- 1Jellagen Limited, Cardiff, United Kingdom
- 2Censo Biotechnologies Ltd., Cambridge, United Kingdom
Accurate disease models are essential for understanding disease pathogenesis and for developing new therapeutics. As stem cells are capable of self-renewal and differentiation, they are ideally suited both for generating these models and for obtaining the large quantities of cells required for drug development and transplantation therapies. Jellyfish collagen is showing great promise as a next generation matrix enabling improved outcomes in 2D and 3D cell culture and regenerative medicine. Here, we report the potential of jellyfish collagen for culturing induced pluripotent stem cell derived cell lines (iPSCs) for modeling human diseases. Jellyfish collagen from Rhizostoma pulmo (Jellagen®) was evaluated for the growth and viability of iPSC-derived microglial-like cells (iMGL) comparing to cells cultured on rat tail collagen 1, laminin-511 and tissue culture plastic. Viability was measured using MTT, XTT, Alamar Blue and Annexin V, since this last assay has the aim of evaluating the onset of apoptosis. Cell ramification was measured using Neurotracker software and ramification measured on the InCucyte S3TM. Cell surface receptor expression was quantified using flow cytometry. Microglia markers used for immunocytochemistry were IBA1, CD11b, TREM2, TMEM119, and P2RY12. We report that iPSC-derived microglia can be successfully cultured on Jellagen® jellyfish collagen demonstrating a more ramified cell morphology compared to cells cultured on mammalian rat tail collagen I and comparable to Laminin-511.
Introduction
Microglia are the innate immune cells of the Central Nervous System (CNS) and play important roles in synaptic plasticity, immune activity, neurogenesis and homeostasis. Microglia are known to be involved in neurological disorders such as Alzheimer’s Disease (AD), yet the study of microglia is proving challenging due to the technical and ethical hurdles of obtaining primary cells from human fetal or adult CNS tissue (Abud et al., 2017). This is leading to a growing requirement to develop alternative sources of human microglia models, such as those derived from induced pluripotent stem cells (iPSCs) (Ryan et al., 2017). Lineage tracing studies have shown that microglia originate from yolk sac erythromyeloid progenitors (EMP) generated during primitive hematopoiesis (Ginhoux et al., 2010; Kierdorf et al., 2013; Prinz and Priller, 2014). EMPs further differentiate into primitive macrophages that migrate into the developing neural tube to become microglial progenitors (Dong et al., 2019). These microglial progenitors mature and develop into tissue resident microglia where they develop ramified processes enabling them to sense and survey their environment, facilitate CNS development, respond to CNS injury and pathology. Generation of microglia from iPSC is complex partly due to their unique developmental origin, however, recent advances have enabled the robust generation of microglia in large numbers, a development essential for the use of these cells in drug discovery (Leitinger and Hohenester, 2007; Figuera-Losadaab et al., 2017). It is therefore essential to understand and test matrices on which cells are regularly cultured to be able to develop robust in vitro cell culture systems.
Collagen is considered to be a good material for the culture and proliferation of cells (Sorushanova et al., 2019). It is the most ubiquitous protein in the mammalian proteome, comprising up to 30% of all proteins. Collagen can stimulate cell attachment and intracellular communication between some cell types (Greegburg et al., 1981; Lee et al., 2001), making it an ideal cell culture research tool. Collagen forms a large part of the extracellular matrix and connective tissue, offering strength and flexibility to tissues in the body. Besides its role in mechanical strength, collagen functions as a signaling molecule, regulating cellular migration (Bosnakovski et al., 2006), differentiation (Pozzi et al., 1998) and proliferation (Farndale et al., 2004) and functions in haemostasis (Silvipriya et al., 2015).
Collagen for cell culture is generally derived from rat tail or from bovine sources. However, during the last 20 years, safety concerns of using collagens derived from mammalian sources is increasing due to perceived risks associated with bovine spongiform encephalopathy (BSE) and viral disease transfer (Browne et al., 2013). This is driving the search for safer alternatives including the production of recombinant collagens in different host models such as bacteria, mouse milk, plants and yeast (Yang et al., 2004). This is not the case with invertebrate collagens which have been shown to share the same characteristics to human collagen leading to new industrial prospects that avoids the use of mammalian tissues and takes into account the sustainable management of natural wastes and ecological problems (Addad et al., 2011).
Jellyfish collagen is an emerging alternative to the provision of collagen for routine cell culture and medical device research (Silva et al., 2014). Jellyfish are often referred to as gelatinous zooplankton made up of collagen rich mesogloea that evolved over 600 m years ago. This makes JFC an interesting molecule for cell culture study. Previous reports have demonstrated the potential of JFC for the culture of human cells in vitro (Song et al., 2006; Addad et al., 2011; Paradiso et al., 2019). Jellyfish collagen when compared against mammalian fibrillar collagen in cell cytotoxicity and cell adhesion assays, showed no statistical difference in cytotoxicity. Human cell adhesion to jellyfish collagen also confirmed that their biological effect on human cells are compatible to that of mammalian type I and type II collagens (Hoyer et al., 2014).
Further studies supporting the potential of jellyfish collagen’s biocompatibility have been conducted that included cytotoxicity tests, measurements of pro-inflammatory cytokine secretion, antibody secretion as well as the population change of immune cells after in vivo implantation (Song et al., 2006). In their study, Song and colleagues found that the number of dendritic cells (CD11c+) and macrophages (F4/80+) were similar in jellyfish collagen implanted into mice as to those of bovine- and gelatin-implanted mice. Hence, they concluded that the jellyfish collagen scaffolds were able to induce a comparable immune response to that caused by bovine collagen or gelatin (Song et al., 2006).
Given the bioavailability of jellyfish collagen and its biological properties, this marine material represents a good candidate for replacing rat tail and bovine or human collagens in selected cell culture applications. Studies have also demonstrated that jellyfish collagen also presents a comparable biological impact on human cells to mammalian type I collagen tested by cytotoxicity and adhesion assays (Pugliano et al., 2017). Further investigations on the mechanisms of cell interaction indicates that both integrins and heparan-sulfate receptors of human cells are able to recognize jellyfish collagen (Pustlauk et al., 2015). Moreover, cells were able to form focal adhesions similar to that observed on mammalian collagens, when they plated on jellyfish collagen.
In this study, we report the comparison of Jellagen® a jellyfish collagen derived from R. pulmo (JC) with other materials to assess their impact on the culture iMGL. Our aim was to assess the suitability of JC as a robust alternative matrix for iMGL cell culture survival and conservation of phenotype and function in comparison with iMGL cells cultured on rat tail vertebrate collagen type I (RTC), tissue culture treated plastic and Laminin-511.
Materials and Methods
Jellyfish Collagen
Acid soluble jellyfish collagen (Jellagen® BT10-01-34-46) was manufactured by Jellagen Limited using internal manufacturing processes.
iPSC-Derived Microglia Differentiation
Differentiation of the “healthy” iPSC line UKBi005-A to macrophage progenitor cells was initiated by mesoderm induction created on a protocol that is based on previously described methods (Abud et al., 2017). Briefly, hemangioblasts were formed and cultured in macrophage media then macrophage progenitor cells were released into the media after 10–14 days. Macrophage progenitors were assessed for the markers CD14 (ImmunoTools Cat. No. 21279143), CD16 (ImmunoTools Cat. No. 21279166) and CD11b (ImmunoTools Cat. No. 21279113) using flow cytometry (Millipore Guava) with a pass threshold of > 90% expression of these marker required to pass quality control. These cells were then matured to iMGL over a period of 7 days in microglia maturation media according to internal protocols of Censo Biotechnologies Ltd.
Coating Methods
Jellyfish collagen (Jellagen® BT10-01-34-46) and rat tail collagen (Cat. no. 3867, lot: SLBS8676, Sigma) were diluted using tissue culture grade water to 100, 50, 10, 5, and 1 μg/mL and used to coat 96-well plates following manufacturers specifications. Controls used were PDL/laminin (Sigma L2020; 10 μg/mL) and uncoated TC plastic. Macrophage progenitor cells were matured over 7 days to microglia-like cells, which were harvested and seeded on pre-coated plates at a density of 100,000 cells/cm2, and cultured for up to 72 h prior to assessing viability, phenotype and function of the cells.
Cell Viability Assays
MTT Assay: 100 μL of 0.5 mg/mL MTT solution (Sigma M2003), diluted in basal media, was added to each well and incubated at 37°C for 4 h. MTT was then removed and 50 μL DMSO added to each well prior to reading absorbance values at 570 nm.
XTT Assay: 1 mg/mL of XTT solution was combined with phenazine methosulfate (PMS – 200 μg/mL) by adding 40 μL of PMS per 1 mL of XTT. Once combined, 50 μL was added to each well of cells without removing the media and incubated at 37°C for 1 h. Absorbance was read at 450 nm. PMS acts as an intermediate electron carrier which helps reduction of XTT and allows for a more sensitive assay. This assay measures cell metabolic activity similar to MTT.
Alamar Blue: Alamar blue was added to each well at 10% of the volume of media in each well (e.g., 10 μL of alarm blue added to 90 μL of media) and incubated at 37°C for 4 h. Absorbance was recorded at 570 and 600 nm, where the value at 600 nm was subtracted from 570 nm. Alamar blue is a reazurin dye which is irreversibly reduced to pink resorufin by live cells.
Annexin V/7AAD
Annexin V and 7-AAD were added to PBS (5 μL of each/100 μL PBS) with DAPI. Media was removed from cells and 50 μL of Annexin V/7-AAD/DAPI solution added per well prior to incubating at room temperature (RT) in the dark for 10 min and then washing in PBS. Staining was quantified using a ThermoFisher ArrayScanTM and data recorded as percentage of cells positive for Annexin V, 7-AAD or double positive. As a positive control of cell death, 10 μM staurosporine was added to positive control wells 2 h prior to performing assay.
Cell Characterization
A concentration of 10 μg/mL for both JFC and rat tail collagen (RTC) was selected to further evaluate cells. Control substrate used throughout the project were Laminin (10 μg/mL) and tissue culture (TC) plastic. Microglia were matured for 7 days, then re-plated on JFC, RTC, laminin or TC plastic for 48 h and cells characterized based on morphology and cell surface receptor expression using imaging and flow cytometry. Cytokine production was measured to establish whether iMGL were activated by any of the substrates tested. Morphology of iMGL was assessed using the InCucyte S3TM with images captured every hour for 24 h. iMGL ramification of cells cultured in each condition was quantified using the InCucyte S3TM neurite tracking module.
Immunocytochemistry
Immunocytochemistry was used to label specific proteins and images taken using the ThermoFisher ArrayScanTM. Microglia markers used were IBA1, CD11b, TREM2, TMEM119 and P2RY12. Cells were fixed in 4% PFA for 20 min, washed in PBS and incubated with appropriate antibodies and DAPI prior to imaging.
Flow Cytometry
Cell surface receptor expression was quantified using flow cytometry (Millipore Guava) on live cells. Cells were blocked in flow buffer (1% FBS + 10 μg/mL human serum IgG) prior to staining for relevant antibodies for 1 h (CD14, CD16, CD11b, HLA-DR, and CD206). Samples were washed and evaluated using a Millipore Guava easyCyte flow cytometer, and 5000 events measured per sample. Cell population was gated to exclude debris and isotype controls used to gate a positive population. Thresholds were set where isotypes showed < 5% positive staining. All data is shown as percent positive expression.
ELISA
For evaluation of cytokine output, cells were cultured on the different matrices for 48 h, microglia were then stimulated with lipopolysaccharide (LPS 100 ng/mL) or INFY (10 ng/mL) for a further 24 h prior to collecting supernatants to be used in ELISA (IL-6 and TNFα – R&D kit).
Statistical Tests
No statistical tests were performed as the data is generated from one repeat with several technical repeats and would therefore not be suitable to perform stats on.
Results and Discussion
The Effect of Cell Culture Matrices on the Viability and Morphology of iMGL
The aim of this study was to investigate if jellyfish collagen could act as a potential alternative to the routine use of existing cell culture matrices for the improved culture of iPSC derived microglia. Cell viability was assessed by performing MTT, XTT, and Alamar Blue assays and an apoptosis assay (Annexin V).
In all cases, data showed that jellyfish collagen (JC) had no effect on cell viability or apoptosis of iMGL when compared to laminin or TC plastic controls (see Figures 1–4). Rat tail collagen (RTC) appeared to decrease cell viability (and showed an increase in Annexin V cell surface expression as well as a decrease in mitochondrial metabolism (MTT) suggesting a decrease in cell viability on this substrate compared to others tested. Viability of cells was confirmed visually by capturing stained images under phase contrast (Figure 1), as well as by quantifying ramification of iMGL using the InCucyte S3TM an indicator of robust microglial morphology (Figure 5). Solid evidence from diverse cell culture studies have shown the potential of jellyfish collagen to successfully culture a wide range of human cell types (Paradiso et al., 2019). In this study, we have successfully demonstrated that jellyfish collagen can also be used as a substrate for the culture of human iPSC-derived Microglia (iMGL) that when cultured, display the morphological, surface marker expression and functional characteristics expected from microglia.
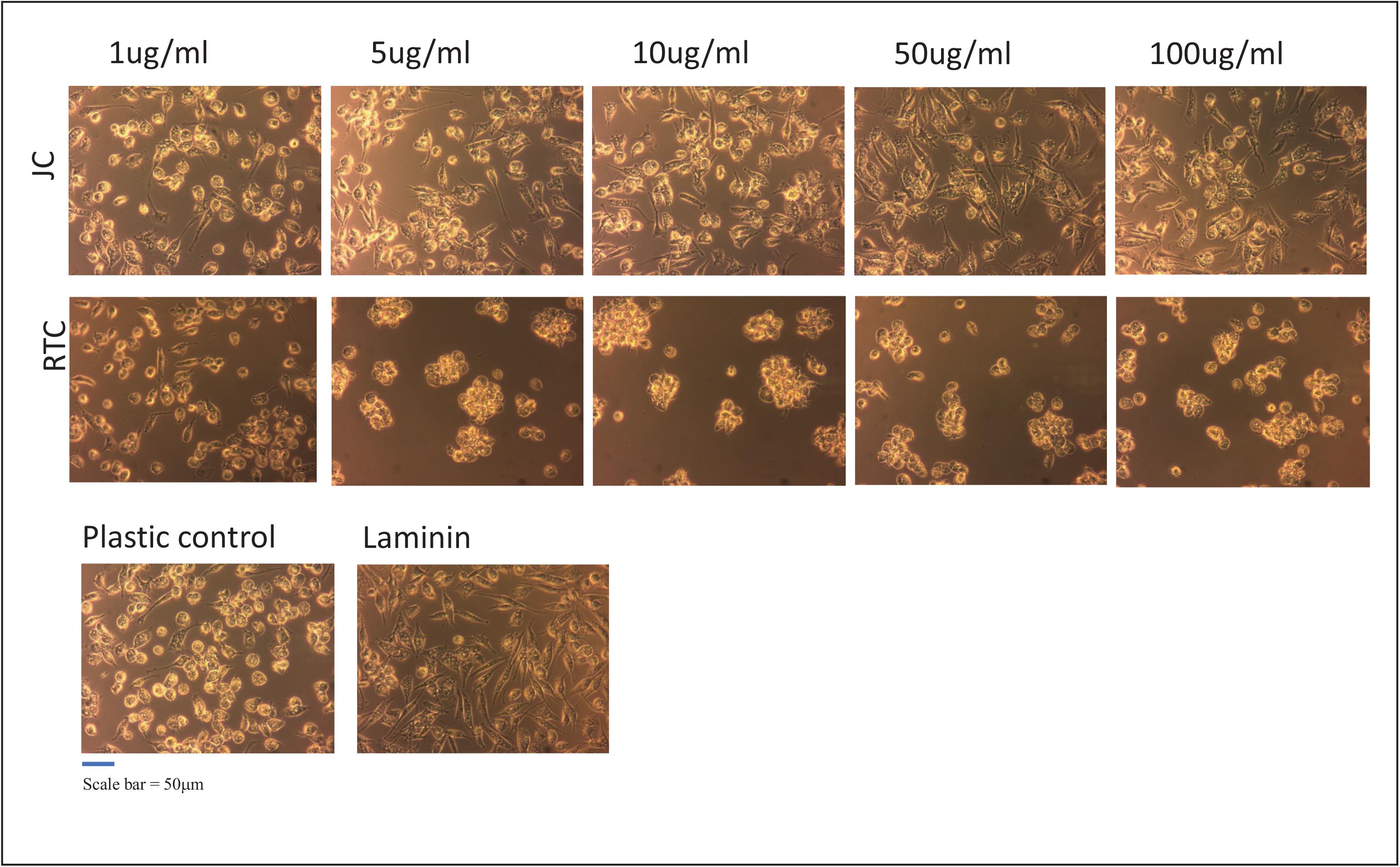
Figure 1. iMGL were cultured on different coating concentrations of Jellagen®. jellyfish collagen (JC), rat tail collagen I (RTC) (1–100 μg/mL), Laminin or Plastic Control (TC plastic) for up to 72 h and morphology observed by phase-contrast imaging at 72 h culture. Scale bar = 50 μm. Images taken at x10 magnification.
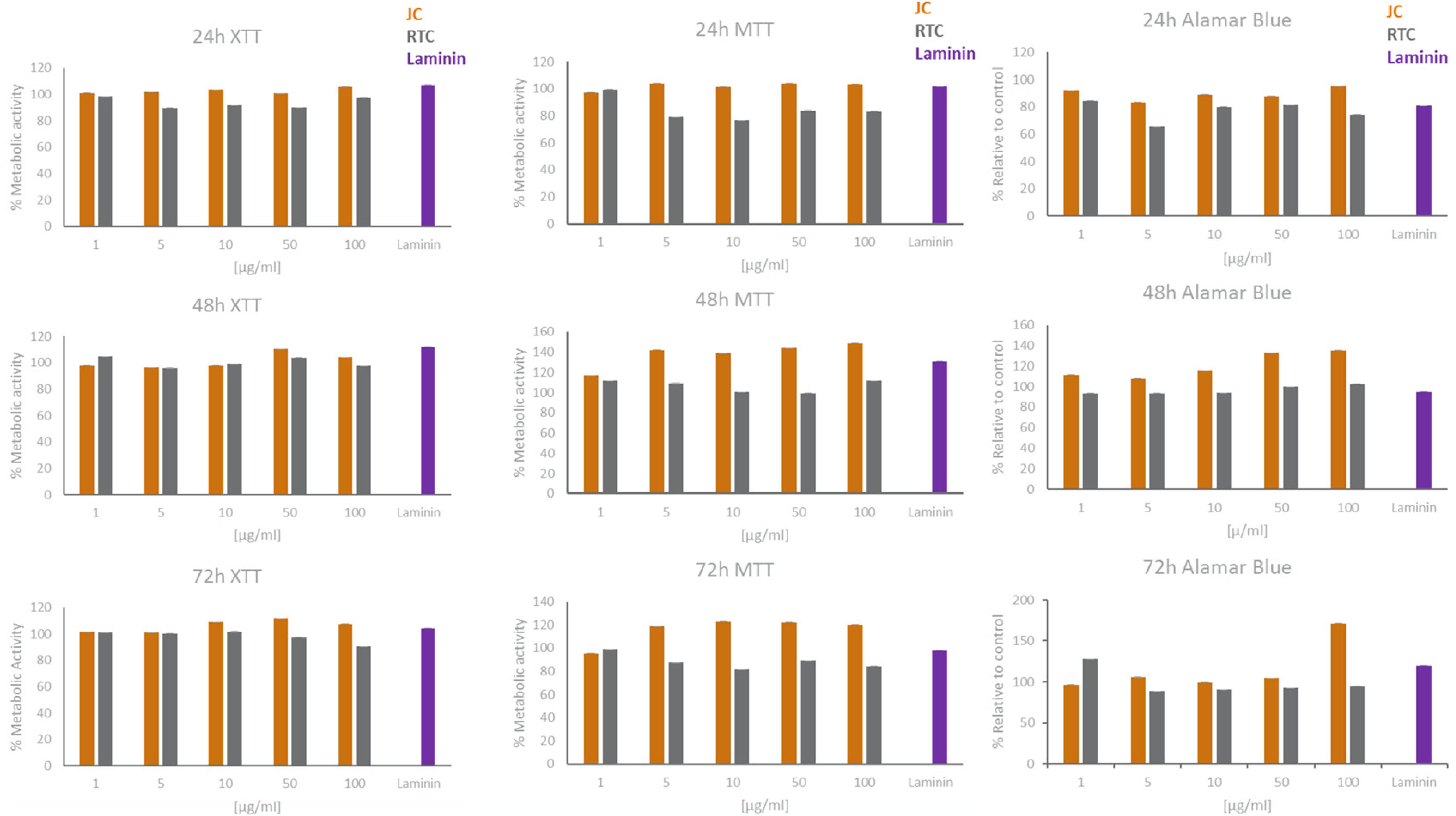
Figure 2. XTT, MTT, and Alamar Blue cell viability results for microglia cultured in different concentrations of JC, RTC, laminin or plastic for 24 h, 48 h or 72 h. All data are normalised to plastic control which is set to 100% metabolic activity. Data represent mean of 3 technical repeats ± SEM.
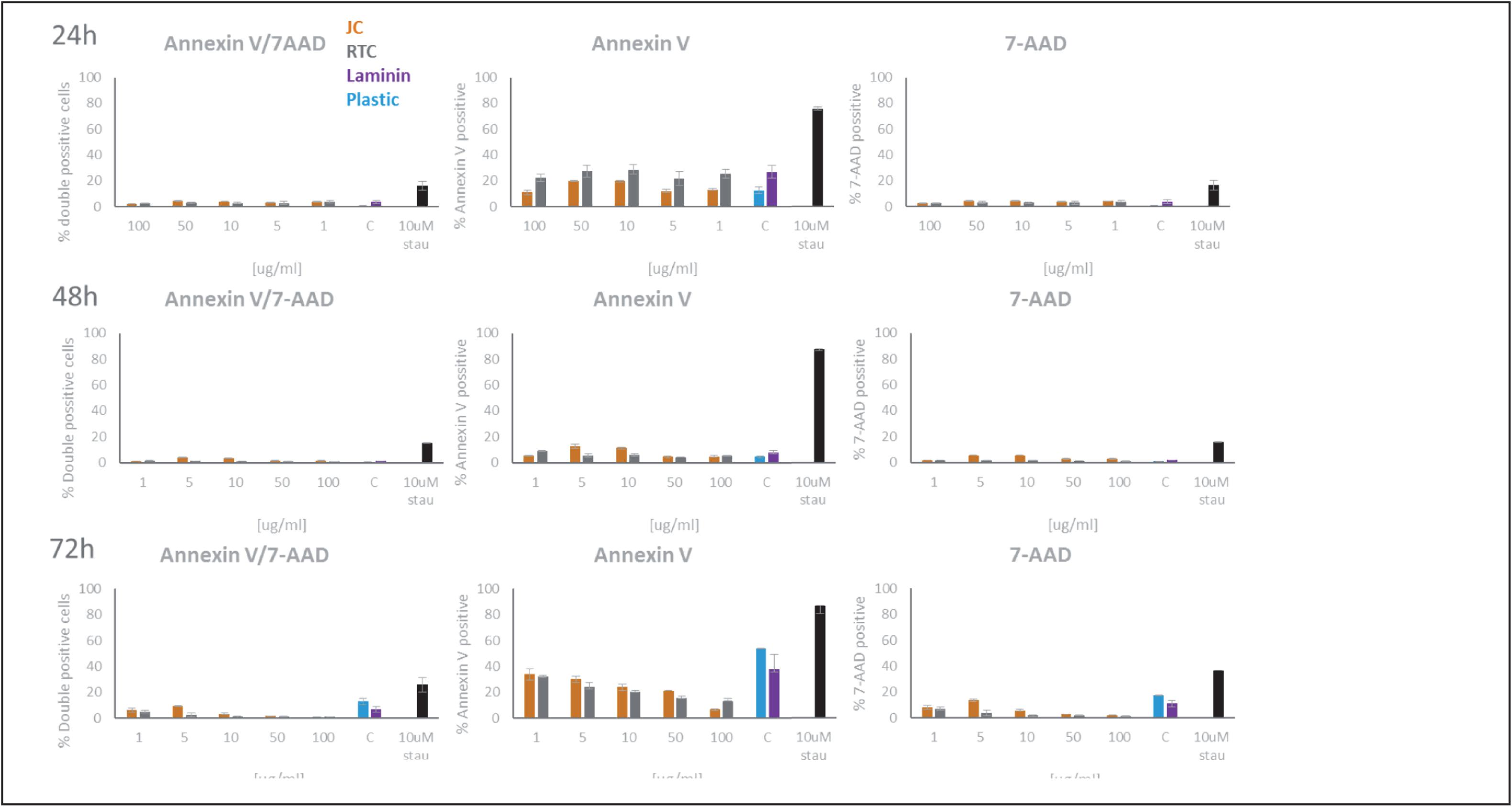
Figure 3. Quantification of Annexin V and 7-AAD staining of microglia following culture on JC, RTC, laminin, or plastic for 24, 48, or 72 h. A positive control of cell death was included by incubating cells with 10 μM Staurosporine for 2 h prior to staining. Data shown are the mean of 3 technical repeats ± SEM.
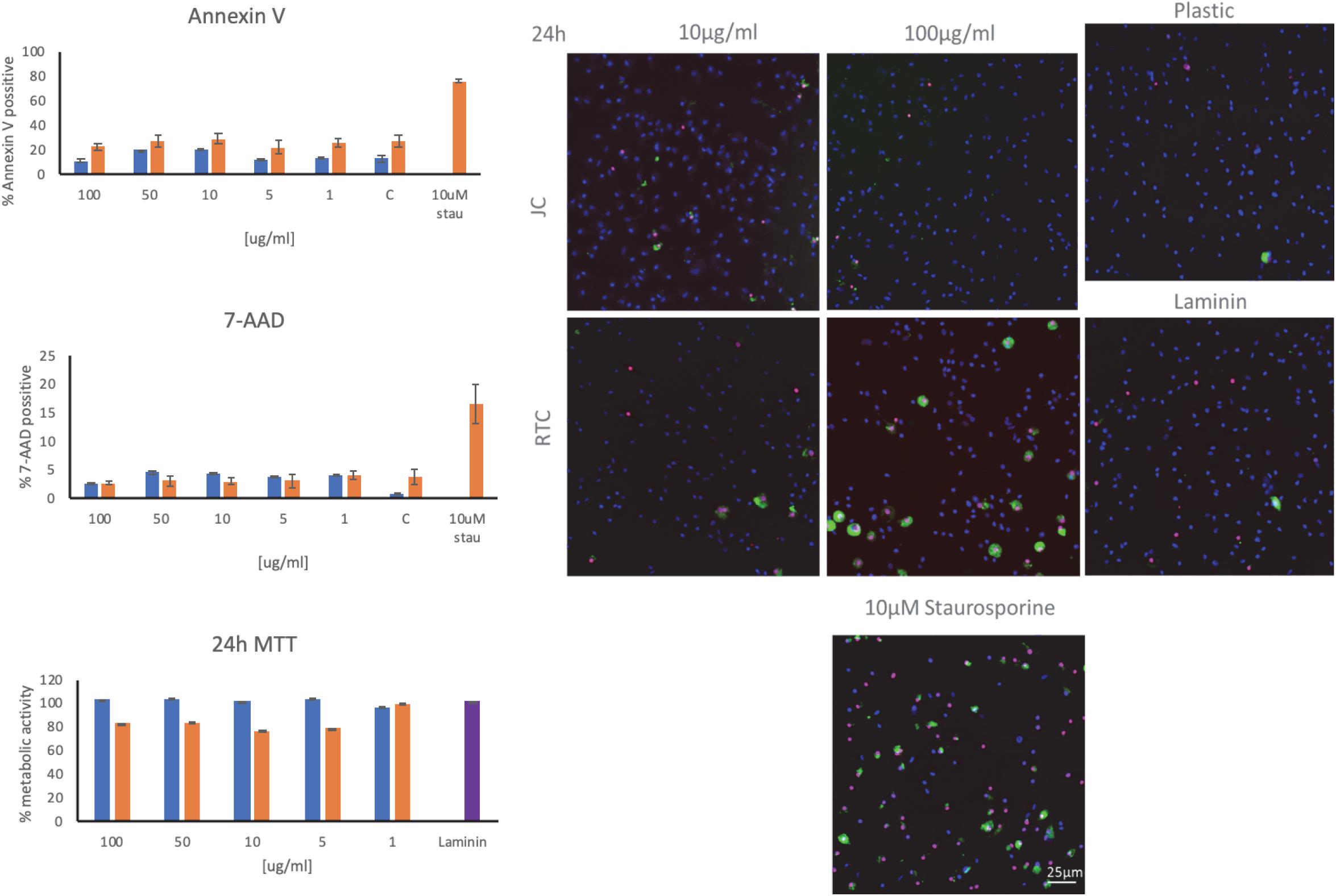
Figure 4. Staining of microglia using annexin V and 7-AAD as markers of apoptosis following culture of microglia on JC, RTC, laminin, or plastic for 24 h. A positive control of cell death was included by incubating cells with 10 μM Staurosporine for 2 h prior to staining. Blue: DAPI, Green.
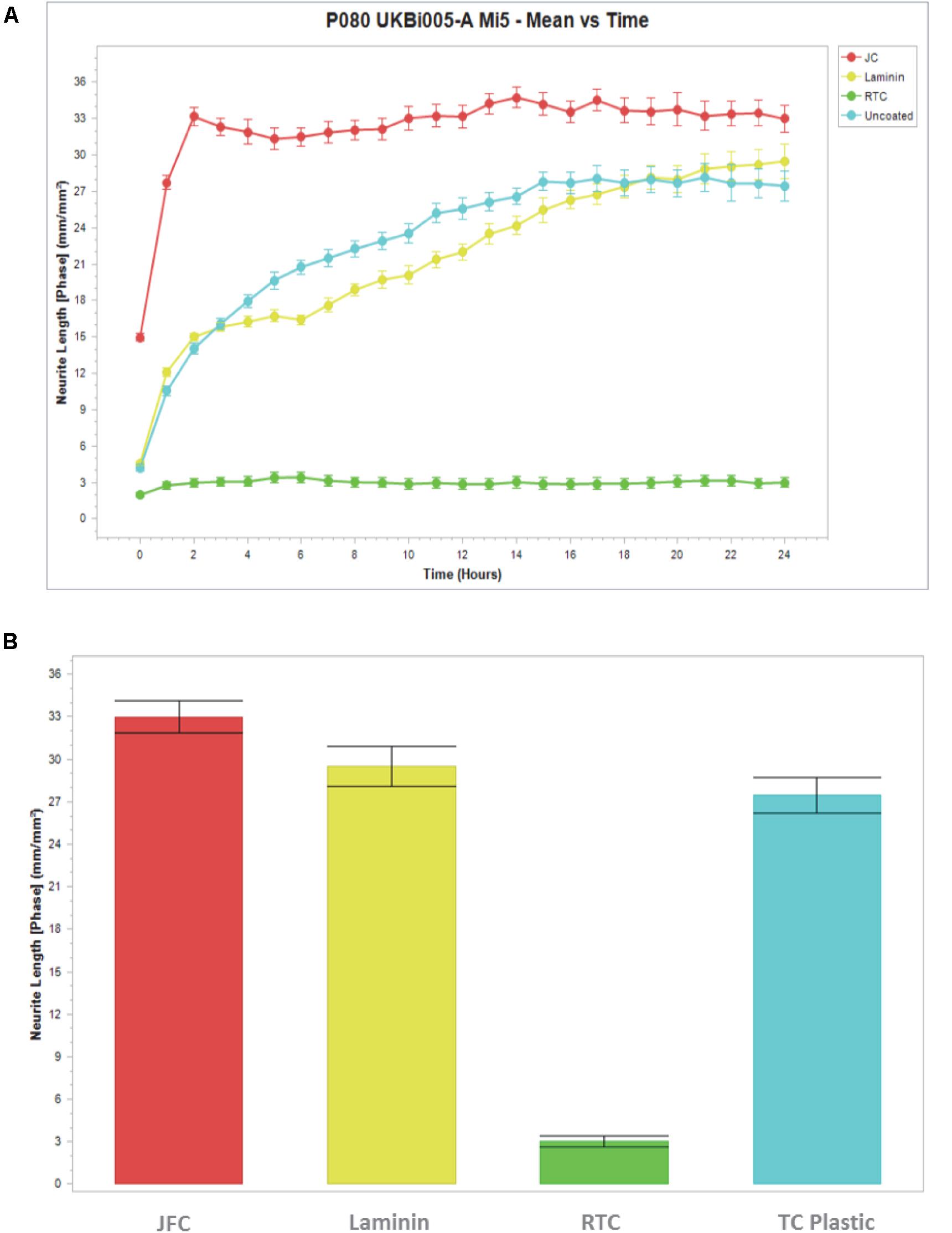
Figure 5. Measure of cell ramification using neurite length software on the Incucyte. Cells were cultured in Jellagen (JFC: 10 μg/mL), Rat tail collagen (RTC 10 μg/mL), laminin or plastic (uncoated) and (A) imaged every hour for 24 h with neurite length quantified. (B) Neurite length quantification after 24 h in culture. Data represent mean ± SEM.
The present study demonstrates that iMGL could be successfully cultured on all concentrations of jellyfish collagen assessed in this study with no loss of cell viability other than homeostatic turnover. Experiments evaluated the viability of iPSC-derived microglia following culture on jellyfish collagen (JC) or rat tail collagen (RTC) compared to controls Laminin-511 or tissue culture plastic for up to 72 h. iMGL cultured on all concentrations of JFC appeared elongated and with a ramified morphology, consistent with standard culture of these cells and similar to cells cultured on laminin or TC plastic. In contrast, microglia cultured on RTC appeared rounded and cells formed clumps as seen in Figure 1. Clumping is not commonly observed in these cells, indicating a change in cell phenotype, activation, function and/or cell health (Cunningham et al., 2013; Tay et al., 2018).
Based on data from dose response experiments, the concentration of 10 μg/mL of both JC and RTC was selected to further to characterize iMGL phenotype and function. Cell morphology was assessed using the neurite tracking module on the InCucyte S3TM as a measure of cell ramification (Figure 5). iMGL cultured on JFC had a time dependent increase in ramification over 24 h, which was better than cells cultured on Laminin-511 or TC plastic. Cells cultured on RTC had much less ramification compared to other conditions assessed and remained rounded, consistent with that observed in images acquired by phase contrast as seen in Figure 1.
Ramification is an important characteristic as during human development, the differentiation of ameboid microglia (brain macrophages) into ramified microglia is marked by a loss of macrophage-like properties and the extension of thin cytoplasmic extracellular matrix proteins (Chamak and Mallat, 1991; Pan et al., 2017). One key interest in the study of microglia is in neuroinflammation, and it is thus imperative that when cells are cultured in vitro they are not activated as this could interfere with additional studies.
The Effect of Cell Culture Matrices on iMGL Cell Surface Receptor Expression
To further characterize iMGL phenotype, immunocytochemistry was performed after 48 h culture on the difference substrates. Cells were stained for the microglia markers, IBA1, TREM2, TMEM119, P2RY12, and CD11b.
Cell surface receptor expression was quantified using flow cytometry (Millipore Guava) on live cells. Cells were blocked in flow buffer (1% FBS + 10 μg/mL human serum IgG) prior to staining for relevant antibodies for 1 h (CD14, CD16, CD11b, HLA-DR, and CD206). Samples were washed and evaluated using a Millipore Guava easyCyte flow cytometer, and 5000 events measured per sample. Cell population was gated to exclude debris and isotype controls used to gate a positive population. Thresholds were set where isotypes showed < 5% positive staining. All data is shown as percent positive expression data summarized on Table 1 but actual graphs not shown.
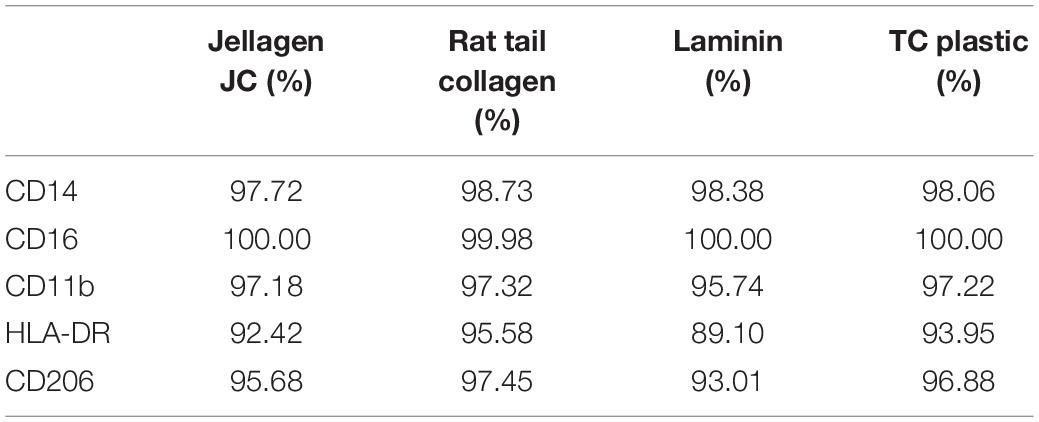
Table 1. Cell surface protein expression. iMGL where cultured on Jellagen® (JFC), rat tail collagen I (RTC), Laminin and TC plastic for 48 h and receptor expression was quantified using flow cytometry.
Culture of iMGL on the different matrices did not drive any changes in cell surface expression of CD14, CD16, CD11b, HLA-DR, or CD206 which were all comparable to cells culture on plastic or laminin (Figure 6 and Table 1). All markers stained positively on the cells, regardless of the matrix they were cultured on (Figure 7).
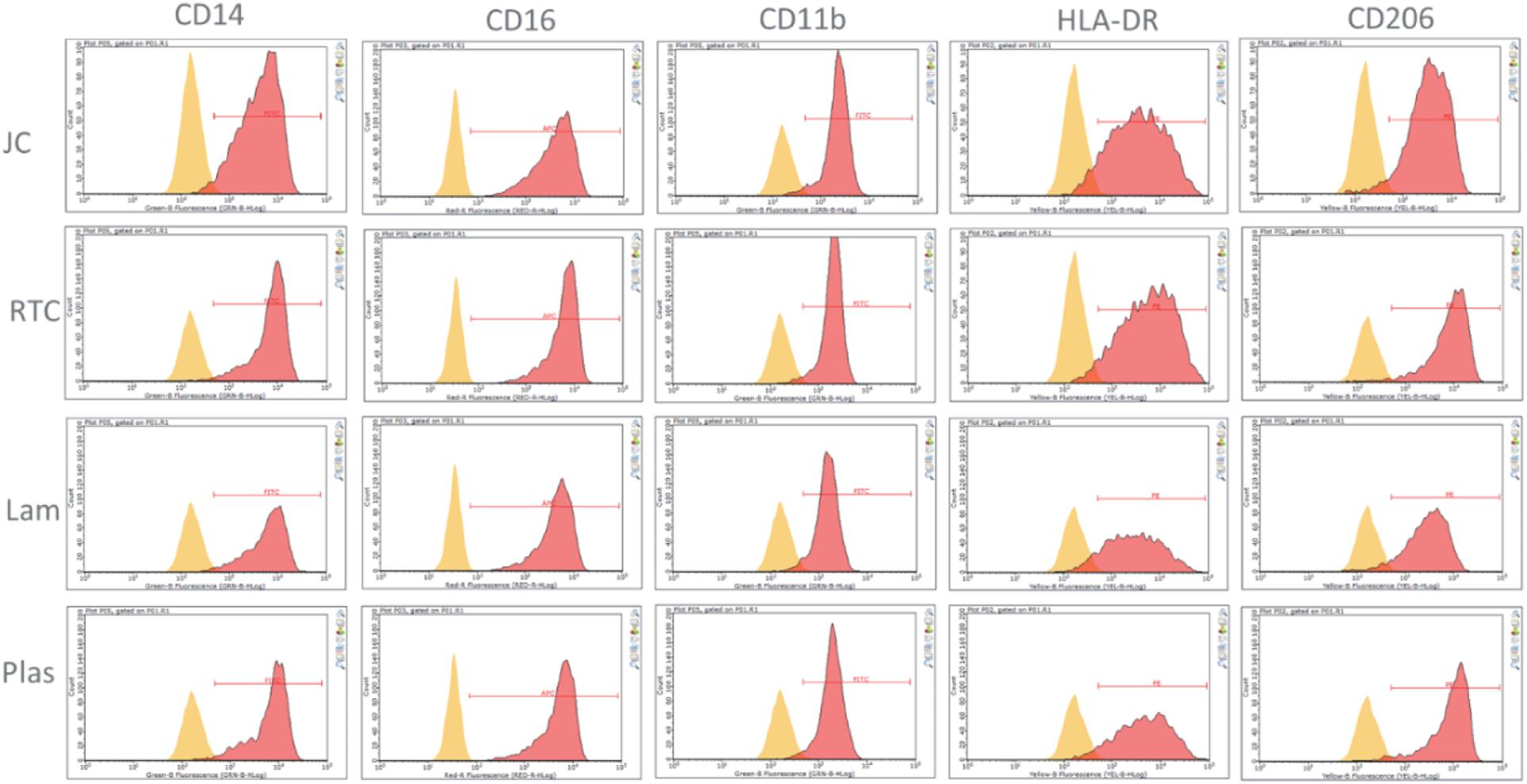
Figure 6. Analysis of cell surface receptors using flow cytometry cultured on Jellyfish collagen (JC), rat tail collagen (RTC), laminin (Lam) and tissue culture plastic (Plas). Microglia cultured on 10 μg/mL JC or RTC or controls for 48 h prior to staining. Yellow: Unstained control, Red: cell surface marker.
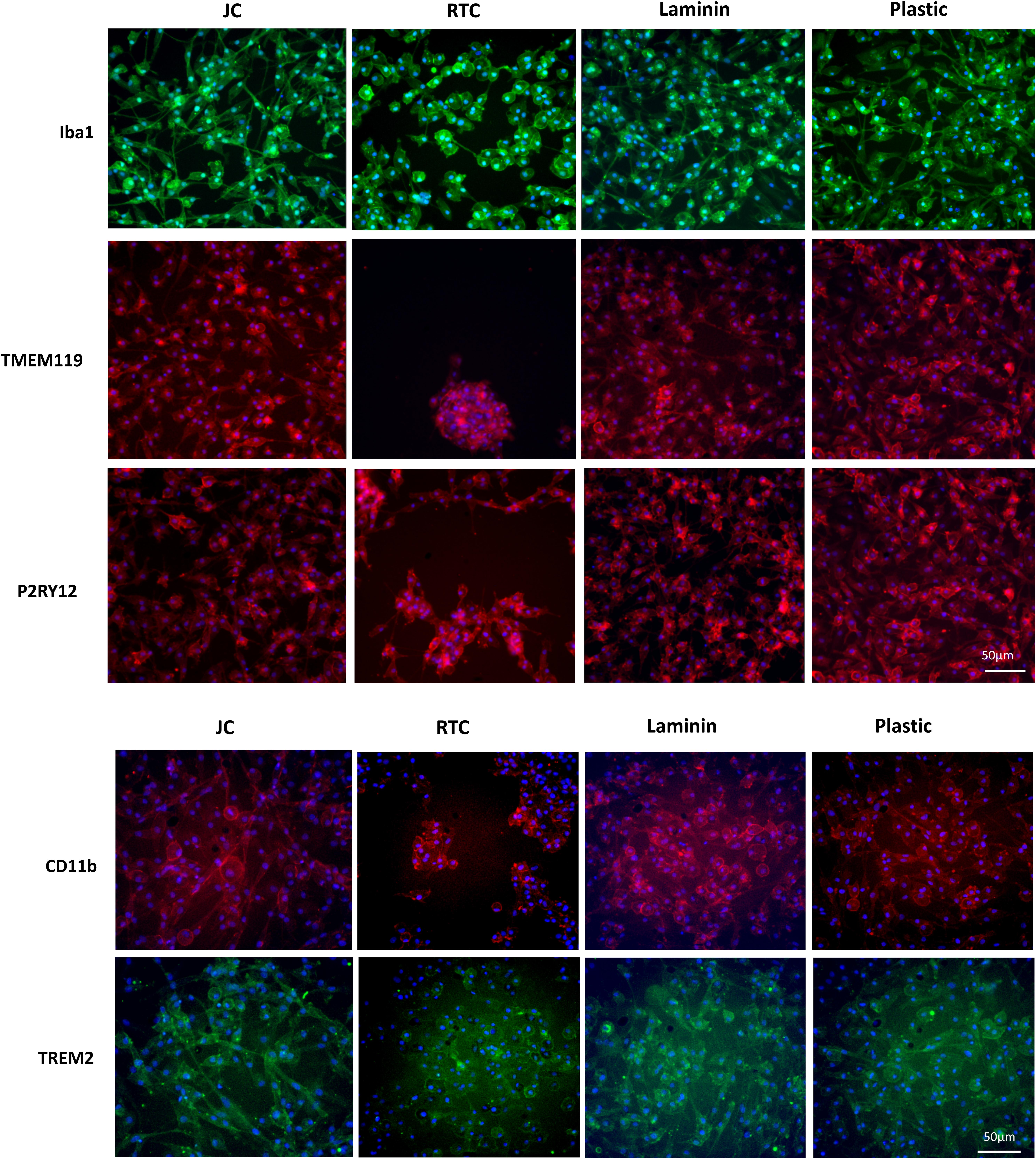
Figure 7. Immunocytochemistry staining of typical microglia markers of iMGL cultured on Jellagen® (JFC), rat tail collagen I (RTC), laminin or TC plastic. Blue: DAPI, red/green: stain of interest. Scale bar = 50 μm.
Effects of Cell Culture Matrices on TNFα and IL-6 Quantification
In order to investigate whether iMGL became activated when cultured on jellyfish collagen (JFC) or RTC, an ELISA was performed to measure cytokine output of IL-6 and TNFα both of which are pro-inflammatory cytokines. Data showed that culture of iMGL on JFC or RTC did not drive increased release of either cytokine at baseline or when stimulated with LPS or INFy compared to controls (Figure 8). Lipopolysaccharide (LPS) and INFY were used to stimulate a pro-inflammatory response form iMGL and cytokine production was measured by ELISA. There were no differences in the levels of TNFα or IL-6 produced by iMGL cultured on the different matrices at baseline or following stimulation.
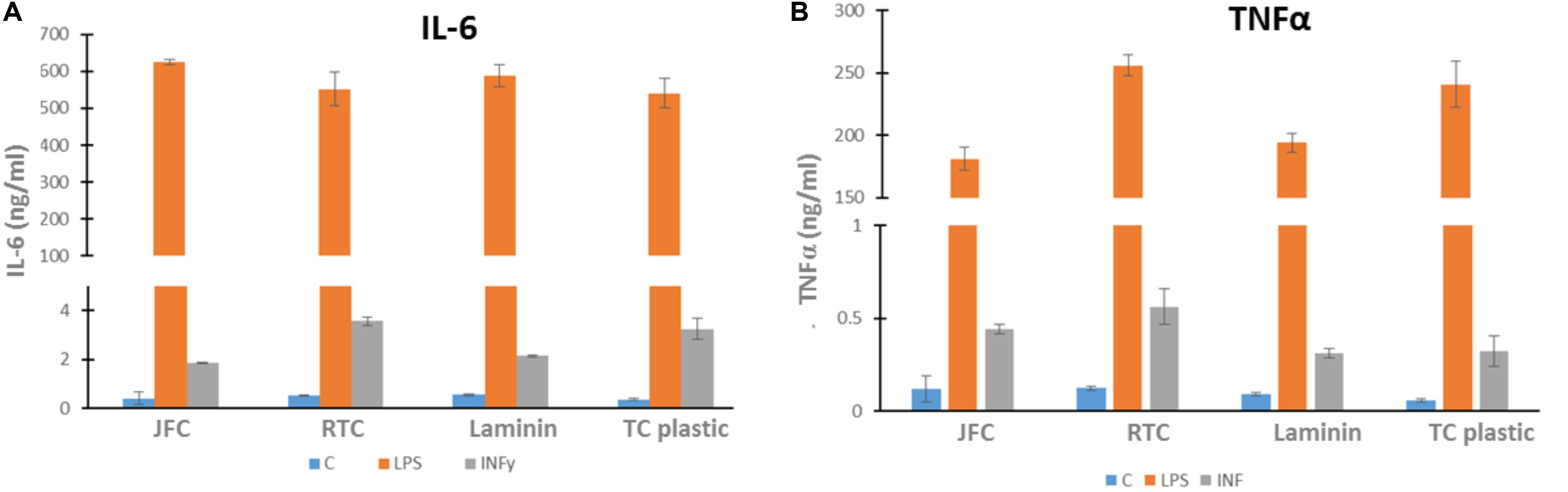
Figure 8. Cytokine output by iMGL at baseline and following 24 h stimulation. iMGL were cultured on Jellagen® (JC), rat tail collagen I (RTC), laminin or TC plastic for 48 h prior to stimulation with LPS (100 ng/mL) or INFY (10 ng/mL) for a further 24 h. Cell supernatants were collected and ELISA performed. Data represent mean ± SEM of 6 technical repeats.
Overall, while microglia can be cultured on plastic, not all cell types can be. As we move toward ever more complex systems of co- and tri-cultures which will be a focus for the development of organoids, it will be important to culture all cell types on one extracellular matrix. Showing that each cell type can grow well on Jellagen® is therefore crucial, as well as showing that the matrix does not cause any activation or cell death of the cells.
In conclusion, we have shown in this study that the jellyfish collagen can be used as a cell matrix for the culture of human iPSC-derived Microglia (iMGL) that display the morphological, surface marker expression and functional characteristics expected from microglia. Jellyfish collagen presents comparable biological impact on human cells to Laminin and in this case was found to be superior to rat tail mammalian type I collagen (RTC) tested by adhesion, cell viability and immunocytochemistry assays. These results may suggest that jellyfish collagen derived from R. pulmo (Jellagen®) offers a potentially inert/non-reactive biomaterial since iMGL cultured on Jellagen did not cause an increase in cytokines and also did not cause cell death (based on several assays and also morphology). Therefore, this matrix shows promise, as the cells are not activated and also doesn’t cause cell death. In contrast, while RTC also did not cause an increase in cytokine release, cells cultured on RTC were observed to produce significant clumping and cell death, so even though the cells are not activated these cells are not healthy on this matrix. The results of this study show clearly that jellyfish collagen offers researchers a biologically compatible and superior alternative to the use of mammalian collagen and other extracellular matrices such as laminin in iMGL cell culture. This data suggests that iMGL did not recognize jellyfish collagen as a foreign substance, however, more tests will be needed to confirm that to be the case.
Data Availability Statement
The datasets generated for this study are available on request to the corresponding author.
Author Contributions
AM-S: conceptualization and writing–original draft. JT: investigation, methodology, writing–reviewing editing, data curation, and visualization. DT: methodology, data curation, software, and image analysis. AB: supervision and writing–reviewing and editing. All authors contributed to the article and approved the submitted version.
Funding
Funding for this project was provided by the Jellagen Limited.
Conflict of Interest
AM-S was employed by the company Jellagen Ltd. JT, DT, and AB were employed by the company Censo Biotechnologies Ltd.
Acknowledgments
AM-S would like to thank JT, DT, and AB from Censo Biotechnologies for supporting this work and for their technical assistance.
Abbreviations
IBA1, Ionized calcium binding adaptor molecule 1; iMGL, human iPSC-derived Microglia; INFy, Interferon gamma; JFC, jellyfish collagen; LPS, lipopolysaccharide; P2RY12, Purinergic receptor P2RY12; RTC, rat tail collagen; TMEM119, Transmembrane Protein 119; TREM2, Triggering receptor expressed on myeloid cells 2.
References
Abud, E. M., Ramirez, R. N., Martinez, E. S., Healy, L. M., Nguyen, C. H. H., Newman, S. A., et al. (2017). iPSC-derived human microglia-like cells to study neurological diseases. Neuron 94, 279–293.
Addad, S., Exposito, J., Faye, C., Ricard-Blum, S., and Lethias, C. (2011). Isolation, characterization and biological evaluation of jellyfish collagen for use in biomedical applications. Mar. Drugs 9, 967–983. doi: 10.3390/md9060967
Bosnakovski, D., Mizuno, M., Kim, G., Takagi, S., Okumura, M., and Fujinaga, T. (2006). Chondrogenic differentiation of bovine bone marrow mesenchymal stem cells (MSCs) in different hydrogels: influence of collagen type II extracellular matrix on MSC chondrogenesis. Biotechnol. Bioeng. 93, 1152–1163. doi: 10.1002/bit.20828
Browne, S., Zeugolis, D. I., and Panditm, A. (2013). Collagen: finding a solution for the source. Tissue Eng. A 19, 1491–1494. doi: 10.1089/ten.tea.2012.0721
Chamak, B., and Mallat, M. (1991). Fibronectin and laminin regulate the in vitro differentiation of microglial cells. Neuroscience 45, 513–527. doi: 10.1016/0306-4522(91)90267-r
Cunningham, C. L., Martínez-Cerdeño, V., and Noctor, S. C. (2013). Microglia regulate the number of neural precursor cells in the developing cerebral cortex. J. Neurosci. 33, 4216–4233. doi: 10.1523/jneurosci.3441-12.2013
Dong, Y., Li, X., Cheng, J., and Hou, L. (2019). Drug development for Alzheimer’s disease: microglia induced neuroinflammation as a target? Int. J. Mol. Sci. 20:558. doi: 10.3390/ijms20030558
Farndale, R. W., Sixma, J. J., Barnes, M. J., and De Groot, P. G. (2004). The role of collagen in thrombosis and hemostasis. J. Thromb. Haemostasis 2, 561–573. doi: 10.1111/j.1538-7836.2004.00665.x
Figuera-Losadaab, M., Thomasa, A. G., Stathisa, M., Stockwell, B. R., Rojasae, C., and Slusher, B. S. (2017). Development of a primary microglia screening assay and its use to characterize inhibition of system xc- by erastin and its analogs. Biochem. Biophys. Rep. 9, 266–272. doi: 10.1016/j.bbrep.2016.12.009
Ginhoux, F., Greter, M., Leboeuf, M., Nandi, S., See, P., Gokhan, S., et al. (2010). Fate mapping analysis reveals that adult microglia derive from primitive macrophages. Science 5, 841–845. doi: 10.1126/science.1194637
Greegburg, J. H., Seppa, A., and Hewitt, T. A. (1981). Role of collagen and fibronectin in neural crest cell adhesion and migration. Dev. Biol. 87, 259–266. doi: 10.1016/0012-1606(81)90149-4
Hoyer, B., Bernhardt, A., Lode, A., Heinemann, S., Sewing, J., Klinger, M., et al. (2014). Jellyfish collagen scaffolds for cartilage tissue engineering. Acta Biomater. 10, 883–892. doi: 10.1016/j.actbio.2013.10.022
Kierdorf, K., Erny, D., Goldmann, T., Sander, V., Schulz, C., Perdiguero, E. G., et al. (2013). Microglia emerge from erythromyeloid precursors via Pu.1- and Irf8- dependent pathways. Nat. Neurosci. 16, 273–280. doi: 10.1038/nn.3318
Lee, C. H., Singla, A., and Lee, Y. (2001). Biomedical applications of collagen. Int. J. Pharm. 221, 1–22. doi: 10.1016/S0378-5173(01)00691-3
Leitinger, B., and Hohenester, E. (2007). Mammalian collagen receptors. Matrix Biol. 26, 146–155. doi: 10.1016/j.matbio.2006.10.007
Pan, N., Bennett, A., and Chi Him, E. M. (2017). Recent advances in the study of bipolar/rod-shaped microglia and their roles in neurodegeneration. Front. Aging Neurosci. 9:128. doi: 10.3389/fnagi.2017.00128
Paradiso, F., Fitzgerald, J., Yao, S., Barry, F., Taraballi, F., Gonzalez, D., et al. (2019). Marine collagen substrates for 2D and 3D ovarian cancer cell systems. Front. Bioeng. Biotechnol. 7:343. doi: 10.3389/fbioe.2019.00343
Pozzi, A., Wary, K. K., Giancotti, F. G., and Gardner, H. A. (1998). Integrin α1β1 mediates a unique collagen-dependent proliferation pathway in vivo. J. Cell Biol. 142, 587–594. doi: 10.1083/jcb.142.2.587
Prinz, M., and Priller, J. (2014). Microglia and brain macrophages in the molecular age: from origin to neuropsychiatric disease. Nat. Rev. Neurosci. 15, 300–312. doi: 10.1038/nrn3722
Pugliano, M., Vanbellinghen, X., Schwinteì, P., Benkirane-Jessel, N., and Keller, L. (2017). Combined jellyfish collagen type II, human stem cells and Tgf-β3 as a therapeutic implant for cartilage repair. J. Stem Cell. Res. Ther. 7:4.
Pustlauk, W., Paul, B., Brueggemeier, S., Gelinsky, M., and Bernhardt, A. (2015). Modulation of chondrogenic differentiation of human mesenchymal stem cells in jellyfish collagen scaffolds by cell density and culture medium. Tissue Eng. Regenerative Med. 11, 1710–1722. doi: 10.1002/term.2065
Ryan, K., White, C., Patel, K., Xu, J., Olah, M., Replogle, J., et al. (2017). A human microglia-like model for assessing the effects of neurodegenerative disease gene variants. Sci. Transl. Med. 20:eaai7635. doi: 10.1126/scitranslmed.aai7635
Silva, T. H., Moreira-Silva, J., Marques, A. L. P., Domingues, A., Bayon, Y., and Reis, R. L. (2014). Marine origin collagens and its potential applications. Mar. Drugs 12, 5881–5901. doi: 10.3390/md12125881
Silvipriya, K. S., Krishna Kumar, K., Bhat, A. R., Dinesh Kumar, B., John, A., and Lakshmanan, P. (2015). Collagen: animal sources and biomedical application. J. Appl. Pharm. Sci. 5, 123–127. doi: 10.7324/japs.2015.50322
Song, E., Yeon Kim, S., Chun, T., Byun, H. J., and Lee, Y. M. (2006). Collagen scaffolds derived from a marine source and their biocompatibility. Biomaterials 27, 2951–2961. doi: 10.1016/j.biomaterials.2006.01.015
Sorushanova, A., Delgado, L. M., Wu, Z., Shologu, N., Kshirsagar, A., and Raghunath, R. (2019). The collagen suprafamily: from biosynthesis to advanced biomaterial development. Adv. Mater. 31:1801651. doi: 10.1002/adma.201801651
Tay, T. L., Béchade, C., D’Andrea, I., St-Pierre, M.-K., Henry, M. S., Roumier, A., et al. (2018). Microglia gone rogue: impacts on psychiatric disorders across the lifespan. Front. Mol. Neurosci. 10:421. doi: 10.3389/fnmol.2017.00421
Keywords: jellyfish, collagen, laminin, iPSC, microglia, cell culture, stem cells
Citation: Mearns-Spragg A, Tilman J, Tams D and Barnes A (2020) The Biological Evaluation of Jellyfish Collagen as a New Research Tool for the Growth and Culture of iPSC Derived Microglia. Front. Mar. Sci. 7:689. doi: 10.3389/fmars.2020.00689
Received: 20 February 2020; Accepted: 29 July 2020;
Published: 31 August 2020.
Edited by:
Arita Dubnika, Riga Technical University, LatviaReviewed by:
Giovanna Romano, University of Naples Federico II, ItalyEhsan Saburi, Zanjan University of Medical Sciences, Iran
Copyright © 2020 Mearns-Spragg, Tilman, Tams and Barnes. This is an open-access article distributed under the terms of the Creative Commons Attribution License (CC BY). The use, distribution or reproduction in other forums is permitted, provided the original author(s) and the copyright owner(s) are credited and that the original publication in this journal is cited, in accordance with accepted academic practice. No use, distribution or reproduction is permitted which does not comply with these terms.
*Correspondence: Andrew Mearns-Spragg, YW5kcmV3Lm1lYXJucy1zcHJhZ2dAamVsbGFnZW4uY28udWs=; YW5kcmV3QGplbGxhZ2VuLmNvLnVr