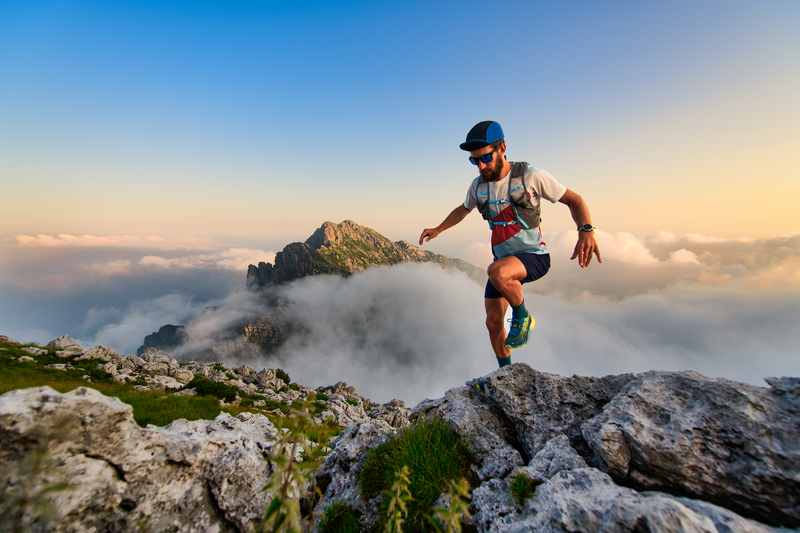
95% of researchers rate our articles as excellent or good
Learn more about the work of our research integrity team to safeguard the quality of each article we publish.
Find out more
ORIGINAL RESEARCH article
Front. Mar. Sci. , 31 July 2020
Sec. Deep-Sea Environments and Ecology
Volume 7 - 2020 | https://doi.org/10.3389/fmars.2020.00608
Studies have shown the importance of submarine canyons as conduits of land-derived organic carbon beyond the coastal shelf into the deep-sea where a single obvious river source can be identified. When there is more than one river source, identifying which rivers contribute to canyon sediment organic matter is technically challenging. Here, we compare two contrasting submarine canyons: the Hokitika Canyon, a long, narrow, and gently sloping canyon on the west coast of New Zealand; and the Kaikōura Canyon, a high productivity, short, steep canyon close to shore on the east coast of New Zealand. Both canyons have multiple potential river sources, so we applied a compound specific stable isotope (CSSI) tracking technique to identify and apportion the contribution from each river at locations along the length of each canyon axis. We found that land-derived organic matter contributed between 74 and 100% of the total organic matter in the sediment of the Hokitika Canyon as far as 200 km from shore and to depths of 2000 m. However, less than 50% of the land-derived organic carbon came from the largest river closest to the canyon head. We hypothesize that longshore drift transported much of the sediment from that river past the Hokitika Canyon, while river inflows farther up-current supplied the bulk of the land-derived organic carbon. In contrast, land-derived organic matter contributed less than 50% of the total organic matter in Kaikōura Canyon sediments with land-derived organic sediment contribution decreasing steeply to less than 15% at about 24 km from shore in 1500 m water depth. Most of the land-derived organic matter (ca. 80%) came from the river with the largest suspended sediment yield, despite another (smaller) river discharging closer to the canyon head. We hypothesize that this difference in carbon source is partly due to the comparatively short and steep, and therefore dynamic, nature of Kaikōura Canyon resulting in efficient sediment through-put. The efficiency with which organic matter is captured and transferred to the deep-sea by canyons demonstrates the potential for such systems to act as natural carbon sinks driven by both geologically episodic and more regular oceanographic processes.
Understanding the transport pathways and fate of organic carbon delivered from continental land areas to the ocean remains a major challenge in marine biogeochemistry (Hedges and Keil, 1995; Goñi et al., 1997; Hedges et al., 1997; Benner et al., 2004; Burdige, 2005; Blair and Aller, 2012). Globally, the annual total flux of terrestrial particulate organic carbon is estimated to be between 0.2 and 0.5 Pg y–1, with most of this material delivered to the ocean by rivers (Degens et al., 1991; Smith and Hollibaugh, 1993; Ludwig et al., 1996; Schlunz and Schneider, 2000; Dagg et al., 2004; Liu et al., 2010). The majority (55–80%) of this exported terrestrial carbon is thought to be remineralized along the continental margins, while the remainder is buried in sediments of deltaic environments and on the continental shelf and slope and in deep sea environments (Hedges and Keil, 1995; Burdige, 2005). The deposition of terrigenous sediment on the continental margin (shelf to slope and rise) is expected to produce a systematic gradient of land-derived material decreasing in concentration away from the sediment sources, such as rivers (O’Brien et al., 2006). Chen (2010) estimates that ∼0.2 Pg y–1 of organic and inorganic carbon is buried in shelf environments, while similar amounts are also transported downslope and deposited on the continental slope (Burdige, 2005; Jahnke, 2010; Kandasamy and Nath, 2016). These global estimates, however, do not take into account the downslope transport of organic carbon across the continental margin as facilitated by submarine canyons, which could have a significant effect on regional carbon budgets.
Submarine canyons are recognized as a major conduit for land derived sediment moving to deep ocean depo-centers (Pasqual et al., 2011; Paull et al., 2018). Canyons eroded into the upper continental slope and continental shelf may link directly to terrestrial sediment sources through riverine input or may receive sediment by longshore transport (littoral zone) processes (Puig et al., 2014). Sediment is commonly staged in submarine canyon head areas and remobilized via mass wasting processes such as landslides and gully erosion and transported downslope as turbidity currents (Pasqual et al., 2011; Paull et al., 2018). Turbidity currents in continental shelf-slope settings can also be generated by plunging hyperpycnal flows off highly turbid, high discharge rivers (Mulder and Syvitski, 1995) and via dilute river plumes with sediment concentrations as low as 1 kg/m3, if the plume locally becomes denser than ambient seawater (e.g., by double diffusion or settling-driven convection) (Hage et al., 2019). The amount of terrestrial organic matter transported to the deep sea by canyons differs widely (see references above) and likely involves a range of different processes (Pickering et al., 1986; Canals et al., 2006; Allen and Durrieu de Madron, 2009; Peakall and Sumner, 2015). The proportion of land-derived organic matter in submarine canyons can be relatively low, such as in the Nazaré Canyon on the Portuguese margin (10–15%; Alt-Epping et al., 2007) or more elevated, as in the Aviles Canyon in the Bay of Biscay and Baltimore Canyon (ca. 40%; Romero-Romero et al., 2016; Prouty et al., 2017). In the Congo deep-sea turbidite system, most (>70%) of the sediment organic matter is land derived (Stetten et al., 2015; Baudin et al., 2017) and originates from the Congo River, which drains the second largest watershed in the world. Similarly, high terrestrial organic matter concentrations are found in the sediments off Taiwan, where pulses of fluvial organic matter associated with typhoon events are transported directly to the deep sea via the Gaoping Canyon (Hsu et al., 2014). Small, mountainous river systems, as found on active tectonic margins such as Taiwan and across Oceania, are now recognized as contributing significantly to global budgets of fine-grained sediment deposition in marine systems (Milliman and Syvitski, 1992; Blair and Aller, 2012; Bao et al., 2015) including, by inference, the deposition of associated bio-reactive constituents, such as terrestrial organic matter (Hastings et al., 2012).
Terrigenous carbon sources in suspended particles and surface sediments from the marine environment have been identified using biomarkers (Bouloubassi et al., 1997; Goñi et al., 1997, 2000; Wakeham et al., 1997; Mannino and Harvey, 1999; Xiao et al., 2013; Prouty et al., 2017) and compound-specific stable isotope analysis (CSIA) (O’Brien et al., 2006; Amiel and Cochran, 2008; Wildhaber et al., 2012; Tolosa et al., 2013; Yunker et al., 2015). These biomarkers, and associated techniques, have identified that land-based organic material is sometimes a major contributor to marine particulate organic matter flux. While many of these and other tracer studies confirm the terrigenous origin of particulate organic matter and sediments, they do not identify the specific catchment sources unless a single major river inflow is in proximity (Vonk et al., 2019).
Using a different approach, Gibbs (2008) was able to identify and apportion the soil source contribution to estuarine sediments by land use, using a compound specific stable isotope (CSSI) technique. In this CSSI technique, isotopic signatures of fatty acid (FA) biomarkers bound to soil particles are used as the tracers of soil origin. All plants produce the same range of saturated FAs, with carbon chain lengths from 14 to 28 and higher (i.e., C14:0 to >C28:0), during photosynthesis. These biomarkers are released from the plant roots into the soil where they bind ionically to the soil particles. However, each plant species produces their FAs with slightly different isotopic signatures because of different levels of isotopic fractionation during their production in the plants photosynthetic pathway. Consequently, each plant community that defines a specific land use (e.g., wetland, native forest, or agricultural land), labels the soil from that land use with a unique isotopic fingerprint that can be traced back to its origin from a sediment deposition zone mixture (Gibbs, 2008, 2014). Once bound to the soil, these compounds are refractory and therefore conservative tracers in the marine sediments, remaining unchanged for hundreds of years (Gibbs, 2008).
The CSSI technique uses a source library of reference land use types and a stable isotope mixing model to disaggregate the sediment mixture into its catchment and/or land use source components. While the CSSI technique has successfully been used to assess soil erosion across landscapes from different land uses (Blake et al., 2012; Brandt et al., 2016, 2018; Bravo-Linares et al., 2018; Mabit et al., 2018) and from land into rivers (Hancock and Revill, 2013; Alewell et al., 2016) and estuaries (Gibbs, 2008; Gibbs and Olsen, 2010; Gibbs et al., 2012) it has not been used to assess the relative proportions of recent terrigenous and marine material in sediment deposits in the deep-sea. The two canyons investigated in this study were the Kaikōura Canyon, on the east coast of the South Island/Te Waipounamu of New Zealand/Aotearoa, and the Hokitika Canyon, on the west coast of the South Island.
The aim of the present study was to investigate the quantity, quality and sources of the organic matter in two contrasting canyons, while a companion study examined the relationships between sediment organic matter parameters and macro- and meiofaunal abundance, biomass and community structure (Leduc et al., 2020).
In this study, we raise the hypothesis that the steep topography and proximity to the coast of Kaikōura Canyon results in higher organic matter enrichment from land sources relative to Hokitika Canyon. This is tested by investigating the contributions of land-derived organic matter to canyon sediments, using bulk stable isotopes of C and N and CSSI signatures of terrigenous biomarkers to identify the riverine sources. This study is the first to apply the CSSI technique (Gibbs, 2008, 2014) to a deep-sea setting to identify and quantify the contribution of several potential source rivers to sediment organic matter of submarine canyon sediments.
The Kaikōura Canyon is incised deeply into the narrow continental shelf, with the canyon head only ∼500 m off the shore (Figure 1). The canyon rim is at just 30 m water depth dropping off into steep (up to 40 degrees) canyon walls to the upper reaches of the canyon floor at 500 m depth. The 60 km long canyon exits into the Hikurangi Channel at approximately 2000 m depth, and is characterized as a deeply incised bedrock canyon with walls up to 1100 m high. The lithology is variable but known bedrock from dredge samples includes greywacke and limestone, while canyon infill sediments include mud sand, gravel and coarse clastics up to boulder size (Barnes et al., 1998). The canyon is near the highly productive Subtropical Front (STF), where warm subtropical surface waters to the north meet cold, high nutrient-low chlorophyll subantarctic surface waters to the south (Shaw and Vennell, 2000; Murphy et al., 2001; Sutton, 2001; Chiswell et al., 2015). Episodic upwelling events may also contribute to the high primary productivity of the surface water above the canyon (Heath, 1982; Chiswell and Schiel, 2001) with annual gross productivity estimated to be in the order of 160 g C m–2 y–1 and seasonal peaks of ∼10 g C m–2 d–1 in spring (Bradford, 1972) compared to high spring values of ∼1 g C m–2 d–1 farther offshore in the STF on the Chatham Rise.
Figure 1. Site and sample location maps of the east coast Kaikōura Canyon, showing: (A) the position relative to New Zealand, (B) the position of the Kaikōura Canyon sampling sites (open circles) relative to the marine sediment source sites (solid circles) on the Chatham Rise (CR) and (C) the positions of the main inflow rivers relative to the Kaikōura Canyon and Conway Trough, and the predominant longshore drift direction. Approximate position of the Subtropical Front (STF) boundaries is shown by gray lines in panel (B).
The North Canterbury region, located immediately south of the Kaikōura Canyon, has several large river catchments that drain from the Marlborough mountains to the coast, and collectively transport 5.68 Mt y–1 of suspended sediment (Hicks et al., 2011). The Hurunui, Waiau and Conway rivers transport 0.53, 2.77, and 0.22 Mt y–1 of suspended sediments to the coast, respectively, and are the main contributors of terrigenous sediments to the coastal waters in the region (Figure 1; Hicks et al., 2011). A northeast littoral drift system transports sediment along the eastern continental shelf, driven by the Southland Current along the shelf-break (Chiswell, 1996; Sutton, 2003; Chiswell et al., 2015; Nokes et al., 2019) and the prevailing south-eastward wind- and storm-waves (Carter and Heath, 1975; Carter and Herzer, 1979). Fine sands and mud transported in the littoral drift system (Nokes et al., 2019) are eventually captured at the heads of submarine canyons, such as the Kaikōura Canyon (Herzer, 1979; Lewis and Barnes, 1999) or dispersed across the continental slope as a mud drape (Carter and Herzer, 1979; Bostock et al., 2019a).
The accumulation of sediment in the head and on the steep sides of the canyon has the potential to fail under severe ground-shaking by earthquakes, which can lead to transport of sediments down the canyon into the deep-sea Hikurangi Channel as turbidity currents (Lewis and Pantin, 2002). During the recent November 2016 Mw 7.8 Kaikōura earthquake (Clark et al., 2017; Hamling et al., 2017; Kaiser et al., 2017), slope failures and substantial erosional scouring of geological materials within the canyon led to the generation of a turbidity current, tracked over 600 km away from the Kaikōura Canyon source along the deep-sea Hikurangi Channel (Mountjoy et al., 2018). The Hikurangi Channel extends 1500 km from the continental margin, with submarine fans identified at its terminus in the Southwest Pacific Basin (Lewis, 1994).
In contrast, the Hokitika Canyon is one of several similar bathymetric features that incise the continental slope and outer shelf along the middle part of the west coast of the South Island (Figure 2). The shelf narrows markedly southwards along the coast from 40 to 80 km width off northwest Nelson, to 30–35 km south of the Hokitika Canyon and to <5–10 km off south Westland farther to the south (Zeldis et al., 2010). The canyons, most of which have high to moderate sinuosity, often coalesce to form deeply incised channels that continue out into the Tasman Sea to beyond 4000 m water depth, with evidence of small depositional fans on the Tasman Abyssal Plain (Mitchell et al., 2012). The ∼1250 km long Hokitika Canyon is the northernmost feature and cuts across the southern flank of the Challenger Plateau. The canyon axis is incised 200–600 m into the seafloor from the shelf-break down the continental slope, with up to 1000 m of incision on the lower slope and continental rise (H. Neil., NIWA, unpublished data). The canyon head is fed by numerous distributary channels across the shelf-break, with the canyon becoming wider (from 10 to 20 km wide) and characterized by geomorphic features reminiscent of a braided river system (e.g., meanders, ox bows) in the upper and mid-slope area. Sediments in the canyon axis are dominated by micaceous silts and fine sands, with turbidite deposits on the abyssal plain levees outside of the incised channel (H. Neil., NIWA, unpublished data; Bostock et al., 2019b).
Figure 2. Site and sample location maps of the west coast Hokitika Canyon, showing: (A) the position relative to New Zealand, (B) the position of the Hokitika Canyon sampling sites (open circles) relative to the marine sediment source sites on the Challenger Plateau (CP) and (C) the positions of the main inflow rivers relative to the Hokitika Canyon and the predominant longshore drift direction. Numbered river catchments are: (1) Mikonui, (2) Waitaha, and (3) Wanganui.
The west coast of the South Island contributes one of the highest annual suspended sediment yields in New Zealand (62.3 Mt y–1), second only to the East Cape, North Island/Te Ika a Māui (68.8 Mt y–1; Hicks et al., 2011), driven by the high exhumation, erosion and rainfall rates in the rapidly uplifted Southern Alps/Kā Tiritiri o te Moana along the Alpine Fault plate boundary (Adams, 1980; Jiao et al., 2017). The prevailing westerly wind leads to periodic coastal upwelling (Stanton and Moore, 1992) storm-driven current flows and generates the northwards-directed Westland Current (Heath, 1982; Zeldis et al., 2010; Chiswell et al., 2015). Biological productivity on the shelf is in the order of ∼180 g C m–2 y–1 (Probert, 1986; Zeldis et al., 2010) with seasonal productivity peaks of 0.2–0.7 g C/m2/d likely in spring-summer (Bradford-Grieve et al., 1997).
In the vicinity and south of the Hokitika Canyon are several large rivers, including the Hokitika, Waitaha, Whataroa, Waiho, Haast and Arawhata rivers, each contributing substantial annual suspended yields in the order of 3–7 Mt y–1 (Griffiths and Glasby, 1985; Hicks et al., 2011). However, Radford (2012) suggests that the Hokitika Canyon is not fed directly by the Hokitika River under modern sea-level conditions, with more direct connection occurring during the glacial lowstand when the rivers debouched at or near the present-day shelf-break. Terrestrial organic matter in the form of high proportions of lignin (Probert and Swanson, 1985) low organic carbon contents (<0.5%) and high C:N ratios (9 to >15) in the shelf sediments (Stoffers et al., 1984) suggest that much of this organic material is not readily assimilable by the shelf benthos (Probert, 1986; Zeldis et al., 2010).
Sediment samples from Kaikōura and Hokitika canyons were collected using an Ocean Instruments MC-800 multi-corer, which provides up to eight undisturbed, 9.52 cm inner diameter cores. Samples from Kaikōura Canyon were collected at six sites from 1017 to 1485 m water depths (voyage TAN1006; April–May 2010). Samples K01, K04, K07 and K12 were located directly in the canyon thalweg. Sample K03 was within a small tributary canyon. Sample K08 was located on the canyon floor but approximately 30 m above the thalweg. Samples from the Hokitika Canyon were collected at six sites from 200 to 2000 m water depths (voyage TAN1311; October 2013) (Table 1); Sites H200, H700, H1200B, H1500 and H2000 were all located in the canyon thalweg, whereas H1000 was located on a terrace 50 m above the thalweg. The top 5-cm layer of sediment from each sample was sampled and sealed in plastic bags and frozen (−20°C) pending analysis.
To characterize the isotopic signature of deep-sea sediments not affected by sedimentation of land-derived organic matter (i.e., purely marine in origin), we obtained sediment samples located offshore from each study canyon at similar depths (Table 2). Samples were obtained from Chatham Rise, offshore from Kaikōura Canyon (voyage TAN1701; January 2017), and from Challenger Plateau (offshore from Hokitika Canyon; voyage TAN0707; May–June 2007). These sediment multi-corer samples were sliced and frozen using the same methods as the canyon samples described above.
River sediment load and flow data were extracted from the New Zealand River Maps database (Booker and Whitehead, 2017) (NZ River Maps© 2016 NIWA, Version 1.0.01). On the east coast, there are three major rivers that could deliver terrigenous sediment to the Kaikōura canyon: the Hurunui, Waiau and Conway rivers. On the west coast, there are five major rivers that could deliver terrigenous sediment to the Hokitika Canyon: the Hokitika, Mikonui, Waitaha, Wanganui and Whataroa rivers (Table 3).
Table 3. Comparison data for the west coast and east coast South Island rivers, extracted from the New Zealand River Maps database (Booker and Whitehead, 2017).
As previously noted, east coast rivers have relatively low sediment yields reflecting the low rainfall and low slope of the land in their catchments. Although the historical land use has been mostly sheep and grain, there are now areas of dairy farming and exotic plantation forestry. Erosion zones in the rolling hills are common but in different proportions in each catchment. Because of these observations, the individual isotopic signatures of each catchment (Hurunui, Waiau and Conway rivers) were used in the modeling.
In contrast, west coast rivers have steep alpine catchments and, coupled with very high rainfall, they have very high sediment yields. The Hokitika River sediment yield is lowest at 58 t ha–1 y–1, which reflects the large lowland farming basin in the middle of that catchment. The other four rivers have mean sediment yields of 77–88 t ha–1 y–1 reflecting their steep mountain slopes with limited lowland areas. The main land use in these four river catchments is native beech forest and, because of this, we assumed that any difference in isotopic signatures would be minimal. Consequently, these four rivers were treated as one source with the isotopic data from the largest, the Whataroa River, being considered as representative for modeling purposes. This approach is consistent with best modeling practice (Phillips et al., 2014).
Sediments from the river mouth of each river were obtained in March 2017 (Table 2). Because rivers and streams transport large percentages of the annual sediment loads from their catchment during a small number of floods that occur over relatively short time periods in a year (Markus and Demissie, 2006) sampling sites were selected in natural deposition zones along the side of the main channel in the flood plain between the low flow river level and the obvious maximum flood level. Sediment deposited in this zone would have come from highly turbulent, well mixed water and would be representative of most suspended sediments being carried out to sea. Sediment from the river mouth sites represents an integration of all sediment transported from the upstream catchment to the sea, an approach consistent with other studies (Belicka and Harvey, 2009; Tolosa et al., 2013). These sites provide the land end-member sediments for the Kaikōura and Hokitika canyon isotope analyses. At each site, ten 100-mm diameter, 20-mm thick surface sediment samples were collected with a hand-corer from an area of about 100 m2. These ten sediment samples were combined to form one composite sample. About 400 g of the homogenized composite mixture was placed in a cool insulated box in the dark for transport to the laboratory. Five composite samples were collected from each site to provide replication.
Each marine and river sediment sample was freeze-dried and sieved through a 500-micron mesh. The proportion of organic matter in each sample was determined in two ways. First as loss on ignition (LOI) by combusting a 5 g aliquot of dried soil at 500°C for 3 h. Total organic carbon content was estimated by assuming a 47% carbon content (Perié and Ouimet, 2008). This measure was used to provide a guide for how much sample was required for the stable isotope analyses. The second method was by elemental analysis as the bulk stable isotopes were determined. These latter values were used in all subsequent data manipulations. Sediment grain-size, carbonate percentage, total particulate organic matter, and phytopigment content (chlorophyll a and phaeopigments) were also determined for the marine samples. Laboratory methods are largely as described in Nodder et al. (2003) with %TOM determined by loss-on-ignition (500°C for 4 h), %CaCO3 by vacuum gasometry, and %TOC, %N and C:N by CHN analyser (CE Instruments NC2500) after acidifying with 8% sulfurous acid (Verardo et al., 1990). Phytopigments were determined by spectrofluorometric techniques after freeze-drying and extraction in 90% acetone, and grain-size determinations were made using Beckman Coulter LS 13 320 Laser Diffraction Particle Size Analyzer (Nodder et al., 2003, 2011).
Stable isotope analyses of carbon (δ13C) and nitrogen (δ15N) of bulk organic matter were carried out on a DELTA V Plus continuous flow isotope ratio mass spectrometer (CF-IRMS) linked to a Flash 2000 elemental analyser via a ConFlo IV, using a MAS 200 R autosampler (EA-IRMS) (Thermo Fisher Scientific, Bremen, Germany) at the NIWA Environmental and Ecological Stable Isotope Facility in Wellington, New Zealand. Prior to the δ13C analyses, a 5 g aliquot of each sediment sample was acidified using 10% hydrochloric acid (HCl) to remove inorganic carbonates. The sample was then rinsed three times with deionised water, freeze-dried and ground before subsampling for analysis. A separate non-acidified aliquot was used for δ15N analyses.
Isotopic abundances are reported as δ-values (“per mil,” ‰) on the VPDB scale (Vienna Pee Dee Belemnite) for δ13C values, and the AIR scale (atmospheric air) for δ15N values, as determined by the International Atomic Energy Agency (Vienna, Austria). Initial isotopic values were calculated by ISODAT software (Thermo Fisher Scientific) by comparison with an ultra-high purity working gas (CO2 or N2). Final isotopic values were determined using data from the daily analysis of solid National Institute of Standards and Technology (NIST) reference materials following Paul et al. (2007). Precision (±0.1‰ for δ13C and ±0.2‰ for δ15N) was determined by the repeat analysis of the working laboratory standard DL-Leucine. Accuracy and precision were checked by the repeat analysis of international reference material USGS65 Glycine. Carbon and nitrogen content (%C and %N) were calculated by ISODAT software from thermal conductivity detector values of a DL-Leucine laboratory standard run as a reference during EA-IRMS analysis.
Fatty acid biomarkers for CSIA were extracted from 20-g, non-acidified aliquots of each dry sample with dichloromethane at 100°C and 2000 psi in an accelerated solvent extraction system (DIONEX ASE200 – Thermo-Fisher Scientific Dionex, Germany), using two 5-min extraction cycles. The extracts were combined before being reduced to dryness on a rotary evaporator in a 35°C water bath. The residue was taken up in 2 ml of dichloromethane and transferred to a 10 ml Kimax screw-top reaction tube and dried in a heating block under a flow of, oxygen-free dry nitrogen. The dry extract was methylated using 2 ml of 5% boron trifluoride (BF3) catalyst in pure methanol. The tube was sealed and heated to 70°C in an air-fan oven for 20 min. The resultant fatty acid methyl esters (FAMEs) where extracted from the reaction mixture in hexane, dried and analyzed at the NIWA isotope facility.
Fatty acid methyl ester samples were analyzed via gas chromatography-combustion-isotope ratio mass spectrometry (GC-C-IRMS) using a Thermo TRACE Ultra Gas Chromatograph with GC IsoLink Interface coupled via a ConFlo IV to a DELTA V Plus CF-IRMS (Thermo-Fisher Scientific, Bremen, Germany). FAME mixtures were separated on a Thermo TraceGold TG-5ms column (30 m × 0.25 mm ID, 0.25 μm film thickness) prior to combustion at 1000°C in a reactor with NiO/CuO and a Pt catalyst. The split/splitless injector was held in splitless mode for 1 min at 280°C with a constant carrier gas (helium) flow of 1.4 ml/min. GC oven conditions were as follows: initial temperature of 50°C held for 1 min, ramp 10°C/min to 120°C, ramp 4°C/min to 220°C, ramp 10°C/min to 290°C, hold for 10 min.
Prior to analysis, the FAME mixtures were spiked with an internal standard (C12:0) with a known isotope composition to normalize isotope values to international standards and to determine the micrograms of carbon (μg C) of each FA in the samples. Several samples from each sample set were run in duplicate alongside a suite of seven saturated FA internal reference materials (C14:0, C16:0, C18:0, C20:0, C22:0, C24:0. C26:0). Precision on replicate reference materials varied by FA but was generally better than ± 1‰. The average standard deviation of replicate measurements of reference materials was < ± 0.5‰ across all FAMEs.
The methanol used in the derivatization process was analyzed for δ13C to allow correction of the FAME isotopic value for the added methyl group. An aliquot of methanol (1 to 2 μL) was transferred into the base of a 4.5 mm × 2.0 mm smooth-walled tin capsule containing a small piece of glass microfiber filter paper (GF/F, Whatman®). The tin capsule was immediately cold-welded and analyzed by EA-IRMS (as described above). Methanol was analyzed in triplicate with a precision of 0.03‰.
Methyl group correction was applied to all FAME data using the equation:
where δ13CMethanol is the bulk δ13C value of the methanol used in the methylation process, δ13CFAME is the δ13C value of the FAME as measured, and X is the fractional contribution of the methyl group to the δ13C of the FAME. The value X was calculated from the number of carbon atoms in the FA molecule divided by the number of carbon atoms in the FAME derived from the FA. For example, the FA stearic acid (C18:0) has 18 carbon atoms whereas the FAME produced, methyl stearate, has 19 carbon atoms, including one added carbon from the methanol, and thus has an X-value of 18/19 or 0.9474. The methanol-corrected FAME results were then used as the CSSI values in the source proportional modeling.
The proportional contribution of land-derived organic matter at each core site in both canyons was determined by comparing to offshore (100% marine-derived organic matter) and riverbed (100% land-derived organic matter) sediments using a simple mixing model based on bulk stable isotope values of carbon (δ13C) and nitrogen (δ15N). The mixing model used was IsoError. These results were used as the quantitative measure of the land-derived (terrigenous) soil component, because there is an intrinsic difference between land and marine derived isotopic signatures of these bulk stable isotopes. However, these results do not identify which river(s) the soil came from. To do this, the CSSI source apportionment technique was used (Gibbs, 2008, 2014).
Identification and apportionment of source river contribution to the sediment organic carbon at each site along the Kaikōura and Hokitika and canyons was estimated using the isotopic mixing model MixSIAR (Stock and Semmens, 2015; Stock et al., 2018) applied to the bulk δ13C data and the δ13C values of the FAs extracted from each sediment sample. These data were used to develop a source library for modeling in MixSIAR following best practices (Phillips et al., 2014). Note that in sediment tracing studies, there is no fractionation of the isotopic signatures of the FAs once they are bound to the fine sediment particles, i.e., the FA signatures are conservative. Consequently, the trophic discrimination factors (TDF) (i.e., the difference between tracer values in consumer (mixture) and source tissues) are set to zero (Stock et al., 2020). The isotopic signatures of the bulk δ15N or nitrogenous organic molecules are not used in the CSSI technique as these compounds do not bind to the soil. This lack of binding means nitrogenous organic molecules can experience isotopic fractionation, which causes their isotopic signatures to change and therefore they are not conservative. Because marine organic matter is transported alongshore by coastal currents, the same suite of isotopic data from offshore surficial sediment samples collected from Chatham Rise (east coast adjacent to the Kaikōura Canyon; Figure 1B), and Challenger Plateau (west coast adjacent to the Hokitika Canyon; Figure 2B), were used as marine sources in the library for modeling. The organic matter being deposited in the canyons is potentially a mixture of all sources.
A stable isotope mixing model (SIMM) was used to deconstruct the deposited sediment into its source proportions. Recent SIMMs are statistical tools that use biotracers such as FAs and stable isotopes to probabilistically estimate the contribution of multiple sources to a mixture. The original mixing model used by Gibbs (2008) was IsoSource which is a less complex but non-probabilistic tool used to estimate the isotopic proportions of feasible sources contributing to a sediment mixture. IsoSource is a useful mixing model where there are limited data and little or no replication of sampling (Mabit et al., 2018). Alternative SIMMs, such as MixSIAR, do have statistical outputs.
MixSIAR is a probabilistic Bayesian mixing model that generates a probability density function using a Markov Chain Monte Carlo (MCMC) method. It produces simulations of plausible values, therefore representing a true probability density function for the proportional contribution of source soils in the mixture being tested (Stock and Semmens, 2015; Stock et al., 2018). While there is essentially no difference between the results from MixSIAR and IsoSource when source-signature variability is ∼zero (Mabit et al., 2018) in this study MixSIAR was chosen to enable a measure of the uncertainty with the results to be estimated.
There are a range of fatty acid tracers which can potentially be used in mixing models. However, only those source tracers that form a polygon around the mixture can be used in the modeling process (Phillips et al., 2014), i.e., point-in-polygon (Figure 3). The model uses the mixing polygons to provide a quantitative basis for model rejection and exclusion for sediment samples which violate the point-in-polygon assumption, i.e., the mixture must lie within the bounds of a polygon of straight lines drawn through and linking the extreme source values to be used in MixSIAR (Phillips et al., 2014; Stock et al., 2018; Figure 3). From the polygon tests, we found that appropriate tracers were the δ13C values of the bulk carbon and the FAs C18:0, C22:0, C24:0 and C26:0 (Supplementary Appendix 1 and 2).
Figure 3. Examples of polygons built using the river mouth sediment and marine sediment CSSI values enclosing all of the (A) Kaikōura Canyon and (B) Hokitika Canyon surface sediment CSSI values. All sediment samples from the canyons (open circles) lie inside their respective polygons (red dashed lines) drawn through the error bar values of the potential sediment sources. The legend labeled “Combined” (Hokitika Canyon) stands for the Whataroa, Mikonui, Waitaha and Wanganui rivers. Error bars stand for one standard deviation.
MixSIAR assumes that the sediment supply is similar from all sources. In this study, the river sources were not similar in catchment area, discharge or sediment yield (Table 2). On the east coast, the Waiau River contributes more than 12 times the mean annual sediment load from the Conway River and 5 times the sediment load from the Hurunui River to the coastal waters. On the west coast, the combined Mikonui, Waitaha, Wanganui and Whataroa rivers contribute about twice the sediment load from the Hokitika River to the coastal waters. To accommodate such large differences in sediment load, the MixSIAR model has a feature that allows non-isotopic information about the sources to be included in the modeling process by the use of “priors.” As a default, the prior for each source is set to 1 as an “Uninformative” prior, i.e., the sediment supply is the same from all sources. However, where this is not the case, the prior values for each source can be changed to reflect the information being passed to the model as an “Informative” prior. In this study informative priors were developed using the relative size of the annual suspended sediment load from each river catchment, or combined river catchments (west coast rivers), and the distance of the river mouth from the head of the canyon. The ‘distance’ factor was calculated from the actual distance (km), the travel time from the river mouth to the head of the canyon and the expected settling rate of land-derived sediment in sea water. The travel time value used was 10 km d–1, which was the average speed of the longshore current measured by current meters (Chiswell, 1996) and drift-card estimates (Brodie, 1960). These gave a range 6–16 km d–1. The average settling velocity value was 1.01 m d–1 as measured in the Motueka River plume (Gibbs, 2001). This value, which was derived using a vertical array of sediment traps, represents the best available estimate of particle settling velocity for rivers in the region. The results were scaled to a numeric total value equal to the number of sources (Table 3) and were found to be less than 2% different from priors derived from the mean annual suspended sediment load alone. Because there are no suspended sediment transport data for the marine sources, and because primary productivity is similar in both regions, the prior values for these sources were left as uninformative with a value of 1. Using this approach allowed an assessment of the relative contribution of terrigenous sediment to the canyon from each river inflow, relative to the magnitude of the sediment load to the coastal waters from the catchment rivers.
The canyon samples used in this study were collected between 2010 and 2013. In order to use contemporary (2017) surficial sediment from the river sources in the MixSIAR modeling of the coastal mixtures, the isotopic values of each sediment mixture were corrected for the Suess effect to year 2017 values using the method of Verburg (2007).
Sediments from the Kaikōura and Hokitika canyons were dominated by silt-sized particles at all locations (80 to >95% silt, sandy silts), except at ∼200 m (Site H200) water depth in the head of the Hokitika Canyon where more sandy (∼50%) sediments were prevalent (Table 1). Organic carbon and nitrogen, calcium carbonate, and phytopigment (chlorophyll a and phaeopigments) concentrations were typically higher in the Kaikōura Canyon, compared to Hokitika, although the sediment C:N molar ratio was relatively elevated in the latter. Total organic carbon content of the sediment increased down-canyon at Kaikōura (0.7 to 1.2%), reflecting a progressive increase in the marine contribution to the organic carbon pool, whereas at Hokitika this parameter varied less (0.2–0.4%), except at 700 m (site H700) where 1.25% total organic carbon was observed (Table 1). Carbonate content increased with depth in Kaikōura Canyon (2–8%) but was lower and more variable in the Hokitika Canyon (0.2–1.6%). Chlorophyll a: phaeopigment ratios were slightly more elevated in the Kaikōura Canyon sediments (0.1–0.3), compared to Hokitika (0.1–0.2) (Table 1), indicating higher overall contributions of ‘fresh’ organic matter, consistent with lower C:N ratios (8–9 cf. 9–15), in Kaikōura Canyon.
The sediment bulk δ13C and δ15N values for the Kaikōura Canyon were intermediate between the lower δ13C isotopic signature of land-derived plant material, and higher δ13C and δ15N marine-derived material (Tables 1, 2 and Figure 4A). Isotopic values of some Kaikōura Canyon sediment samples lie closer to the marine source than the river source implying a greater input of organic material of marine origin. In contrast, the sediment bulk δ13C and δ15N measurements from the Hokitika Canyon samples lie within the range of isotopic values from the river sediments, implying relatively little influence from the marine source (Figure 4B).
Figure 4. Bulk δ13C and δ15N values of river, canyon and marine sediment from: (A) Kaikōura Canyon (single samples have no error bars) and (B) Hokitika Canyon. Error bars (±1 standard deviation) are shown.
Spatial representation of the contribution of land-derived particulate organic matter as a proportion of the total organic matter in the sediment (%TOM, Table 1) down the canyons as estimated with the IsoError mixing model (Phillips and Gregg, 2001) using bulk δ13C data, are shown in Figure 5. There is a relatively steep decrease in the percent contribution of terrigenous particulate organic matter moving offshore down the axis of Kaikōura Canyon (Figure 5A). The deepest canyon site, Site K12 (at 1485 m depth), is only 25 km away from the coast and comprised only 13% terrigenous total organic matter, compared to about 50% in the upper reaches of the canyon closer to the coast (Sites K1, K3, K4). In contrast, there was very little decrease in the terrigenous organic matter down the Hokitika Canyon (Figure 5B). The contribution of terrigenous organic matter was high at all Hokitika sites (74–100%), particularly at the shallowest sites, Sites H200 and H700 (200 and 700 m depth), and was still high (79%) at the deepest site, Site H2000 (2000 m depth), some 200 km away from the coast.
Figure 5. Spatial contribution (%) of land-derived organic matter to total sediment organic matter in: (A) Kaikōura Canyon and (B) Hokitika Canyon. Error terms (standard deviation) were < 5% (not shown for clarity). Numbered river catchments are: (1) Mikonui, (2) Waitaha, and (3) Wanganui.
The terrigenous organic carbon component from each of the river sources at each canyon site, as determined by MixSIAR, are presented in Figure 6 and Table 4. Across all the sites in Kaikōura Canyon, most of the terrigenous organic carbon originated from the Waiau River, which contributed at least twice as much as the Conway and Hurunui rivers combined (Figure 6A and Table 4). The proportional contribution of terrigenous organic carbon from the Hokitika River was around 35% at the nearshore site H200 (200 m depth) and increased to around 54% at Site H700 (700 m depth), before decreasing farther offshore. In contrast, the proportional contribution of terrigenous organic carbon from the other four rivers was consistently around 50% and increased to about 65% at the farthest site off shore, Site H2000 (Figure 6B).
Table 4. Land derived organic matter proportions, derived from IsoError, and source river proportions in that land derived organic matter as estimated by MixSIAR.
Figure 6. Spatial proportional contribution of terrigenous organic material from the river inflows in: (A) Kaikōura Canyon, showing the proportional contribution from the Waiau River relative to the river inputs combined, and (B) Hokitika Canyon, showing the proportional contribution from the Hokitika River relative to the other river inputs combined. Results are mean values with error terms of ± 0.7–20% as standard deviations about the mean (errors not shown for clarity). Numbered river catchments are: (1) Mikonui, (2) Waitaha, and (3) Wanganui.
It has long been recognized that substantial amounts of terrestrial sediment are discharged from New Zealand rivers into coastal waters (Milliman and Syvitski, 1992; Milliman and Farnsworth, 2013). This material is then laterally redistributed along the coast by longshore currents (Stoffers et al., 1984; Griffiths and Glasby, 1985; Hicks et al., 2011; Kuehl et al., 2016; Stevens et al., 2019). However, where that sediment finally settles is often uncertain (Foster and Carter, 1997). The present study has used stable isotopes of bulk carbon, nitrogen and individual FAs to demonstrate that the Kaikōura and Hokitika canyons are conduits for transferring land-derived organic matter to the deep ocean.
Global budgets suggest that on the order of 0.2 Pg of carbon is deposited and buried annually in continental shelf sediments (Chen, 2010) and on the adjacent slope (Jahnke, 2010) accounting for 25% of the total riverine flux of carbon to the coast (Liu et al., 2010). Submarine canyons provide a vector for transporting organic carbon to the deep ocean, and organic matter in canyon sediments has also been shown to have strong terrigenous affinities throughout the sedimentary system [Nazaré Canyon – Masson et al. (2010) and Kiriakoulakis et al. (2011); Scripps and La Jolla canyons – Vetter and Dayton (1999); Lacaze-Duthier and Cap de Creus canyons, Gulf of Lions – Schmiedl et al. (2004) and Pasqual et al. (2013); Gaoping Canyon, SW Taiwan – Kao et al. (2014); Selvaraj et al. (2015), and Liu et al. (2016)].
In the present study of two contrasting canyon systems, the terrigenous component of the organic matter in canyon sediments may represent as much as 80–100% of the total organic matter pool (Hokitika Canyon) or as little as ∼13% (Kaikōura Canyon). Although the canyons were sampled 3 years apart (2010 and 2013), this time lag is unlikely to have substantially affected our results as samples were obtained from the top 5 cm of sediment, which integrates several years to decades of sedimentation in the study areas (Probert and Swanson, 1985; Gomez et al., 2004; Alexander et al., 2010; Radford, 2012).
Deep-sea sediments within Hokitika Canyon have greater concentrations of land-derived organic matter than Kaikōura Canyon, with high (>70%) proportions of terrestrial material throughout its initial 200 km reach, presumably fed by the continuous supply of annually high suspended sediment loads (Hicks et al., 2011) and associated high fluxes of particulate organic carbon (Scott et al., 2006) delivered to the coast by west coast rivers. Because of the low gradient, sediment transport in the canyon is most likely associated with regular inputs of shelf material via longshore drift and the transport of this material by grain-flow and other gravitational mechanisms down the canyon axis, similar to La Jolla (Paull et al., 2013) and Monterey canyons (Symons et al., 2017; Paull et al., 2018) on the west coast of the United States.
The persistence of terrestrially derived organic matter throughout the Hokitika Canyon system, however, is reminiscent of other locations where steep, mountainous river catchments deliver significant quantities of sediment and associated organic matter to nearby submarine canyons, such as the Gaoping River-Canyon system in SW Taiwan (Kao et al., 2014; Liu et al., 2016). In the case of Hokitika Canyon, this observation is facilitated by soil erosion rates in the uplifted hinterland, with the west coast river catchments having sediment yields of 58 to 88 t ha–1 y–1 compared to just 2 to 8 t ha–1 y–1 in the east coast river catchments. This high sediment load is a function of the higher rainfall intensity and steeper land slopes on the west coast of South Island (Adams, 1980; Hicks et al., 2011; Jiao et al., 2017). Consequently, because the west coast river catchments are very much smaller and have higher mean annual flows than those on the east coast with more flood events per year (Booker and Whitehead, 2017) the water discharged from the west coast rivers is likely to have higher energy, potentially pushing the sediment load farther from shore where it can be intercepted by the longshore drift system and transported into the Hokitika Canyon.
High freshwater contributions in offshore environments have been recognized previously along the west coast of South Island, New Zealand, with salinity sections showing a freshwater influence out to ∼100 km from the coast (Stanton, 1976), with associated enhanced vertical stratification and water column stability, leading to localized increases in surface primary productivity, extending well offshore (Moore and Murdoch, 1993). The combination of cooler freshwater delivered from the large alpine catchments following prolonged drought and summer ocean warming may generate conditions to drive dense-water cascading which can initiate dense sediment-laden flows at the canyon heads and submarine gullies (Micallef and Mountjoy, 2011). The conditions for dense water formation are poorly known here and have not been quantified with respect to down canyon flows on the west coast of South Island but may be an important sediment transport process. Similarly, other oceanographic processes that are known to operate on the west coast, such as coastal wind-driven upwelling and coastally trapped wave activity (Cahill et al., 1991; Stevens et al., 2019) have not been investigated to determine their relevance to canyon processes as observed elsewhere (Inman et al., 1976; She and Klinck, 2000; Allen and Durrieu de Madron, 2009; Mountjoy et al., 2014).
Only 50% of the organic carbon in Kaikōura Canyon head is land-derived, decreasing to 13% over the 25 km length of the sampled canyon axis. This finding suggests that Kaikōura Canyon transports less terrigenous organic matter to the deep sea than Hokitika Canyon, although it is also possible that the steeper topography of Kaikōura canyon results in more efficient flushing of terrigenous organic matter to deeper parts of the canyon (>1500 m) over short timescales (inter-seismic), much like steep mountain rivers accelerate carbon transfer (Hilton et al., 2011). Major sediment movement in the Kaikōura Canyon is driven by large earthquakes resulting in canyon flushing, as occurred most recently with the November 2016 MW7.8 Kaikōura earthquake with a calculated inter-seismic event time of 140 years (Clark et al., 2017; Hamling et al., 2017; Mountjoy et al., 2018). Based on littoral zone sediment budgets previous studies have hypothesized that sand and gravel turbidity currents may have a recurrence of 150 years (Barnes, 1996). Mountjoy et al. (2018) calculated that the equivalent of two to four times the annual terrestrial load from all New Zealand rivers (Hicks et al., 2011) was delivered from the upper canyon to the deep ocean in the single earthquake event in November 2016, accounting for up to 7 Mt of carbon, or twice the annual terrestrial particulate organic carbon riverine flux for New Zealand (Scott et al., 2006).
Since the sediment sampling in the Kaikōura Canyon was undertaken prior to the 2016 Kaikōura earthquake, it is relevant to consider sediment transport processes that most likely occur between such episodic events. Lewis and Barnes (1999) describe the movement of silt and sand-sized particles along the continental shelf in the longshore drift system, where the material is then captured by the head of the canyon. Contrary to Lewis and Barnes (1999), however, Lane et al. (2018) argue that the accumulation of a potentially unstable mass of sediment at the rim of the canyon head was not occurring, suggesting that deeper, larger canyon-margin and slope failures were more apparent. The rapid dilution of a terrigenous signal in the organic carbon pool in the Kaikōura Canyon sediments is consistent with the accumulation and staging of land-derived sediment in the upper parts of the canyon, which can then be excavated and removed by episodic earthquake events such as during the 2016 Kaikōura earthquake (i.e., up to 50 m of vertical erosion in the canyon head, with annualized local incision rates of 40 ± 11 mm y–1; Mountjoy et al., 2018). Important inter-seismic sediment transport processes within the canyon are likely to include large storm events and internal tidal pumping (Puig et al., 2014).
With respect to similar tectonic events on the Alpine Fault or other faults in the proximity of the Hokitika Canyon, it is anticipated that potentially more substantial and catastrophic episodes are likely to occur in this canyon and others on the west coast of South Island, especially given the level of ground-shaking expected with a future rupture scenario on the Alpine Fault (Sutherland et al., 2007; Howarth et al., 2018). The reverse Cape Foulwind Fault extends across the shelf east of the Hokitika Canyon head and parallel to the coast and may also be capable of generating sizeable earthquakes (MW7.5) with large single event displacements (∼5 m) (Barnes and Ghisetti, 2016). Therefore, while presently high accumulation and transport of terrestrial organic matter appears to occur down the length of Hokitika Canyon, and potentially the other canyons along the west coast of South Island (Mitchell et al., 2012), random or episodic tectonic events may also be a factor in the long term transfer of organic carbon to the deep ocean in this region.
Although there is little difference in annual primary productivity between the two systems (i.e., ∼160 to 180 g C m–2 y–1; Bradford, 1972; Probert, 1986; Zeldis et al., 2010) there is a proportionally higher influence of marine deposition in Kaikōura Canyon, compared to the Hokitika Canyon. This inconsistency likely stems from the proximity of the Kaikōura Canyon to the productive Southland-Current and Subtropical Front, and the occurrence of production-favorable canyon upwelling (Heath, 1982; Sutherland, 1995; Chiswell and Schiel, 2001; Chiswell et al., 2015). Greater inputs of marine organic matter to the seabed in Kaikōura Canyon could help explain the relatively rapid dilution of the land organic matter signal, particularly in the deeper parts of the canyon. The short and steep topography of Kaikōura Canyon may also capture and funnel sinking marine particulates more proximally and efficiently than the comparatively long and narrow Hokitika Canyon.
The CSSI results suggest that the majority of the sediment in the Hokitika Canyon on the west coast of South Island comes from the four alpine rivers that were combined for modeling purposes (i.e., Mikonui, Waitaha, Wanganui and Whataroa rivers) south of the canyon, although the Hokitika River is the largest (and closest) individual river source, contributing 35 to 54% at the inshore end and <30% farther down the canyon. It seems likely, however, that a relatively high proportion of contemporary Hokitika River sediment is bypassing the Hokitika Canyon due to the local north-easterly longshore flow (Radford, 2012).
The four alpine rivers were modeled as a combined source using the isotopic data from the Whataroa River only. While this approach may increase the uncertainty in the model output, we consider that the isotopic data from the Whataroa River to be reasonably representative of the four alpine rivers, since they have essentially the same land use and morphology in their catchments and experience the same weather patterns. In addition, with isotope mixing models, it is regarded as best practice to combine sources where they are similar and difficult to discriminate (Phillips et al., 2014). Because the Whataroa River was farthest south and was positively identified in the sediment from all sites along the Hokitika canyon, the other three rivers between the Whataroa and Hokitika rivers must also be contributing. Consequently, we have chosen to express the proportional contribution as that of the combined rivers relative to the proportion from the Hokitika River, which we were able to discriminate individually as a source.
Of interest is that the highest proportion of organic matter from the combined rivers was found at the farthest offshore site, which also had the lowest proportion of Hokitika River sediment. Because the longshore drift along this section of coast is from south to north (Chiswell et al., 2015; Stevens et al., 2019) this result may reflect a northerly drift of a proportion of the Hokitika River sediment away from the canyon, consistent with the expectations of Radford (2012). Another possibility is that the sediment discharged from the Hokitika River, which has a relatively large area of pasture farmland, has a higher proportion of agricultural soil that could be less dense than the organic matter from beneath native beech forest in the catchments of the combined alpine rivers. This difference in land use may lead to more of this organic material staying in suspension and being transported along-shore in the littoral drift system and/or Westland Current, thus lowering the likelihood of entering the canyon. Further work is required to better understand this phenomenon.
Notwithstanding these observations, a similar decrease in terrigenous soil proportions was found in the Kaikōura Canyon from land which is predominantly pastoral farmland. While the expectation was for a high proportion of the sediment in the Kaikōura Canyon to be derived from the more proximal Conway River (Carter and Herzer, 1979; Carter et al., 1982) the CSSI results indicate that the majority of the land-derived sediment was from the Waiau River farther south of the canyon. Overall, the Waiau River contributed about 75% of the land-derived organic matter to the sediments in the canyon, which is in keeping with the large catchment, higher river flows and higher sediment yield of this river compared to other potential river sources.
The present study adds to the growing evidence showing that the footprint of human activities on land extends well beyond the coast and continental shelf through the transport of sediments and associated materials (including potential pollutants) via a variety of pathways including canyon transport (Ramirez-Llodra et al., 2011; Kane and Clare, 2019). As we have identified earlier, a variety of processes are likely to be involved in determining which river(s) contribute most of the terrestrial organic matter transported to the deep-sea. Regardless of which mechanism(s) are involved, the transport of organic matter to the deep is likely to have two main consequences, namely: (1) an impact on secondary productivity through the provision of an additional food source to benthic animals (see companion paper by Leduc et al., 2020) and (2) providing a significant carbon sink through the transfer, burial and sequestration of relatively refractory terrestrial carbon to the deep seabed.
Short mountainous rivers like those on the west coast of the South Island contribute disproportionately to the delivery of freshwater and sediment to the global coastal ocean (Milliman and Syvitski, 1992; Milliman and Farnsworth, 2013; Bao et al., 2015). This is also the case in New Zealand, where the Hokitika and other west coast rivers (Mikonui, Waitaha, Wanganui and Whataroa) contribute a disproportionate amount of sediment (Hicks et al., 2011) and organic carbon (dissolved and particulate; Scott et al., 2006) to national inventories (Milliman and Farnsworth, 2013). Riverine particulate organic carbon yield from these rivers represents 20–40 t C km–2 y–1, well in excess of the average for all New Zealand rivers of 10 ± 3 t C km–2 y–1, which together add up to a total of 2.7 ± 1.0 × 106 t C y–1 (Scott et al., 2006). The latter figure represents 25–34% of the estimate for all Oceania (Kao et al., 2014). It has been suggested that Oceania’s small mountainous rivers are globally significant conduits of terrestrial particulate organic carbon to the deep sea (Bao et al., 2015). Coupled with the fact that the remineralization of terrestrial organic matter in the oceans is much less efficient than that of more labile marine organic matter (Thomas et al., 2002; Blair et al., 2004), it appears likely that small mountainous rivers play an important role in the burial and sequestration of atmospheric CO2, thus impacting the global carbon cycle (Burdige, 2005; Muller-Karger et al., 2005; Chen, 2010; Jahnke, 2010). Furthermore, the run-out distance and turbulent transport mechanisms within turbidity currents that transport terrestrial materials offshore via submarine canyons and other sediment gravity flow processes are likely to be reduced by the incorporation of organic matter, promoting organic carbon burial and preservation (Craig et al., 2020). Further work is needed to determine the rate of terrestrial particulate organic carbon transport to the deep ocean by New Zealand rivers and thus gauge the magnitude of this carbon sink in the region. However, if we consider that New Zealand’s total riverine particulate organic carbon yield is equivalent to about a third of the country’s total fossil fuel emissions (Scott et al., 2006), then sequestration of land carbon by the deep ocean seabed may be substantial.
Climate change is likely to impact the transport of terrestrial organic matter to the ocean. As climate change alters the rainfall patterns across New Zealand (Reisinger et al., 2010), the expectation is for the west coast to become wetter therefore increasing the potential erosion and transport of land-derived organic carbon to the coast and hence to the deep-sea. Conversely, the east coast is expected to become drier, which could reduce the amount of land-derived organic carbon reaching the Kaikōura Canyon. In addition to potentially changing the rate of carbon sequestration by the deep-sea, shifts in the magnitude of terrestrial carbon transport may also have implications for food webs. Leduc et al. (2020) provide evidence supporting a trophic link between terrestrial organic matter and benthic communities in Kaikōura Canyon. Therefore, decreased land-to-ocean subsidies could reduce the productivity of this unique ecosystem (De Leo et al., 2010).
Our results show that the Hokitika Canyon on the west coast of South Island, New Zealand has an efficient axial transport mechanism for moving terrigenous material from the continental shelf into the deep-sea. West coast South Island rivers transfer substantial amounts of terrigenous material to the ocean, which is delivered to the Hokitika Canyon via along shore processes in the coastal zone. In-canyon processes transport terrigenous organic material at least 200 km offshore. In contrast, the Kaikōura Canyon is more strongly influenced by other processes down the canyon axis. Nearby rivers deliver relatively limited amounts of terrigenous organic matter, which is diluted rapidly by increasing marine production-derived material down the short length of the canyon, only ∼25 km from the coast. Episodic events (e.g., earthquake-triggered canyon flushing) also play a significant role in moving material farther into the deep ocean, as evinced with recent events in Kaikōura Canyon, but as yet unquantified for Hokitika Canyon. The majority of organic matter from rivers in submarine canyon sediments does not necessarily originate from the river closest to the canyon heads. Instead, our results show that the relative magnitude of river suspended sediment loads and along-shore processes are more important determinants. Linking the proportional contribution of terrigenous sediment and organic matter from each river source at various locations down a submarine canyon provides new understanding of how these mechanisms may be affected by land use in the river catchment. We found evidence of advection of the sediment by longshore drift currents, showing that oceanographic processes on the shelf also have an influence on the distribution and delivery of sediments to canyons. Sedimentation in submarine canyons is affected by a variety of tectonic, climatic and oceanographic processes. The efficiency with which organic matter is captured and transferred to the deep-sea by canyons demonstrates the potential for such systems to act as natural carbon sinks, driven by both geologically episodic and more regular oceanographic processes.
The raw data supporting the conclusions of this article will be made available by the authors, without undue reservation.
MG, DL, SN, and AS designed the study. MG, AS, DL, AR, and JM collected the samples and data. MG, AK, SB, JB, GO, RO, and BG analyzed the samples and data. MG, AK, AS, DL, SN, and SB interpreted the results. MG, DL, SN, and AR wrote first drafts of the manuscript text and produced the figures. All authors read, commented, and edited the final draft.
Funding for this study was provided by the Ministry of Business, Innovation and Employment through the National Science Challenge Sustainable Seas project (C01X1515 “Submarine canyons: how important are they for connecting coastal and deep-sea ecosystems?”). Samples that were used for this study were collected on several voyages, funded by various research programs over several years. These included New Zealand-based projects (e.g., Core and Strategic Science Investment Funds: Ocean Flows and Productivity and Marine Geological Processes) funded and administered presently by NIWA’s National Centre for Coasts and Oceans, and those funded by international agencies, in particular the RENEWZ project (NOAA Office of Ocean Exploration grant no. NA05OAR4171076).
The authors declare that the research was conducted in the absence of any commercial or financial relationships that could be construed as a potential conflict of interest.
We thank student assistants on TAN1006 (Richard O’Rorke, Simon Connell, both University of Auckland), Joe Prebble (Victoria University of Wellington, now GNS Science), Krystyna Muller (Bonn University, Germany). Anna Kilimnik and Josette Delgado for processing stable isotope samples, and Lisa Northcote (all NIWA) for analyzing sediment samples, Sarah Fraser (NIWA) for her support with publication, and NIWA science staff on TAN1311 for help at-sea processing samples. We are grateful to three reviewers for providing constructive criticisms on the manuscript.
The Supplementary Material for this article can be found online at: https://www.frontiersin.org/articles/10.3389/fmars.2020.00608/full#supplementary-material
Adams, J. (1980). Contemporary uplift and erosion of the Southern Alps, New Zealand. GSA Bull. 91, 1–114. doi: 10.1130/gsab-p2-91-1
Alewell, C., Birkholz, A., Meusburger, K., Schindler Wildhaber, Y., and Mabit, L. (2016). Quantitative sediment source attribution with compound-specific isotope analysis in a C3 plant-dominated catchment (central Switzerland). Biogeosciences 13, 1587–1596. doi: 10.5194/bg-13-1587-2016
Alexander, C., Walsh, J., and Orpin, A. (2010). Modern sediment dispersal and accumulation on the outer Poverty continental margin. Mar. Geol. 270, 213–226. doi: 10.1016/j.margeo.2009.10.015
Allen, S. E., and Durrieu de Madron, X. (2009). A review of the role of submarine canyons in deep-ocean exchange with the shelf. Ocean Sci. 5, 607–620. doi: 10.5194/os-5-607-2009
Alt-Epping, U., Mil-Homens, M., Hebbeln, D., Abrantes, F., and Schneider, R. R. (2007). Provenance of organic matter and nutrient conditions on a river- and upwelling influenced shelf: a case study from the Portugese Margin. Mar. Geol. 243, 169–179. doi: 10.1016/j.margeo.2007.04.016
Amiel, D., and Cochran, J. K. (2008). Terrestrial and marine POC fluxes derived from 234Th distributions and 13C measurements on the Mackenzie Shelf. J. Geophys. Res. 113:C03S06.
Bao, H., Lee, T. Y., Huang, C., Feng, X., Dai, M., and Kao, S. J. (2015). Importance of Oceanian small mountainous rivers (SMRs) in global land-to-ocean output of lignin and modern biospheric carbon. Sci. Rep. 5:16217.
Barnes, P. M. (1996). Active folding of Pleistocene unconformities on the edge of the Australian-Pacific plate boundary zone, offshore North Canterbury, New Zealand. Tectonics 15, 623–640. doi: 10.1029/95tc03249
Barnes, P. M., and Ghisetti, F. C. (2016). Structure, late Quaternary slip rate and earthquake potential of marine reverse faults along the North Westland deformation front, New Zealand. N. Z. J. Geol. Geophys. 59, 157–175. doi: 10.1080/00288306.2015.1112816
Barnes, P. M., Lépinay, B. M., Collot, J. Y., Delteil, J., and Audru, J. C. (1998). Strain partitioning in the transition area between oblique subduction and continental collision, Hikurangi margin, New Zealand. Tectonics 17, 534–557. doi: 10.1029/98tc00974
Baudin, F., Stetten, E., Schnyder, J., Charlier, K., Martinez, P., Dennielou, B., et al. (2017). Origin and distribution of the organic matter in the distal lobe of the Congo deep-sea fan – A rock-eval survey. Deep Sea Res. Part II Top. Stud. Oceanogr. 142, 75–90. doi: 10.1016/j.dsr2.2017.01.008
Belicka, L. L., and Harvey, H. R. (2009). The sequestration of terrestrial organic carbon in Arctic Ocean sediments: a comparison of methods and implications for regional carbon budgets. Geochim. Cosmochim. Acta 73, 6231–6248. doi: 10.1016/j.gca.2009.07.020
Benner, R., Benitez-Nelson, B., Kaiser, K., and Amon, R. M. W. (2004). Export of young terrigenous dissolved organic carbon from rivers to the Arctic Ocean. Geophys. Res. Lett. 31:L05305.
Blair, N. E., and Aller, R. C. (2012). The fate of terrestrial organic carbon in the marine environment. Ann. Rev. Mar. Sci. 4, 401–423. doi: 10.1146/annurev-marine-120709-142717
Blair, N. E., Leithold, E. L., and Aller, R. C. (2004). From bedrock to burial: the evolution of particulate organic carbon across coupled watershed-continental margin systems. Mar. Chem. 92, 141–156. doi: 10.1016/j.marchem.2004.06.023
Blake, W. H., Ficken, K. J., Taylor, P., Russell, M. A., and Walling, D. E. (2012). Tracing crop-specific sediment sources in agricultural catchments. Geomorphology 139, 322–329. doi: 10.1016/j.geomorph.2011.10.036
Booker, D. J., and Whitehead, A. L. (2017). NZ River Maps: An Interactive Online Tool for Mapping Predicted Freshwater Variables Across New Zealand. Christchurch: NIWA.
Bostock, H., Jenkins, C., Mackay, K., Carter, L., Nodder, S., Orpin, A., et al. (2019a). Distribution of surficial sediments in the ocean around New Zealand/Aotearoa. Part A: continental slope and deep ocean. N. Z. J. Geol. Geophys. 62, 1–23. doi: 10.1080/00288306.2018.1523198
Bostock, H., Jenkins, C., Mackay, K., Carter, L., Nodder, S., Orpin, A., et al. (2019b). Distribution of surficial sediments in the ocean around New Zealand/Aotearoa. Part B: continental shelf. N. Z. J. Geol. Geophys. 62, 24–45. doi: 10.1080/00288306.2018.1523199
Bouloubassi, I., Lipiatou, E., Saliot, A., Tolosa, I., Bayona, J. M., and Albaiges, J. (1997). Carbon sources and cycle in the western Mediterranean – the use of molecular markers to determine the origin of organic matter. Deep Sea Res. Part II Top. Stud. Oceanogr. 44, 781–799. doi: 10.1016/s0967-0645(96)00094-x
Bradford, J. M. (1972). Systematics and Ecology of New Zealand Central East Coast Plankton Sampled at Kaikoura. New Zealand Oceanographic Institute Memoir 54. Wellington: New Zealand Oceanographic Institute, 89.
Bradford-Grieve, J. M., Chang, F. H., Gall, M., and Pickmere, R. F. (1997). Size-fractionated phytoplankton standing stocks and primary production during austral winter and spring 1993 in the Subtropical Convergence region near New Zealand. N. Z. J. Mar. Freshwater Res. 31, 201–224. doi: 10.1080/00288330.1997.9516759
Brandt, C., Benmansour, M., Walz, L., Nguyen, L. T., Cadisch, G., and Rasche, F. (2018). Integrating compound-specific δ13C isotopes and fallout radionuclides to retrace land use type-specific net erosion rates in a small tropical catchment exposed to intense land use change. Geoderma 310, 53–64. doi: 10.1016/j.geoderma.2017.09.008
Brandt, C. G., Cadisch, L. T., Nguyen, T., Vien, D., and Rasche, F. (2016). Compound-specific δ13C isotopes and Bayesian inference for erosion estimates under different land use in Vietnam. Geoderma Reg. 7, 311–322. doi: 10.1016/j.geodrs.2016.06.001
Bravo-Linares, C., Schuller, P., Castillo, A., Ovando-Fuentealba, L., Muñoz-Arcos, E., Alarcón, O., et al. (2018). First use of a compound-specific stable isotope (CSSI) technique to trace sediment transport in upland forest catchments of Chile. Sci. Total Environ. 618, 1114-1124. doi: 10.1016/j.scitotenv.2017.09.163
Brodie, J. W. (1960). Coastal currents around New Zealand. N. Z. J. Geol. Geophys. 3, 235–252. doi: 10.1080/00288306.1960.10423596
Burdige, D. J. (2005). Burial of terrestrial organic matter in marine sediments: a re-assessment. Glob. Biogeochem. Cycles 19:GB4011.
Cahill, M. L., Middleton, J. H., and Stanton, B. R. (1991). Coastal-trapped waves on the west coast of South Island, New Zealand. J. Phys. Oceanogr. 21, 541–557. doi: 10.1175/1520-0485(1991)021<0541:ctwotw>2.0.co;2
Canals, M., Puig, P., Durrieude Madron, X., Heussner, S., Palanques, A., and Fabres, J. (2006). Flushing submarine canyons. Nature 444, 354–357. doi: 10.1038/nature05271
Carter, L., Carter, R. M., and Griggs, G. B. (1982). Sedimentation in the Conway Trough, a deep near-shore marine basin at the junction of the Alpine transform and Hikurangi subduction plate boundary, New Zealand. Sedimentology 29, 475–497. doi: 10.1111/j.1365-3091.1982.tb01731.x
Carter, L., and Heath, R. A. (1975). Role of mean circulation, tides, and waves in the transport of bottom sediment on the New Zealand continental shelf. N. Z. J. Mar. Freshw. Res. 9, 423–448. doi: 10.1080/00288330.1975.9515579
Carter, L., and Herzer, R. H. (1979). The hydraulic regime and its potential to transport sediment on the Canterbury continental shelf. N. Z. Oceanogr. Inst. Memoir 83:33.
Chen, C.-T. A. (2010). “Cross-boundary exchanges of carbon and nitrogen in continental margins,” in Carbon and Nutrient Fluxes in Continental Margins: A Global Synthesis. Global Change - The IGBP Series, eds K.-K. Liu, L. Atkinson, R. A. Quiñones, and L. Talaue-McManus (Berlin: Springer), 561–574. doi: 10.1007/978-3-540-92735-8_13
Chiswell, S. M. (1996). Variability in the Southland Current, New Zealand. N. Z. J. Mar. Freshw. Res. 30, 1–17. doi: 10.1080/00288330.1996.9516693
Chiswell, S. M., Bostock, H. C., Sutton, P. J. H., and Williams, M. J. M. (2015). Physical oceanography of the deep seas around New Zealand: a review. N. Z. J. Mar. Freshw. Res. 49, 286–317. doi: 10.1080/00288330.2014.992918
Chiswell, S. M., and Schiel, D. R. (2001). Influence of along-shore advection and upwelling on coastal temperature at Kaikoura Peninsula, New Zealand. N. Z. J. Mar. Freshw. Res. 35, 307–317. doi: 10.1080/00288330.2001.9517000
Clark, K. J., Nissen, E. K., Howarth, J. D., Hamling, I. J., Mountjoy, J. J., Ries, W. F., et al. (2017). Highly variable coastal deformation in the 2016 MW7.8 Kaikôura earthquake reflects rupture complexity along a transpressional plate boundary. Earth Planet. Sci. Lett. 474, 334–344. doi: 10.1016/j.epsl.2017.06.048
Craig, M. J., Baas, J. H., Amos, K. J., Strachan, L. J., Manning, A. J., Paterson, D. M., et al. (2020). Biomediation of submarine sediment gravity flow dynamics. Geology 48, 72–76. doi: 10.1130/g46837.1
Dagg, M., Benner, R., Lohrenz, S., and Lawrence, D. (2004). Transformation of dissolved and particulate materials on continental shelves influenced by large rivers: plume processes. Cont. Shelf Res. 24, 833–858. doi: 10.1016/j.csr.2004.02.003
De Leo, F. C., Smith, C. R., Rowden, A. A., Bowden, D. A., and Clark, M. R. (2010). Submarine canyons: hotspots of benthic biomass and productivity in the deep sea. Proc. Biol. Sci. 277, 2783–2792. doi: 10.1098/rspb.2010.0462
Degens, E. T., Kempe, S., and Richey, J. E. (1991). Summary: Biogeochemistry of Major World Rivers. SCOPE 42. New York, NY: Wiley, 356.
Foster, G., and Carter, L. (1997). Mud sedimentation on the continental shelf at an accretionary margin—Poverty Bay, New Zealand. N. Z. J. Geol. Geophys. 40, 157–173. doi: 10.1080/00288306.1997.9514750
Gibbs, M., and Olsen, G. (2010). Bay of Islands OS20/20 Survey Report Chapter 05: Determining Sediment Sources and Dispersion in the Bay of Islands. NIWA Client Report WLG2010-38. Christchurch: NIWA, 30
Gibbs, M., Olsen, G., Swales, A., and He, S. (2012). Kaipara Harbour Sediment Tracing: Sediment Dispersion Across the Harbour. NIWA Client Report Number HAM2011-091 Prepared for the Integrated Kaipara Harbour Management Group. Available online at: http://www.kaiparaharbour.net. nz/Content/Publications/Gibbsetal2012KaiparaHarbourSedimentTracingFinal.pdf (accessed March 1, 2020).
Gibbs, M. M. (2001). Sedimentation, suspension, and resuspension in Tasman Bay and Beatrix Bay, New Zealand, two contrasting coastal environments which thermally stratify in summer. N. Z. J. Mar. Freshw. Res. 35, 951–970. doi: 10.1080/00288330.2001.9517056
Gibbs, M. M. (2008). Identifying source soils in contemporary estuarine sediments: a new compound-specific isotope method. Estuaries Coast. 31, 344–359. doi: 10.1007/s12237-007-9012-9
Gibbs, M. M. (2014). Protocols on the use of Compound-Specific Stable Isotopes to iDentify and Apportion Soil Sources from Land Use. Revised 2013. Contract report to Joint FAO/IAEA Division of Nuclear Techniques in Food and Agriculture. Available online at: http://www-naweb.iaea.org/nafa/swmn/public/CSSI-technique-protocols-revised-2013.pdf (accessed March 1, 2020).
Gomez, B., Carter, L., Trustrum, N. A., Palmer, A. S., and Roberts, A. P. (2004). El Niño–Southern Oscillation signal associated with middle Holocene climate change in intercorrelated terrestrial and marine sediment cores, North Island, New Zealand. Geology 32, 653–656.
Goñi, M. A., Ruttenberg, K. C., and Eglinton, T. I. (1997). Sources and contribution of terrigenous organic carbon to surface sediments in the Gulf of Mexico. Nature 389, 275–278. doi: 10.1038/38477
Goñi, M. A., Yunker, M. B., Macdonald, R. W., and Eglinton, T. I. (2000). Distribution and sources of organic biomarkers in arctic sediments from the Mackenzie River and Beaufort Shelf. Mar. Chem. 71, 23–51. doi: 10.1016/s0304-4203(00)00037-2
Griffiths, G. A., and Glasby, G. P. (1985). Input of river-derived sediment to the New Zealand continental shelf: I. Mass. Estuar. Coastal Shelf Sci. 21, 773–787. doi: 10.1016/0272-7714(85)90072-1
Hage, S., Cartigny, M. J. B., Sumner, E. J., Clare, M. A., Hughes Clarke, J. E., Talling, P. J., et al. (2019). Direct monitoring reveals initiation of turbidity currents from extremely dilute river plumes. Geophys. Res. Lett. 46, 11310–11320. doi: 10.1029/2019GL084526
Hamling, I. J., Hreinsdóttir, S., Clark, K., Elliott, J., Liang, C., Fielding, E., et al. (2017). Complex multifault rupture during the 2016 Mw 7.8 Kaikôura earthquake, New Zealand. Science 356:eaam7194.
Hancock, G. J., and Revill, A. T. (2013). Erosion source discrimination in a rural Australian catchment using compound-specific isotope analysis (CSIA). Hydrol. Process. 27, 923–932. doi: 10.1002/hyp.9466
Hastings, R. H., Goñi, M. A., Wheatcroft, R. A., and Borgeld, J. C. (2012). A terrestrial organic matter depocenter on a high-energy margin: the Umpqua River system, Oregon. Cont. Shelf Res. 39-40, 78–91. doi: 10.1016/j.csr.2012.04.002
Heath, R. A. (1982). What drives the mean circulation on the New Zealand west coast continental shelf. N. Z. J. Mar. Freshw. Res. 16, 215–226. doi: 10.1080/00288330.1982.9515964
Hedges, J. I., and Keil, R. G. (1995). Sedimentary organic matter preservation: an assessment and speculative synthesis. Mar. Chem. 49, 81–115. doi: 10.1016/0304-4203(95)00008-f
Hedges, J. I., Keil, R. G., and Benner, R. (1997). What happens to terrestrial organic matter in the ocean? Org. Geochem. 27, 195–212. doi: 10.1016/s0146-6380(97)00066-1
Herzer, R. H. (1979). Submarine slides and submarine canyons on the continental slope off Canterbury, New Zealand. N. Z. J. Geol. Geophys. 22, 391–406. doi: 10.1080/00288306.1979.10424107
Hicks, D. M., Shankar, U., McKerchar, A. I., Basher, L., Lynn, I., Page, M., et al. (2011). Suspended sediment yields from New Zealand rivers. J. Hydrol. 50, 81–142.
Hilton, R. G., Galy, A., Hovius, N., Horng, M. J., and Chen, H. (2011). Efficient transport of fossil organic carbon to the ocean by steep mountain rivers: an orogenic carbon sequestration mechanism. Geology 39, 71–74. doi: 10.1130/g31352.1
Howarth, J. D., Cochran, U. A., Langridge, R. M., Clark, K., Fitzsimons, S. J., Berryman, K., et al. (2018). Past large earthquakes on the Alpine Fault: paleoseismological progress and future directions. N. Z. J. Geol. Geophys. 61, 309–328. doi: 10.1080/00288306.2018.1464658
Hsu, F. H., Su, C. C., Wang, C. H., Lin, S., Liu, J., and Huh, C. A. (2014). Accumulation of terrestrial organic carbon on an active continental margin offshore southwestern Taiwan: source-to-sink pathways of river-borne organic particles. J. Asian Earth Sci. 91, 163–173. doi: 10.1016/j.jseaes.2014.05.006
Inman, D. L., Nordstrom, C. E., and Flick, R. E. (1976). Currents in submarine canyons: an air-sea-land interaction. Annu. Rev. Fluid Mech. 8, 275–310. doi: 10.1146/annurev.fl.08.010176.001423
Jahnke, R. A. (2010). “Global synthesis,” in Carbon and Nutrient fluxes in Continental Margins: A Global Synthesis. Global Change - The IGBP Series, eds K.-K. Liu, L. Atkinson, R. A. Quiñones, and L. Talaue-McManus (Berlin: Springer), 597–615.
Jiao, R., Herman, F., and Seward, D. (2017). Late Cenozoic exhumation model of New Zealand: impacts from tectonics and climate. Earth Sci. Rev. 166, 286–298. doi: 10.1016/j.earscirev.2017.01.003
Kaiser, A., Balfour, N., Fry, B., Holden, C., Litchfield, N., Gerstenberger, M., et al. (2017). The 2016 Kaikôura, New Zealand, earthquake: preliminary seismological report. Seismol. Res. Lett. 88, 727–739. doi: 10.1785/0220170018
Kandasamy, S., and Nath, B. N. (2016). Perspectives on the terrestrial organic matter transport and burial along the land-deep sea continuum: caveats in our understanding of biogeochemical processes and future needs. Front. Mar. Sci. 3:259. doi: 10.3389/fmars.2016.00259
Kane, I. A., and Clare, M. A. (2019). Dispersion, accumulation, and the ultimate fate of microplastics in deep-marine environments: a review and future directions. Front. Earth Sci. 7:80. doi: 10.3389/feart.2019.00080
Kao, S. J., Hilton, R. G., Selvaraj, K., Dai, M., Zehetner, F., Huang, J. C., et al. (2014). Preservation of terrestrial organic carbon in marine sediments offshore Taiwan: mountain building and atmospheric carbon dioxide sequestration. Earth Surf. Dyn. 2, 127–139. doi: 10.5194/esurf-2-127-2014
Kiriakoulakis, K., Blackbird, S., Ingels, J., Vanreusel, A., and Wolff, G. A. (2011). Organic geochemistry of submarine canyons: The Portuguese Margin. Deep Sea Res. Part II Top. Stud. Oceanogr. 58, 2477–2488. doi: 10.1016/j.dsr2.2011.04.010
Kuehl, S. A., Alexander, C. R., Blair, N. E., Harris, C. K., Marsaglia, K. M., Ogston, A. S., et al. (2016). A source-to-sink perspective of the Waipaoa River margin. Earth Sci. Rev. 153, 301–334. doi: 10.1016/j.earscirev.2015.10.001
Lane, E., Mountjoy, J., Orpin, A., and Rowden, A. (2018). “Submarine landslides and turbidity currents,” in Shaky Shores - Coastal Impacts & Responses to the 2016 Kaikoura earthquakes., eds C. Hendtlass, J. Borrero, D. Neale, and T. Shand (Wellington: New Zealand Coastal Society), 12–14.
Leduc, D., Nodder, S. D., Rowden, A. A., Gibbs, M., Berkenbusch, K., Wood, A., et al. (2020). Structure of infaunal communities in New Zealand submarine canyons is linked to origins of sediment organic matter. Limnol. Oceanogr. doi: 10.1002/lno.11454
Lewis, K. B. (1994). The 1500-km-long Hikurangi Channel: trench-axis channel that escapes its trench, crosses a plateau, and feeds a fan drift. Geomar. Lett. 14, 19–28. doi: 10.1007/bf01204467
Lewis, K. B., and Barnes, P. M. (1999). Kaikoura Canyon, New Zealand: active conduit from near-shore sediment zones to trench-axis channel. Mar. Geol. 162, 39–69. doi: 10.1016/s0025-3227(99)00075-4
Lewis, K. B., and Pantin, H. M. (2002). Channel-axis, overbank and drift sediment waves in the southern Hikurangi Trough, New Zealand. Mar. Geol. 192, 123–151. doi: 10.1016/s0025-3227(02)00552-2
Liu, J. T., Hsu, R. T., Hung, J. J., Chang, Y.-P., Wang, Y.-H., Rendle-Bühring, R. H., et al. (2016). From the highest to the deepest: the Gaoping River–Gaoping Submarine Canyon dispersal system. Earth Sci. Rev. 153, 274–300. doi: 10.1016/j.earscirev.2015.10.012
Liu, K.-K., Atkinson, L., Quiñones, R. A., and Talaue-McManus, L. (2010). “Biogeochemistry of continental margins in a global context,” in Carbon and Nutrient Fluxes in Continental Margins: A Global Synthesis. Global Change – The International Geosphere-Biosphere Programme Series, eds K.-K. Liu, L. Atkinson, R. A. Quiñones, and L. Talaue-McManus (Berlin: Springer), 3–24. doi: 10.1007/978-3-540-92735-8_1
Ludwig, W., Probst, J. L., and Kempe, S. (1996). Predicting the oceanic input or organic carbon by continental erosion. Glob. Biogeochem. Cycles 10, 23–41. doi: 10.1029/95gb02925
Mabit, L., Gibbs, M., Mbaye, M., Meusburger, K., Toloza, A., Resch, C., et al. (2018). Novel application of a Compound Specific Stable Isotope (CSSI) technique to investigate on-site sediment origins across arable fields. Geoderma 316, 19–26. doi: 10.1016/j.geoderma.2017.12.008
Mannino, A., and Harvey, H. R. (1999). Lipid composition in particulate and dissolved organic matter in the Delaware Estuary: sources and diagenetic patterns. Geochim. Cosmochim. Acta 63, 2219–2235. doi: 10.1016/s0016-7037(99)00128-3
Markus, M., and Demissie, M. (2006). Predictability of annual sediment loads based on flood events. J. Hydrol. Eng. 11, 354–361. doi: 10.1061/(asce)1084-0699(2006)11:4(354)
Masson, D. G., Huvenne, V. A. I., de Stigter, H. C. F., Wolff, G. A., Kiriakoulakis, K., Arzola, R. G., et al. (2010). Efficient burial of carbon in a submarine canyon. Geology 38, 831–834. doi: 10.1130/g30895.1
Micallef, A., and Mountjoy, J. J. (2011). A topographic signature of a hydrodynamic origin for submarine gullies. Geology 39, 115–118. doi: 10.1130/g31475.1
Milliman, J. D., and Farnsworth, K. L. (2013). River Discharge to the Coastal Ocean: A Global Synthesis. Cambridge: Cambridge University Press.
Milliman, J. D., and Syvitski, J. P. M. (1992). Geomorphic/tectonic control of sediment discharge to the ocean: the importance of small mountainous rivers. J. Geol. 100, 525–544. doi: 10.1086/629606
Mitchell, J. S., Mackay, K. A., Neil, H. L., Mackay, E. J., Pallentin, A., and Notman, P. (2012). Undersea New Zealand, 1:5,000,000. NIWA Chart, Miscellaneous Series No. 92. Available online at: https://www.niwa.co. nz/our-science/oceans/bathymetry/further-informationLicence: https://www. niwa.co.nz/environmental-information/licences/niwa-open-data-licence-by-n n-nc-sa-version-1Alternative (accessed March 1, 2020).
Moore, M. I., and Murdoch, R. C. (1993). Physical and biological observations of coastal squirts under non-upwelling conditions. J. Geophys. Res. Oceans 98, 20043–20061.
Mountjoy, J. J., Howarth, J. D., Orpin, A. R., Barnes, P. M., Bowden, D. A., Rowden, A. A., et al. (2018). Earthquakes drive large-scale submarine canyon development and sediment supply to deep-ocean basins. Sci. Adv. 4:eaar3748. doi: 10.1126/sciadv.aar3748
Mountjoy, J. J., Micallef, A., Stevens, C. L., and Stirling, M. W. (2014). Holocene sedimentary activity in a non-terrestrially coupled submarine canyon: cook Strait Canyon system, New Zealand. Deep Sea Res. Part II Top. Stud. Oceanogr. 104, 120–133. doi: 10.1016/j.dsr2.2013.09.001
Mulder, T., and Syvitski, J. P. M. (1995). Turbidity currents generated at river mouths during exceptional discharges to the world oceans. J. Geol. 103, 285–299. doi: 10.1086/629747
Muller-Karger, F. E., Varela, R., Thunell, R., Luerssen, R., Hu, C., and Walsh, J. J. (2005). The importance of continental margins in the global carbon cycle. Geophys. Res. Lett. 32:L01602.
Murphy, R. J., Pinkerton, M. H., Richardson, K. M., Bradford-Grieve, J. M., and Boyd, P. W. (2001). Phytoplankton distributions around New Zealand derived from SeaWiFS remotely-sensed ocean colour data. N. Z. J. Mar. Freshwater Res. 35, 343–362. doi: 10.1080/00288330.2001.9517005
Nodder, S., Maas, E., Bowden, D., and Pilditch, C. A. (2011). ). Physical, biogeochemical, and microbial characteristics of sediment samples from the Chatham Rise and Challenger Plateau. New Zealand Aquatic Environment and Biodiversity, Report No 70. Wellington: Ministry of Fisheries, 34.
Nodder, S. D., Pilditch, C. A., Probert, P. K., and Hall, J. A. (2003). Variability in benthic biomass and activity beneath the subtropical front, Chatham Rise, SW Pacific Ocean. Deep Sea Res. Part I Oceanogr. Res. Pap. 50, 959–985. doi: 10.1016/s0967-0637(03)00094-3
Nokes, C. R., Bostock, H. C., Strachan, L. J., and Hadfield, M. G. (2019). Hydrodynamics and sediment transport on the North Canterbury Shelf, New Zealand. N. Z. J. Mar. Freshw. Res. 1–20 doi: 10.1080/00288330.2019.1699584
O’Brien, M. C., Macdonald, R. W., Melling, H., and Iseki, K. (2006). Particle fluxes and geochemistry on the Canadian Beaufort Shelf: implications for sediment transport and deposition. Cont. Shelf Res. 26, 41–81. doi: 10.1016/j.csr.2005.09.007
Pasqual, C., Goñi, M. A., Tesi, T., Sanchez-Vidal, A., Calafat, A., and Canals, M. (2013). Composition and provenance of terrigenous organic matter transported along submarine canyons in the Gulf of Lion (NW Mediterranean Sea). Prog. Oceanogr. 118, 81–94. doi: 10.1016/j.pocean.2013.07.013
Pasqual, C., Lee, C., Goñi, M., Tesi, T., Sanchez-Vidal, A., Calafat, A., et al. (2011). Use of organic biomarkers to trace the transport of marine and terrigenous organic matter through the southwestern canyons of the Gulf of Lion. Mar. Chem. 126, 1–12. doi: 10.1016/j.marchem.2011.03.001
Paul, D., Skrzypek, G. I., and Fórizs, R. (2007). Normalization of measured stable isotopic compositions to isotope reference scales - a review. Rapid Commun. Mass Spectrom. 21, 3006–3014. doi: 10.1002/rcm.3185
Paull, C. K., Caress, D. W., Lundsten, E., Gwiazda, R., Anderson, K., McGann, M., et al. (2013). Anatomy of the La Jolla submarine canyon system; offshore Southern California. Mar. Geol. 335, 16–34. doi: 10.1016/j.margeo.2012.10.003
Paull, C. K., Talling, P. J., Maier, K. L., Parsons, D., Xu, J., Caress, D. W., et al. (2018). Powerful turbidity currents driven by dense basal layers. Nat. Commun. 9, 4114–4123.
Peakall, J., and Sumner, E. J. (2015). Submarine channel flow processes and deposits: a process-product perspective. Geomorphology 244, 95–120. doi: 10.1016/j.geomorph.2015.03.005
Perié, C., and Ouimet, R. (2008). Organic carbon, organic matter and bulk density relationships in boreal forest soils. Can. J. Soil Sci. 88, 315–325. doi: 10.4141/cjss06008
Phillips, D L., and Gregg, J W. (2001). Uncertainty in source partitioning using stable isotopes. Oecologia 127, 171–179. doi: 10.1007/s004420000578
Phillips, D. L., Inger, R., Bearhop, S., Jackson, A. L., Moore, J. W., Parnell, A. C., et al. (2014). Best practices for use of stable isotope mixing models in food-web studies. Can. J. Zool. 92, 823–835. doi: 10.1139/cjz-2014-0127
Pickering, K., Stow, D., Watson, M., and Hiscott, R. (1986). Deep-water facies, processes and models: a review and classification scheme for modern and ancient sediments. Earth Sci. Rev. 23, 75–174. doi: 10.1016/0012-8252(86)90001-2
Probert, P. K. (1986). Energy transfer through the shelf benthos off the west coast of South Island, New Zealand. N. Z. J. Mar. Freshw. Res. 20, 407–417. doi: 10.1080/00288330.1986.9516160
Probert, P. K., and Swanson, K. M. (1985). Sediment texture of the continental shelf and upper slope off the west coast of South Island, New Zealand. N. Z. J. Mar. Freshw. Res. 19, 563–573. doi: 10.1080/00288330.1985.9516119
Prouty, N. G., Mienis, F., Campbell-Swarzenski, P., Roark, E. B., Davies, A. J., Robertson, C. M., et al. (2017). Seasonal variability in the source and composition of particulate matter in the depositional zone of Baltimore Canyon, U.S. Mid-Atlantic Bight. Deep Sea Res. Part I Oceanogr. Res. Pap. 127, 77–89. doi: 10.1016/j.dsr.2017.08.004
Puig, P., Palanques, A., and Martín, J. (2014). Contemporary sediment-transport processes in submarine canyons. Ann. Rev. Mar. Sci. 6, 53–77. doi: 10.1146/annurev-marine-010213-135037
Radford, J. (2012). Shelf-to-Canyon Sedimentation on the South Westland Continental Margin, Westland, New Zealand. Master’s thesis, University of Canterbury, Christchurch.
Ramirez-Llodra, E., Tyler, P. A., Baker, M. C., Bergstad, O. A., Clark, M. R., Escobar, E., et al. (2011). Man and the last great wilderness: human impact on the deep sea. PLoS One 6:e22588. doi: 10.1371/journal.pone.0022588
Reisinger, A., Mullan, A. B., Manning, M., Wratt, D. W., and Nottage, R. (2010). “Global and local climate change scenarios to support adaptation in New Zealand,” in Climate Change Adaptation in New Zealand: Future Scenarios and Some Sectoral Perspectives, eds R. A. C. Nottage, D. S. Wratt, J. F. Bornman, and K. Jones (Wellington: New Zealand Climate Change Centre), 26–43.
Romero-Romero, S., Molina-Ramírez, A., Höfer, J., Duineveld, G., Rumín-Caparrós, A., Sanchez-Vidal, A., et al. (2016). Seasonal pathways of organic matter within the Aviles submarine canyon: food web implications. Deep Sea Res. I 117, 1–10. doi: 10.1016/j.dsr.2016.09.003
Schlunz, B., and Schneider, R. R. (2000). Transport of terrestrial organic carbon to the oceans by rivers: re-estimating flux- and burial rates. Int. J. Earth Sci. 88, 599–606. doi: 10.1007/s005310050290
Schmiedl, G., Pfeilsticker, M., Hemleben, C., and Mackensen, A. (2004). Environmental and biological effects on the stable isotope composition of recent deep-sea benthic foraminifera, from the western Mediterranean Sea. Mar. Micropaleontol. 51, 129–152. doi: 10.1016/j.marmicro.2003.10.001
Scott, D. T., Baisden, W. T., Davies-Colley, R., Gomez, B., Hicks, D. M., Page, M. J., et al. (2006). Localized erosion affects national carbon budget. Geophys. Res. Lett. 33:L0140.
Selvaraj, K., Lee, T. Y., Yang, J. Y. T., Canuel, E. A., Huang, J. C., Dai, M., et al. (2015). Stable isotopic and biomarker evidence of terrigenous organic matter export to the deep sea during tropical storms. Mar. Geol. 364, 32–42.
Shaw, A. G. P., and Vennell, R. (2000). Variability of water masses through the Mernoo saddle, South Island, New Zealand. N. Z. J. Mar. Freshw. Res. 34, 103–116. doi: 10.1080/00288330.2000.9516918
She, J., and Klinck, J. M. (2000). Flow near submarine canyons driven by constant winds. J. Geophys. Res. Oceans 105, 28671–28694. doi: 10.1029/2000jc900126
Smith, S. V., and Hollibaugh, J. T. (1993). Coastal metabolism and the oceanic organic carbon balance. Rev. Geophys. 31, 75–89. doi: 10.1029/92rg02584
Stanton, B. R. (1976). Circulation and hydrology off the west coast of the South Island, New Zealand. N. Z. J. Mar. Freshw. Res. 10, 445–467. doi: 10.1080/00288330.1976.9515629
Stanton, B. R., and Moore, M. I. (1992). Hydrographic observations during the Tasman boundary experiment off the west coast of South Island, New Zealand. N. Z. J. Mar. Freshw. Res. 26, 339–358. doi: 10.1080/00288330.1992.9516528
Stetten, E., Baudin, F., Reyss, J. L., Martinez, P., Charlier, K., Schnyder, J., et al. (2015). Organic matter characterization and distribution in sediments of the terminal lobes of the Congo deep-sea fan: evidence for the direct influence of the Congo River. Mar. Geol. 369, 182–195. doi: 10.1016/j.margeo.2015.08.020
Stevens, C. L., O’Callaghan, J. M., Chiswell, S. M., and Hadfield, M. G. (2019). Physical oceanography of New Zealand/Aotearoa shelf seas – a review. N. Z. J. Mar. Freshw. Res. 1–40. doi: 10.1080/00288330.2019.1588746
Stock, B., Semmens, B., Ward, E., Parnell, A., Jackson, A., and Phillips, D. (2020). Package ‘MixSIAR’. Bayesian Mixing Models in R. Available online at: https://cran.r-project.org/web/packages/MixSIAR/MixSIAR.pdf (accessed March 1, 2020).
Stock, B. C., Jackson, A. L., Ward, E. J., Parnell, A. C., Phillips, D. L., and Semmens, B. X. (2018). Analyzing mixing systems using a new generation of Bayesian tracer mixing models. PeerJ 6:e5096. doi: 10.7717/peerj.5096
Stock, B. C., and Semmens, B. X. (2015). MixSIAR User Manual, version 3.0. Available online at: https://github.com/brianstock/MixSIAR/ (accessed March 1, 2020).
Stoffers, P., Plüger, W., and Walter, P. (1984). Geochemistry and mineralogy of continental margin sediments from Westland, New Zealand. N. Z. J. Geol. Geophys. 27, 351–365.
Sutherland, R. (1995). The Australia-Pacific boundary and Cenozoic plate motions in the SW Pacific: some constraints from Geosat data. Tectonics 14, 819–831. doi: 10.1029/95tc00930
Sutherland, R., Eberhart-Phillips, D., Harris, R. A., Stern, T., Beavan, J., Ellis, S., et al. (2007). “Do great earthquakes occur on the Alpine Fault in central South Island, New Zealand?” in A Continental Plate Boundary: Tectonics at South Island, New Zealand. Geophysical Monograph, eds D. Okaya, T. Stern, and F. Davey (Washington, DC: American Geophysical Union), 235–251. doi: 10.1029/175gm12
Sutton, P. (2001). Detailed structure of the subtropical front over Chatham Rise, east of New Zealand. J. Geophys. Res. 106, 31045–31056. doi: 10.1029/2000jc000562
Sutton, P. J. H. (2003). The Southland Current: a subantarctic current. N. Z. J. Mar. Freshw. Res. 37, 645–652. doi: 10.1080/00288330.2003.9517195
Symons, W. O., Sumner, E. J., Paull, C. K., Cartigny, M. J. B., Xu, J. P., Maier, K. L., et al. (2017). A new model for turbidity current behavior based on integration of flow monitoring and precision coring in a submarine canyon. Geology 45, 367–370. doi: 10.1130/g38764.1
Thomas, C. J., Blair, N. E., Alperin, M. J., DeMaster, D. J., Jahnke, R. A., Martens, C. S., et al. (2002). Organic carbon deposition on the North Carolina continental slope off Cape Hatteras (USA). Deep Sea Res. Part II Top. Stud. Oceanogr. 49, 4687–4709. doi: 10.1016/s0967-0645(02)00135-2
Tolosa, I., Fiorini, S., Gasser, B., Martin, J., and Miquel, J. C. (2013). Carbon sources in suspended particles and surface sediments from the Beaufort Sea revealed by molecular lipid biomarkers and compound-specific isotope analysis. Biogeosciences 10, 2061–2087. doi: 10.5194/bg-10-2061-2013
Verardo, D. J., Froelich, P. N., and McIntyre, A. (1990). Determination of organic carbon and nitrogen in marine sediments using the Carlo Erba NA-1500 Analyzer. Deep Sea Res. Part A Oceanogr. Res. Pap. 37, 157–165. doi: 10.1016/0198-0149(90)90034-s
Verburg, P. (2007). The need to correct for the Suess effect in the application of δ13C in sediment of autotrophic Lake Tanganyika, as a productivity proxy in the Anthropocene. J. Paleolimnol. 37, 591–602.
Vetter, E. W., and Dayton, P. K. (1999). Organic enrichment by macrophyte detritus, and abundance patterns of megafaunal populations in submarine canyons. Mar. Ecol. Prog. Ser. 186, 137–148. doi: 10.3354/meps186137
Vonk, J. E., Drenzek, N. J., Hughen, K. A., Stanley, R. H. R., McIntyre, C., Montluçon, D. B., et al. (2019). Temporal deconvolution of vascular plant-derived fatty acids exported from terrestrial watersheds. Geochim. Cosmochim. Acta 244, 502–521. doi: 10.1016/j.gca.2018.09.034
Wakeham, S. G., Hedges, J. I., Lee, C., Peterson, M. L., and Hernes, P. J. (1997). Compositions and transport of lipid biomarkers through water column and surficial sediments of the equatorial Pacific Ocean. Deep Sea Res. Part II Top. Stud. Oceanogr. 44, 2131–2162. doi: 10.1016/s0967-0645(97)00035-0
Wildhaber, Y. S., Liechti, R., and Alewell, C. (2012). Organic matter dynamics and stable isotope signatures of tracers of the sources of suspended sediment. Biogeosciences 9, 1985–1996. doi: 10.5194/bg-9-1985-2012
Xiao, X., Fahl, K., and Stein, R. (2013). Biomarker distributions in surface sediments from the Kara and Laptev seas (Arctic Ocean): indicators for organic-carbon sources and sea-ice coverage. Quat. Sci. Rev. 79, 40–52. doi: 10.1016/j.quascirev.2012.11.028
Yunker, M. B., Belick, L. L., Harvey, H. R., and Macdonald, R. W. (2015). Tracing the inputs and fate of marine and terrigenous organic matter in Arctic Ocean sediments: a multivariate analysis of lipid biomarkers. Deep Sea Res. Part II Top. Stud. Oceanogr. 52, 3478–3508. doi: 10.1016/j.dsr2.2005.09.008
Zeldis, J., Hicks, M., Trustrum, N., Orpin, A., Nodder, S., Probert, K., et al. (2010). “New Zealand continental margin fluxes,” in Carbon and Nutrient Fluxes in Continental Margins: A Global Synthesis. Global Change – the IGBP Series, eds K.-K. Liu, L. Atkinson, R. Quinoñes, and L. Talaue-Mcmanus (New York, NY: Springer-Verlag), 273–287.
Keywords: land-to-sea connectivity, compound specific stable isotopes, fatty acid biomarkers, sediment transport, Kaikōura Canyon, Hokitika Canyon, small mountainous rivers
Citation: Gibbs M, Leduc D, Nodder SD, Kingston A, Swales A, Rowden AA, Mountjoy J, Olsen G, Ovenden R, Brown J, Bury S and Graham B (2020) Novel Application of a Compound-Specific Stable Isotope (CSSI) Tracking Technique Demonstrates Connectivity Between Terrestrial and Deep-Sea Ecosystems via Submarine Canyons. Front. Mar. Sci. 7:608. doi: 10.3389/fmars.2020.00608
Received: 19 March 2020; Accepted: 01 July 2020;
Published: 31 July 2020.
Edited by:
Erik Cordes, Temple University, United StatesReviewed by:
Amanda Demopoulos, United States Geological Survey (USGS), United StatesCopyright © 2020 Gibbs, Leduc, Nodder, Kingston, Swales, Rowden, Mountjoy, Olsen, Ovenden, Brown, Bury and Graham. This is an open-access article distributed under the terms of the Creative Commons Attribution License (CC BY). The use, distribution or reproduction in other forums is permitted, provided the original author(s) and the copyright owner(s) are credited and that the original publication in this journal is cited, in accordance with accepted academic practice. No use, distribution or reproduction is permitted which does not comply with these terms.
*Correspondence: Daniel Leduc, RGFuaWVsLkxlZHVjQG5pd2EuY28ubno=
†Present address: Andrew Kingston, Geological Survey of Canada, Natural Resources Canada, Calgary, AB, Canada
Disclaimer: All claims expressed in this article are solely those of the authors and do not necessarily represent those of their affiliated organizations, or those of the publisher, the editors and the reviewers. Any product that may be evaluated in this article or claim that may be made by its manufacturer is not guaranteed or endorsed by the publisher.
Research integrity at Frontiers
Learn more about the work of our research integrity team to safeguard the quality of each article we publish.