- 1IFREMER, Physiology and Biotechnology of Algae Laboratory, Nantes, France
- 2PAPPSO, Micalis Institute, INRA, AgroParisTech, Université Paris-Saclay, Jouy-en-Josas, France
- 3IFREMER, Phycotoxins Laboratory, Nantes, France
Cobalamin (vitamin B12) is a cobalt-containing enzymatic cofactor involved in methionine synthesis. Provided only by select bacteria and archaea in marine systems, this vitamin is known to limit primary production in different oceanic areas. Understanding the consequences of cobalamin limitation on phytoplankton physiology is of great interest, notably for cobalamin-dependent haptophytes that significantly contribute to oceanic carbon fixation and sulfur cycle through dimethyl sulfonio propionate (DMSP) synthesis. Here, the effect of cobalamin limitation was compared to nitrogen limitation on the model haptophyte Tisochrysis lutea grown in chemostats, combining comparative proteomics with the analysis of major macromolecules and specific osmolytes. Our results highlight the interconnection of carbon and DMSP metabolisms through the cobalamin-dependent methionine synthesis by showing that cobalamin scarcity impacts the mechanisms of carbon allocation and reduces DMSP quota. Conversely, proline production seemed to anticorrelate with cobalamin availability. In a boarder context, analysis of transcriptomes or genomes of main DMSP producers from different phytoplankton lineages suggests that most of them are cobalamin-dependent, which means that prokaryotic cobalamin synthesis exerts an important control on phytoplankton DMSP production in some regions of the world ocean.
Introduction
Haptophytes are microalgae with ubiquitous representatives (Jordan and Chamberlain, 1997; O’Brien et al., 2013) that dominate pico-planktonic and nano-planktonic communities in oligotrophic open oceans (Thomsen et al., 1994; Reynolds, 2006; Not et al., 2008). Haptophytes contribute in various ways to global carbon cycle by photosynthetically fixing carbon (Liu et al., 2009; Jardillier et al., 2010) and by producing calcified coccoliths, the formation of which is thought to be accountable for half of the total calcium carbonate precipitations in marine systems (Milliman, 1993). They play an important role in sulfur biogeochemistry as well, due to their high production of dimethyl sulfonio propionate (DMSP) (Keller et al., 1989; Malin et al., 1992; Malin and Kirst, 1997; Sunda et al., 2007; Franklin et al., 2010), the precursor of gas dimethyl sulfide (DMS), a climatically important sulfur compound contributing to around 10–40% of the total global sulfur budget and mainly emitted from oceanic reservoirs (Lovelock et al., 1972; Charlson et al., 1987).
The energetic cost of the catalytic reactions involved in methionine synthesis make it energetically expensive for the cell to produce, requiring between 18 and 20 mol of ATP per molecule (Kaleta et al., 2013). Apart from its role as a building-block of proteins, this amino-acid is also the precursor of S-adenosyl methionine (SAM). SAM is an important C1-donor of the methionine cycle and is involved in numerous functions such as DNA methylation, amino-acid metabolism, and potentially DMSP synthesis (Gage et al., 1997; Struck et al., 2012; Fujimori, 2013; Sauter et al., 2013; Curson et al., 2018; Liao and Seebeck, 2019). Methionine is produced in one-carbon metabolism by the conversion of tetrahydrofolate and homocysteine by means of the methionine synthase enzymes (Figure 1). Two enzymatic isoforms have been described: a cobalamin-dependent methionine synthase (METH) necessitating the cofactor as a methyl donor, and a cobalamin-independent (METE) isoform requiring zinc as a cofactor (Pejchal and Ludwig, 2004). While adding cobalamin is mandatory for cultivating microalgae encoding only METH, some other species possess the additional METE, or METE only, and are able to grow without the vitamin (Croft et al., 2005; Helliwell et al., 2011). In the environment, cobalamin, a cobalt-containing molecule, is synthesized only by a subset of bacteria and archaea (Martens et al., 2002; Warren et al., 2002) and has been reported to be available in oceanic systems in femtomolar to picomolar concentrations depending on its chemical form (Panzeca et al., 2009; Sañudo-Wilhelmy et al., 2012; Heal et al., 2014; Suffridge et al., 2018). As a consequence, cobalamin is considered as a co-limiting or secondary limiting element for phytoplankton growth in several areas of the ocean (Panzeca et al., 2006; Bertrand et al., 2007; Gobler et al., 2007; Koch et al., 2011; Browning et al., 2017, 2018; Suffridge et al., 2018).
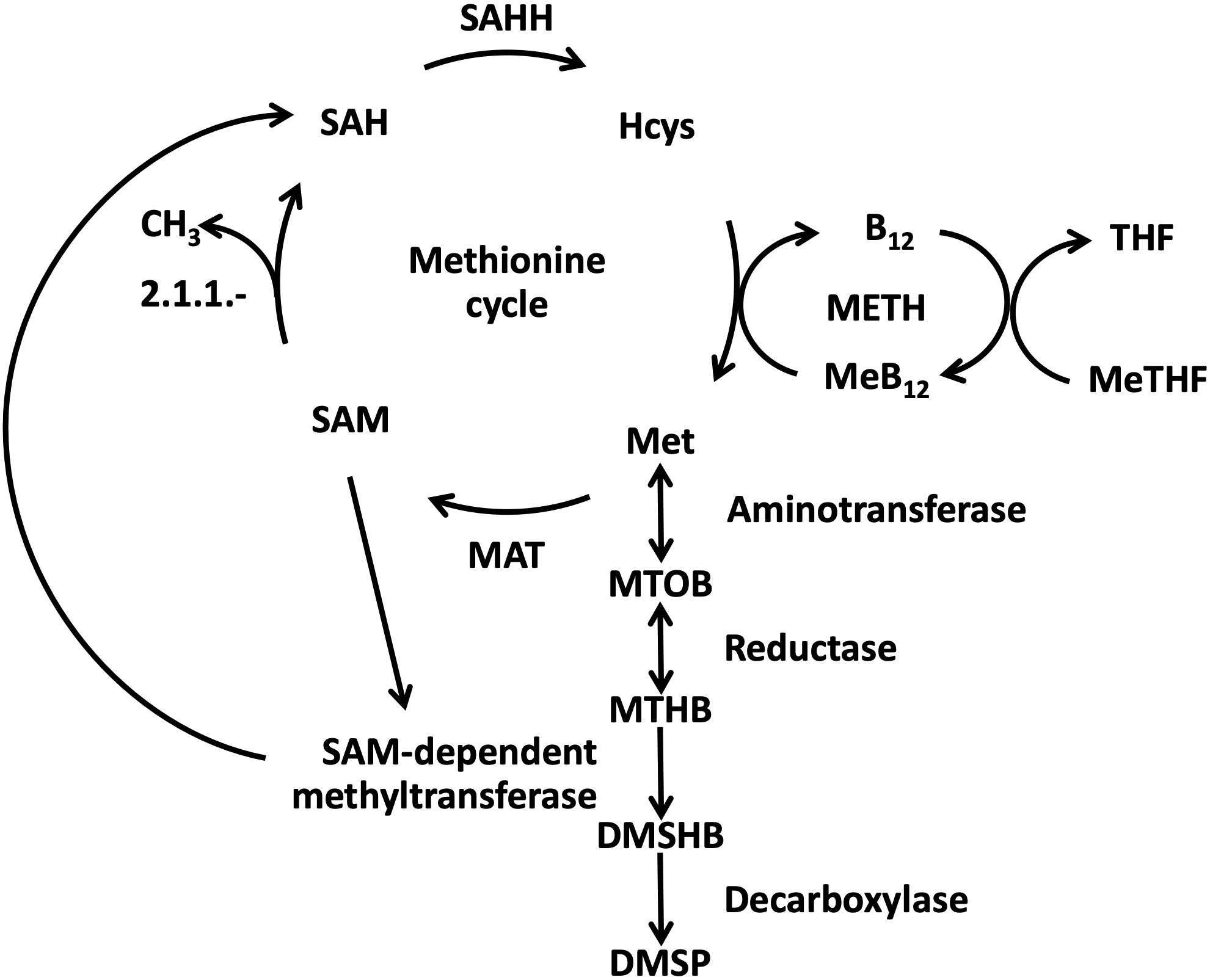
Figure 1. Schematic representation of the links between methionine synthesis and DMSP biosynthetic pathway in cobalamin-dependent algae [modified based on Gage et al. (1997), Stefels (2000), Curson et al. (2018)]. DMSHB: 4-dimethylsulfonio-2-hydroxybutyrate; DMSP: dimethyl sulfonio propionate; Hcys: homocysteine; MAT: methionine adenosyl transferase; MeB12: methylated form of cobalamin; Met: methionine; METH: cobalamin-dependent methionine synthase; MeTHF: methyltetrahydrofolate; MTHB: 4-methylthio-2-hydroxybutyrate; MTOB: 4-methylthio-2-oxobutyrate; SAH: S-adenosyl homocysteine; SAHH: S-adenosyl homocysteine hydrolase; SAM: S-adenosyl methionine; THF: tetrahydrofolate.
How microalgae cope with cobalamin scarcity has been explored in diatoms with the examples of the cobalamin-dependent (auxotrophic) Thalassiosira pseudonana and the cobalamin-independent Phaeodactylum tricornutum (Bertrand et al., 2012, 2013; Heal et al., 2019). The authors managed to identify in both species the only cobalamin acquisition protein known to date in microalgae. Based on transcriptomic and proteomic analyses, they proposed that both diatoms increase cobalamin acquisition and reduce methionine synthase activity when grown in cobalamin scarcity. In addition, they observed an increase of METE transcript and protein in P. tricornutum. Metabolomic analyses of T. pseudonana showed that cobalamin deprivation impacted the compatible solute pool, the methionine metabolism and the trans-sulfuration pathway (Heal et al., 2019). In the cobalamin-independent chlorophyte Chlamydomonas reinhardtii, Helliwell et al. (2015) revealed that prolonged growth of the microalgae with cobalamin addition led to a shift to cobalamin dependency probably due to a transposable element integration in the METE gene. More recently, cobalamin auxotrophy in haptophytes has been investigated using sequence similarity searches to identify methionine synthase isoforms for 19 haptophyte strains (16 transcriptomes, 3 genomes) from 9 families (Nef et al., 2019b). Based on the presence of METH and on the absence of METE in the survey, it has been proposed that Haptophyta phylum harbors only cobalamin-auxotrophic species. This was confirmed by the absence of the METE haptophyte sequence in the MATOU database of planktonic metagenomes and metatranscriptomes, that included data from cobalamin-limited areas. A first targeted gene expression analysis suggested that the model haptophyte Tisochrysis lutea may mitigate cobalamin scarcity by finely regulating cobalamin-associated genes, and potentially assimilating dissolved methionine if available (Nef et al., 2019b).
However, little is known about the effects of cobalamin limitation on haptophyte molecular physiology and intracellular carbon allocation. Therefore, the present study aimed at investigating the influence of cobalamin availability on carbon partitioning in the marine haptophyte T. lutea using comparative proteomic and by measuring major macromolecules. Given the potential link between carbon, DMSP and cobalamin in haptophytes and their ability to produce high DMSP amounts, a focus was set on this osmolyte. To study the specific metabolic modifications linked directly to cobalamin availability, chemostat steady-state under cobalamin limitation was characterized and compared with nitrogen limitation. By removing the growth rate changes inherent of batch cultures, the use of chemostat steady-states enable the unequivocal definition of the species’ physiological state (Hoskisson and Hobbs, 2005; Bull, 2010; Adamberg et al., 2015). As both cobalamin and nitrogen are involved in protein build-up, the nitrogen-limited control allowed for the identification of impacts specific to cobalamin scarcity versus those more generally related to changes in protein synthesis. This chemostat experiment was combined with the measurement of major macromolecules on both conditions to decipher carbon allocation mechanisms. DMSP, its precursor methionine and other N-containing osmolytes glycine betaine (GBT) and proline were examined in all the nutrient limitation conditions and following resupply of the limiting nutrients. We provide the evidence that carbon allocation is differently affected by cobalamin and N scarcity and concluded that a lack of the vitamin can particularly decrease DMSP synthesis. As proline production appeared to anticorrelate with cobalamin availability, we suggest that it could act as substitute to DMSP. Combined with a survey of cobalamin auxotrophy across high DMSP producers from different lineages, we propose that cobalamin limitation could reduce phytoplanktonic DMSP production in some oceanic areas.
Materials and Methods
Cultures and Sampling
Experiments in controlled photobioreactors in chemostat mode were chosen to analyze steady state ecophysiological conditions. The culture conditions were previously detailed in Nef et al. (2019b). Briefly, an axenic strain of T. lutea CCAP 927/14 was grown in chemostat continuous cultures set either in cobalamin limitation [modified Walne’s medium (Walne, 1970) with 25.3 pmol L–1 cobalamin (cyanocobalamin)] or in nitrogen (N) limitation (modified Walne’s medium with 294 μmol L–1 NaNO3) both in biological duplicates at the same dilution rate (0.5 day–1). The N-limited culture was taken as a control to identify from a culture with the same growth rate if some metabolic changes were specifically related to cobalamin scarcity. Axenic condition was frequently verified by plating culture samples on Marine Agar (BD DifcoTM, Becton Dickinson Company, United States) and performing cytometric analyses and epifluorescence microscopy both using SYBRTM Green staining (Lonza, United States). The temperature was set at 27 ± 1°C with continuous light (400 μmol photons m–2 s–1) and pH maintained at 8.2 by CO2 injection. Samples were collected at three time points during steady-state (days 14, 21, and 25, corresponding to ss1, ss2, and ss3) (Figure 2). After several days at equilibrium, a discreet input of the limiting nutrients was realized either with 25.3 pmol L–1 cobalamin or 294 μmol L–1 NaNO3 for the cobalamin-limited and N-limited chemostats, respectively. A previous work on the effect of nitrogen limitation on T. lutea physiology showed that some biochemical modifications appeared 1 h after the addition of the limiting nutrient (Garnier et al., 2016). We expected similar dynamics to occur regarding cobalamin availability, particularly for proteins involved in cobalamin transport and metabolism, and therefore chose to collect samples 1 h after nutrient resupply (day 25, corresponding to resupply + 1 h or sp1) (Figure 2). As discussed in Nef et al. (2019b), cobalamin quotas of the chemostats were monitored to characterize their physiological state regarding cobalamin availability with the cobalamin-limited samples showing eight times lower cobalamin quota then the sp1 sample, and the N-limited and N-resupplied samples showing 50 times higher values than the cobalamin-limited ones. The samples used for the following analyses were taken during cobalamin-limited and N-limited steady-states (ss1, ss2, and ss3) and 1 h after cobalamin and N resupply (sp1). The biochemical and comparative proteomic analyses of these samples are presented in the following sections.
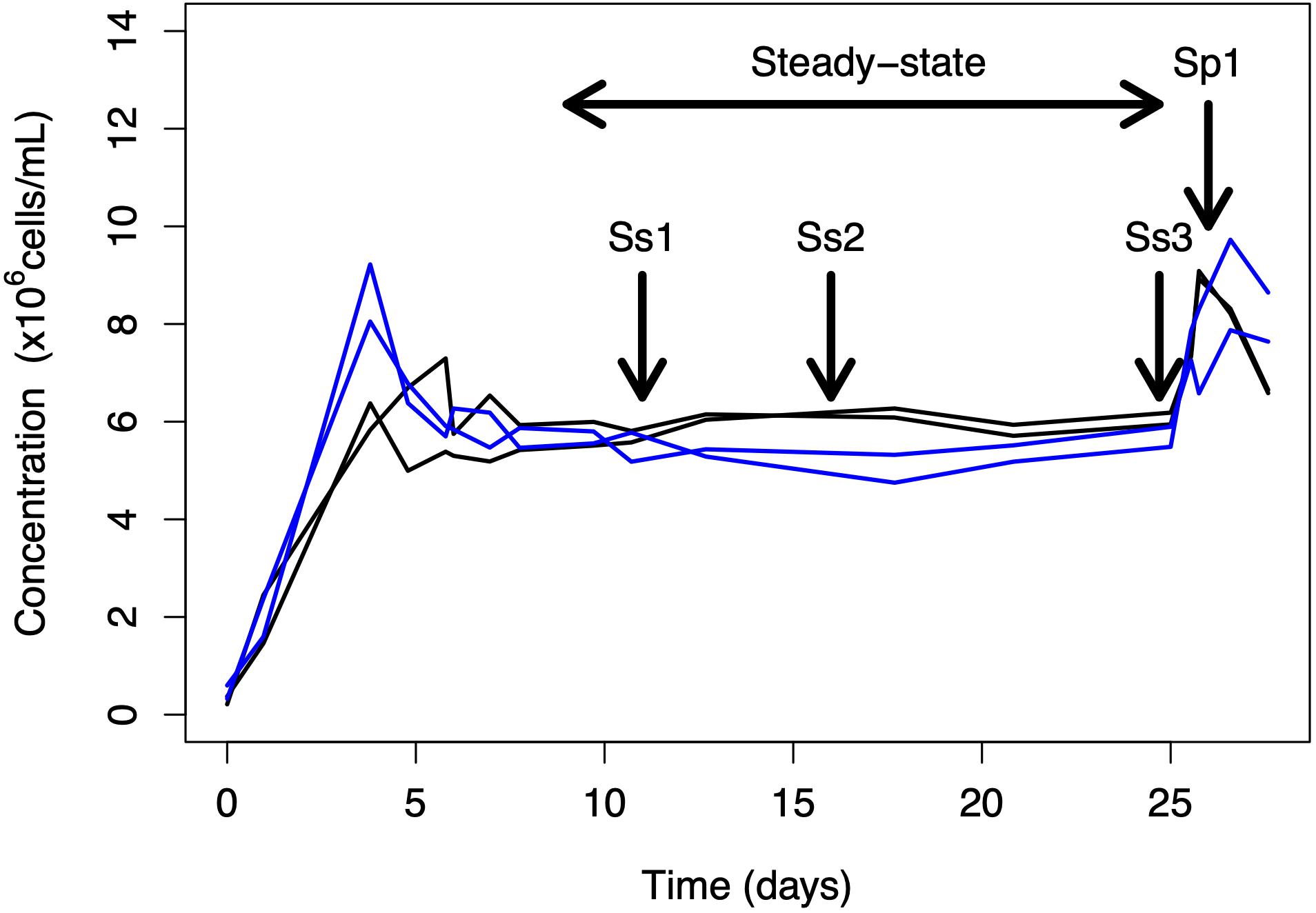
Figure 2. Overview of the chemostat experiment. Sampling was performed during steady-state (at days 14, 21, and 25; samples ss1, ss2, and ss3) and 1 h after nutrient spike (day 25; sample sp1). Blue lines correspond to cobalamin-limited replicates and black lines to N-limited replicates.
Biochemical Analyses
To identify whether carbon allocation changed in the different physiological conditions, particulate carbon was measured with a CN elemental analyser (Flash 2000, Thermo Fisher Scientific, Waltham, MA, United States) on a total of 20 × 106 cells filtered on 25 mm pre-combusted GF/F microfibers filters (0.7 μm, Whatman, United Kingdom). Total proteins and carbohydrates were quantified using the PierceTM BCA Protein Assay Kit and Dubois assay (Dubois et al., 1956), respectively, as previously described for T. lutea (Garnier et al., 2016). Free amino-acids extraction was performed on cellular pellets of 80 × 106 cells suspended in 800 μL freshly distilled water. The cells were disrupted by sonication and the supernatants were harvested after centrifugation. Free amino-acids were quantified in the supernatants by HPLC at P2M2 platform (Rennes, France). For total lipid extraction, pellets of 250 × 106 cells were suspended in 3 mL chloroform:methanol (2:1 v:v) then sonicated 10 min in ice bath (pulse mode). Three milliliters of chloroform:methanol (1:2 v:v) and 2.7 mL distilled water were then added in each sample and the tubes were centrifuged to separate organic and aqueous phases, this step was repeated twice, each time transferring the organic phase into a pre-combusted glass tube. The combined organic phases were dried under nitrogen flux at room temperature for 30 min, then dried at 60°C for several days until their weight was stable. The pigments analyses were based on the protocol described in Serive et al. (2017) using high performance liquid chromatography. Concentrations of chlorophyll c2 (Chl c2), chlorophyll a (Chl a), and carotenoids fucoxanthin (Fuco) diatoxanthin (Diato), diadinoxanthin (Diadino), echinenone (Echin), and beta-carotene (β-Car) in samples were determined from DHI (Denmark) standards. Protein, lipid, carbohydrate and pigment measurements were converted to % of total carbon using the following conversion factors: 0.53 g C g–1 proteins; 0.4 g C g–1 carbohydrates; 0.76 g C g–1 lipids; 0.74 g C g–1 chlorophyll a; 0.69 g C g–1 chlorophyll c; 0.80 g C g–1 carotenoid or xanthophyll (Geider and La Roche, 2002). For free amino-acids quantification, each mole of the corresponding amino-acid was converted into its carbon equivalent.
Target metabolite analyses were realized to measure DMSP and its nitrogenous analog GBT, together with proline as all these compounds were affected by cobalamin availability levels in the cobalamin-dependent diatom T. pseudonana (Heal et al., 2019). The method also allowed to measure free methionine, the backbone of DMSP biosynthesis. The compatible solutes were extracted from 80 × 106 cells previously stored as cell pellets at −80°C, then suspended into 1 mL methanol, centrifuged and filtered on 0.2 μm filters. Methionine, DMSP, GBT and proline analyses were performed on a LC System (model UFLC XR, Shimadzu) coupled to a triple-quadrupole mass spectrometer (4000Qtrap, AB Sciex). Based on Curson et al. (2018), the chromatography was performed with a Hypersil GOLD HILIC column (150 × 2.1 mm, 3 μm, Thermo Fisher Scientific) with a guard column (10 × 2.1 mm, 3 μm). Details of the LC-MS/MS method are described in Georges des Aulnois et al. (2019). The mobile phase flow rate was modified to 0.25 mL min–1 for this chromatographic column. The compounds were quantified against their standards (Sigma Aldrich) prepared in methanol to cover calibration levels from 50 to 5000 nM. Algal extracts were diluted (100 to 600-fold) to fit within the standard curve.
Comparative Proteomics
The methods used for proteomics using high resolution mass spectrometry analyses are fully described in Nef et al. (2019a). Briefly, proteins were extracted and purified from 350 × 106 cells. A total of 20 μg proteins were fractionated on SDS-PAGE 10% acrylamide gel. In-gel digestion was conducted with 50 mM ammonium bicarbonate pH 8.0 and 0.1 μg trypsin per sample. After trypsin digestion, the peptides were analyzed by LC-MS/MS at the PAPPSO platform1 using an Orbitrap FusionTM LumosTM TribridTM (Thermo Fisher Scientific) coupled to an UltiMateTM 3000 RSLCnano System (Thermo Fisher Scientific). Protein identification with X!TandemPipeline (PAPPSO, version 0.2.21)2 was conducted by using the T. lutea in silico proteome database (Garnier et al., 2016, version 2018, 17373 entries) as reference. The identified proteins were filtered as follows: (1) peptide e-value < 0.01 with a minimum of two peptides per protein and (2) protein e-value < 10–4. A reversed sequence database was used to calculate False Discovery Rates (FDR). A 0.05% FDR was achieved at peptide level and 0.00% at protein level. Peptide quantities of the proteome were analyzed by extracted ion chromatograms (XICs) using MassChroQ “Black Caiman” version 0.3.7 (Valot et al., 2011).
Considering analytical depth, 6 and 4 samples for cobalamin and nitrogen limitations were, respectively considered for further proteomic analyses and two samples 1 h after cobalamin resupply. These samples were used to compare cobalamin limitation vs. N limitation and cobalamin limitation vs. cobalamin resupply. The abundance of proteins with at least three measured peptides were calculated as the sum of peptide intensities. Missing peptide intensities and missing proteins were assigned the lowest recorded values. The dataset was normalized to take technical variability between injections into account. One factor ANOVA was performed to determine the significance of variation between the different conditions. Factor effect on protein accumulation was considered significant when p < 0.05 with a fold change superior to 2.0 or inferior to −2.0.
Determination of Cobalamin Auxotrophy in Phytoplankton High DMSP-Producers
As previously detailed (Nef et al., 2019b), the presence of MetH and MetE encoding genes was searched by Blastx in available genomes and transcriptomes downloaded from https://www.imicrobe.us/. High DMSP producers were selected by using a threshold of 10 mmol DMSP/L of cell volume by considering Keller et al. (1989) and Caruana and Malin (2014) as references. METE from C. reinhardtii (XP_001702934.1, NCBI) and P. tricornutum (B7G1X4, UniProt) and METH from T. lutea and P. tricornutum (B7GBG7, UniProt) were used as query sequences.
Results
Carbon Allocation and Photosynthetic Capacity
Tisochrysis lutea composition in major macromolecules was compared between a cobalamin-limited and a nitrogen-limited chemostats both at steady-state. Carbon allocation was analyzed by measuring cellular contents in proteins, carbohydrates, free amino-acids, lipids, pigments (chlorophylls and carotenoids) and major osmolytes (DMSP and its precursor methionine, together with proline and GBT). The raw results are presented in Supplementary Table S1. For cobalamin-limited cells, 32.9% of carbon was allocated to proteins, 2.5% to free amino-acids, 11.0% to carbohydrates and 34.6% to lipids (Figures 3A–D). Compared to N limitation, the carbon allocated to carbohydrates in cobalamin limitation was 2.3 times lower and the carbon allocated to lipids 1.4 times higher (Figures 3B,D), implying that the two nutrient limitations differently affect carbon allocation between the main carbon-rich compounds. Regarding nitrogen-rich compounds, carbon allocation to proteins and free amino-acids was found 1.3 and 6.5 times higher, respectively in cobalamin limitation compared to N limitation (Figures 3A,C). The carbon allocation in total pigments was found similar (1.90%) in both conditions (Figure 3E), suggesting that cobalamin and N limitation similarly impacted carbon allocation to photosynthetic apparatus. The general carbon allocation 1 h after cobalamin resupply was quite similar to that of the cobalamin-limited steady state whereas higher carbon allocation to free amino acids and lower carbon allocation to carbohydrates were observed 1 h after nitrogen resupply (Figures 3A–E). It appears likely that the delay necessary to observe carbon allocation modifications following cobalamin resupply is greater than an hour.
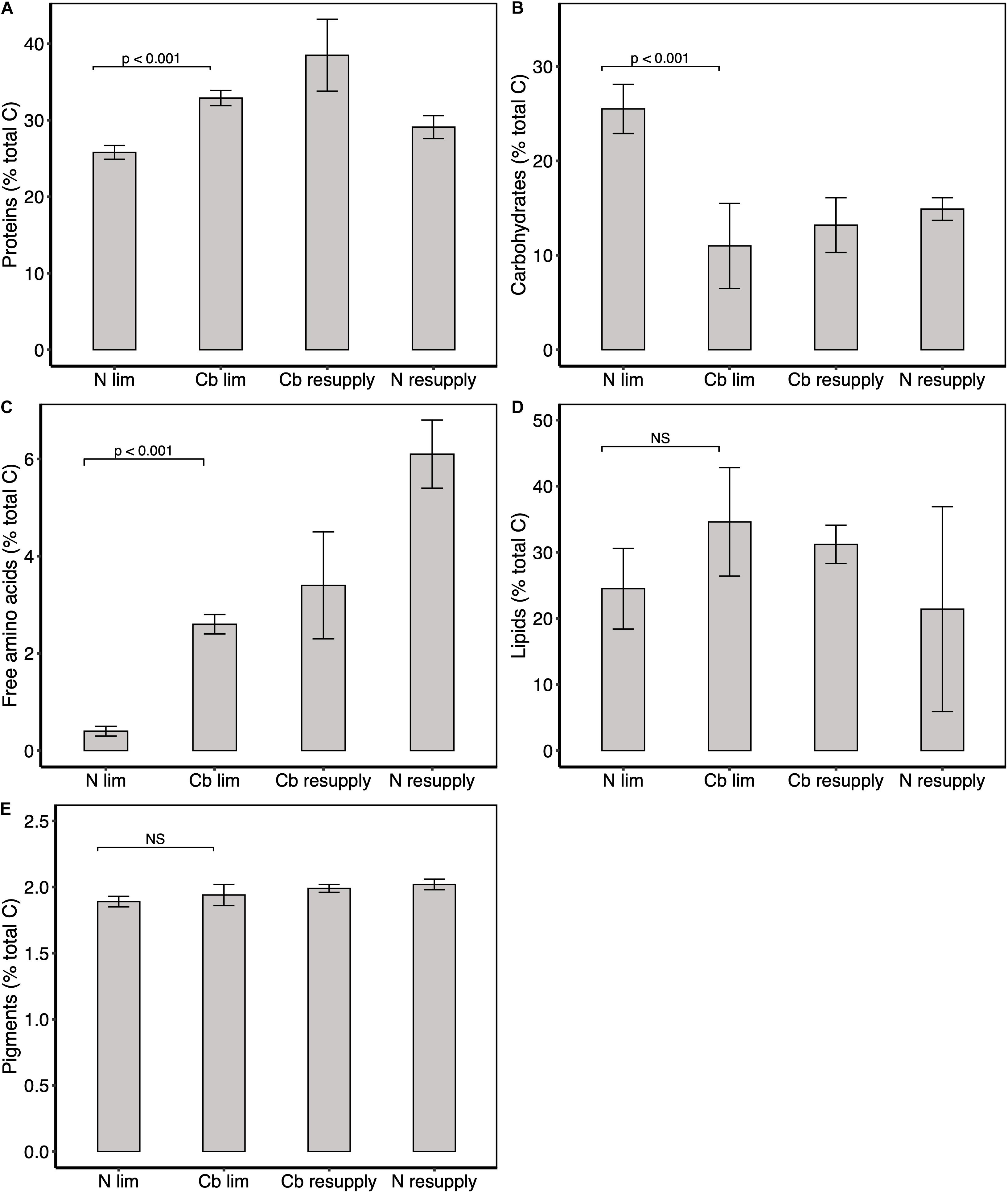
Figure 3. Carbon partitioning between major metabolites. Percentage of total carbon in (A) proteins, (B) carbohydrates, (C) free amino-acids, (D) lipids, and (E) pigments. Pigments correspond to the sum of Chl a, c2 and xanthophylls. Values represent means ± one standard deviation for n = 6 replicates in cobalamin (Cb) and N limitation (ss1, ss2, and ss3), and means ± one range for n = 2 replicates in cobalamin and N resupply (sp1). Significance levels of one factor ANOVA are represented; NS: not significant.
Tisochrysis lutea photosynthetic capacity was also investigated by considering pigment ratios as a proxy of the photosynthetic status. Total chlorophyll (the sum of Chl c2 and Chl a) per nitrogen unit was not impacted in cobalamin limitation compared to N limitation, indicating comparable photosynthetic capacity in both physiological states (Figure 4A). The ratio of pigments diatoxanthin (DT) and diadinoxanthin (DD) (DT/(DD + DT)) was lower in cobalamin limitation vs. N limitation (Figure 4B), which suggested higher photoprotective response in the N-limited condition. No clear change in total chlorophyll and photoprotective pigments ratio was observed following cobalamin or nitrogen resupply (Figures 4A,B). Effort was made in the experiment to maintain each culture at saturating light. Equal light was measured behind the cultures (50 μmol photons m–2 s–1) as a means to ensure the same light availability and a slight difference of 10 μmol photons m–2 s–1 was observed in front of the chemostats. Because this difference was very low, we considered that the change in pigment ratio was mainly due to the effect of nutrient limitation.
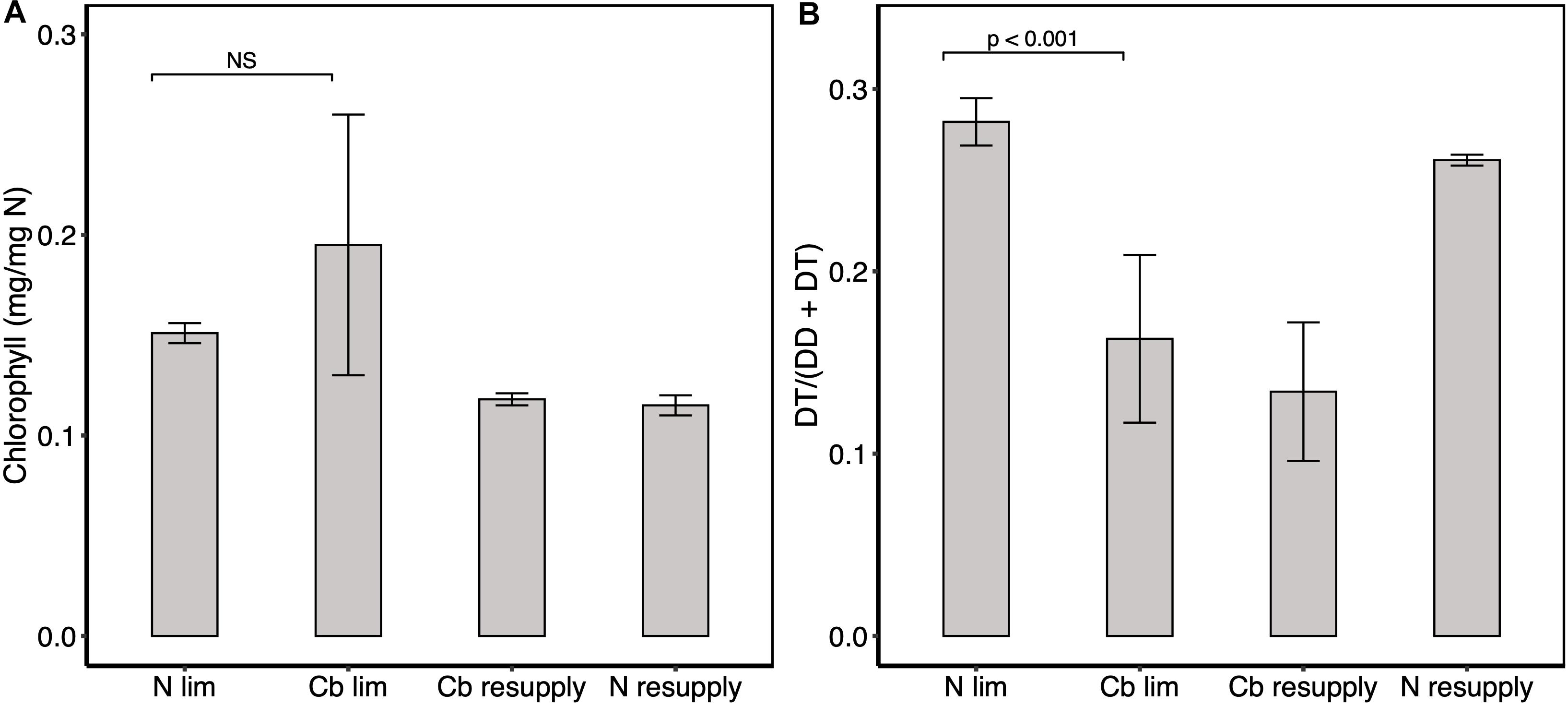
Figure 4. Intracellular concentration of pigments. (A) Total chlorophyll (Chl c2 + Chl a) quota. (B) Ratio of photoprotective pigments diadinoxanthin and diatoxanthin. Values represent means ± one standard deviation for n = 6 replicates for cobalamin (Cb) and N limitation (ss1, ss2, and ss3), and means ± one range for n = 2 replicates in cobalamin and N resupply (sp1). Significance levels of one factor ANOVA are represented; NS: not significant.
Osmolyte Quotas
An analysis of the content in major osmolytes GBT, proline, DMSP and its precursor methionine was conducted to decipher whether changes in cobalamin availability could control DMSP production, and could consequently lead to a rearrangement in compatible solute pool. The DMSP quota was five times lower in cobalamin-limited cultures (0.076 nmol μg–1 C) than in the N-limited ones (0.419 nmol μg–1 C) and did not significantly change 1 h after cobalamin spike (0.099 nmol μg–1 C) nor after nitrogen resupply (0.484 nmol μg–1 C) (Figure 5A). DMSP represented 0.46 and 2.53% total algal carbon in cobalamin and N limitation, respectively. This result is in agreement with the 5–7% cell carbon allocated to DMSP measured by Sunda et al. (2007) and (Simó et al., 2002) in Emiliania huxleyi and with the average 2–5% proportion of cell carbon in DMSP in haptophytes (Stefels et al., 2007). Conversely to DMSP, GBT and proline quotas were higher in cobalamin limitation (0.096 and 0.030 nmol μg–1 C) whilst almost absent in N-limited cultures (<LOQ and 0.001 nmol μg–1 C) and a decrease in proline quota was observed 1 h after cobalamin resupply (0.030 nmol μg–1 C) compared to cobalamin limitation (Figures 5C,D). 1 h after N resupply, GBT quota was significantly higher (0.097 nmol μg–1 C) than in N limitation whereas proline quota did not show clear modification (0.025 nmol μg–1 C). Free methionine was also found significantly higher following N resupply but not after cobalamin amendment (Figure 5B). Taken together, these results show that the compatible solute production dynamics in T. lutea are different depending on the limiting nutrient. Cobalamin limitation may potentially induce a replacement of DMSP by N-containing osmolytes, and specifically by proline that exhibited swift metabolic linkage regarding the vitamin availability. Conversely, GBT and methionine production seem to be tightly controlled by nitrogen level. As DMSP quota did not change 1 h upon resupply of any of the limiting nutrients, we infer that its production dynamics may take more than an hour to be observed in T. lutea, potentially because the cell has to produce a lot more before it is noticeable given the large amount observed.
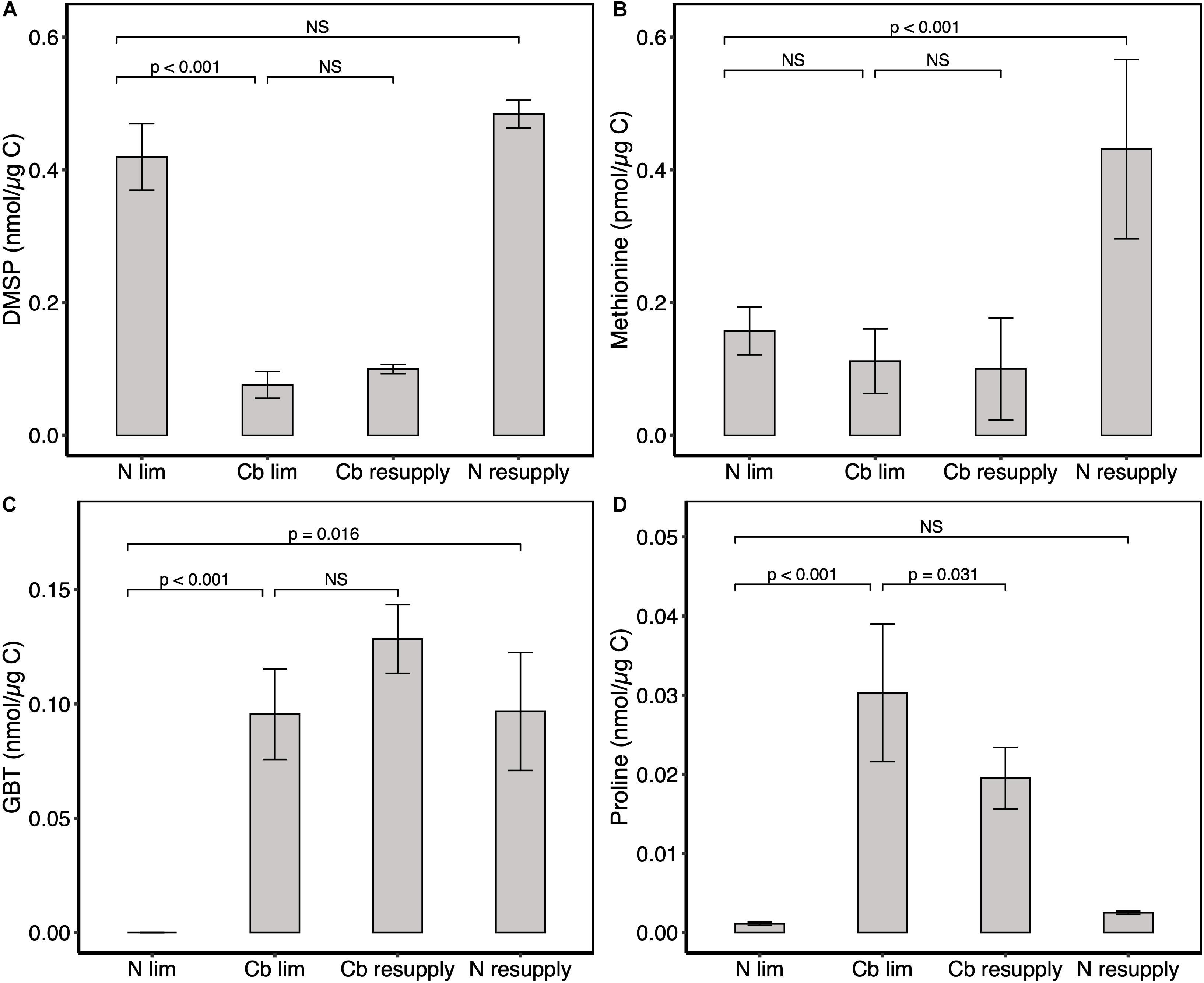
Figure 5. Intracellular concentration of osmolytes. (A) DMSP, (B) methionine, (C) GBT, and (D) proline. Values represent means ± one standard deviation for n = 18 technical replicates for cobalamin (Cb) and N limitation (ss1, ss2, and ss3), and means ± one standard deviation for n = 6 technical replicates in cobalamin and N resupply (sp1) (except for methionine in cobalamin resupply with n = 5 replicates). GBT content for N limitation was below the limit of quantification. Significance levels of one factor ANOVA are represented; NS: not significant.
Comparative Proteomics
Overview of Comparative Proteomic Results
Large-scale comparative proteomic analysis on the cobalamin-limited and N-limited samples was carried out to evaluate whether changes in key metabolic pathways impacted by cobalamin limitation could explain observed biochemical modifications. The raw proteomic data are publicly available in Nef et al. (2019a). A total of 2636 proteins were identified, corresponding to about 15% in silico proteome. Six samples from cobalamin limitation, four from N limitation (two of the former six were discarded due to poor analytical depth) and two from cobalamin resupply (1 h after cobalamin adding) were used to compare cobalamin limitation vs. N limitation and cobalamin limitation vs. cobalamin resupply. The abundance of 1726 and 1765 proteins was analyzed for both comparative analyses, respectively. Most of the differentially abundant proteins had a fold change between −2 and −5 with the highest fold change reaching a factor 3 and the lowest −20 (Supplementary Figure S1). In cobalamin limitation vs. N limitation, a total of 108 proteins were found differentially abundant (84 decrease in abundance and 24 increase in abundance), corresponding to 6.26% variation in the detected proteome (Supplementary Table S2). In cobalamin limitation vs. resupply, 75 proteins were differentially abundant (61 decrease and 14 increase in abundance), accounting for a rearrangement of 4.25% measured proteome (Supplementary Table S3). Over the differentially abundant proteins, only seven were the same between the two comparative analyses. Functional annotation allowed to identify the putative function of 70% of the differentially abundant proteins.
We investigated whether methionine-rich proteins would be among the ones depleted during cobalamin limitation given the direct involvement of this cofactor in methionine synthesis. Methionine-rich proteins are considered containing at least 6% methionine with a total length of at least 95 amino-acids (Chu et al., 2016). Among the 49 methionine-rich proteins identified this way in the detected proteome, only two (6.90 and 8.50% methionine) were depleted in cobalamin limitation vs. N limitation and one (6.73% methionine) was depleted compared to cobalamin resupply. This indicated that a decrease in cobalamin availability did not impact protein quality regarding methionine or that there might be some compensation mechanism. The most impacted pathways with a link to phenotypic variations are discussed in the following sections.
Cobalamin Limitation Modifies the Abundance of Proteins Involved in Photosynthesis, Central Carbon Metabolism and Protein Catabolism
Whereas the small Rubisco subunit was not found differentially abundant, the large subunit was found five times depleted in cobalamin limitation vs. N limitation (Figure 6 and Supplementary Table S2), suggesting a global reduction of Rubisco activity. Proteomic analyses revealed that six proteins of photosynthetic light-harvesting complex (LHC) and cytochrome b6f were depleted in cobalamin limitation vs. N limitation and a similar trend was observed comparing cobalamin limitation with resupply (Supplementary Figure S2 and Supplementary Table S3). When taking the pigment analyses into account, that suggested that cobalamin-limited cultures exhibited lower photoprotection and respiration compared to N-limited controls despite same chlorophyll content, it was possible to infer that the lower excess energy dissipation observed as a result of cobalamin limitation was potentially due to lower light acquisition. A notable depletion of proteins involved in glycolysis/gluconeogenesis (five proteins involved in four out of twelve metabolic steps), TCA cycle (four proteins), oxidative phosphorylation (seven proteins) and β-oxidation of lipids (two proteins both having two homologs) was observed in cobalamin limitation vs. N limitation (Figure 6 and Supplementary Table S2) and the same proteins were found differentially abundant for oxidative phosphorylation in cobalamin limitation vs. resupply (Supplementary Figure S2 and Supplementary Table S3). All these metabolic pathways are involved in intracellular recruitment and transport of carbon skeletons, with the TCA cycle acting as a hub to allocate carbon skeletons between lipids, carbohydrates and proteins.
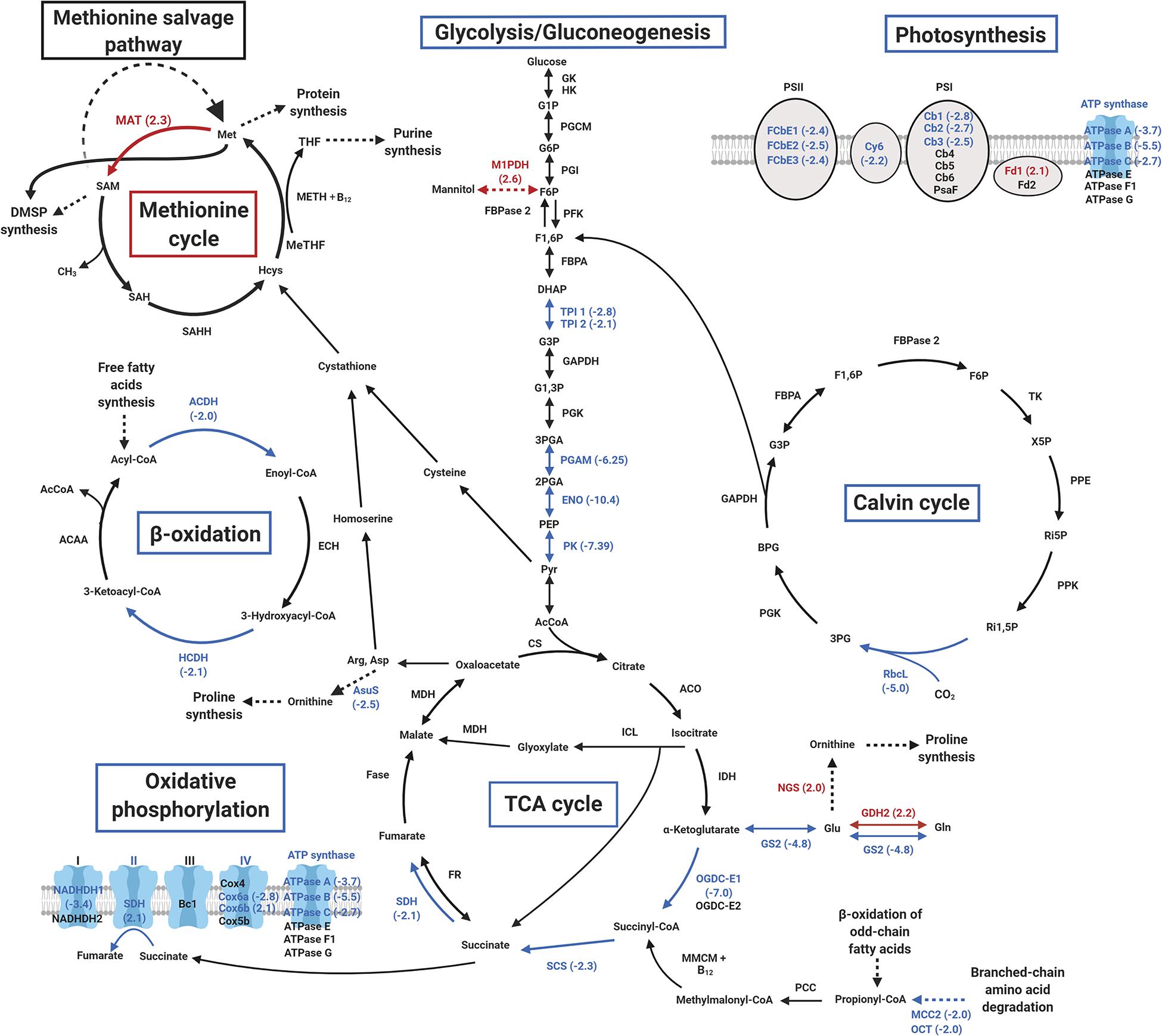
Figure 6. Overview of major metabolic pathways differentially impacted in cobalamin limitation vs. N limitation. Enriched proteins (p < 0.05 and fold > 2.0) are shown in red and depleted proteins (p < 0.05 and fold < –2.0) are shown in blue (protein abbreviations are shown in Supplementary Table S2).
Interestingly, 17 proteins involved in nitrogen metabolism were also differentially accumulated in cobalamin limitation vs. N limitation (Supplementary Table S2). Among these, six proteins with protease or peptidase activity were depleted, including a methylcrotonyl-CoA carboxylase that has been shown to provide acetyl-CoA through leucine degradation in the diatom P. tricornutum (Ge et al., 2014; Supplementary Table S2), potentially indicating that the cobalamin-limited phenotype presents lower catabolism at this level. 1 h after cobalamin resupply no clear difference was observed at protein level for β-oxidation, TCA cycle and glycolysis/gluconeogenesis, and carbohydrate and lipid content did not change. It is likely that mechanisms regulating intracellular carbon allocation after cobalamin resupply may take longer than an hour before having observable effects. Seven proteins involved in nitrogen metabolism were also depleted in cobalamin limitation vs. resupply (Supplementary Table S3), suggesting that the ability of cobalamin-limited cells to produce all the proteins they require is impaired compared to cobalamin repletion condition.
Impact of Cobalamin Limitation on Proteins Related to Cobalamin and Methionine Metabolism
We further investigated whether a limitation in cobalamin induced a change in key methionine cycle proteins, namely the cobalamin-dependent methionine synthase (METH), methionine adenosyltransferase (MAT), which allows the conversion of methionine into SAM and the S-adenosyl homocysteine hydrolase (SAHH), involved in homocysteine regeneration (Figure 1). No clear change of METH and SAHH accumulation was observed in cobalamin limitation vs. N limitation nor when comparing cobalamin limitation vs. resupply. However, MAT was found enriched by 2.3 and by 1.9 in cobalamin limitation vs. N limitation and in cobalamin limitation vs. resupply, respectively (Figure 6, Supplementary Figure S2 and Supplementary Table S2 and in the dataset available in Nef et al. (2019a)). In some microorganisms, a methionine salvage pathway (MSP) can constitute an alternate way to get methionine back after its conversion into ASM (Albers, 2009). Though we did find four proteins potentially involved in MSP in T. lutea, none of them was differently abundant. The accumulation of Class II ribonucleotide reductase (RNRII) was also investigated because this enzyme catalyzes the reversible conversion of dNTP into NDP for DNA synthesis using cobalamin as a cofactor (Sando et al., 1975). No RNRII protein was retrieved in T. lutea proteome, but a hypothetical cobalamin-independent class I RNR (RNRI) was found enriched by 1.9 in cobalamin limitation vs. N limitation [see the full comparative proteomic dataset available in Nef et al. (2019a)].
Proteins Involved in Osmolyte Synthesis
To identify proteins potentially responsible for the observed changes in osmolytes content, a first search for proteins involved in DMSP biosynthesis was realized. In microalgae, DMSP production uses methionine and requires two reversible steps of transamination and reduction, a transfer of methyl by a methyltransferase and a final step of decarboxylation requiring SAM (Gage et al., 1997; Rhodes et al., 1997; Curson et al., 2018; Figure 1). The corresponding enzymes are poorly described for haptophytes, except for DSYB, the methylthiohydroxybutyrate methyltransferase, localized in the chloroplasts and mitochondria in the haptophyte Prymnesium parvum, and identified in many phytoplankton (Curson et al., 2018). A putative T. lutea DSYB (P813.01) identified here presented 68.0% identity with the one of P. parvum but did not show difference of accumulation between conditions, despite differences in DMSP content. In the environment, DMSP can be cleaved into DMS by the enzyme DMSP lyase in certain phytoplankton species and bacteria (Steinke et al., 1998; Stefels, 2000; Franklin et al., 2010). No DMSP lyase candidate was identified in T. lutea by looking for homologs to Alma DMSP lyases as described in E. huxleyi (P0DN21, P0DN23, R1F9H0, R1CW23, R1ERP2, R1F493, R1ENF4, and R1G6M4) and Symbiodinium sp. (P0DN22) (Alcolombri et al., 2015).
A focus was set also on proteins involved in the synthesis of N-containing osmolytes GBT and proline. Choline is converted by choline dehydrogenase (CDH) into betaine aldehyde that is in turn converted into GBT by betaine aldehyde dehydrogenase (BADH) (Aguilar et al., 2017). A putative CDH was identified but it did not display differential accumulation in this study. Proline can be produced from both glutamate and ornithine in plants (Majumdar et al., 2016) but in haptophytes and diatoms proline synthesis appears to be achieved through the ornithine-urea cycle from carbamoyl phosphate (Allen et al., 2011). An N-acetylglutamate synthase (NGS, Tiso_v2_Prot_14921) involved in the synthesis of ornithine was found enriched in cobalamin limitation vs. N limitation (Supplementary Table S2). Another urea cycle protein, argininosuccinate synthase (ASuS) which converts aspartate and citruline into argininosuccinate was depleted by 2.5 in cobalamin limitation vs. N limitation (Figure 6 and Supplementary Table S2). No other homolog of these proteins was found in the proteome of T. lutea.
Cobalamin Auxotrophy Among Phytoplankton High DMSP Producers
We investigated cobalamin auxotrophy of marine high DMSP producers from different lineages (including haptophytes, dinoflagellates, diatoms, chrysophytes, and prasinophytes) that were identified in previous studies (Caruana and Malin, 2014; Spiese and Tatarkov, 2014; Nef et al., 2019b) considering species with available sequenced transcriptome or genome (Table 1). The cobalamin-independent isoform of methionine synthase (MetE) was only found in the transcriptomes of 3 over the 11 dinoflagellates investigated and not in the other 10 species. Although MetE can be down-regulated in microalgae encoding both methionine synthase isoforms when grown with cobalamin supply, MetE transcripts and peptides appear still detectable at a lower level (e.g., in the diatom P. tricornutum (Bertrand et al., 2013; Helliwell et al., 2014, 2016), and the chlorophyte C. reinhardtii (Helliwell et al., 2014)). It was therefore concluded that the absence of MetE in the investigated trancriptomes corresponded to the absence of MetE-encoding gene. Moreover, all these species encode the cobalamin-dependent methionine synthase, indicating that they require this cofactor.
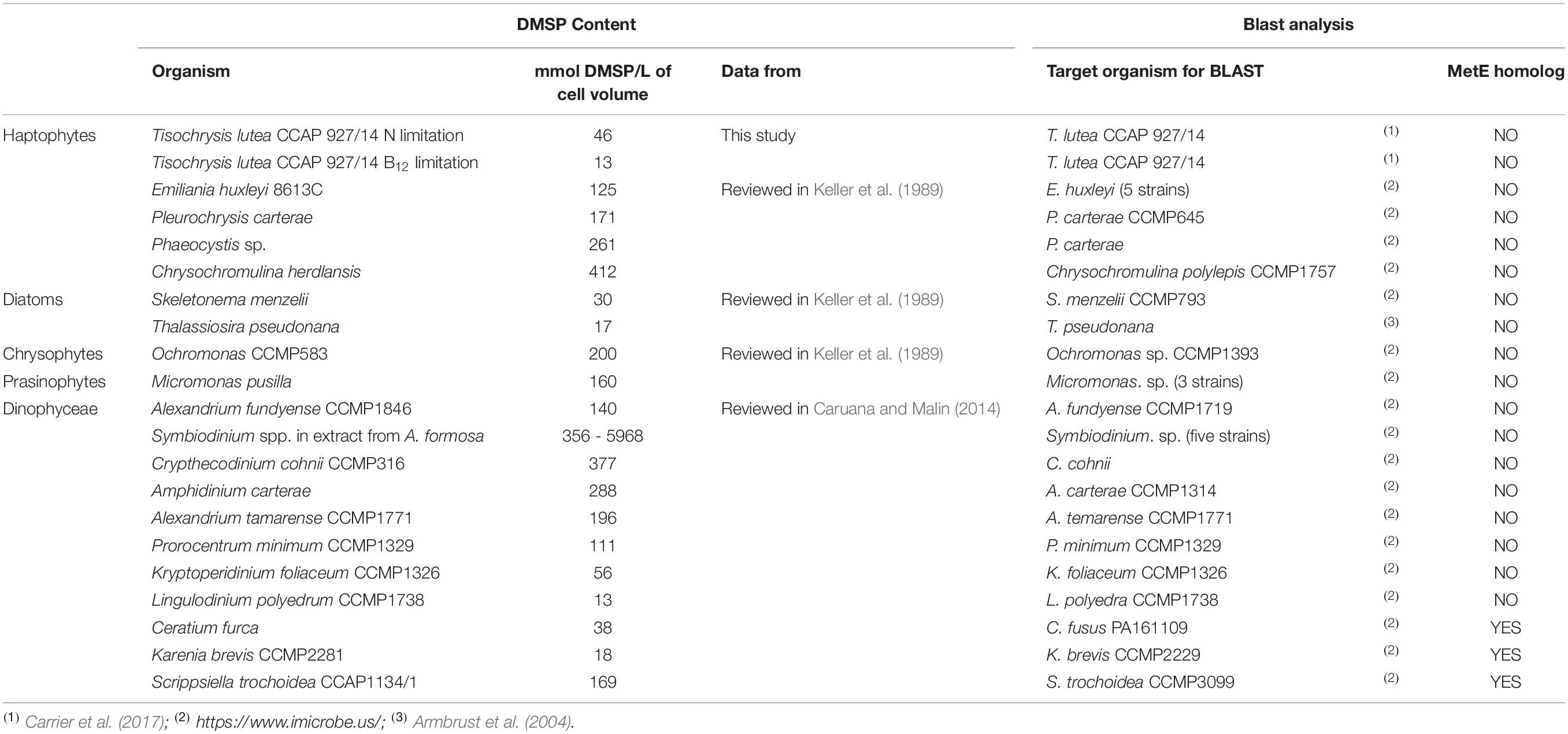
Table 1. Analysis of the repartition of cobalamin auxotrophy in high DMSP producers from different phytoplankton lineages.
Discussion
Information about how haptophytes mediate cobalamin limitation is rare even though cobalamin controls phytoplankton development in numerous marine systems either as a co-limiting or secondary limiting nutrient (Panzeca et al., 2006; Bertrand et al., 2007; Gobler et al., 2007; Koch et al., 2011; Browning et al., 2017; Suffridge et al., 2018). Haptophyte microalgae are key contributors to phytoplankton communities (Thomsen et al., 1994), to oceanic DMSP production (Keller et al., 1989), and appear to all require cobalamin for their development (Nef et al., 2019b). Therefore, deciphering the effect of vitamin cobalamin scarcity on fixed carbon allocation and DMSP production of these microalgae should provide important insights into their contribution to primary productivity and sulfur cycle in cobalamin-limited areas. The marine haptophyte T. lutea was grown in cobalamin-limited and nitrogen (N)-limited chemostats. Cultures in continuous chemostat mode guaranteed their stability at equilibrium and a fine estimation of the effects due to even a small variation of one of the factors controlling the culture. This method allowed to accurately compare the cultures’ biochemical composition and conduct thorough comparative proteomic analyses to understand the effect of cobalamin limitation on carbon allocation, with a special emphasis on specific compatible solutes.
Relatively few differentially abundant proteins were noted in the comparative proteomic analyses (between 4 and 6% detected proteome variation), which resulted from our controlled experiment where the same growth rate was applied. Given that some phenotypic traits including carbohydrate, protein and osmolyte contents were clearly dissimilar, these differentially abundant proteins were expected to be strongly linked to the observed trait variations, as chemostat cultures are relevant to finely study metabolic mechanisms in microalgae (Hoskisson and Hobbs, 2005). In particular, changes in carbon allocation between the main biochemical compounds were clearly observed, together with a different enrichment of enzymes involved in carbon skeletons transport and conversion, demonstrating a different effect of cobalamin and N limitations on intracellular carbon allocation. Lipid catabolism produces carbon skeletons that can be recruited in the TCA cycle. Here, our proteomic analyses suggested a lower allocation of carbon originating from lipid catabolism to the synthesis of carbohydrates in cobalamin limitation, which appeared consistent with the biochemical measurements. N limitation is known to affect the production of N-rich compounds such as proteins and free amino-acids, as was observed in N-deprived cultures of P. tricornutum (Alipanah et al., 2015), Nannochloropsis oceanica (Dong et al., 2013) and T. lutea (Garnier et al., 2014), and its availability controls carbon allocation to lipids in T. lutea (Garnier et al., 2016). Here, we observed that cobalamin availability appears also to have an impact on carbon allocation to lipids, at least equal to the one of nitrogen.
Lower light acquisition and dissipation were observed when comparing cobalamin limitation to N limitation, together with a general decrease of mitochondrial energetic metabolism (TCA cycle and oxidative phosphorylation), showing that photosynthetic and respiratory characteristics were differently affected by both limitations, while the growth rate was the same. In our proteomic analyses, the comparison between both conditions is based on the same amount of proteins. Given that protein content was higher in cobalamin limitation, more carbon should be fixed in N limitation per quantity of proteins, which could partly explain the increase of carbon fixation and central metabolism in this condition. Additionally, nitrogen limitation is known to induce an important N turnover (Levitan et al., 2015; Remmers et al., 2018) that fuels and requires the TCA cycle. Therefore, central metabolism activity appears generally higher in nitrogen limitation, which can explain the comparatively reduced TCA cycle and glycolysis/gluconeogenesis activity in the cobalamin-limited phenotype. Such differences of energetic metabolism have been reported for the chlorophyte Dunaliella tertiolecta grown in chemostat cultures at the same dilution rate either N- or phosphorus-limited (Geider et al., 1998), demonstrating that chemostat culture comparisons may provide a more accurate picture of how algae acclimate in various nutrient limitations. N limitation can reduce the synthesis of proteins associated to light acquisition in microalgae (Levitan et al., 2015; Shang et al., 2017; Remmers et al., 2018). Therefore, the comparatively lower synthesis of LHC proteins observed in cobalamin limitation suggests that the cofactor has similar and maybe more important impact on these proteins production. Cobalamin is known to bind to an antirepressor complex that regulates the bacteriochlorophyll pathway of the purple bacteria Rhodobacter capsulatus (Cheng et al., 2014). The results obtained here for T. lutea might indicate similar mechanism of cobalamin on LHC synthesis and such hypothesis should be more deeply explored in eukaryotic phytoplankton.
Methionine is known to be the most expensive amino-acid (Kaleta et al., 2013) because its synthesis presents one of the most important enzymatic costs in terms of ATP (as characterized in Escherichia coli by Li et al., 2014). This amino-acid has an important role through its conversion into SAM, a methylating agent involved in numerous pathways such as transfer of methyl groups to secondary metabolites, DNA and proteins through the activity of SAM-dependent methyltransferases (Chiang et al., 1996; Kozbial and Mushegian, 2005; Struck et al., 2012; Fujimori, 2013). SAM appears particularly important also for phospholipid production in green algae and other eukaryotes (Hirashima et al., 2017) and for the synthesis of polyamines, present in all forms of life (Roje, 2006; Sauter et al., 2013). A previous study on T. pseudonana showed that cobalamin scarcity induces an imbalance in the methionine cycle with a depletion of SAM content, an increased activity of the trans-sulfuration pathway through higher content of cystathione, potentially to compensate for the possible cytotoxicity of methionine cycle intermediates homocysteine and SAH, and a depletion of both DMSP and GBT (Heal et al., 2019). Bertrand et al. (2012) also reported that T. pseudonana accumulates much more MAT enzymes when grown with low cobalamin input. We previously observed an enrichment in transcripts of the methionine cycle proteins METH, MAT and SAHH in cobalamin-limited cultures of T. lutea (Nef et al., 2019b). Here, we were not able to differentiate cystathionine-β-synthase, cystathionine-β-lyase, cystathionine-γ-synthase and cystathionine-γ-lyase due to high sequence similarity in algae (Bromke and Hesse, 2015), that prevented us from concluding on a possible higher activity of the trans-sulfuration pathway, and no clear change of METH and SAHH accumulation was observed in cobalamin limitation vs. N limitation nor when comparing cobalamin limitation vs. resupply. We nonetheless noticed an enrichment in MAT level when comparing both cobalamin and N limitations and cobalamin limitation with resupply, which confirmed the hypothesis of a strong link between this protein and cobalamin availability in T. lutea. Also, no other cobalamin-dependent protein, such as RNRII, was retrieved, which suggests that methionine metabolism is the most impacted by cobalamin scarcity, with no clear change in MSP proteins. Based on these results, it is conceivable that cobalamin-dependent microalgae might share a common acclimation mechanism to cobalamin limitation by increasing the ability to produce SAM, probably to try to maintain physiologically important methylation reactions when the enzymatic cofactor is scarce, or to reduce the accumulation of potentially toxic homocysteine and SAH (Roe et al., 2002; Jakubowski, 2004).
As methionine is involved in DMSP synthesis through both its transamination and conversion into SAM that provides a methyl group (Gage et al., 1997; Stefels, 2000; Curson et al., 2018), our hypothesis was that reduced cobalamin availability would lead to decreased DMSP production in cobalamin-auxotrophic haptophytes in the same way that it was observed for T. pseudonana (Spiese and Tatarkov, 2014; Heal et al., 2019). The authors were not able to rule out that the changes in DMSP content between the cobalamin treatments was due to cobalamin availability alone as a decrease in DMSP was also observed in a low light treatment were cobalamin was replete and the patterns of DMSP quotas followed the growth rate variations (Heal et al., 2019). Here, we specifically designed chemostat cultures that ensured the same growth rate and light availability between treatments, and clearly observed a sharp DMSP decrease in cobalamin limitation compared to N limitation in T. lutea. Batch cultures of the cobalamin-dependent haptophyte Isochrysis galbana, a species close to T. lutea (Bendif et al., 2013), displayed DMSP concentrations ranging from 0.25 to 0.9 nmol DMSP/μg C in non-limited exponential phase, corresponding to 1.5–5.4% of the cellular carbon (Tang and Simó, 2003; Archer et al., 2011). These values are the same order than those found in the N-limited chemostats presented here and higher than those in cobalamin limitation, supporting our N-limited cultures as a reference against which to compare the cobalamin-limited ones. However, because many compounds require methionine (mainly in the form of SAM) for their synthesis, we were not able to tell whether changes in DMSP production could be due to lower methionine synthesis. The impact of cobalamin limitation on methionine cycle and fluxes may affect many pathways through SAM rearrangements, which may be partly responsible for the phenotypic variations. We observed that DMSP quota did not change 1 h upon resupply of cobalamin nor nitrogen, which made us infer that its production dynamics may take more than an hour until they are observed in T. lutea. Also, no Alma DMSP lyase homolog was identified, supporting the idea that this enzyme is restricted to the Noelaerhabdaceae family in haptophytes (Franklin et al., 2010) and suggesting that DMSP was not cleaved. Additional measures of dissolved DMSP, which could not be performed here, should help clarifying whether it was partly excreted. Knowing that DMSP excretion mainly occurs in the late stationary phase of discontinuous phytoplankton cultures (Keller, 1991; Keller et al., 1999), it is likely that in the present experiment the main part of DMSP was intracellular. Finally, taking our results into account, it appears that cobalamin-dependent microalgae from different lineages exhibit a decline in DMSP production as a result of the cofactor scarcity.
Dimethyl sulfonio propionate is thought to play different roles in algal cells, such as osmoregulation, cryoprotection in polar algae and as an antioxidant (Keller et al., 1989; Stefels, 2000; Sunda et al., 2002; Gebser and Pohnert, 2013; Petrou and Nielsen, 2018). This osmolyte is also considered a major infochemical in the phycosphere (Seymour et al., 2010), the zone surrounding algal cells where microorganisms are influenced by algal extracellular compounds (Bell and Mitchell, 1972). Several studies have shown that this molecule constitutes a carbon and sulfur source preferentially consumed by Rhodobacteraceae (González et al., 1999, 2000; Howard et al., 2008; Zeng et al., 2016) and other Proteobacteria (Kiene et al., 1999, 2000; Tripp et al., 2008; del Valle et al., 2015). DMSP may also mediate algal interactions with bacteria such as for Ulva and its associated microbiota, which enables morphogenesis of the macroalga (Kessler et al., 2018). In addition, it has been observed that the strains of the haptophyte E. huxleyi producing the highest DMSP amounts had higher probabilities to be killed by bacterial algicidal molecules, suggesting that DMSP can act as a signal molecule allowing bacteria to localize algae (Barak-Gavish et al., 2018). Here, DMSP quota appeared much lower in cobalamin limitation compared to N limitation, but it was still present in low amounts. Based on this, we hypothesize that DMSP could either play a key physiological role in sulfur storage (Stefels, 2000), to ensure balanced global metabolism or/and play a role as an infochemical allowing microbial interactions. Phytoplanktonic DMSP production could increase the attraction of bacteria, which might release cobalamin that in turn could potentially increase microalgal DMSP quota. This might explain DMSP production in very small quantities even when cobalamin is rare, as a way to initiate the positive interaction loop.
A dramatic GBT decrease under low cobalamin input was noted for T. pseudonana (Heal et al., 2019), supporting the idea that GBT synthesis involves multiple SAM molecules in diatoms (Bertrand and Allen, 2012). Here, the pattern we observed was different for T. lutea, with GBT levels higher in cobalamin limitation compared to N limitation, and equal to the ones after N resupply. No significant change in GBT was noticed following cobalamin resupply, though it is possible that GBT concentration may increase more than an hour after cobalamin addition. Based on this result, it appears possible that SAM may not be involved in GBT synthesis, or that SAM fluxes to GBT production did not decrease in our study. Interestingly, proline quotas showed patterns tightly linked to cobalamin availability, being higher in cobalamin limitation and decreasing after resupply. Though proteins possibly involved in GBT synthesis did not display significant change in cobalamin limitation, several proteins potentially involved in proline production showed significantly different abundances. More specifically, the enrichment in N-acetylglutamate synthase and the depletion in argininosuccinate synthase in cobalamin limitation (see Figure 6) may suggest that aspartate was preferentially used as substrate in favor of homocysteine production, therefore replenishing the methionine cycle, rather than for ornithine and proline synthesis in the urea cycle. These changes in protein abundances could potentially explain the observed higher proline content in cobalamin limitation. Such a switch in osmolytes content was proposed to occur in DMSP-producing dinoflagellates and haptophytes (Keller et al., 1999, 2004) but was not clearly observed in previous studies until now. More information regarding the functional characterization of the candidate proteins is required to understand the mechanisms regulating the balance of osmolytes. We must note that we have chosen to focus our measurements on DMSP, its nitrogenous analog GBT, together with proline as these compounds were found affected by both cobalamin availability levels and light in T. pseudonana (Heal et al., 2019). Gebser and Pohnert (2013) showed that DMSP and GBT are the most abundant zwitterionic osmolytes in E. huxleyi, supporting the relevance of our compatible solute analysis, though other described osmolytes found in haptophytes, including for instance homarine and gonyol (Keller et al., 1989; Gebser and Pohnert, 2013), were not measured here. Having a more complete picture of the link between SAM production and osmolyte synthesis depending on cobalamin availability in haptophytes would be valuable, and achievable for instance by coupling our approach to metabolomics.
Previous results suggested that all haptophytes are cobalamin-dependent (Nef et al., 2019b). A similar method using molecular data was applied to evaluate cobalamin dependency of marine high DMSP producers from different lineages (Caruana and Malin, 2014; Spiese and Tatarkov, 2014; Nef et al., 2019b). The cobalamin-independent methionine synthase was not found in the transcriptomes nor genomes of most of these species but the cobalamin-dependent isoform was. The present results suggest that the majority of marine phytoplankton producing high DMSP amounts require an exogenous source of cobalamin for their growth. This includes for instance species such as the haptophyte Phaeocystis spp., globally distributed from pole to pole (Rellinger et al., 2009; Vogt et al., 2012), with arctic Phaeocystis pouchetii strains and Phaeocystis antarctica able to accumulate up to 6–20% cellular carbon in DMSP (Matrai et al., 1995; Stefels and van Leeuwe, 1998; Bertrand et al., 2007), or Symbiodinium spp., the endosymbiont of reef-building corals producing most DMSP production in tropical regions (Raina et al., 2013). On the basis of these results, a phylogenetic correlation between cobalamin dependency and DMSP production would be valuable, especially taking the recent progress made on DMSP biosynthetic pathways in phytoplankton (Curson et al., 2018) into account. Globally, the auxotrophy for cobalamin in high DMSP synthesizers implies that limitation of this cofactor could reduce DMSP production in numerous oceanic regions. As cobalamin production is performed only by a subset of bacteria and archaea in marine systems, it appears that these prokaryotic communities exert an important control on phytoplankton DMSP synthesis in some oceanic regions. The present study participates in expanding the knowledge of the links between cobalt and sulfur biogeochemical cycles.
Data Availability Statement
The datasets presented in this study can be found in online repositories. The names of the repository/repositories and accession number(s) can be found in the article/Supplementary Material.
Author Contributions
CN, FM, RK, and MG conceived and designed the experiment. CN, CH, ÉN, J-BB, FH, and AC performed the experiments. CN, ÉN, FM, and MG analyzed the data. CN conceived the figures and wrote the manuscript with inputs from all authors. All authors read and approved the final version of the manuscript.
Funding
This work was part of the Atlantic Microalgae project, we acknowledge funding by the region Pays de la Loire, France. Proteomics analyses were performed on the PAPPSO platform (http://pappso.inra.fr) which is supported by INRA (http://www.inra.fr), the Ile-de-France regional council (https://www.iledefrance.fr/education-recherche), IBiSA (https://www.ibisa.net), and CNRS (http://www.cnrs.fr).
Conflict of Interest
The authors declare that the research was conducted in the absence of any commercial or financial relationships that could be construed as a potential conflict of interest.
Acknowledgments
Part of this work has been published previously in the Ph.D. thesis of CN (Nef, 2019) and is available online.
Supplementary Material
The Supplementary Material for this article can be found online at: https://www.frontiersin.org/articles/10.3389/fmars.2020.569560/full#supplementary-material
Footnotes
References
Adamberg, K., Valgepea, K., and Vilu, R. (2015). Advanced continuous cultivation methods for systems microbiology. Microbiology 161, 1707–1719. doi: 10.1099/mic.0.000146
Aguilar, C., Raina, J.-B., Motti, C. A., Fôret, S., Hayward, D. C., Lapeyre, B., et al. (2017). Transcriptomic analysis of the response of Acropora millepora to hypo-osmotic stress provides insights into DMSP biosynthesis by corals. BMC Genomics 18:612. doi: 10.1186/s12864-017-3959-0
Albers, E. (2009). Metabolic characteristics and importance of the Universal Methionine Salvage Pathway Recycling Methionine from 5 ’-Methylthioadenosine. IUBMB Life 61, 1132–1142. doi: 10.1002/iub.278
Alcolombri, U., Ben-Dor, S., Feldmesser, E., Levin, Y., Tawfik, D. S., and Vardi, A. (2015). Identification of the algal dimethyl sulfide-releasing enzyme: a missing link in the marine sulfur cycle. Science 348, 1466–1469. doi: 10.1126/science.aab1586
Alipanah, L., Rohloff, J., Winge, P., Bones, A. M., and Brembu, T. (2015). Whole-cell response to nitrogen deprivation in the diatom Phaeodactylum tricornutum. J. Exp. Bot. 66, 6281–6296. doi: 10.1093/jxb/erv340
Allen, A. E., Dupont, C. L., Oborník, M., Horák, A., Nunes-Nesi, A., McCrow, J. P., et al. (2011). Evolution and metabolic significance of the urea cycle in photosynthetic diatoms. Nature 473, 203–207. doi: 10.1038/nature10074
Archer, S. D., Tarran, G. A., Stephens, J. A., Butcher, L. J., and Kimmance, S. A. (2011). Combining cell sorting with gas chromatography to determine phytoplankton group-specific intracellular dimethylsulphoniopropionate. Aquat. Microb. Ecol. 62, 109–121. doi: 10.3354/ame01464
Armbrust, E. V., Berges, J. A., Bowler, C., Green, B. R., Martinez, D., Putnam, N. H., et al. (2004). The genome of the diatom Thalassiosira pseudonana: ecology, evolution, and metabolism. Science 306, 79–86. doi: 10.1126/science.1101156
Barak-Gavish, N., Frada, M. J., Ku, C., Lee, P. A., DiTullio, G. R., Malitsky, S., et al. (2018). Bacterial virulence against an oceanic bloom-forming phytoplankter is mediated by algal DMSP. Sci. Adv. 4:eaau5716. doi: 10.1126/sciadv.aau5716
Bell, W., and Mitchell, R. (1972). Chemotactic and growth responses of marine bacteria to algal extracellular products. Biol. Bull. 143, 265–277. doi: 10.2307/1540052
Bendif, E. M., Probert, I., Schroeder, D. C., and de Vargas, C. (2013). On the description of Tisochrysis lutea gen. nov. sp. nov. and Isochrysis nuda sp. nov. in the Isochrysidales, and the transfer of Dicrateria to the Prymnesiales (Haptophyta). J. Appl. Phycol. 25, 1763–1776. doi: 10.1007/s10811-013-0037-0
Bertrand, E. M., and Allen, A. E. (2012). Influence of vitamin B auxotrophy on nitrogen metabolism in eukaryotic phytoplankton. Front. Microbiol. 3:375. doi: 10.3389/fmicb.2012.00375
Bertrand, E. M., Allen, A. E., Dupont, C. L., Norden-Krichmar, T. M., Bai, J., Valas, R. E., et al. (2012). Influence of cobalamin scarcity on diatom molecular physiology and identification of a cobalamin acquisition protein. Proc. Natl. Acad. Sci. U.S.A. 1762–1771.
Bertrand, E. M., Moran, D. M., McIlvin, M. R., Hoffman, J. M., Allen, A. E., and Saito, M. A. (2013). Methionine synthase interreplacement in diatom cultures and communities: implications for the persistence of B12 use by eukaryotic phytoplankton. Limnol. Oceanogr. 58, 1431–1450. doi: 10.4319/lo.2013.58.4.1431
Bertrand, E. M., Saito, M. A., Rose, J. M., Riesselman, C. R., Lohan, M. C., Noble, A. E., et al. (2007). Vitamin B12 and iron colimitation of phytoplankton growth in the Ross Sea. Limnol. Oceanogr. 52, 1079–1093. doi: 10.4319/lo.2007.52.3.1079
Bromke, M. A., and Hesse, H. (2015). Phylogenetic analysis of methionine synthesis genes from Thalassiosira pseudonana. SpringerPlus 4:391. doi: 10.1186/s40064-015-1163-8
Browning, T. J., Achterberg, E. P., Rapp, I., Engel, A., Bertrand, E. M., Tagliabue, A., et al. (2017). Nutrient co-limitation at the boundary of an oceanic gyre. Nature 551, 242–246. doi: 10.1038/nature24063
Browning, T. J., Rapp, I., Schlosser, C., Gledhill, M., Achterberg, E. P., Bracher, A., et al. (2018). Influence of iron, cobalt, and vitamin B 12 supply on phytoplankton growth in the tropical East Pacific during the 2015 El Niño. Geophys. Res. Lett. 45, 1–10. doi: 10.1029/2018GL077972
Bull, A. T. (2010). The renaissance of continuous culture in the post-genomics age. J. Ind. Microbiol. Biotechnol. 37, 993–1021. doi: 10.1007/s10295-010-0816-4
Carrier, G., Baroukh, C., Rouxel, C., Duboscq-Bidot, L., Schreiber, N., and Bougaran, G. (2017). Draft Genomes of the Algae Tisochrysis lutea Strains. Available online at: https://www.seanoe.org/data/00361/47171/ (accessed September 20, 2020).
Caruana, A. M. N., and Malin, G. (2014). The variability in DMSP content and DMSP lyase activity in marine dinoflagellates. Prog. Oceanogr. 120, 410–424. doi: 10.1016/j.pocean.2013.10.014
Charlson, R. J., Lovelock, J. E., Andreae, M. O., and Warren, S. G. (1987). Oceanic phytoplankton, atmospheric sulphur, cloud albedo and climate. Nature 326, 655–661. doi: 10.1038/326655a0
Cheng, Z., Li, K., Hammad, L. A., Karty, J. A., and Bauer, C. E. (2014). Vitamin B12 regulates photosystem gene expression via the CrtJ antirepressor AerR in Rhodobacter capsulatus. Mol. Microbiol. 91, 649–664. doi: 10.1111/mmi.12491
Chiang, P. K., Gordon, R. K., Tal, J., Zeng, G. C., Doctor, B. P., Pardhasaradhi, K., et al. (1996). S-Adenosylmethionine and methylation. FASEB J. 10, 471–480.
Chu, H. D., Le, Q. N., Nguyen, H. Q., and Le, D. T. (2016). Genome-wide analysis of genes encoding methionine-rich proteins in arabidopsis and soybean suggesting their roles in the adaptation of plants to abiotic stress. Int. J. Genomics 2016:5427062. doi: 10.1155/2016/5427062
Croft, M. T., Lawrence, A. D., Raux-Deery, E., Warren, M. J., and Smith, A. G. (2005). Algae acquire vitamin B12 through a symbiotic relationship with bacteria. Nature 483, 90–93. doi: 10.1038/nature04056
Curson, A. R. J., Williams, B. T., Pinchbeck, B. J., Sims, L. P., Martínez, A. B., Rivera, P. P. L., et al. (2018). DSYB catalyses the key step of dimethylsulfoniopropionate biosynthesis in many phytoplankton. Nat. Microbiol. 3, 430–439. doi: 10.1038/s41564-018-0119-5
del Valle, D., Martínez-García, S., Sañudo-Wilhelmy, S. A., Kiene, R., and Karl, D. (2015). Methionine and dimethylsulfoniopropionate as sources of sulfur to the microbial community of the North Pacific Subtropical Gyre. Aquat. Microb. Ecol. 75, 103–116. doi: 10.3354/ame01750
Dong, H.-P., Williams, E., Wang, D., Xie, Z.-X., Hsia, R., Jenck, A., et al. (2013). Responses of Nannochloropsis oceanica IMET1 to Long-term nitrogen starvation and recovery1[C][W][OA]. Plant Physiol. 162, 1110–1126. doi: 10.1104/pp.113.214320
Dubois, M., Gilles, K. A., Hamilton, J. K., Rebers, P. A., and Smith, F. (1956). Colorimetric method for determination of sugars and related substances. Anal. Chem. 28, 350–356. doi: 10.1021/ac60111a017
Franklin, D., Steinke, M., Young, J., Probert, I., and Malin, G. (2010). Dimethylsulphoniopropionate (DMSP), DMSP-lyase activity (DLA) and dimethylsulphide (DMS) in 10 species of coccolithophore. Mar. Ecol. Prog. Ser. 410, 13–23. doi: 10.3354/meps08596
Fujimori, D. G. (2013). Radical SAM-mediated methylation reactions. Curr. Opin. Chem. Biol. 17, 597–604. doi: 10.1016/j.cbpa.2013.05.032
Gage, D. A., Rhodes, D., Nolte, K. D., Hicks, W. A., Leustek, T., Cooper, A. J. L., et al. (1997). A new route for synthesis of dimethylsulphoniopropionate in marine algae. Nature 387, 891–894. doi: 10.1038/43160
Garnier, M., Bougaran, G., Pavlovic, M., Bérard, J.-B., Carrier, G., Charrier, A., et al. (2016). Use of a lipid rich strain reveals mechanisms of nitrogen limitation and carbon partitioning in the haptophyte Tisochrysis lutea. Algal Res. 20, 229–248. doi: 10.1016/j.algal.2016.10.017
Garnier, M., Carrier, G., Rogniaux, H., Nicolau, E., Bougaran, G., Saint-Jean, B., et al. (2014). Comparative proteomics reveals proteins impacted by nitrogen deprivation in wild-type and high lipid-accumulating mutant strains of Tisochrysis lutea. J. Proteomics 105, 107–120. doi: 10.1016/j.jprot.2014.02.022
Ge, F., Huang, W., Chen, Z., Zhang, C., Xiong, Q., Bowler, C., et al. (2014). Methylcrotonyl-CoA Carboxylase Regulates Triacylglycerol Accumulation in the Model Diatom Phaeodactylum tricornutum. Plant Cell 26, 1681–1697. doi: 10.1105/tpc.114.124982
Gebser, B., and Pohnert, G. (2013). Synchronized regulation of different zwitterionic metabolites in the osmoadaption of phytoplankton. Mar. Drugs 11, 2168–2182. doi: 10.3390/md11062168
Geider, R., and La Roche, J. (2002). Redfield revisited: variability of C:N:P in marine microalgae and its biochemical basis. Eur. J. Phycol. 37, 1–17. doi: 10.1017/S0967026201003456
Geider, R., Macintyre, Graziano, L., and McKay, R. M. (1998). Responses of the photosynthetic apparatus of Dunaliella tertiolecta (Chlorophyceae) to nitrogen and phosphorus limitation. Eur. J. Phycol. 33, 315–332. doi: 10.1080/09670269810001736813
Georges des Aulnois, M., Roux, P., Caruana, A., Réveillon, D., Briand, E., Hervé, F., et al. (2019). Physiological and metabolic responses of freshwater and brackish strains of Microcystis aeruginosa acclimated to a salinity gradient: insight into salt tolerance. Appl. Environ. Microbiol. 85:e01614-19. doi: 10.1128/AEM.01614-19
Gobler, C. J., Norman, C., Panzeca, C., Taylor, G. T., and Sañudo-Wilhelmy, S. A. (2007). Effect of B-vitamins (B1, B12) and inorganic nutrients on algal bloom dynamics in a coastal ecosystem. Aquat. Microb. Ecol. 49, 181–194. doi: 10.3354/ame01132
González, J. M., Kiene, R. P., and Moran, M. A. (1999). Transformation of sulfur compounds by an abundant lineage of marine bacteria in the alpha-subclass of the class Proteobacteria. Appl. Environ. Microbiol. 65, 3810–3819. doi: 10.1128/aem.65.9.3810-3819.1999
González, J. M., Simo, R., Massana, R., Covert, J. S., Casamayor, E. O., Pedros-Alio, C., et al. (2000). Bacterial community structure associated with a Dimethylsulfoniopropionate-Producing north Atlantic algal bloom. Appl. Environ. Microbiol. 66, 4237–4246. doi: 10.1128/AEM.66.10.4237-4246.2000
Heal, K. R., Carlson, L. T., Devol, A. H., Armbrust, E. V., Moffett, J. W., Stahl, D. A., et al. (2014). Determination of four forms of vitamin B12 and other B vitamins in seawater by liquid chromatography/tandem mass spectrometry. Rapid Commun. Mass Spectrom. 28, 2398–2404. doi: 10.1002/rcm.7040
Heal, K. R., Kellogg, N. A., Carlson, L. T., Lionheart, R. M., and Ingalls, A. E. (2019). Metabolic Consequences of Cobalamin Scarcity in the Diatom Thalassiosira pseudonana as Revealed Through Metabolomics. Protist 170, 328–348. doi: 10.1016/j.protis.2019.05.004
Helliwell, E. K., Wheeler, G. L., Leptos, K. C., Goldstein, R. E., and Smith, A. G. (2011). Insights into the evolution of vitamin B12 auxotrophy from sequenced algal genomes. Trends Genet. 29, 469–478.
Helliwell, K. E., Collins, S., Kazamia, E., Wheeler, G. L., and Smith, A. G. (2015). Fundamental shift in vitamin B12 eco-physiology of a model alga demonstrated by experimental evolution. ISME J. 9, 1446–1455. doi: 10.1038/ismej.2014.230
Helliwell, K. E., Lawrence, A. D., Holzer, A., Kudahl, U. J., Sasso, S., Kräutler, B., et al. (2016). Cyanobacteria and eukaryotic algae use different chemical variants of vitamin B12. Curr. Biol. 26, 999–1008. doi: 10.1016/j.cub.2016.02.041
Helliwell, K. E., Scaife, M. A., Sasso, S., Ulian Araujo, A. P., Purton, S., and Smith, A. G. (2014). Unraveling vitamin B12-responsive gene regulation in algae. Plant Physiol. 165, 388–397. doi: 10.1104/pp.113.234369
Hirashima, T., Toyoshima, M., Moriyama, T., Nakamura, Y., and Sato, N. (2017). Characterization of phosphoethanolamine-N-methyltransferases in green algae. Biochem. Biophys. Res. Commun. 488, 141–146. doi: 10.1016/j.bbrc.2017.05.026
Hoskisson, P. A., and Hobbs, G. (2005). Continuous culture - making a comeback? Microbiology 151, 3153–3159. doi: 10.1099/mic.0.27924-0
Howard, E. C., Sun, S., Biers, E. J., and Moran, M. A. (2008). Abundant and diverse bacteria involved in DMSP degradation in marine surface waters. Environ. Microbiol. 10, 2397–2410. doi: 10.1111/j.1462-2920.2008.01665.x
Jakubowski, H. (2004). Molecular basis of homocysteine toxicity in humans. Cell. Mol. Life Sci. 61, 470–487. doi: 10.1007/s00018-003-3204-7
Jardillier, L., Zubkov, M. V., Pearman, J., and Scanlan, D. J. (2010). Significant CO2 fixation by small prymnesiophytes in the subtropical and tropical northeast Atlantic Ocean. ISME J. 4, 1180–1192. doi: 10.1038/ismej.2010.36
Jordan, R. W., and Chamberlain, A. H. L. (1997). Biodiversity among haptophyte algae. Biodivers. Conserv. 6, 131–152.
Kaleta, C., Schäuble, S., Rinas, U., and Schuster, S. (2013). Metabolic costs of amino acid and protein production in Escherichia coli. Biotechnol. J. 8, 1105–1114. doi: 10.1002/biot.201200267
Keller, M. D. (1991). Dimethyl sulfide production and marine phytoplankton: the importance of species composition and cell size. Biol. Oceanogr. 6, 375–382. doi: 10.1080/01965581.1988.10749540
Keller, M. D., Bellows, W. K., and Guillard, R. R. L. (1989). “Dimethyl sulfide production in marine phytoplankton,” in Biogenic Sulfur in the Environment, eds E. S. Saltzman and W. J. Cooper (Washington, DC: American Chemical Society), 167–182. doi: 10.1021/bk-1989-0393.ch011
Keller, M. D., Kiene, R. P., Matrai, P. A., and Bellows, W. K. (1999). Production of glycine betaine and dimethylsulfoniopropionate in marine phytoplankton. I. Batch cultures. Mar. Biol. 135, 237–248. doi: 10.1007/s002270050621
Keller, M. D., Matrai, P. A., Kiene, R. P., and Bellows, W. K. (2004). Responses of coastal phytoplankton populations to nitrogen additions: dynamics of cell-associated dimethylsulfoniopropionate (DMSP), glycine betaine (GBT), and homarine. Can. J. Fish. Aquat. Sci. 61, 685–699. doi: 10.1139/f04-058
Kessler, R. W., Weiss, A., Kuegler, S., Hermes, C., and Wichard, T. (2018). Macroalgal-bacterial interactions: Role of dimethylsulfoniopropionate in microbial gardening by Ulva (Chlorophyta). Mol. Ecol. 27, 1808–1819. doi: 10.1111/mec.14472
Kiene, R. P., Linn, L. J., and Bruton, J. A. (2000). New and important roles for DMSP in marine microbial communities. J. Sea Res. 43, 209–224. doi: 10.1016/S1385-1101(00)00023-X
Kiene, R. P., Linn, L. J., González, J., Moran, M. A., and Bruton, J. A. (1999). Dimethylsulfoniopropionate and methanethiol are important precursors of methionine and protein-sulfur in marine bacterioplankton. Appl. Environ. Microbiol. 65, 4549–4558. doi: 10.1128/aem.65.10.4549-4558.1999
Koch, F., Marcoval, M. A., Panzeca, C., Bruland, K. W., Sañudo-Wilhelmy, S. A., and Gobler, C. J. (2011). The effect of vitamin B12 on phytoplankton growth and community structure in the Gulf of Alaska. Limnol. Oceanogr. 56, 1023–1034. doi: 10.4319/lo.2011.56.3.1023
Kozbial, P. Z., and Mushegian, A. R. (2005). Natural history of S-adenosylmethionine-binding proteins. BMC Struct. Biol. 5:19. doi: 10.1186/1472-6807-5-19
Levitan, O., Dinamarca, J., Zelzion, E., Lun, D. S., Guerra, L. T., Kim, M. K., et al. (2015). Remodeling of intermediate metabolism in the diatom Phaeodactylum tricornutum under nitrogen stress. Proc. Natl. Acad. Sci. U.S.A. 112, 412–417. doi: 10.1073/pnas.1419818112
Li, G.-W., Burkhardt, D., Gross, C., and Weissman, J. S. (2014). Quantifying absolute protein synthesis rates reveals principles underlying allocation of cellular resources. Cell 157, 624–635. doi: 10.1016/j.cell.2014.02.033
Liao, C., and Seebeck, F. P. (2019). In vitro reconstitution of bacterial DMSP biosynthesis. Angew. Chem. 131, 3591–3594. doi: 10.1002/ange.201814662
Liu, H., Probert, I., Uitz, J., Claustre, H., Aris-Brosou, S., Frada, M., et al. (2009). Extreme diversity in noncalcifying haptophytes explains a major pigment paradox in open oceans. Proc. Natl. Acad. Sci. U.S.A. 106, 12803–12808. doi: 10.1073/pnas.0905841106
Lovelock, J. E., Maggs, R. J., and Rasmussen, R. A. (1972). Atmospheric dimethyl sulphide and the natural sulphur cycle. Nature 237, 452–453. doi: 10.1038/237452a0
Majumdar, R., Barchi, B., Turlapati, S. A., Gagne, M., Minocha, R., Long, S., et al. (2016). Glutamate, ornithine, arginine, proline, and polyamine metabolic interactions: the pathway is regulated at the post-transcriptional level. Front. Plant Sci. 7:78. doi: 10.3389/fpls.2016.00078
Malin, G., and Kirst, G. O. (1997). Algal production of dimethyl sulfide and its atmospheric role. J. Phycol. 33, 889–896. doi: 10.1111/j.0022-3646.1997.00889.x
Malin, G., Turner, S. M., and Liss, P. S. (1992). Sulfur: the plankton/climate connection. J. Phycol. 28, 590–597. doi: 10.1111/j.0022-3646.1992.00590.x
Martens, J.-H., Barg, H., Warren, M. J., and Jahn, D. (2002). Microbial production of vitamin B12. Appl. Microbiol. Biotechnol.
Matrai, P. A., Vernet, M., Hood, R., Jennings, A., Brody, E., and Saemundsdóttir, S. (1995). Light-dependence of carbon and sulfur production by polar clones of the genus Phaeocystis. Mar. Biol. 124, 157–167. doi: 10.1007/BF00349157
Milliman, J. D. (1993). Production and accumulation of calcium carbonate in the ocean: Budget of a nonsteady state. Glob. Biogeochem. Cycles 7, 927–957. doi: 10.1029/93GB02524
Nef, C. (2019). Métabolisme et Interactions Bactériennes en lien Avec la Vitamine B12 Chez la Microalgue Haptophyte Tisochrysis lutea. Ph.D. thesis, Université de Nantes, Nantes.
Nef, C., Henry, C., Mairet, F., and Garnier, M. (2019a). Proteome of T. lutea in B12 and N limitation. Available online at: https://www.seanoe.org/data/00508/62006/ (accessed September 20, 2020).
Nef, C., Jung, S., Mairet, F., Kaas, R., Grizeau, D., and Garnier, M. (2019b). How haptophytes microalgae mitigate vitamin B12 limitation. Sci. Rep. 9:8417. doi: 10.1038/s41598-019-44797-w
Not, F., Latasa, M., Scharek, R., Viprey, M., Karleskind, P., Balagué, V., et al. (2008). Protistan assemblages across the Indian Ocean, with a specific emphasis on the picoeukaryotes. Deep Sea Res. Part Oceanogr. Res. Pap. 55, 1456–1473. doi: 10.1016/j.dsr.2008.06.007
O’Brien, C. J., Peloquin, J. A., Vogt, M., Heinle, M., Gruber, N., Ajani, P., et al. (2013). Global marine plankton functional type biomass distributions: coccolithophores. Earth Syst. Sci. Data 5, 259–276. doi: 10.5194/essd-5-259-2013
Panzeca, C., Beck, A. J., Tovar-Sanchez, A., Segovia-Zavala, J., Taylor, G. T., Gobler, C. J., et al. (2009). Distributions of dissolved vitamin B12 and Co in coastal and open-ocean environments. Estuar. Coast. Shelf Sci. 85, 223–230. doi: 10.1016/j.ecss.2009.08.016
Panzeca, C., Tovar-Sanchez, A., Agusti, S., Reche, I., Duarte, C. M., Taylor, G. T., et al. (2006). B vitamins as regulators of phytoplankton dynamics. EOS Trans. Am. Geophys. Union 49, 594–596.
Pejchal, R., and Ludwig, M. L. (2004). Cobalamin-independent methionine synthase (MetE): a face-to-face double barrel that evolved by gene duplication. PLoS Biol. 3:e31. doi: 10.1371/journal.pbio.0030031
Petrou, K., and Nielsen, D. A. (2018). Uptake of dimethylsulphoniopropionate (DMSP) by the diatom Thalassiosira weissflogii: a model to investigate the cellular function of DMSP. Biogeochemistry 141, 265–271. doi: 10.1007/s10533-018-0507-1
Raina, J.-B., Tapiolas, D. M., Forêt, S., Lutz, A., Abrego, D., Ceh, J., et al. (2013). DMSP biosynthesis by an animal and its role in coral thermal stress response. Nature 502, 677–680. doi: 10.1038/nature12677
Rellinger, A. N., Kiene, R. P., del Valle, D. A., Kieber, D. J., Slezak, D., Harada, H., et al. (2009). Occurrence and turnover of DMSP and DMS in deep waters of the Ross Sea, Antarctica. Deep Sea Res. Part Oceanogr. Res. Pap. 56, 686–702. doi: 10.1016/j.dsr.2008.12.010
Remmers, I. M., D’Adamo, S., Martens, D. E., de Vos, R. C. H., Mumm, R., America, A. H. P., et al. (2018). Orchestration of transcriptome, proteome and metabolome in the diatom Phaeodactylum tricornutum during nitrogen limitation. Algal Res. 35, 33–49. doi: 10.1016/j.algal.2018.08.012
Rhodes, D., Gage, D. A., Cooper, A. J. L., and Hanson, A. D. (1997). S-Methylmethionine Conversion to Dimethylsulfoniopropionate: evidence for an Unusual Transamination Reaction. Plant Physiol. 115, 1541–1548. doi: 10.1104/pp.115.4.1541
Roe, A. J., O’Byrne, C., McLaggan, D., and Booth, I. R. (2002). Inhibition of Escherichia coli growth by acetic acid: a problem with methionine biosynthesis and homocysteine toxicity. Microbiology 148, 2215–2222. doi: 10.1099/00221287-148-7-2215
Roje, S. (2006). S-adenosyl-l-methionine: beyond the universal methyl group donor. Phytochemistry 67, 1686–1698. doi: 10.1016/j.phytochem.2006.04.019
Sando, G., Blakley, R., Hogenkamp, H., and Hoffmann, P. (1975). Studies on mechanism of adenosylcobalamin-dependent ribonucleotide reduction by use of analogs of coenzyme. J. Biol. Chem. 250, 8774–8779.
Sañudo-Wilhelmy, S. A., Cutter, L. S., Durazo, R., Smail, E. A., Gómez-Consarnau, L., Webb, E. A., et al. (2012). Multiple B-vitamin depletion in large areas of the coastal ocean. Proc. Natl. Acad. Sci. U.S.A. 109, 14041–14045. doi: 10.1073/pnas.1208755109
Sauter, M., Moffatt, B., Saechao, M. C., Hell, R., and Wirtz, M. (2013). Methionine salvage and S-adenosylmethionine: essential links between sulfur, ethylene and polyamine biosynthesis. Biochem. J. 451, 145–154. doi: 10.1042/BJ20121744
Serive, B., Nicolau, E., Berard, J.-B., Kaas, R., Pasquet, V., Picot, L., et al. (2017). Community analysis of pigment patterns from 37 microalgae strains reveals new carotenoids and porphyrins characteristic of distinct strains and taxonomic groups. PLoS One 12:e0171872. doi: 10.1371/journal.pone.0171872
Seymour, J. R., Simo, R., Ahmed, T., and Stocker, R. (2010). Chemoattraction to dimethylsulfoniopropionate throughout the marine microbial food web. Science 329, 342–345. doi: 10.1126/science.1188418
Shang, C., Zhu, S., Wang, Z., Qin, L., Alam, M. A., Xie, J., et al. (2017). Proteome response of Dunaliella parva induced by nitrogen limitation. Algal Res. 23, 196–202. doi: 10.1016/j.algal.2017.01.016
Simó, R., Archer, S. D., Pedrós-Alió, C., Gilpin, L., and Stelfox-Widdicombe, C. E. (2002). Coupled dynamics of dimethylsulfoniopropionate and dimethylsulfide cycling and the microbial food web in surface waters of the North Atlantic. Limnol. Oceanogr. 47, 53–61. doi: 10.4319/lo.2002.47.1.0053
Spiese, C., and Tatarkov, E. (2014). Dimethylsulfoxide reduction activity is linked to nutrient stress in Thalassiosira pseudonana NCMA 1335. Mar. Ecol. Prog. Ser. 507, 31–38. doi: 10.3354/meps10842
Stefels, J. (2000). Physiological aspects of the production and conversion of DMSP in marine algae and higher plants. J. Sea Res. 43, 183–197. doi: 10.1016/s1385-1101(00)00030-7
Stefels, J., Steinke, M., Turner, S., Malin, G., and Belviso, S. (2007). Environmental constraints on the production and removal of the climatically active gas dimethylsulphide (DMS) and implications for ecosystem modelling. Biogeochemistry 83, 245–275. doi: 10.1007/s10533-007-9091-5
Stefels, J., and van Leeuwe, M. A. (1998). Effects of iron and light stress on the biochemical composition of antarctic Phaeocystis Sp. (prymnesiophyceae). I. Intracellular Dmsp Concentrations. J. Phycol. 34, 486–495. doi: 10.1046/j.1529-8817.1998.340486.x
Steinke, M., Wolfe, G., and Kirst, G. (1998). Partial characterisation of dimethylsulfoniopropionate (DMSP) lyase isozymes in 6 strains of Emiliania huxleyi. Mar. Ecol. Prog. Ser. 175, 215–225. doi: 10.3354/meps175215
Struck, A.-W., Thompson, M. L., Wong, L. S., and Micklefield, J. (2012). S-adenosyl-methionine-dependent methyltransferases: highly versatile enzymes in biocatalysis, biosynthesis and other biotechnological applications. ChemBioChem 13, 2642–2655. doi: 10.1002/cbic.201200556
Suffridge, C. P., Gómez-Consarnau, L., Monteverde, D. R., Cutter, L., Arístegui, J., Alvarez-Salgado, X. A., et al. (2018). B vitamins and their congeners as potential drivers of microbial community composition in an oligotrophic marine ecosystem. J. Geophys. Res. Biogeosci. 123, 2890–2907. doi: 10.1029/2018jg004554
Sunda, W., Kieber, D. J., Kiene, R. P., and Huntsman, S. (2002). An antioxidant function for DMSP and DMS in marine algae. Nature 418, 317–320. doi: 10.1038/nature00851
Sunda, W. G., Hardison, R., Kiene, R. P., Bucciarelli, E., and Harada, H. (2007). The effect of nitrogen limitation on cellular DMSP and DMS release in marine phytoplankton: climate feedback implications. Aquat. Sci. 69, 341–351. doi: 10.1007/s00027-007-0887-0
Tang, K. W., and Simó, R. (2003). Trophic uptake and transfer of DMSP in simple planktonic food chains. Aquat. Microb. Ecol. 31, 193–202. doi: 10.3354/ame031193
Thomsen, H., Buck, K. R., and Chavez, F. P. (1994). “Haptophytes as components of marine phytoplankton,” in The Haptophyte Algae, eds J. C. Green, and B. S. C. Leadbeater (Oxford: Clarendon Press), 187–208.
Tripp, H. J., Kitner, J. B., Schwalbach, M. S., Dacey, J. W. H., Wilhelm, L. J., and Giovannoni, S. J. (2008). SAR11 marine bacteria require exogenous reduced sulphur for growth. Nature 452, 741–744. doi: 10.1038/nature06776
Valot, B., Langella, O., Nano, E., and Zivy, M. (2011). MassChroQ: a versatile tool for mass spectrometry quantification. Proteomics 11, 3572–3577. doi: 10.1002/pmic.201100120
Vogt, M., O’Brien, C., Peloquin, J., Schoemann, V., Breton, E., Estrada, M., et al. (2012). Global marine plankton functional type biomass distributions: Phaeocystis spp. Earth Syst. Sci. Data 4, 107–120. doi: 10.5194/essd-4-107-2012
Walne, P. R. (1970). Studies on the Food Value of Nineteen Genera of Algae to Juvenile Bivalves of the Genera Ostrea, Crassostrea, Mercenaria and Mytilus. (London: Fishery Investigations), 1–62.
Warren, M. J., Raux, E., Schubert, H. L., and Escalante-Semeran, J. C. (2002). The biosynthesis of adenosylcobalamin (vitamin B12). Nat. Prod. Rep. 19, 390–412. doi: 10.1039/b108967f
Keywords: cobalamin, vitamin B12, haptophyte microalgae, Tisochrysis lutea, DMSP, chemostat, comparative proteomics, metabolism
Citation: Nef C, Henry C, Nicolau É, Bérard J-B, Hervé F, Caruana AMN, Kaas R, Mairet F and Garnier M (2020) Cobalamin Scarcity Modifies Carbon Allocation and Impairs DMSP Production Through Methionine Metabolism in the Haptophyte Microalgae Tisochrysis lutea. Front. Mar. Sci. 7:569560. doi: 10.3389/fmars.2020.569560
Received: 04 June 2020; Accepted: 22 September 2020;
Published: 08 October 2020.
Edited by:
Haiwei Luo, The Chinese University of Hong Kong, ChinaReviewed by:
Anitra E. Ingalls, University of Washington, United StatesBryndan Paige Durham, University of Florida, United States
Rafel Simó, Consejo Superior de Investigaciones Científicas (CSIC), Spain
Copyright © 2020 Nef, Henry, Nicolau, Bérard, Hervé, Caruana, Kaas, Mairet and Garnier. This is an open-access article distributed under the terms of the Creative Commons Attribution License (CC BY). The use, distribution or reproduction in other forums is permitted, provided the original author(s) and the copyright owner(s) are credited and that the original publication in this journal is cited, in accordance with accepted academic practice. No use, distribution or reproduction is permitted which does not comply with these terms.
*Correspondence: Charlotte Nef, bmVmY2hhcmxvdHRlQHlhaG9vLmZy; Y25lZkBiaW8uZW5zLnBzbC5ldQ==; Matthieu Garnier, bWF0dGhpZXUuZ2FybmllckBpZnJlbWVyLmZy; bWdhcm5pZXJAaWZyZW1lci5mcg==
†Present address: Charlotte Nef, Institut de Biologie de l’École Normale Supérieure (IBENS), École Normale Supérieure, CNRS, INSERM, PSL Université Paris, Paris, France