- 1Marine Benthic Ecology and Ecophysiology (MABEE) Lab, Bermuda Institute of Ocean Sciences, St. George’s, Bermuda
- 2Bazaruto Center for Scientific Studies, Benguerra Island, Mozambique
- 3GEOMAR Helmholtz Centre for Ocean Research Kiel, Kiel, Germany
Global warming is considered to be the most severe threat to coral reefs globally, which makes it important for scientists to develop novel strategies that mitigate the impact of warming on corals and associated habitats. Artificial upwelling of cooler deep water to the surface layer may be a possible mitigation/management tool. In this study, we investigated the effect of simulated artificial upwelling with deep water off Bermuda collected at 50 m (24°C) and 100 m (20°C) on coral symbiont biology of 3 coral species (Montastrea cavernosa, Porites astreoides, and Pseudodiploria strigosa) in a temperature stress experiment. The following treatments were applied over a period of 3 weeks: (i) control at 28°C (ii) heat at 31°C, (iii) heat at 31°C+ deep water from 50 m depth, and (iv) heat at 31°C+ deep water from 100 m depth. Artificial upwelling was simulated over a period of 25 min on a daily basis resulting in a reduction of temperature for 2 h per day and the following degree-heating-weeks: 5.7°C-weeks for ii, 4.6°C-weeks for iii and 4.2°C-weeks for iv. Comparative analysis of photosynthetic rate, chlorophyll-a concentration and zooxanthellae density revealed a reduction of heat stress responses in artificial upwelling treatments in 2 of the 3 investigated species, and a stronger positive effect of 100-m water than 50-m water. These results indicate that artificial upwelling could be an effective strategy to mitigate coral bleaching during heat stress events allowing corals to adjust to increasing temperatures more gradually. It will still be necessary to further explore the ecological benefits as well as potential ecosystem impacts associated with different artificial upwelling scenarios to carefully implement an effective in situ artificial upwelling strategy in coral reefs.
Introduction
Global warming is the most severe threat to coral reefs worldwide (Hughes et al., 2017; Gattuso et al., 2018), since most reef-building corals are highly susceptible to abnormally high sea surface temperatures (SSTs). This is evident in coral bleaching, the loss of the coral’s symbiotic algae (zooxanthellae) inside the coral tissue, occurring during periods of increased SST in the form of heat waves over weeks to months (Brown, 1997; Weis, 2008). Temperature-sensitive species can die quickly when exposed to heat stress (few weeks; Jones, 2008; Hughes et al., 2018b), while others die as a lack of energy through photosynthesis, if deprived of their symbiotic algae for too long (weeks to months; Grottoli et al., 2006). To date, the most devastating coral bleaching event on record occurred from 2014 to 2017 affecting numerous coral reefs worldwide (Heron et al., 2017; NOAA Coral Reef Watch,, 2018; Hughes et al., 2018a). For example, in the Great Barrier Reef in 2016, 85% of the 171 individual reefs surveyed experienced bleaching, of which 31% were exposed to 8 – 16°C-weeks (degree heating weeks – DHW), which resulted in bleaching of 70–90% of all corals (Hughes et al., 2017). Furthermore, it was found that the degree of reef protection and water quality had very little effect on bleaching susceptibility, and that previous bleaching events did not seem to have made corals less susceptible to repeated heat stress in 2016 (Hughes et al., 2017). Considering that current climate change models predict increasing frequency and severity of abnormally high SSTs (e.g., heat waves) in many regions of the world over the course of this century (Cai et al., 2014; ICPP, 2014), and considering that reefs take at least 15–25 years to recover from major destructive events (Baker et al., 2008), the future of coral reefs is on a steep trajectory of decline. This highlights the need to develop novel and unconventional reef management strategies that either equip corals with the necessary toolkit to cope with SST rise as proposed via assisted evolution approaches or that allow corals to adjust more gradually to SST rise via heat stress mitigation (Rau et al., 2012; Anthony et al., 2017; Gattuso et al., 2018; National Academics of Sciences, Engineering, and Medicine [NAS], 2019).
While rearing heat resilient coral may be a viable solution to counter reef decline (van Oppen et al., 2015; Chakravarti et al., 2017; Van Oppen et al., 2017), the research is still in its infancy. Furthermore, this approach needs to account for other unforeseen future changes and potentially is not applicable to the broad range of coral species forming coral reefs and for larger reef areas due to logistical constrains. Hence, this approach will require substantial time to be applicable and may still have its limitations. Concepts that will reduce the occurrence of thermal stress in order to allow corals to survive heat waves and to adjust more gradually to global warming may be more feasible in the near future. Different approaches of heat stress mitigation have been suggested like reef shading achieved through marine cloud brightening (cloud production from seawater aerosols; Latham et al., 2012; Kravitz et al., 2013) or large shade cloths (Rau et al., 2012). Furthermore, naturally occurring turbid waters in some near shore reefs were suggested to act as refuges from coral bleaching (Cacciapaglia and Van Woesik, 2016). Shading has two effects: Firstly, it reduces the ocean’s uptake of solar energy or heat (Latham et al., 2012), and it also reduces the stress caused by the synergetic effect of high temperature and intense light (Mumby et al., 2001; Coelho et al., 2017). While most of this research is based on modeling approaches, not much testing of their applicability has been conducted in the lab or in situ so far (but see, Coelho et al., 2017). An alternative way of heat stress mitigation is artificial upwelling (AU), which brings cooler deep water into warm shallow reef waters, thereby reducing SST. So far, this geoengineering technology has mainly been considered for fertilizing purposes, where nutrient-rich deep water (deeper than 100 m) is introduced into nutrient-poor surface waters to increase primary production (Williamson et al., 2009; Fan et al., 2015; Pan et al., 2016). The increase in primary production via AU aims to increase the yield of fisheries (Kirke, 2003; Viúdez et al., 2016), or to increase carbon sequestration, which reduces CO2 in the ocean and atmosphere (Lovelock and Rapley, 2007; Pan et al., 2015; Zhang et al., 2017). A so far unstudied application of AU is surface water cooling (shown to occur by a modeling study of Oschlies et al., 2010). This may be a viable tool to mitigate thermal stress on corals, as indicated by studies in natural upwelling areas.
Localized natural upwelling has been found to reduce coral bleaching during periods of SST anomalies (Jiménez et al., 2001; Chollett et al., 2010; Bayraktarov et al., 2012; Wall et al., 2015; Schmidt et al., 2016; Riegl et al., 2018). For example, short-term cold-water intrusions due to large internal waves in the Andaman Sea, Thailand, significantly reduced coral bleaching and death on exposed reefs compared to sheltered reefs during a major bleaching event in 2010 (Wall et al., 2015; Schmidt et al., 2016). Furthermore, a modeling study proposed that certain upwelling regions in the Caribbean may be less prone to coral bleaching due to overall lower rate of SST rise (Chollett and Mumby, 2013). Although these findings are promising, studies are now required that investigate the effectiveness of different artificial upwelling regimes, including depth of upwelled water, and the rate and duration of upwelling.
If AU would be applied to cool surface waters of reefs during heat waves, it would be critical to choose a depth with a temperature that causes meaningful SST reductions, while matching as much as possible the seawater chemical composition of surface waters (e.g., inorganic nutrients, pH, dissolved inorganic carbon, oxygen). Unlike when AU is applied to fertilize surface waters, AU application for surface water cooling should minimize nutrient enrichment, which may otherwise lead to negative side effects of AU on reef communities (e.g., McCook, 1999). Furthermore, reductions of pH and changes in the seawater carbonate chemistry should be minimal to avoid negative effects on coral calcification (Schneider and Erez, 2006); and overcooling should be avoided, since this can, as well, lead to stress and bleaching in corals (Saxby et al., 2003; Kemp et al., 2011). In summer, decreased wind-induced water mixing often leads to a steep gradient in temperature with depth coinciding with the season of highest likelihood for heat stress. In the euphotic zone (>1% light of surface intensity), this is accompanied by overall low nutrient concentration and high oxygen concentration, and an increase of phytoplankton with depth (Lalli and Parsons, 1997). Below the euphotic zone, respiration and re-mineralization decrease oxygen concentration, increase CO2 and inorganic nutrients, and decrease seawater pH (Lalli and Parsons, 1997). Depending on region these changes are more or less pronounced, and depending on water clarity the depth of the euphotic zone varies.
In order to test the potential use of AU in mitigating coral bleaching during thermal stress, a laboratory study was carried out testing the effect of deep-water pulses from 50 m (∼24°C) and 100 m (∼20°C) depth on three different coral species. The 20-day study took place in Bermuda in late summer, when maximum annual SST of ∼28°C was reached. Thermal stress was simulated by exposing the corals to 31°C, and pulses of upwelling were applied once a day at midday reducing the temperature for a total period of 2 h. We hypothesized that both upwelling scenarios decelerate coral bleaching, while the water from 100 m is more efficient than the water from 50 m depth. Furthermore, we hypothesize that the efficiency of upwelling will vary between species. The goal of the study was to gain a first understanding about whether pulsed upwelling (sudden temperature drops) has the potential to mitigate coral bleaching in corals that are not naturally exposed to upwelling events, and – in case of a positive result - to stimulate further in-depth studies that would allow determining the most effective AU scenario(s). To facilitate the latter, the results are also discussed in a broader context, which includes further considerations for AU implementations and the potential benefits and risks that remain to be tested.
Materials and Methods
Study Site and Study Species
The Bermuda platform is a circular pseudo-atoll (∼900 km2) comprised by a large lagoon with numerous patch reefs surrounded by a tract of shallow rim reefs that drops quickly to the mesophotic reefs and to the deep ocean (Figure 1A). Bermuda’s reefs rank among the highest coral cover in the wider Caribbean with an estimated cover of 38.6% (Jackson et al., 2014). The oligotrophic waters surrounding the Bermuda platform are typically stratified during the summer months, with a temperature of ∼28°C at the surface, ∼24°C at 50 m depth and ∼20°C in 100 m depth (Steinberg et al., 2001). Inorganic nutrient concentration (nitrate + nitrite < 0.1 μmol kg–1, phosphate < 0.5 μmol kg–1) and concentrations of total organic carbon (60–70 μM with >95% being dissolved organic carbon) and total organic nitrogen (4–5 μM) are rather consistent within the first 100 m depth, while chlorophyll-a values are elevated at 100 m depth (0–50 m: <0.1 μg kg–1, 100 m: ∼0.4 μg kg–1) (Hansell and Carlson, 2001; Steinberg et al., 2001). Although no major bleaching event imposing significant mortality has been observed in Bermuda so far, coral bleaching and subsequent recovery has been observed fairly frequently in rather susceptible coral species, such as Millepora alcicornis (hydrozoan), Diploria labyrinthiformis and Montastrea cavernosa (Cook et al., 1990; Smith et al., 2013). Temperature threshold for coral bleaching in Bermuda is >28°C at the rim reef and >30°C at lagoonal and coastal reefs (Cook et al., 1990).
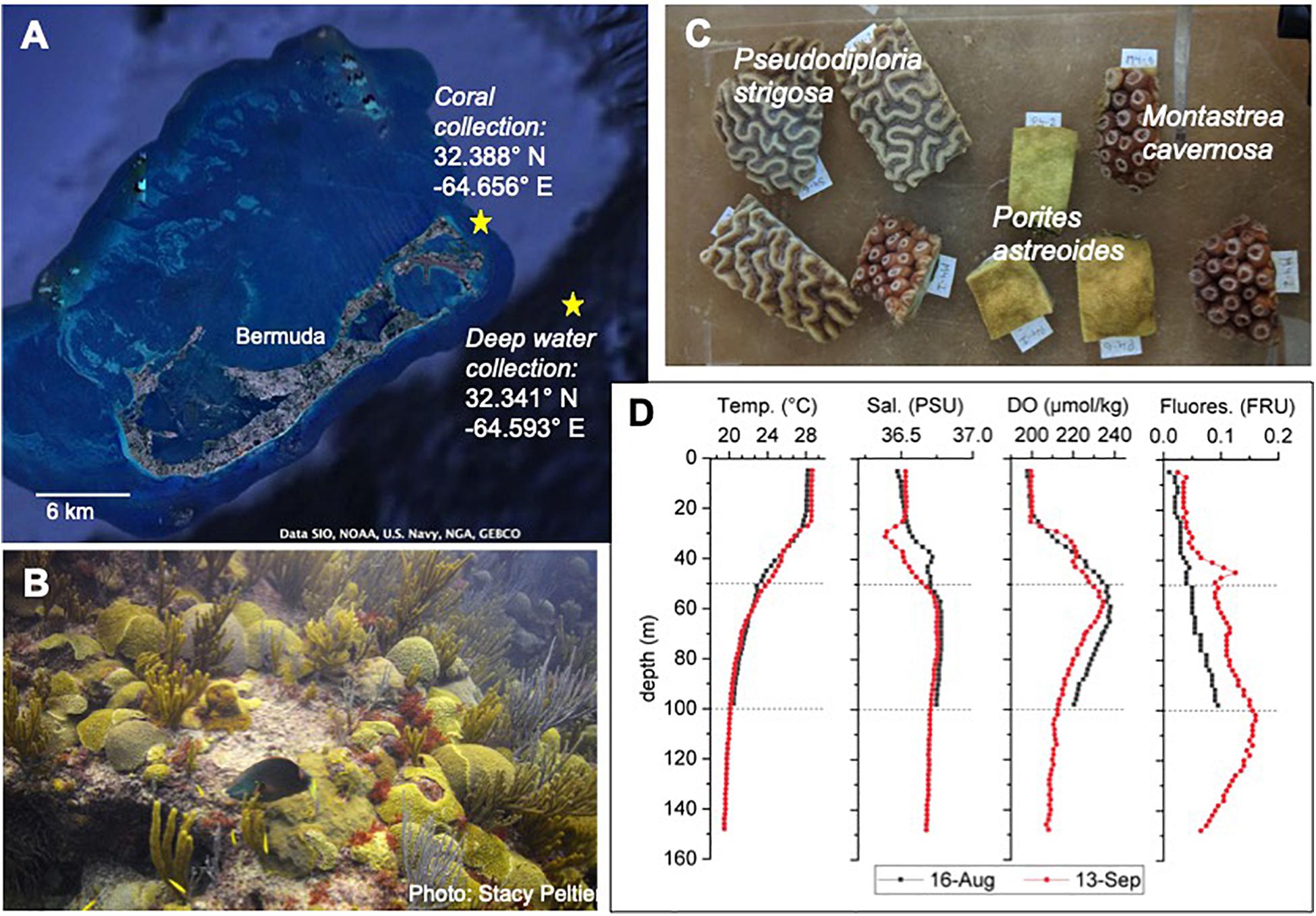
Figure 1. Overview of study site and species. (A) Google Earth image of Bermuda and the Bermuda platform with extensive coral reefs at the edges of the platform and at places throughout the platform. Site of coral collection and of deep-water collection are indicated by yellow stars. (B) Typical benthic community of a shallow water coral reef in Bermuda. (C) Coral fragments cut from coral colonies for the experiment. (D) Depth profiles at the site of water collection on 16-August and 13-September 2018. Temp., temperature; Sal., salinity; DO, dissolved oxygen; Fluores., fluorescence in fluorescence relative units (RFU). Calibrated data for the depth profiles provided by the Dr. Rod Johnson as part of the Bermuda Atlantic Time Series (BATS).
The common shallow water coral species Montastrea cavernosa, Porites astreoides, and Pseudodiploria strigosa (formerly Diploria strigosa; Budd et al., 2012) were used in the experiment, due to their importance as dominant reef builders in Bermuda reefs (Figure 1B). In August 2018, 6 colonies (diameter ∼20 cm) per species were collected (permit no. 2018071710, Department of Environment and Natural Resources, Bermuda government) with a hammer and chisel in 5 m depth from Sea Venture Shoals, a reef that is close to open ocean water (Figure 1A). Corals were brought to the Bermuda Institute of Ocean Sciences (BIOS) in coolers filled with seawater within 1h after collection. Each colony was divided into 9 fragments using a wet-cutting diamond saw and kept in in-door aquaria with continuous seawater flow-through for 3 weeks for recovery and acclimatization (Figure 1C). Seawater is constantly pumped from 2 m depth in Ferry Reach in front of BIOS. Temperature was kept at 28°C using chillers (TECO TK500) and aquarium heaters (Accu-therm, Cobalt Aquatics), and light (photosynthetic active radiation – PAR: 220 – 240 μE m–2 s–1) was provided for 13 h each day (Indoor grow light, Sun Blaze T5 Fluorescent 44).
Experimental Setup
To test the effect of deep cold water pulses (AU) during thermal stress, the following temperature scenarios were applied, each on a subset of five replicate aquaria filled with 6 L of seawater: (i) Control condition – surface water at 28°C, which is the average summer temperature at shallow rim reefs; (ii) “Heat” treatment – surface water at 31°C, which is known to cause thermal stress and bleaching in Bermuda corals; (iii) “Heat + 50” m AU treatment – surface water at 31°C mixed with cooler water from 50 m depth once a day; (iv) “Heat + 100” m AU treatment – surface water at 31°C mixed with cooler water from 100 m depth once a day. Each subset of aquaria was kept inside a larger water basin, which was temperature-controlled by a chiller and a heater connected to a temperature controller (28°C or 31°C). Five of the 6 coral colonies per species (replicates) were used in the experiment (the 6th colony served as a back-up) and the 9 clones (fragments) of each coral colony were distributed between the 4 treatments, with 3 fragments in the control and 2 fragments in each of the heat treatments. Water flow was supplied by small aquarium pumps within each aquarium and a slow flow-through of unfiltered seawater was applied in the night after the “new” seawater has been adjusted to target temperature during the day.
AU Simulation
Prior to the experiment, a preliminary test was conducted to determine the AU regime to be applied, while considering the amount of deep water available. Deep water was collected during 2 cruises of the RV Atlantic Explorer, and the total amount of water available allowed a maximum addition of ∼5.5 L of deep water per AU aquarium per day, for a period of 20 days. For the preliminary test, we used surface water cooled down to the same temperature as in 50 m (24°C) and 100 m (20°C) depth, respectively, and we added the chilled water to 31°C warm water in different mixing ratios and flow speeds. The strongest and longest cooling effect with the available amount of water was achieved by removing 3 L of the 6 L of water in the aquarium, and then adding 5.5 L of cool water over a period of 22–24 min. This resulted in a 1:1 mixing ratio of “hot” and cool water after about 15 min, followed by continued cooling for another 7–9 min. Water warmed up again over a period of about 1.5 h until reaching the initial temperature. This AU scenario was applied to the AU treatments.
Three weeks prior to the start (16-August 2018, Batch 1) and in the middle of the experiment (13-September 2018, Batch 2), ∼260 L of water per depth (50 and 100 m) were collected 3 km off the Bermuda platform (Figure 1A) with a rosette of 24 × 12-L Niskin bottles on board of the RV Atlantic Explorer. A connected CTD (regularly used and calibrated as part of the Bermuda Atlantic Time Series) allowed depth profiles of temperature, salinity, dissolved oxygen and fluorescence (Figure 1D). The water was filled into 25-L dark water storage containers, brought to BIOS within 3 h after collection and stored in the dark at 4°C to minimize biological activity and hence changes in water chemistry. During each day of the experiment, the amount of deep water required for one AU simulation (one per day) was poured into a larger container and was allowed to slowly come up to room temperature. Just prior to the daily AU simulation at ∼1300 h, the water from 50 m depth was adjusted to 24°C and the water from 100 m depth to 20°C with a cooler or heater. Then the deep water was added to the Heat + 50 and Heat + 100 treatment aquaria, respectively, following the AU scenario described above.
Measurement of Water Quality
Temperature was monitored continuously in one of the five replicate aquaria, as well as in three replicate aquaria during AU simulation, using temperature loggers (HOBO Pendant Temperature/Light loggers, ONSET). Salinity was measured twice per day (morning and evening) and small amounts of distilled water were added to the aquaria in case salinity increased as a consequence of evaporation. pH was measured in parallel to salinity, as well as just before AU simulation and after AU simulation in the AU aquaria. Conductivity (salinity) and pH sensors (Orion Star, Thermo Fisher Scientific) were calibrated weekly with standard calibration solutions. Environmental parameters of the deep water were measured, during deep-water retrieval and every 3rd day throughout the experiment immediately before AU simulation. Those included salinity, pH, concentration of dissolved inorganic carbon (DIC) and total alkalinity (TA). For DIC and TA analysis, water samples were taken, preserved with HgCl2 and later measured using two automated high-precision VINDTA systems at BIOS (model VINDTA 3C; built by L. Mintrop at Marianda Co.; Bates, 2017). TA and DIC were calibrated by measuring certified reference material (CRM; prepared by A.G. Dickson, Scripps Institution of Oceanography).
Measurements of Coral Response Parameters
Thermal stress can cause a cascade of physiological response in corals, while the loss of zooxanthellae and their photo-harvesting pigments (chlorophyll-a, c2 and peridinin) is the most obvious sign of a thermal stress response (coral bleaching). A number of studies suggested that the loss of zooxanthellae is the consequence of their malfunctioning photosystem caused by stress-related production of oxygen radicals in the chloroplasts (e.g., Warner et al., 1999; Lesser, 2006). More recent studies, however, indicate that the leaching of oxygen radicals from the zooxanthellae to the host – imposing a risk of cell damage to the host – triggers expulsion of zooxanthellae even if the photosystems are still intact (Gardner et al., 2017).
In this study, net photosynthetic rate was measured and standardized to surface area representing the product of zooxanthellae photosystem functioning and the amount of zooxanthellae and photo-pigments per surface area. Net photosynthetic rate was measured repetitively of one fragment per colony and per treatment (5 colonies [replicates] × 3 species × 4 treatments = 60 fragments). Always the same fragment was measured on day 3, 7, 12, 15, and 20 of the experiment, using 1 L-glass incubation chambers with a plastic lid (Glasslock USA, Inc.). The chambers were placed upside down to guarantee maximum light penetration (through glass instead of plastic) and were equipped with an oxygen sensor spot (PyroScience), a magnetic stirring bar and a grid for coral placement above the stirring bar. A tub of water was placed on a magnetic stirring plate and the chambers were submerged inside the tub to stabilize the temperature inside the chambers (28° or 31°C). Twelve fragments were incubated simultaneously over a period of 45 min starting with the fragments of the AU treatments in order to avoid interference with the AU pulse conducted in the afternoon. Five to six rounds of incubations were conducted per day until measurements of all 60 fragments were completed. Light during incubations was equal to the experimental light intensity (220–240 μE m–2 s–1). Oxygen was measured in the beginning and at the end of the incubation by holding a fiber optic cable connected to an Optical Oxygen Meter (FireSting, PyroScience, Germany; calibrated with air-bubbled seawater as a 100% dissolved oxygen standard) from the outside of the incubation chamber against the oxygen sensor spot inside the chambers for 30 s. The change in oxygen concentration was used to calculate net photosynthesis standardized to coral surface area (μmol O2 cm–2 h–1). Surface area of the coral fragment was determined by photography and subsequent picture analysis using Image J (fragments had a rather flat surface, since all experimental coral species feature a massive growth form; Figure 1C).
Chlorophyll-a concentration and zooxanthellae density were determined as a metric for coral bleaching. For this, one fragment of each coral colony was harvested from the control treatment on day 3 (5 colonies × 3 species × 1 treatment = 15 fragments) and from all four treatments on day 12 and day 20 of the experiment (5 colonies × 3 species × 4 treatments = 60 fragments per sampling day). The tissue was removed from the skeleton with an airbrush and filtered seawater (0.47 μm pore size; ∼25 ml) inside a Ziploc bag, and homogenized using an Ultraturrax homogenizer. Aliquots of the tissue slurry were frozen at −80°C (1.5 ml – chlorophyll-a) and −20°C (1.5 ml – zooxanthellae density) until further processing. For chlorophyll-a measurements, the tissue slurry was defrosted on ice, centrifuged (3100 rpm, 2 min) to collect the zooxanthellae in the pellet, the supernatant was discarded, and the pellet was re-dissolved in 0.5 ml of distilled water and mixed with 4.5 ml of 100% acetone (final concentration of acetone 90%). Chlorophyll extraction took place over 24 h at 4°C in the dark. Extract was centrifuged (3100 rpm, 4 min) and the absorbance of the supernatant containing the chlorophyll was measured in a spectrophotometer at wavelengths 663, 630 nm and 750 (absorbance at 750 is an indicator for turbidity, which was subtracted from 663 and 630), using 1 cm glass cuvettes (Parsons et al., 1984). Chlorophyll-a concentration was calculated following Parsons et al. (1984): Chlorophyll-a [μg] = (11.43 × Absorbance663)-(0.64 × Absorbance630). For zooxanthellae density, tissue slurry was defrosted, homogenized again, and drops of the slurry were placed on a hemocytometer and counted under the microscope (e.g., Sawall et al., 2014). The average of 6 replicate counts (6 drops) was used to calculate zooxanthellae density. Chlorophyll-a and zooxanthellae density were standardized to coral surface area.
Data Analysis
Degree-heating-weeks (DHW) were calculated from the temperature data following the description from NOAA (National Oceanic and Atmospheric Administration, United States). Temperature data were decomposed into weekly means and temperature anomalies per week calculated by subtracting the long-term climatological maximum monthly mean SST (28°C). The heat stress experienced by the corals in terms of DHW were derived by summing up all weekly anomalies for the experimental period only if they were ≥1°C (Strong et al., 1997; Liu et al., 2003).
One-way ANOVA and the Tukey’s HSD post hoc test were performed with the software OriginPro2018 to examine differences between treatments at the end of the experiment (day 20). Each response parameter was tested separately for each coral species.
Results
Temperature Regimes
Temperature in the Control treatment varied around the target temperature of 28°C with a minimum temperature of 27.1°C and a maximum temperature of 28.8°C (Figure 2A and Table 1). Minimum temperatures occurred later in the night after several hours of fresh seawater supply, which was slightly cooler than the target temperature. Temperature in the heat treatments (Heat, Heat + 50, Heat + 100) varied around the target temperature of 31°C (Table 1), except during AU pulses (Figure 2A). During AU pluses, in Heat + 50, temperature dropped by about 3.5°C (minimum ∼27.5°C) and by about 5°C (minimum ∼26°C) in the Heat + 100 (Figures 2B,C). In both AU treatments the drop in temperature occurred over a period of 25 min and the subsequent increase in temperature to 31°C required 1.5 to 2 h (Figures 2B,C). This resulted in daily mean temperatures of 30.9 ± 0.2°C (mean ± SD; Heat), 30.6 ± 0.2°C (Heat + 50) and 30.4 ± 0.2°C (Heat + 100). AU simulations were consistent between replicate aquaria (Figure 2C). Degree heating weeks were 5.73 ± 0.47°C-weeks for Heat treatment, which was reduced by 1.1°C-weeks in Heat + 50 and by 1.56°C-weeks in Heat + 100 (Supplementary Figure S1).
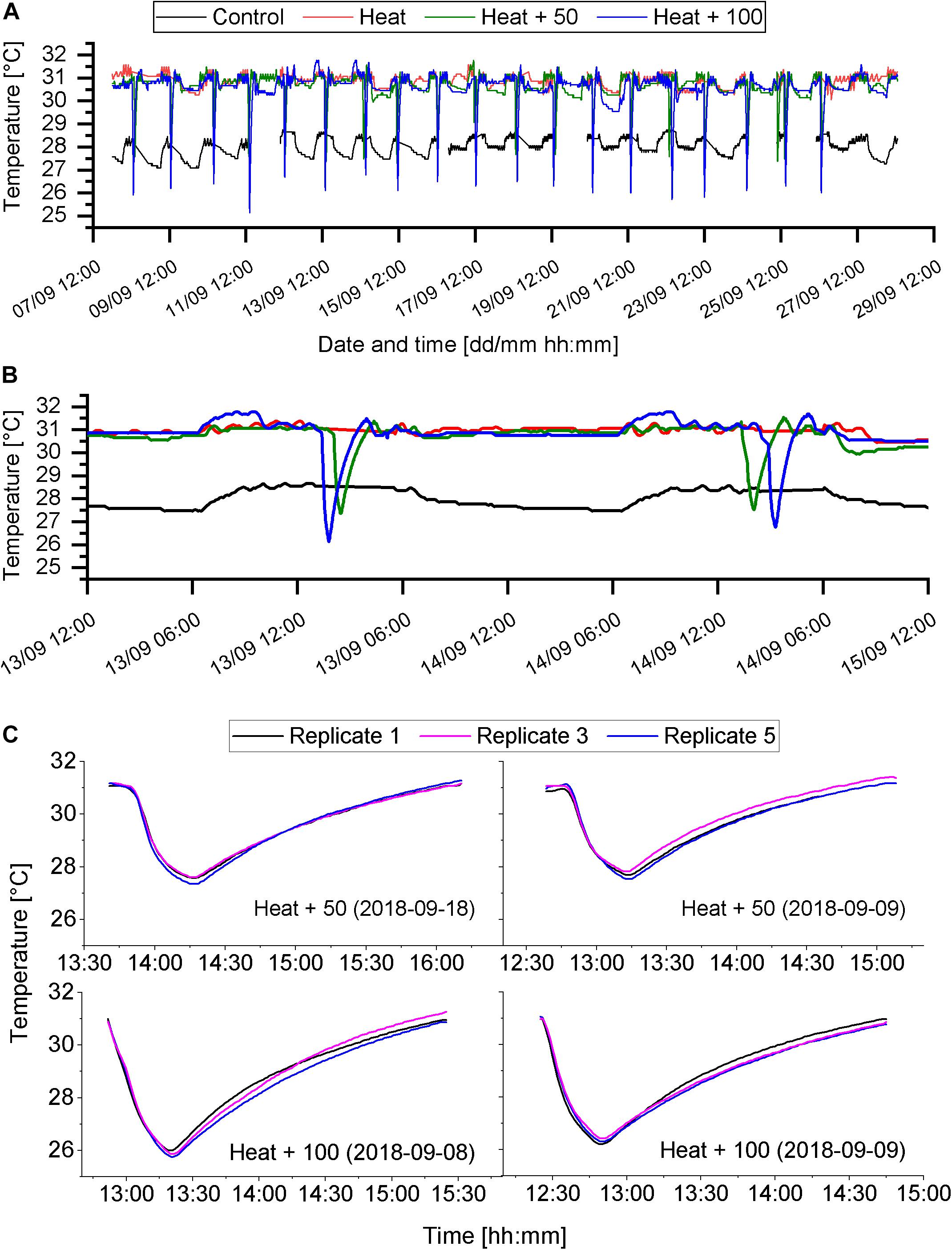
Figure 2. Temperature regimes of the different treatments. (A) Temperature of each of the four treatments throughout the experimental phase (8th to 28th of September 2018). Sudden drops of temperature indicate AU events. Gaps in the data set indicate gaps in temperature recordings. (B) Close up of temperature regime of 2 experimental days (13th and 14th of September). (C) Examples of AU events, showing the temperature of 3 of the 5 replicate aquaria.
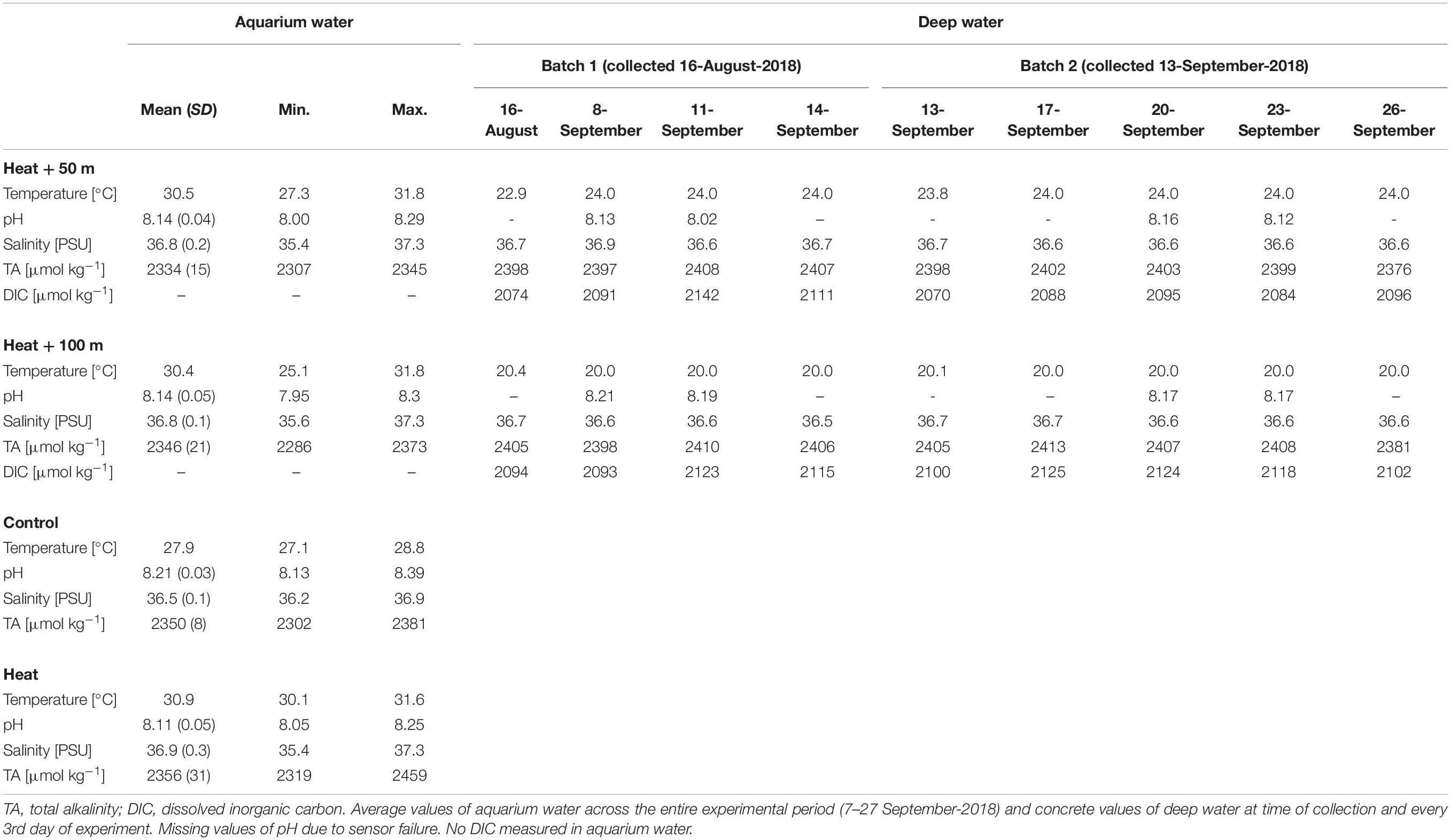
Table 1. Environmental conditions of the aquarium water (experimental units) and the deep water collected at 50 and 100 m depth.
pH, Salinity, Total Alkalinity, Dissolved Inorganic Carbon
Parameters other than temperature were rather consistent throughout the treatments and over the duration of the experiment (Table 1). Mean pHNBS varied between 8.11 ± 0.05 (Heat, mean ± SD) and 8.21 ± 0.03 (Control). Mean salinity ranged from 36.5 ± 0.1 (Control) to 36.9 ± 0.3 PSU (Heat), while salinities up to 37.3 PSU occurred occasionally due to evaporation in the 31°C-treatments. Short-term drops in salinities (<36 PSU) occurred in some cases during manual salinity adjustments. TA ranged from 2334 ± 15 (Heat + 50) to 2356 ± 31 μmol kg–1 (Heat). In the deep water, temperature of Batch 1 was 22.9°C in 50 m depth and 20.4°C in 100 m depth, while it was closer to the target temperature of 24°C (50 m: 23.8°C) and 20°C (100 m: 20.1°C) in Batch 2. The chemical properties of the deep water were similar to the aquarium water (shallow water) and varied little over time (Table 1). Salinity was 36.7 PSU in both depths, TA was around 2400 μmol kg–1 in both depths (∼50 μmol kg–1 higher than in the aquarium water), and DIC varied around 2100 μmol kg–1 (Table 1).
Coral Response Parameters
Most coral fragments survived the experimental period of 20 days (Figures 3A–C – numbers behind values of day 20). Among the few corals that died, most of them belonged to the species P. astreoides, where 2 of 5 fragments died in Heat, and 1 of 5 in both Heat + 50 and Heat + 100 (Figure 3B). M. cavernosa and P. strigosa each experienced a loss of 1 fragment in Heat + 100 (Figures 3A,C). Paling (=bleaching) of corals was evident in corals in the Heat and Heat + AU treatments including corals that have died, but has not been quantified explicitly. The results of the statistical tests are presented in Figure 3 with details provided as Supplementary Table S1.
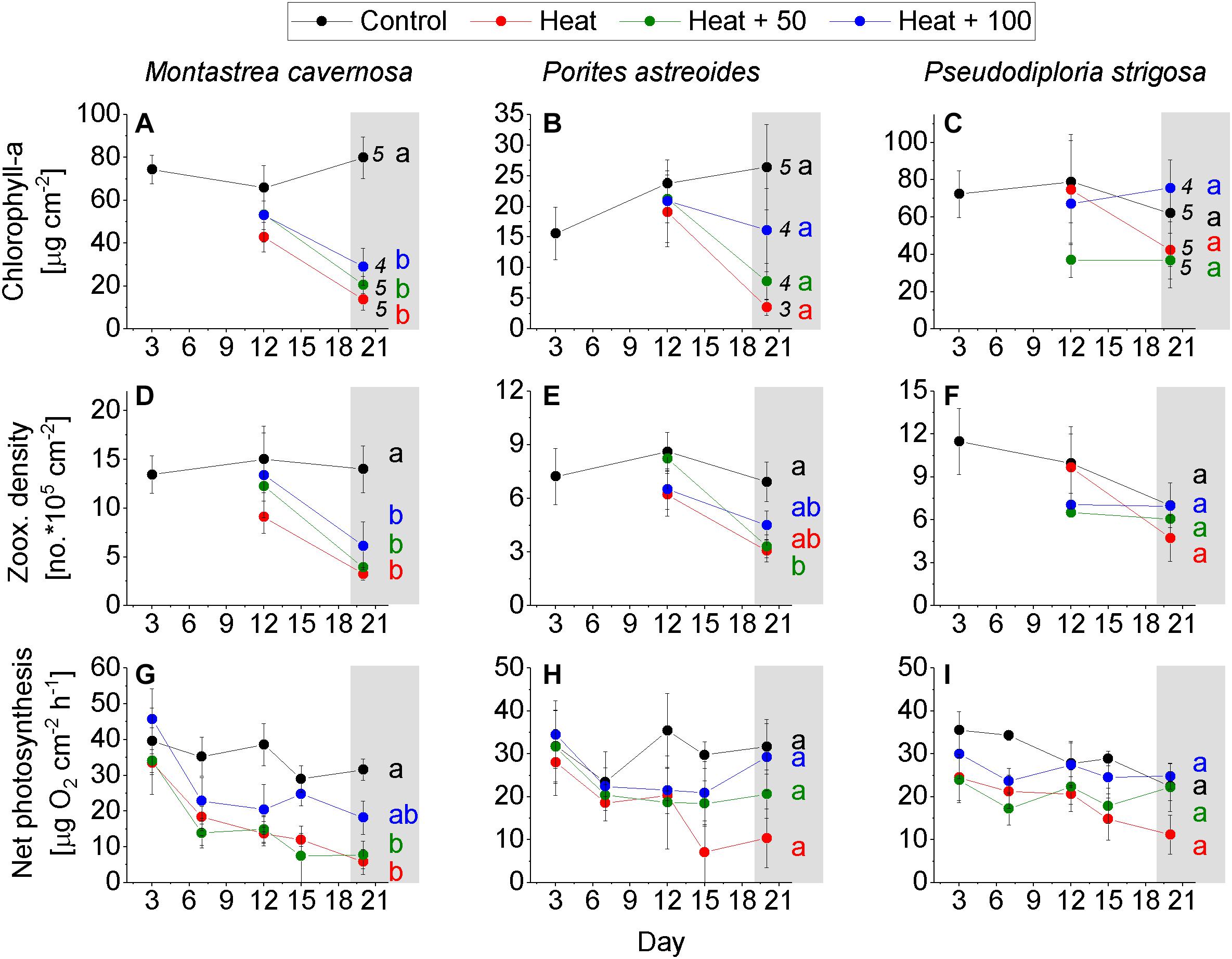
Figure 3. Coral response over the experimental period. (A–C) Chlorophyll-a concentration of the control corals on day 3 and of corals of all treatments on day 12 and 20. (D–F) Zooxanthellae density of the control corals on day 3 and of the corals of all treatments on day 12 and 20. (G–I) Net photosynthesis measured on day 3, 6, 9, 12, 15, 18, and 20 on coral of all treatments. Mean ± SE. Numbers behind data points of day 20 = number of replicates on day 20 (initial number of replicates = 5). Letters indicate statistical results with equal letters indicating p > 0.05 between treatments on day 20 and different letters indicating < 0.05 (one-way ANOVA, Tukey HSD post hoc).
Montastrea cavernosa experienced a significant loss of chlorophyll-a in all heat treatments, while remaining rather constant in Control with a final concentration of 79.9 ± 9.7 μg cm–2 (mean ± SE). Heat featured the lowest chlorophyll-a concentration at the end of the experiment (13.8 ± 5.1), followed by Heat + 50 (20.6 ± 4.0) and Heat + 100 (29.1 ± 8.7; Figure 3A). A similar pattern was evident in zooxanthellae density where Control remained constant throughout the experiment with a final density of 14.0 ± 2.4 × 105 cells cm–2, while the heat treatments had significantly reduced densities at the end of the experiment, albeit less pronounced in Heat + 100 (Heat: 3.3 ± 0.7 × 105, Heat + 50: 3.9 ± 0.6 × 105, Heat + 100: 6.1 ± 2.4 × 105; Figure 3D). Net photosynthesis of Control slightly decreased over the course of the experiment (from 39.2 ± 8.7 to a final rate of 31.6 ± 3.0 μg O2 cm–2 h–1), rates were significantly lower in Heat + 50 (7.7 ± 3.8) and Heat (5.8 ± 3.5) than Control, and were less reduced in Heat + 100 with values lying between Control and Heat + 50 (18.2 ± 4.6; Figure 3G). A decoupling between the trajectories of zooxanthellae density and net photosynthesis rate seems to be evident in the heat treatments where net photosynthesis decreased before zooxanthellae densities declined (Figures 3D,G).
Porites astreoides showed similar trends in the response parameters as M. cavernosa. A high variability between replicates and a reduced number of replicates in all heat treatments (due to coral death), however, led to non-significant results in most cases. Chlorophyll-a increased in Control over the duration of the experiment (final: 26.4 ± 6.9 μg cm–2) and was higher than in all heat treatments. Second highest average chlorophyll-a concentration occurred in Heat + 100 (16.1 ± 6.8) followed by Heat + 50 (7.7 ± 3.0) and Heat (3.5 ± 1.3) at the end of the experiment (Figure 3B). Zooxanthellae density of Control remained high throughout the experiment with 6.9 × 105 cells cm–2 after 20 days, while densities decreased in all heat treatments with Heat + 50 (3.3 ± 0.6 × 105) being amongst the lowest and the only one significantly lower than Control (Figure 3E). Net photosynthesis of Control was rather consistent throughout the experiment (except on day 6) with 31.7 ± 6.3 μg O2 cm–2 h–1 at the end of the experiment. Net photosynthesis was similarly high in Heat + 100 (29.3 ± 7.8), and lower in Heat + 50 (20.6 ± 5.6) and Heat (10.3 ± 6.8), although not statistically significant (Figure 3H). Like in M. cavernosa, a decrease in net photosynthesis in the heat treatments appeared to be earlier in the experiment and remained similar or even increased (Heat + 100) toward the end of the experiment, while zooxanthellae density decreased later in the experiment (Figures 3E,H).
Of all study species, P. strigosa showed the weakest response to the treatments. Also none of the tested differences between treatments were statistically significant. Chlorophyll-a concentration in Control was rather constant throughout the duration of the experiment, but also highly variable between replicates (62.0 ± 28.6 μg cm–2 at the end of the experiment). In Heat + 100, chlorophyll-a was similarly high than in Control at the end of the experiment (75 ± 14.5), followed by Heat (42.2 ± 15.2) and Heat + 50 (36.8 ± 14.7; Figure 3C). Zooxanthellae density decreased in Control over the experimental duration from 11.5 ± 2.3 × 105 (day 3) to 7.0 ± 1.5 × 105 cells cm–2 (day 20). At the end of the experiment, zooxanthellae density in Heat + 100 was equally high as in Control (7.0 ± 2.1 × 105), but lower in Heat + 50 (6.1 ± 1.7 × 105) and Heat (4.7 ± 1.6 × 105; Figure 3F). Net photosynthesis decreased in Control over the experimental duration from 35.5 ± 4.4 (day 3) to 22.5 ± 3.4 μg O2 cm–2 h–1 (day 20). Net photosynthesis in Heat + 50 (22.3 ± 12.6) and Heat + 100 (24.8 ± 3.0) were similar as in Control, but lower in Heat (11.1 ± 4.6; Figure 3I) at the end of the experiment. Net photosynthesis varied slightly more between treatments on day 3 already, if compared to M. cavernosa and P. astreoides (Figures 3G–I).
Data repository: CTD data/depth profiles collected on the research vessel are available on the BIOS website from the BATS Data Portal (cast IDs: 10350022, 10350023, 10351019, 10351020). All other data (environmental and coral responses) are available on the PANGAEA website1.
Discussion
Artificial upwelling could be an emerging reef management tool by locally reducing SST during heat waves and hence providing a temporal refugium for corals from thermal stress. The results of our study support the hypotheses (i) that pulses of cooler deep water can reduce thermal stress responses during a heat stress event, and (ii) that the water from a deeper and cooler layer in the ocean (100 m) is more efficient than from a more shallow and warmer layer (50 m). Furthermore, it is evident that species-specific differences in responses occur. Mitigation of heat stress occurred despite the rather short pulses of deep-water supply, which restricted temperature reduction (<31°C) to less than 2 h per day and led to an overall reduction of DHW by 1.1–1.6°C-weeks. In the following, we discuss the results in more detail, and we provide a broader picture on the implications of our results with respect to AU utilization, and what else needs to be considered and investigated from an ecological standpoint.
Efficiency of Simulated Upwelling in Coral Bleaching Mitigation
The two coral species M. cavernosa and P. astreoides showed the strongest response to the treatments with respect to both heat stress and mitigation of heat stress under simulated upwelling. Being aware of the fact that our low replication of n = 5 likely precludes statistically significant results between Heat and Heat + AU, the trends in zooxanthellae characteristics are reasonably clear to draw the conclusion that pulsed AU has the potential to mitigate heat stress responses in these two coral species.
Heat stress responses were first evident in a reduction of net photosynthetic rates followed by a loss of zooxanthellae (and pigments; M. cavernosa and P. astreoides). This indicates that photo-damage may be a rapid response to heat, which is followed by the coral’s expulsion of the malfunctioning zooxanthellae (Lesser, 2006; Hennige et al., 2011). Contrasting results were recently found in a heat stress experiment, where the branching corals Acropora millepora and Stylophora pistillata experienced a loss of zooxanthellae several days before a reduction in photosynthesis and photosynthetic efficiency was observed (Gardner et al., 2017), indicating species-specific heat stress responses. Interestingly, in the AU treatments net photosynthesis remained rather stable throughout the second half of the experiment (while they declined further in the Heat treatment, but also in Heat + 50 of M. cavernosa) and even increased in Heat + 100 in P. astreoides, despite the loss of zooxanthellae. The reason for this could be that a moderate loss of zooxanthellae may be counterbalanced by an increase of light available for the remaining symbionts thereby maintaining a comparatively high photosynthetic rate (non-linear relationship between cell density and photosynthesis; e.g., Hoogenboom et al., 2010; Gardner et al., 2017), in particular under AU conditions. It may also be that thermal stress led to a selection of prevalent thermally tolerant Symbiodinium types with potentially improved photosynthetic efficiency, or that highly abundant endolithic algae increased their photosynthetic rates due to increased availability of light (Pernice et al., 2020). In P. astreoides, it is worthwhile mentioning that this species experienced the highest number of dead fragments due to heat. This could be due to lower energy reserves (less biomass), where a shortage of photosynthetic energy quickly leads to starvation (Thornhill et al., 2011). Alternatively, this could also be due to a higher susceptibility to colony fragmentation (3 weeks prior the experiment), which only becomes evident during concomitant heat stress. The consequent reduction in the number of replicates (survivors) likely explains the lack of statistical significance between treatments, despite rather clear trends.
Bleaching susceptibility of M. cavernosa and P. astreoides are generally considered to be moderate, while it is considered rather high in P. strigosa, as studies have shown during large-scale bleaching events in the wider Caribbean (Florida Keys – Brandt, 2009; Little Cayman – van Hooidonk et al., 2012; US Virgin Islands – Miller et al., 2009). This seems to be in contradiction with our finding, where P. strigosa revealed a high coral survival and the lowest differences between treatments at the end of the experiment. However, it needs to be considered that the corals in Control experienced a decline in zooxanthellae density and net photosynthesis over the course of the experiment as well (maybe due to fragmentation or continued acclimatization to laboratory conditions), which may distort visible changes due to heat. Therefore, the observed small differences found between treatments at the end of the heat stress experiment (lower net photosynthesis in Heat, compared to Control and Heat + AU conditions) may indicate beneficial effects of AU on P. strigosa under thermal stress, however, further studies are required to confirm this conclusion. We suggest that follow-up studies with this coral species consider larger fragment sizes (>5 cm in diameter) and longer periods for recovery from fragmentation and for acclimatization to laboratory conditions.
Implications of Results for AU Utilization and Further Considerations
In accordance with our study, pulsed natural upwelling has previously found to reduce heat stress and signs of coral bleaching during a large-scale bleaching event in Thailand (Wall et al., 2015; Schmidt et al., 2016). Large amplitude internal waves (LAIWs) deliver short-pulses of deep water into shallow reef areas of the Similan Islands (Andaman Sea, Thailand) with drops in temperature of up to 6–9°C for a period of 15–30 min (Schmidt et al., 2012). In a follow-up experiment, Buerger et al. (2015) simulated pulsed upwelling (2 pulses/day, 30 min/pulse, temperature drop of 5–6°C, pH drop 0.6 units), and found that the coral Porites lutea from both, exposed and sheltered reefs, experienced significantly less bleaching under upwelling conditions when exposed to thermal stress (2.3°C above average summer temperatures). These examples together with our study show that even short pulses of cold-water upwelling can substantially reduce thermal stress in corals during heat waves. One mechanism behind this could be reduced production and increased neutralization of cell damaging oxygen radicals during cool periods (Lesser, 1997). Pulsed AU, instead of sustained AU, is expected to be a preferable AU approach, since it likely reduces the risk of undesirable side effects (which, however, remains to be tested).
Undesirable side effects of AU on reefs, as well as on neighboring ecosystems, could be caused by a range of factors that are manipulated due to artificially mixing of two water bodies with different physical, chemical and biological properties. The magnitude of changes in water properties strongly depends on upwelling depth and intensity (upwelling rate and duration), as well as on the local conditions. In Bermuda, the euphotic zone extends deeper than 100 m, and the chemical properties of the water used for our experiment deviated little from the surface conditions. Therefore, the water taken at 50 and 100 m depth harbors relatively low risk of negative side effects of AU on corals and coral reefs, although we can not completely rule out the possibility that other parameters than temperatures have confounded the AU effects observed. Only chlorophyll-a was elevated substantially at 100 m depth as indicated by fluorescence in our study (Figure 1D) and as previously described (from <0.1 at surface to ∼0.4 μg kg–1 at 100 m depth; Steinberg et al., 2001). The influx of phytoplankton, depending on AU intensity, may lead to increased feeding and biomass of corals (Roder et al., 2010, 2011), which is considered beneficial for corals, in particular when photosynthetic energy supply decreases due to bleaching (Grottoli et al., 2006; Borell and Bischof, 2008; Buerger et al., 2015). However, sustained supply of plankton may lead to indirect negative effects on corals when it dies, since it can promote microbial activity that is potentially harmful (e.g., fostering the spread of benthic cyanobacteria mats; Brocke et al., 2015).
Upwelled water from a depth below the euphotic zone could cause substantial changes in the surface water chemistry, which might lead to negative effects overruling the benefits of cooling. More acidic water (lower pH, higher CO2, lower aragonite saturation state) is known to hamper coral growth (calcification) and to promote bioerosion (Schneider and Erez, 2006; Jokiel et al., 2008; Schmidt and Richter, 2013). While pulses of acidic deep water in Thailand did not show a measurable effect on P. lutea in a laboratory study (Buerger et al., 2015), it may be responsible (together with temperature reductions) for a generally lower coral growth at LAIW-exposed reefs (Schmidt and Richter, 2013). Hence, under a more continuous upwelling scenario, more acidic deep water could certainly impact coral growth. Furthermore, CO2-enrichment may foster (benthic) algal growth (Wu et al., 2008), and it could lead to higher CO2-flux into the atmosphere, thereby contributing to the greenhouse effect. Decreased oxygen concentrations of deep water could lead to low oxygen stress in particular in the night when corals and other reef photosynthesizers (algae) do not produce oxygen (Murphy and Richmond, 2016). Therefore, if AU is conducted with water from below the euphotic zone, it might be desirable to limit AU applications to the daytime only. Another reason for conducting AU during the daytime is that the most stressful situation for corals occurs when high temperature coincides with high light conditions (Mumby et al., 2001; Anthony et al., 2007).
Increased nutrient supply is usually known to be either neutral or beneficial for corals by increasing zooxanthellae densities and/or zooxanthellae pigmentation, which increases photosynthetic capacity and hence energy supply (Fabricius, 2005). Negative effects of nutrients, however, have been found in the reproductive success of corals (Loya et al., 2004) and in the thermal tolerance (e.g., Wooldridge, 2009). A decrease in thermal tolerance can be caused by an unbalanced nutrient supply (high nitrogen: phosphate ratio), where phosphate starvation produces symbionts with a low thermal tolerance (Wiedenmann et al., 2013; Ezzat et al., 2015). Furthermore, nutrient enrichment may lead to a reduced carbon translocation to the coral host, which can lead to energy shortage of the coral host in particular under thermal stress (Wooldridge, 2013). The lack of energy may consequently lead to a reduction of CO2 supply to the symbionts thereby triggering the expulsion of symbionts (Wooldridge, 2013). At an ecosystem level, nutrient enrichment can lead to changes in the benthic community structure, where faster growing algae may spatially outcompete slower growing corals, in particular when the abundance of grazers is low (Shulman and Robertson, 1996; Murphy and Richmond, 2016). Although periodic natural upwelling has been found to be an important source of nutrients in a number of reefs (e.g., Florida – Leichter et al., 2003; Galapagos – Riegl et al., 2018; Great Barrier Reef – Andrews and Gentien, 1982), in case of AU, it will be important to assess the natural nutrient dynamics, in order to determine the upper limits of potentially harmful nutrient concentrations.
The bathymetry and hydrology of a given location determines the accessibility of cooler deep water for AU in coral reefs. In Bermuda, the most extensive coral reefs occur at the rim of the Bermuda platform. The platform drops off quickly into depths below 200 m. Here, deep water is comparatively easy to access and a suitable depth for AU could be chosen based on seawater properties. In contrast, in the central Great Barrier Reef many coral reefs are located on a wide continental shelf that is 40-80 m deep. The shelf edge, and consequently the location of deep water, is up to 50 km away from many reefs. Furthermore, large parts of the shelf experience a rather homogenous temperature throughout the water column all year around (Furnas and Mitchell, 1986), which exempt these reefs from AU applications. However, it has been found that deep-water intrusions from the adjacent deep Coral Sea can sometimes occur far onto the shelf. They occur also during summer months and can persist for several weeks, although they do not necessarily reach the surface layers of reef waters (Andrews and Gentien, 1982; Furnas and Mitchell, 1986). In case these intrusions happen concomitantly with a heat wave, this water could be targeted for AU, although no selection for the “type” of water could be done. Therefore it would be important to carefully choose an appropriate AU intensity.
Conclusion
Our results along with previous studies on the effect of cold water upwelling or pulses during heat stress indicate that reductions of SST via AU could be an effective tool to provide temporal refuges for corals from thermal stress. We have shown, that even short intrusions of cooler deep water (<2 h per day) are likely to mitigate thermal stress as evident in enhanced zooxanthellae performance in corals exposed to heat and AU compared to corals exposed to heat only. This effect seemed stronger in AU simulations with water from 100 m depth (temperature reduction of 0.5°C per day; DHW 4.2°-weeks) than with water from 50 m depth (0.3°C per day; DHW 4.6°C-weeks), despite the small difference in DHW between treatments. This suggests that the absolute change in temperature during AU pulses might be a better predictor for heat stress response during pulsed AU than DHW (see also McClanahan et al., 2019). Our study also shows that the responses to AU were still comparatively small, therefore, an increase of AU duration or frequency may likely be required to mitigate heat stress and coral bleaching effectively. In order to avoid unwanted and unforeseeable side effects of AU caused by changes in other physical as well as chemical and biological parameters, pulsed AU instead of steady AU might be the desirable mode of application. While our results are promising, there are a number of studies that need to be conducted to advance our understanding on the benefits and risks of AU addressing ecological, engineering and societal aspects, before this can be applied on large scales in situ. Ecological aspects involve, but are not limited to (i) evaluating the effect of deep-water intrusions on reef communities rather than on individual corals, (ii) exploring the most effective and concomitantly least risky upwelling regime with respect to depth of water retrieval, volume of water upwelled, frequency and timing, (iii) assessing the effect of AU on plankton communities in the water column, (iv) evaluating the effect of AU on the hydrodynamics of reefs, and (v) assessing potential longer-term effects of AU on coral physiology (e.g., do AU induced temperature fluctuations promote higher thermal tolerance over time?).
Ocean warming and thus, the occurrence of heat waves will increase in frequency and intensity over the next decades and we will need to consider more permanent unconventional solutions to protect and sustain coral reefs. In future reef management strategies AU may be considered in combination with more conventional tools (strategies that aim to minimize local pressures such as overfishing and pollution) as well as other unconventional approaches like assisted evolution. For instance, AU and reef shading are both active ways to mitigate thermal stress and coral bleaching, while they may have different logistical constrains. Therefore, one or the other may be favorable at a given location. Traditional reef management strategies such as marine protected areas and fishing regulations are essential and will help to buffer future changes and minimize cumulative effects caused by pollution, overfishing and physical destruction. Yet we urgently need to carefully develop, test and assess unconventional approaches as novel management strategies, which are still far from being applied in the field at scale.
Data Availability Statement
All datasets generated for this study are included in the article/Supplementary Material.
Author Contributions
EF initiated the idea of the study. YS, EF, MW, and ML contributed to the conception and design of the study. YS and MH conducted the study and performed the data analysis. YS wrote the manuscript. All the authors contributed to manuscript revision, read and approved the submitted version.
Funding
This project was funded by the Cluster of Excellence “The Future Ocean” (CP1780), funded within the framework of the Excellence Initiative by the Deutsche Forschungsgemeinschaft (DFG, German Research Foundation). Deep-water retrieval was funded by the National Science Foundation (NSF) through BATS (OCE-1756105) and Hydrostation S (OCE-1633125) time series.
Conflict of Interest
The authors declare that the research was conducted in the absence of any commercial or financial relationships that could be construed as a potential conflict of interest.
Acknowledgments
We thank the BATS team in particular PI Dr. Rod Johnson for collecting the deep water during two cruises with the RV Atlantic Explorer as part of the ongoing BATS and Hydrostation S time series at BIOS. Furthermore, we thank the interns Hannah Lampit and Khalil Smith for assistance with coral collection and experimental maintenance.
Supplementary Material
The Supplementary Material for this article can be found online at: https://www.frontiersin.org/articles/10.3389/fmars.2020.00720/full#supplementary-material
Footnotes
References
Andrews, J., and Gentien, P. (1982). Upwelling as a source of nutrients for the Great Barrier Reef ecosystem: a solution to Darwin’s question? Mar. Ecol. Prog. Ser. 8, 257–269. doi: 10.3354/meps008257
Anthony, K., Bay, L. K., Costanza, R., Firn, J., Gunn, J., Harrison, P., et al. (2017). New interventions are needed to save coral reefs. Nat. Ecol. Evol. 1:1420.
Anthony, K. R., Connolly, S. R., and Hoegh-Guldberg, O. (2007). Bleaching, energetics, and coral mortality risk: effects of temperature, light, and sediment regime. Limnol. Oceanogr. 52, 716–726. doi: 10.4319/lo.2007.52.2.0716
Baker, A. C., Glynn, P. W., and Riegl, B. (2008). Climate change and coral reef bleaching: an ecological assessment of long-term impacts, recovery trends and future outlook. Estuar. Coast. Shelf Sci. 80, 435–471. doi: 10.1016/j.ecss.2008.09.003
Bates, N. R. (2017). Twenty years of marine carbon cycle observations at Devils Hole Bermuda provide insights into seasonal hypoxia, coral reef calcification, and ocean acidification. Front. Mar. Sci. 4:36. doi: 10.3389/fmars.2017.00036
Bayraktarov, E., Pizarro, V., Eidens, C., Wilke, T., and Wild, C. (2012). “Upwelling mitigates coral bleaching in the Colombian Caribbean,” in Proceedings of the 12th International Coral Reef Symposium, Cairns.
Borell, E. M., and Bischof, K. (2008). Feeding sustains photosynthetic quantum yield of a scleractinian coral during thermal stress. Oecologia 157, 593–601. doi: 10.1007/s00442-008-1102-2
Brandt, M. (2009). The effect of species and colony size on the bleaching response of reef-building corals in the Florida Keys during the 2005 mass bleaching event. Coral Reefs 28, 911–924. doi: 10.1007/s00338-009-0548-y
Brocke, H. J., Polerecky, L., de Beer, D., Weber, M., Claudet, J., and Nugues, M. M. (2015). Organic matter degradation drives benthic cyanobacterial mat abundance on Caribbean coral reefs. PLoS One 10:e0125445. doi: 10.1371/journal.pone.0125445
Budd, A. F., Fukami, H., Smith, N. D., and Knowlton, N. (2012). Taxonomic classification of the reef coral family Mussidae (Cnidaria: Anthozoa: Scleractinia). Zool. J. Linnean Soc. 166, 465–529. doi: 10.1111/j.1096-3642.2012.00855.x
Buerger, P., Schmidt, G., Wall, M., Held, C., and Richter, C. (2015). Temperature tolerance of the coral Porites lutea exposed to simulated large amplitude internal waves (LAIW). J. Exper. Mar. Biol. Ecol. 471, 232–239. doi: 10.1016/j.jembe.2015.06.014
Cacciapaglia, C., and Van Woesik, R. (2016). Climate-change refugia: shading reef corals by turbidity. Glob. Chang. Biol. 22, 1145–1154. doi: 10.1111/gcb.13166
Cai, W., Borlace, S., Lengaigne, M., van Rensch, P., Collins, M., Vecchi, G., et al. (2014). Increasing frequency of extreme El Niño events due to greenhouse warming. Nat. Clim. Chang. 4:111.
Chakravarti, L. J., Beltran, V. H., and van Oppen, M. J. (2017). Rapid thermal adaptation in photosymbionts of reef-building corals. Glob. Chang. Biol. 23, 4675–4688. doi: 10.1111/gcb.13702
Chollett, I., and Mumby, P. J. (2013). Reefs of last resort: locating and assessing thermal refugia in the wider Caribbean. Biol. Conserv. 167, 179–186. doi: 10.1016/j.biocon.2013.08.010
Chollett, I., Mumby, P. J., and Cortes, J. (2010). Upwelling areas do not guarantee refuge for coral reefs in a warming ocean. Mar. Ecol. Prog. Ser. 416, 47–56. doi: 10.3354/meps08775
Coelho, V., Fenner, D., Caruso, C., Bayles, B., Huang, Y., and Birkeland, C. (2017). Shading as a mitigation tool for coral bleaching in three common Indo-Pacific species. J. Exper. Mar. Biol. Ecol. 497, 152–163. doi: 10.1016/j.jembe.2017.09.016
Cook, C. B., Logan, A., Ward, J., Luckhurst, B., and Berg, C. J. (1990). Elevated temperatures and bleaching on a high latitude coral reef: the 1988 Bermuda event. Coral Reefs 9, 45–49. doi: 10.1007/bf00686721
Ezzat, L., Maguer, J. F., Grover, R., and Ferrier-Pagès, C. (2015). New insights into carbon acquisition and exchanges within the coral-dinoflagellate symbiosis under NH4+ and NO3- supply. Proc. R. Soc. Biol. Sci. 282:20150610. doi: 10.1098/rspb.2015.0610
Fabricius, K. E. (2005). Effects of terrestrial runoff on the ecology of corals and coral reefs: review and synthesis. Mar. Pollut. Bull. 50, 125–146. doi: 10.1016/j.marpolbul.2004.11.028
Fan, W., Pan, Y., Liu, C. C., Wiltshire, J. C., Chen-Tung, A. C., and Chen, Y. (2015). Hydrodynamic design of deep ocean water discharge for the creation of a nutrient-rich plume in the South China Sea. Ocean Eng. 108, 356–368. doi: 10.1016/j.oceaneng.2015.08.006
Furnas, M. J., and Mitchell, A. W. (1986). Phytoplankton dynamics in the central Great Barrier Reef—I. Seasonal changes in biomass and community structure and their relation to intrusive activity. Continent. Shelf Res. 6, 363–384. doi: 10.1016/0278-4343(86)90078-6
Gardner, S. G., Raina, J. B., Nitschke, M. R., Nielsen, D. A., Stat, M., Motti, C. A., et al. (2017). A multi-trait systems approach reveals a response cascade to bleaching in corals. BMC Biol. 15:117. doi: 10.1186/s12915-017-0459-2
Gattuso, J.-P., Magnan, A. K., Bopp, L., Cheung, W. W., Duarte, C. M., Hinkel, J., et al. (2018). Ocean solutions to address climate change and its effects on marine ecosystems. Front. Mar. Sci. 5:337. doi: 10.3389/fmars.2017.00337
Grottoli, A. G., Rodrigues, L. J., and Palardy, J. E. (2006). Heterotrophic plasticity and resilience in bleached corals. Nature 440, 1186–1189. doi: 10.1038/nature04565
Hansell, D. A., and Carlson, C. A. (2001). Biogeochemistry of total organic carbon and nitrogen in the Sargasso Sea: control by convective overturn. Deep Sea Res. Part II Top. Stud. Oceanogr. 48, 1649–1667. doi: 10.1016/s0967-0645(00)00153-3
Hennige, S. J., McGinley, M. P., Grottoli, A. G., and Warner, M. E. (2011). Photoinhibition of Symbiodinium spp. within the reef corals Montastraea faveolata and Porites astreoides: implications for coral bleaching. Mar. Biol. 158, 2515–2526. doi: 10.1007/s00227-011-1752-1
Heron, S. F., Eakin, C. M., Douvere, F., Anderson, K. L., Day, J. C., Geiger, E., et al. (2017). Impacts of Climate Change on World Heritage Coral Reefs: A First Global Scientific Assessment. Paris: UNESCO World Heritage Centre.
Hoogenboom, M., Beraud, E., and Ferrier-Pagès, C. (2010). Relationship between symbiont density and photosynthetic carbon acquisition in the temperate coral Cladocora caespitosa. Coral Reefs 29, 21–29. doi: 10.1007/s00338-009-0558-9
Hughes, T. P., Kerry, J. T., Álvarez-Noriega, M., Álvarez-Romero, J. G., Anderson, K. D., Baird, A. H., et al. (2017). Global warming and recurrent mass bleaching of corals. Nature 543, 373–377.
Hughes, T. P., Anderson, K. D., Connolly, S. R., Heron, S. F., Kerry, J. T., Lough, J. M., et al. (2018a). Spatial and temporal patterns of mass bleaching of corals in the Anthropocene. Science 359, 80–83. doi: 10.1126/science.aan8048
Hughes, T. P., Kerry, J. T., Baird, A. H., Connolly, S. R., Dietzel, A., Eakin, C. M., et al. (2018b). Global warming transforms coral reef assemblages. Nature 556, 492–496. doi: 10.1038/s41586-018-0041-2
ICPP (2014). Climate Change 2014: Synthesis Report. Contribution of Working Groups I, II and III to the Fifth Assessment Report of the Intergovernmental Panel on Climate Change. Geneva: IPCC.
Jackson, J., Donovan, M., Cramer, K., and Lam, V. (2014). Status and Trends of Caribbean Coral Reefs. Gland: Global Coral Reef Monitoring Network. Switzerland: IUCN. Available online at: http://cmsdata. iucn. org/downloads/caribbean_coral_reefs___status_report_1970_2012. pdf
Jiménez, C., Cortés, J., León, A., and Ruíz, E. (2001). Coral bleaching and mortality associated with the 1997-98 El Niño in an upwelling environment in the eastern Pacific (Gulf of Papagayo, Costa Rica). Bull. Mar. Sci. 69, 151–169.
Jokiel, P., Rodgers, K., Kuffner, I., Andersson, A., Cox, E., and Mackenzie, F. (2008). Ocean acidification and calcifying reef organisms: a mesocosm investigation. Coral Reefs 27, 473–483. doi: 10.1007/s00338-008-0380-9
Jones, R. J. (2008). Coral bleaching, bleaching-induced mortality, and the adaptive significance of the bleaching response. Mar. Biol. 154, 65–80. doi: 10.1007/s00227-007-0900-0
Kemp, D. W., Oakley, C. A., Thornhill, D. J., Newcomb, L. A., Schmidt, G. W., and Fitt, W. K. (2011). Catastrophic mortality on inshore coral reefs of the Florida Keys due to severe low-temperature stress. Glob. Chang. Biol. 17, 3468–3477. doi: 10.1111/j.1365-2486.2011.02487.x
Kirke, B. (2003). Enhancing fish stocks with wave-powered artificial upwelling. Ocean Coast. Manag. 46, 901–915. doi: 10.1016/s0964-5691(03)00067-x
Kravitz, B., Forster, P. M., Jones, A., Robock, A., Alterskjær, K., Boucher, O., et al. (2013). Sea spray geoengineering experiments in the geoengineering model intercomparison project (GeoMIP): Experimental design and preliminary results. J. Geophys. Res. Atmos. 118, 175–111.
Lalli, C., and Parsons, T. R. (1997). Biological Oceanography: An Introduction. Amsterdam: Elsevier.
Latham, J., Bower, K., Choularton, T., Coe, H., Connolly, P., Cooper, G., et al. (2012). Marine cloud brightening. Philos. Trans. R. Soc. A 370, 4217–4262.
Leichter, J. J., Stewart, H. L., and Miller, S. L. (2003). Episodic nutrient transport to Florida coral reefs. Limnol. Oceanogr. 48, 1394–1407. doi: 10.4319/lo.2003.48.4.1394
Lesser, M. P. (1997). Oxidative stress causes coral bleaching during exposure to elevated temperatures. Coral Reefs 16, 187–192. doi: 10.1007/s003380050073
Lesser, M. P. (2006). Oxidative stress in marine environments: biochemistry and physiological ecology. Annu. Rev. Physiol. 68, 253–278. doi: 10.1146/annurev.physiol.68.040104.110001
Liu, G., Strong, A. E., and Skirving, W. J. (2003). Remote sensing of sea surface temperature during 2002 Barrier Reef coral bleaching. EOS 84, 137–144.
Lovelock, J. E., and Rapley, C. G. (2007). Ocean pipes could help the Earth to cure itself. Nature 449:403. doi: 10.1038/449403a
Loya, Y., Lubinevsky, H., Rosenfeld, M., and Kramarsky-Winter, E. (2004). Nutrient enrichment caused by in situ fish farms at Eilat, Red Sea is detrimental to coral reproduction. Mar. Pollut. Bull. 49, 344–353. doi: 10.1016/j.marpolbul.2004.06.011
McClanahan, T. R., Darling, E. S., Maina, J. M., Muthiga, N. A., D’agata, S., Jupiter, S. D., et al. (2019). Temperature patterns and mechanisms influencing coral bleaching during the 2016 El Niño. Nat. Clim. Chang. 9, 845–851. doi: 10.1038/s41558-019-0576-8
McCook, L. J. (1999). Macroalgae, nutrients and phase shifts on coral reefs: scientific issues and management consequences for the Great Barrier Reef. Coral Reefs 18, 357–367. doi: 10.1007/s003380050213
Miller, J., Muller, E., Rogers, C., Waara, R., Atkinson, A., Whelan, K., et al. (2009). Coral disease following massive bleaching in 2005 causes 60% decline in coral cover on reefs in the US Virgin Islands. Coral Reefs 28:925. doi: 10.1007/s00338-009-0531-7
Mumby, P. J., Chisholm, J. R., Edwards, A. J., Andrefouet, S., and Jaubert, J. (2001). Cloudy weather may have saved Society Island reef corals during the 1998 ENSO event. Mar. Ecol. Prog. Ser. 222, 209–216. doi: 10.3354/meps222209
Murphy, J. W. A., and Richmond, R. H. (2016). Changes to coral health and metabolic activity under oxygen deprivation. PeerJ 4:e1956. doi: 10.7717/peerj.1956
National Academics of Sciences, Engineering, and Medicine [NAS] (2019). Ocean Studies Board Committee on Interventions to Increase the Resilience of Coral Reefs. A Decision Framework for Interventions to Increase the Persistence and Resilience of Coral Reefs. Washington, DC: The National Academies Press.
NOAA Coral Reef Watch, (2018). Status Report. Available at: https://coralreefwatch.noaa.gov/satellite/analyses_guidance/global_coral_bleaching_2014-17_status.php (accessed August 2019).
Oschlies, A., Pahlow, M., Yool, A., and Matear, R. J. (2010). Climate engineering by artificial ocean upwelling: channelling the sorcerer’s apprentice. Geophys. Res. Lett. 37:961.
Pan, Y., Fan, W., Huang, T.-H., Wang, S.-L., and Chen, C.-T. A. (2015). Evaluation of the sinks and sources of atmospheric CO2 by artificial upwelling. Sci. Total Environ. 511, 692–702. doi: 10.1016/j.scitotenv.2014.11.060
Pan, Y., Fan, W., Zhang, D., Chen, J., Huang, H., Liu, S., et al. (2016). Research progress in artificial upwelling and its potential environmental effects. Sci. China Earth Sci. 59, 236–248. doi: 10.1007/s11430-015-5195-2
Parsons, T., Maita, Y., and Lalli, C. (1984). “Determination of chlorophylls and total carotenoids: spectrophotometric method,” in A Manual of Chemical and Biological Methods for Seawater Analysis, eds T. R. Parsons, Y. Maita, and C. M. Lalli, (Oxford: Pergamon Press), 101–112.
Pernice, M., Raina, J., Rädecker, N., Cárdenas, A., Pogoreutz, C., and Voolstra, C. R. (2020). Down to the bone: the role of overlooked endolithic microbiomes in reef coral health. ISME J. 14, 325–334. doi: 10.1038/s41396-019-0548-z
Rau, G. H., McLeod, E. L., and Hoegh-Guldberg, O. (2012). The need for new ocean conservation strategies in a high-carbon dioxide world. Nat. Clim. Chang. 2, 720–724. doi: 10.1038/nclimate1555
Riegl, B., Glynn, P. W., Banks, S., Keith, I., Rivera, F., Vera-Zambrano, M., et al. (2018). Heat attenuation and nutrient delivery by localized upwelling avoided coral bleaching mortality in northern Galapagos during 2015/2016 ENSO. Coral Reefs 38, 1–13.
Roder, C., Fillinger, L., Jantzen, C., Schmidt, G., Khokiattiwong, S., and Richter, C. (2010). Trophic response of corals to large amplitudal internal waves. Mar. Ecol. Prog. Ser. 412, 113–128. doi: 10.3354/meps08707
Roder, C., Jantzen, C., Schmidt, G., Kattner, G., Phongsuwan, N., and Richter, C. (2011). Metabolic plasticity of the corals Porites lutea and Diploastrea heliopora exposed to large amplitude internal waves. Coral Reefs 30, 57–69. doi: 10.1007/s00338-011-0722-x
Sawall, Y., Al-Sofyani, A., Banguera-Hinestroza, E., and Voolstra, C. (2014). Spatio-temporal analyses of zooxanthellae physiology of the coral Pocillopora verrucosa along large-scale nutrient and temperature gradients in the Red Sea. PLoS One 9:e103179. doi: 10.1371/journal.pone.103179
Saxby, T., Dennison, W. C., and Hoegh-Guldberg, O. (2003). Photosynthetic responses of the coral Montipora digitata to cold temperature stress. Mar. Ecol. Prog. Ser. 248, 85–97. doi: 10.3354/meps248085
Schmidt, G. M., Phongsuwan, N., Jantzen, C., Roder, C., Khokiattiwong, S., and Richter, C. (2012). Coral community composition and reef development at the Similan Islands, Andaman Sea, in response to strong environmental variations. Mar. Ecol. Prog. Ser. 456, 113–126. doi: 10.3354/meps09682
Schmidt, G. M., and Richter, C. (2013). Coral growth and bioerosion of Porites lutea in response to large amplitude internal waves. PLoS One 8:e73236. doi: 10.1371/journal.pone.073236
Schmidt, G. M., Wall, M., Taylor, M., Jantzen, C., and Richter, C. (2016). Large-amplitude internal waves sustain coral health during thermal stress. Coral Reefs 35, 869–881. doi: 10.1007/s00338-016-1450-z
Schneider, K., and Erez, J. (2006). The effect of carbonate chemistry on calcification and photosynthesis in the hermatypic coral Acropora eurystoma. Limnol. Oceanogr. 51, 1284–1293. doi: 10.4319/lo.2006.51.3.1284
Shulman, M. J., and Robertson, D. R. (1996). Changes in the coral reefs of San Blas, Caribbean Panama: 1983 to 1990. Coral Reefs 15, 231–236. doi: 10.1007/s003380050048
Smith, S. R., Sarkis, S., Murdoch, T. J., Weil, E., Croquer, A., Bates, N. R., et al. (2013). “Threats to coral reefs of Bermuda,” in Coral Reefs of the United Kingdom Overseas Territories, ed. C. R. C. Sheppard, (Cham: Springer), 173–188. doi: 10.1007/978-94-007-5965-7_13
Steinberg, D. K., Carlson, C. A., Bates, N. R., Johnson, R. J., Michaels, A. F., and Knap, A. H. (2001). Overview of the US JGOFS Bermuda Atlantic Time-series Study (BATS): a decade-scale look at ocean biology and biogeochemistry. Deep Sea Res. Part II Top. Stud. Oceanogr. 48, 1405–1447. doi: 10.1016/s0967-0645(00)00148-x
Strong, A. E., Barrientos, C. B., Duda, C., and Sapper, J. (1997). “Improved satellite techniques for monitoring coral reef bleaching,” in Procedings of the 8th International Coral Reef Symposium, Panama City, FL.
Thornhill, D. J., Rotjan, R. D., Todd, B. D., Chilcoat, G. C., Iglesias-Prieto, R., Kemp, D. W., et al. (2011). A connection between colony biomass and death in Caribbean reef-building corals. PLoS One 6:e29535. doi: 10.1371/journal.pone.029535
van Hooidonk, R. J., Manzello, D. P., Moye, J., Brandt, M. E., Hendee, J. C., McCoy, C., et al. (2012). Coral bleaching at little cayman, Cayman Islands 2009. Estuar. Coas. Shelf Sci. 106, 80–84. doi: 10.1016/j.ecss.2012.04.021
Van Oppen, M. J., Gates, R. D., Blackall, L. L., Cantin, N., Chakravarti, L. J., Chan, W. Y., et al. (2017). Shifting paradigms in restoration of the world’s coral reefs. Glob Chang. Biol. 23, 3437–3448.
van Oppen, M. J., Oliver, J. K., Putnam, H. M., and Gates, R. D. (2015). Building coral reef resilience through assisted evolution. Proc. Natl. Acad. Sci. U.S.A. 112, 2307–2313. doi: 10.1073/pnas.1422301112
Viúdez, Á, Balsells, M. F.-P., and Rodríguez-Marroyo, R. (2016). Artificial upwelling using offshore wind energy for mariculture applications. Sci. Mar. 80, 235–248. doi: 10.3989/scimar.04297.06b
Wall, M., Putchim, L., Schmidt, G., Jantzen, C., Khokiattiwong, S., and Richter, C. (2015). Large-amplitude internal waves benefit corals during thermal stress. Proc. R. Soc. Lond. B Biol. Sci. 282:20140650. doi: 10.1098/rspb.2014.0650
Warner, M. E., Fitt, W. K., and Schmidt, G. W. (1999). Damage to photosystem II in symbiotic dinoflagellates: a determinant of coral bleaching. Proc. Natl. Acad. Sci. U.S.A. 96, 8007–8012. doi: 10.1073/pnas.96.14.8007
Weis, V. M. (2008). Cellular mechanisms of Cnidarian bleaching: stress causes the collapse of symbiosis. J. Exper. Biol. 211, 3059–3066. doi: 10.1242/jeb.009597
Wiedenmann, J., D’Angelo, C., Smith, E. G., Hunt, A. N., Legiret, F.-E., Postle, A. D., et al. (2013). Nutrient enrichment can increase the susceptibility of reef corals to bleaching. Nat. Clim. Chang. 3, 160–164. doi: 10.1038/nclimate1661
Williamson, N., Komiya, A., Maruyama, S., Behnia, M., and Armfield, S. W. (2009). Nutrient transport from an artificial upwelling of deep sea water. J. Oceanogr. 65, 349–359. doi: 10.1007/s10872-009-0032-x
Wooldridge, S. A. (2009). Water quality and coral bleaching thresholds: formalising the linkage for the inshore reefs of the Great Barrier Reef, Australia. Mar. Pollut. Bull. 58, 745–751. doi: 10.1016/j.marpolbul.2008.12.013
Wooldridge, S. A. (2013). Breakdown of the coral-algae symbiosis: towards formalising a linkage between warm-water bleaching thresholds and the growth rate of the intracellular zooxanthellae. Biogeosciences 10, 1647–1658. doi: 10.5194/bg-10-1647-2013
Wu, H., Zou, D., and Gao, K. (2008). Impacts of increased atmospheric CO2 concentration on photosynthesis and growth of micro- and macro-algae. Sci. China Ser. C Life Sci. 51, 1144–1150. doi: 10.1007/s11427-008-0142-5
Keywords: coral bleaching, bleaching mitigation, artificial upwelling, pulsed upwelling, global warming, sea surface temperature rise, heat waves
Citation: Sawall Y, Harris M, Lebrato M, Wall M and Feng EY (2020) Discrete Pulses of Cooler Deep Water Can Decelerate Coral Bleaching During Thermal Stress: Implications for Artificial Upwelling During Heat Stress Events. Front. Mar. Sci. 7:720. doi: 10.3389/fmars.2020.00720
Received: 02 October 2019; Accepted: 06 August 2020;
Published: 28 August 2020.
Edited by:
Hajime Kayanne, The University of Tokyo, JapanReviewed by:
Gang Liu, National Oceanic and Atmospheric Administration (NOAA), United StatesDouglas Fenner, Independent Researcher, Pago Pago, American Samoa
Ines D. Lange, University of Exeter, United Kingdom
Copyright © 2020 Sawall, Harris, Lebrato, Wall and Feng. This is an open-access article distributed under the terms of the Creative Commons Attribution License (CC BY). The use, distribution or reproduction in other forums is permitted, provided the original author(s) and the copyright owner(s) are credited and that the original publication in this journal is cited, in accordance with accepted academic practice. No use, distribution or reproduction is permitted which does not comply with these terms.
*Correspondence: Yvonne Sawall, eXZvbm5lLnNhd2FsbEBiaW9zLmVkdQ==; Ellias Yuming Feng, eWZlbmdAZ2VvbWFyLmRl