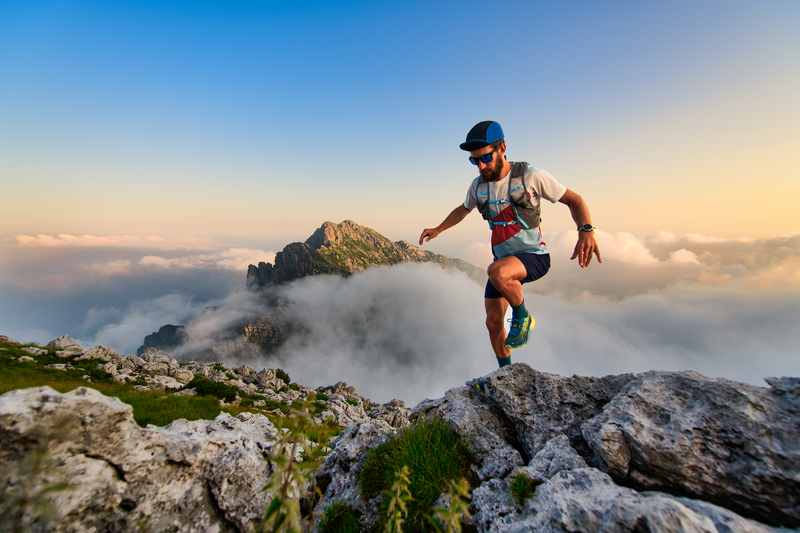
94% of researchers rate our articles as excellent or good
Learn more about the work of our research integrity team to safeguard the quality of each article we publish.
Find out more
ORIGINAL RESEARCH article
Front. Mar. Sci. , 28 August 2020
Sec. Marine Megafauna
Volume 7 - 2020 | https://doi.org/10.3389/fmars.2020.00725
Reef manta rays (Mobula alfredi) are capable of long-distance dispersal when habitat is continuous. In the Ningaloo Reef World Heritage Area located on Australia’s mid-west coast, M. alfredi is sighted year-round and is a focal species for ecotourism in the region. Despite value to local tourism and demographic vulnerability to exploitation, basic information regarding M. alfredi movements and area use in this region is lacking. Here we used satellite tags and a long-term photographic-identification database collected by researchers and citizen scientists to examine the species’ visitation patterns and regional space use. Tagged M. alfredi remained in coastal shelf waters, preferentially occupying shallow depths < 20 m (76% of depth observations). Comparison of real tracks against those produced by correlated random walk simulations revealed directional dispersal and connectivity between the Ningaloo and Shark Bay World Heritage Areas. The greatest linear distance an individual moved after tagging was ∼700 km. This movement range is comparable to seasonal M. alfredi migration observed at similar latitudes on Australia’s east coast. Photographic-identification of 1121 individual M. alfredi, from 5146 sighting events, revealed that some individuals exhibited long-term site affinity to locations within Ningaloo Marine Park, with the longest spanning 15.16 years (9.8% individuals > 10 years). Lagged Identification Rate analysis suggested site visitation was best described by a pattern of emigration and reimmigration. While our observations of movement and residency establishes basic information previously unknown for the species in this region, the overlap of core area use with existing UNESCO World Heritage Areas demonstrates the potential for well-positioned marine parks to provide protection of critical habitat for M. alfredi.
Marine protected areas (MPAs) are increasingly established around the world to protect biodiversity, but the degree to which they can help conserve mobile species remains contentious. Highly mobile and widely ranging species pose a considerable challenge for spatial management as protection across ranges of hundreds to thousands of kilometres is often impractical, while protecting too small an area or failing to protect key habitats may be ineffectual (see Dunn et al., 2019; Dwyer et al., 2020). To assess the effectiveness of spatial management or habitat protection, it is necessary to have a good understanding of residency, habitat use and movement scale (Cooke, 2008). Simple numbered tags have historically been attached to a range of taxa, such as fish, birds and turtles, with recapture of marked individuals giving some indication of movements (e.g., Wood, 1945; Kohler and Turner, 2001). Where individuals are uniquely identifiable by natural physical markings that do not change over the period of interest, such features may serve as a ‘visual tag’ in place of a physical tag. However, it should be noted that these approaches are biased by heterogenous effort to capture or re-sight individuals in different locations, and provide limited insight on movements in-between capture and re-capture occasions. For many widely ranging species, particularly those in the marine environment, aquatic telemetry can facilitate the acquisition of spatial information without the limitation of relying on re-capture (Hussey et al., 2015).
While satellite tracking of tagged animals provides new insights into movements and habitat use, the integration and application of such data to conservation and management remains underutilised (Hays et al., 2016). Recent studies have showcased examples where movement data has informed aquatic conservation and management issues (Hays et al., 2019), including species stock boundaries and structure (Neilson et al., 2014), fishing mortality estimates (Byrne et al., 2017), identification of illegal and non-declared fishing vessels (Weimerskirch et al., 2020), and species behaviour and area utilisation (Schofield et al., 2013; Queiroz et al., 2016; Daly et al., 2018). Knowledge of species movements thus has the potential to provide valuable supporting information for the effective management of marine species.
Ningaloo Reef on Australia’s remote mid-west coast is one of the longest fringing reefs in the world, encompassed by multiple-use State and Commonwealth MPAs and in 2011 was designated a UNESCO World Heritage Area (WHA). The waters of the Ningaloo WHA are a productivity hotspot along the west Australian coastline, with seasonal upwelling and locally elevated productivity supporting one of the few predictable aggregations of whale sharks (Rhincodon typus) in the world (Wilson et al., 2002; Hanson et al., 2005; Norman et al., 2016; Xu et al., 2016). The region attracts tourists who participate in recreational fishing and swim-with-megafauna experiences, specifically with R. typus, humpback whales (Megaptera novaeangliae) and reef manta rays (Mobula alfredi). Tourism activity in the area is increasing, with in-water R. typus interactions rising by 370% over just four years (Anderson et al., 2014), and a steady increase in activity surrounding M. alfredi since the 1990s (Venables et al., 2016). While studies on space use, tourism impacts and species-specific tourism management plans exist for R. typus and M. novaeangliae (Sanzogni et al., 2015; Norman et al., 2016; Norman et al., 2017; Lester et al., 2019; Sprogis et al., 2020), such local information or management and monitoring plans for M. alfredi are yet to be established (Venables et al., 2016).
In Australia, M. alfredi is coastally distributed to ∼30°S on the east and west coasts, with a continuous northerly distribution between coastlines (Armstrong et al., 2020, Couturier et al., 2012). Although capable of long-distance movements of at least 1100 km (Armstrong et al., 2019), records for M. alfredi suggest they typically traverse shorter distances and display strong site affinity (Clark, 2010; Couturier et al., 2011; Germanov and Marshall, 2014; Germanov et al., 2019; Jaine et al., 2014; Peel, 2019; Venables et al., 2020). With an exceptionally low maximum population growth rate (Dulvy et al., 2014), M. alfredi is particularly susceptible to human impacts and is classified as “Vulnerable” on the International Union for the Conservation of Nature Red List of Threatened Species. Although the World Heritage Area and Marine Park status of select areas along the mid-west coast likely reduces threats to fauna and flora, such constructs cannot entirely eliminate the influence of human activity. For example, recent studies conducted in the Ningaloo Reef WHA have reported short-term behavioural changes in M. alfredi in up to a third of tourism interactions (Venables et al., 2016), as well as a possible increase in vessel strike (McGregor et al., 2019). The lack of information on M. alfredi area use and spatial connectivity in the region hinders the guidance and evaluation of future management approaches for threat identification and reduction (Stewart et al., 2018).
To better understand patterns of movement and site use by M. alfredi along the remote and productive west Australian coastline we use satellite telemetry alongside a decade of photographic identification records. The combination of approaches allows us to specifically investigate: (1) whether M. alfredi movements extend outside of the Ningaloo WHA, offshore or to other regions along the coast and if so, the similarity to east coast movement trends; (2) trends in sighting peaks at monitored aggregation sites; (3) demographic parameters such as site affinity and residency.
We tagged M. alfredi (n = 22) with miniature pop-up archival (hereafter miniPAT) satellite tags (Wildlife Computers, Seattle WA) at sites along the West Australian coastline (Figure 1). Tag deployments in or adjacent to the Ningaloo Marine Park were timed to coincide with reported sighting peaks during May 2016 in Coral Bay (n = 10 deployments; 23.10°S, 113.94°E) and September 2016 for the Exmouth Gulf (n = 10 deployments; 21.93°S, 114.14°E). Two additional tags were deployed opportunistically near Steep Point, Shark Bay (25.56°S, 113.02°E) in March 2019. Tags were attached to free-swimming M. alfredi with a modified Hawaiian sling used to insert a nylon Domeier dart or titanium dart (for 2016 and 2019 deployments, respectively) anchor into the pectoral fin base on the dorsum of the animal. At the time of tagging, a ventral photograph of the ray was taken for individual identification (Photo ID). Tag tethers consisted of 15 cm braided stainless-steel wire looped through the anchor and crimped in position beneath a heat shrink coating. Tags were programmed to remain attached for a maximum of 120 or 180 days for 2016 and 2019 deployments respectively, with conditional release programmed to occur if remaining at a constant depth (larger than the local tidal range) for > 24-hours (indicative of mortality or premature tag shedding). Tags that were physically recovered contained temperature, depth and light level observations every 3 seconds, whilst data from non-recovered transmitting tags were summarised into 450-second windows and recovered via the Advanced Research and Global Observation Satellite (ARGOS) network. We examined depth data for tags for which light-based geolocation was possible using the R package ‘Rchivaltag’ (Bauer, 2018).
Figure 1. The Ningaloo and Shark Bay World Heritage areas (inset) of Australia’s western coastline (outset) span vast regions of largely uninhabited coastline.
As miniPAT tags do not broadcast or log locations throughout their deployment, tag locations are estimated post-deployment using tag-based observations of light and temperature. Twelve hourly maximum likelihood location were calculated using the manufacturers’ proprietary hidden Markov model as implemented in Wildlife Computers Global Position Estimator 3. Briefly, this approach considers a 0.25° gridded probability surface with location estimates informed by initial deployment location, pop-up location, dawn and dusk light-level curves, sea surface temperature, and bathymetry. Output from the model includes a maximum likelihood location (point location) as well the 50%, 95% and 99% likelihood areas. To confirm accurate deployment end dates, we examined temperature, depth and light-level data, with tag pop-off confirmed as the last point prior to consistent observations of a floating tag. No spatial end points were assigned to tags with a first ARGOS location fix > 24 h post detachment. For dawn and dusk light-level observations, we removed duplicate events (i.e., two dawns or dusks for a given date) and removed events that featured an entirely flat light-level curve (i.e., due to sustained dives during crepuscular periods). For tagged individuals that were re-sighted during the tag deployment period (photo-ID via visual matching), we included the fine scale sighting location and time as a known tag location into the state-space movement models, and compared these to tracks without the re-sighting observations. Diffusion speed through the gridded probability space is constrained by a maximum daily movement value representing animal speed. Active tracking observations in Hawaii show M. alfredi movement reached speeds of 1.7 m.s–1 (Clark, 2010), while the maximum estimated velocity of tracked individuals through an acoustic array in the Ningaloo Marine Park reached only 1.2 m.s–1 (FM unpublished data). In the interest of not artificially constraining modelled movements, we set the diffusion value for all tracks to a midpoint of these estimates, 1.5m.s–1, but reran the analysis for each track with multiple velocity scenarios to ensure stability of the maximum likelihood track. Core area use was calculated from the maximum likelihood tag tracks using kernel utilisation density analyses as implemented in the R package ‘adehabitatHR’ (Calenge, 2006). Core area use and maximum likelihood tracks were visualised using the R package ‘marmap’ (Pante and Simon-Bouhet, 2013).
We tested for habitat selectivity by comparing the observed proportion of time that the observed tracks occupied three predefined regions, with the expected occupation time derived from simulated random walks for each tag. We divided the coastline into three regions based on the location of the Ningaloo Reef WHA, with regions classified as north, south or within the latitudinal bounds of the WHA. We based coastline division off latitude only, as the positional error associated with light-based geolocation precludes confident assignment to fine-scale locations. We simulated 1000 random tracks that mirrored deployment durations for observed tags, using a similar method to analyses for other planktivorous elasmobranchs (Sims et al., 2006; Rohner et al., 2018, Jaine et al., 2014). Simulated tracks sourced step lengths from the associated observed tag track by randomly sampling observed step lengths without replacement and then assigning a random movement angle (360° range) to each step. Step lengths were determined as the distance between 12 hourly interpolated locations. To more accurately represent M. alfredi movement behaviour, we constrained the model by depth using a raster layer with the ‘raster’ R package (Hijmans, 2019) generated from ∼1 km resolution bathymetric data. Bathymetric data were sourced from the NOAA ETOPO1 dataset using the R package ‘marmap’ (Pante and Simon-Bouhet, 2013). The first depth constraint in the raster layer prevented positions from being either on-land or crossing land, while the second depth constraint limited the available movement space to locations where maximum depth was < 500 m, akin to true movements observed in other M. alfredi tracking (Jaine et al., 2014). We considered that manta rays were being selective in their habitat use if observed mantas either spent significantly more time in an area than random manta tracks (i.e., > 975 out of 1000 modelled mantas) or spent significantly less time in an area than random manta tracks (i.e., < 25 out of 1000 modelled mantas). Note that this is a two-tailed test because we did not know a priori whether manta rays would prefer or avoid areas. Core area use was also calculated for simulated tracks for comparison with area use derived from observed tracks.
To investigate drivers of movement, we extracted sea-surface temperature (SST) and an index of phytoplankton biomass (chlorophyll-a; Chl-a) values for all positions from random model tracks and observed tracks. As in previous studies investigating movement in planktivorous marine megafauna (Sims et al., 2003; Sleeman et al., 2007, Graham et al., 2012; Rohner et al., 2018), we used Chl-a data as a proxy for zooplankton abundance. Time-series of Chl-a from the Visible and Infrared Imager/Radiometer Suite onboard the Suomi-NPP satellite were accessed via the NOAA ERDDAP server and contained weekly composite observations at ∼4 km resolution. As excessive cloud cover in the region of interest throughout the tag deployments rendered daily Chl-a data products unsuitable for use, weekly composite observations were used. Track positions were assigned a Chl-a observation based on the maximum likelihood location as well as through matching simulated and observed track points to the nearest associated time point in the satellite product. Temperature time-series produced by the Australian Bureau of Meteorology from the Advanced Very High-Resolution Radiometer onboard the NOAA-19 satellite were accessed via the Australian Ocean Data network1 and contained daily (night-time) observations at ∼2 km spatial resolution.
Ventral identification photographs of M. alfredi individuals sighted on Ningaloo Reef have been catalogued for over a decade in a photo-ID image database along with metadata such as date and location of sighting (McGregor et al., 2019). In Coral Bay, identification photos have been collected primarily by tourism operators who run in-water megafauna interaction tours year-round (2002-2018), with image cataloguing and collection efforts beginning in earnest during 2006. Photographs from Exmouth-based tourism operators are associated with the peak tourism season (April – September), while citizen-scientist observations from private vessels occur year-round in the Exmouth Gulf.
The unique patterning on the ventral surface of M. alfredi facilitates the generation of catalogues recording sightings and re-sightings of individuals through space and time (Marshall et al., 2009; Marshall and Pierce, 2012). Identifications based on images that clearly showed the ventral surface of the individual were matched by eye with the assistance of hierarchical filtering software (‘MantaUtil’, Winstanley, 2016). Sightings were either matched to an existing identification, or if no match was found the image was added to the catalogue as a new identification; all sightings were checked by trained observers. The sex of each individual was determined through the presence (male) or absence (female) of claspers. The behaviour displayed by each individual when sighted was categorised (as in Germanov et al., 2019), and was informed by ancillary observations reported by tourism operators or trained observers, or through indications of behaviour evident in the photographs. Sightings of Mobula birostris at Ningaloo Reef are relatively infrequent (Armstrong et al., 2020) and as such the species was excluded from this study.
Although resightings of M. alfredi individuals may be separated by long periods of time, information on whether individuals visit the same or different locations can provide insight into spatial connectivity and residency. Therefore, for individuals with more than one sighting, we extracted the date and location details of each encounter for database entries up to the end of 2018. To examine trends in sightings across locations, we tallied the number of unique identifications per month, per site. To examine site use, we grouped encounters from the four primary behavioural classifications and examined the proportions of each activity observed across all sighting records, as well as activity type by location.
To provide an indication of temporal area use, we used a lagged identification rate (LIR) analysis as implemented in the software SOCPROG 2.9 (Whitehead, 2009). For this analysis we used identifications from Coral Bay only, as survey effort at this site is year-round and not tied to a fixed tourism season as occurs at Exmouth. The LIR approach allows an estimate of residency by calculating the probability of re-sighting an individual in the study area following a given time lag (Whitehead, 2001). The observed LIR can be compared to exponential mathematical models that describe various patterns of movement including: no movement; emigration and mortality; emigration and re-immigration, and; the latter with mortality. The quasi-Akaike information criterion (QAIC) values are used to determine the model of best-fit (Burnham and Anderson, 2002). The rate at which the LIR declines and the shape of the curve through time describes how quickly animals leave the study site and whether or not they remain resident or later re-immigrate.
Twelve of the twenty-two tags deployed between 2016–2019 reported enough observations for light-based geolocation with their deployments spanning 12 to 112 days (mean = 62.6 days, SD = 31.9 days; Table 1). Deployments where light-based geolocation was not possible involved premature tag shedding (< 4 days duration; n = 2), non-reporting tags (n = 3) and receipt of insufficient messages (n = 5). Tag pop-off locations were available for 17 of 22 tags, and within 24 hours of detachment for 12 of 22 tags (Table 1). The greatest duration between suspected tag detachment and first ARGOS location was ∼2 weeks (Tag 146004), where time-series observations indicate a period of likely tag ingestion. Tag ingestion is suspected from the time at which the observed light-level decreased to constant darkness, and the temperature observations for the suspected ingestion period became relatively constant despite depth variance, and depth observations did not fit with the pattern of previous diving activity (Supplementary Figure S1). Four tagged individuals were re-sighted through photo-ID during tag deployment, with inclusion of resightings as known locations for three geolocation models (Table 1). For the two tracks with sufficient deployment duration and a mid-deployment photographic recapture, comparison between tracks modelled with and without re-sighting observations differed in their maximum likelihood locations surrounding the re-sighting period, with the general track trajectory remaining similar (Supplementary Figures S2, S3). We observed little change to maximum likelihood locations or model scores when running light-based geolocation models across the range of diffusion parameters (2 m.s–1 to 1 m.s–1). Of the 22 M. alfredi fitted with miniPAT tags, 10 have subsequently been re-sighted with no reported tether retention, signs of dorsal damage or any other apparent ill-effects.
Table 1. Metadata for 22 pop-up satellite archival tags deployed on M. alfredi in Western Australia between May 2016 and March 2019.
Maximum likelihood locations indicating tagged M. alfredi remained predominantly on the shelf (Figures 2, 3), are supported by time-series observations, where 93.9% of depth records are < 50 m (Figure 4). We observed considerable southerly latitudinal displacement in 8 of 12 tag tracks, with only one tag track moving outside the northern latitudinal bounds of the Ningaloo WHA, before moving southwards along the coast (Supplementary Figures S4, S15). The maximum displacement (great circle distance) between tag deployment locations and the farthest point in the light-based geolocation tracks was 483 km (mean = 261.9 km; Figures 2, 3). Although recovered light-level observations were too sparse throughout the deployment to support confident geolocation of the single transmitting 2019 deployment, recovered depth time series in combination with light observations confirm the tag remained attached for the full deployment duration. Therefore, the distance between initial deployment location and final pop-up location was ∼700 km (Table 1). The greatest track length (based on the cumulative sum of each step length) was 1817 km across 98 days (18.5 km.day–1; 0.77 km.hr–1) completed by individual #1024 (PTT 66705). The mean track length for all tags was 839 km (range = 118–1817 km), with a mean daily distance of 12.3 km.day–1 (range = 6.9–18.6 km.day–1; 0.3-0.77 km.hr–1). The core area use estimated by kernel density analysis of M. alfredi tag tracks showed the main activity hotspot centered in the Shark Bay WHA, with weaker hotspots extending northwards to Coral Bay in the Ningaloo WHA (Figure 5). While core area use differed by tag deployment period, with October deployments featuring a southerly shift compared to May/June deployments, the 95% kernel utilisation density areas remained similarly shelf associated (Figure S16).
Figure 2. Maximum likelihood tracks for M. alfredi (n = 6) tagged in Coral Bay, Western Australia during May/June 2016. Track points represent 12-hourly maximum likelihood locations from light-based geolocation analysis implemented in the Wildlife Computers Global Position Estimator (v3), and consecutive observations are connected by the shortest straight-line distance.
Figure 3. Maximum likelihood tracks for M. alfredi (n = 6) tagged in the Exmouth Gulf, Western Australia during October 2016. Track points represent 12-hourly maximum likelihood locations from light-based geolocation analysis implemented in the Wildlife Computers Global Position Estimator (v3), and consecutive observations are connected by the shortest straight-line distance.
Figure 4. Depth occupation frequency grouped by deployment period for Mobula alfredi (n = 12) tagged off the mid-west Australian coast. Depth observations are from recovered PSAT time-series and recovered archival tag data.
Figure 5. Core area use for all tag observations (left) and tag simulations (right) represented by the ⚫ 25%, ⚫ 50%, ⚫ 75% and ⚫ 95% kernel utilisation density estimates.
All tagged individuals showed a preference for occupying shallow depths, with 76.7% (SD = 14.4%) and 94.4% (SD = 6.4%) of observed depths < 20 m and < 50 m, respectively. Although M. alfredi occupied shallow depths much of the time, we observed infrequent deeper dives that differed between tag deployment periods with tags deployed in May/June recording significantly greater depths (Mean = 177 m, Range = 144–269 m) than those deployed in October (Mean = 62.9 m, Range = 24–118 m); t-test P < 0.01).
Observed M. alfredi spent significantly more time south of the Ningaloo WHA, and significantly less time in latitudes north and encompassing the Ningaloo WHA than compared to modelled tracks (Table 2, example: Figures 6, 7). Significant mismatches between simulated and observed area occupation occurred for 6 of 8 tags recorded in latitudes south of the Ningaloo WHA (i.e., < 50 of 1000 simulations spend the same or more time in the southern region). When comparing the proportion of the southerly area occupation for all 8 tags recorded south of Ningaloo WHA with simulations, just 152 of 8000 simulations occupied the area for the same or more time than observed, implying that the overall observed trend is unlikely due to chance alone (Proportion test, p < 0.001). There were also significant mismatches between simulated and observed northerly occupation for the October deployment, where 5 of 6 M. alfredi occupied northern latitudes for significantly less time than simulations (i.e., < 50 of 1000 simulations spend the same or less time in the northern region, P < 0.05).
Table 2. Time spent south of the Ningaloo WHA for tagged M. alfredi (observed) compared to simulated tracks, including the number of tracks that spent more or less time than observed and the associated p-value.
Figure 6. Example of simulated tracks (left panel; n = 1000) based on Tag 165338 deployment duration vs modelled track (blue) and (right panel) the proportion of time (grey bars) and mean time (orange line) simulated tracks occupy latitudes to the north of Ningaloo Marine Park (top), encompassing Ningaloo Marine Park (middle) or south of Ningaloo marine park (bottom) vs. area occupation by the observed track (blue line). Depth contours indicate 500 m (innermost) and 2000 m (outermost), while the open white circle indicates the starting location for track simulations.
Figure 7. Tracks for 6 miniPAT tags deployed in October (A) and the proportion of time (B) simulated tracks (grey bars) and observed tracks (coloured lines) occupy latitudes to the north of Ningaloo Marine Park (top right), encompassing Ningaloo Marine Park (mid right) or south of Ningaloo marine park (bottom right). Map contours indicate 500 m (innermost) and 2000 m (outermost) isobath.
Vertically integrated tag-based temperature observations indicated M. alfredi experienced temperatures between 12.8–27.3°C (Supplementary Figure S17), with a mean temperature of 23.2°C (SD = 1.3°C). The majority of the observations originated from a narrow thermal band, with 50.9% of observations from between 22–23°C, and > 98% of observations between 21–25°C. Vertically integrated temperature observations closely matched satellite derived SST for the observed satellite tags (mean = 23.0°C, SD = 1.2°C) and were not significantly different (t-test p = 0.06), suggesting appropriate thermal habitat can likely be assessed from satellite derived products. Tagged individuals spent significantly more time in cooler waters than modelled tracks (Figure 8) and less time in waters with lower Chl-a concentration than modelled tracks (Figure 9, t-tests p < 0.05).
Figure 8. Sea surface temperature preferences for satellite tracked M. alfredi (white) and modelled tracks (grey).
Figure 9. Chlorophyll-a concentration preferences for satellite tracked M. alfredi (white) and modelled tag tracks (grey).
The Ningaloo photo-ID database contained 5146 sightings of 1121 uniquely identifiable M. alfredi. For the individuals where sex was identifiable, the sex ratio was 1.26 females (n = 583) to males (n = 464), with sex for 6.6% individuals (n = 74) indeterminable from catalogue images. The discovery curve of sightings showed a steady rate of increase in newly identified individuals through time, with no indication of an asymptote (Supplementary Figure S18). Mobula alfredi showed strong site-affinity to the region, with 51% (n = 574) of individuals sighted on more than one occasion. Although most sightings originated from Coral Bay (91%, n = 4697), M. alfredi were also sighted around the Exmouth Gulf (< 4%, n = 202), Muiron Islands (< 0.1%, n = 12), along the Ningaloo Reef (North West Cape, west of Exmouth; n = 213, 4%) and in Shark Bay (< 0.1%, n = 4). The timing of sighting peaks throughout the year varied by site (Table 3).
Table 3. Total number of unique monthly sighting events for M. alfredi between 2005–2018 from Coral Bay, Exmouth (Ningaloo Reef) and the Exmouth Gulf.
For M. alfredi in Coral Bay, the lagged identification rate fell rapidly, reaching a minimum at ∼190 days before increasing again at ∼1 year and levelling off above zero at longer time lags > 12 years (Figure 10). The levelling off of the LIR above zero for time lags > 1 year suggests individuals may either remain resident for long periods of time or re-immigrate years after the initial sighting. Sightings of M. alfredi in Coral Bay were best described by a pattern of emigration and re-immigration as indicated by QAIC support for Model F (Table 4). This movement model indicated that approximately 46 M. alfredi (46.4 ± 8.6, 95% CI 37.6–62.5) were in Coral Bay at any one time, remaining in the area for 56 days (56.7 ± 215.6, 95% CI 40.6–109.7) before leaving for 92 days (92.8 ± 553.4, 95% CI 70.7–148.6) followed by re-immigration at a later time.
Figure 10. Lagged identification rates (blue circles), standard errors (bars), and the expected lagged identification rate (line) from the most parsimonious maximum likelihood model incorporating emigration and re-immigration for M. alfredi in Coral Bay, Western Australia. Numbers beside lagged identification rates represent the mean time lag in days for each estimated point.
Table 4. Movement models and their fit (ΔQAIC) to the Lagged Identification Rate of M. alfredi in Coral Bay, Western Australia.
Individuals recorded at more than one location (26%, n = 151) support tag observations of connectivity within the region (Figure 11). Only two large-scale movements between Ningaloo sites and any other location were captured by photo-ID. Two individuals were recorded (#0965, #0886) moving between the Exmouth Gulf and Steep Point in Shark Bay (∼530 km), with the fastest (#0886) undertaking the northbound journey in a maximum of 111 days at an average velocity of ∼4.7 km.day–1. It should be noted that this journey time is a maximum duration as the individual may have been in the area earlier, but not detected. Database records show repeated sightings of individuals within the Ningaloo region over multiple years, with 19% (n = 110) of individual re-sighting durations spanning > 10 years. The individual with the longest period of site affinity was first photographed in Coral Bay on 9th June 2003 and has been re-sighted in the region 4 more times engaging in feeding and courtship activity, most recently on 8th August 2018 (Sighting span = 15 years 2 months; Manta #0070).
Figure 11. Movements (lines) between locations (points) for M. alfredi in Western Australia, as detected through individual re-sightings from photographic identification. Lines show connections between re-sighting locations and the line colour indicates the number of movements detected. Point size indicates the total number of sighting records for a given location. The dotted and solid black lines to the left of the coastline represent the 500 m and 1000 m depth contours.
Behavioural classification of identification images (Table 5) revealed feeding to be the most frequently observed behaviour (60.5%), followed by cruising (18.8%), cleaning (17.6%) and courtship (4.9%). The proportion of observed behavioural classes varied by site, with up to 94% Exmouth Gulf sightings associated with feeding, with proportionally less feeding observed along Exmouth/Ningaloo Reef sites (79%) and Coral Bay (56%). Although courtship behaviours were rarely captured (4.9% of all sightings), this behaviour was reported from all sites except Shark Bay, for which there were few sightings overall (n = 4).
Table 5. Behavioural observations for M. alfredi from photo-ID records at various locations in Western Australia.
The work presented here demonstrates for the first time that: (1) directional and broad-scale coastally associated movements of M. alfredi along Australia’s western subtropical coastline are comparable to those observed on the east coast; (2) movements connect the Ningaloo Reef and Shark Bay World Heritage areas; (3) M. alfredi have long-lasting site affinity to sites on the west coast; and 4) the proportion of observed behaviours vary by location. Our use of simulated track movements facilitated discrimination between observed movement patterns and those that may occur through chance alone. The difference between observed and simulated tracks suggests that directionality of movement is behaviourally mitigated. Prior to these findings, most information on M. alfredi movements and dynamics in the region was restricted to unpublished records, and heavily focused on a single aggregation site. The directionality of movement identified herein highlights previously unidentified core use areas outside of the Ningaloo WHA. These areas are chiefly located in the northern end of the Shark Bay WHA which is of interest given the spatial overlap with strong oceanographic frontal features. Our use of a long-term photo ID database complemented the short temporal scale of tagging observations, allowing us to further examine patterns of site use, revealing long term site affinity of individuals to the Ningaloo WHA over a 15-year period. Photo ID database sightings suggest a differential seasonal pattern in peak sightings across the survey sites, with peaks occurring in Coral Bay, Ningaloo Reef and the Exmouth Gulf in austral Autumn, Winter and Spring respectively.
Understanding connectivity between locations and populations is crucial for the conservation of migratory species (Dunn et al., 2019). The spatial and temporal dynamics of movements documented herein add to the growing body of literature highlighting connectivity between the Shark Bay and Ningaloo Reef WHA sites. Spatial data detailing marine megafauna movements along the west Australian coastline shows that these two WHA sites feature in the migratory pathways of dusky sharks (Carcharhinus obscurus; Braccini et al., 2018), humpback whales (Megaptera novaeangliae; Jenner et al., 2001), pygmy blue whales (Balaenoptera musculus brevicauda; Double et al., 2014), M. alfredi (this study), tiger sharks (Galeocerdo cuvier; Ferreira et al., 2015) and whale sharks (Rhincodon typus; Norman et al., 2016), with connectivity suggested but not yet formally established for dugong (Dugong dugon) (Gales et al., 2004). These connections highlight an MPA success story, where the two WHAs with their various levels of conservation zoning, provide protection for 27,059 km2 of habitat. World Heritage Area designations seek to protect locations with outstanding natural and cultural heritage, and the listing process encourages local participation as well as the establishment by state parties of management plans and reporting systems (UNESCO). For M. alfredi, WHAs appear to offer protection for habitat and locations that fulfil various needs throughout the life of individuals, as well as encompassing regions of high productivity as seen by observations of feeding behaviour.
The abundance of vulnerable or threatened marine megafauna in these parks may be in part due to bathymetry and currents. For R. typus, locations with upwelling, steep shelf slopes and narrow shelf edges have been identified as key habitats (Copping et al., 2018), and the same is likely for M. alfredi. Both WHAs include or are located near such bathymetric features. The abundance of large megafauna in these regions may be driven by the productivity and abundance of food as illustrated by the proximity of the WHAs to three of the state’s most valuable fisheries; the Shark Bay and Exmouth Gulf prawn fisheries and the Shark Bay scallop fishery (Western Australia Department of Fisheries, 2017). The Exmouth Gulf prawn fishery is adjacent to the Ningaloo WHA, with prawn spawning occurring multiple times throughout the year, but peaking between August-October (White, 1975; Penn and Caputi, 1986). This spawning peak coincides with a short window of time in which large aggregations of feeding M. alfredi are reported in the Exmouth Gulf and around the Muiron Islands. Although the short peak in Exmouth Gulf reef manta ray sightings may be linked to spawning, further work is needed to investigate the composition of prey items and to ensure the peak is not a result of survey effort bias.
The primary core use area identified in our study is located at the northern end of the Shark Bay WHA. This area is oceanographically dynamic and contains persistent frontal features (Nahas et al., 2005). Frontal zones are an ecologically significant ocean feature for a variety of taxa (Wolanski and Hamner, 1988) and are priority conservation areas for mobile marine fauna (see review by Scales et al., 2014). Movements and feeding aggregations of planktivorous elasmobranchs are typically tied to seeking and exploiting better foraging grounds or ephemeral pulses of prey items that are often associated with frontal features (Rohner et al., 2018; Weeks et al., 2015; Anderson et al., 2011; Wilson et al., 2001; Sims et al., 2006). Sighting records from Shark Bay note the presence of multiple manta rays and whale sharks in the same core activity use area identified by our study (Preen et al., 1997; Armstrong et al., 2020), overlapping spatially with areas elevated primary productivity at the northern opening to the bay (Hanson et al., 2005; Nahas et al., 2005). Although M. alfredi likely gains considerable nutrition from deep water plankton (Couturier et al., 2013), the overlap of multiple tag tracks with an oceanographically dynamic but shallow region may be indicative of larger aggregations of M. alfredi at this location. The frequency of feeding behaviours observed within the Ningaloo WHA and surrounding locations for M. alfredi, indicates that productivity exists at sufficient levels to support continuous occupation by mega planktivores, as demonstrated through year-round low-level occupation of the region by R. typus (Norman et al., 2017). It has been suggested that previously established seasonality in R. typus visitation may be a consequence of search effort, and that R. typus may continue to occupy the coastline, albeit with a southerly shifted distribution (Reynolds et al., 2017). Our results suggest that like other pelagic species, M. alfredi may be exploiting persistent oceanic fronts for their dense resource availability. Longer-term and finer spatial resolution monitoring coupled with environmental observations will be necessary to help resolve the underlying drivers of movement and behaviour associated with the core use areas identified by this study. Finer spatial scale monitoring may also assist with identifying likely behaviours predominately associated with areas, especially since we’ve demonstrated that areas with high sightings (e.g., Coral Bay) are used for cleaning and reproductive behaviours in addition to feeding. Although it should be noted that as with other satellite tracking studies, our results will be impacted by tagging site bias, i.e., there will be a bias for high use areas to appear closer to the tagging site. To mitigate this bias future work could increase the tag deployment period and tag individuals in broader array of locations (e.g., Queiroz et al., 2019).
The final pop-up location for the single reporting tag deployed in Shark Bay occurred near Dampier, home to Australia’s second largest shipping port. Dampier is at the southern end of a coastal movement corridor for many migratory marine species and is in close proximity to increasing volumes of shipping traffic (see Pendoley et al., 2014). Although feeding aggregations of M. alfredi have been previously reported in the region (Armstrong et al., 2020), the likely origin of the animals visiting this location had not been established. Ship strike is a threat to many megafaunal species, including M. alfredi, and given the species’ behavioural preference of occupancy of the upper 20 m of the water column (Jaine et al., 2014, Peel, 2019) and the increasing boat traffic in regions adjacent to the Ningaloo WHA (see Bejder et al., 2019), it is perhaps unsurprising that ship strike injuries have come to recent attention. In the Ningaloo Reef WHA, there have been recent graphic examples of damage to M. alfredi from vessel strike, the rate of which is likely underestimated (McGregor et al., 2019), while for R. typus, the proportion of the population detected bearing injuries consistent with ship strike is increasing (Speed et al., 2008; Lester et al., 2020). As M. alfredi has a naturally low rate of potential population growth, an increase in the rate of injury or mortality due ship strike is concerning, and therefore implementation of hazard mitigation strategies for core use areas should be considered. There may be opportunities to implement strategies under the overarching framework of protection and threat minimization required by World Heritage status. Examples of successfully implemented management actions include the network of ‘Go Slow’ zones, such as those established to minimise ship strike risk for dugong and turtles (Family: Cheloniidae and Dermochelyidae) in the Moreton Bay and the Great Barrier Reef Marine Parks on Australia’s east coast (Maitland et al., 2006; Shimada et al., 2017).
While our observed southerly dispersal among multiple independent tag tracks combined with photographic identifications provides additional confidence in reported regional movements for M. alfredi, the scale of the maximum likelihood error margins for light-based geolocation remains relatively large (Musyl et al., 2001; Nielsen et al., 2006) and may not be suitable for informing localised management actions. The scale of confirmed positional error we observed (Supplementary Figures S2, S3) is similar to that reported for double-tagged R. typus, in the Gulf of Mexico (Hueter et al., 2013) and for M. alfredi the Seychelles (Peel, 2019). The preferential shallow depth occupation of M. alfredi makes it a prime candidate for Fastloc-capable towed tag systems. Indeed, tethered Fastloc-GPS ARGOS tags have been used with good success on M. alfredi, albeit in low sample sizes (Kessel et al., 2017). The primary advantage of the system is the fast acquisition (and storage) of GPS ephemeris (with locations accurate to a few 10s of metres; Dujon et al., 2014) that are subsequently relayed back via the ARGOS system. However, deployment of such a system on enough individuals to generate a robust understanding of a species’ movement behaviour may preclude its use in the study of many species. Our identification of core use areas through satellite tracking could guide the appropriate positioning of a more affordable acoustic array for which representative sample sizes, longer tracking durations and fine-scale site use information may be more realistically obtained.
When deploying instrumentation for the purposes of gathering information to support conservation and biological understanding, managing the balance between data acquisition and the impact on the animal is an ongoing challenge (Hammerschlag et al., 2014; Hays et al., 2016). As a likely consequence of our anchor choice (nylon umbrella anchor), all 2016 tag deployments were shed prematurely. Other studies using the nylon anchoring system with satellite telemetry packages on mobulid rays also report high rates of premature tag shedding (Croll et al., 2012; Graham et al., 2012; Jaine et al., 2014; Francis and Jones, 2017). A switch to the titanium anchor in 2019 resulted in the full 180 days deployment period being achieved for the single reporting tag. Results for titanium tag anchors with larger satellite packages are mixed with premature tag detachment still observed (e.g., Peel, 2019), although full deployment times on M. alfredi have been achieved in work on the GBR (180 days, Adam Barnett pers comm) and Indonesia (> 120 days, Mark Erdmann pers comm). Resightings of previously tagged individuals (tagged with both anchor types) suggest that there is little or no long-term impact of tagging, with dorsal photographs revealing no evidence of retained tag tether, injury, or scarring at the tag attachment site (Project Manta Database, unpublished data). This observation is important with regards to the welfare of tagged M. alfredi as inappropriate tag and anchor systems have the potential to cause damage to the tagged animal as well as increase conflict with stakeholders (i.e., tourism operators at aggregation sites; Hammerschlag et al., 2014).
The use of multiple tools to explore movement patterns for pelagic or migratory species can help overcome limitations in temporal or spatial observational capacity associated with any one method. The combination of satellite telemetry with photo-identification studies has revealed previously unknown locations of core area use outside of the Ningaloo WHA which may host important habitat or foraging grounds for M. alfredi. We have established that the geographic range of reef manta rays visiting the Ningaloo WHA extends ∼700 km from Shark Bay in the south to the Dampier archipelago in the North, and is comparable to movements observed in the east Australian population at similar latitudes. The long-term site affinity to and movements between WHAs, suggest that on Australia’s west coast, these two WHAs provide protection for important habitat and likely foraging areas for reef manta rays.
The raw data supporting the conclusions of this article will be made available by the authors, without undue reservation.
Tagging procedures were compliant with ethics guidelines from the University of Queensland’s Animal Ethics Committee and conducted under approval certificate SBS/319/14/ARC/EA/LEIER, while tagging activity in Ningaloo Marine Park was compliant with the Western Australia Department of Biodiversity, Conservation and Attractions licences FO25000030 and CE005886.
AR, FM, GH, KT, MB, and MK secured funding. AJA, AOA, CD, FM, KT, and JS conducted field work. AJA performed the data analysis. AJA, AR, and CD wrote the manuscript. All authors contributed to manuscript revisions.
This research was funded by the Australian Research Council Linkage Grant LP110100712 in partnership with Austral Fisheries, TG Kailis Marine Conservation Fund, Murdoch University Coral Bay Research Station, Ningaloo Marine Interactions, Lady Elliot Island Eco Resort, and University of Queensland Scholarships to AOA and AJA. Funding partners provided direct financial (Australian Research Council, Austral Fisheries, TG Kailis Marine Conservation Fund) or in-kind support and were not directly involved in study design or data analysis and interpretation.
FM has a managing interest in funding partner Ningaloo Marine Interactions which provided in-kind support in the form of vessel time and photo-ID contributions from an onboard photographer.
The remaining authors declare that the research was conducted in the absence of any commercial or financial relationships that could be construed as a potential conflict of interest.
We would like to acknowledge the team at the Department of Biodiversity, Conservation and Attractions Exmouth Office for assisting with various field logistics; Gerard Smith and Adam Wall for supporting tag recovery efforts; Giles Winstanley for assistance with track simulations; and Samuel Williams for earlier tag modelling advice. We thank Brad Norman, Samantha Reynolds, and the Ecocean team for assisting the study by deploying two satellite tags in Shark Bay. Lastly, we thank all the onboard photographers, Ningaloo tourism industry operators, and the many citizen scientists who have generously contributed photographs to the Project Manta Western Australia ID database. HRPT AVHRR SST skin retrievals were produced by the Australian Bureau of Meteorology as a contribution to the Integrated Marine Observing System – an initiative of the Australian Government being conducted as part of the National Collaborative Research Infrastructure Strategy and the Super Science Initiative. Satellite derived Chl-a data were provided by NOAA’s Centre for Satellite Applications & Research (STAR) and the CoastWatch Program, and distributed by NOAA/NMFS/SWFSC/ERD.
The Supplementary Material for this article can be found online at: https://www.frontiersin.org/articles/10.3389/fmars.2020.00725/full#supplementary-material
Anderson, D. J., Kobryn, H. T., Norman, B. M., Bejder, L., Tyne, J. A., and Loneragan, N. R. (2014). Spatial and temporal patterns of nature-based tourism interactions with whale sharks (Rhincodon typus) at Ningaloo Reef, Western Australia. Estuar. Coast. Shelf Sci. 148, 109–119. doi: 10.1016/j.ecss.2014.05.023
Anderson, R. C., Adam, M. S., and Goes, J. I. (2011). From monsoons to mantas: seasonal distribution of Manta alfredi in the Maldives. Fish Oceanogr. 20, 104–113. doi: 10.1111/j.1365-2419.2011.00571.x
Armstrong, A. J., Armstrong, A. O., Bennett, M. B., McGregor, F., Abrantes, K. G., Barnett, A., et al. (2020). The geographic distribution of reef and oceanic manta rays (Mobula alfredi and Mobula birostris) in Australian coastal waters. J. Fish Biol. 96, 835–840. doi: 10.1111/jfb.14256
Armstrong, A. O., Armstrong, A. J., Bennett, M. B., Richardson, A. J., Townsend, K. A., and Dudgeon, C. L. (2019). Photographic identification and citizen science combine to reveal long distance movements of individual reef manta rays Mobula alfredi along Australia’s east coast. Mar. Biodivers. Rec. 12, 10–15. doi: 10.1186/s41200-019-0173-6
Bauer, R. (2018). RchivalTag: Analyzing Archival Tagging Data. R package version 0.0.7. Available online at: https://CRAN.R-project.org/package=RchivalTag (accessed April 5, 2020).
Bejder, L., Videsen, S., Hermannsen, L., Simon, M., Hanf, D., and Madsen, P. T. (2019). Low energy expenditure and resting behaviour of humpback whale mother-calf pairs highlights conservation importance of sheltered breeding areas. Sci. Rep. 9, 1–11. doi: 10.1038/s41598-018-36870-7
Braccini, M., de Lestang, S., and McAuley, R. (2018). Dusky sharks (Carcharhinus obscurus) undertake large-scale migrations between tropical and temperate ecosystems. Can. J. Fish. Aquat. Sci. 75, 1525–1533. doi: 10.1139/cjfas-2017-0313
Burnham, K. P., and Anderson, D. R. (2002). Model Selection and Multimodel Inference: A Practical Information-Theoretic Approach. New York, NY: Springer-Verlag.
Byrne, M. E., Cortés, E., Vaudo, J. J., Harvey, G. C. M. M., Sampson, M., Wetherbee, B. M., et al. (2017). Satellite telemetry reveals higher fishing mortality rates than previously estimated, suggesting overfishing of an apex marine predator. Proc. R. Soc. B Biol. Sci. 284:20170658. doi: 10.1098/rspb.2017.0658
Calenge, C. (2006). The package “adehabitat” for the R software: a tool for the analysis of space and habitat use by animals. Ecol. Model. 197, 516–519. doi: 10.1016/j.ecolmodel.2006.03.017
Clark, T. B. (2010). Abundance, Home Range, and Movement patterns of Manta Rays (Manta alfredi, M birostris) in Hawai’i. Master’s thesis. University of Hawai’i, Mãnoa, HI.
Cooke, S. (2008). Biotelemetry and biologging in endangered species research and animal conservation: relevance to regional, national, and IUCN Red List threat assessments. Endanger Species Res. 4, 165–185. doi: 10.3354/esr00063
Copping, J. P., Stewart, B. D., McClean, C. J., Hancock, J., and Rees, R. (2018). Does bathymetry drive coastal whale shark (Rhincodon typus) aggregations? PeerJ 6:e4904. doi: 10.7717/peerj.4904
Couturier, L. I. E., Jaine, F. R. A., Townsend, K. A., Weeks, S. J., Richardson, A. J., and Bennett, M. B. (2011). Distribution, site affinity and regional movements of the manta ray, Manta alfredi (Krefft, 1868), along the east coast of Australia. Mar. Freshw. Res. 62, 628–637. doi: 10.1071/MF10148
Couturier, L. I. E., Marshall, A. D., Jaine, F. R. A., Kashiwagi, T., Pierce, S. J., Townsend, K. A., et al. (2012). Biology, ecology and conservation of the Mobulidae. J. Fish Biol. 80, 1075–1119. doi: 10.1111/j.1095-8649.2012.03264.x
Couturier, L. I. E., Rohner, C. A., Richardson, A. J., Pierce, S. J., Marshall, A. D., Jaine, F. R. A., et al. (2013). Unusually high levels of n-6 polyunsaturated fatty acids in whale sharks and reef manta rays. Lipids 48, 1029–1034. doi: 10.1007/s11745-013-3829-8
Croll, D., Newton, K., Weng, K., Galván-Magaña, F., O’Sullivan, J., and Dewar, H. (2012). Movement and habitat use by the spine-tail devil ray in the Eastern Pacific Ocean. Mar. Ecol. Prog. Ser. 465, 193–200. doi: 10.3354/meps09900
Daly, R., Smale, M. J., Singh, S., Anders, D., Shivji, M., Daly, C. A., et al. (2018). Refuges and risks: evaluating the benefits of an expanded MPA network for mobile apex predators. Divers. Distrib. 24, 1217–1230. doi: 10.1111/ddi.12758
Double, M. C., Andrews-Goff, V., Jenner, K. C. S., Jenner, M.-N., Laverick, S. M., Branch, T. A., et al. (2014). Migratory movements of pygmy blue whales (Balaenoptera musculus brevicauda) between Australia and Indonesia as revealed by satellite telemetry. PLoS One 9:e93578. doi: 10.1371/journal.pone.0093578
Dujon, A. M., Lindstrom, R. T., and Hays, G. C. (2014). The accuracy of Fastloc-GPS locations and implications for animal tracking. Methods Ecol. Evol. 5, 1162–1169. doi: 10.1111/2041-210X.12286
Dulvy, N. K., Pardo, S. A., Simpfendorfer, C. A., and Carlson, J. K. (2014). Diagnosing the dangerous demography of manta rays using life history theory. PeerJ 2:e400. doi: 10.7717/peerj.400
Dunn, D. C., Harrison, A.-L., Curtice, C., DeLand, S., Donnelly, B., Fujioka, E., et al. (2019). The importance of migratory connectivity for global ocean policy. Proc. R. Soc. B Biol. Sci. 286:20191472. doi: 10.1098/rspb.2019.1472
Dwyer, R. G., Krueck, N. C., Udyawer, V., Heupel, M. R., Chapman, D., Pratt, H. L., et al. (2020). Individual and population benefits of marine reserves for reef sharks. Curr. Biol. 30, 480.e–489.e. doi: 10.1016/j.cub.2019.12.005
Ferreira, L. C., Thums, M., Meeuwig, J. J., Vianna, G. M. S., Stevens, J., McAuley, R., et al. (2015). Crossing latitudes-long-distance tracking of an apex predator. PLoS One 10:e0116916. doi: 10.1371/journal.pone.0116916
Francis, M. P., and Jones, E. G. (2017). Movement, depth distribution and survival of spinetail devilrays (Mobula japanica) tagged and released from purse-seine catches in New Zealand. Aquat. Conserv. Mar. Freshw. Ecosyst. 27, 219–236. doi: 10.1002/aqc.2641
Gales, N., McCauley, R. D., Lanyon, J., and Holley, D. (2004). Change in abundance of dugongs in Shark Bay, Ningaloo and Exmouth Gulf, Western Australia: evidence for large-scale migration. Wildl. Res. 31:283. doi: 10.1071/WR02073
Germanov, E. S., Bejder, L., Chabanne, D. B. H., Dharmadi, D., Hendrawan, I. G., Marshall, A. D., et al. (2019). Contrasting habitat use and population dynamics of reef manta rays within the Nusa Penida marine protected area, Indonesia. Front. Mar. Sci. 6:215. doi: 10.3389/fmars.2019.00215
Germanov, E. S., and Marshall, A. D. (2014). Running the gauntlet: regional movement patterns of Manta alfredi through a complex of parks and fisheries. PLoS One 9:e110071. doi: 10.1371/journal.pone.0110071
Graham, R. T., Witt, M. J., Castellanos, D. W., Remolina, F., Maxwell, S., Godley, B. J., et al. (2012). Satellite tracking of manta rays highlights challenges to their conservation. PLoS One 7:e36834. doi: 10.1371/journal.pone.0036834
Hammerschlag, N., Cooke, S. J., Gallagher, A. J., and Godley, B. J. (2014). Considering the fate of electronic tags: interactions with stakeholders and user responsibility when encountering tagged aquatic animals. Methods Ecol. Evol. 5, 1147–1153. doi: 10.1111/2041-210X.12248
Hanson, C. E., Pattiaratchi, C. B., and Waite, A. M. (2005). Sporadic upwelling on a downwelling coast: phytoplankton responses to spatially variable nutrient dynamics off the Gascoyne region of Western Australia. Cont. Shelf Res. 25, 1561–1582. doi: 10.1016/j.csr.2005.04.003
Hays, G. C., Bailey, H., Bograd, S. J., Bowen, W. D., Campagna, C., Carmichael, R. H., et al. (2019). Translating marine animal tracking data into conservation policy and management. Trends Ecol. Evol. 34, 459–473. doi: 10.1016/j.tree.2019.01.009
Hays, G. C., Ferreira, L. C., Sequeira, A. M. M., Meekan, M. G., Duarte, C. M., Bailey, H., et al. (2016). Key questions in marine megafauna movement ecology. Trends Ecol. Evol. 31, 463–475. doi: 10.1016/j.tree.2016.02.015
Hijmans, R. J. (2019). raster: Geographic Data Analysis and Modeling. R package version 3.0-7. Available online at: https://CRAN.R-project.org/package=raster (accessed April 5, 2020).
Hueter, R. E., Tyminski, J. P., and de la Parra, R. (2013). Horizontal movements, migration patterns, and population structure of whale sharks in the gulf of Mexico and Northwestern Caribbean Sea. PLoS One 8:e71883. doi: 10.1371/journal.pone.0071883
Hussey, N. E., Kessel, S. T., Aarestrup, K., Cooke, S. J., Cowley, P. D., Fisk, A. T., et al. (2015). Aquatic animal telemetry: a panoramic window into the underwater world. Science 6240, 1255642–1255642. doi: 10.1126/science.1255642
Jaine, F. R. A., Rohner, C. A., Weeks, S. J., Couturier, L. I. E., Bennett, M. B., Townsend, K. A., et al. (2014). Movements and habitat use of reef manta rays off eastern Australia: offshore excursions, deep diving and eddy affinity revealed by satellite telemetry. Mar. Ecol. Prog. Ser. 510, 73–86. doi: 10.3354/meps10910
Jenner, K. C. S., Jenner, M.-N. M., and McCabe, K. A. (2001). Geographical and temporal movements of humpback whales in western Australian waters. APPEA J. 41:749. doi: 10.1071/AJ00044
Kessel, S. T., Elamin, N. A., Yurkowski, D. J., Chekchak, T., Walter, R. P., Klaus, R., et al. (2017). Conservation of reef manta rays (Manta alfredi) in a UNESCO world heritage site: large-scale island development or sustainable tourism? PLoS One 12:e0185419. doi: 10.1371/journal.pone.0185419
Kohler, N. E., and Turner, P. A. (2001). Shark tagging: a review of conventional methods and studies. Environ. Biol. Fishes 60, 191–223. doi: 10.1007/978-94-017-3245-1_12
Lester, E., Meekan, M., Barnes, P., Raudino, H., Rob, D., Waples, K., et al. (2020). Multi-year patterns in scarring, survival and residency of whale sharks in Ningaloo Marine Park, Western Australia. Mar. Ecol. Prog. Ser. 634, 115–125. doi: 10.3354/meps13173
Lester, E., Speed, C., Rob, D., Barnes, P., Waples, K., and Raudino, H. (2019). Using an electronic monitoring system and photo identification to understand effects of tourism encounters on whale sharks in Ningaloo Marine Park. Tour Mar. Environ. 14, 121–131. doi: 10.3727/154427319X15634581669992
Maitland, R. N., Lawler, I. R., and Sheppard, J. K. (2006). Assessing the risk of boat strike on Dugongs Dugong dugon at burrum heads, Queensland, Australia. Pacific Conserv. Biol. 12:321. doi: 10.1071/PC060321
Marshall, A. D., Compagno, L. J. V., and Bennett, M. B. (2009). Redescription of the genus Manta with resurrection of Manta alfredi (Krefft, 1868) (Chondrichthyes; Myliobatoidei; Mobulidae). Zootaxa 2301, 1–28. doi: 10.11646/zootaxa.2301.1.1
Marshall, A. D., and Pierce, S. J. (2012). The use and abuse of photographic identification in sharks and rays. J. Fish Biol. 80, 1361–1379. doi: 10.1111/j.1095-8649.2012.03244.x
McGregor, F., Richardson, A. J., Armstrong, A. J., Armstrong, A. O., and Dudgeon, C. L. (2019). Rapid wound healing in a reef manta ray masks the extent of vessel strike. PLoS One 14:e0225681. doi: 10.1371/journal.pone.0225681
Musyl, M. K., Brill, R. W., Curran, D. S., Gunn, J. S., Hartog, J. R., Hill, R. D., et al. (2001). “Ability of archival tags to provide estimates of geographical position based on light intensity,” in Electronic Tagging and Tracking in Marine Fisheries. Reviews: Methods and Technologies in Fish Biology and Fisheries, Vol. 1, eds J. R. Sibert and J. L. Nielsen (Dordrecht: Springer), 343-367.
Nahas, E. L., Pattiaratchi, C. B., and Ivey, G. N. (2005). Processes controlling the position of frontal systems in Shark Bay, Western Australia. Estuar. Coast Shelf Sci. 65, 463–474. doi: 10.1016/j.ecss.2005.06.017
Neilson, J. D., Loefer, J., Prince, E. D., Royer, F., Calmettes, B., Gaspar, P., et al. (2014). Seasonal distributions and migrations of northwest atlantic swordfish: inferences from integration of pop-up satellite archival tagging studies. PLoS One 9:e112736. doi: 10.1371/journal.pone.0112736
Nielsen, A., Bigelow, K. A., Musyl, M. K., and Sibert, J. R. (2006). Improving light-based geolocation by including sea surface temperature. Fish. Oceanogr. 15, 314–325. doi: 10.1111/j.1365-2419.2005.00401.x
Norman, B. M., Reynolds, S., and Morgan, D. L. (2016). Does the whale shark aggregate along the Western Australian coastline beyond Ningaloo Reef? Pacific Conserv. Biol. 22:72. doi: 10.1071/PC15045
Norman, B. M., Whitty, J. M., Beatty, S. J., Reynolds, S. D., and Morgan, D. L. (2017). Do they stay or do they go? acoustic monitoring of whale sharks at Ningaloo Marine Park, Western Australia. J. Fish. Biol. 91, 1713–1720. doi: 10.1111/jfb.13461
Pante, E., and Simon-Bouhet, B. (2013). marmap: a package for importing, plotting and analyzing bathymetric and topographic data in R. PLoS One 8:e73051. doi: 10.1371/journal.pone.0073051
Peel, L. R. (2019). Movement Patterns and Feeding Ecology of the reef manta ray (Mobula alfredi) in Seychelles. Doctoral thesis. University of Western Australia, Crawley WA.
Pendoley, K. L., Schofield, G., Whittock, P. A., Ierodiaconou, D., and Hays, G. C. (2014). Protected species use of a coastal marine migratory corridor connecting marine protected areas. Mar. Biol. 161, 1455–1466. doi: 10.1007/s00227-014-2433-7
Penn, J. W., and Caputi, N. (1986). Spawning stock-recruitment relationships and environmental influences on the brown tiger prawn (Penaeus esculentus) fishery in Exmouth Gulf, Western Australia. Austr. J. Mar. Freshw. Res. 37, 491–505. doi: 10.1071/mf9860491
Preen, A. R., Marsh, H., Lawler, I. R., Prince, R. I. T., and Shepherd, R. (1997). Distribution and abundance of dugongs, turtles, dolphins and other megafauna in Shark Bay, Ningaloo Reef and Exmouth Gulf, Western Australia. Wildl. Res 24:185. doi: 10.1071/WR95078
Queiroz, N., Humphries, N. E., Couto, A., Vedor, M., da Costa, I., Sequeira, A. M. M., et al. (2019). Global spatial risk assessment of sharks under the footprint of fisheries. Nature 572, 461–466. doi: 10.1038/s41586-019-1444-4
Queiroz, N., Humphries, N. E., Mucientes, G., Hammerschlag, N., Lima, F. P., Scales, K. L., et al. (2016). Ocean-wide tracking of pelagic sharks reveals extent of overlap with longline fishing hotspots. Proc. Natl. Acad. Sci. U.S.A. 113, 1582–1587. doi: 10.1073/pnas.1510090113
Reynolds, S. D., Norman, B. M., Beger, M., Franklin, C. E., and Dwyer, R. G. (2017). Movement, distribution and marine reserve use by an endangered migratory giant. Divers. Distrib. 23, 1268–1279. doi: 10.1111/ddi.12618
Rohner, C. A., Richardson, A. J., Jaine, F. R. A., Bennett, M. B., Weeks, S. J., Cliff, G., et al. (2018). Satellite tagging highlights the importance of productive Mozambican coastal waters to the ecology and conservation of whale sharks. PeerJ 6:e4161. doi: 10.7717/peerj.4161
Sanzogni, R. L., Meekan, M. G., and Meeuwig, J. J. (2015). Multi-year impacts of ecotourism on whale shark (Rhincodon typus) sisitation at Ningaloo Reef, Western Australia. PLoS One 10:e0127345. doi: 10.1371/journal.pone.0127345
Scales, K. L., Miller, P. I., Hawkes, L. A., Ingram, S. N., Sims, D. W., and Votier, S. C. (2014). REVIEW: on the front line: frontal zones as priority at-sea conservation areas for mobile marine vertebrates. J. Appl. Ecol. 51, 1575–1583. doi: 10.1111/1365-2664.12330
Schofield, G., Scott, R., Dimadi, A., Fossette, S., Katselidis, K. A., Koutsoubas, D., et al. (2013). Evidence-based marine protected area planning for a highly mobile endangered marine vertebrate. Biol. Conserv. 161, 101–109. doi: 10.1016/j.biocon.2013.03.004
Shimada, T., Limpus, C., Jones, R., and Hamann, M. (2017). Aligning habitat use with management zoning to reduce vessel strike of sea turtles. Ocean Coast Manag. 142, 163–172. doi: 10.1016/j.ocecoaman.2017.03.028
Sims, D., Southall, E., Richardson, A. J., Reid, P., and Metcalfe, J. (2003). Seasonal movements and behaviour of basking sharks from archival tagging: no evidence of winter hibernation. Mar. Ecol. Prog. Ser. 248, 187–196. doi: 10.3354/meps248187
Sims, D. W., Witt, M. J., Richardson, A. J., Southall, E. J., and Metcalfe, J. D. (2006). Encounter success of free-ranging marine predator movements across a dynamic prey landscape. Proc. R. Soc. B Biol. Sci. 273, 1195–1201. doi: 10.1098/rspb.2005.3444
Sleeman, J. C., Meekan, M. G., Wilson, S. G., Jenner, C. K. S., Jenner, M. N., Boggs, G. S., et al. (2007). Biophysical correlates of relative abundances of marine megafauna at Ningaloo Reef, Western Australia. Mar. Freshw. Res. 58, 608–623. doi: 10.1071/MF06213
Speed, C. W., Meekan, M. G., Rowat, D., Pierce, S. J., Marshall, A. D., and Bradshaw, C. J. A. (2008). Scarring patterns and relative mortality rates of Indian Ocean whale sharks. J. Fish Biol. 72, 1488–1503. doi: 10.1111/j.1095-8649.2008.01810.x
Sprogis, K. R., Bejder, L., Hanf, D., and Christiansen, F. (2020). Behavioural responses of migrating humpback whales to swim-with-whale activities in the Ningaloo Marine Park, Western Australia. J. Exp. Mar. Biol. Ecol. 522, 151254. doi: 10.1016/j.jembe.2019.151254
Stewart, J. D., Jaine, F. R. A., Armstrong, A. J., Armstrong, A. O., Bennett, M. B., Burgess, K. B., et al. (2018). Research priorities to support effective manta and devil ray conservation. Front. Mar. Sci. 5:314. doi: 10.3389/fmars.2018.00314
Venables, S., McGregor, F., Brain, L., and van Keulen, M. (2016). Manta ray tourism management, precautionary strategies for a growing industry: a case study from the Ningaloo Marine Park, Western Australia. Pacific Conserv. Biol. 22:295. doi: 10.1071/PC16003
Venables, S., van Duinkerken, D., Rohner, C. A., and Marshall, A. (2020). Habitat use and movement patterns of reef manta rays Mobula alfredi in southern Mozambique. Mar. Ecol. Prog. Ser. 634, 99–114. doi: 10.3354/meps13178
Weeks, S., Magno-Canto, M., Jaine, F., Brodie, J., and Richardson, A. (2015). Unique sequence of events triggers manta ray feeding frenzy in the southern great barrier reef, Australia. Remote Sens. 7, 3138–3152. doi: 10.3390/rs70303138
Weimerskirch, H., Collet, J., Corbeau, A., Pajot, A., Hoarau, F., Marteau, C., et al. (2020). Ocean sentinel albatrosses locate illegal vessels and provide the first estimate of the extent of nondeclared fishing. Proc. Natl. Acad. Sci. U.S.A. 117, 3006–3014. doi: 10.1073/pnas.1915499117
Western Australia Department of Fisheries (2017). Department of Fisheries Annual Report to Parliament 2016/17∗. Available online at: https://www.fish.wa.gov.au/Documents/annual_reports/annual_report_2016-17.pdf (accessed April 5, 2020).
White, T. F. C. (1975). Population Dynamics of the Tiger Prawn Penaeus esculentus in the Exmouth Gulf Prawn Fishery, and Implications for the Management of the Fishery. PhD thesis, University of Western Australia, Crawley, WA.
Whitehead, H. (2001). Analysis of animal movement using opportunistic individual identifications: application to sperm whales. Ecology 82, 1417–1432. doi: 10.1890/0012-9658(2001)082[1417:aoamuo]2.0.co;2
Whitehead, H. (2009). SOCPROG programs: analysing animal social structures. Behave. Ecol. Sociobiol. 63, 765–778. doi: 10.1007/s00265-008-0697-y
Wilson, S. G., Pauly, T., and Meekan, M. G. (2001). Daytime surface swarming by Pseudeuphausia latifrons (Crustacea, euphausiacea) off Ningaloo reef, Western Australia. Bull. Mar. Sci. 68, 157–162.
Wilson, S. G., Pauly, T., and Meekan, M. G. (2002). Distribution of zooplankton inferred from hydroacoustic backscatter data in coastal waters off Ningaloo Reef, Western Australia. Mar. Freshw. Res. 53:1005. doi: 10.1071/MF01229
Winstanley, G. W. (2016). MantaUtil: Manta Database Utility. Version 4.2.1. Available online at: http://www.pelagicon.com/software/mantautil/ (accessed April 5, 2020).
Wolanski, E., and Hamner, W. M. (1988). Topographically controlled fronts in the ocean and their biological influence. Science 241, 177–181. doi: 10.1126/science.241.4862.177
Keywords: site affinity, Mobula alfredi, Ningaloo Reef, satellite telemetry, citizen science
Citation: Armstrong AJ, Armstrong AO, McGregor F, Richardson AJ, Bennett MB, Townsend KA, Hays GC, van Keulen M, Smith J and Dudgeon CL (2020) Satellite Tagging and Photographic Identification Reveal Connectivity Between Two UNESCO World Heritage Areas for Reef Manta Rays. Front. Mar. Sci. 7:725. doi: 10.3389/fmars.2020.00725
Received: 07 April 2020; Accepted: 10 August 2020;
Published: 28 August 2020.
Edited by:
Jeremy Kiszka, Florida International University, United StatesReviewed by:
Mourier Johann, Institut de Recherche Pour le Développement (IRD), FranceCopyright © 2020 Armstrong, Armstrong, McGregor, Richardson, Bennett, Townsend, Hays, van Keulen, Smith and Dudgeon. This is an open-access article distributed under the terms of the Creative Commons Attribution License (CC BY). The use, distribution or reproduction in other forums is permitted, provided the original author(s) and the copyright owner(s) are credited and that the original publication in this journal is cited, in accordance with accepted academic practice. No use, distribution or reproduction is permitted which does not comply with these terms.
*Correspondence: Amelia J. Armstrong, YW1lbGlhLmFybXN0cm9uZ0B1cWNvbm5lY3QuZWR1LmF1
Disclaimer: All claims expressed in this article are solely those of the authors and do not necessarily represent those of their affiliated organizations, or those of the publisher, the editors and the reviewers. Any product that may be evaluated in this article or claim that may be made by its manufacturer is not guaranteed or endorsed by the publisher.
Research integrity at Frontiers
Learn more about the work of our research integrity team to safeguard the quality of each article we publish.