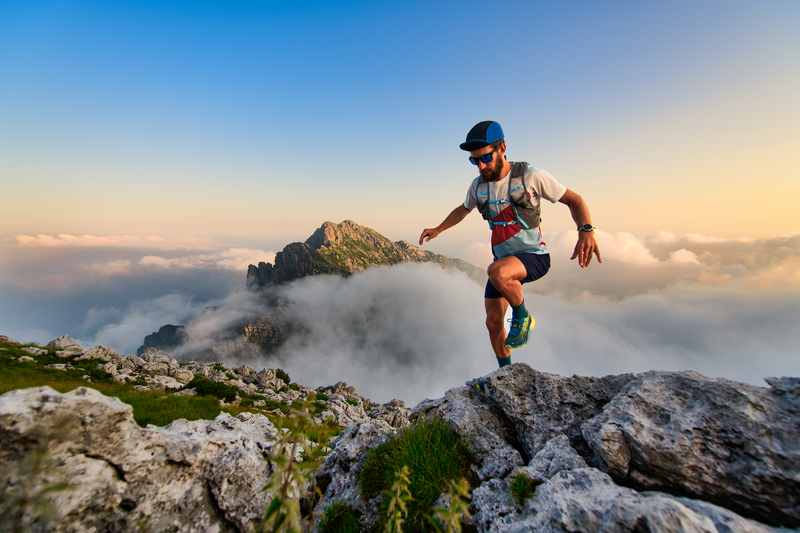
95% of researchers rate our articles as excellent or good
Learn more about the work of our research integrity team to safeguard the quality of each article we publish.
Find out more
ORIGINAL RESEARCH article
Front. Mar. Sci. , 17 September 2020
Sec. Marine Biology
Volume 7 - 2020 | https://doi.org/10.3389/fmars.2020.547276
Acidification and deoxygenation are two consequences of climate change that also co-occur in eutrophied coastal zones and can have deleterious effects on marine life. While the effects of hypoxia on marine herbivores have been well-studied, how ocean acidification combined with hypoxia affects herbivory is poorly understood. This study examined how herbivory and survival by the gastropod Lacuna vincta grazing on the macroalgae Ulva rigida was influenced by hypoxia and ocean acidification, alone and in combination, with and without food limitation. Experiments exposed L. vincta to a range of environmentally realistic dissolved oxygen (0.7 – 8 mg L–1) and pH (7.3 – 8.0 total scale) conditions for 3 – 72 h, with and without a starvation period and quantified herbivory and survival. While acidified conditions (pH < 7.4) reduced herbivory when combined with food limitation, low oxygen conditions (< 4 mg L–1) reduced herbivory and survival regardless of food supply. When L. vincta were starved and grazed in acidified conditions herbivory was additively reduced, whereas starvation and hypoxia synergistically reduced grazing rates. Overall, low oxygen had a more inhibitory effect on herbivory than low pH. Shorter exposure times (9, 6, and 3 h) were required to reduce grazing at lower DO levels (∼2.4, ∼1.6, and ∼0.7 mg L–1, respectively). Herbivory ceased entirely following a three-hour exposure to DO of 0.7 mg L–1 suggesting that episodes of diurnal hypoxia disrupt grazing by these gastropods. The suppression of herbivory in response to acidified and hypoxic conditions could create a positive feedback loop that promotes ‘green tides’ whereby reduced grazing facilitates the overgrowth of macroalgae that cause nocturnal acidification and hypoxia, further disrupting herbivory and promoting the growth of macroalgae. Such feedback loops could have broad implications for estuarine ecosystems where L. vincta is a dominant macroalgal grazer and will intensify as climate change accelerates.
The world’s oceans are acidifying and experiencing deoxygenation. The continued delivery of CO2 into surface oceans is lowering levels of pH, CO32–, and calcite and aragonite saturation states (Doney et al., 2009; Feely et al., 2009). Ocean warming in response to excessive atmospheric CO2 (Solomon et al., 2009) has the added consequence of deoxygenation through decreased oxygen solubility and increased water column stratification (Gruber, 2011; Breitburg et al., 2018). It is estimated that global ocean dissolved oxygen (DO) levels will continue to decrease over the next century, particularly in the 200 – 400 m depth range of North Pacific and Southern Oceans, as well as in the tropical Atlantic and Pacific Oceans (Stramma et al., 2008; Keeling et al., 2010; Falkowski et al., 2011).
Beyond the open ocean, coastal ecosystems and estuaries can also be subjected to long-term and seasonal declines in oxygen and pH levels (Diaz and Rosenberg, 2008; Gilbert et al., 2010; Wallace et al., 2014; Breitburg et al., 2018). Coastal marine ecosystems are both ecologically and economically productive and as human coastal populations expand, these critical habitats have become subject to a suite of anthropogenic stressors, including acidification and hypoxia (Gobler and Baumann, 2016). Deoxygenation is exacerbated in estuarine ecosystems by eutrophication that can contribute to increased primary production and subsequent microbial respiration of the decaying algal biomass (Diaz and Rosenberg, 2008; Falkowski et al., 2011). Microbial respiration of phytoplankton biomass also releases respiratory CO2 that can elevate pCO2 in some coastal zones to levels not expected in open ocean systems for more than a century (> 1,000 μatm; Cai et al., 2011; Wallace et al., 2014; Cai et al., 2017). Intensified wind-driven upwelling via increased atmospheric warming can also introduce acidified and hypoxic waters into coastal zones and estuaries (Waldbusser and Salisbury, 2014; Levin and Breitburg, 2015). Temperate regions are most vulnerable to acidified and hypoxic conditions during the warmer months when respiration rates and water column stratification are maximal (Gobler and Baumann, 2016). In stratified coastal ecosystems, bottom waters are more likely to experience acidified and hypoxic conditions, which suggests that benthic organisms are subjected to these stressors for longer periods of time than pelagic organisms (Wallace et al., 2014; Breitburg et al., 2018). In shallow, well-mixed coastal ecosystems, hypoxia, and acidification can occur diurnally, with the cumulative effects of nighttime respiration significantly decreasing DO and pH levels and exposing pelagic and benthic organisms to these stressors (Wootton et al., 2008; Baumann et al., 2015; Gobler and Baumann, 2016).
Independently and combined, acidification and hypoxia can have deleterious effects on a variety of estuarine organisms, including bivalves (Miller et al., 2002; Talmage and Gobler, 2010; Gobler et al., 2014), fish (Baumann et al., 2011; DePasquale et al., 2015; Morrell and Gobler, 2020), and gastropods (McMahon and Russell-Hunter, 1978; Shirayama and Thorton, 2005; Young et al., 2019). These broad effects across multiple trophic levels organisms that represent important fisheries, or are prey that support them, can result in a significant reduction in the production of these fisheries in estuaries (Gobler and Baumann, 2016; Grear et al., 2020). For gastropods, elevated pCO2 or reduced pH levels have been shown to decrease shell growth (Shirayama and Thorton, 2005), fertility (Hendriks et al., 2010), and metabolism (Melatunan et al., 2011). Similarly, exposure of gastropods to low DO concentrations has been shown to slow the rates of shell growth and feeding (Cheung et al., 2008), as well as reduce growth rates and metabolism (Kapper and Stickle, 1987; Li and Chiu, 2013). Furthermore, for both stressors, decreased metabolism are potentially related to an increased reliance on anaerobic metabolism (Bishop and Brand, 2000; Seibel and Walsh, 2003; Tripp-Valdez et al., 2017).
Common to estuarine ecosystems of the Northwest Atlantic Ocean, the northern Lacuna snail, Lacuna vincta (Gastropoda), is known to graze on various genera of macroalgae, including Laminaria, Saccharina, and Ulva (Brady-Campbell et al., 1984; Nelson et al., 2008; Krumhansl and Scheibling, 2011). The snail can consume up to 25% of macroalgal biomass and thus can serve as an effective top-down control on macroalgal growth (Nelson et al., 2008; Molis et al., 2010). The extent to which grazing by L. vincta, or other estuarine grazers, is affected by combined acidified and hypoxic conditions, however, is not well understood. Given that some genera of macroalgae, such as Ulva, undergo enhanced growth under elevated pCO2 (Olischläger et al., 2013; Young and Gobler, 2016; Ober and Thornber, 2017) and grazers such as L. vincta experience depressed herbivory rates when exposed to elevated pCO2 in the absence of food (Young et al., 2019), the potential stress of hypoxia alone or combined with ocean acidification could alter consumption of macroalgal biomass, which may influence the proliferation of the algal biomass.
Following our previous study (Young et al., 2019), which demonstrated that ocean acidification and food limitation can combine to reduce rates of herbivory in L. vincta, this study sought to assess how hypoxic conditions, with and without acidification and with and without food limitation, affect the grazing and survival of L. vincta feeding on Northwest Atlantic populations of the macroalgae, Ulva rigida. A series of experiments were performed to quantify changes in grazing and survival following direct exposure to low pH and/or low DO with and without food limitation prior to experiments. Acidification and hypoxia have been shown to have additive or synergistic effects on marine life (Gobler et al., 2014; Gobler and Baumann, 2016), including gastropods (Kim et al., 2013), with synergistic effects yielding outcomes more intense that would be predicted based on exposure to low DO and low pH individually. We hypothesized that the effects of low pH and low DO would be additive. Prior studies have demonstrated that adequate food supply can make individuals resilient to ocean acidification (Melzner et al., 2011; Thomsen et al., 2013; Pansch et al., 2014). We, therefore, hypothesized that the effects of hypoxia and acidification on L. vincta would manifest only when individuals were starved. Experiments were also performed using a gradient of low DO intensities (i.e., concentrations) and exposure durations to identify the precise DO conditions required to alter grazing behavior and survival in L. vincta. Responses to such gradients of low DO can follow a threshold effect (i.e., a level below which effects are manifested) or can be dose-dependent response (i.e., responses proportional to changes in DO; Levin, 2003; Vaquer-Sunyer and Duarte, 2008). We hypothesized that L. vincta would respond in a dose-dependent manner to both low DO intensity and duration of low DO exposure.
Lacuna vincta and U. rigida used for this study were collected from Shinnecock Bay, NY, United States (40.85°N, 72.50°W; Supplementary Figure S1) during low tides. Large, well-pigmented fronds of U. rigida and similarly sized L. vincta (∼3 mm) were collected by hand, placed in containers filled with seawater, and transported to the Stony Brook Southampton Marine Science Center within 15 min of collection. Upon arrival to the facility, L vincta were placed in a 20 L polycarbonate vessel filled with filtered (0.2 μm polysulfone filter capsule, Pall©) seawater obtained from the collection site. The vessel was provided with aeration and recently collected U. rigida was supplied as a food source until experiments were initiated. Consistent with our previous sequencing and microscopy (Young and Gobler, 2016) Ulva samples were confirmed as U. rigida. L. vincta is an abundant macroalgae grazer in estuaries of the northern United States (Chavanich and Harris, 2002; Janiak and Whitlatch, 2012) and were identified based on morphology.
For each experiment, circular section samples (∼3.5 cm in diameter) of U. rigida were cut from large thalli with care taken to avoid the potentially reproductive outer region of the algae (Wallace and Gobler, 2015). The samples were placed in a salad spinner to remove debris and epiphytes, extensively rinsed with filtered seawater, and spun again to further remove any debris and epiphytes (Young and Gobler, 2016). Excess seawater was removed by placing the samples on a lint-free Kimwipe and samples were weighed on a Scientech ZSA-120 digital microbalance (± 0.0001 g) to obtain initial wet weights. All samples were kept in 100 mL filtered seawater-filled containers after cleaning and weighing to prevent desiccation prior to introduction of U. rigida to experiments.
Nine experiments were performed to assess the effects of low DO and/or low pH on the herbivory rates of L. vincta on U. rigida as well as the survival of the former. Each experiment was performed in 1 L polycarbonate vessels that were acid-washed (10% HCl) and liberally rinsed with deionized water prior to use. All experimental vessels were placed in an environmental control chamber set to a temperature (19 – 22°C), light intensity (∼250 μE m–2 s–1), and duration (14 h light: 10 h dark cycle) that was similar to ambient conditions at the collection site and near-optimal for animals and algae. Experimental vessels were filled with filtered seawater and randomly assigned, in quadruplicate, to each treatment, which varied based on the experiment performed. For each experimental treatment, two additional containers with U. rigida were filled with filtered seawater without L. vincta to assess residual growth. To minimize U. rigida growth during the grazing periods, the lights of the environmental control chamber were turned off 24 h prior to the introduction of L. vincta (Nelson et al., 2008; Young et al., 2019). For each treatment within each experiment, eight mature L. vincta (∼3 mm) were added to each replicate. Based on the amount of U. rigida used in experiments (∼8 – 9 cm2 per sample), the appropriate number of L. vincta was added. The number of L. vincta used mimicked densities found on U. rigida at the collection site (0.5 – 1 grazer cm–2) and reported in the literature (Chenelot and Konar, 2007; Dubois and Iken, 2012; Young et al., 2019).
Dissolved gases were delivered into each experimental vessel through aeration via air diffusers (Pentair) connected a 1 mL polystyrene serological pipette inserted into the bottom of each vessel and connected via Tygon tubing to an air source. Containers were subjected to varying DO and pH conditions via multitube gas proportioner systems (Cole Parmer® Flowmeter) that mixed ambient air with 5% CO2 and pure N2 gases (Clark and Gobler, 2016). The gases were mixed and delivered through gang valves into the serological pipettes that were fit through an opening in the plexiglass used to cover the experimental vessels. Bubbling was initiated 2 – 3 d prior to the start of each experiment to allow DO concentrations and carbonate chemistry to reach a state of equilibrium. A Honeywell DuraFET III ion-sensitive field-effect transistor-based pH sensor (± 0.01 pH unit, total scale) and a YSI Professional Plus multiparameter handheld meter were used to measure pH and DO, respectively, within vessels daily. Initial and final water samples were taken at the beginning and conclusion of experiments to directly measure dissolved inorganic carbon (DIC) within experimental vessels. Samples were preserved using a saturated mercuric chloride solution and stored at ∼4°C until analysis on a VINDTA 3D delivery system coupled with a UIC Inc. coulometer (model CM5017O) as per Young and Gobler (2018). CO2 levels (Table 1) were calculated from measured levels of DIC, pH, temperature, and salinity as well as the first and second dissociation constants of carbonic acid in seawater (Millero, 2010) using the program CO2SYS1. We used certified reference material (CRM; provided by Andrew Dickson, Scripps Institution of Oceanography, University of California, San Diego; batch 180 = 2224.47 μmol DIC kg–1 seawater) for quality assurance of measured samples, and analyses only proceeded when recovery of CRM was 99.9 – 100%.
Table 1. Values of temperature (°C), salinity (g kg–1), dissolved oxygen (DO; mg L–1), pH (total scale), pCO2 (μatm), total DIC (μmol kgSW–1), total alkalinity (TA; μmol kgSW–1), HCO3– (μmol kgSW–1), and the saturation state of aragonite (Ωarag) for Experiments 1 – 9.
Experiments presented here covered three topics: 1. The individual and combined effects of low pH and low DO, 2. Reciprocal transplant experiments that identified whether effects of low DO and low pH on L. vincta were important prior to grazing, during grazing, or during both periods, and 3. An assessment of the effective minimal dose of, and duration of exposure to, low DO that altered performance of L. vincta. For the first set of experiments, two experiments (designated as Experiments 1 and 2; Table 2) were performed to gauge the herbivory rates and survival of L. vincta exposed to low DO and/or low pH. For Experiment 1, L. vincta were moved to vessels without U. rigida (i.e., starved) for 24 h prior to exposure to one of four treatments: a control with ambient levels of DO and pH (7.98 ± 0.14 mg L–1 and 7.96 ± 0.05, respectively), a treatment with low DO (3.88 ± 0.20 mg L–1) and ambient pH (7.89 ± 0.06), a treatment with low pH (7.31 ± 0.05) and ambient DO (7.89 ± 0.11), and a treatment with low DO (3.60 ± 0.11 mg L–1) and pH (7.31 ± 0.04). At the end of the starvation period, the lights in the incubation chamber were turned off, U. rigida was introduced, and L. vincta were allowed to graze for 24 h. Experiment 2 followed a similar procedure as Experiment 1, except that DO levels were lower (2.42 ± 0.13 mg L–1), and L. vincta were not starved prior to the introduction of U. rigida and individuals were simply placed in their respective treatments (Tables 1, 2). This experiment, therefore, explored the extent to which lower DO levels changed effects on grazing and to assess if absence of food would exacerbate the effects. At the end of the grazing periods for both experiments, L. vincta and U. rigida were removed from the vessels, L. vincta survival was quantified, and U. rigida samples were weighed as described above. L. vincta that had expired during the experiment were non-responsive to touch and not anchored to U. rigida or the wall of the experimental vessel. Weights of U. rigida samples grazed by L. vincta were corrected for residual growth using the difference in weight of U. rigida samples without L. vincta. Herbivory rates, or the mean consumption rates of all snails per replicate, were calculated by obtaining the difference in the initial and final corrected algal weights and multiplied by 1,000 to convert weight to mg, then divided by the number of remaining grazers at the end of the grazing period and the time elapsed of the grazing period (mg grazer–1 d–1).
Food limitation can alter the response of L. vincta (Young et al., 2019) and other invertebrates (Pechenik et al., 2002; Melzner et al., 2011; Thomsen et al., 2013) to environmental stressors. For the second set of experiments, the two reciprocal transplant experiments were performed to quantify herbivory rates and survival of L. vincta when starved under ambient conditions or low DO (Experiment 3; Table 2) or low pH (Experiment 4; Table 2) and then allowed to graze on U. rigida under ambient (pH and DO; 7.97 ± 0.03 and 8.15 ± 0.06 mg L–1, respectively), low DO (pH and DO; 8.01 ± 0.07 and 2.49 ± 0.14 mg L–1, respectively), or low pH (pH and DO; 7.27 ± 0.07 and 8.09 ± 0.07 mg L–1, respectively) conditions. L. vincta were placed in ambient or low DO conditions, with pH being ambient for both (Experiment 3), or in ambient or low pH conditions, with DO being ambient for both (Experiment 4) and starved for 24 h. At the end of the starvation period, the lights of the environmental chamber were turned off, and half of the L. vincta from each treatment were introduced into U. rigida-containing vessels and allowed to graze for 72 h under ambient, low DO, or low pH conditions (Table 2). At the end of the grazing periods, L. vincta and U. rigida were removed, L. vincta survival was assessed, and herbivory rates were calculated.
The third set of experiments examined the effective minimal dose and duration of low DO exposures in five separate experiments gauging herbivory rates and survival of L. vincta when exposed to varying DO concentrations for varying durations. The fifth experiment (Experiment 5; Table 2) was performed to quantify herbivory rates and survival of L. vincta when exposed to DO concentrations of 7.85 ± 0.08, 5.94 ± 0.22, 3.85 ± 0.44, 1.75 ± 0.13 mg L–1. For all treatments, pH was, on average, 7.93 ± 0.04. L. vincta were starved in their respective treatments for 24 h. Following the starvation period, U. rigida was introduced, and L. vincta were allowed to graze for 72 h. At the end of the grazing period, L. vincta and U. rigida were removed from the vessels, L. vincta survival was quantified, and herbivory rates were calculated. Three experiments (Experiments 6 – 8; Table 2) were performed to quantify the effective minimum duration of low DO exposure required to reduce herbivory rates and survival in L. vincta. For Experiments 6, L. vincta were starved for 24 h in ambient conditions (pH and DO; 7.93 ± 0.04 and 7.88 ± 0.07 mg L–1, respectively) before being placed in one of five treatments: a control with L. vincta allowed to graze on U. rigida for 12 h (no exposure), and treatments of 3, 6, 9, and 12 h of exposure to 2.36 ± 0.08 mg DO L–1 and a pH of 7.94 ± 0.02. Experiment 7 followed a similar procedure as Experiment 6, with the only modification being that DO concentrations for the low DO treatments were 1.55 ± 0.07 mg L–1. For Experiment 8, L. vincta were starved for 24 h in ambient conditions before being placed in one of three treatments: a control with L. vincta allowed to graze on U. rigida for 6 h (no exposure), a treatment with L. vincta allowed to graze in ambient DO and low DO (pH and DO; 7.93 ± 0.03 and 0.72 ± 0.04 mg L–1, respectively) for 3 h each, and a treatment with L. vincta allowed to graze in low DO for 6 h.
A final experiment was performed to gauge if food limitation under low DO affects herbivory rates or survival. For Experiment 9, L. vincta were placed in one of two treatments: a treatment that received U. rigida (non-starved) and a treatment that did not receive U. rigida (starved). Both treatments received ambient pH/DO (pH and DO = 7.97 ± 0.02 and 8.09 ± 0.02 mg L–1, respectively) and were kept at those conditions for 24 h. At the end of the 24 h incubation period, L. vincta were placed into containers with low DO concentrations (0.75 ± 0.06 mg L–1) and allowed to graze on U. rigida samples for 3 h. At the end of the 3 h grazing period, L. vincta and U. rigida were removed from the vessels, L. vincta survival was quantified, and herbivory rates were calculated.
Two-way ANOVA were performed within SigmaPlot 11.0 for Experiments 1 – 4 to assess differences in herbivory rates or survival, where the main treatment effects, for Experiments 1 and 2, were pH levels (ambient or low) and DO concentrations (ambient or low), and, for Experiments 3 and 4, DO or pH levels during the starvation and grazing periods (ambient or low for both) (Table 2). For all two-way ANOVAs, significant interactions between pH and DO were deemed synergistic when the outcome of the combined treatment yielded an outcome more intense than would be expected based on the results of the individual treatments. Alternatively, significant interactions that yielded outcomes less intense than the expectation based on the individual treatments would be deemed antagonistic, although we did not observe such an interaction during this study. One-way ANOVAs were performed within SigmaPlot for Experiments 5 – 9 to assess significant differences in herbivory rates or survival between treatments within their respective experiments. Equal variance (via Brown -Forsythe tests) were and normality (via Shapiro-Wilk tests) of data sets were assessed using SigmaPlot 11.0. Survival percentages were arc-sin-square root transformed before testing to ensure equal variance and normality were met. If significant differences were detected, a Tukey Honest Significant Difference (HSD) test using R 3.4.0 within RStudio 1.0.143 was performed.
For Experiment 1, herbivory rates were sensitive to changes in DO and pH levels following an initial starvation period. Herbivory was 50% and significantly lower in treatments where L. vincta were exposed to low DO concentrations relative to treatments with ambient DO concentrations following the prior 24 h starvation period (Two-way ANOVA and Tukey HSD; p < 0.05; Figure 1; Supplementary Tables S1, S2). Herbivory rates were also significantly lower in low pH treatments than in ambient pH treatments by ∼50% (Two-way ANOVA and Tukey HSD; p < 0.05; Figure 1; Supplementary Tables S1, S2). The treatment with low pH and low DO had significantly lower herbivory rates than the other treatments in the experiment with the two factors additively reducing rates by more than 80% (Tukey HSD; p < 0.05; Figure 1; Supplementary Table S2). There was 100% survival for all treatments. For Experiment 2 when snails were not starved and DO levels were lower (2.42 ± 0.13 mg L–1), herbivory rates were significantly lower by ∼95% when L. vincta were exposed to low DO levels, regardless of pH level (Two-way ANOVA and Tukey HSD; p < 0.05; Figure 2; Supplementary Tables S3, S4). Furthermore, in the low DO treatment, herbivory rates were ∼90% lower in Experiment 2 compared to Experiment 1 (Figures 1, 2). The combined low pH/DO treatment had herbivory rates near 0 mg grazer–1 d–1, and while it was significantly lower than the control and low pH treatments (Two-way ANOVA and Tukey HSD; p < 0.05 for both; Figure 2; Supplementary Tables S3, S4), it was not significantly different compared to the ambient pH and low DO treatment (Two-way ANOVA and Tukey HSD; p > 0.05; Figure 2; Supplementary Tables S3, S4). In contrast to herbivory rates, L. vincta survival was sensitive to changes in DO and pH levels, being significantly lower in treatments with low DO and low pH (Two-way ANOVA and Tukey HSD; p < 0.05 for both; Figure 2; Supplementary Tables S3, S4). Furthermore, the combined low DO and low pH treatment additively depressed survival, having rates significantly lower than all other treatments in the experiment (Tukey HSD; p < 0.05; Figure 2; Supplementary Table S4).
Figure 1. Herbivory rates of L. vincta grazing on U. rigida with initial starvation under control (ambient pH/DO), low DO, low pH, and low pH/DO (Experiment 1; Table 2). Columns represent means ± standard deviation. Statistical analyses: Two-way ANOVA and post hoc Tukey Honest Significant Difference tests performed; significant differences between treatments represent p-values less than 0.05.
Figure 2. Herbivory rates and survival of L. vincta grazing on U. rigida without initial starvation under control (ambient pH/DO), low DO, low pH, and low pH/DO (Experiment 2; Table 2). Columns represent means ± standard deviation. Statistical analyses: Two-way ANOVA and post-hoc Tukey Honest Significant Difference tests performed; significant differences between treatments represent p-values less than 0.05.
For L. vincta starved in either ambient DO or low DO conditions and transplanted into ambient, low DO, or low pH treatments (Experiment 3), herbivory rates and survival were sensitive to the conditions that the organisms grazed in, and not the conditions they were initially starved in. There was no significant difference in herbivory rates or survival when L. vincta were starved in either ambient or low DO conditions (Two-way ANOVA and Tukey HSD; p < 0.05 for all; Figure 3; Supplementary Tables S5, S6). In contrast, herbivory rates and survival were significantly lower when L. vincta grazed in the low DO treatments relative to the treatments where L. vincta grazed in ambient pH, ambient DO, or low pH conditions (Two-way ANOVA and Tukey HSD; p < 0.05; Figure 3; Supplementary Tables S5, S6). Specifically, herbivory ceased entirely (0 mg grazer–1 d–1) in the treatment where L. vincta were starved in ambient DO and grazed in low DO, although it was not significantly different than herbivory rates in the treatment where L. vincta were starved and grazed in low DO (Two-way ANOVA and Tukey HSD; p > 0.05; Figure 3; Supplementary Tables S5 and S6). There were no significant differences in herbivory rates or survival between the treatments where L. vincta grazed in ambient pH/DO conditions or in low pH conditions (Two-way ANOVA and Tukey HSD; p < 0.05 for all; Supplementary Tables S5, S6).
Figure 3. Herbivory rates and survival of L. vincta grazing on U. rigida starved under ambient or low DO conditions and allowed to graze on U. rigida under ambient, low dissolved oxygen, or low pH conditions (Experiment 3; Table 2). Columns represent means ± standard deviation. Statistical analyses: Two-way ANOVA and post hoc Tukey Honest Significant Difference tests performed; significant differences between treatments represent p-values less than 0.05.
For L. vincta starved in ambient pH or low pH conditions and transplanted into ambient, low DO, or low pH treatments (Experiment 4), herbivory rates were sensitive to the conditions that the organisms were starved and grazed in. When L. vincta were starved under low pH conditions, herbivory was significantly lower compared to herbivory of snails starved under ambient conditions (Two-way ANOVA and Tukey HSD; p < 0.05; Figure 4; Supplementary Tables S7, S8). When snails were starved under low pH conditions, herbivory was reduced by 60, 75, and ∼70%, when grazing under ambient, low DO, and low pH conditions, respectively (Figure 4). Furthermore, herbivory rates were significantly lower when L. vincta grazed under low DO conditions but were starved under ambient conditions (Two-way ANOVA and Tukey HSD; p < 0.05; Figure 4; Supplementary Tables S7, S8). On average, grazing under low DO conditions reduced herbivory rates by ∼65 and ∼60% relative to herbivory rates in ambient and low pH treatments, respectively (Figure 4). Comparing individual treatments, there was no difference between the control and the treatment with L. vincta grazing in low pH after being starved in ambient conditions (Tukey HSD; p > 0.05; Figure 4; Supplementary Table S8) but all other treatments had significantly lower herbivory rates (Tukey HSD; p < 0.05 for all; Figure 4; Supplementary Table S8). Herbivory rates in the treatments with L. vincta grazing at ambient or low pH after being starved in low pH and the treatment that had L. vincta graze in low DO after being starved in ambient conditions were not significantly different between each other (Tukey HSD; p < 0.05 for all; Figure 4; Supplementary Table S8). However, herbivory was significantly and synergistically decreased in the treatment that had L. vincta graze in low DO following starvation in low pH conditions compared to all other treatments, with rates dropping to nearly zero in these treatments (Tukey HSD; p < 0.05 for all; Figure 4; Supplementary Table S8). Survival rates were not significantly affected by the conditions in which L. vincta were starved in (Two-way ANOVA and Tukey HSD; p < 0.05; Figure 4; Supplementary Tables S7, S8) but were significantly decreased when snails grazed in low DO treatments (Two-way ANOVA and Tukey HSD; p < 0.05; Figure 4; Supplementary Tables S7, S8). On average, survival was ∼30% lower when L. vincta grazed in low DO compared to ambient and low pH treatments (Figure 4).
Figure 4. Herbivory rates and survival of L. vincta grazing on U. rigida starved under ambient or low pH and allowed to graze on U. rigida under ambient, low dissolved oxygen, or low pH conditions (Experiment 4; Table 2). Columns represent means ± standard deviation. Statistical analyses: Two-way ANOVA and post hoc Tukey Honest Significant Difference tests performed; significant differences between treatments represent p-values less than 0.05.
For Experiment 5, low DO concentrations significantly reduced herbivory rates and survival. Herbivory rates were significantly lower at the 1.8 and 3.9 mg L–1 DO treatments than in the ambient (∼7.9 mg L–1) and 5.9 mg L–1 DO treatments (One-way ANOVA and Tukey HSD; p < 0.05 for both; Figure 5; Supplementary Tables S9, S10) with no significant differences between the two lower DO levels and the two higher DO levels (One-way ANOVA and Tukey HSD; p > 0.05 for all; Figure 5; Supplementary Tables S9, S10). Paralleling herbivory, survival was significant lower at 1.8 and 3.9 mg L–1 DO treatments (50 and 70%, respectively) than the 5.9 and 7.9 mg L–1 DO treatments (90%; One-way ANOVA and Tukey HSD; p < 0.05 for all; Figure 5; Supplementary Tables S9, S10). While there was no significant difference in survival between the 5.9 and 7.9 mg L–1 DO treatments (One-way ANOVA and Tukey HSD; p > 0.05; Figure 5; Supplementary Tables S9, S10), survival was significantly lower in the 1.8 mg L–1 DO treatment than in the 3.9 mg L–1 DO treatment (One-way ANOVA and Tukey HSD; p < 0.05; Figure 5; Supplementary Tables S9, S10).
Figure 5. Herbivory rates and survival of L. vincta initially starved and allowed to graze on U. rigida under ambient (7.85 mg L–1), medium (5.94 mg L–1), low (3.85 mg L–1), and very low (1.75 mg L–1) DO concentrations for 72 h (Experiment 5; Table 2). Statistical analyses: One-way ANOVA and post hoc Tukey Honest Significant Difference tests performed; significant differences between treatments represent p-values less than 0.05.
For Experiment 6, nine and twelve hours of exposure to ∼2.4 mg L–1 DO significantly decreased herbivory rates, while 0, 3, and 6 h exposure did not (One-way ANOVA and Tukey HSD; p > 0.05 for all; Figure 6; Supplementary Tables S11, S12). However, there was no significant difference in herbivory rates between the 9 and 12 h exposure treatments nor between 0, 3, and 6 h exposure treatments (One-way ANOVA and Tukey HSD; p > 0.05; Figure 6; Supplementary Tables S11, S12). Herbivory ceased entirely in the 12 h exposure treatment (Figure 6). L. vincta survival was not different among treatments.
Figure 6. Herbivory rates of L. vincta initially starved for 24 h under ambient pH/DO and allowed to graze on U. rigida for 12 h with varying durations of exposure (0 – 12 h) to low DO concentrations (2.36 mg L–1) (Experiment 6; Table 2). Statistical analyses: One-way ANOVA and post hoc Tukey Honest Significant Difference tests performed; significant differences between treatments represent p-values less than 0.05.
In Experiment 7, the minimum exposure time to negatively affect herbivory rates at ∼1.6 mg L–1 DO was lower than at 2.6 mg L–1 DO. Herbivory in the 6 h exposure treatment was significantly lower than in treatments with 0 and 3 h exposure (One-way ANOVA and Tukey HSD; p < 0.05 for both; Figure 7; Supplementary Tables S13, S14). Furthermore, herbivory rates in the 9 and 12 h exposure treatments were nearly zero and significantly lower than in the 0, 3, and 6 h exposure treatments (One-way ANOVA and Tukey HSD; p < 0.05 for all; Figure 7; Supplementary Tables S13, S14). However, there was no significant difference in herbivory rates between the 0 and 3 h exposure treatments or between the 9 and 12 h exposure treatments (One-way ANOVA and Tukey HSD; p > 0.05 for all; Figure 7; Supplementary Tables S13, S14). Survival in the 12 h exposure treatment was significantly lower than in the 0, 3, 6, and 9 h exposure treatments (One-way ANOVA and Tukey HSD; p < 0.05 for all; Figure 7; Supplementary Tables S13, S14). However, there was no significant difference between the 0, 3, 6, and 9 h exposure treatments (One-way ANOVA and Tukey HSD; p > 0.05 for all; Figure 7; Supplementary Tables S13, S14).
Figure 7. Herbivory rates of L. vincta initially starved for 24 h under ambient pH/DO and allowed to graze on U. rigida for 12 h with varying durations of exposure (0 – 12 h) to low DO concentrations (1.55 mg L–1) (Experiment 7; Table 2). Statistical analyses: One-way ANOVA and post-hoc Tukey Honest Significant Difference tests performed; significant differences between treatments represent p-values less than 0.05. Statistical analyses: One-way ANOVA and post hoc Tukey Honest Significant Difference tests performed; significant differences between treatments represent p-values less than 0.05.
For Experiment 8, three hours of exposure to ∼0.7 mg L–1 significantly reduced herbivory rates relative to no exposure and there was effectively no grazing after six hours of exposure leading to a rate significantly lower than the three hour exposure treatment as well as the no exposure control (One-way ANOVA and Tukey HSD; p < 0.05; Figure 8; Supplementary Tables S15, S16). L. vincta survival was 100% in all treatments. Finally, for Experiment 9, starving snails prior to low DO exposure did not alter herbivory rates of snails when exposed to low DO (One-way ANOVA; p > 0.05; Figure 9; Supplementary Table S17). There was 100% survival for all treatments.
Figure 8. Herbivory rates of L. vincta initially starved for 24 h under ambient pH/DO and allowed to graze on U. rigida for 6 h with varying durations of exposure (0 – 6 h) to low DO concentrations (0.72 mg L–1) (Experiment 8; Table 2). Statistical analyses: One-way ANOVA and post hoc Tukey Honest Significant Difference tests performed; significant differences between treatments represent p-values less than 0.05.
Figure 9. Herbivory rates of L. vincta either starved or not starved for 24 h under ambient DO and then allowed to graze on U. rigida for 3 h under low DO concentrations (0.75 mg L–1) (Experiment 9; Table 2).
During this study, herbivory and survival rates of L. vincta were significantly reduced when snails were exposed to hypoxic conditions and reduced further when previously deprived of food in acidified conditions. Food supply did not alter herbivory or survival under hypoxic conditions. Herbivory and survival were significantly reduced at DO concentrations below 4 mg L–1 and the duration of exposure needed to suppress herbivory and survival were shortened at lower DO concentrations. Under very low DO concentrations (< 1 mg L–1), herbivory decreased after only 3 h of exposure. Collectively, this study revealed how acidification and hypoxia can individually, additively, and synergistically disrupt gastropod herbivory on macroalgae, a fundamental process in estuarine ecosystems.
The continued delivery of CO2 into coastal zones is expected to have negative effects on the growth and survival of calcifying organisms, including gastropods, through decreases in calcium carbonate saturation states (Gazeau et al., 2013). Exposure to elevated pCO2 or low pH for extended periods of time (i.e., days to weeks) has been shown to slow shell rates of growth and repair, as well as disrupt the physiology of several species of marine gastropods (Shirayama and Thorton, 2005; Bibby et al., 2007; Manno et al., 2012). Beyond the effects of acidification, hypoxia has also been shown to have deleterious effects on marine gastropods. Among major classes of marine organisms, gastropods have the lowest median lethal oxygen threshold (Vaquer-Sunyer and Duarte, 2008). Reduced DO levels (< 3 mg L–1) have been shown to significantly reduce shell growth, somatic growth, feeding, and aerobic respiration (Kapper and Stickle, 1987; Cheung et al., 2008; Li and Chiu, 2013; Tripp-Valdez et al., 2017). During this study, herbivory was even more sensitive to hypoxia than these previously studied attributes, being significantly reduced at DO levels ≤ 4 mg L–1. While the effects of combined acidification and hypoxia on gastropods is poorly understood, these combined stressors have been shown to reduce somatic growth and survival in juvenile red abalone (Haliotis rufescens; Kim et al., 2013) and were found to both additively and synergistically reduce herbivory and survival in the current study.
The direct responses of L. vincta to low DO and low pH differed in some respects. While low pH had deleterious effects on herbivory only when L. vincta were starved, low DO reduced herbivory regardless of food limitation. While low pH almost never caused mortality, low DO often yielded significantly reduced survival, collectively demonstrating hypoxia had a stronger physiological impact on these gastropods. Consequently, the mechanisms by which each factor reduces herbivory and/or survival may have differed. Exposure to elevated pCO2 or low pH can cause acidosis, which can disrupt homeostatic functions and divert energy away from other metabolic processes (Lindinger et al., 1984; Pörtner et al., 1998; Marchant et al., 2010). When combined with food limitation, acidification lowers respiration rates and metabolism (Young et al., 2019), likely representing a survival strategy to match lowered energy supply from food limitation and potentially resulting in an increased reliance on anaerobic respiration (Pörtner et al., 1998; Melatunan et al., 2011). In a similar manner, when confronted with hypoxia, marine gastropods also undergo lowered respiration and metabolic rates, and an increased reliance on anaerobic metabolism to conserve energy (Kapper and Stickle, 1987; Wu, 2002; Cheung et al., 2008; Liu et al., 2014). Reductions in foraging and feeding may occur to further conserve limited energy reserves under hypoxic conditions and the depletion of those reserves can result in mortality (Liu et al., 2014) as was observed during this study. The combination of low pH and low DO commonly led to an additive reduction in herbivory and survival, supporting the hypothesis that they linearly promote a metabolic suppression, perhaps via the induction of anaerobic respiration (Pörtner et al., 1998; Melatunan et al., 2011). In the fourth experiment, however, starvation under acidified conditions followed by an exposure to hypoxia synergistically reduced herbivory, suggesting that this succession of events can overwhelm L. vincta physiology in a stronger, a non-linear manner yielding an outcome not predicted by either individual stressor.
In an ecosystem setting, marine gastropods sensitive to low pH and DO levels may be affected by the seasonal onsets of hypoxia and acidification. Seasonally, temperate estuaries become vulnerable to eutrophication-driven hypoxia and acidification during warmer months when respiration rates and water column stratification are maximal and oxygen saturation is minimal (Diaz and Rosenberg, 2008; Gilbert et al., 2010; Wallace et al., 2014; Gobler and Baumann, 2016; Young et al., 2019). In stratified water bodies, seasonal, benthic hypoxia and acidification can persist for days-to-months at pH and DO levels shown during this study to significantly reduce grazing by L. vincta (Melzner et al., 2013; Wallace et al., 2014; Baumann et al., 2015). In other cases, seasonally driven upwelling can move hypoxic and acidified water into coastal zones and may disrupt herbivory by gastropods (Feely et al., 2008, 2010).
The diurnal occurrence of hypoxia and acidification is also most likely to emerge in warmer, eutrophic ecosystems due to higher temperatures causing more rapid respiration rates and lower dissolved oxygen saturation (Gobler and Baumann, 2016). In the present study, L. vincta herbivory rates were significantly reduced following prolonged (24 h) exposure to DO levels of 4 mg L–1 with shorter durations of exposure (∼9, ∼6 and ∼3 h) required to reduce herbivory and survival at lower DO concentrations at (∼2.4, ∼1.6, and ∼0.7 mg L–1). We have previously demonstrated that food limitation and acidification can lower herbivory after 18 h of exposure to pCO2 levels of 1,500 μatm or greater (Young et al., 2019). The intensity and duration of diurnal hypoxia and acidification shown to reduce grazing rates in L. vincta and other marine gastropods (Kim et al., 2013) has been frequently documented in coastal ecosystems (Ringwood and Keppler, 2002; O’Boyle et al., 2013; Wallace et al., 2014; Baumann et al., 2015; Clark and Gobler, 2016; Gobler and Baumann, 2016) suggesting nocturnal disruption of herbivory due to low DO and low pH may be a common occurrence in estuaries.
Exposure to low pH only affected L. vincta herbivory rates when combined with food limitation. Resistance to acidification due to an abundance of food has been documented in other calcifying organisms (Pansch et al., 2014) and in forage fish (Gobler et al., 2018), bivalves (Melzner et al., 2011; Thomsen et al., 2013). Additionally, given that L. vincta utilizes macroalgae as both a habitat and a food source (Martel and Chia, 1991; Chavanich and Harris, 2002), the buffering capacity of macroalgae may minimize the harmful effects of exposure to acidification (Young and Gobler, 2018). In the present study, however, feeding did not provide a refuge from the negative effects of hypoxia, suggesting that the protection food offers against acidification may not occur in an ecosystem setting since hypoxia and acidification commonly coincide in space and time (Wallace et al., 2014; Baumann et al., 2015; Cai et al., 2017).
Ulva spp. can experience enhanced growth under conditions of elevated pCO2 and nutrient concentrations (Olischläger et al., 2013; Young and Gobler, 2016; Ober and Thornber, 2017). Beyond the negative effects of elevated pCO2 on gastropod grazers (Shirayama and Thorton, 2005; Bibby et al., 2007; Manno et al., 2012), excessive nutrients have been shown to decrease abundances of common estuarine grazers due to potentially toxic ammonia concentrations (Atalah and Crowe, 2012). Ulva serves as an important food source for L. vincta and other macroalgae-consuming estuarine gastropod, with the former accounting for the consumption of up to 25% of macroalgal biomass (Giannotti and McGlathery, 2001; Nelson et al., 2008; Zamprogno et al., 2013). Decreased herbivory on algal biomass wrought by hypoxia and acidification could synergistically facilitate the proliferation of Ulva blooms known as ‘green tides’ (Smetacek and Zingone, 2013; Zhao et al., 2013). Eutrophication, therefore, may initiate a positive feedback loop by promoting elevated nutrients and pCO2 coupled with hypoxia (Wallace et al., 2014), which could reduce herbivory rates by L. vincta that could facilitate the overgrowth of Ulva and, through decay, promote the additional accumulation of CO2, sulfides, and ammonia, and anoxia events (Valiela et al., 1997; Valiela and Cole, 2002; Liu et al., 2009) that would further inhibit L. vincta herbivory and, thus, further promote macroalgal overgrowth. Indeed, blooms of Ulva spp. are known to be associated with periods of hypoxia and anoxia (Valiela et al., 1992), and can promote acidification (pH < 7.2; Wallace, 2020).
The reductions in herbivory and survival of gastropods such as L. vincta in response to acidification and hypoxia have numerous ecosystem implications. L. vincta is a common grazer in ecosystems of the coastal Northwest Atlantic (Chavanich and Harris, 2002) and is the only mesograzer in the region known to consume macroscopic kelp sporophytes (Brady-Campbell et al., 1984; Johnson and Mann, 1986). The grazer’s preference for the commercially important sugar kelp (Saccharina saccharina), as well as other laminarialean kelps, can result in significant consumption of kelp blades and loss of kelp beds in Northwest Atlantic ecosystems when L. vincta populations increase (Fralick et al., 1974; Chavanich and Harris, 2002; Krumhansl and Scheibling, 2011). L. vincta has also been shown to consume other commercially-important macroalgae, such as Chondrus crispus (Shacklock and Croft, 1981; Janiak and Whitlatch, 2012) and Fucus serratus (Southgate, 1982; Jackson, 2007) as well as invasive macroalgae, such Sargassum muticum and Grateloupia turuturu (Buschbaum et al., 2006; Britton-Simmons et al., 2011; Janiak and Whitlatch, 2012). Many of the aforementioned macroalgal species have been shown to benefit from elevated pCO2 (Hepburn et al., 2011; Hofmann et al., 2012; Saderne, 2012; Xu et al., 2016; Xu et al., 2017). As such, future pCO2 increases coupled with decreased grazing pressure due to acidification and/or hypoxia may promote the growth of commercially important macroalgae as well as some invasive species.
In conclusion, the combination of low DO and low pH had both additive and synergistically negative effects on herbivory, with herbivory and survival being most impaired when snails were initially starved in low pH conditions and then were grazing under low DO. Reduced herbivory in response to acidification and hypoxia may create a positive feedback loop that facilitates an overgrowth of macroalgal biomass that may further promote hypoxia and the accumulation of respiratory CO2. Given the potential for macroalgae to buffer carbonate chemistry and release oxygen via photosynthesis, the extent to which the harmful effects of acidification and hypoxia on gastropods are realized may be influenced by the physiological status of macroalgae and certainly warrants further investigation. Regardless, climate change-induced reductions in DO and pH will make the disruption of gastropod herbivory a more common occurrence in the future.
The raw data supporting the conclusions of this article will be made available by the authors, without undue reservation, to any qualified researcher.
CG and CY conceived and designed the experiments. CY performed the experiments. CY and CG analyzed the data. CG contributed reagents, materials, and analysis tools. CY and CG wrote the paper.
This work was supported by the Rauch Foundation, Laurie Landeau Foundation, Simons Foundation, and New York Sea Grant (R-FBM-38).
The authors declare that the research was conducted in the absence of any commercial or financial relationships that could be construed as a potential conflict of interest.
We are appreciative of the logistical support provided by the Stony Brook Southampton Marine Science staff throughout the study. We thank Andrew Lundstrom for assistance on the analysis of samples for DIC.
The Supplementary Material for this article can be found online at: https://www.frontiersin.org/articles/10.3389/fmars.2020.547276/full#supplementary-material
Atalah, J., and Crowe, T. P. (2012). Nutrient enrichment and variation in community structure on rocky shores: the potential of molluscan assemblages for biomonitoring. Estuar. Coast. Shelf Sci. 99, 162–170. doi: 10.1016/j.ecss.2011.12.034
Baumann, H., Talmage, S. C., and Gobler, C. J. (2011). Reduced early life growth and survival in a fish in direct response to increased carbon dioxide. Nat. Clim. Change 2, 38–41. doi: 10.1038/nclimate1291
Baumann, H., Wallace, R. B., Tagliaferri, T., and Gobler, C. J. (2015). Large natural pH, CO2 and O2 fluctuations in a temperate tidal salt marsh on diel, seasonal, and interannual time scales. Estuar. Coasts 38, 220–231. doi: 10.1007/s12237-014-9800-y
Bibby, R., Cleall-Harding, P., Rundle, S., Widdicombe, S., and Spicer, J. (2007). Ocean acidification disrupts induced defences in the intertidal gastropod Littorina littorea. Biol. Lett. 3, 699–701. doi: 10.1098/rsbl.2007.0457
Bishop, T., and Brand, M. D. (2000). Processes contributing to metabolic depression in hepatopancreas cells from the snail Helix aspersa. J. Exp. Biol. 203, 3603–3612.
Brady-Campbell, M. M., Campbell, D. B., and Harlin, M. M. (1984). Productivity of kelp (Laminaria spp.) near the southern limit in the Northwestern Atlantic ocean. Mar. Ecol. Prog. Ser. 18, 79–88. doi: 10.3354/meps018079
Breitburg, D., Levin, L. A., Oschlies, A., Grégoire, M., Chavez, F. P., Conley, D. J., et al. (2018). Declining oxygen in the global ocean and coastal waters. Science 359:eaam7240. doi: 10.1126/science.aam7240
Britton-Simmons, K. H., Pister, B., Sánchez, I., and Okamoto, D. (2011). Response of a native, herbivorous snail to the introduced seaweed Sargassum muticum. Hydrobiologia 661, 187–196. doi: 10.1007/s10750-010-0523-1
Buschbaum, C., Chapman, A. S., and Saier, B. (2006). How an introduced seaweed can affect epibiota diversity in different coastal ecosystems. Mar. Biol. 148, 743–754. doi: 10.1007/s00227-005-0128-9
Cai, W.-J., Hu, X., Huang, W.-J., Murrell, M. C., Lehrter, J. C., Lohrenz, S. E., et al. (2011). Acidification of subsurface coastal waters enhanced by eutrophication. Nat. Geosci. 4, 766–770. doi: 10.1038/ngeo1297
Cai, W.-J., Huang, W.-J., Luther, G. W., Pierrot, D., Li, M., Testa, J., et al. (2017). Redox reactions and weak buffering capacity lead to acidification in the Chesapeake Bay. Nat. Commun. 8, 369. doi: 10.1038/s41467-017-00417-7
Chavanich, S., and Harris, L. G. (2002). The influence of macroalgae on seasonal abundance and feeding preference of a subtidal snail, Lacuna vincta (Montagu) (Littorinidae) in the Gulf of Maine. J. Molluscan Stud. 68, 73–78. doi: 10.1093/mollus/68.1.73
Chenelot, H., and Konar, B. (2007). Lacuna vincta (Mollusca, Neotaenioglossa) herbivory on juvenile and adult Nereocystis luetkeana (Heterokontophyta, Laminariales). Hydrobiologia 583, 107–118. doi: 10.1007/s10750-006-0484-6
Cheung, S. G., Chan, H. Y., Liu, C. C., and Shin, P. K. S. (2008). Effect of prolonged hypoxia on food consumption, respiration, growth and reproduction in marine scavenging gastropod Nassarius festivus. Mar. Pollut. Bull. 57, 280–286. doi: 10.1016/j.marpolbul.2008.03.039
Clark, H. R., and Gobler, C. J. (2016). Diurnal fluctuations in CO2 and dissolved oxygen concentrations do not provide a refuge from hypoxia and acidification for early-life-stage bivalves. Mar. Ecol. Prog. Ser. 558, 1–14. doi: 10.3354/meps11852
DePasquale, E. L., Baumann, H., and Gobler, C. J. (2015). Vulnerability of early life stage Northwest Atlantic forage fish to ocean acidification and low oxygen. Mar. Ecol. Prog. Ser. 523, 145–156. doi: 10.3354/meps11142
Diaz, R. J., and Rosenberg, R. (2008). Spreading dead zones and consequences for marine ecosystems. Science 321, 926–929. doi: 10.1126/science.1156401
Doney, S. C., Fabry, V. J., Feely, R. A., and Kleypas, J. A. (2009). Ocean acidification: the other CO2 problem. Annu. Rev. Mar. Sci. 1, 169–192. doi: 10.1146/annurev.marine.010908.163834
Dubois, A., and Iken, K. (2012). Seasonal variation in kelp phlorotannins in relation to grazer abundance and environmental variables in the Alaskan sublittoral zone. Algae 27, 9–19. doi: 10.4490/algae.2012.27.1.009
Falkowski, P. G., Algeo, T., Codispoti, L., Deutsch, C., Emerson, S., Hales, B., et al. (2011). Ocean deoxygenation: past, present, and future. Eos, Transactions American Geophysical Union 92, 409–410. doi: 10.1029/2011EO460001
Feely, R. A., Alin, S. R., Newton, J., Sabine, C. L., Warner, M., Devol, A., et al. (2010). The combined effects of ocean acidification, mixing, and respiration on pH and carbonate saturation in an urbanized estuary. Estuar. Coast. Shelf Sci. 88, 442–449. doi: 10.1016/j.ecss.2010.05.004
Feely, R. A., Doney, S. C., and Cooley, S. R. (2009). Ocean acidification: present conditions and future changes in a high-CO2 world. Oceanography 22, 36–47. doi: 10.5670/oceanog.2009.95
Feely, R. A., Sabine, C. L., Hernandez-Ayon, J. M., Ianson, D., and Hales, B. (2008). Evidence for upwelling of corrosive “acidified” water onto the continental shelf. Science 320, 1490–1492. doi: 10.1126/science.1155676
Fralick, R. A., Turgeon, K. W., and Mathieson, A. C. (1974). Destruction of kelp populations by Lacuna vincta (Montagu). Nautilus 88, 112–114.
Gazeau, F., Parker, L. M., Comeau, S., Gattuso, J.-P., O’Conner, W. A., Martin, S., et al. (2013). Impacts of ocean acidification on marine shelled molluscs. Mar. Biol. 160, 2207–2245. doi: 10.1007/s00227-013-2219-3
Giannotti, A. L., and McGlathery, K. J. (2001). Consumption of Ulva lactuca (Chlorophyta) by the omnivorous mud snail Hyanassa obsoleta (Say.). J. Phycol. 37, 209–215. doi: 10.1046/j.1529-8817.2001.037002209.x
Gilbert, D., Rabalais, N. N., Diaz, R. J., and Zhang, J. (2010). Evidence for greater oxygen decline rates in the coastal ocean than in the open ocean. Biogeosciences 7, 2283–2296.
Gobler, C. J., and Baumann, H. (2016). Hypoxia and acidification in ocean ecosystems: coupled dynamics and effects on marine life. Biol. Lett. 12:20150976. doi: 10.1098/rsbl.2015.0976
Gobler, C. J., DePasquale, E. L., Griffith, A. W., and Baumann, H. (2014). Hypoxia and acidification have additive and synergistic negative effects on the growth, survival, and metamorphosis of early life stage bivalves. PLoS One 9:e83648. doi: 10.1371/journal.pone.0083648
Gobler, C. J., Merlo, L. R., Morell, B. K., and Griffith, A. W. (2018). Temperature, acidification, and food supply interact to negatively affect the growth and survival of the forage fish, Menidia beryllina (inland silverside) and Cyprinodon variegatus (sheepshed minnow). Front. Mar. Sci. 5:86. doi: 10.3389/fmars.2018.00086
Grear, J. S., O’Leary, C. A., Nye, J. A., Tettelbach, S. T., and Gobler, C. J. (2020). Effects of coastal acidification on North Atlantic bivalves: interpreting laboratory responses in the context of in situ populations. Mar. Ecol. Prog. Ser. 633, 89–104. doi: 10.3354/meps13140
Gruber, N. (2011). Warming up, turning sour, losing breath: ocean biogeochemistry under global change. Philos. Trans. R. Soc. A 369, 1980–1996. doi: 10.1098/rsta.2011.0003
Hendriks, I. E., Duarte, C. M., and Álvarez, M. (2010). Vulnerability of marine biodiversity to ocean acidification: a meta-analysis. Estuar. Coast. Shelf Sci. 86, 157–164. doi: 10.1016/j.ecss.2009.11.022
Hepburn, C. D., Pritchard, D. W., Cornwall, C. E., McLeod, R. J., Beardall, J., and Raven, J. A. (2011). Diversity of carbon use strategies in a kelp forest community: implications for a high CO2 ocean. Global Change Biology 17, 2488–2497. doi: 10.1111/j.1365-2486.2011.02411.x
Hofmann, L. C., Straub, S., and Bischof, K. (2012). Competition between calcifying and noncalcifying temperate marine macroalgae under elevated CO2 levels. Mar. Ecol. Prog. Ser. 464, 89–105. doi: 10.3354/meps09892
Jackson, A. (2007). “Lacuna vincta Banded chink shell,” in Marine Life Information Network: Biology and Sensitivity Key Information Reviews, eds H. Tyler-Walters, and K. Hiscock, (Plymouth: Marine Biological Association of the United Kingdom), 1–13.
Janiak, D. S., and Whitlatch, R. B. (2012). Epifaunal and algal assemblages associated with the native Chondrus crispus (Stackhouse) and the non-native Grateloupia turuturu (Yamada) in eastern Long Island Sound. J. Exp. Mar. Biol. Ecol. 413, 38–44. doi: 10.1016/j.jembe.2011.11.016
Johnson, C. R., and Mann, K. H. (1986). The importance of plant defence abilities to the structure of subtidal seaweed communities: the kelp Lamanaria longicruris de la Pylaie survives grazing by the snail Lacuna vincta (Montagu) at high population densities. J. Exp. Mar. Biol. Ecol. 97, 231–267. doi: 10.1016/0022-0981(86)90244-3
Kapper, M. A., and Stickle, W. B. (1987). Division of comparative physiology and biochemistry, society for integrative and comparative biology. Physiol. Zool. 60, 159–173. doi: 10.1086/physzool.60.1.30158637
Keeling, R. F., Körtzinger, A., and Gruber, N. (2010). Ocean deoxygenation in a warming world. Ann. Rev. Mar. Sci. 2, 199–229. doi: 10.1146/annurev.marine.010908.163855
Kim, T. W., Barry, J. P., and Micheli, F. (2013). The effects of intermittent exposure to low-pH and low-oxygen conditions on survival and growth of juvenile red abalone. Biogeosciences 10, 7255–7262. doi: 10.5194/bg-10-7255-2013
Krumhansl, K. A., and Scheibling, R. E. (2011). Spatial and temporal variation in grazing damage by the gastropod Lacuna vincta in Nova Scotian kelp beds. Aquat. Biol. 13, 163–173. doi: 10.3354/ab00366
Levin, L. A. (2003). Oxygen minimum zone benthos: adapatation and community response to hypoxia. Oceanogr. Mar. Biol. Ann. Rev. 41, 1–45.
Levin, L. A., and Breitburg, D. L. (2015). Linking coasts and seas to address ocean deoxygenation. Nat. Clim. Change 5, 401–403. doi: 10.1038/nclimate2595
Li, A., and Chiu, J. M. Y. (2013). Latent effects of hypoxia on the gastropod Crepidula onyx. Mar. Ecol. Prog. Ser. 480, 145–154. doi: 10.3354/meps10213
Lindinger, M. I., Lauren, D. J., and McDonald, D. G. (1984). Acid-base balance in the sea mussel, Mytilus edulis. III. Effects of environmental hypercapnia on intra- and extracellular acid-base balance. Mar. Biol. Lett. 5, 371–381.
Liu, C. C., Shin, P. K. S., and Cheung, S. G. (2014). Comparisons of the metabolic responses of two subtidal nassariid gastropods to hypoxia and re-oxygenation. Mar. Pollut. Bull. 82, 109–116. doi: 10.1016/j.marpolbul.2014.03.013
Liu, D., Keesing, J. K., Xing, Q., and Shi, P. (2009). World’s largest macroalgal bloom caused by expansion of seaweed aquaculture in China. Mar. Pollut. Bull. 58, 888–895. doi: 10.1016/j.marpolbul.2009.01.013
Manno, C., Morata, N., and Primicerio, R. (2012). Limacina retroversa’s response to combined effects of ocean acidification and sea water freshening. Estuar. Coast. Shelf Sci. 113, 163–171. doi: 10.1016/j.ecss.2012.07.019
Marchant, H. K., Calosi, P., and Spicer, J. I. (2010). Short-term exposure to hypercapnia does not compromise feeding, acid-base balance or respiration of Patella vulgata but surprisingly is accompanied by radula damage. J. Mar. Biol. Assoc. U.K. 90, 1379–1384. doi: 10.1017/S0025315410000457
Martel, A., and Chia, F. S. (1991). Oviposition, larval abundance, in situ larval growth, and recruitment of the herbivore gastropod Lacuna vincta in kelp canopies in Barkely Sound, Vancouver Island (Britain Columbia). Mar. Biol. 110, 237–247. doi: 10.1007/BF01313709
McMahon, R. F., and Russell-Hunter, W. D. (1978). Respiratory responses to low oxygen stress in marine littoral and sublittoral snails. Physiol. Zool. 51, 408–424. doi: 10.1086/physzool.51.4.30160965
Melatunan, S., Calosi, P., Rundle, S. D., Moody, A. J., and Widdicombe, S. (2011). Exposure to elevated temperature and pCO2 reduces respiration rate and energy status in the periwinkle Littorina littorea. Physiol. Biochem. Zool. 84, 583–594. doi: 10.1086/662680
Melzner, F., Jörn, T., Koeve, W., Oschlies, A., Gutowska, M. A., Bange, H. W., et al. (2013). Future ocean acidification will be amplified by hypoxia in coastal habitats. Mar. Biol. 160, 1875–1888. doi: 10.1007/s00227-012-1954-1
Melzner, F., Stange, P., Trübenbach, K., Thomsen, J., Casties, I., Panknin, U., et al. (2011). Food supply and seawater pCO2 impact calcification and internal shell dissolution in the blue mussel Mytilus edulis. PLoS One 6:e24223. doi: 10.1371/journal.pone.0024223
Miller, D., Poucher, S., and Coiro, L. (2002). Determination of lethal dissolved oxygen levels for selected marine and estuarine fishes, crustaceans, and a bivalve. Mar. Biol. 140, 287–296. doi: 10.1007/s002270100702
Millero, F. J. (2010). History of the equation of state of seawater. Oceanography 23, 18–33. doi: 10.5670/oceanog.2010.21
Molis, M., Enge, A., and Karsten, U. (2010). Grazing impact of, and indirect interactions between mesograzers associated with kelp (Laminaria digitata). J. Phycol. 46, 76–84. doi: 10.1111/j.1529-8817.2009.00787.x
Morrell, B. K., and Gobler, C. J. (2020). Negative effects of diurnal changes in acidification and hypoxia on early-life stage estuarine fishes. Diversity 12, 25. doi: 10.3390/d12010025
Nelson, T. A., Haberlin, K., Nelson, A. V., Ribarich, H., Hotchkiss, R., Van Alstyne, K. L., et al. (2008). Ecological and physiological controls of species composition in green macroalgal blooms. Ecology 89, 1287–1298. doi: 10.1890/07-0494.1
Ober, G. T., and Thornber, C. S. (2017). Divergent responses in growth and nutritional quality of coastal macroalgae to the combination of increased pCO2 and nutrients. Mar. Environ. Res. 131, 69–79. doi: 10.1016/j.marenvres.2017.09.003
O’Boyle, S., McDermott, G., Noklegaard, T., and Wilkes, R. (2013). A simple index of trophic status in estuaries and coastal bays based on measurements of pH and dissolved oxygen. Estuar. Coast. 36, 158–173. doi: 10.1007/s12237-012-9553-4
Olischläger, M., Bartsch, I., Gutow, L., and Wiencke, C. (2013). Effects of ocean acidification on growth and physiology of Ulva lactuca (Chlorophyta) in a rockpool-scenario. Phycol. Res. 61, 180–190. doi: 10.1111/pre.12006
Pansch, C., Schaub, I., Havenhand, J., and Wahl, M. (2014). Habitat traits and food availability determine the response of marine invertebrates to ocean acidification. Glob. Change Biol. 20, 765–777. doi: 10.1111/gcb.12478
Pechenik, J. A., Jarrett, J. N., and Rooney, J. (2002). Relationships between larval nutritional experience, larval growth rates, juvenile growth rates, and juvenile feeding rates in the prosobranch gastropod Crepidula fornicata. J. Exp. Mar. Biol. Ecol. 280, 63–78. doi: 10.1016/S0022-0981(02)00367-2
Pörtner, H.-O., Reipschläger, A., and Heisler, N. (1998). Acid-base regulation, metabolism and energetics in Sipunculus nudus as a function of ambient carbon dioxide level. J. Exp. Biol. 201, 43–55.
Ringwood, A. H., and Keppler, C. J. (2002). Water quality variation and clam growth: is pH really a non-issue in estuaries. Estuaries 25, 901–907. doi: 10.1007/BF02691338
Saderne, V. (2012). The Ecological Effect of CO2 on the Brown Algae Fucus Serratus and its Epibionts: From the Habitat to the Organismic Scale. Kiel: Christian Albrecht’s University of Kiel. Ph.D Dissertation.
Seibel, B. A., and Walsh, P. J. (2003). Biological impacts of deep-sea carbon dioxide injection inferred from indices of physiological performance. J. Exp. Biol. 206, 641–650. doi: 10.1242/jeb.00141
Shacklock, P. F., and Croft, G. B. (1981). Effect of grazers on Chondrus crispus in culture. Aquaculture 22, 331–342. doi: 10.1016/0044-8486(81)90159-9
Shirayama, Y., and Thorton, H. (2005). Effect of increased atmospheric CO2 on shallow water marine benthos. J. Geophys. Res. 110:C09S08. doi: 10.1029/2004JC002618
Smetacek, V., and Zingone, A. (2013). Green and golden seaweed tides on the rise. Nature 504, 84–88. doi: 10.1038/nature12860
Solomon, S., Plattner, G.-K., Knutti, R., and Friedlingstein, P. (2009). Irreversible climate change due to carbon dioxide emissions. Proc. Natl. Acad. Sci. U.S.A. 106, 1704–1709. doi: 10.1073/pnas.0812721106
Southgate, T. (1982). A comparative study of Lacuna vincta and Lacuna pallidula (Gastropoda: Prosobranchia) in littoral algal turfs. J. Mollus. Stud. 48, 302–309. doi: 10.1093/oxfordjournals.mollus.a065652
Stramma, L., Johnson, G. C., Sprintall, J., and Mohrholz, V. (2008). Expanding oxygen-minimum zones in the tropical oceans. Science 320, 655–658. doi: 10.1126/science.1153847
Talmage, S. C., and Gobler, C. J. (2010). Effects of past, present, and future ocean carbon dioxide concentrations on the growth and survival of larval shellfish. Proc. Natl. Acad. Sci. U.S.A. 107, 17246–17251. doi: 10.1073/pnas.0913804107
Thomsen, J., Casties, I., Pansch, C., Körtzinger, A., and Melzner, F. (2013). Food availability outweighs ocean acidification effects in juvenile Mytilus edulis: laboratory and field experiments. Glob. Change Biol. 19, 1017–1027. doi: 10.1111/gcb.12109
Tripp-Valdez, M. A., Bock, C., Lucassen, M., Lluch-Cota, S. E., Sicard, M. T., Lannig, G., et al. (2017). Metabolic response and thermal tolerance of green abalone juveniles (Haliotis fulgens: Gastropoda) under acute hypoxia and hypercapnia. J. Exp. Mar. Biol. Ecol. 497, 11–18. doi: 10.1016/j.jembe.2017.09.002
Valiela, I., and Cole, M. L. (2002). Comparative evidence that salt marshes and mangroves may protect seagrass meadows from land-derived nitrogen loads. Ecosystems 5, 92–102. doi: 10.1007/s10021-001-0058-4
Valiela, I., Foreman, K., LaMontagne, M., Hersh, D., Costa, J., Peckol, P., et al. (1992). Couplings of watersheds and coastal waters: Sources and consequences of nutrient enrichment in Waquoit Bay, Massachusetts. Estuaries 15, 443–457. doi: 10.2307/1352389
Valiela, I., McClelland, J., Hauxwell, J., Behr, P. J., Hersh, D., and Foreman, K. (1997). Macroalgal blooms in shallow estuaries: controls and ecophysiological and ecosystem consequences. Limnol. Oceanogr. 42, 1105–1118. doi: 10.4319/lo.1997.42.5_part_2.1105
Vaquer-Sunyer, R., and Duarte, C. M. (2008). Thresholds of hypoxia for marine biodiversity. Proc. Natl. Acad. Sci. U.S.A. 105, 15452–15457. doi: 10.1073/pnas.0803833105
Waldbusser, G. G., and Salisbury, J. E. (2014). Ocean acidification in the coastal zone from an organism’s perspective: multiple system parameters, frequency domains, and habitats. Ann. Rev. Mar. Sci. 6, 221–247. doi: 10.1146/annurev-marine-121211-172238
Wallace, R. B. (2020). Coastal ocean acidification: Dynamics and potential to affect marine mollusks. Stony Brook, NY: Stony Brook University. Ph.D Dissertation.
Wallace, R. B., Baumann, H., Grear, J. S., Aller, R. C., and Gobler, C. J. (2014). Coastal ocean acidification: the other eutrophication problem. Estuar. Coast. Shelf Sci. 148, 1–13. doi: 10.1016/j.ecss.2014.05.027
Wallace, R. B., and Gobler, C. J. (2015). Factors controlling blooms of microalgae and macroalgae (Ulva rigida) in a eutrophic, urban estuary: Jamaica Bay, NY, USA. Estuar. Coast. 38, 519–533. doi: 10.1007/s12237-014-9818-1
Wootton, J. T., Pfister, C. A., and Forester, J. D. (2008). Dynamic patterns and ecological impacts of declining ocean pH in a high-resolution multi-year dataset. Proc. Natl. Acad. Sci. U.S.A. 105, 18848–18853. doi: 10.1073/pnas.0810079105
Wu, R. S. S. (2002). Hypoxia: from molecular responses to ecosystem responses. Mar. Pollut. Bull. 45, 35–45. doi: 10.1016/S0025-326X(02)00061-9
Xu, D., Schaum, C.-E., Lin, F., Sun, K., Munroe, J. R., Zhang, X. W., et al. (2016). Acclimation of bloom-forming and perennial seaweeds to elevated pCO2 conserved across levels of environmental complexity. Glob. Change Biol. 23, 4828–4839. doi: 10.1111/gcb.13701
Xu, Z., Gao, G., Xu, J., and Wu, H. (2017). Physiological response of a golden tide alga (Sargassum muticum) to the interaction of ocean acidification and phosphorus enrichment. Biogeosciences 14, 671–681. doi: 10.5194/bg-14-671-2017
Young, C. S., and Gobler, C. J. (2016). Ocean acidification accelerates the growth of two bloom-forming macroalgae. PLoS One 11:e0155152. doi: 10.1371/journal.pone.0155152
Young, C. S., and Gobler, C. J. (2018). The ability of macroalgae to mitigate the negative effects of ocean acidification on four species of North Atlantic bivalve. Biogeosciences 15, 6167–6183.
Young, C. S., Lowell, A., Peterson, B. J., and Gobler, C. J. (2019). Ocean acidification and food limitation combine to suppress herbivory by the gastropod Lacuna vincta. Mar. Ecol. Prog. Ser. 627, 83–94. doi: 10.3354/meps13087
Zamprogno, G. C., Costa, M. B., Barbiero, D. C., Ferreira, B. S., and Souza, F. T. V. M. (2013). Gastropod communities associated with Ulva spp. in the littoral zone in southeast Brazil. Lat. Am. J. Aquat. Res. 41, 968–978.
Keywords: hypoxia, ocean acidification, gastropods, macroalgae, grazing
Citation: Young CS and Gobler CJ (2020) Hypoxia and Acidification, Individually and in Combination, Disrupt Herbivory and Reduce Survivorship of the Gastropod, Lacuna vincta. Front. Mar. Sci. 7:547276. doi: 10.3389/fmars.2020.547276
Received: 31 March 2020; Accepted: 28 August 2020;
Published: 17 September 2020.
Edited by:
Ben P. Harvey, University of Tsukuba, JapanReviewed by:
Jason Michael Hall-Spencer, University of Plymouth, United KingdomCopyright © 2020 Young and Gobler. This is an open-access article distributed under the terms of the Creative Commons Attribution License (CC BY). The use, distribution or reproduction in other forums is permitted, provided the original author(s) and the copyright owner(s) are credited and that the original publication in this journal is cited, in accordance with accepted academic practice. No use, distribution or reproduction is permitted which does not comply with these terms.
*Correspondence: Christopher J. Gobler, Q2hyaXN0b3BoZXIuZ29ibGVyQHN0b255YnJvb2suZWR1
Disclaimer: All claims expressed in this article are solely those of the authors and do not necessarily represent those of their affiliated organizations, or those of the publisher, the editors and the reviewers. Any product that may be evaluated in this article or claim that may be made by its manufacturer is not guaranteed or endorsed by the publisher.
Research integrity at Frontiers
Learn more about the work of our research integrity team to safeguard the quality of each article we publish.