983). “Physiological energetics of marine mollusc,” in The Mollusca, eds A. S. M. Salenium and K. M. Wilbur (New York, NY: Academic Press), 407–515. doi: 10.1016/b978-0-12-751404-8.50017-7
Bayne, B. L., Salkeld, P. N., and Worrall, C. M. (1983). Reproductive effort and value in different populations of the marine mussel, Mytilus edulis L. Oecologia 59, 18–26. doi: 10.1007/bf00388067
Bayne, B. L., Hedgecock, D., McGoldrick, D., and Rees, R. (1999a). Feeding behaviour and metabolic efficiency contribute to growth heterosis in Pacific oysters [Crassostrea gigas (Thunberg)]. J. Exper. Mar. Biol. Ecol. 233, 115–130. doi: 10.1016/s0022-0981(98)00125-7
Bayne, B. L., Svensson, S., and Nell, J. A. (1999b). The physiological basis for faster growth in the Sydney rock oyster, Saccostrea commercialis. Biol. Bull. 197, 377–387. doi: 10.2307/1542792
Bayne, B. L., Thompson, R. J., and Widdows, J. (1973). “Some effects of temperature and food on the rate of oxygen consumption by Mytilus edulis L,” in Effects of Temperature on Ectothermic Organisms, ed. W. Wieser (Berlin: Springer), 181–193. doi: 10.1007/978-3-642-65703-0_15
Beiras, R., Camacho, A. P., and Albentosa, M. (1995). Short-term and long-term alterations in the energy budget of young oyster Ostrea edulis L. in response to temperature change. J. Exper. Mar. Biol. Ecol. 186, 221–236. doi: 10.1016/0022-0981(94)00159-b
Buckley, B. A., Owen, M. E., and Hofmann, G. E. (2001). Adjusting the thermostat: the threshold induction temperature for the heat-shock response in intertidal mussels (genus Mytilus) changes as a function of thermal history. J. Exper. Biol. 204, 3571–3579.
Buxton, C. D., Newell, R. C., and Field, J. G. (1981). Response-surface analysis of the combined effects of exposure and acclimation temperatures on filtration, oxygen consumption and scope for growth in the oyster Ostrea edulis. Mar. Ecol. Prog. Ser. 6, 73–82. doi: 10.3354/meps006073
Conover, R. J. (1966). Assimilation of organic matter by zooplankton. Limnol. Oceanogr 11, 338–345. doi: 10.4319/lo.1966.11.3.0338
Cusson, M., Tremblay, R., Daigle, G., and Roussy, M. (2005). Modeling the depuration potential of blue mussels (Mytilus spp.) in response to thermal shock. Aquaculture 250, 183–193. doi: 10.1016/j.aquaculture.2005.03.045
Dunphy, B. J., Wells, R. M., and Jeffs, A. G. (2006). Oxygen consumption and enzyme activity of the subtidal flat oyster (Ostrea chilensis) and intertidal Pacific oyster (Crassostrea gigas): responses to temperature and starvation. N. Zeal. J. Mar. Freshw. Res. 40, 149–158. doi: 10.1080/00288330.2006.9517409
Fernández-Reiriz, M. J., Irisarri, J., and Labarta, U. (2016). Flexibility of physiological traits underlying inter-individual growth differences in intertidal and subtidal mussels Mytilus galloprovincialis. PLoS One 11:e0148245. doi: 10.1371/journal.pone.0148245
Fields, P. A., Zuzow, M. J., and Tomanek, L. (2012). Proteomic responses of blue mussel (Mytilus) congeners to temperature acclimation. J. Exper. Biol. 215, 1106–1116. doi: 10.1242/jeb.062273
Frederich, M., and Pörtner, H. O. (2000). Oxygen limitation of thermal tol- erance defined by cardiac and ventilatory performance in the spider crab, Maja squinado. Am. J. Physiol. 279, R1531–R1538.
Gnaiger, E. (1983). Heat dissipation and energetic efficiency in animal anoxibiosis: economy contra power. J. Exper. Zool. Part A Ecol. Genet. Physiol. 228, 471–490. doi: 10.1002/jez.1402280308
Hawkins, A. J. S. (1985). Relationships between the synthesis and breakdown of protein, dietary absorption and turnovers of nitrogen and carbon in the blue mussel, Mytilus edulis L. Oecologia 66, 42–49. doi: 10.1007/bf00378550
Hawkins, A. J. S., and Bayne, B. L. (1992). “Physiological processes, and the regulation of production,” in The Mussel Mytilus: Ecology, Physiology, Genetics and Culture, ed. E. Gosling (Amsterdam: Elsevier Science Publishers), 171–222.
Hawkins, A. J. S., Wilson, I. A., and Bayne, B. L. (1987). Thermal responses reflect protein turnover in Mytilus edulis L. Funct. Ecol. 1, 339–351. doi: 10.2307/2389790
Hildreth, D. I., and Crisp, D. J. (1976). A corrected formula for calculation of filtration rate of bivalve molluscs in an experimental flowing system. J. Mar. Biol. Assoc.U. K. 56, 111–120. doi: 10.1017/s0025315400020476
Hochachka, P. M., and Somero, G. N. (2002). Biochemical Adaptation. Oxford: Princeton University Press.
Holley, M. E., and Foltz, D. W. (1987). Effect of multiple-locus heterozybosity and salinity on clearance rate in a brackish-water clam, Rangia cuneata (Sowerby). J. Exper. Mar. Biol. Ecol. 111, 121–131. doi: 10.1016/0022-0981(87)90050-5
Ibarrola, I., Larretxea, X., Navarro, E., Iglesias, J. I. P., and Urrutia, M. B. (2008). Effects of body-size and season on digestive organ size and the energy balance of cockles fed with a constant diet of phytoplankton. J. Compar. Physiol. B 178, 501–514. doi: 10.1007/s00360-007-0243-7
Jones, H. D., Richards, O. G., and Southern, T. A. (1992). Gill dimensions, water pumping rate and body size in the mussel Mytilus edulis L. J. Exper. Mar. Biol. Ecol. 155, 213–237. doi: 10.1016/0022-0981(92)90064-h
Kang, H. Y., Lee, Y. J., Choi, K. S., Park, H. J., Yun, S. G., and Kang, C. K. (2016). Combined effects of temperature and seston concentration on the physiological energetics of the manila clam Ruditapes philippinarum. PLoS One 11:e0152427. doi: 10.1371/journal.pone.0152427
Lesser, M. P., and Kruse, V. A. (2004). Seasonal temperature compensation in the horse mussel, Modiolus modiolus: metabolic enzymes, oxidative stress and heat shock proteins. Compar. Biochem. Physiol. Part A 137, 495–504. doi: 10.1016/j.cbpb.2003.10.022
MacDonald, B. A., and Thompson, R. J. (1985). Influence of temperature and food availability on the ecological energetics of the giant scallop Placopecten magellanicus. I. Growth rates of shell and somatic tissue. Mar. Ecol. Prog. Ser. 72, 279–294. doi: 10.3354/meps025279
Newell, R. C., Johson, L. G., and Kofoed, L. H. (1977). Adjustment of the components of energy balance in response to temperature change in Ostrea edulis. Oecologia 30, 97–110. doi: 10.1007/bf00345414
Pace, D. A., Marsh, A. G., Leong, P. K., Green, A. J., Hedgecock, D., and Manahan, D. T. (2006). Physiological bases of genetically determined variation in growth of marine invertebrate larvae: a study of growth heterosis in the bivalve Crassostrea gigas. J. Exper. Mar. Biol. Ecol. 335, 188–209. doi: 10.1016/j.jembe.2006.03.005
Pérez-Camacho, A., Labarta, U., and Navarro, E. (2000). Energy balance of mussels Mytilus galloprovincialis: the effect of length and age. Mar. Ecol. Prog. Ser. 199, 149–158. doi: 10.3354/meps199149
Pernet, F., Tremblay, R., Comeau, L., and Guderley, H. (2007). Temperature adaptation in two bivalve species from different thermal habitats: energetics and remodelling of membrane lipids. J. Exper. Biol. 210, 2999–3014. doi: 10.1242/jeb.006007
Pernet, F., Tremblay, R., Gionet, C., and Landry, T. (2006). Lipid remodeling in wild and selectively bred hard clams at low temperatures in relation to genetic and physiological parameters. J. Exper. Biol. 209, 4663–4675. doi: 10.1242/jeb.02581
Pernet, F., Tremblay, R., Redjah, I., Sévigny, J. M., and Gionet, C. (2008). Physiological and biochemical traits correlate with differences in growth rate and temperature adaptation among groups of the eastern oyster Crassostrea virginica. J. Exper. Biol. 211, 969–977. doi: 10.1242/jeb.014639
Pörtner, H. (2001). Climate change and temperature-dependent biogeography: oxygen limitation of thermal tolerance in animals. Naturwissenschaften 88, 137–146. doi: 10.1007/s001140100216
Pörtner, H. O. (2002). Climate variations and the physiological basis of temperature dependent biogeography: systemic to molecular hierarchy of thermal tolerance in animals. Compar. Biochem. Physiol. Part A Mol. Integr. Physiol. 132, 739–761. doi: 10.1016/s1095-6433(02)00045-4
Pörtner, H. O. (2010). Oxygen-and capacity-limitation of thermal tolerance: a matrix for integrating climate-related stressor effects in marine ecosystems. J. Exper. Biol. 213, 881–893. doi: 10.1242/jeb.037523
Prieto, D., Markaide, P., Urrutxurtu, I., Navarro, E., Artigaud, S., Fleury, E., et al. (2019). Gill transcriptomic analysis in fast-and slow-growing individuals of Mytilus galloprovincialis. Aquaculture 511:734242. doi: 10.1016/j.aquaculture.2019.734242
Prieto, D., Tamayo, D., Urrutxurtu, I., Navarro, E., Ibarrola, I., and Urrutia, M. B. (2020). Nature more than nurture affects the growth rate of mussels. Sci. Rep. 10, 1–13.
Prieto, D., Urrutxurtu, I., Navarro, E., Urrutia, M. B., and Ibarrola, I. (2018). Mytilus galloprovincialis fast growing phenotypes under different restrictive feeding conditions: fast feeders and energy savers. Mar. Environ. Res. 140, 114–125. doi: 10.1016/j.marenvres.2018.05.007
Prosser, C. L. (ed.) (1991). Comparative Animal Physiology, Environmental, and Metabolic Animal Physiology. Hoboken, NJ: John Wiley & Sons.
Resgalla, C. Jr., Brasil, E. D. S., and Salomão, L. C. (2007). The effect of temperature and salinity on the physiological rates of the mussel Perna perna (Linnaeus 1758). Braz. Arch. Biol. Technol. 50, 543–556. doi: 10.1590/s1516-89132007000300019
Somero, G. N. (2010). The physiology of climate change: how potentials for acclimatization and genetic adaptation will determine ‘winners’ and ‘losers’. J. Exper. Biol. 213, 912–920. doi: 10.1242/jeb.037473
Sommer, A., Klein, B., and Pörtner, H. O. (1997). Temperature induced an- aerobiosis in two populations of the polychaete worm Arenico- la marina (L.). J. Comp. Physiol. 167B, 25–35. doi: 10.1007/s003600050044
Tamayo, D., Azpeitia, K., Markaide, P., Navarro, E., and Ibarrola, I. (2016). Food regime modulates physiological processes underlying size differentiation in juvenile intertidal mussels Mytilus galloprovincialis. Mar. Biol. 163, 1–13.
Tamayo, D., Ibarrola, I., Cigarría, J., and Navarro, E. (2015). The effect of food conditioning on feeding and growth responses to variable rations in fast and slow growing spat of the Manila clam (Ruditapes philippinarum). J. Exper. Mar. Biol. Ecol. 471, 92–103. doi: 10.1016/j.jembe.2015.05.017
Tamayo, D., Ibarrola, I., and Navarro, E. (2013). Thermal dependence of clearance and metabolic rates in slow-and fast-growing spats of manila clam Ruditapes philippinarum. J. Compar. Physiol. B 183, 893–904. doi: 10.1007/s00360-013-0764-1
Tamayo, D., Ibarrola, I., Urrutia, M. B., and Navarro, E. (2011). The physiological basis for inter-individual growth variability in the spat of clams (Ruditapes philippinarum). Aquaculture 321, 113–120. doi: 10.1016/j.aquaculture.2011.08.024
Tamayo, D., Ibarrola, I., Urrutxurtu, I., and Navarro, E. (2014). Physiological basis of extreme growth rate differences in the spat of oyster (Crassostrea gigas). Mar. Biol. 161, 1627–1637. doi: 10.1007/s00227-014-2447-1
Toro, J. E., Alcapan, A. C., Ojeda, J. A., and Vergara, A. M. (2004). Selection response for growth rate (Shell height and live weight) in the Chilean blue mussel (Mytilus chilensis Hupe 1854). J. Shellf. Res. 23, 753–758.
Toro, J. E., and Vergara, A. M. (1998). Growth and heterozygosity in a 12-Month-Old Cohort of Ostrea chilensis obtained by mass spawning in the laboratory. Mar. Ecol. 19, 311–323. doi: 10.1111/j.1439-0485.1998.tb00470.x
Vahl, O. (1973). Pumping and oxygen consumption rates of Mytilus edulis L. of different sizes. Ophelia 12, 45–52. doi: 10.1080/00785326.1973.10430118
Whyte, J. N. (1987). Biochemical composition and energy content of six species of phytoplankton used in mariculture of bivalves. Aquaculture 60, 231–241. doi: 10.1016/0044-8486(87)90290-0
Widdows, J. (1978). Combined effects of body size, food concentration and season on the physiology of Mytilus edulis. J. Mar. Biol. Assoc. U. K. 58, 109–124. doi: 10.1017/s0025315400024449
Widdows, J., and Bayne, B. L. (1971). Temperature acclimation of Mytilus edulis with reference to its energy budget. J. Mar. Biol. Assoc. U. K. 51, 827–843. doi: 10.1017/s0025315400018002
Keywords: growth, clearance rate, metabolic rate, temperature, thermal compensation, Mytilus galloprovincialis
Citation: Prieto D, Arranz K, Urrutxurtu I, Navarro E, Urrutia MB and Ibarrola I (2020) Variable Capacity for Acute and Chronic Thermal Compensation of Physiological Rates Contributes to Inter-Individual Differences in Growth Rate in Mussels (Mytilus galloprovincialis). Front. Mar. Sci. 7:577421. doi: 10.3389/fmars.2020.577421
Received: 29 June 2020; Accepted: 03 September 2020;
Published: 24 September 2020.
Edited by:
Samad Rahimnejad, University of South Bohemia in České Budějovice, CzechiaReviewed by:
Vincenzo Parrino, University of Messina, ItalyWeiqun Lu, Shanghai Ocean University, China
Copyright © 2020 Prieto, Arranz, Urrutxurtu, Navarro, Urrutia and Ibarrola. This is an open-access article distributed under the terms of the Creative Commons Attribution License (CC BY). The use, distribution or reproduction in other forums is permitted, provided the original author(s) and the copyright owner(s) are credited and that the original publication in this journal is cited, in accordance with accepted academic practice. No use, distribution or reproduction is permitted which does not comply with these terms.
*Correspondence: Daniel Prieto, RGFuaWVsLnByaWV0b0BlaHUuZXVz