- 1Department of Marine Zoology, Senckenberg Research Institute and Natural History Museum, Frankfurt, Germany
- 2Institute of Ecology, Diversity and Evolution, Goethe University Frankfurt, Frankfurt, Germany
- 3British Antarctic Survey, Cambridge, United Kingdom
- 4Alfred Wegener Institute for Polar and Marine Research, Bremerhaven, Germany
- 5Ocean Biodiversity Information System (OBIS), Frankfurt, Germany
Climate change is influencing some environmental variables in the Southern Ocean (SO) and this will have an effect on the marine biodiversity. Peracarid crustaceans are one of the dominant and most species-rich groups of the SO benthos. To date, our knowledge on the influence of environmental variables in shaping abundance and species composition in the SO’s peracarid assemblages is limited, and with regard to ice coverage it is unknown. The aim of our study was to assess the influence of sea ice coverage, chlorophyll-a, and phytoplankton concentrations on abundance, distribution and assemblage structure of peracarids. In addition, the influence of other physical parameters on peracarid abundance was assessed, including depth, temperature, salinity, sediment type, current velocity, oxygen, iron, nitrate, silicate and phosphate. Peracarids were sampled with an epibenthic sledge (EBS) in different areas of the Atlantic sector of the SO and in the Weddell Sea. Sampling areas were characterized by different regimes of ice coverage (the ice free South Orkney Islands, the seasonally ice-covered Filchner Trough and the Eastern Antarctic Peninsula including the Prince Gustav Channel which was formerly covered by a perennial ice shelf). In total 64766 individuals of peracarids were collected and identified to order level including five orders: Amphipoda, Cumacea, Isopoda, Mysidacea, and Tanaidacea. Amphipoda was the most abundant taxon, representing 32% of the overall abundances, followed by Cumacea (31%), Isopoda (29%), Mysidacea (4%), and Tanaidacea (4%). The Filchner Trough had the highest abundance of peracarids, while the South Orkney Islands showed the lowest abundance compared to other areas. Ice coverage was the main environmental driver shaping the abundance pattern and assemblage structure of peracarids and the latter were positively correlated with ice coverage and chlorophyll-a concentration. We propose that the positive correlation between sea ice and peracarid abundances is likely due to phytoplankton blooms triggered by seasonal sea ice melting, which might increase the food availability for benthos. Variations in ice coverage extent and seasonality due to climate change would strongly influence the abundance and assemblage structure of benthic peracarids.
Introduction
Peracarids play an important role in marine ecosystems; they can influence the structure and composition of benthic communities (Duffy and Hay, 2000) and they are an important converter of biomass and organic matter in the biogeochemical cycles (Karlson et al., 2007; Dunn et al., 2009). Burrows built by sediment-living peracarids allow oxygenated water to pass through the sediment layer, consequently promoting the mineralization of organic matter by other organisms (Pelegrí and Blackburn, 1994; Lehtonen and Andersin, 1998). Furthermore, peracarid crustaceans can also directly consume organic matter as deposit-feeders and also feed on dead organisms from the sea bottom as scavengers; the assimilated biomass can be then transferred to the higher trophic levels via direct consumption (Jeong et al., 2009; Thiel and Hinojosa, 2009; Duffy et al., 2012). Peracarids are also an important source of food for benthic organisms as well as pelagic fauna such as fish and squid (Mouat et al., 2001; Padovani et al., 2012; Xavier et al., 2020). For example, amphipods represent a large percentage in the diet of many Antarctic species, from benthic invertebrates such as the polynoid polychaete Harmothoe spinosa, to bentho- and bathy-pelagic predators such as cephalopods (e.g., Galiteuthis glacialis), notothenioid fish (e.g., Notothenia coriiceps), and megafaunal predators like penguins (e.g., Eudyptes chrysolophus), and baleen whales (e.g., Balaenoptera borealis) (Dauby et al., 2003). It has been estimated that about 60 million tons of amphipods are consumed every year within the Antarctic food web (Dauby et al., 2003).
Among invertebrates from the SO, Peracarida are one of the dominant and most species-rich groups of benthic fauna (De Broyer and Jazdzewski, 1996; Brandt et al., 2007b; De Broyer and Jazdzewska, 2014; De Broyer and Koubbi, 2014; Kaiser, 2014; Legezyñska et al., 2020). They show high levels of endemism, ranging from 51% (Cumacea) to 88% (Amphipoda) (Brandt, 2000; Brökeland et al., 2007). This might be because the SO’s peracarids are characterized by low dispersal ability due to their reproductive biology (Brandt, 1999). Peracarids have undergone a long period of isolation during their evolution, which was strongly influenced by geological and climatic events over the last 40 Ma (Clarke and Crame, 1992; Lawver et al., 2011). During the Eocene-Oligocene, the opening of a seaway between Australia and East Antarctica and the subsequent opening of the Drake Passage in the Northern Antarctic Peninsula initiated the onset of the Antarctic Circumpolar Current (Lawver et al., 2011). The Antarctic Circumpolar Current is the largest current in the world and promoted the biogeographic isolation of the SO by forming a dispersal barrier for marine species (Barker et al., 2007). Furthermore, during the glacial period of the late Cenozoic, the variation in size and extent of the continental ice sheet influenced the benthic community by forcing the organisms to take refuge on the shelf, and/or to shift their distribution ranges into the deep sea (Thatje et al., 2005). Such events caused a reduced gene flow between the newly separated communities and enhanced speciation. As a result, new species from the deep sea could then recolonize the shallow waters following the glacial retreat (Brey et al., 1996; Hodgson et al., 2003). For example, recent studies showed that different families of isopods underwent multiple colonization events from the shelf to the deep sea; some hypotheses also supported these using molecular experiments (Brandt et al., 2007c; Raupach et al., 2009; Riehl et al., 2020). Cooling events likely caused the extinction of some groups of decapods in the SO (Thatje and Arntz, 2004; Aronson et al., 2009). Consequently, the lack of benthic predators such as lobsters or brachyuran crabs in Antarctica offered new ecological niches to the peracarid crustaceans (Brandt, 1999).
The success of the SO’s peracarids can be further explained by their highly diverse lifestyle and feeding biology (Brökeland et al., 2007; Thiel and Hinojosa, 2009; Brusca et al., 2016). For example, mysidaceans are strictly distributed in the water column, isopods and amphipods have benthic, pelagic, or bentho-pelagic lifestyle (Brökeland et al., 2007). Isopods and amphipods are one of the most dominant components of the emerging benthos (Alldredge and King, 1985; Vallet and Dauvin, 2001; Kiljunen et al., 2020). They perform vertical migrations into the water column during the night, moving benthic resources to the pelagos, thus playing an important role in the benthic-pelagic coupling (Vallet and Dauvin, 2001; Pacheco et al., 2013; Kiljunen et al., 2020). Peracarids include mobile swimmers, bottom-dwelling, and sediment-living species feeding on a wide variety of different food sources. Besides being prey by themselves, they can also be predators, scavengers, suspension-feeders and, among isopods and amphipods (e.g., whale lice), there even are ectoparasites. Cumaceans and tanaidaceans represent a smaller range of lifestyles being more strictly related to the sediment type, they mainly include suspension-feeders and deposit-feeders, but also predators (Thiel and Hinojosa, 2009; Brusca et al., 2016). Despite their high abundance and dominance, the composition pattern of the orders of Peracarida along the SO is still far from being comprehensively understood.
Sediment characteristics and depth have been identified as the most important factors driving faunal abundance and composition patterns in the SO’s peracarids (Brandt et al., 2007b; Rehm et al., 2007). In addition, temperature, oxygen, salinity, primary productivity, and quantity of food influence their diversity and community structure (Brandt et al., 2007a; Ingels et al., 2012; Meyer-Löbbecke et al., 2014). Some of these factors in turn could be affected by sea-ice dynamics: the extent and duration of ice coverage affect the amount of light penetrating the water column, which can positively influence phytoplanktonic activity (Dayton et al., 1994; Runcie and Riddle, 2006; Clark et al., 2017). Moreover, ice melting can also influence the salinity of the upper water column (Haumann et al., 2016).
Apart from sea ice, SO’s peracarids can also be affected by glacial ice, in particular by floating ice shelves, icebergs, or marine terminating glaciers. Ice shelves around Antarctica cover more than 1.561 million km2 (Rignot et al., 2013; Smith et al., 2019) creating conditions of permanent limited light penetration and food depletion, which can last for millennia (Domack et al., 2005; Pudsey et al., 2006). Benthic communities living beneath the ice shelf rely on the lateral advection of food particles (Riddle et al., 2007; Gutt et al., 2011; Smith et al., 2019). Due to limited food and light, benthic communities that live under the ice shelves are more similar to those living in the deep sea (Rose et al., 2015). Icebergs calving from ice shelves can play an important role as a source of physical disturbance in shallow Antarctic benthic marine systems (Gutt and Starmans, 2001; Rack and Rott, 2004; Barnes and Souster, 2011). Iceberg scouring events are one of the main physical processes affecting shallow benthic communities which can be catastrophic (Gutt et al., 1996; Peck et al., 1999; Barnes and Souster, 2011; Valdivia et al., 2020). Iceberg calving events are episodic. Although, in the recent decades rising temperatures and in particular the regional warming along the Antarctic Peninsula caused destabilization leading to disintegration and break-up of several ice shelves (e.g., Larsen A and B and most recently Larsen C in the Eastern Antarctic Peninsula; Rott et al., 1996; Rack and Rott, 2003). Consequently, this caused an increase in the rate of iceberg calving events in the area (Rack and Rott, 2004; Massom et al., 2018).
Sea ice coverage (pack and fast ice) is characterized by strong seasonality, forming in winter and retreating or breaking out during austral summer, and it has a strong influence on the biota underneath. As aforementioned, during its retreat sea ice disperses and allows more light penetration into the upper water column, strongly increasing primary production and triggering phytoplankton blooms. Furthermore, when the ice melts, sea-ice biota are released and enhance the primary productivity and the organic matter input in the water column (e.g., fecal pellets produced by zooplankton). Released algae and fecal pellets can ultimately sink to the sea floor serving as food for the benthos, being able to reach also greater depths (Vanhove et al., 1995; Boetius et al., 2013; Wing et al., 2018). In addition, the land-fast sea ice along the coast prevents drifting icebergs from scouring the seabed (Smale et al., 2008; Smith, 2011; Collares et al., 2018).
Satellite observations show that the overall ice coverage in the Weddell Sea experienced a gradual increase since the early 1980s, particularly in summer. However, long-term trends are superimposed by large multi-year variability, with a recent strong decline beginning in 2016 (Parkinson, 2019; Vernet et al., 2019). The sea ice development is somewhat contradictory to the strong warming experienced by the Antarctic Peninsula region, which is considered one of the most rapidly warming regions of the world (Hansen et al., 2010).
In light of all this, improving our knowledge on composition and distribution of Antarctic benthic communities and their interactions with the environmental abiotic factors is important for prediction of the potential ecological impact induced by on-going climate change. The knowledge of its influence in shaping abundances and species composition in benthic communities of the deep sea is limited and in peracarid crustaceans still remains unknown. This study therefore aims to describe the composition of peracarid crustaceans in different areas of the Weddell Sea, and to investigate the importance of ice coverage and potential driving environmental variables on their abundance, distribution patterns and assemblage structure.
Materials and Methods
Study Area
The peracarid samples were collected from different areas of the Atlantic sector of the SO, mainly in the Weddell Sea, during expeditions of the RRS James Clark Ross and RV Polarstern (Figure 1). The entire area of the Weddell Sea is dominated by the cyclonic Weddell Gyre, which branches off from the warmer and more saline Antarctic Circumpolar Current north of the Antarctic Peninsula going southwards into the Antarctic continental shelves (Fahrbach et al., 1995). In this area, colder deep and bottom waters (Weddell Sea Bottom Water) are produced, released into the gyre and transported back to the north along the Eastern Antarctic Peninsula. Another water mass that contributes to the formation of the Weddell Gyre is the Weddell Sea Deep Water, which originates by mixing processes between surface water masses and a component of the Antarctic Circumpolar Current, the Circumpolar Deep Water (Fahrbach et al., 1995; Vernet et al., 2019).
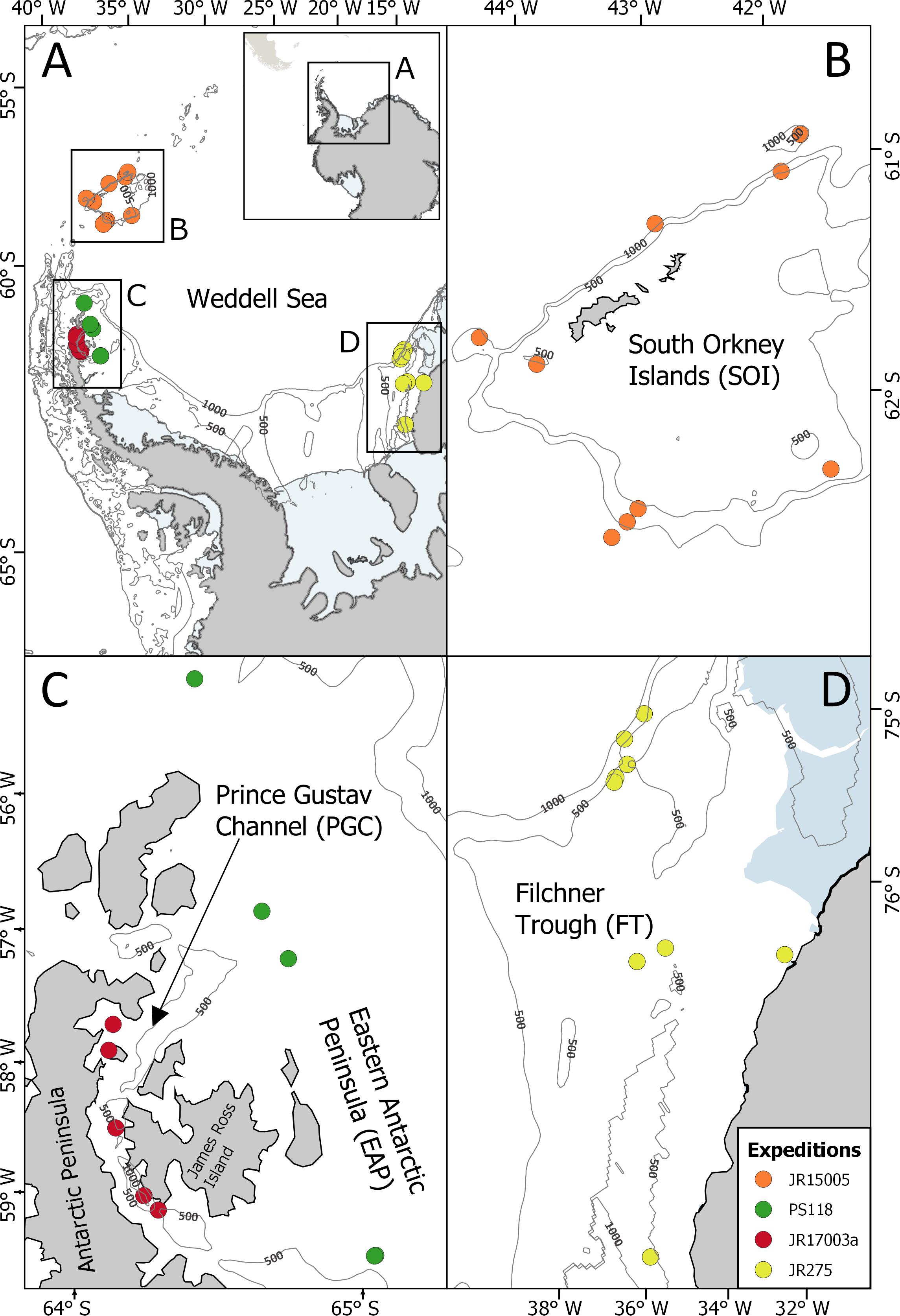
Figure 1. Locations of the EBS stations sampled during the expeditions in the SO (A); JR15005, South Orkney Island, SOI (B); PS118, Eastern Antarctic Peninsula, EAP (C); JR17003a, Prince Gustav Channel, PGC, (C); JR275, Filchner Trough, FT, (D).
The expedition JR15005 with the RRS James Clark Ross worked at the South Orkney Islands in February-March 2016, a small archipelago located in the Northeast of the Antarctic Peninsula (Figures 1A,B, Griffiths, 2017). It is characterized by a great variability in the duration of ice coverage (Murphy et al., 1995; Meredith et al., 2011), the presence of the Antarctic Circumpolar Current in the north and the Weddell Sea Deep Water in the south (Meredith et al., 2011).
During the expedition JR17003a on the RRS James Clarke Ross, sampling was carried out in February–March 2018 in the Prince Gustav Channel, situated in the Eastern Antarctic Peninsula (Figures 1A,C) because the Larsen-C ice shelf, where iceberg A68 calved off in July 2017 (Hogg and Gudmundsson, 2017), could not be reached as originally planned due to heavy pack-ice conditions (Linse, 2018). The Prince Gustav Channel was formerly covered by ice shelf but in 1995, an almost total collapse of the ice shelf (Rott et al., 1998; Pudsey et al., 2001) exposed the area to new environmental conditions, leading to an increase in primary production (Bertolin and Schloss, 2009). The area, especially in the deeper parts, is characterized by drapes of diatom-bearing glacial marine mud, which are typical of the presence of floating ice shelves (Pudsey et al., 2001).
The PS118 expedition of the RV Polarstern in February/March 2019 aimed to reach the Larsen-C ice shelf but also failed to reach this area as well due to very heavy sea-ice conditions (Dorschel, 2019). Therefore, sampling was done to the east of James Ross Island along the Eastern Antarctic Peninsula following a latitudinal gradient transect at an average depth of about 400 m from 63° to 64° south (Figures 1AA,C).
Previously, epibenthic sledge (EBS) samples were taken during the expedition JR275 on the RRS James Clark Ross (February–March 2012) in the Filchner Trough area (South-Eastern Weddell Sea), located in front of the Filchner Ice Shelf (Figures 1A,D) (Griffiths, 2012). The South-Eastern Weddell Sea is relatively inaccessible and under-sampled as it is mostly covered with perennial sea ice and is characterized by the presence of very large icebergs (Årthun et al., 2013; Darelius and Sallée, 2018). The sampled area, situated in the north of the Filchner Ice Shelf, is characterized by seasonality in the ice coverage (Yi et al., 2011) and is affected by super-cooled Ice Shelf Water. The latter originates from the Filchner Ice Shelf and flows northwards mixing with the Weddell Sea Bottom Water, contributing to the formation of the Weddell Gyre (Gordon et al., 2001). Ice Shelf Water and Weddell Sea Bottom Water are characterized by very low temperatures (with Ice Shelf Water reaching −2.3°C) and high level of oxygen concentration (Orsi et al., 1993; Makinson et al., 2011).
Sampling Protocol
During each expedition, peracarid crustaceans were collected using an EBS which consisted of a suprabenthic- and an epibenthic net with a mesh size of 500 μm (cod-ends 300 μm) and was deployed as described by Brenke (2005). The epibenthic net extended from 27 to 60 cm above the seafloor, while the suprabenthic net extended from 100 to 133 cm above the bottom. The sledge was provided with an opening-closing mechanism so that box supra- and epibenthic meshes would immediately close once the gear was lifted (Brenke, 2005). The deployment was carried out for 10 min at a mean velocity of about one knot. Trawling distances were then calculated on the basis of velocity of ship and winch from the start of the trawling until the sledge left the ground, following the equation #4 reported in Brenke (2005). Since the trawling distance between stations was not always the same, in order to compare the different stations, numbers of individuals where standardized to 1000 m haul distances (Tables 1, 2).
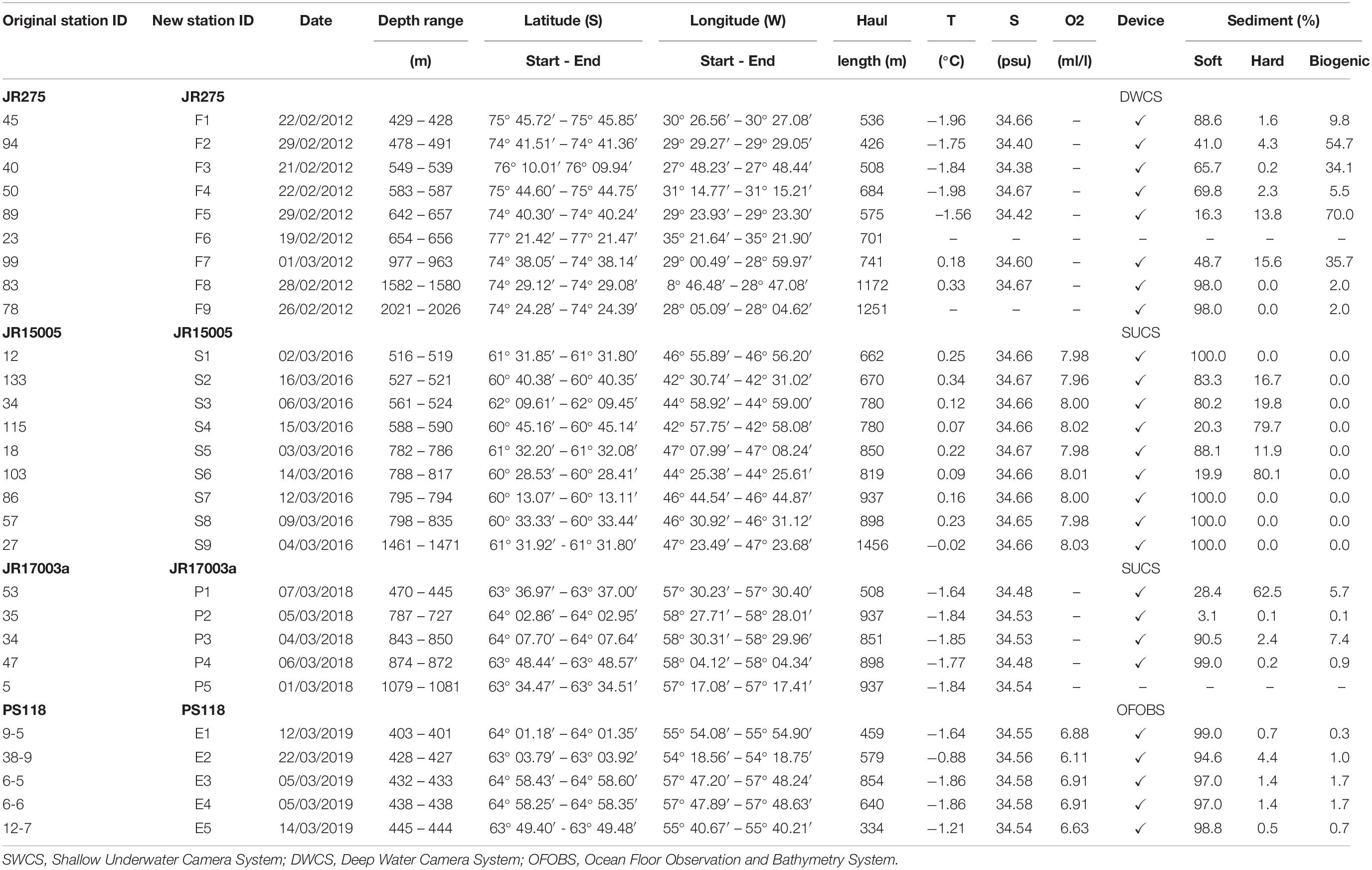
Table 1. Station list of analyzed EBS deployments ordered by depth, CTD and environmental data measured at seafloor.
In order to make station numbers more intuitive and easier to read, original station IDs were changed and reported in Table 1 as “New Station ID.” Nevertheless, original station names from each expedition were included as well (Table 1; “Original Station ID”) to make our results comparable with published data in which the original names were used. A total of 28 EBS and 26 CTDs were deployed at 28 stations in the areas of the Filchner Trough (9 EBS/7 CTDs), the Prince Gustav Channel (5/5), the Eastern Antarctic Peninsula (4/4) and the South Orkney islands (9/9) (Table 1). CTD sensors attached to the EBS collected data about temperature and salinity, except for station F6 and F9 in the Filchner Trough. The characterization of the type of sediment was derived from the analysis of video footage, following the same protocol as in Brasier et al. (2018) for the geomorphologic classification (Table 1). Video footage was recorded using the Shallow Underwater Camera System during expeditions JR15005 and JR17003a, the Deep Water Camera System during JR275 and the Ocean Floor Observation and Bathymetry System during the expedition PS118 (Table 1).
On board samples were sieved with a mesh size of 300 μm and/or directly transferred into precooled (−20°C) 96% ethanol. All ethanol-preserved samples were then stored at −20°C for at least 48 h before further processing, to avoid DNA degradation. On board and later in the laboratory, ethanol-preserved peracarids were further sorted to order level. The number of individuals per sample was counted (raw data; Supplementary Table 1) and compared with the number of individuals standardized to 1000 m haul distance.
Environmental Data
Environmental data not collected during the expeditions were obtained from the “global environmental datasets for marine species distribution modeling” Bio-ORACLE1 (Tyberghein et al., 2012; Assis et al., 2017) with a resolution of 5arcmin (0.0833°). The latter were compiled from combinations of satellite and in situ observations, gathering data for a period of 14 years (2000–2014; Assis et al., 2017).
The layers downloaded for the present paper included data about annual-mean value of chlorophyll-a (mg/m3), current velocity (m–1), oxygen concentration (mol/m3), iron (μmol/m3), nitrate (mol/m3), silicate (mol/m3), phosphate (mol/m3), phytoplankton (μmol/m3), primary production (g/m3d–1). All values referred only to the maximum depth at the sea bottom except for primary production which included only the pelagic data. Besides, ice concentration data (fractions from 0 to 1), salinity, and temperature (C°) were also downloaded in order to assess the reliability of Bio-ORACLE data in comparison with the CTD data. Bio-ORACLE data were used to replace the two missing values of CTD data of temperature and salinity in station F6 and F9 from the Filchner Trough. Ice concentration data from all study areas since the year 1978 were obtained from the meereisportal data base of the Alfred Wegener Institute2 (Grosfeld et al., 2016). Ice concentration is given using the unit interval (fractions) from 0 to 1, where 0 indicates absence of ice and 1 indicates a completely ice-covered area.
Data Analysis
To determine the distribution patterns of the assemblage of peracarid crustaceans between stations and in relation to environmental variables, abundance data were analyzed by means of ordination analysis. Prior to analyses, a draftsman plot was used to check for multicollinearity between environmental variables and to assess the presence of heavily skewed ones. Heavily skewed variables were then transformed following Clarke and Gorley (2006). The following variables were removed: phytoplankton, nitrate, oxygen, silicate. In addition, depth, chlorophyll-a, current velocity, iron and primary productivity were log transformed. When a couple of variables presented mutual Pearson correlation coefficients averaging more than 0.90 and less than −0.90, only one for each couple was selected for further analyses (Supplementary Table 2).
Ordinate analysis was performed using the non-metric multidimensional scaling (nMDS) based on Bray–Curtis dissimilarity matrix and on square rooted transformed abundance data in order to visualize dissimilarities in assemblages′ structure among samples. The similarity profile permutation test (SIMPROF) was used to visually identify significant dissimilarities among samples by superimposing significant SIMPROF clusters on nMDS plots. Principal Component Analysis (PCA) based on normalized environmental data was used to graphically represent correlations between peracarid assemblages and environmental parameters.
The BIOENV procedure (BEST) was used to identify the subset of variables that best explained the dissimilarity patterns observed. BIOENV computed a Spearman rank correlation (Rho) between the Bray–Curtis similarity matrix of peracarid’s abundances and the similarity matrix of transformed and normalized environmental variables based on Euclidean distance. In order to examine the statistical significance of observed correlation, the global BEST match permutation test (999 permutations) was used.
The seriation with replication test of the RELATE routine was used to test whether the dissimilarity in assemblages’ structure observed in the nMDS followed a sequential pattern of change. This analysis applies a Spearman rank correlation (Rho) between dissimilarities among samples and a perfect seriated model matrix based on a linear sequence of values equally spaced along a line (Clarke and Gorley, 2006). A Spearman rank correlation coefficient Rho close to one indicates high seriation, while a coefficient Rho close to zero corresponds to the null hypothesis of no seriation. To reject the null hypothesis of a complete absence of seriation, a permutation test was applied to the matching coefficient (Rho; 999 permutations). The null hypothesis was rejected at a significance level of at least 1 in 10000 (p < 0.0001).
Ordination analysis including nMDS and PCA, BIOENV and RELATE analyses were performed using the multivariate software PRIMER v6 (Clarke and Gorley, 2006).
Ultimately, correlations between environmental variables and total peracarid abundances were analyzed by Pearson correlation analyses using the statistic software RStudio and the package “ggpubr” (Kassambara, 2017).
Statistical analyses by means of Pearson correlation were carried out on selected stations from the continental shelf (depth range 400–899 m) excluding those from the deep sea. The latter usually starts at about 200 m but in the SO where the continental shelf is usually deeper, it starts at a depth of 1000 m (Clarke, 2003). It was also shown that a shift between shelf and deep-sea isopod and sponge communities occurred only at about 1500 m in the Powell Basin (Brandt et al., 2007c). The depth range 400–899 m was chosen because it allowed us to have the larger dataset having the smallest difference in depth.
Results
Peracarid Abundance
A total of 64766 peracarids were sorted and identified to order level, five orders were identified (Amphipoda, Cumacea, Isopoda, Mysidacea, Tanaidacea; Table 2). Standardized abundance data showed that the sampled areas had different levels of maximum abundance per station. Noteworthy was station F5 in the Filchner Trough which had the highest abundance with 13740 peracarid ind./1000 m haul, while abundance from station F9 was the lowest with only 234 ind./1000 m haul (Table 2).
In total, amphipods were the most abundant taxon with 34868 ind./1000 m haul, representing 32% of the total abundance, while Mysidacea and Tanaidacea were the least abundant with 4909 and 4953 ind./1000 m haul respectively, each only representing 4% of the total abundance (Figure 2A).
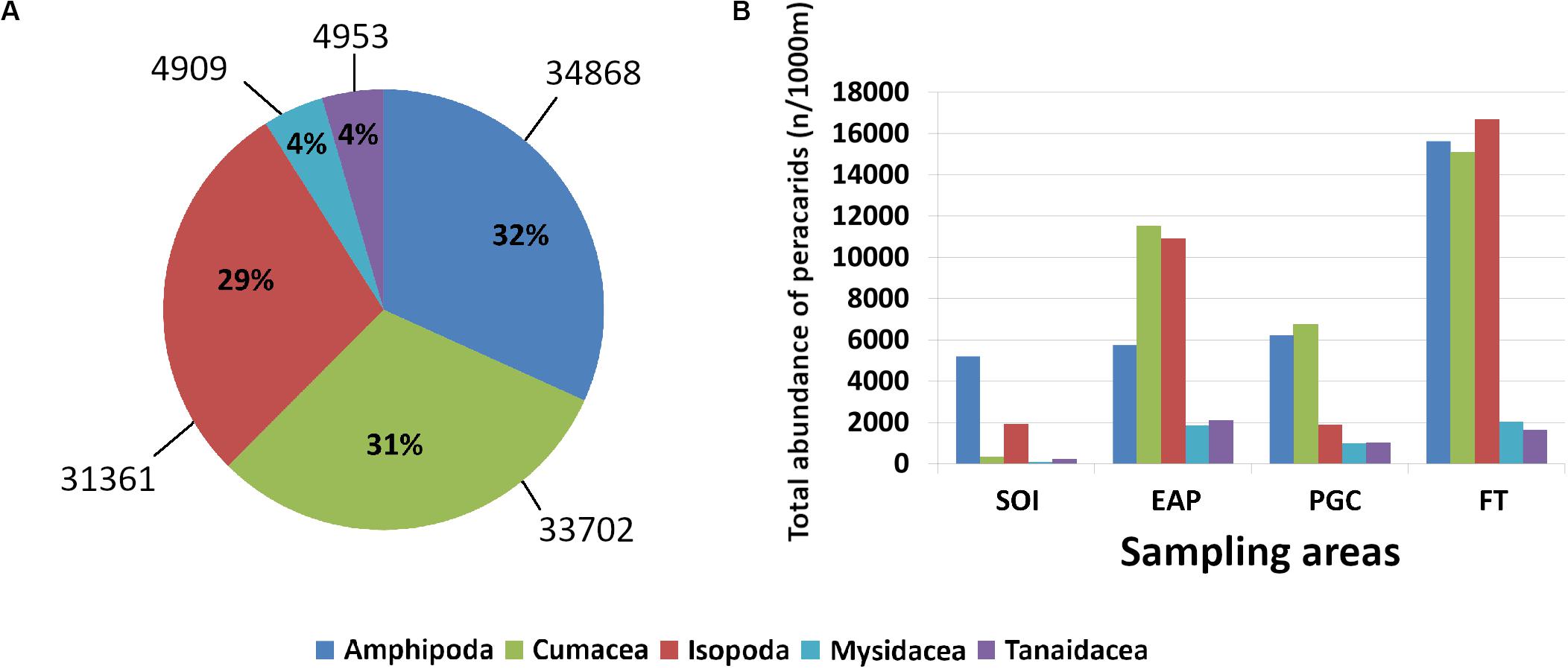
Figure 2. Total relative abundance of peracarids (A); total abundance of peracarids in each sampling area (B). SOI, South Orkney Islands; EAP, Eastern Antarctic Peninsula; PGC, Prince Gustav Channel; FT, Filchner Trough.
The number of individuals in each order of peracarids varied regardless of the depth in all sampling areas. In the Eastern Antarctic Peninsula, abundances from station E5 (445 m depth) were the highest with 20686 ind./1000 m haul, while abundances from station E4 (438 m) were only 196 ind./1000 m haul (Table 2 and Figure 3C). The other stations from the same area and with similar depth showed a much lower number of peracarids (Table 2 and Figures 3A,E,G).
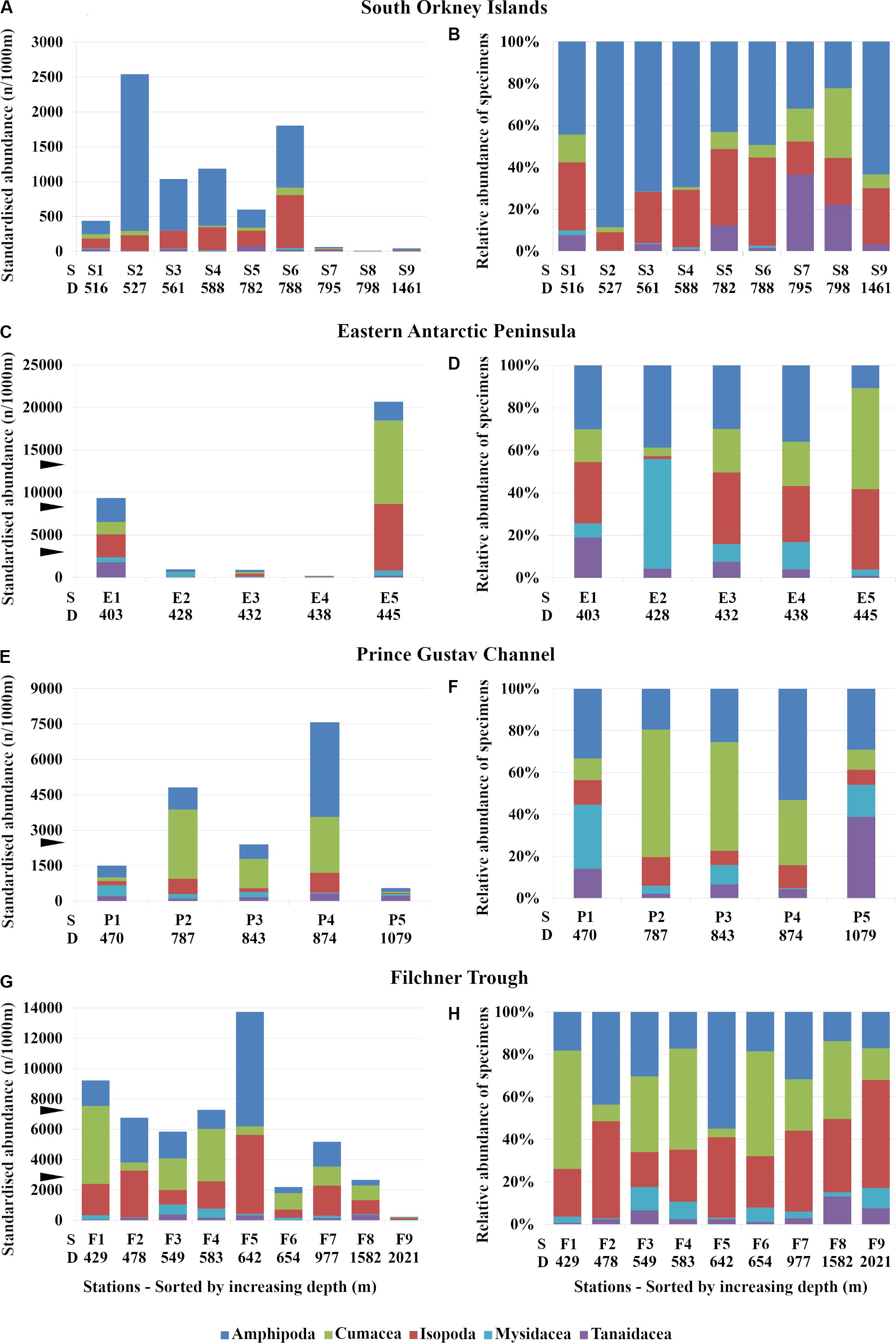
Figure 3. Standard (A,C,E,G) and relative abundance (B,D,F,H) of peracarid orders in the study areas. (A,B) South Orkney Islands, (C,D) Eastern Antarctic Peninsula, (E,F) Prince Gustav Channel, (G,H) Filchner Trough. ▶, maximum standardized abundance at comparative regions; S, station number; D, depth (m).
The total abundance of the five peracarid orders varied between different areas. Whilst in the Filchner Trough amphipods, isopods, and cumaceans were similar in abundance, in the Prince Gustav Channel isopods were less abundant (Figure 2B). In the Eastern Antarctic Peninsula and in the South Orkney Islands the least abundant of these three taxa were the amphipods and cumaceans, respectively (Figure 2B).
One of the most striking results was the very high abundance of cumaceans at station E5 off the Eastern Antarctic Peninsula, dominating with 9823 ind./1000 m haul (Figure 3C), while at station S3 off the South Orkney Islands only one single ind./1000 m haul was found (Table 2). A similar trend was observed also for the other groups; although, none of them showed such high abundance in one single station. Cumaceans were also dominant at five of nine stations in the Filchner Trough and at two stations from the Prince Gustav Channel (Figures 3E–H).
Relative abundances showed different patterns between the different areas and regardless of depth. In the South Orkney Islands amphipods were the most dominant group, representing up to more than 80% of the total in the different stations (Figure 3B). A consistent relationship between depth and the abundance of amphipods was not observed. In the Eastern Antarctic Peninsula amphipods showed a similar pattern between stations (Figure 3D). In the Prince Gustav Channel, Cumacea were the dominant order, representing up to 61% of the total abundance among the different stations (Figure 3F). In the Filchner Trough almost all orders showed similar abundance patterns at all stations (Figure 3H).
Peracarid Assemblages and Environmental Variables
In order to assess the abundance, distribution patterns and assemblage structure of peracarids between the different stations and in relation to environmental variables, ordinate statistical analyses were performed.
The nMDS analysis showed a dissimilarity in assemblages’ structure among sampling sites gradually increasing from low ice concentration (<0.5) to high ice concentration (>0.5). Stations S7, S8, S9 were very dissimilar compared to the rest of the cluster due to their extremely low abundances (59, 9 and 41 ind./1000 m haul, respectively; Figure 4 and Table 2). In contrast, depth did not explain the patterns of dissimilarity in assemblages’ structure observed. Stations from the same depth ranges were evenly distributed among the different clusters (Figure 4).
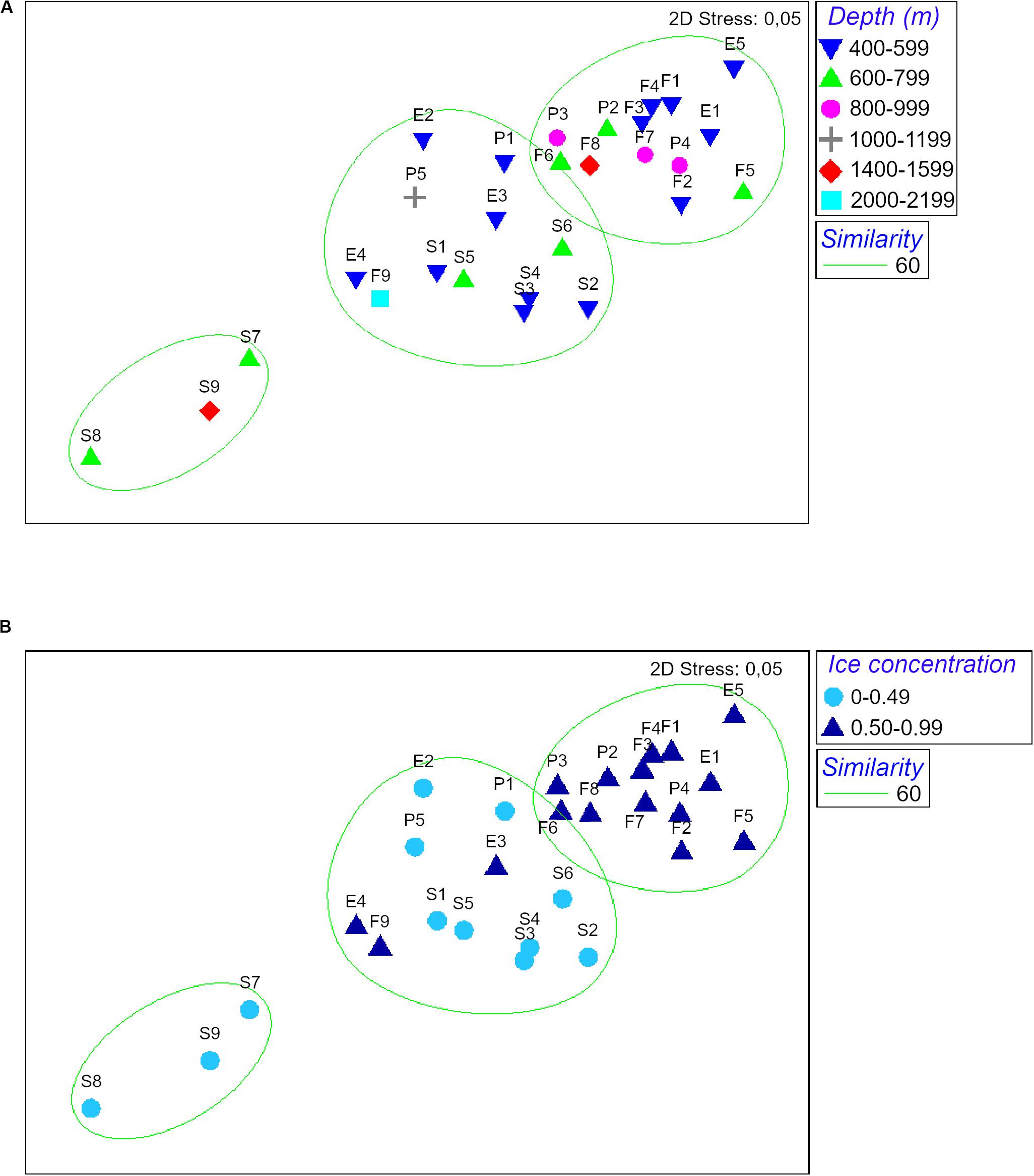
Figure 4. nMDS of ice concentration and depth of peracarid assemblages from all stations. Depth is expressed in meters, ice concentration in fractions from 0 to 1 (where 0 indicates no ice coverage and 1 indicates complete ice coverage).
Principal Component Analysis produced a total of five principal components, the first three of which explained 80.1% of the total variance (eigenvalue > 1). Analysis of the eigenvectors showed that the major contribution in the first PCA axis (PC1) was given by phosphate concentration and temperature, while the predominant variables in the second axis (PC2) were salinity and primary productivity. Ice coverage and depth were the most significant variables in the third axis (PC3; Table 3; Figure 5). BIOENV analysis showed that the ice coverage was the variable which was most highly correlated with the similarity matrix derived from peracarid abundances (p = 0.008; ρ = 0.334). The presence of a gradual change of dissimilarities in assemblage’s structure among samples with increasing/decreasing ice concentration was tested using the “seriation with replication” test implemented in the RELATE routine. The analysis showed a significant result (p = 0.0001; ρ = 0.355). The same test carried out on a depth gradient did not show any significant result (p = 0.17; ρ = 0.077).
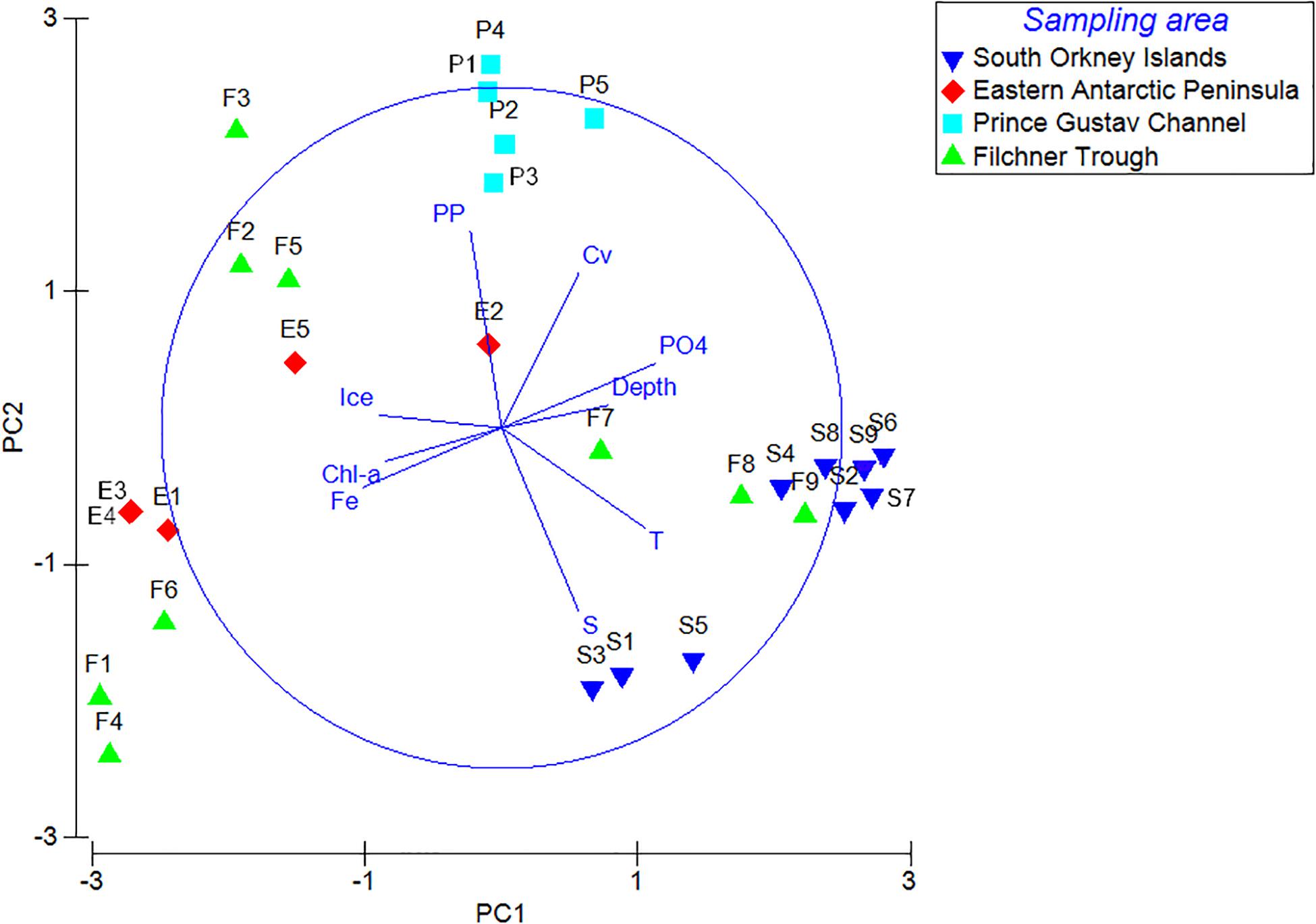
Figure 5. Principal component analysis (PCA) of peracarid assemblages and environmental variables from all stations. PP, primary productivity; Chl, chlorophyll-a; S, salinity; T, temperature; Fe, iron; Cv, current velocity; PO4, phosphate; Ice, ice concentration.
Total abundance of peracarids from all the stations investigated in the present study (400–2021 m) decreased with depth (R = −0.67, p = 0.033; Figure 6A and Table 4). When the correlation was tested including only stations from the continental shelf (400–899 m) the result was not significant (R = −0.82, p = 0.092; Table 4), showing that the correlation between abundances and other environmental variables was not affected by the depth intervals (100 m) chosen in the analysis.
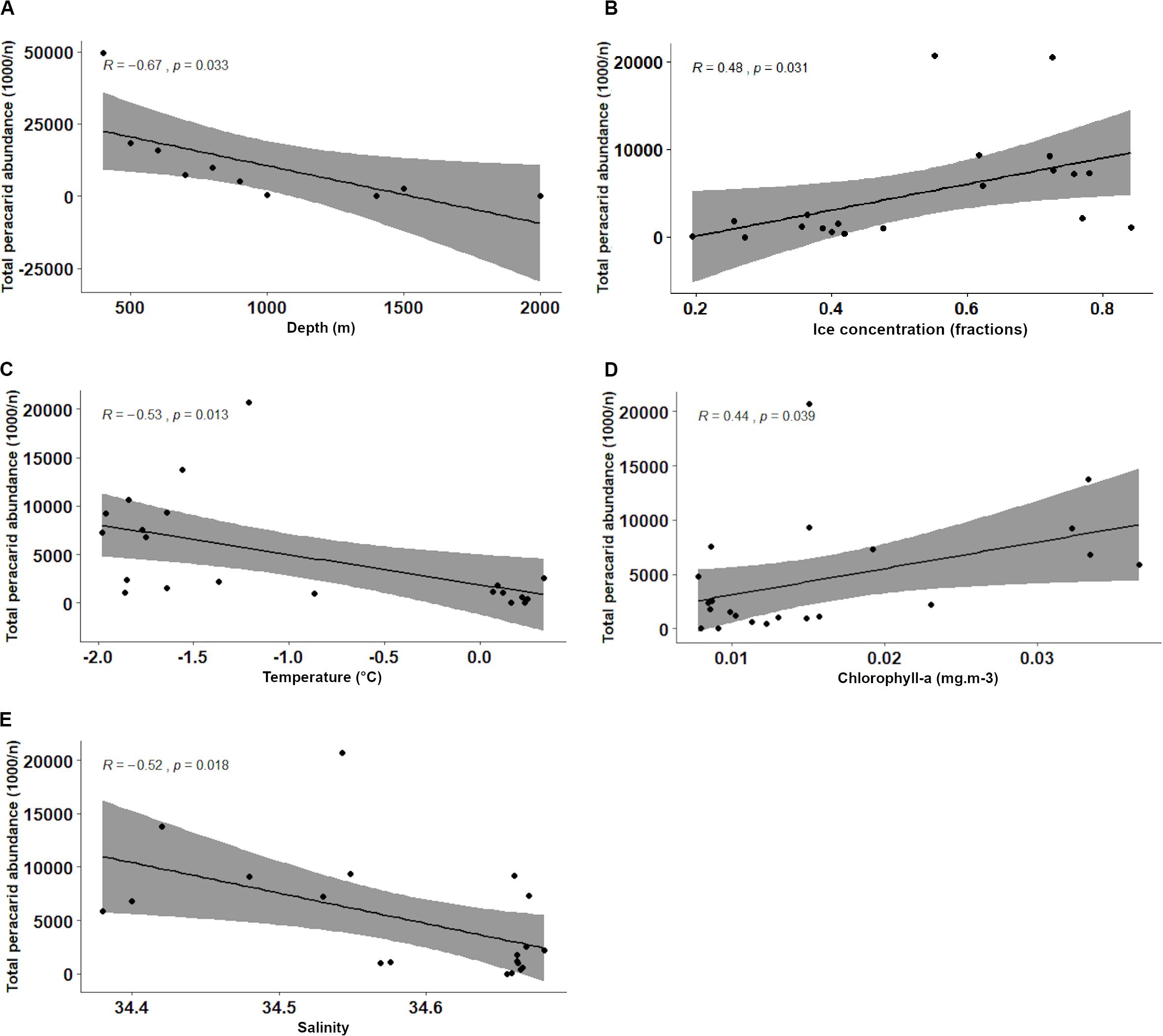
Figure 6. Pearson’s correlation analyses of peracarid abundances and environmental variables: depth (A), ice concentration (B), temperature (C), chlorophyll-a (D), salinity (E).
A negative correlation was found with increasing temperature and salinity (R = −0.53, p = 0.013 and R = −0.52, p = 0.018 respectively; Figures 6C,E and Table 4). Peracarids showed higher abundances at lower values of the two parameters. Conversely, peracarid abundances significantly increased with increasing ice and clorophyll concentration (Figures 6B,D and Table 4). No significant correlation with the other environmental parameters investigated in this study (current velocity, iron, phosphate, primary productivity; Table 4) was found.
When comparing Bio-ORACLE data with shipboard CTD measurements, both sources produced similar results.
Discussion
Abundance of Peracarid Crustaceans
Results from this study showed that peracarid abundances varied in different study areas and between stations within the same area. Such a trend is in line with a previous study from the SO continental shelf, in which Kaiser et al. (2008) reported that total abundance of peracarids between different stations (South Sandwich Islands) ranged from 11 to 4123 ind./1000 m haul (samples collected with an EBS, the deepest station was about 1000 m). In our study, overall, shelf stations from the Filchner Trough showed much higher abundances compared to those recorded in the South Orkney Islands. A similar result was observed when comparing the Filchner Trough with the South Sandwich Islands (Kaiser et al., 2008). Total abundances of peracarids from the latter (4361 ind./1000 m haul) more closely resembled those from the South Orkney Islands (Table 2). The higher abundances in the Filchner Trough could be explained by the high frequencies of icebergs that can lead to more open water, broken-up ice, enhanced primary productivity and also impact benthic communities promoting the spread of opportunistic species, which in turn can become very abundant (Gerdes et al., 2003; Årthun et al., 2013). Our finding is in line with a previous study in which Brandt et al. (2007c) indicated an increase in abundance and species richness from the Scotia Arc area toward the Southern Weddell Sea.
Amphipods showed the highest relative abundance compared to other peracarid orders, which corresponds to previous studies where amphipods were also the most abundant group sampled in the SO (Brökeland et al., 2007; Kaiser et al., 2008). Nevertheless, a striking result was the high abundance recorded for cumaceans from many stations and from different areas (Filchner Trough, Prince Gustav Channel, Eastern Antarctic Peninsula). This is unusual, as in previous studies, cumaceans were rarely dominant, except for two stations sampled in the Ross Sea (Rehm et al., 2007).
Besides, the high relative abundance of cumaceans recorded in our study (31%) was never reported before. In Brandt (2001; Eastern Weddell Sea), they represented about 21%, in Brökeland et al. (2007; different areas of the SO) 16%, in Rehm et al. (2007; Ross Sea) 7%, in Kaiser et al. (2008; South Sandwich Islands) they represented only the 2% of the entire peracarid assemblage. The high abundance of cumaceans recorded in the present study may be explained by their life style. Cumaceans are inbenthic (only males move into the water column during circadial migrations; Mühlenhardt-Siegel, 2014), they specially occur in very fine and silty sediments which characterized most of the sampling sites.
Influence of Environmental Variables
Temperature, Salinity, Depth, Sediment Type
Previous studies that investigated the influence of environmental parameters on peracarid assemblages′ abundance and composition in the SO mainly considered factors such as depth, salinity, temperature and sediment type. However, previous results did not always show the same patterns or correlations between peracarid assemblages and environmental parameters. Meyer-Löbbecke et al. (2014) suggested that salinity, temperature, chlorophyll-a, and depth might influence the number of isopod specimens and the isopod assemblages, while in Brandt et al. (2007a) depth was the main driver shaping the structure of isopod assemblages. These different results could be due to the fact that sampling was done in areas influenced by different water bodies and thus by different environmental conditions, besides the different number of stations and depth range. A full understanding of the dynamics governing the composition of benthic communities is difficult, since general distribution patterns can result from several different factors and from the spatial scales investigated (e.g., if local or regional scale; Kaiser et al., 2007).
In our study, the abundance of peracarids from all stations (depth range 400–2021 m) was negatively correlated with temperature, salinity, and depth. The negative correlation with the latter can be explained by a decrease in food availability and quality from shallower to deeper waters (Hessler, 1974; Gage and Tyler, 1991; Schnack-Schiel and Isla, 2005; Brökeland et al., 2007). The high number of peracarids recorded at stations from the deep sea showed that depth is not the only factor affecting food availability at the bottom (Brökeland et al., 2007). This possibly documents the importance of the Antarctic bottom-water production, which might enhance food availability in deeper waters (Brandt et al., 2007b). For example, in East Antarctica regions it was shown that downslope flow of Antarctic bottom-water rich in organic matter and generated in shelf polynyas can supply food to seafloor slope benthos (Jansen et al., 2018). It was shown to be also one of the key factors sustaining benthic communities from the continental shelf, down to about 900 m (Post et al., 2010).
Ice Coverage and Chlorophyll-a Concentration
In our study, ice concentration was the parameter which best explained the pattern of dissimilarity in assemblages’ structure of peracarids. Dissimilarities among samples increased with decreasing percentage of ice concentration. In spite of the lack of studies in this regard in the Southern Ocean, the influence of sea ice on peracarid assemblages was reported in a previous study performed in the Northern Hemisphere (Greenland; Brandt, 1995). Composition, abundance and diversity of benthic peracarids reflected the availability of food (phytoplankton and ice algae) which was linked to the presence of a polynya and ice-edge primary production. The polynya opened in spring and enhanced the primary productivity of the area throughout the summer months. The increased primary productivity in the water column affects the benthos by the increasing amounts of organic matter reaching the seafloor. For example, Brandt (1995) linked the presence of high isopod abundances to the availability of fresh phytoplankton material on the seafloor, derived from phytoplankton blooms initiated by melting sea ice. In our study, noteworthy was the significant positive correlation observed between sea ice concentration and peracarid abundance and between chlorophyll-a and peracarid abundance. In the SO a previous study investigated the correlation between deep-sea isopod assemblage and chlorophyll-a concentration, although a clear pattern was not observed (Meyer-Löbbecke et al., 2014). An explanation for this result could be the use of surface chlorophyll-a in the analysis. The organic matter produced at the surface could be rapidly consumed by the zooplankton communities and in part also be laterally transferred by currents. Therefore, only a small amount of organic matter might reach the seafloor and be available for the benthic communities. A consideration of the concentration of chlorophyll-a and organic matter at the bottom might thus yield a more reliable result.
Mean sea ice concentration and peracarid abundances of continental shelf stations from present and previous studies combined (same sampling protocol and data standardization; Arntz and Gutt, 1999; Fütterer et al., 2003; Fahrbach, 2006; Linse, 2006; Brökeland et al., 2007; Kaiser et al., 2008) also showed a significant correlation. In contrast, the same analysis carried out at deeper stations did not show any significant result (Table 4). This suggests that benthic communities may be more strongly influenced by the presence of sea ice only within a certain depth range (up to about 1000 m from results of the present study). The reason could be the different response between deep-sea and shelf communities to seasonal organic inputs derived by the release of ice biota in the water column. Benthic shelf communities can show a quick response to the input of organic matter in productive shallow waters (Covazzi Harriague et al., 2007; Zhang et al., 2015); whereas, in the deep sea, results can be contrasting. Several studies showed that the benthic macrofauna from abyssal depths can quickly react to phytodetritus pulses (Sorbe, 1999; Aberle and Witte, 2003; Witte et al., 2003), although in other studies no clear response was observed (Pfannkuche, 1993; Gooday, 2002).
Concentration of chlorophyll-a in the water column can be influenced by sea ice, the break-up and melt of the latter might trigger phytoplankton blooms and enhance the production of sea-ice algae in the surrounding environment (Gradinger, 1996; Jin et al., 2007; Gradinger, 2009). In a previous study, it was also shown that the contribution of sea-ice algae to the general primary production in the water column increased in areas with more extensive ice coverage, reaching up to 57% of the total primary productivity in the ice of the Central Arctic Ocean (Leu et al., 2011). Besides, sea ice melting processes may cause a stabilization of the surface mixed layer, which can lead to higher primary production (Dunbar et al., 1998; Vernet et al., 2019) and thus higher abundances and more diverse benthic communities. In marginal sea ice zones or polynyas, higher abundances of benthic fauna were reported due to the enhanced primary productivity (Brandt, 1995, 1996; Fonseca and Soltwedel, 2007). This also proved true for peracarid assemblages from the continental shelf in the North Atlantic, where higher abundances were recorded in response to an increase in sedimentation of phytoplankton and ice algae to the sea floor (Brandt, 1995, 1996).
Sea ice retreat influences primary productivity also indirectly by the increase of open water areas whose ice-free surface is affected by wind action. During ice-free periods winds can change the depth of the mixed layer in the water column leading to a mixed-layer deepening, which in turn decreases the mean value of light available per water volume for phytoplankton photosynthesis, thus reducing the chlorophyll-a concentration (Ikeda, 1989; Dunbar et al., 1998; Montes-Hugo et al., 2009; Rainville et al., 2011). These results highlight the importance of seasonal ice coverage variations. Wlodarska-Kowalczuk et al. (2004) showed, for example, that areas characterized by seasonal ice coverage had higher abundances of the benthic macrofauna compared to areas characterized by perennial ice coverage. Moreover, the lack of sea ice break-up events strongly alters the benthic food-web structure, causing a shift on the diet of the benthic organisms (Michel et al., 2019).
Sea ice is characterized not only by seasonality but also by an interannual variability, which can drive changes in the annual rate of primary production in different regions of the SO (Arrigo et al., 2008). The latter authors showed an interannual variability in annual primary production in the Weddell Sea for the period range 1997–2006. They addressed higher annual primary production rates to anomalies in sea ice extent. Sea ice coverage around the Antarctic Peninsula has undergone a gradual increase during the last three decades and a strong decline starting from 2014 (Parkinson, 2019). In light of this, it is possible that the peracarid distribution pattern, abundance and assemblage structure observed in our study could be also explained by different sampling years (from 2012 to 2019), which may have experienced different interannual productivity rates due to changes in ice cover extent. Similarly, Meyer-Löbbecke et al. (2014) indicated the difference in sampling years as a possible factor explaining the different patterns observed in their study on isopod abundance.
Conclusion
Our study suggests that varying regimes of ice coverage and chlorophyll-a concentration strongly influence the abundance and assemblages’ structure of benthic peracarids from the continental shelf of the SO. On one hand, the sea-ice break-up and retreat in summer is a key element for peracarid abundance and distribution since the ice-melting process releases a large amount of ice algae in the water column. These, in turn, increase the local primary productivity and thus enhance the amount of organic matter available for the benthic communities. On the other hand, ice shelves with their continuous ice coverage allow the existence of a benthic fauna adapted to live in an environment characterized by very low sedimentation rate and which resembles that of the deep sea.
The retreat of sea ice and the disintegration of ice shelves caused by the increase of temperatures (Rott et al., 1996; Rack and Rott, 2003; Cook et al., 2016) can alter these equilibriums and impact the composition and abundance of the benthic fauna. Peracarid assemblages are subject to change drastically in the future due to such variations. Given the important role that peracarid crustaceans play among benthic communities, the complete retreat of sea ice and the consequent strong decrease of their abundance would negatively impact the benthic ecosystem. For a better understanding of interactions between sea-ice coverage and benthic communities, it is fundamental to study the ecological impact of such events on faunal compositions. Only if we understand these correlations might we be able to predict faunal alterations induced by climate change.
Data Availability Statement
The raw data supporting the conclusions of this article will be made available by the authors, without undue reservation.
Author Contributions
AB, KL, and HG developed and designed the study. DD, AB, KL, and HG collected the samples. DD, AB, and KL sorted the samples and identified the specimens to order level. CH provided the sea ice analyses. HS provided the environmental data and helped with the statistical analyses. DD prepared the figures and tables, performed the statistical analyses, and drafted the original manuscript which was revised and improved by AB, KL, HG, CH, and HS. All the authors contributed to the article and approved the submitted version.
Funding
DD and AB and their participation in the RV Polarstern expedition PS118 were funded by the Deutsche Forschungsgemeinschaft (DFG; Br1121/51-1). KL and HG are part of the British Antarctic Survey Polar Science for Planet Earth Programme. It was funded by the Natural Environment Research Council (NERC) (NC-Science), and included the funding for the RSS James Clark Ross expeditions JR275 and JR15005. The RSS James Clark Ross expedition JR17003a was funded by the NERC urgency grant NE/R012296/1. Multiyear ice data from 15/10/1978 to 15/03/2019 were obtained from https://www.meereisportal.de (grant: REKLIM-2013-04).
Conflict of Interest
The authors declare that the research was conducted in the absence of any commercial or financial relationships that could be construed as a potential conflict of interest.
Acknowledgments
We would like to thank the scientists and crew that were on board of the RRS James Clark Ross and the RV Polarstern expeditions, and the people who helped to collect the samples. We thank Oliver S. Ashford and Camille Moreau, who sorted the EBS samples to order level from the expeditions JR15005 and JR275, and Autumn Purser and the AWI OFOBS Team, who provided high quality images of the sea floor from the expedition PS118. We are grateful to Dilay Ergül, Katharina M. Krüger, Alexander H. Knorrn, Emily B. Riemer, Marissa Adler, and students of the Goethe University in Frankfurt who contributed to sort further samples to order and in peracarids to morphospecies level.
Supplementary Material
The Supplementary Material for this article can be found online at: https://www.frontiersin.org/articles/10.3389/fmars.2020.554663/full#supplementary-material
Footnotes
References
Aberle, N., and Witte, U. (2003). Deep-sea macrofauna exposed to a simulated sedimentation event in the abyssal NE Atlantic: in situ pulse-chase experiments using 13C-labelled phytodetritus. Mar. Ecol. Prog. Ser. 251, 37–47. doi: 10.3354/meps251037
Alldredge, A. L., and King, J. M. (1985). The distance demersal zooplankton migrate above the benthos: implications for predation. Mar. Biol. 84, 253–260. doi: 10.1007/BF00392494
Arntz, W. E., and Gutt, J. (1999). “The expedition ANTARKTIS XV/3 (EASIZ II) of RV Polarstern in 1998,” in Berichte zur Polarforschung (Reports on Polar Research), Vol. 301, (Bremerhaven: Alfred Wegener Institute for Polar and Marine Research), 135–149. doi: 10.2312/BzP_0301_1999
Aronson, R. B., Moody, R. M., Ivany, L. C., Blake, D. B., Werner, J. E., and Glass, A. (2009). Climate change and trophic response of the Antarctic Bottom Fauna. PLoS One 4:e4385. doi: 10.1371/journal.pone.0004385
Arrigo, K. R., van Dijken, G. L., and Bushinsky, S. (2008). Primary production in the Southern Ocean, 1997–2006. J. Geophys. Res. 113:C08004. doi: 10.1029/2007JC004551
Årthun, M., Nicholls, K. W., and Boehme, L. (2013). Wintertime water mass modification near an Antarctic Ice Front. J. Phys. Oceanogr. 43, 359–365. doi: 10.1175/JPO-D-12-0186.1
Assis, J., Tyberghein, L., Bosch, S., Verbruggen, H., Serrão, E. A., and De Clerck, O. (2017). Bio-ORACLE v2.0: extending marine data layers for bioclimatic modelling. Global Ecol. Biogeogr. 27, 277–284. doi: 10.1111/geb.12693
Barker, P. F., Filippelli, G. M., Florindo, F., Martin, E. E., and Scher, H. D. (2007). Onset and role of the Antarctic circumpolar current. Deep Sea Res. Part II Top. Stud. Oceanogr. 54, 2388–2398. doi: 10.1016/j.dsr2.2007.07.028
Barnes, D. K. A., and Souster, T. (2011). Reduced survival of Antarctic benthos linked to climate-induced iceberg scouring. Nat. Clim. Chang. 1, 365–368. doi: 10.1038/nclimate1232
Bertolin, M. L., and Schloss, I. R. (2009). Phytoplankton production after the collapse of the Larsen A Ice Shelf, Antarctica. Polar Biol. 32, 1435–1446. doi: 10.1007/s00300-009-0638-x
Boetius, A., Albrecht, S., Bakker, K., Bienhold, C., Felden, J., Fernandez-Mendez, M., et al. (2013). Export of algal biomass from the melting Arctic sea ice. Science 339, 1430–1432. doi: 10.1126/science.1231346
Brandt, A. (1995). Peracarid fauna (Crustacea, Malacostraca) of the Northeast Water Polynya off Greenland: documenting close benthic-pelagic coupling in the Westwind Trough. Mar. Ecol. Prog. Ser. 121, 39–51. doi: 10.3354/meps121039
Brandt, A. (1996). Peracarid Crustaceans (Malacostraca) from a “time-series station” in the Westwind Trough of the New-Polynya (Greenland): a benthic response to productivity? Crustaceana 69, 985–1004. doi: 10.1163/156854096X00420
Brandt, A. (1999). On the origin and evolution of Antarctic Peracarida (Crustacea, Malacostraca). Sci. Mar. 63, 261–274. doi: 10.3989/scimar.1999.63s1261
Brandt, A. (2000). Hypotheses on Southern Ocean peracarid evolution and radiation (Crustacea, Malacostraca). Antarct. Sci. 12, 269–275. doi: 10.1017/S095410200000033X
Brandt, A. (2001). Great differences in peracarid crustacean density between the Arctic and Antarctic deep sea. Polar Biol. 24, 785–789. doi: 10.1007/s003000100290
Brandt, A., Brix, S., Brökeland, W., Choudhury, M., Kaiser, S., and Malyutina, M. (2007a). Deep-sea isopod biodiversity, abundance, and endemism in the Atlantic sector of the Southern Ocean—Results from the ANDEEP I–III expeditions. Deep Sea Res. Part II Top. Stud. Oceanogr. 54, 1760–1775. doi: 10.1016/j.dsr2.2007.07.015
Brandt, A., De Broyer, C., De Mesel, I., Ellingsen, K. E., Gooday, A. J., Hilbig, B., et al. (2007b). The biodiversity of the deep Southern Ocean benthos. Phil. Trans. R. Soc. B 362, 39–66. doi: 10.1098/rstb.2006.1952
Brandt, A., Gooday, A. J., Brandão, S. N., Brix, S., Brökeland, W., Cedhagen, T., et al. (2007c). First insights into the biodiversity and biogeography of the Southern Ocean deep sea. Nature 447, 307–311. doi: 10.1038/nature05827
Brasier, M. J., Grant, S. M., Trathan, P. N., Allcock, L., Ashford, O., Blagbrough, H., et al. (2018). Benthic biodiversity in the South Orkney islands southern shelf marine protected area. Biodiversity 2018:148821. doi: 10.1080/14888386.2018.1468821
Brenke, N. (2005). An Epibenthic Sledge for operations on marine soft bottom and bedrock. Mar. Technol. Soc. J. 39, 10–21. doi: 10.4031/002533205787444015
Brey, T., Dahm, C., Gorny, M., Klages, M., Stiller, M., and Arntz, W. E. (1996). Do Antarctic benthic invertebrates show an extended level of eurybathy? Antarct. Sci. 8, 3–6. doi: 10.1017/S0954102096000028
Brökeland, W., Choudhury, M., and Brandt, A. (2007). Composition, abundance and distribution of Peracarida from the Southern Ocean deep sea. Deep Sea Res. Part II Top. Stud. Oceanogr. 54, 1752–1759. doi: 10.1016/j.dsr2.2007.07.014
Brusca, R. C., Moore, W., and Schuster, M. (2016). Invertebrates. Sunderland, MA: Sinauer Associated, Inc.
Clark, G. F., Stark, J. S., Palmer, A. S., Riddle, M. J., and Johnston, E. L. (2017). The roles of sea-ice, light and sedimentation in structuring shallow Antarctic benthic communities. PLoS One 12:e0173939. doi: 10.1371/journal.pone.0173939
Clarke, A. (2003). “The polar deep seas,” in Ecosystems of the Deep Oceans, ed. P. A. Tyler (Amsterdam: Elsevier), 239–260.
Clarke, A., and Crame, A. (1992). The Southern Ocean benthic fauna and climate change: a historical perspective. Phil. Trans. R. Soc. Lond. B 338, 299–309. doi: 10.1098/rstb.1992.0150
Collares, L. L., Mata, M. M., Kerr, R., Arigony-Neto, J., and Barbat, M. M. (2018). Iceberg drift and ocean circulation in the northwestern Weddell Sea, Antarctica. Deep Sea Res. Part II Top. Stud. Oceanogr. 149, 10–24. doi: 10.1016/j.dsr2.2018.02.014
Cook, A. J., Holland, P. R., Meredith, M. P., Murray, T., Luckman, A., and Vaughan, D. G. (2016). Ocean forcing of glacier retreat in the western Antarctic Peninsula. Science 353, 283–286. doi: 10.1126/science.aae0017
Covazzi Harriague, A., Albertelli, G., Bonomi, A., Fabiano, M., and Zunini-Sertorio, T. (2007). Pelagic–benthic coupling in a subtidal system of the North-Western Mediterranean. Chem. Ecol. 23, 263–277. doi: 10.1080/02757540701379527
Darelius, E., and Sallée, J. B. (2018). Seasonal outflow of Ice Shelf Water across the front of the Filchner Ice Shelf, Weddell Sea, Antarctica. Geophys. Res. Lett. 45, 3577–3585. doi: 10.1002/2017GL076320
Dauby, P., Nyssen, F., and Broyer, C. D. (2003). “Amphipods as food sources for higher trophic levels in the Southern Ocean: a synthesis,” in Antarctic Biology in a Global Context, eds A. H. L. Huiskes, W. C. C. Gieskes, J. Rozema, R. M. L. Schorno, S. M. van der Vies, and W. J. Wolff (Leiden: Backhuys Publishers), 129–134. doi: 10.13140/RG.2.1.3879.0163
Dayton, P. K., Mordida, B. J., and Bacon, F. (1994). Polar marine communities. Am. Zool. 34, 90–99. doi: 10.1093/icb/34.1.90
De Broyer, C., and Jazdzewska, A. (2014). “Biogeographic patterns of Southern Ocean benthic amphipods,” in Biogeographic Atlas of the Southern Ocean, eds C. De Broyer, P. Koubbi, H. J. Griffiths, B. Raymond, C. d’Udekem d’Acoz, A. Van de Putte, et al. (Cambridge: The Scientific Committee on Antarctic Research), 155–165. doi: 10.13140/RG.2.1.1716.3289
De Broyer, C., and Jazdzewski, K. (1996). Biodiversity of the Southern Ocean: towards a new synthesis for the Amphipoda (Crustacea). Boll. Mus. Civ. Stor. Nat. Verona 20, 547–568.
De Broyer, C., and Koubbi, C. P. (2014). Biogeographic Atlas of the Southern Ocean. Cambridge: Scientific Committee on Antarctic Research.
Domack, E., Duran, D., Leventer, A., Ishman, S., Doane, S., McCallum, S., et al. (2005). Stability of the Larsen B ice shelf on the Antarctic Peninsula during the Holocene epoch. Nature 436, 681–685. doi: 10.1038/nature03908
Dorschel, B. (2019). The expedition PS118 of the research vessel Polarstern to the Weddell Sea in 2019. Berichte zur Polar Meeresforschung 735:149.
Duffy, G. A., Horton, T., and Billett, D. S. M. (2012). Deep-sea scavenging amphipod assemblages from the submarine canyons of the Western Iberian Peninsula. Biogeosciences 9, 4861–4869. doi: 10.5194/bg-9-4861-2012
Duffy, J. E., and Hay, M. E. (2000). Strong impacts of grazing amphipods on the organization of a benthic community. Ecol. Monogr. 70, 237–263.
Dunbar, R. B., Leventer, A. R., and Mucciarone, D. A. (1998). Water column sediment fluxes in the Ross Sea, Antarctica: atmospheric and sea ice forcing. J. Geophys. Res. 103, 30741–30759. doi: 10.1029/1998JC900001
Dunn, R. J. K., Welsh, D. T., Jordan, M. A., Teasdale, P. R., and Lemckert, C. J. (2009). Influence of natural amphipod (Victoriopisa australiensis) (Chilton, 1923) population densities on benthic metabolism, nutrient fluxes, denitrification and DNRA in sub-tropical estuarine sediment. Hydrobiologia 628, 95–109. doi: 10.1007/s10750-009-9748-2
Fahrbach, E. (2006). The expedition ANTARKTIS-XXII/3 of the research vessel Polarstern in 2005. Berichte zur Polar Meeresforschung 533, 127–134. doi: 10.2312/BzPM_0533_2006
Fahrbach, E., Rohardt, G., Scheele, N., Schröder, M., Strass, V., and Wisotzki, A. (1995). Formation and discharge of deep and bottom water in the northwestern Weddell Sea. J. Mar. Res. 53, 515–538. doi: 10.1357/0022240953213089
Fonseca, G., and Soltwedel, T. (2007). Deep-sea meiobenthic communities underneath the marginal ice zone off Eastern Greenland. Polar Biol. 30, 607–618. doi: 10.1007/s00300-006-0220-8
Fütterer, D., Brandt, A., and Poore, G. (2003). The expeditions ANTARKTIS-XIX/3-4 of the research vessel POLARSTERN in 2002: (ANDEEP I and II: Antarctic benthic deep-sea biodiversity - colonization history and recent community patterns). Berichte zur Polar Meeresforschung 470, 66–71. doi: 10.2312/BzPM_0470_2003
Gage, J. D., and Tyler, P. A. (1991). Deep-Sea Biology: A Natural History of Organisms at the Deep-Sea Floor. Cambridge: Cambridge University Press.
Gerdes, D., Hilbig, B., and Montiel, A. (2003). Impact of iceberg scouring on macrobenthic communities in the high-Antarctic Weddell Sea. Polar Biol. 26, 295–301. doi: 10.1007/s00300-003-0484-1
Gooday, A. J. (2002). Biological responses to seasonally varying fluxes of organic matter to the ocean floor: a review. J. Oceanogr. 58, 305–332. doi: 10.1023/A:1015865826379
Gordon, A. L., Visbeck, M., and Huber, B. (2001). Export of Weddell Sea deep and bottom water. J. Geophys. Res. 106, 9005–9017. doi: 10.1029/2000JC000281
Gradinger, R. (1996). Occurrence of an algal bloom under Arctic pack ice. Mar. Ecol. Prog. Ser. 131, 301–305. doi: 10.3354/meps131301
Gradinger, R. (2009). Sea-ice algae: major contributors to primary production and algal biomass in the Chukchi and Beaufort Seas during May/June 2002. Deep Sea Res. Part II Top. Stud. Oceanogr. 56, 1201–1212. doi: 10.1016/j.dsr2.2008.10.016
Griffiths, H. J. (2012). Cruise Report JR275. Available online at: https://www.bodc.ac.uk (accessed February 20, 2020).
Griffiths, H. J. (2017). Cruise Report JR15005. Available online at: https://www.bodc.ac.uk (accessed February 20, 2020).
Grosfeld, K., Treffeisen, R., Asseng, J., Bartsch, A., Bräuer, B., Fritzsch, B., et al. (2016). Online sea-ice knowledge and data platform <www.meereisportal.de>. Polarforschung 85, 143–155. doi: 10.2312/polfor.2016.011
Gutt, J., Barratt, I., Domack, E., d’Udekem d’Acoz, C., Dimmler, W., Grémare, A., et al. (2011). Biodiversity change after climate-induced ice-shelf collapse in the Antarctic. Deep Sea Res. Part II Top. Stud. Oceanogr. 58, 74–83. doi: 10.1016/j.dsr2.2010.05.024
Gutt, J., and Starmans, A. (2001). Quantification of iceberg impact and benthic recolonisation patterns in the Weddell Sea (Antarctica). Polar Biol. 24, 615–619. doi: 10.1007/s003000100263
Gutt, J., Starmans, A., and Dieckmann, G. (1996). Impact of iceberg scouring on polar benthic habitats. Mar. Ecol. Prog. Ser. 137, 311–316. doi: 10.3354/meps137311
Hansen, J., Ruedy, R., Sato, M., and Lo, K. (2010). Global surface temperature change. Rev. Geoph. 48, 1–29. doi: 10.1029/2010RG000345
Haumann, F. A., Gruber, N., Münnich, M., Frenger, I., and Kern, S. (2016). Sea-ice transport driving Southern Ocean salinity and its recent trends. Nature 537, 89–92. doi: 10.1038/nature19101
Hessler, R. R. (1974). “The structure of deep benthic communities from central oceanic waters,” in The Biology of the Oceanic Pacific, ed. C. B. Miller (Corvallis, OR: Oregon State University Press), 79–93.
Hodgson, D. A., McMinn, A., Kirkup, H., Cremer, H., Gore, D., Melles, M., et al. (2003). Colonization, succession, and extinction of marine floras during a glacial cycle: a case study from the Windmill Islands (east Antarctica) using biomarkers. Paleoceanography 18, 12. doi: 10.1029/2002PA000775
Hogg, A. E., and Gudmundsson, G. H. (2017). Impacts of the Larsen-C Ice Shelf calving event. Nat. Clim. Chang. 7, 540–542. doi: 10.1038/nclimate3359
Ikeda, M. (1989). A coupled ice-ocean mixed layer model of the marginal ice zone responding to wind forcing. J. Geophys. Res. 94, 9699. doi: 10.1029/JC094iC07p09699
Ingels, J., Vanreusel, A., Brandt, A., Catarino, A. I., David, B., De Ridder, C., et al. (2012). Possible effects of global environmental changes on Antarctic benthos: a synthesis across five major taxa. Ecol. Evol. 2, 453–485. doi: 10.1002/ece3.96
Jansen, J., Hill, N. A., Dunstan, P. K., McKinlay, J., Sumner, M. D., Post, A. L., et al. (2018). Abundance and richness of key Antarctic seafloor fauna correlates with modelled food availability. Nat. Ecol. Evol. 2, 71–80. doi: 10.1038/s41559-017-0392-3
Jeong, S. J., Yu, O. H., and Suh, H.-L. (2009). Reproductive patterns and secondary production of Gammaropsis japonicus (Crustacea, Amphipoda) on the seagrass Zostera marina of Korea. Hydrobiologia 623, 63–76. doi: 10.1007/s10750-008-9648-x
Jin, M., Deal, C., Wang, J., Alexander, V., Gradinger, R., Saitoh, S., et al. (2007). Ice-associated phytoplankton blooms in the southeastern Bering Sea. Geophys. Res. Lett. 34, L06612. doi: 10.1029/2006GL028849
Kaiser, S. (2014). “Antarctic and Sub-Antarctic isopod crustaceans (Peracarida, Malacostraca),” in Biogeographic Atlas of the Southern Ocean, eds C. De Broyer, P. Koubbi, H. J. Griffiths, B. Raymond, C. d’Udekem d’Acoz, A. Van de Putte, et al. (Cambridge: The Scientific Committee on Antarctic Research), 166–172.
Kaiser, S., Barnes, D. K. A., and Brandt, A. (2007). Slope and deep-sea abundance across scales: Southern Ocean isopods show how complex the deep sea can be. Deep Sea Res. Part II Top. Stud. Oceanogr. 54, 1776–1789. doi: 10.1016/j.dsr2.2007.07.006
Kaiser, S., Barnes, D. K. A., Linse, K., and Brandt, A. (2008). Epibenthic macrofauna associated with the shelf and slope of a young and isolated Southern Ocean island. Antarct. Sci. 20, 281–290. doi: 10.1017/S0954102008001107
Karlson, K., Hulth, S., and Rosenberg, R. (2007). Density of Monoporeia affinis and biogeochemistry in Baltic Sea sediments. J. Exp. Mar. Biol. Ecol. 344, 123–135. doi: 10.1016/j.jembe.2006.11.016
Kassambara, A. (2017). ggpubr: “ggplot2” Based Publication Ready Plots. Available online at: https://CRAN.R-project.org/package=ggpubr (accessed March 16, 2020).
Kiljunen, M., Peltonen, H., Lehtiniemi, M., Uusitalo, L., Sinisalo, T., Norkko, J., et al. (2020). Benthic-pelagic coupling and trophic relationships in northern Baltic Sea food webs. Limnol. Oceanogr. 9999, 1–17. doi: 10.1002/lno.11413
Lawver, L. A., Gahagan, L. M., and Dalziel, I. W. D. I. W. D. (2011). “A different look at gateways: drake passage and Australia/Antarctica,” in Special Publications, eds J. B. Anderson and J. S. Wellner (Washington, DC: American Geophysical Union), 5–33. doi: 10.1029/2010SP001017
Legezyñska, J., De Broyer, C., and Wesławski, J. M. (2020). “Invasion of the poles,” in The Natural History of the Crustacea: Evolution and Biogeography of the Crustacea, eds M. Thiel and G. Poore (New York, NY: Oxford University Press), 216–246.
Lehtonen, K. K., and Andersin, A.-B. (1998). Population dynamics, response to sedimentation and role in benthic metabolism of the amphipod Monoporeia affinis in an open-sea area of the northern Baltic Sea. Mar. Ecol. Prog. Ser. 168, 71–85.
Leu, E., Søreide, J. E., Hessen, D. O., Falk-Petersen, S., and Berge, J. (2011). Consequences of changing sea-ice cover for primary and secondary producers in the European Arctic shelf seas: timing, quantity, and quality. Progr. Oceanogr. 90, 18–32. doi: 10.1016/j.pocean.2011.02.004
Linse, K. (2006). Cruise report JR144, JR145, JR146, JR147 and JR149. Available online at: https://www.bodc.ac.uk (accessed February 20, 2020).
Linse, K. (2018). Cruise report JR17003a. Available online at: https://www.bodc.ac.uk (accessed February 20, 2020).
Makinson, K., Holland, P. R., Jenkins, A., Nicholls, K. W., and Holland, D. M. (2011). Influence of tides on melting and freezing beneath Filchner-Ronne Ice Shelf, Antarctica. Geophys. Res. Lett. 38:L06601. doi: 10.1029/2010GL046462
Massom, R. A., Scambos, T. A., Bennetts, L. G., Reid, P., Squire, V. A., and Stammerjohn, S. E. (2018). Antarctic ice shelf disintegration triggered by sea ice loss and ocean swell. Nature 558, 383–389. doi: 10.1038/s41586-018-0212-1
Meredith, M. P., Nicholls, K. W., Renfrew, I. A., Boehme, L., Biuw, M., and Fedak, M. (2011). Seasonal evolution of the upper-ocean adjacent to the South Orkney Islands, Southern Ocean: results from a “lazy biological mooring.”. Deep Sea Res. Part II Top. Stud. Oceanogr. 58, 1569–1579. doi: 10.1016/j.dsr2.2009.07.008
Meyer-Löbbecke, A., Brandt, A., and Brix, S. (2014). Diversity and abundance of deep-sea Isopoda along the Southern Polar Front: results from the SYSTCO I and II expeditions. Deep Sea Res. Part II Top. Stud. Oceanogr. 108, 76–84. doi: 10.1016/j.dsr2.2014.06.006
Michel, L. N., Danis, B., Dubois, P., Eleaume, M., Fournier, J., Gallut, C., et al. (2019). Increased sea ice cover alters food web structure in East Antarctica. Sci. Rep. 9:8062. doi: 10.1038/s41598-019-44605-5
Montes-Hugo, M., Doney, S. C., Ducklow, H. W., Fraser, W., Martinson, D., Stammerjohn, S. E., et al. (2009). Recent changes in Phytoplankton communities associated with rapid regional climate change along the western Antarctic Peninsula. Science 323, 1470–1473. doi: 10.1126/science.1164533
Mouat, B., Collins, M. A., and Pompert, J. (2001). Patterns in the diet of Illex argentinus (Cephalopoda: Ommastrephidae) from the Falkland Islands jigging fishery. Fish. Res. 52, 41–49. doi: 10.1016/S0165-7836(01)00229-6
Mühlenhardt-Siegel, U. (2014). “Southern ocean Cumacea,” in Biogeographic Atlas of the Southern Ocean, eds C. De Broyer, P. Koubbi, H. J. Griffiths, B. Raymond, C. d’Udekem d’Acoz, A. Van de Putte, et al. (Cambridge: The Scientific Committee on Antarctic Research), 181–184.
Murphy, E. J., Clarke, A., Symon, C., and Priddle, J. (1995). Temporal variation in Antarctic sea-ice: analysis of a long term fast-ice record from the South Orkney Islands. Deep Sea Res. Part I Oceanogr. Res. Pap. 42, 1045–1062. doi: 10.1016/0967-0637(95)00057-D
Orsi, A. H., Nowlin, W. D., and Whitworth, T. (1993). On the circulation and stratification of the Weddell Gyre. Deep Sea Res. Part I Oceanogr. Res. Pap. 40, 169–203. doi: 10.1016/0967-0637(93)90060-G
Pacheco, A. S., Goméz, G. E., and Riascos, J. M. (2013). First records of emerging benthic invertebrates at a sublittoral soft-bottom habitat in northern Chile. Rev. Biol. Mar. Oceanogr. 48, 387–392. doi: 10.4067/S0718-19572013000200018
Padovani, L. N., Viñas, M. D., Sánchez, F., and Mianzan, H. (2012). Amphipod-supported food web: Themisto gaudichaudii, a key food resource for fishes in the southern Patagonian Shelf. J. Sea Res. 67, 85–90. doi: 10.1016/j.seares.2011.10.007
Parkinson, C. L. (2019). A 40-y record reveals gradual Antarctic sea ice increases followed by decreases at rates far exceeding the rates seen in the Arctic. Proc. Natl. Acad. Sci. U.S.A. 116, 14414–14423. doi: 10.1073/pnas.1906556116
Peck, L., Brockington, S., Vanhove, S., and Beghyn, M. (1999). Community recovery following catastrophic iceberg impacts in a soft-sediment shallow-water site at Signy Island, Antarctica. Mar. Ecol. Prog. Ser. 186, 1–8.
Pelegrí, S. P., and Blackburn, T. H. (1994). Bioturbation effects of the amphipod Corophium volutator on microbial nitrogen transformations in marine sediments. Mar. Biol. 121, 253–258. doi: 10.1007/BF00346733
Pfannkuche, O. (1993). Benthic response to the sedimentation of particulate organic matter at the BIOTRANS station, 47°N, 20°W. Deep Sea Res. Part II Top. Stud. Oceanogr. 40, 135–149. doi: 10.1016/0967-0645(93)90010-K
Post, A. L., O’Brien, P. E., Beaman, R. J., Riddle, M. J., and De Santis, L. (2010). Physical controls on deep water coral communities on the George V Land slope, East Antarctica. Antarct. Sci. 22, 371–378. doi: 10.1017/S0954102010000180
Pudsey, C. J., Evans, J., Domack, E. W., Morris, P., and Valle, R. A. D. (2001). Bathymetry and acoustic facies beneath the former Larsen-A and Prince Gustav ice shelves, north-west Weddell Sea. Antarct. Sci. 13, 312–322. doi: 10.1017/S095410200100044X
Pudsey, C. J., Murray, J. W., Appleby, P., and Evans, J. (2006). Ice shelf history from petrographic and foraminiferal evidence, Northeast Antarctic Peninsula. Quat. Sci. Rev. 25, 2357–2379. doi: 10.1016/j.quascirev.2006.01.029
Rack, W., and Rott, H. (2003). “Further retreat of the northern Larsen Ice Shelf and collapse of Larsen B,” in Proceedings of the 16th International Workshop of the Forum for Research on Ice Shelf Processes (FRISP), (Bergen: University of Bergen).
Rack, W., and Rott, H. (2004). Pattern of retreat and disintegration of the Larsen B ice shelf, Antarctic Peninsula. Ann. Glaciol. 39, 505–510. doi: 10.3189/172756404781814005
Rainville, L., Lee, C., and Woodgate, R. (2011). Impact of wind-driven mixing in the Arctic Ocean. Oceanography 24, 136–145. doi: 10.5670/oceanog.2011.65
Raupach, M. J., Mayer, C., Malyutina, M., and Wägele, J.-W. (2009). Multiple origins of deep-sea Asellota (Crustacea: Isopoda) from shallow waters revealed by molecular data. Proc. R. Soc. B 276, 799–808. doi: 10.1098/rspb.2008.1063
Rehm, P., Thatje, S., Mühlenhardt-Siegel, U., and Brandt, A. (2007). Composition and distribution of the peracarid crustacean fauna along a latitudinal transect off Victoria Land (Ross Sea, Antarctica) with special emphasis on the Cumacea. Polar Biol. 30, 871–881. doi: 10.1007/s00300-006-0247-x
Riddle, M. J., Craven, M., Goldsworthy, P. M., and Carsey, F. (2007). A diverse benthic assemblage 100 km from open water under the Amery Ice Shelf, Antarctica. Paleoceanography 22:A1204. doi: 10.1029/2006PA001327
Riehl, T., Brandão, S. N., and Brandt, A. (2020). “Conquering the ocean depths over three geological eras,” in The Natural History of the Crustacea: Evolution and Biogeography of the Crustacea, eds M. Thiel and G. Poore (New York, NY: Oxford University Press), 155–182.
Rignot, E., Jacobs, S., Mouginot, J., and Scheuchl, B. (2013). Ice-shelf melting around Antarctica. Science 341, 266–270. doi: 10.1126/science.1235798
Rose, A., Ingels, J., Raes, M., Vanreusel, A., and Arbizu, P. M. (2015). Long-term iceshelf-covered meiobenthic communities of the Antarctic continental shelf resemble those of the deep sea. Mar. Biodiv. 45, 743–762.
Rott, H., Rack, W., Nagler, T., and Skvarca, P. (1998). Climatically induced retreat and collapse of northern Larsen Ice Shelf, Antarctic Peninsula. Ann. Glaciol. 27, 86–92. doi: 10.3189/S0260305500017262
Rott, H., Skvarca, P., and Nagler, T. (1996). Rapid collapse of northern Larsen Ice Shelf, Antarctica. Science 271, 788–792. doi: 10.1126/science.271.5250.788
Runcie, J. W., and Riddle, M. J. (2006). Photosynthesis of marine macroalgae in ice-covered and ice-free environments in East Antarctica. Eur. J. Phycol. 41, 223–233. doi: 10.1080/09670260600645824
Schnack-Schiel, S. B., and Isla, E. (2005). The role of zooplankton in the pelagic-benthic coupling of the Southern Ocean. Sci. Mar. 69, 39–55. doi: 10.3989/scimar.2005.69s239
Smale, D. A., Brown, K. M., Barnes, D. K. A., Fraser, K. P. P., and Clarke, A. (2008). Ice ccour disturbance in Antarctic waters. Science 321, 371–371. doi: 10.1126/science.1158647
Smith, J. A., Graham, A. G. C., Post, A. L., Hillenbrand, C.-D., Bart, P. J., and Powell, R. D. (2019). The marine geological imprint of Antarctic ice shelves. Nat. Commun. 10:5635. doi: 10.1038/s41467-019-13496-5
Smith, K. L. (2011). Free-drifting icebergs in the Southern Ocean: an overview. Deep Sea Res. Part II Top. Stud. Oceanogr. 58, 1277–1284. doi: 10.1016/j.dsr2.2010.11.003
Sorbe, J. C. (1999). Deep-sea macrofaunal assemblages within the Benthic Boundary Layer of the Cap-Ferret Canyon (Bay of Biscay, NE Atlantic). Deep Sea Res. Part II Top. Stud. Oceanogr. 46, 2309–2329. doi: 10.1016/S0967-0645(99)00064-8
Thatje, S., and Arntz, W. E. (2004). Antarctic reptant decapods: more than a myth? Polar Biol. 27, 195–201. doi: 10.1007/s00300-003-0583-z
Thatje, S., Hillenbrand, C.-D., and Larter, R. (2005). On the origin of Antarctic marine benthic community structure. Trends Ecol. Evol. 20, 534–540. doi: 10.1016/j.tree.2005.07.010
Thiel, M., and Hinojosa, I. (2009). “Peracarida–amphipods, isopods, tanaidaceans & cumaceans,” in Marine Benthic Fauna of Chilean Patagonia: Illustrated Identification Guide, eds V. Häussermann and G. Försterra (Santiago del Chile, CL: Nature in Focus), 671–738.
Tyberghein, L., Verbruggen, H., Pauly, K., Troupin, C., Mineur, F., and Clerck, O. D. (2012). Bio-ORACLE: a global environmental dataset for marine species distribution modelling. Glob. Ecol. Biogeogr. 21, 272–281. doi: 10.1111/j.1466-8238.2011.00656.x
Valdivia, N., Garrido, I., Bruning, P., Piñones, A., and Pardo, L. M. (2020). Biodiversity of an Antarctic rocky subtidal community and its relationship with glacier meltdown processes. Mar. Env. Res. 159, 104991. doi: 10.1016/j.marenvres.2020.104991
Vallet, C., and Dauvin, J.-C. (2001). Biomass changes and bentho-pelagic transfers throughout the Benthic Boundary Layer in the English Channel. J. Plankton Res. 23, 903–922. doi: 10.1093/plankt/23.9.903
Vanhove, S., Wittoeck, J., Desmet, G., Van den Berghe, B., Herman, R., Bak, R. P. M., et al. (1995). Deep-sea meiofauna communities in Antarctica: structural analysis and relation with the environment. Mar. Ecol. Prog. Ser. 127, 65–76. doi: 10.3354/meps127065
Vernet, M., Geibert, W., Hoppema, M., Brown, P. J., Haas, C., Hellmer, H. H., et al. (2019). The Weddell Gyre, Southern Ocean: present knowledge and future challenges. Rev. Geophys. 57, 623–708. doi: 10.1029/2018RG000604
Wing, S. R., Leichter, J. J., Wing, L. C., Stokes, D., Genovese, S. J., McMullin, R. M., et al. (2018). Contribution of sea ice microbial production to Antarctic benthic communities is driven by sea ice dynamics and composition of functional guilds. Glob. Chang. Biol. 24, 3642–3653. doi: 10.1111/gcb.14291
Witte, U., Wenzhöfer, F., Sommer, S., Boetius, A., Heinz, P., Aberle, N., et al. (2003). In situ experimental evidence of the fate of a phytodetritus pulse at the abyssal sea floor. Nature 424, 763–766. doi: 10.1038/nature01799
Wlodarska-Kowalczuk, M., Kendall, M. A., Marcin Weslawski, J., Klages, M., and Soltwedel, T. (2004). Depth gradients of benthic standing stock and diversity on the continental margin at a high-latitude ice-free site (off Spitsbergen, 79°N). Deep Sea Res. Part I Oceanogr. Res. Pap. 51, 1903–1914. doi: 10.1016/j.dsr.2004.07.013
Xavier, J. C., Cherel, Y., Boxshall, G., Brandt, A., Coffer, T., Forman, J., et al. (2020). Crustacean Guide for Predator Studies in the Southern Ocean. Cambridge: Scientific Committee on Antarctic Research, 255.
Yi, D., Zwally, H. J., and Robbins, J. W. (2011). ICESat observations of seasonal and interannual variations of sea-ice freeboard and estimated thickness in the Weddell Sea, Antarctica (2003–2009). Ann. Glaciol. 52, 43–51. doi: 10.3189/172756411795931480
Keywords: the Weddell Sea, Southern Ocean, ice coverage, environmental variables, Peracarida, Crustacea, abundance, distribution pattern
Citation: Di Franco D, Linse K, Griffiths HJ, Haas C, Saeedi H and Brandt A (2020) Abundance and Distributional Patterns of Benthic Peracarid Crustaceans From the Atlantic Sector of the Southern Ocean and Weddell Sea. Front. Mar. Sci. 7:554663. doi: 10.3389/fmars.2020.554663
Received: 22 April 2020; Accepted: 16 September 2020;
Published: 07 October 2020.
Edited by:
Christian Marcelo Ibáñez, Andres Bello University, ChileReviewed by:
Aldo S. Pacheco, National University of San Marcos, PeruJavier Sellanes, Catholic University of the North, Chile
Martin Thiel, Catholic University of the North, Chile
Copyright © 2020 Di Franco, Linse, Griffiths, Haas, Saeedi and Brandt. This is an open-access article distributed under the terms of the Creative Commons Attribution License (CC BY). The use, distribution or reproduction in other forums is permitted, provided the original author(s) and the copyright owner(s) are credited and that the original publication in this journal is cited, in accordance with accepted academic practice. No use, distribution or reproduction is permitted which does not comply with these terms.
*Correspondence: Davide Di Franco, ZGF2aWRlLmRpLWZyYW5jb0BzZW5ja2VuYmVyZy5kZQ==