- 1Institute of Ocean Sciences, Fisheries and Oceans Canada, Sidney, BC, Canada
- 2Arctic Research Centre, Aarhus University, Aarhus, Denmark
- 3Department of Bioscience, Aarhus University, Silkeborg, Denmark
The Arctic marine system is large and heterogeneous, harsh and remote, and now changing very rapidly, all of which contribute to our current inadequate understanding of its basic structures and functions. In particular, many key processes within and external to the Arctic Ocean are intrinsically linked to its freshwater system, which itself is undergoing rapid and uncertain change. The role of the freshwater system (delivery, disposition, storage, and export) in the Arctic Ocean has recently received significant attention; however, due to the fact that few studies are able to cover all regions and seasons equally, we still lack an accessible, unified pan-Arctic representation generalizing the impacts of freshwater on the upper Arctic Ocean where many biological and geochemical interactions occur. This work seeks to distill our current understanding of the Arctic freshwater system, and its impacts, into conceptual diagrams which we use as a basis to speculate on the impact of future changes. We conclude that an understanding of regional and seasonal variability is required in order to gain a pan-Arctic perspective on the physical-geochemical-biological state of the upper Arctic Ocean. As an example of regionality, enhanced stratification due to freshening will be more important in the Pacific influenced Amerasian Basin, which stores the bulk of the freshwater burden, while the Atlantic influenced Eurasian Basin will experience more consequences related to increased heating from advective sources. River influenced coastal regions will experience a mosaic of these and other biogeochemical effects, whereas glacial fjords may follow their own unique trajectories due to the loss of upwelling mechanisms at glacial fronts. As an example of seasonality, the continued modulation of the sea ice freeze-melt cycle has increased the seasonal freshwater burden in the deep basins dramatically as the system progresses toward ice-free summer conditions, but will eventually reverse, reducing the seasonal flux of freshwater by more than half in a future, perennially ice-free ocean. It is our goal that these conceptualizations, based on the current state-of-the-art, will drive hypothesis-based research to investigate the physical-biogeochemical response to a changing freshwater cycle in a future Arctic Ocean with greatly reduced ice cover.
Introduction: The Arctic Ocean Has Two Freshwater Lids, and Both Are Changing
The Arctic Ocean (AO, Figure 1) is a “beta” ocean, in that its salt-stratified halocline constrains and shapes its fundamental processes and functions related to stratification, circulation, and mixing (cf. Carmack, 2007). It is this freshwater (FW) feature that, in fact, allows an ice cover to form and persist by limiting the depth of seasonal heat exchange and mixing, and constrains the upward flux of nutrients to the euphotic zone. Together, the ice cover and halocline provide a shield (the solid and the liquid “lids”) to limit the vertical exchange of heat and wind energy with the upper AO. Under recent climate warming both lids are changing rapidly, but not in uniform, predictable, or well-understood ways. For example, an intensified hydrological cycle is anticipated to follow in a warming climate, and with sea ice decline, a greater fraction of the internal FW burden now cycles seasonally between the solid (ice) and liquid (halocline) lids, the latter of which has both a seasonal and perennial structure, as emphasized here. Further, it is now established that responses to climate change are decidedly regional, and that effects will be felt disproportionally among seasons (Polyakov et al., 2017, 2018, 2020). We focus here on these two “lids” as a central location for FW exchanges and cycling on seasonal and regional time and space scales, with particular emphasis on the upper ocean. Since we focus our discussion on the coupling between physics, geochemistry, and biology, we define the “upper” ocean as the seasonal surface mixed layer, where many of the FW-relevant interactions among these systems take place. Our aim is not to provide a review of the Arctic FW system, as this has been done recently (Carmack et al., 2015c; Haine et al., 2015; AMAP, 2017), but rather, our goal is to distill these syntheses and current state-of-the-art knowledge into a conceptualized view of the regionality and seasonality of FW in the Arctic Ocean. This is in order to make generalizations about how FW is affecting physical-geochemical-biological interactions from a pan-Arctic perspective, where possible. It also provides a starting point for hypotheses-driven research to address knowledge gaps and test future scenarios of an intensified hydrological cycle impacting an Arctic ocean with greatly reduced sea ice. Specifically we address: (1) the present role of the two FW lids and how they are already changing (section “Present State of the Regionality and Seasonality of Freshwater in the Arctic Ocean”); (2) the consequences of these changes to the physics of the upper ocean (section “Changing State of Freshwater in the Arctic Ocean”); and (3) how the changing upper ocean physics (warmer, altered stratification patterns) influences AO wide geochemical and biological functions and responses (section “Changing Upper Ocean Physics Influences Geochemistry and Biology Across the Arctic Ocean”). We dedicate the remainder of the paper to describing the regionality and seasonality of the effects of changing FW regimes on geochemical and biological systems of the upper AO (section “Regionality and Seasonality of Freshwater Sources Set the Geochemistry and Biology of the Seasonal Mixed Layer”), and project, via conceptualization, recent observations of freshening impacts on these systems into the future (section “Impacts of Continued Freshening of the Arctic Ocean on Physics, Geochemistry, and Biota”). We end by speculating as to the response of the upper AO systems to continued change to FW cycles that may ultimately lead to an ice-free AO (section “Potential Future States of the Upper Arctic Ocean Under a Changing Freshwater System”).
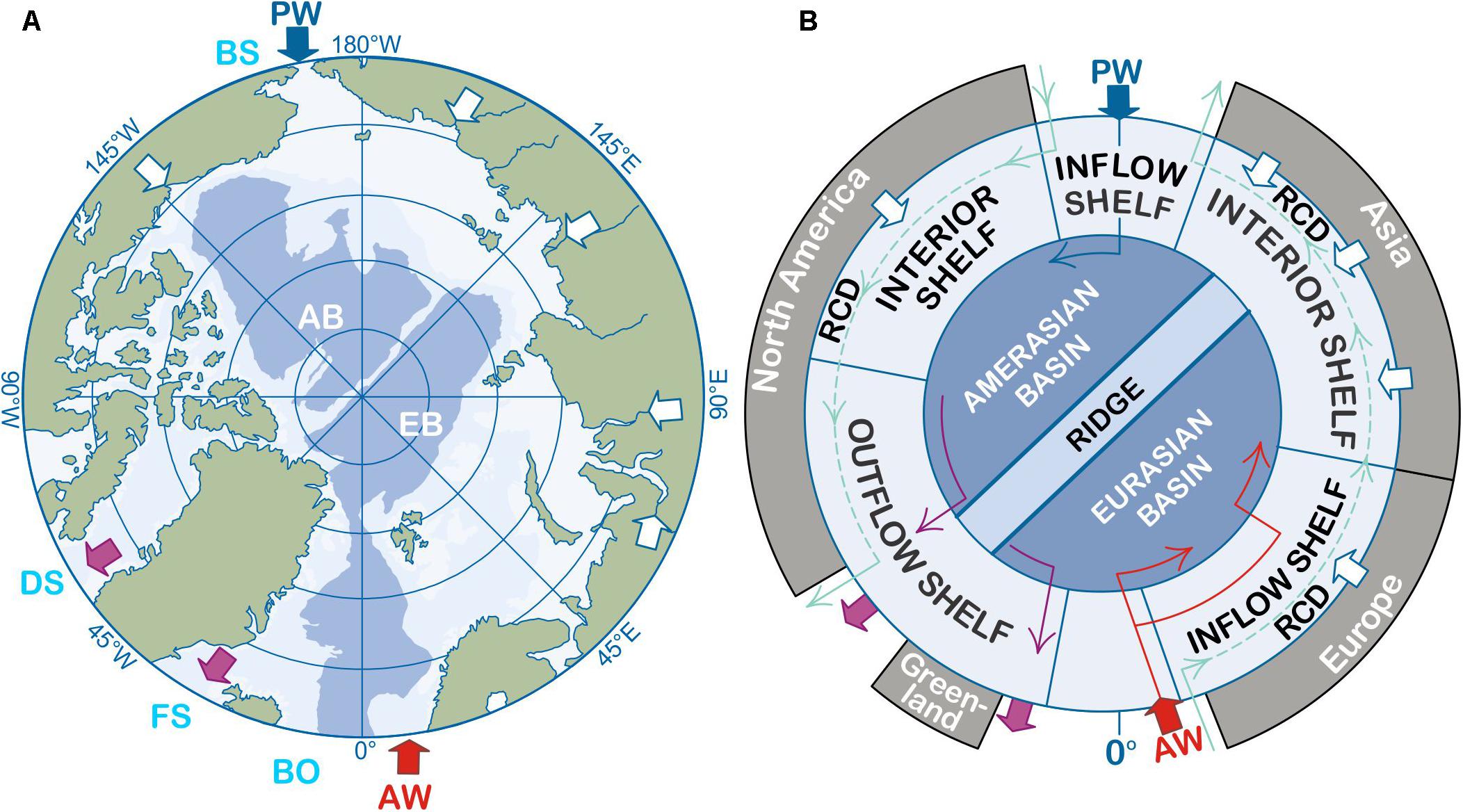
Figure 1. Map of the arctic marine system. (A) Polar projection of the Arctic Ocean showing a simplified version of bathymetry, highlighting the deep Amerasian and Eurasian Basins (AB, EB; dark shading) and shallow shelves (light shading), along with idealized Pacific Water (PW) inputs via Bering Strait (BS), Atlantic Water (AW) inflows via the Barents Opening (BO), and major river inputs with blue, red, and white arrows, respectively. Outflows from the AO via the Davis Strait (DS) and Fram Strait (FS) gateways are illustrated with purple arrows; and (B) the highly idealized hydromorphological domains redrawn from Bluhm et al. (2015), where the RCD refers to the Riverine Coastal Domain (see Carmack et al., 2015b, c), major inflow and outflow arrows as in (A).
Present State of the Regionality and Seasonality of Freshwater in the Arctic Ocean
The AO is a mediterranean sea that is forced by, and interacts with, the polar atmosphere, the vast surrounding drainage basins, and the subarctic Atlantic and Pacific oceans to the south (Prowse et al., 2015a, b). A fundamental difference, however, exists between the two marine source waters entering the AO: flows from the Atlantic side entering through the Nordic Seas are alpha-ocean derived, and remain temperature stratified within the West Spitsbergen current as far as 80oN, while those entering through the Bering Strait are beta-ocean derived and salt stratified, drawing upper ocean waters from the North Pacific well north of the Subarctic Front. Upon entering the AO, the Atlantic water (AW) subducts below the surface and forms the base of the AO halocline, while the Pacific water (PW), itself drawn from waters above the North Pacific halocline, subducts to add additional low salinity waters to what is termed “the halocline complex,” which spans the upper ∼225 m or more of the water column, depending on location. Together this sets the background for the so-called “double estuary” circulation of the AO, with incoming subarctic waters becoming both lighter and denser during their passage through the AO (Stigebrandt, 1985; Carmack and Wassmann, 2006), and thus, becomes the first determinant of AO regionality. Variations throughout the year in FW inputs and disposition, both external (rivers, net precipitation, and Pacific inflow) and internal (the sea ice freeze-melt cycle) set conditions for physical-chemical-biological coupling and thus underscore the requirement to also consider the seasonality of the FW cycle.
The AO FW system is traditionally examined through budget considerations, with efforts to reconcile and balance FW inputs, storage, and export of various sources via net precipitation, river discharge, Pacific inflow, Arctic outflow, and sea ice export (Aagaard and Carmack, 1989; Serreze et al., 2006; Tsubouchi et al., 2012; Carmack et al., 2015c; Haine et al., 2015). Limitations should be acknowledged. For example, budgets are most often calculated as an anomaly based on a reference salinity, typically relative to a salinity of 34.8, but regional constraints in the use of a reference salinity exist (cf. Carmack et al., 2008; Bacon et al., 2015; Schauer and Losch, 2019). Still, even with the crude resolution of budget integrations, the importance of regionality of FW storage is strikingly clear (see Figure 4 of Aagaard and Carmack, 1989). Furthermore, Proshutinsky et al. (2009) documented interannual variability in FW content in the Canada Basin in relation to atmospheric forcing, and Bacon et al. (2015) used a model-based approach to examine the seasonal variability of FW fluxes and storage within the AO. They show a summer peak in storage associated with snow melt derived river inflow, and a tendency to store FW in summer and release it in winter, hypothesized to be due to changes in wind-stress curl and to variations in Ekman pumping related to seasonal changes in air-sea-ice coupling.
Estimation of exchanges with the subarctic seas are most frequently based on measurements of transport through four major “gateways” (Fram Strait, Barents Sea, Bering Strait, and Davis Strait; Figure 1A), but the importance of expanding the FW system beyond the classical gateways to the subtropics and even the tropics has recently been stressed (Carmack et al., 2015c; Prowse et al., 2015a, b). Likewise, a full understanding of the atmosphere’s role in the AO FW system requires inclusion of the transport of moisture from the Atlantic to the Pacific by the northeast trade winds, and the subsequent transport of moisture by westerly winds into the Arctic drainage basins (Carmack and McLaughlin, 2011). Shifts in storm track patterns and jet stream structure will likewise alter the delivery of FW to the AO (Prowse et al., 2015a, b). Within the AO, winds and vorticity conservation control surface ocean circulation and therefore FW transport rates and pathways (as summarized by Haine et al., 2015). Although a primary FW input into the Arctic, no significant long-term change has been observed in atmospheric moisture transport over the last three decades (1979–2016), however, warming over the Arctic has resulted in the increase in precipitation in all seasons over this period, and a shift in the seasons has been observed, with the Arctic getting drier over boreal winter, spring, and fall; but wetter over boreal summer (Oshima and Yamazaki, 2017).
Changing State of Freshwater in the Arctic Ocean
The AO receives FW from three different external sources: direct precipitation (balanced by evaporation, P-E), runoff from land (RO), primarily via rivers, and through the inflow of Pacific Ocean water via Bering Strait (PW), which account for roughly 25, 47, and 28% of the contemporary liquid FW delivery to the Arctic Ocean, respectively (cf. Serreze et al., 2006; Holland et al., 2007; Haine et al., 2015). Pan-Arctic observations have indicated that FW inputs from all three sources have increased over the last several decades (e.g., Peterson et al., 2002; Overeem and Syvitski, 2010; Vihma et al., 2016; Woodgate, 2018). While within the AO, the liquid FW content has been increasing since the mid-1990s; concomitant with a persistent decline in sea ice extent, thickness, and volume (e.g., Kwok et al., 2009; Stroeve et al., 2012b; Krishfield et al., 2014; Rabe et al., 2014; Proshutinsky et al., 2015). These additional FW inputs, combined with a decline in storage within solid sea ice, and relatively constant FW export, have resulted in an 8 % increase in the liquid FW content of the AO over the first decade of the 2000s, compared to the 20 years previous; a trajectory which is projected to continue for the foreseeable future (Haine et al., 2015).
Internally, sea ice and the halocline both act as storage reservoirs for FW (Figure 2), which, coupled with a redistribution of solid FW from multi-year sea ice into seasonal sea ice, has consequences for FW distributions under changing circulation pathways. For example, more FW is stored in the central basins than on the shelves, and more in the Amerasian Basin (AB) than Eurasian Basin (EB; Carmack, 2000). Summer sea ice melt increases the liquid FW content of the surface ocean across the pan-Arctic by moving the FW burden from the sea ice reservoir into the halocline reservoir. This seasonal redistribution between the ocean’s solid and liquid “lids,” combined with changing circulation pathways that redirect FW from the EB (saltier) to the AB (fresher), act to regionally redistribute FW across the AO without increasing the total FW content within the AO (e.g., Wang et al., 2019). As summer sea ice extent continues to diminish, the FW burden from ice melt, recycled on an annual basis, has more than doubled since the 1970s and 1980s and now exceeds that of the other (external) sources (Table 1). In the 1980s, freeze-melt (F/M) was estimated to cycle ∼ 4 × 103 km3 yr–1 of FW into and out of the seasonal mixed layer of the deep polar basins (cf. Aagaard et al., 1981), on par with other FW inputs (Table 1). Currently, F/M cycles about ∼9 × 103 km3 yr–1 over the same area, and by ∼2050, assuming an ice-free summer (<10% ice cover) and full winter freeze up of the basin, the volume of FW cycled through the sea ice F/M system will reach a maximum (∼13 × 103 km3 yr–1), more than triple that of the next largest input to the AO (Table 1). The critical distinction here is that FW from sea ice melt creates a strong shallow halocline, essentially separating the euphotic zone from the permanent halocline, while brine released by freezing drives penetrative convection and mixing to the depth of the permanent halocline (Figure 3; e.g., Rosenblum et al., submitted). This is especially important in the central AB, as the nutricline is associated with the deeper, permanent halocline (see section “Regionality and Seasonality of Freshwater Dictates the Accessibility of Nutrients and Organic Carbon”), it is effectively separated from the euphotic zone, thereby strongly limiting primary productivity associated with the spring bloom without convective mixing. In a future sea-ice free state, the AO may no longer shunt FW through the sea ice freeze-melt cycle, with potential consequences of removing this FW component altogether (see discussion in section “Potential Future States of the Upper Arctic Ocean Under a Changing Freshwater System”), and reducing the seasonal surface ocean FW flux by 60%, effectively making the future AO functionally similar to its neighboring subarctic oceans, particularly the North Pacific. This change in the annual freeze-melt cycle will likely overwhelm all other FW source changes with respect to processes controlling nutrient availability and physical-geochemical-biological coupling.
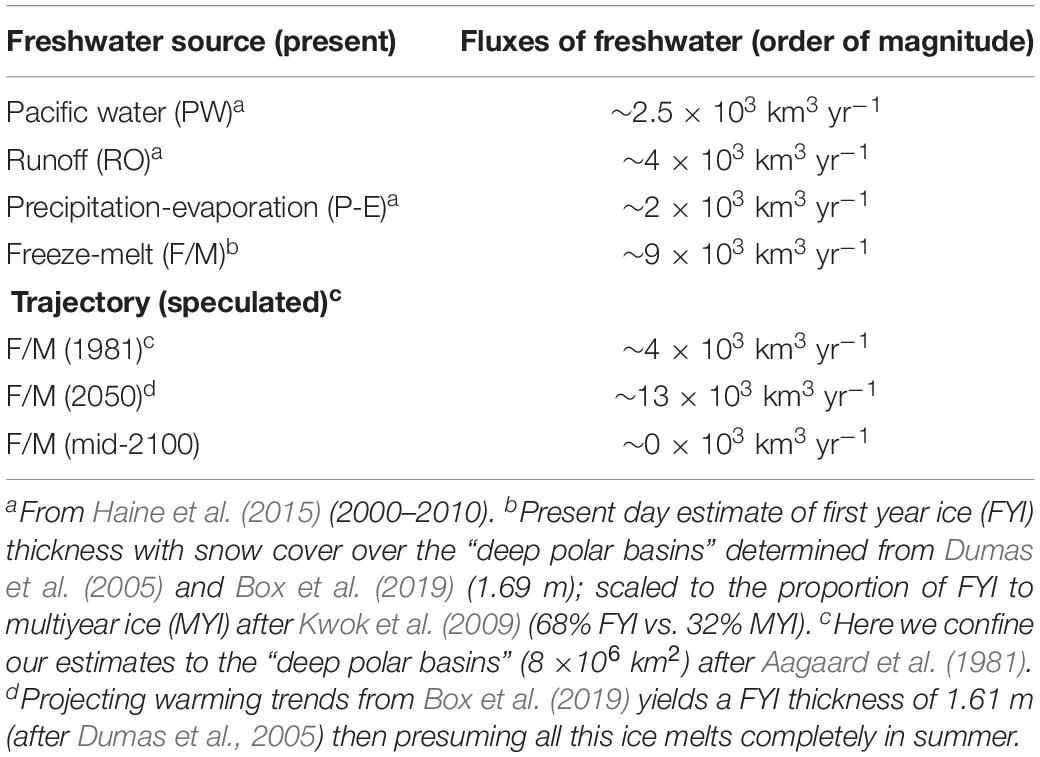
Table 1. Freshwater fluxes to the Arctic Ocean, shown in Figure 2.
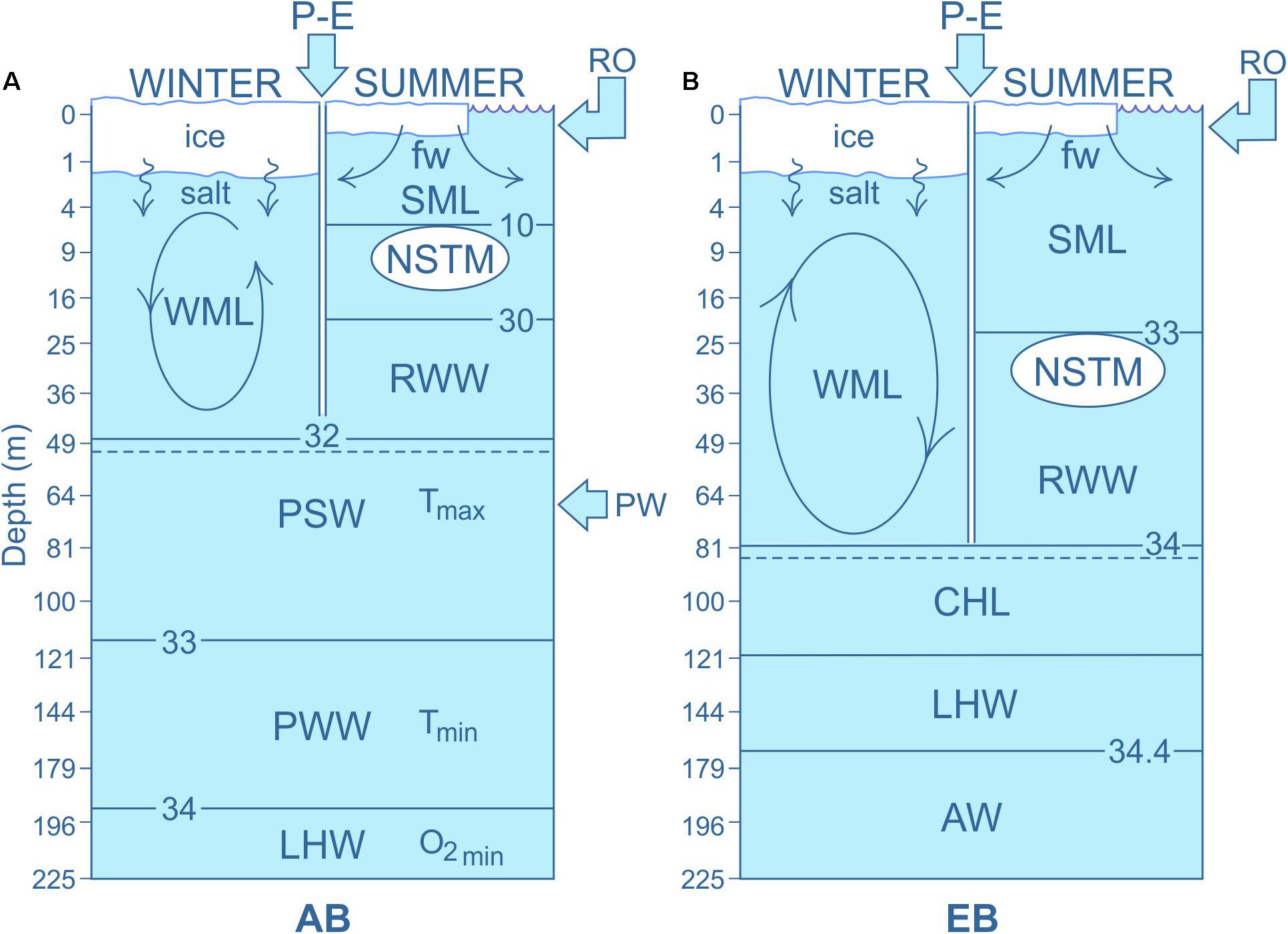
Figure 2. The Arctic Ocean’s two “lids”: sea ice and the halocline. Sea ice growth in winter adds salt to the surface ocean, driving penetrative convection to form the winter mixed layer (WML). In summer, freshwater (FW) is added to the mixed layer through seasonal contributions of sea ice melt, terrestrial runoff (RO) and the balance of precipitation-evaporation (P-E), which strongly stratify the surface ocean. This stratification limits the depth of wind mixing, allowing formation of a shallow summer mixed layer (SML). Below the SML, fractions of the previous season’s processes may remain; specifically, a cool remnant winter water (RWW) immediately above the base of the WML from the previous year. In the presence of sea ice with sufficient open water, a near-surface temperature maximum (NSTM) may form immediately below the SML by solar heating, capped by the cold, low salinity surface layer. These exchanges between the surface ocean layer and the sea ice “lid” occur across the Arctic Ocean, however, the additional FW associated with Pacific water (PW) enhances surface stratification in the Amerasian Basin (AB) and contributes to the difference between the halocline “lid” within the deep AB and that of the Eurasian Basin (EB); represented conceptually in (A,B), respectively. In both the Pacific and Atlantic sectors of the Arctic Ocean, warm Atlantic-derived waters (AW) form the base of the halocline. The water column above is comprised of multiple layers that define the seasonal and permanent haloclines, constrained by the horizontal interleaving of seasonally modified FW inputs. (A) In the Pacific sector halocline, PW inputs from the Bering Sea interflow, increasing stratification, and further constraining the depth of convection. Here, the winter convection penetrates down to the base of the Pacific Summer Water (PSW) and the sub-surface temperature maximum (Tmax), which in turn lies above the Pacific Winter Water (PWW) and the sub-surface temperature minimum (Tmin). Below PW lies lower halocline water (LHW), which likely forms on Siberian shelves. (B) In the Atlantic sector, the thickness and distribution of halocline layers is highly variable across the EB, but includes a cold halocline layer (CHL) and LHW layer above the warm AW layer. Both form as a consequence of sea ice formation and winter convective mixing events; CHL mostly within the basin and LHW on mostly on shelves with subsequent drainage into the basins (after Aagaard et al., 1981; Rudels et al., 1996; Steele and Boyd, 1998; Polyakov et al., 2013; Carmack et al., 2015a; Peralta-Ferriz and Woodgate, 2015).
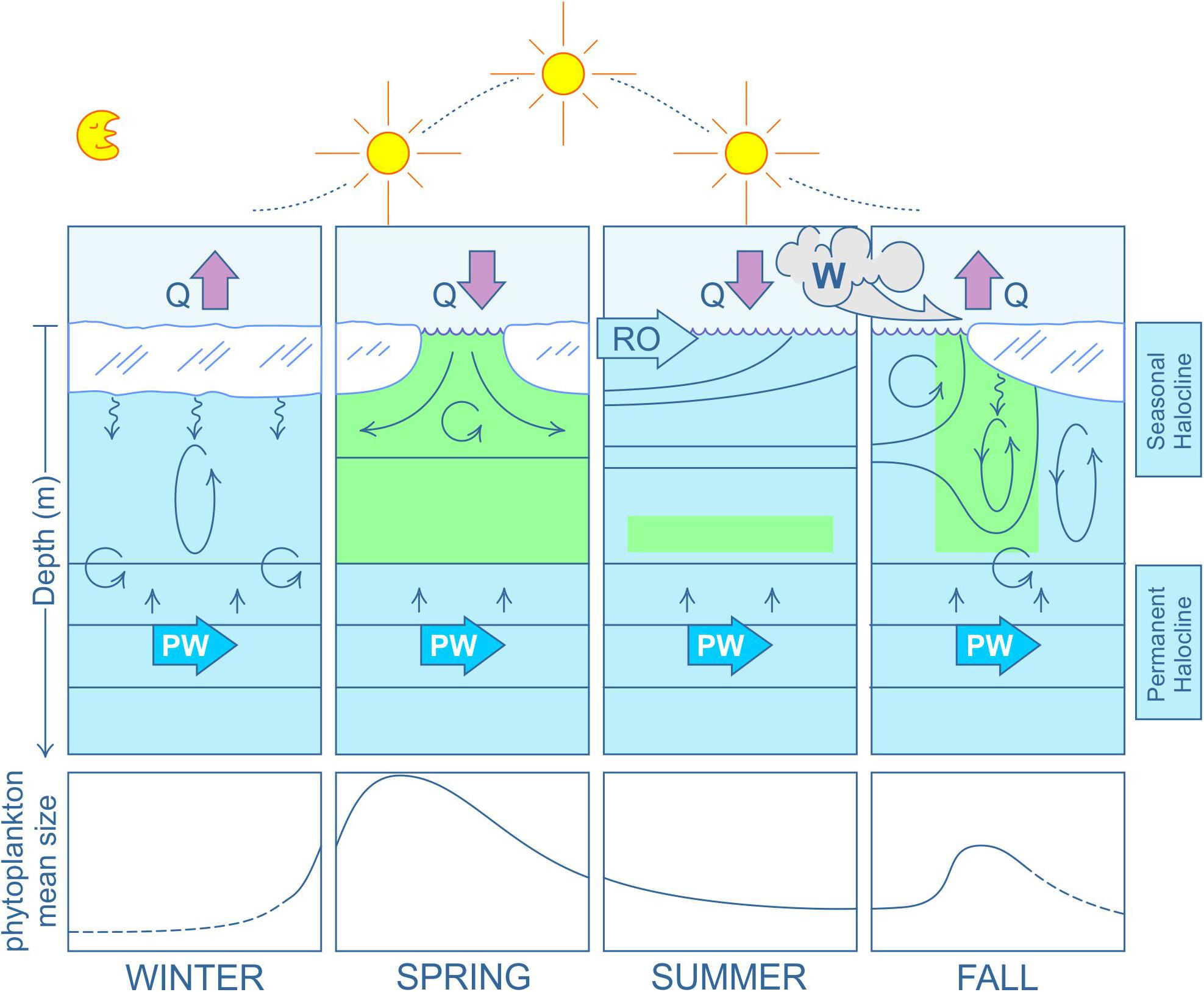
Figure 3. Seasonal evolution of the Arctic Ocean “lids.” Cartoon depicting an idealized, 1-D seasonal progression of upper ocean stratification through the freeze-melt sea ice cycle, illustrating the formation of the seasonal halocline and its separation from the permanent halocline complex below. The structures shown are specific to the Amerasian Basin, but analogous reasoning holds for the Eurasian Basin. Here, vertical block arrows denote net heat flux (Q) out of (winter and fall) and into (spring and summer) the upper ocean; horizontal arrows labeled RO denote spreading of the runoff plume waters and horizontal arrows labeled PW denote interflowing Pacific Water; blue isolines illustrate the seasonal cycle of salinity stratification (see text); straight, upwards-directed arrows denote compensation upwelling from the PW (see text); wiggly, downward-directed arrows denote dense, brine rejection during sea ice formation (winter and fall); ellipses with arrows denote brine-driven convection; circles with arrows denote penetrative convection and entrainment across the upper boundary of the permanent halocline due to convection and shear; W is wind, shown here as intensified during fall transition. Green highlighted areas illustrate the relative location of phytoplankton biomass in the water column, while the lower panels illustrate the mean size distribution of phytoplankton within each season, based on an extrapolation of the nanoplankton/picoplankton ratio discussed by Li et al. (2009, 2013).
Many of the exchanges and modifications of FW in the AO occur within the seasonal mixed layer (ML) of the upper ocean (Figure 2). For the purposes of this paper, we consider the ML to have two seasonal configurations, summer and winter. Here the “summer mixed layer” (SML) is a near-homogeneous layer within which low salinity water from ice melt, runoff, and net precipitation is mixed downward in summer, primarily by the winds. The “seasonal halocline” is the layer of strong salinity gradient that lies immediately below the ML. Likewise, the “winter mixed layer” (WML) is the near-homogeneous layer of water that is mixed downward in winter, primarily by brine convection and winds (Figure 2). The permanent halocline is the suite of layers of strong salinity gradient immediately below the WML, that is formed and maintained primarily by advective mechanisms. The depth of the ML is directly influenced by its liquid FW content and has consequences for the amount of heat from air-sea exchange that is trapped in the surface ocean. As stratification increases with FW input, more heat can be trapped in progressively shallower layers. This in turn strengthens the ice-albedo feedback, such that when ice is removed, incoming heat is stored as sensible heat in the water column, rather than used as latent heat to melt ice (cf. Carmack et al., 2015a). Together, these two mechanisms mean that surface waters will warm faster in summer. For example, increased stratification associated with the accumulation of FW (meteoric water and sea ice melt) in the Beaufort Gyre (Toole et al., 2010) has resulted in the storage of heat from solar radiation in the near surface temperature maximum (NSTM) that now persists through the winter (Figure 2A; Jackson et al., 2010). Similar evidence of sub-surface warming is now also observed as NSTM formation in the EB (Figure 2B; Polyakov et al., 2013); however, the presence of this layer will likely be short-lived as weakening stratification in the EB may act to increase heat and nutrient fluxes from below (e.g., Polyakov et al., 2017). This strengthening of the stability of the upper water column may give a double whammy of (1) increasing total stratification (salinity and temperature), and (2) warming of the surface ocean, both of which have consequences for geochemical processes and biological systems.
Changing Upper Ocean Physics Influences Geochemistry and Biology Across the Arctic Ocean
Observation- and model-based discussions of biological change in the Arctic Ocean have generally followed two lines of inquiry. The first involves the joint but counter opposing roles of light and nutrient availability under conditions of sea ice decline and altered salt-stratification (e.g., Ardyna et al., 2011; Table 2). Implicit in these works is often the presumption of homogeneity in the lateral, vertical and temporal distributions of photosynthesizing species, and more-or-less uniform conditions of nutrient availability. The second involves the northward invasion of subarctic species or borealization (e.g., Polyakov et al., 2020). Implicit in these works is the presumption that new species entering local food webs take on the ecological function of the species they replace, with minimal cascading effects, and that regionality plays no constraining role. Our discussions below address these issues.
The seasonal dynamics of water column stability and the development and growth of primary producers in the upper AO over the freeze-melt cycle are described in a conceptual model in Figure 3. This model is based on water mass conditions found in the AB, where nutrient-rich PW interflows to form the uppermost permanent halocline and nutricline (but note that similar reasoning for the separation of the seasonal halocline/euphotic zone and permanent halocline/nutricline holds for conditions in the EB). In winter (Figure 3, left-hand panel) surface heat loss and sea ice formation releases plumes of dense salty water into the underlying water column, resulting in a negative buoyance flux. These plumes then sink and entrain ambient surface layer waters, thus mixing downwards to the depth of the permanent halocline and nutricline. The combined effect of such penetrative convection and shear-induced mixing (cf. Farmer, 1975) allows mixing across the interface and some reset of nutrients into the upper AO. In spring (Figure 3, second panel), sea ice melt re-stratifies the upper ocean, and this, combined with light availability, results in a brief spring bloom, quickly drawing down nutrients made available during winter reset. In this case, however, the positive buoyancy flux greatly impedes the depth of effective mechanical mixing by the wind, thus separating the euphotic zone from the deeper lying permanent halocline and nutricline. In summer (Figure 3, third panel), low nutrient levels in the uppermost ocean suppress new production, resulting in the formation of a subsurface chlorophyll maximum (SCM) immediately above the nutricline maintained by interflowing PW (cf. McLaughlin and Carmack, 2010) where turbulent diffusion (cf. Randelhoff et al., 2020) between the two layers brings some nutrients just above the pycnocline. Finally, in fall (Figure 3, right-hand panel), the onset of freezing again rejects dense brine into the water column, first eroding the summer halocline, and then reaching deeper until limited by the permanent halocline. As such, enhanced productivity, e.g., a fall bloom, will not occur until the summer halocline is fully eroded (Nishino et al., 2020). A key point to note is that the volume of liquid FW involved in the seasonal stratification cycle is currently increasing (with increased summer melting of sea-ice, but current refreezing in winter). But, that cycle may soon reach a maximum (under ice-free summer conditions), and then vanish altogether should the AO enter a perennial ice-free state (see discussion in section “Potential Future States of the Upper Arctic Ocean Under a Changing Freshwater System”).
Of course, increasing the total stratification of the halocline and warming the surface ocean will have consequences for geochemical processes and biological systems. The initial effects of increased stratification on primary producers has already been reported. Numerous studies indicate both a decrease in primary production and chlorophyll a biomass (Table 2; Coupel et al., 2012, 2015; Yun et al., 2016) and a shift to smaller phytoplankton (Table 2; Li et al., 2009; Tremblay et al., 2009; Ardyna et al., 2011; Fujiwara et al., 2014) in fresher, more stratified systems (mainly the Beaufort Sea, Canada Basin, and Canadian Arctic Archipelago). Warming has additional consequences. For example, warmer waters increase the metabolism of plankton communities, favoring picoplankton, or species with lower carbon biomass, over larger phytoplankton (Table 2; Tremblay et al., 2009; Coello-Camba et al., 2014a; Fujiwara et al., 2014; Neeley et al., 2018). Furthermore, due to lower activation energies, heterotrophic processes are likely to prevail with a warming surface ocean (Table 2; Vaquer-Sunyer et al., 2010; Holding et al., 2013). Increased temperatures, especially if the heat uptake is confined to shallower layers, may push surface water temperatures beyond the thermal limits of existing vertebrate taxa (Drost et al., 2016), and further stress Arctic residents by the northward migration of boreal species (see section “Potential Future States of the Upper Arctic Ocean Under a Changing Freshwater System”). Numerous examples of boreal species invasions have already been documented (e.g., Friis Møller and Nielsen, 2019; Polyakov et al., 2020) whereby traditionally more sub-Arctic species are finding comfortable living conditions in warming waters. Blooms of the North Atlantic calcifying algae Emiliania huxleyi have been observed to follow the ever-encroaching polar front (Neukermans et al., 2018) possibly advected there due to increased current velocities (Oziel et al., 2020).
Regionality and Seasonality of Freshwater Sources Set the Geochemistry and Biology of the Seasonal Mixed Layer
As reviewed above, FW inputs to the AO from all sources have increased over the past few decades. These FW contributions are not distributed evenly, however, resulting in both regionality and seasonality of FW inputs across the AO. Importantly, this means that future changes to FW inputs will impact different regions differently, as summarized in Figure 4A and Table 3. Referenced to a salinity of 34.8, PW contributions through Bering Strait dominate the FW composition of inputs across the Pacific inflow shelf, but upon entering the deep basins, PW contributions remain mostly restricted to the AB and the North American interior and outflow shelves, making up only a small component of what exits the AO via Fram Strait. The absence of PW in the Atlantic sector (Atlantic inflow shelf, Eurasian interior shelves, and EB) results in regionally greater relative roles for direct inputs of P-E and RO components on upper ocean properties.
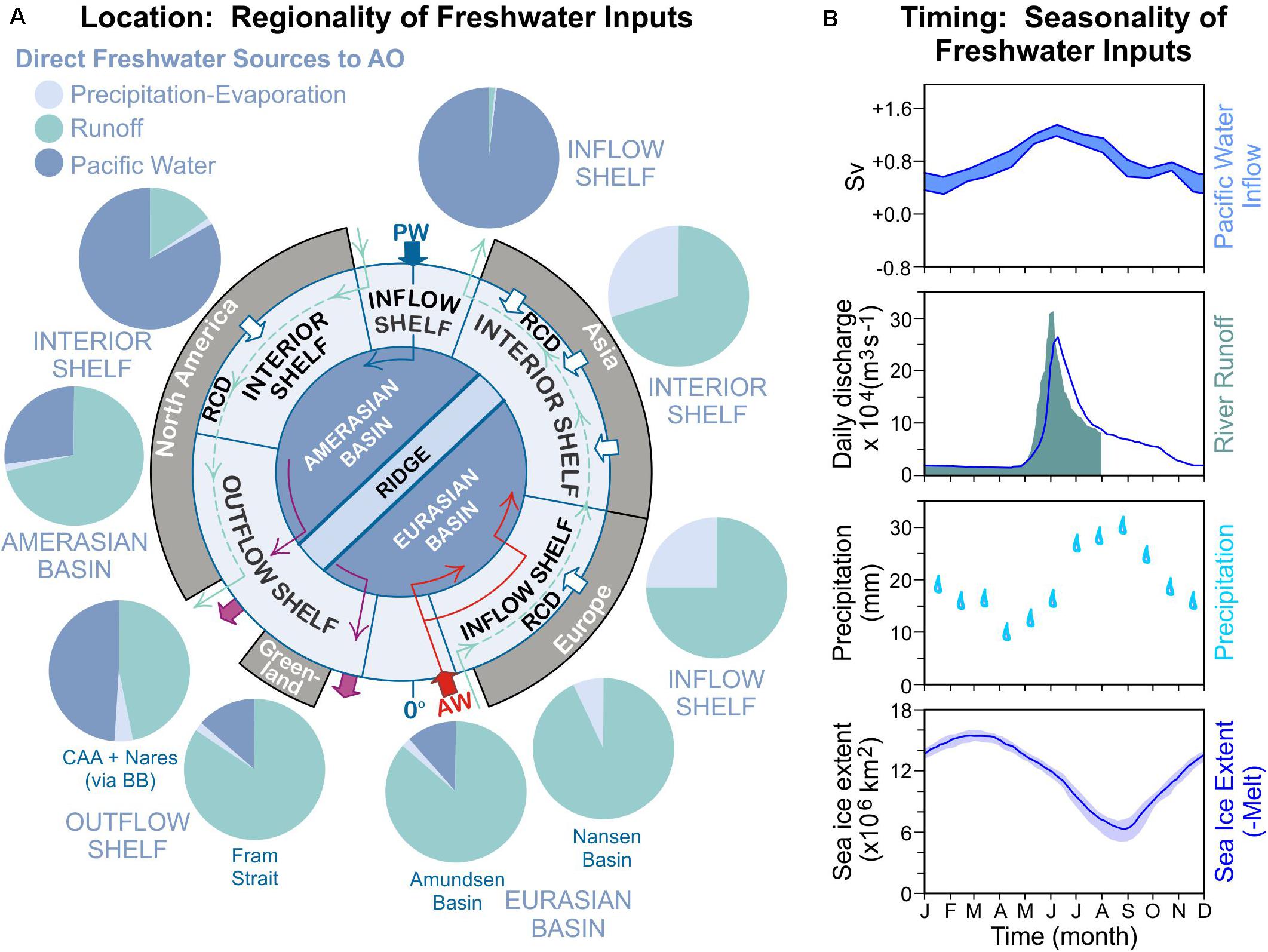
Figure 4. Regionality and seasonality of freshwater sources to the Arctic Ocean. (A) Regionality of freshwater sources: Conceptual representation of the regionality of freshwater sources (Annual runoff, RO, primarily from rivers; Precipitation-Evaporation, P-E; and Pacific Water, PW) to the Arctic Ocean (AO) showing the fractional contribution of each local freshwater source, with literature sources in Table 3. Note: Pacific Inflow shelves average freshwater inputs were estimated based on mean annual RO and P-E as a component of the 1 Sv of PW entering through Bering Strait annually. Pacific Interior shelf freshwater components were estimated with RO and P-E inputs scaled to an average meteoric water content of 20% for Polar Mixed Layer. Amerasian Basin freshwater components were determined by averaging estimates for the Makarov Basin and the Canada Basin. Depending on the location of the Pacific-Atlantic front, the amount of PW in the Eurasian Basin can be quite variable. Here we have separated the Nansen Basin and the Amundsen Basin to illustrate the presence-absence of PW. For the Atlantic Outflow shelves, we separate the CAA and Nares Strait outflow via Baffin Bay and Fram Strait. In all regions, Sea Ice Melt is considered a mixture of freshwater sources and would have the same fractional composition as surface waters. It should also be noted that the freshwater composition of each region is, at best, a qualitative description based on the time interval and regional coverage of the referenced study, and, in most cases, the components were determined using different geochemical tracers, data sets from different seasons, and different methods. For further insight, please see the referenced studies. Central map as in Figure 1B. (B) Seasonality of freshwater sources: Conceptual representation of the seasonality of freshwater inputs to the AO, as in (a), with literature sources as in Table 3. Pacific Water inflow climatology from 1990 to 2004 (purple shaded area; top panel). River runoff to the AO (second panel from the top), including average combined daily discharge for Eurasian rivers: Severnaya Dvina, Pechora, Ob’, Yenisey, Lena, and Kolyma for the period of January 1st to July 31st, 2015 (green shaded area); 1980–1989 average for January to July (dark blue line). Arctic precipitation climatology (third panel from the top) from 1957 to 1990. Arctic Sea Ice Extent (bottom panel) includes 1981–2010 median (dark blue line) and interdecile range (shaded area).
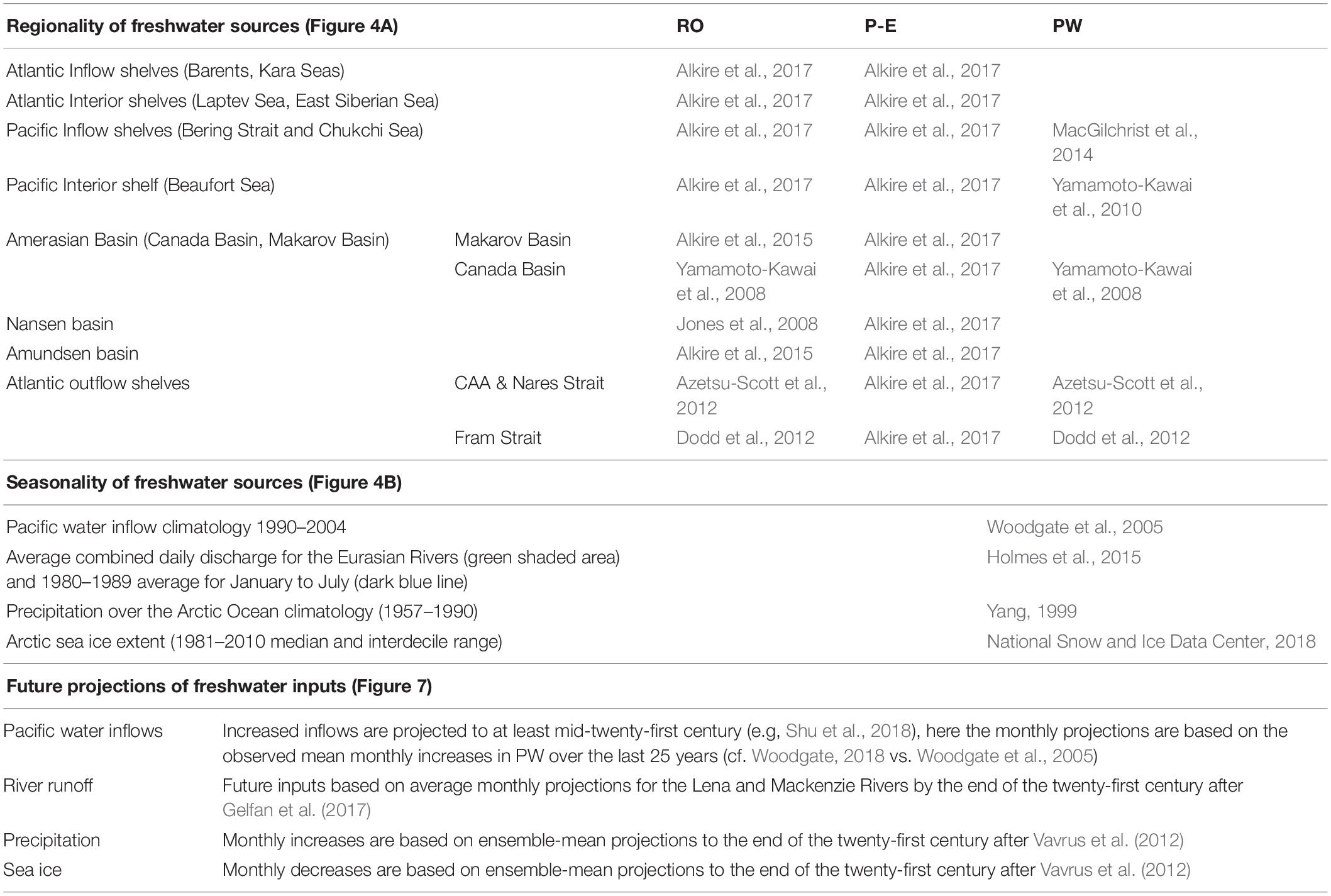
Table 3. Regionality and seasonality of freshwater sources to the Arctic Ocean and projections for the future, literature sources for Figures 4, 7.
Freshwater enters the AO in pulses, both to the shelf seas and central basins, and seasonal cycles dictate the delivery of FW from all sources, including PW inputs (Figure 4B and Table 3; Moore et al., 2018; note the PW FW flux is highly correlated with the volume flux through Bering Strait, Woodgate, 2018). River and PW inputs peak in early summer (June), whereas direct precipitation on the AO peaks later in September. Minima in FW inflows occur in early spring (April-May) for both rivers and precipitation sources, however PW inflows reach their minima in mid-winter (January). Freshwater is further cycled through the freeze-melt of sea ice while in the AO (Figure 4B), intermittently storing and releasing about 12% of the FW reservoir over seasonal and interannual time scales (Haine et al., 2015).
Expected future changes to the seasonality of inputs (e.g., increase RO in winter, Liljedahl et al., 2017; change in PW pathways into the central AO, Alkire et al., 2019; Krumpen et al., 2019) will have consequences not only for when FW enters each region, but for where these inputs are released and stored. For example, FW incorporated into sea ice within the Canada Basin is more quickly exported (>6 year residence time) compared to the liquid FW residing in the surface ocean (<11 year residence time; Yamamoto-Kawai et al., 2009). As well, an increased flux of RO into frozen estuaries with shallow coastal bathymetry can result in the increased retention of FW behind the landfast stamukhi ice zone in winter (McClelland et al., 2012), enhancing sea ice melt once this barrier breaks up in the spring (Nghiem et al., 2014). Overall, the regionality and seasonality of FW inputs, combined with the seasonal cycle of sea ice formation-melt, generate spatial and temporal heterogeneity in FW distributions across the AO, ultimately impacting the ML biogeochemistry most relevant for primary producers. In particular, FW inputs affect access to light (section “Regionality and Seasonality of Freshwater Dictates Access to Light”), nutrient and organic carbon availability (section “Regionality and Seasonality of Freshwater Dictates the Accessibility of Nutrients and Organic Carbon”), and the inorganic carbon composition of the surface ocean (section “Regionality and Seasonality of Freshwater Dictates Inorganic Carbon Composition of the Upper Ocean”).
Regionality and Seasonality of Freshwater Dictates Access to Light
In the AO, primary producers are constantly faced with trade-offs between the limited availability of light (discussed herein) and nutrients (see section “Regionality and Seasonality of Freshwater Dictates the Accessibility of Nutrients and Organic Carbon”). Access to light in surface waters is controlled by day length (time of year, latitude), by ice cover, and by turbidity in near-shore environments. The first major consequences of the changing FW “lids” is the effect of sea ice thinning and retreat on the light environment at a given latitude. Indeed, decreasing sea ice cover increases the ocean surface area available for primary producers to harvest light, and satellite-derived estimates of primary production (PP) indicate an increase in some areas experiencing more open water (e.g., Table 2; Arrigo et al., 2008; Arrigo and van Dijken, 2015), though there are many disputes about the robustness of estimates of PP from satellite derived products and their ability to predict harvestable or “new” production (cf. Randelhoff et al., 2020). Furthermore, light availability in the ever-expanding seasonal ice zone (SIZ) can be difficult to predict due to variability in light transmittance through different ice surfaces (Frey et al., 2011). However, simply adding more light does not translate into new PP everywhere. In situ observations fail to corroborate the direct light-PP relationship in some regions, in particular in those which are fresher and more nutrient depleted (Ardyna et al., 2011; Coupel et al., 2012). Furthermore, modeling studies also show disparities in the proportionality of new production and increase in annual photosynthetically active radiation (PAR; see Slagstad et al., 2015). The consequences of sea ice retreat, however, go beyond just changes in bulk productivity, as earlier sea ice retreat has the potential to change the phenology of blooms (Ji et al., 2013) and phytoplankton species composition (Neeley et al., 2018). Phytoplankton blooms have been reported to occur up to 50 days earlier in some areas of extreme ice retreat (Table 2; Kahru et al., 2011) which can create a mismatch situation for secondary consumers with established migration and life cycle patterns whom rely on spring blooms, particularly for the annual pulse of food rich in fatty acids (Søreide et al., 2010).
The place that regionality and seasonality may have the most pronounced impact on light availability is along the contiguous Riverine Coastal Domain (RCD, Carmack et al., 2015b; Figure 4A), which experiences large regional and seasonal fluctuations in both sea ice cover and terrestrial inputs. For ease of discussion, here we consider Greenland and other glacial fjords as part of the RCD, though there are physical mechanisms that control mixing present in some fjords that are not present along the majority of coastal river inputs (see discussion in section “Regionality and Seasonality of Freshwater Dictates the Accessibility of Nutrients and Organic Carbon” below), nor are they necessarily contiguous with the broader pan-Arctic domain. Positioned at the marine and terrestrial interface, the RCD is an intermittent but contiguous, counter-clockwise propagating feature which aggregates multiple riverine inputs along a narrow (<15 km) band of the shallow (<10 m) Arctic coastal zone, thus creating a virtually continuous pathway for terrestrial inputs of sediment, nutrients, and carbon (see Carmack et al., 2015b). The regionality and seasonality of FW inputs to this zone has direct geochemical and biological consequences. River inputs peak when sea ice extent is only part way through its seasonal decline (Figure 4B). With this peak in discharge comes an increased flux of sediments into river estuaries and fjords (Holmes et al., 2002; Overeem et al., 2017), impacting organic material availability and light climate (via turbidity; Wiktor et al., 1998; Murray et al., 2015).
Regionality in drainage basin geomorphic features plays an important role in sediment delivery and dispersal by rivers. For example, rivers that drain areas of tectonism and active glaciation are associated with high annual sediment fluxes (e.g., Mackenzie river) whereas those draining lowlands tend to have low annual sediment fluxes (e.g., Yenisey, Lena, Ob’ rivers; Holmes et al., 2002). Coastal erosion is further responsible for a significant amount of sediment (and particulate organic carbon) along the coastal ocean. In regions with glacial drainage systems, both tidewater and inland glaciers also contribute sediments to the coastal ocean (Table 2; Murray et al., 2015; Overeem et al., 2017; Halbach et al., 2019), much of which contains calcium carbonate and thus can impact the alkalinity of glacial runoff (see section “Regionality and Seasonality of Freshwater Dictates Inorganic Carbon Composition of the Upper Ocean”). Light can be extremely limiting in high turbidity fjords, with photic depths <10 m (Murray et al., 2015) and extremely steep pycnoclines during the runoff season (Holding et al., 2019). Although the nutricline may be located much deeper, the phytoplankton in these situations are generally concentrated in the upper 10 m due to light limitation, making it difficult to establish an SCM (Holding et al., 2019) and hence primary productivity tends to be minimal and dominated by regenerated, rather than new, production (Randelhoff et al., 2020). Phytoplankton subject to high turbidity, however, may be highly adapted to low light conditions, and hence in the fall, when runoff ceases and turbid inputs lessen, phytoplankton move further down in the water column, close to the nutricline, and maintain minimal production despite short day length and low light levels from seasonally low sun-angle (Table 2; Holding et al., 2019).
Although an important contributor to the light climate of the RCD, much of the suspended sediment delivered by rivers, glaciers, and coastal erosion will be retained in the deltas and estuaries of the coastal shelf, with limited delivery off-shelf and into the deep basins. But there is regionality in this retention as well. For example, river-dominated delta systems can accumulate 8–10 times more material than marine-dominated estuary systems (Bring et al., 2016). Filtration of this suspended material through deltaic and estuary systems (and their inhabitants) represents a significant sink for terrestrial sediments in the nearshore region (Wassmann et al., 2004), and in some situations, these inputs have proven to be detrimental to zooplankton and benthic consumers (White and Dagg, 1989; Wlodarska-Kowalczuk and Pearson, 2004; Thrush et al., 2004; Arendt et al., 2011; Hutchison et al., 2016) although effects are generally local at the point source of sediment input. Still, transport of material out of the RCD can occur through tidal and turbidity currents, as well as through incorporation into sea ice (e.g., Nürnberg et al., 1994; Eicken et al., 2005), which can increase turbidity in the ML upon melt away from the shelf.
Regionality and Seasonality of Freshwater Dictates the Accessibility of Nutrients and Organic Carbon
Even when light is not limiting, the presence or absence of FW in the surface ocean directly impacts the penetrating depth of seasonal mixing (see section “Introduction: The Arctic Ocean Has Two Freshwater Lids, and Both Are Changing,” Figure 2), with consequences for the accessibility of nutrients to sunlit surface waters. As described above, the depth of the ML is sensitive both to the physical conditions of the ocean surface (temperature, wind) and the stratifying effect of freshening, so it varies both seasonally and regionally (Figure 5). For example, the AO ML is deeper in winter (∼25 to > 50 m) than in summer (∼5–30 m), and its average extremes are greater in the eastern AO (up to 100+m maximum in winter in the EB), than the western AO (∼30 m maximum in winter in Canada Basin; Peralta-Ferriz and Woodgate, 2015). Freshening has been shown to have contributed to ML shoaling over the last three decades, with a larger impact observed in winter than in summer, and in the AB (20–40 m) compared to the EB (10 m; Peralta-Ferriz and Woodgate, 2015; Wang et al., 2019). And these extreme differences in stratification between the AB and EB may continue to diverge into the future (Polyakov et al., 2020). As FW is removed from the ML by sea ice formation over winter, the insulating effects of the sea ice cover progressively limit heat loss to the atmosphere (Rudels et al., 1996) and reduce wind-driven mixing, whereas penetrative convection by brine rejection helps to mix and homogenize the upper water column (Figure 3). Thus as the ML fluctuates over seasonal cycles, its ability to reach the depth of the nutricline to replenish surface nutrients is not assured (Figure 3). Without the annual disintegration of the summer mixed layer through convection induced by sea ice formation and brine export, nutrients cannot be replenished in the surface ocean (e.g., Nishino et al., 2020).
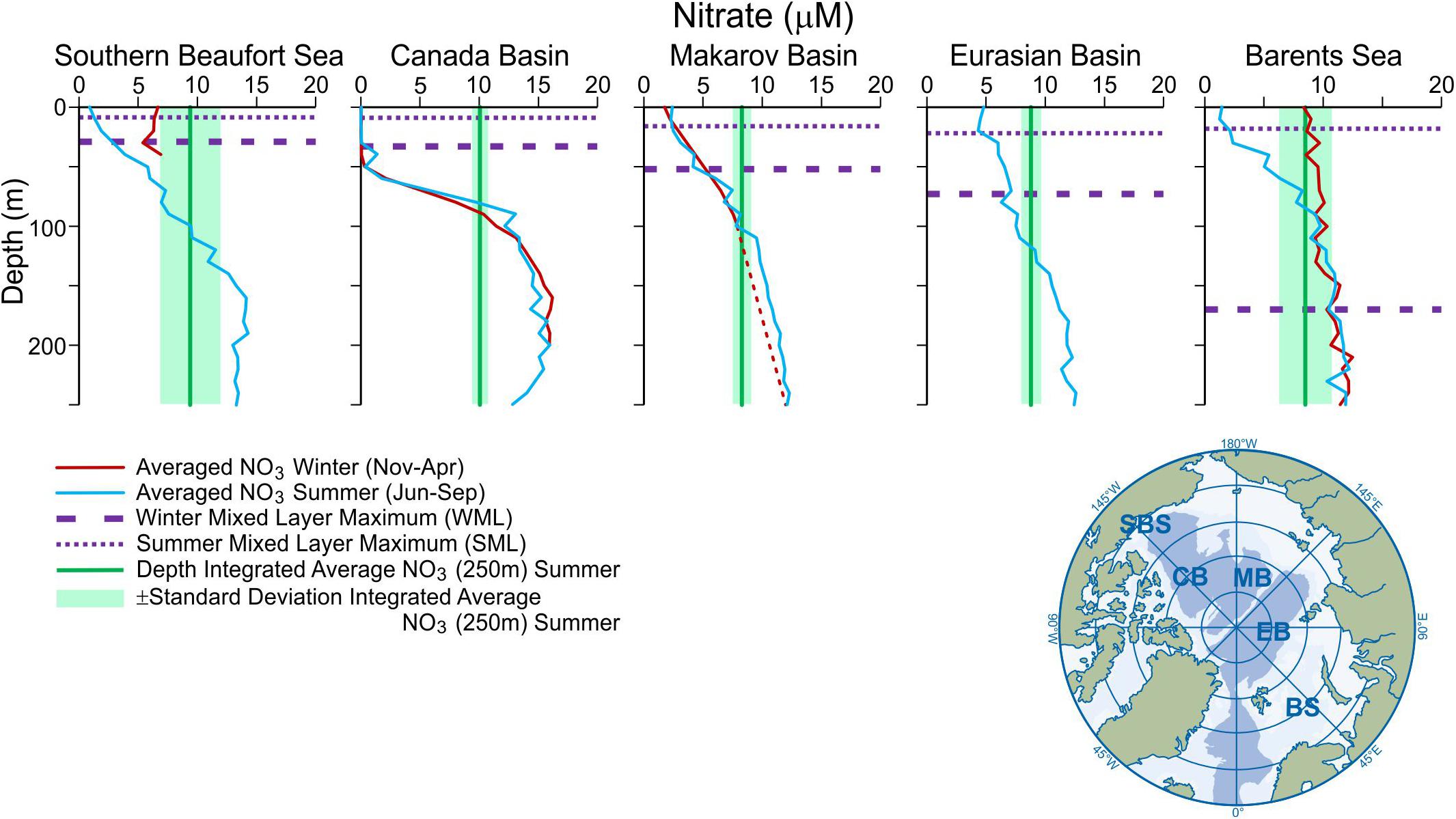
Figure 5. Seasonal mixed layers across the basins. Basin averaged Nitrate (NO3, μM) profiles from “summer” (June-Sept; blue lines) and “winter” (Nov-Apr; red lines) plotted along a transect through the Arctic Ocean from the Southern Beaufort Sea (SBS) to the Barents Sea (BS). Nitrate data were sourced from the Codispoti Arctic Nutrient Atlas (Codispoti et al., 2011), and have been binned over 10 m depth intervals and averaged for their respective seasons, where data were available. Purple dashed lines indicate the average depth of the seasonal mixed layer “summer minimum” (thin line) and “winter maximum” (thick line) after Peralta-Ferriz and Woodgate (2015), green vertical lines (± SD) indicate the depth integrated average NO3 in the upper 250 m from summer data.
Away from terrestrial (rivers and coastlines) and shallow shelf (remineralization) inputs, discussed below, the accessible “store” of nutrients in the AO basins resides at different depths (Figure 5). If both light and nutrients are available, primary producers will utilize all available nitrate in the ML. So it is this combination of the phytoplankton uptake of nitrate, and the physical conditions of stratification which constrains nitrate from being mixed up from below, that define the nutricline; practically, this is the depth at which inorganic nitrate begins to increase >1 μM (Codispoti et al., 2013). Primary producers reduce nitrate concentrations in the ML annually, necessitating access to nutrient stores held below the ML to fuel new PP in the subsequent year (cf. Figures 3, 5). The SCM depth is the observable result of this balance, which is created when limits are reached in the water column from below (by nutrient limitation) and above (by light limitation), or as a result of lateral advection. Although depth-integrated nitrate inventories in the upper 250 m are relatively consistent across the pan-Arctic basins (green vertical lines, Figure 5), their accessibility by primary producers is set by the upper ocean FW inventory (through stratification), resulting in a non-uniform nutricline depth across the AO (Figure 5). The nutricline itself is “leaky” due to turbulent diffusion (Randelhoff et al., 2020) but this surface FW stratification limits the extent. More nutrients diffuse into the upper water column when stratification is reduced (e.g., by brine rejection and deepening of the ML; Figure 3), but may be further deepened when ice cover is reduced under a convergent wind-field. As an example, wind-driven convergence of FW in the Beaufort Gyre resulted in the deepening of the nutricline in the Canada Basin, driving the SCM deeper in the water column over time (Table 2; McLaughlin and Carmack, 2010).
Regionality and seasonality can also play dual roles in limiting ML nutrient concentrations across the AO. While the Atlantic side gateways deliver a greater loading of nitrate (and phosphate), these loadings are partitioned through a water column ∼80–800 m deep, limiting accessibility to the euphotic zone (Torres-Valdes et al., 2013). In contrast, Pacific-sourced nitrate (and phosphate) through Bering Strait has higher concentrations and is delivered to a shallower and thinner layer ∼60–220 m deep (Tremblay et al., 2015). Pacific water inflow to the AO reaches a maximum in the summer months (June, July; Figure 4B), when phytoplankton in the Bering and Chukchi Seas are able to efficiently utilize available nitrate (Cooper et al., 1997; Brown Z. W. et al., 2015), limiting major nutrient influx to the winter season when PW inputs are lower (Figure 4B). The volume flux of PW inputs also impacts the residence time of waters on the Chukchi Sea inflow shelf, with corresponding implications for nutrient availability. Observations of reduced flushing time (∼7.5–4.5 months) associated with increased PW volume flux (1990–2015) could ultimately have far-field implications on nutrient availability and primary productivity, due to shorter bottom water residence times (e.g., reducing the time for organic matter remineralization) and faster currents (e.g., increasing turbidity with sediment resuspension, more rapid advection of plankton; Woodgate, 2018). Adjacent to the Pacific and Atlantic gateways, winter mixing in the shelf seas plays an important role in replenishing ML nutrients by tapping into mid-depth reservoirs of Pacific and Atlantic derived nutrients (SBS, BS; Figure 5), as well as bottom waters that have accumulated nutrients through remineralization in shelf sediments (Brown Z. W. et al., 2015; Granger et al., 2018). Within the deep basins, the EB maintains the highest year-round surface nitrate concentrations, likely due to the persistence of ice cover for much of the year (Randelhoff et al., 2020), whereas deep winter mixing in both the Canada and Makarov Basins is unsuccessful at replenishing surface nutrients as stratification limits penetration to the nutricline (Figure 5).
Should nutrients be replenished in the ML over winter, stabilization of the upper water column with sea ice melt aids in forming a phytoplankton bloom in the spring. This is the classic condition along the ice edge or the Marginal Ice Zone (MIZ), especially in the Barents Sea (Wassmann and Reigstad, 2011). This is also seen in the shelf seas, where upwelling at the Beaufort shelf-break can replenish ML nutrients in the fall, and nutrients not used up by late season blooms precondition the surface ocean for primary producers the following spring (Table 2). This translates directly into an increase in the abundance of secondary consumers, however, immediately off-shore, salinity stratification remains unaffected (Tremblay et al., 2011).
Similarly, it has also been suggested that delayed freezing and increased wind strength in fall may promote additional nutrient availability for PP in the shelves or over the deep basins (see Loeng et al., 2005 their Figure 9.13 for background hypothesis). Indeed, an increase in occurrence of fall blooms has been observed over the last decade (Table 2; Ardyna et al., 2014). But in more strongly stratified regions (e.g., AB) the strength of the summer halocline will likely constrain wind mixing at shallower depths until sea ice begins to form and thus delay mixing across the nutricline until light conditions inhibit a productive fall bloom (Figure 3, right-hand panel). Thus, wind mixing alone will not break the stratification of the seasonal halocline in these regions (Nishino et al., 2020). Furthermore, there are large areas of the AO where an increased occurrence of fall blooms has not been observed (e.g., Barents Sea, Baffin Bay; Ardyna et al., 2014), as they are already commonplace. In AW-influenced regions, such as the Barents Sea, weaker stratification causes ice formation to occur later, or not at all, and winter vertical mixing is driven by convective cooling forces rather than brine rejection (Loeng, 1991).
Along the RCD, seasonal inputs of terrestrial nutrients can be regionally important to satisfy deficiencies in the marine nutrient budget. Inorganic nutrient concentrations tend to be highest during winter baseflow (Holmes et al., 2012), providing a source of nutrients for PP in the RCD and shelf seas as spring advances and light-limitation is reduced (e.g., day length increases, sea ice thins, sedimentation and erosion are low; McClelland et al., 2012). But these contributions of riverine nitrogen to new PP have been shown to be small at a regional scale, and estimated to be negligible at a pan-Arctic scale (Le Fouest et al., 2013). On the other hand, slow remineralization of riverine dissolved organic nitrogen makes riverine nitrogen a potentially important source for PP later in summer, and in open ocean waters away from the RCD (Tank et al., 2012b; Le Fouest et al., 2015). Many unknowns remain about the present and future importance of terrestrial nutrient input from rivers and there is an urgent need for more research on this topic as runoff becomes and ever-increasing source of FW to the AO.
Inputs from glacial melt have also been suggested as a source of macro- and micro-nutrients (e.g., silicate, iron, nitrogen, organic matter) to the RCD (Table 2; e.g., Hood and Scott, 2008; Bhatia et al., 2013; Lawson et al., 2014; Meire et al., 2016). However, apart from iron and silicate, other macronutrients concentrations are considerably lower in glacial melt compared with marine waters, and glacial melt may thus act to dilute rather than enrich the surface ocean (Hopwood et al., 2020). Moreover, as nitrate is generally the limiting nutrient in the AO, additional fluxes of iron are unlikely to have significant positive effects on PP, even at a local fjord scale (Hopwood et al., 2020). On the other hand, tidewater glacial fjords can have locally important mechanisms of upwelling at glacial fronts (Hopwood et al., 2018), bringing nutrients up from depth to contribute to prolonged late summer blooms (Juul-Pedersen et al., 2015), which can support entire ecosystem production (Table 2; Meire et al., 2017).
As discussed above, rivers also carry terrestrially derived organic carbon into the RCD, a nutritive food source for microbial communities. Particulate sediment delivery into river estuaries and deltas peaks in spring with FW discharge and dissolved organic carbon (cf. Holmes et al., 2002, 2013). Furthermore, POC fluxes due to erosion can be highly regional and temporally variable, and may exceed riverine inputs by as much as sevenfold (cf. Lantuit et al., 2013; McClelland et al., 2016). River inputs can also bring heat, facilitating the early melt-back of ice and sea surface warming (Dean et al., 1994), potentially pre-conditioning permafrost thaw in the advance of sea ice retreat and contributing to further sediment export.
Organic carbon inputs from permafrost thaw (coastal erosion, river inputs) and glacier and ice sheet melt have been the topic of much attention recently, due to the potential for these vast, labile carbon stores to be exported to the coastal ocean (Hood et al., 2015; McClelland et al., 2016; Le Fouest et al., 2018; Wadham et al., 2019). Carbon in river runoff, eroded permafrost sediments, and glaciers has been found to be highly labile to microbial communities (Table 2; Vonk et al., 2013; Paulsen et al., 2017; Sipler et al., 2017), causing both the quantity and quality of these inputs to influence ecosystem carbon cycling, and potentially converting large areas of coastal ocean into sources, rather than sinks, of CO2 (discussed further below; Terhaar et al., 2019). However, as with inorganic nutrients, glacial and ice sheet organic carbon concentrations are orders of magnitude lower than runoff from the large Arctic Rivers and coastal erosion (Hood et al., 2015; Paulsen et al., 2017; Hopwood et al., 2018; Wadham et al., 2019). Thus, while labile, glacial runoff contributions of allochthonous carbon are likely acting to dilute dissolved organic carbon concentrations (Paulson and Robson, 2019; Hopwood et al., 2020), and enhance particulate organic carbon in glacial fjords (Paulson and Robson, 2019). Organic carbon and nutrients from the Greenland Ice Sheet are likely to have little impact offshore; rather, their influence is likely limited to within local fjords (Hopwood et al., 2020).
Regionality and Seasonality of Freshwater Dictates Inorganic Carbon Composition of the Upper Ocean
Low temperature and salinity, combined with highly productive inflow shelves, predispose the AO to be a sink for atmospheric CO2 (e.g., Bates et al., 2006; Bates and Mathis, 2009; Cai et al., 2010; MacGilchrist et al., 2014). Persistent ice cover in the central basins (e.g., Canada Basin), however, limits air-sea CO2 exchange and acts as a semi-permeable barrier (Bates et al., 2006; Miller et al., 2011), and contributes to sustained pCO2 under-saturation (e.g., Jutterström and Anderson, 2010). Satellite observations and modeling studies suggest that the increased seasonally ice-free area over the shelf seas has allowed increased PP in recent decades (e.g., Zhang et al., 2010; Arrigo and van Dijken, 2015), thus enhancing the biological carbon pump and increasing the CO2 sink across the Pacific inflow and interior shelves (Manizza et al., 2013). However, similar increases in open water area on the Atlantic inflow shelf have seen a decrease in CO2 uptake capacity with warming. These observations reveal that a reduced ice cover does not necessarily lead to an increased CO2 sink (Manizza et al., 2013), in particular in the central AO basins (Cai et al., 2010; Else et al., 2013). Along the shelf seas, the net balance of CO2 sources and sinks is maintained by terrestrial organic matter (OM) input, marine OM production and respiration, retention or export of OM off-shelf, and the net air-sea exchange (Bates and Mathis, 2009).
The coastal zone, in particular the RCD, is a major site of both inorganic carbon inputs and modification, with implications for the inorganic carbon balance both within and away from the RCD. River and glacial runoff are generally characterized by low alkalinity, carbonate, and bicarbonate ion concentrations compared to marine waters, resulting in under-saturated conditions for aragonite along mixing gradients into coastal estuaries (Chierici and Fransson, 2009; Mathis et al., 2011; Yamamoto-Kawai et al., 2013; Fransson et al., 2015). Aragonite is the major building block of carbonate shelled organisms and its saturation state (Ω-aragonite) determines the ease at which CaCO3 mineral precipitates can be formed in solution. Regionality and seasonality further modulate the generally under-statured Ω-aragonite condition of river inputs. North American rivers tend to have higher alkalinity (higher Ω-aragonite values) than Eurasian rivers (Cooper et al., 2008; Tank et al., 2012a); furthermore, Ω-aragonite values are more diluted by high flows in spring compared to later in summer, with the highest values expected under-ice in winter (Tank et al., 2012a). Seasonal temperature fluctuations, and episodic events like storm-induced upwelling, will further influence these patterns, potentially causing some areas to transition between under-saturated and saturated states over the seasonal cycle (e.g., Mathis et al., 2011, 2012). In regions with glacial drainage systems, sediment contributions from both tidewater and inland glaciers can contain large quantities of calcium carbonate, contributing to increasing the alkalinity of the mixing marine waters, increasing Ω, and reducing the partial pressure of CO2 (pCO2; e.g., Fransson et al., 2015).
Both biogeochemical (e.g., respiration, photosynthesis) and physical (e.g., temperature fluctuations, air-sea exchange) processes contribute to altering the CO2 content, and thus the CaCO3 saturation state, of coastal surface waters as they are transported into the deep AO basins. In spite of this, the impacts of the regionality of river runoff are not confined to the local RCD. Due to differences in the composition of their drainage systems, North American and Eurasian river inputs can be traced by their (total) alkalinity concentrations (e.g., Yamamoto-Kawai et al., 2009); as such, terrestrially sourced alkalinity propagates across the AO with river waters and contributes to setting the CO2 uptake capacity of the ML in the central basins far away from the RCD (e.g., Yamamoto-Kawai et al., 2009; Tremblay et al., 2015).
The seasonal cycle of sea ice formation and melt further acts to redistribute inorganic carbon across the atmosphere-ice-ocean interface (Miller et al., 2011; Rysgaard et al., 2011). This leaves the sea ice depleted in CO2 but with excess carbonate (alkalinity) going into the melt season (Rysgaard et al., 2011; Brown K. A. et al., 2015). In spite of this excess alkalinity, sea ice melt is even more diluted than river inputs, imparting a greater effect of lowering marine Ω-aragonite values upon melting (e.g., Yamamoto-Kawai et al., 2009). River runoff and sea ice melt will contribute to surface ocean freshening at different seasonal intervals, however, with rivers reaching their peak discharge along the coastal margins in spring and sea ice melt reaching a maximum in late summer (Figure 4B), extending the input of low-Ω waters to the entire open water season.
Due to the complex and sensitive interplay between the FW cycle and the inorganic carbon composition of the upper ocean, the AO has been considered as a “bellwether” for the effects of global ocean acidification on biota (e.g., Fabry et al., 2009). The impacts of ocean acidification on biota are likely to appear in the AO before other areas of the global ocean, and include the potential fertilization of primary producers via increased CO2 (e.g., Hein and Sand-Jensen, 1997) and the dissolution effect of CaCO3 shelled organisms via acidification (e.g., Feely et al., 2004). There is evidence for and against fertilization effects (Table 2; Engel et al., 2013; Holding et al., 2015; Hoppe et al., 2018). Potential fertilization by CO2 is likely temperature dependent (Holding et al., 2015) and any positive effects on heterotrophic processes (e.g., Vaqué et al., 2019) may preclude effects on autotrophic processes resulting in a net-zero effect (i.e., Hoppe et al., 2018). Community composition change will likely also be altered as some taxa are favored in a high-CO2 environment (Coello-Camba et al., 2014b; Dutkiewicz et al., 2015; Schulz et al., 2017). Evidence for dissolution effects of ocean acidification on biota have largely been experimental (Hendriks et al., 2010), and few, if any, studies have observed impacts of OA on organisms in situ. Multiple lines of evidence are necessary to attribute impacts of environmental change (O’Connor et al., 2015) and as we have already begun to observe corrosive conditions in some areas of the Arctic (e.g., Mathis et al., 2015; Zhang et al., 2020), it will be important to look for evidence of OA affecting organisms in situ as well as experimentally. The recent AMAP Assessment on Arctic Ocean Acidification (AMAP, 2018) provides an overview of some of the possible impacts of future acidification on biota, including: changes in food quality altering trophic energy transfer; decreased calcification and shell diameter of foraminifera and pteropods; and general negative responses to acidification by gastropods, bivalves, copepods, and other crustaceans, including their larval stages (see Falkenberg et al., 2018 and references therein). While no observable impact or positive impacts have been documented in cold water corals and macroalgae, only a small proportion of these studies were performed with Arctic species.
Impacts of Continued Freshening of the Arctic Ocean on Physics, Geochemistry, and Biota
Projections from global coupled climate models indicate that the upper Arctic Ocean will continue to freshen over the twenty-first century (e.g., Kattsov et al., 2007; Vihma et al., 2016; Shu et al., 2018), with consequences for the physics, geochemistry, and biota of the upper AO. From a pan-Arctic perspective, FW storage in the upper AO is anticipated to increase proportionally with FW input, impacting regional distributions of FW across the AO. Increased FW input and residence time is predicted to result in an overall shoaling of the seasonal halocline and a diversion of FW outputs from the Canadian Arctic Archipelago (CAA) toward Fram Strait (Pemberton and Nilsson, 2015). Outflow shelves continue to be the point of exit for all FW from the AO, with export of liquid FW via Fram and Davis Straits predicted to increase by the end of the century (Shu et al., 2018).
In addition to FW, further heat input to the AO is also anticipated. From the Pacific side, increasingly earlier arrival of warmer waters entering Bering Strait has been observed over the last two decades (Woodgate, 2018; Danielson et al., 2020) and is likely to continue, while the storage of heat in the near-surface of these waters advancing from the Pacific is expected to increase (e.g., NSTM, Jackson et al., 2010). On the Atlantic side, the suppression of vertical heat flux due to increased stratification is predicted to result in the advection of a warmer Atlantic layer (Pemberton and Nilsson, 2015; Nummelin et al., 2016). Within the AO, the seasonal cycle of sea surface temperature is predicted to amplify most in regions historically covered by sea ice, moving the seasonal heat sink of the F/M cycle directly into the surface ocean and contributing to continued surface warming through the twenty-first century (Carton et al., 2015).
Regionality
These anticipated changes in FW inputs, outputs, and cycling will exert different pressures on different AO hydromorphological domains (Figure 6). For example, inflow shelves will experience more prolonged exposure to solar radiation as sea ice extent continues to retreat. This may have the effect of increasing the surface area for PP (cf. Arrigo and van Dijken, 2015) at least until surface nutrient supplies are depleted (e.g., Vancoppenolle et al., 2013; Slagstad et al., 2015). Atlantic waters are anticipated to continue to warm and the redistribution of boreal species along this warming gradient will contribute to the “borealizetion” of the Arctic along the Atlantic water flow path (Polyakov et al., 2020). Sea ice retreat from the shelves will increase wind-driven shelf-basin exchange, thus contributing to increased nutrient fluxes and increased currents. In particular, some shelf-break slopes will experience increased upwelling, bringing deep nutrients up into exposed surface waters fueling PP in the fall and storing nutrients for the following spring (e.g., Tremblay et al., 2011; Slagstad et al., 2015), though this is not necessarily ubiquitous for all shelf break slopes across the Arctic (Randelhoff and Sundfjord, 2018).
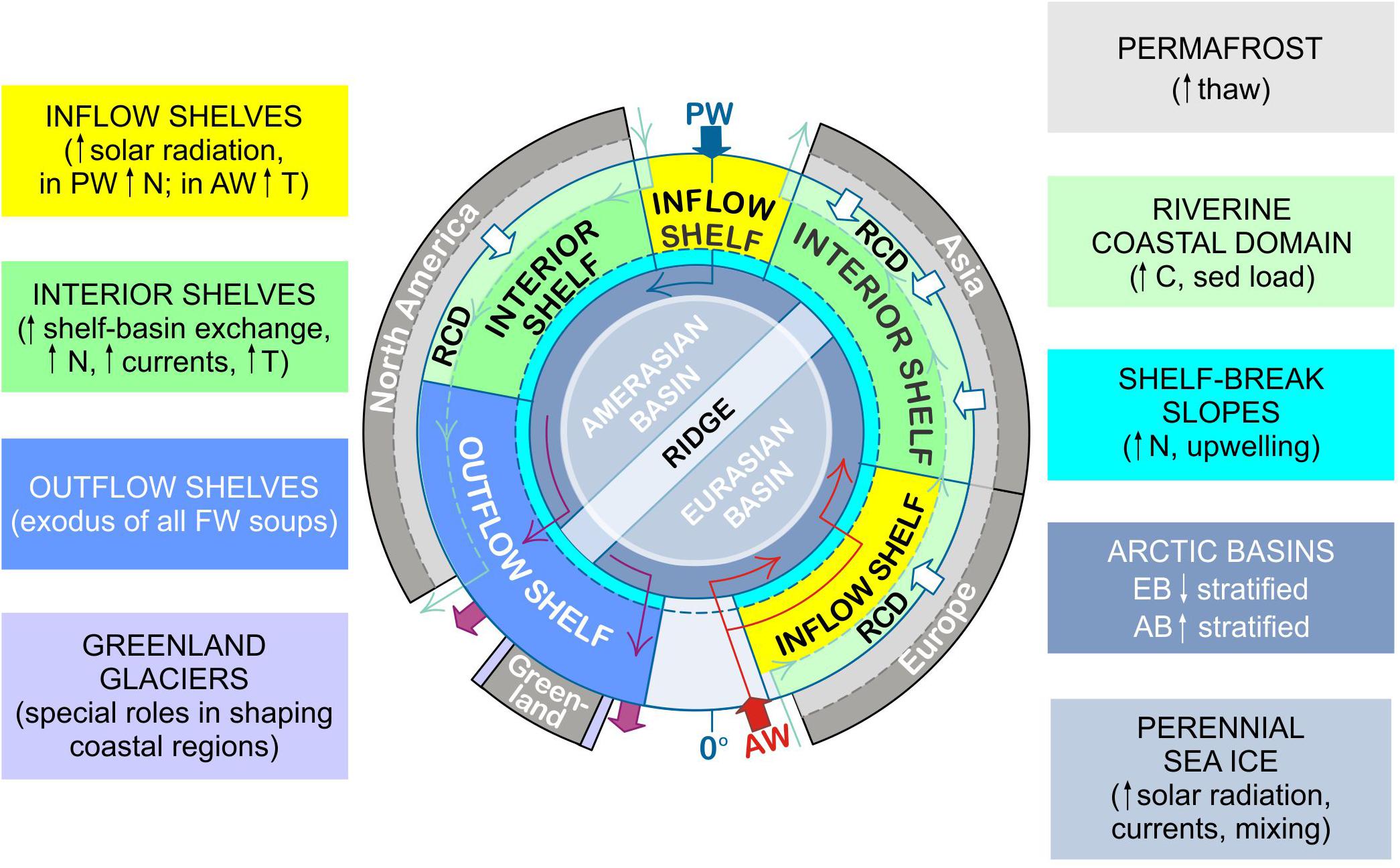
Figure 6. Regional impacts of future freshwater change. Predicted future changes in freshwater inputs and warming will have differing regional impacts to the upper ocean across the hydromorphological domains as discussed in the text (section “Regionality”). For example, the interior basins are anticipated to experience increased (Amerasian Basin, AB) and decreased (Eurasian Basin, EB) stratification due to the redistribution of sea ice melt water from the EB into the AB. Central map as in Figure 1B.
Much of the future freshening of the AO will be observable in the central basins (Canada, Makarov, and Amundsen; Shu et al., 2018), and as such, the deep Arctic basins will continue to diverge, with the AB further stratifying and the EB becoming less so (Polyakov et al., 2020). Further stratification in the AB may push primary producers further from the surface away from light toward exploitable nutrients (cf. McLaughlin and Carmack, 2010), while turbulent nutrient supply may be enhanced in the EB. The continued loss of sea ice and melt water stratification in the central basins are predicted to increase the AO CO2 – sink in the short term (Bates et al., 2006; Arrigo et al., 2008). However, there is evidence that this CO2 uptake capacity is limited (Cai et al., 2010; Else et al., 2013) and may already have been reached in the CB (Zhang et al., 2020). Continued warming of the stratified mixed layer (Carton et al., 2015), dilution of alkalinity (Woosley and Millero, 2020), increased microbial remineralization of organic material (Bates and Mathis, 2009), and a reduced biological pump (Li et al., 2009) will further reduce the surface ocean’s ability to take up CO2 from the atmosphere as FW inputs increase.
As the receiving zone for the majority of riverine input to the AO, the RCD will be the first zone impacted by up to an anticipated 50% increase in river discharge to the coastal margins projected in the coming decades (Figure 6; Bring et al., 2017). Continued freshening and warming of the terrestrial system will further result in changing sedimentation patterns. As discussed above, increased discharge will likely increase within-river sediment flux proportionally, which, combined with warming of underlying permafrost in the drainage basin, is likely to result in increased suspended sediment and organic matter fluxes to the coastal AO. Facilitated by permafrost thaw, coastal erosion is further susceptible to the changing seasonality of sea ice retreat and ocean warming. An earlier sea ice retreat in the spring, closer to peak river discharge, can result in higher insolation and warming of the coastal ocean, which contributes to increased permafrost thaw (Barnhart et al., 2014), whereas expansion of the ice free period in the fall increases the impact of fall storms and enhances coastal erosion rates due to increased wave action, in particular in areas where ground-ice content is high (Overeem et al., 2011; Barnhart et al., 2014). Warming and release of carbon and coastal erosion may enhance bacterial breakdown of this allochthonous material (e.g., Vonk et al., 2013; Sipler et al., 2017), while increased turbidity from sediment inputs may affect the light environment for primary producers (e.g., Wiktor et al., 1998; Holding et al., 2019).
Glacial fjords, especially around Greenland, are expected to experience continued glacier retreat. Retreat of tidewater glaciers will reduce the occurrence of upwelling (Hopwood et al., 2018), which bring nutrients to the surface waters of fjords and can support significant late summer productivity (e.g., Juul-Pedersen et al., 2015) and large marine foodwebs (e.g., Meire et al., 2017). Without these mechanisms for mixing, fjords may switch to lower-productivity systems, which are controlled by strong stratification and light limitation from turbid inputs (e.g., Holding et al., 2019).
Seasonality
Shifting seasonality in FW inputs are also anticipated to alter the timing of FW delivery to the upper ocean (Figure 7 and Table 3). Pacific water inputs to the AO are projected to increase for at least the first half of the twenty-first century (Shu et al., 2018), and although future shifts in seasonality are not clear, increases could be anticipated in virtually all seasons if trends continue to follow mooring observations from the last 25 years (cf. 2003–2015 climatology from Woodgate, 2018 vs. 1990–2004 climatology from Woodgate et al., 2005). The importance of the Pacific-Arctic pressure head in driving the volume flux (Woodgate et al., 2010), implies that increased inflows may follow projected seasonal decreases in Arctic sea level pressure, which are lowest in Nov-Dec (Vavrus et al., 2012). Note, however, that strengthened stratification as freshening continues to increase across the AO may contribute to a decreased pressure gradient, potentially leading to reduced PW influx (e.g., Nummelin et al., 2016).
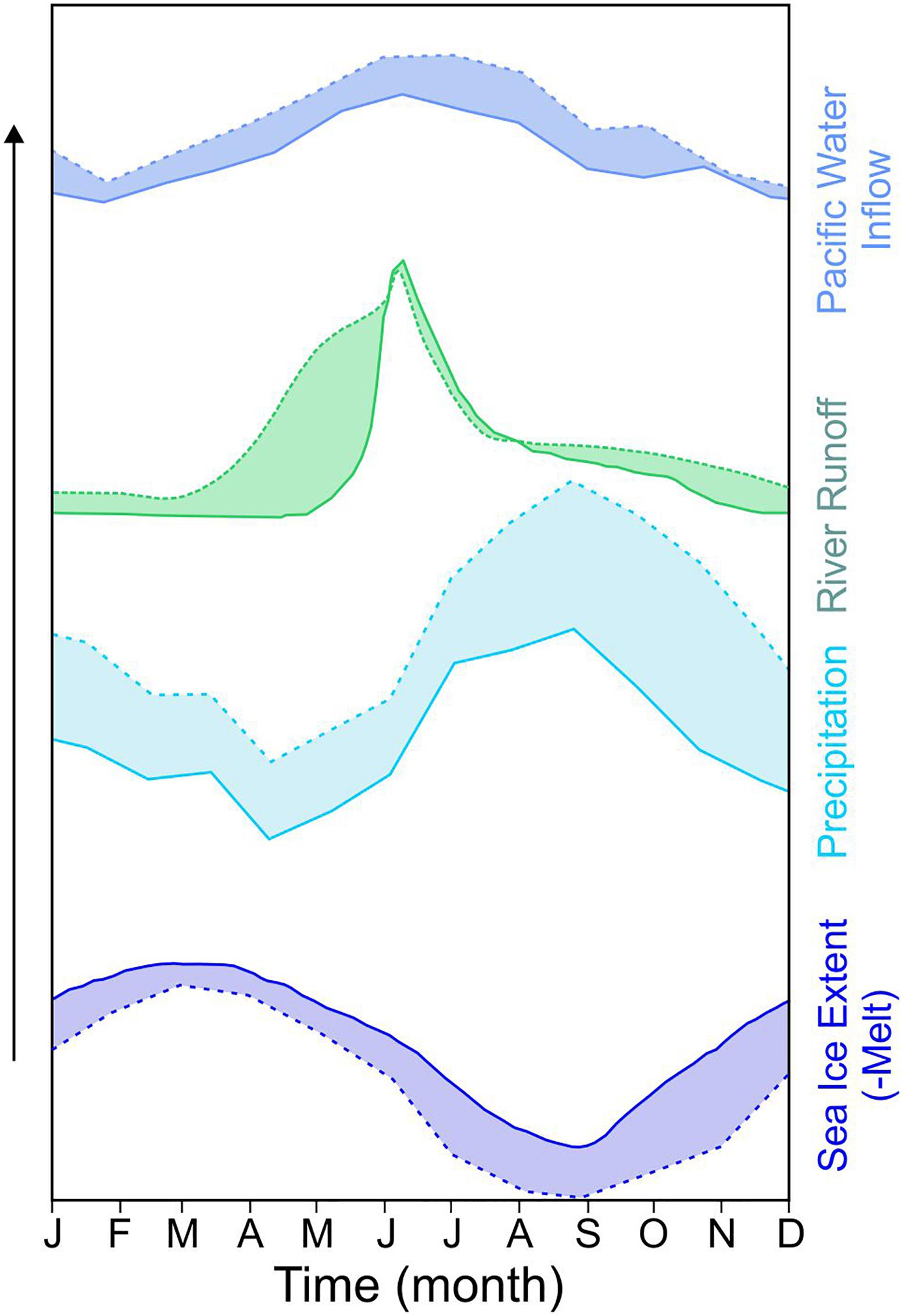
Figure 7. Seasonal impacts of future freshwater change. Predicted seasonal shifts in freshwater inputs to the Arctic Ocean will alter the timing of freshwater delivery to the upper ocean, as discussed in the text (section “Seasonality”). Here, dashed lines and shaded areas indicate the predicted relative seasonal changes with respect to the present-day conceptual representations presented in Figure 4B, following literature cited in Table 3. Pacific Water inflows (purple shading, top panel) are predicted to increase at least until the mid-twenty-first century, with anticipated increases in virtually all seasons if trends continue to follow mooring observations from the last 25 years. River runoff (green shading, second panel from top) can be expected to increase overall with a seasonal shift to increased discharge in early spring and late fall. Overall precipitation (light blue shading, third panel from the top) is predicted to continue to increase in all seasons, with the largest increases in the autumn and winter months. Sea ice concentration (dark blue shading, bottom panel) is anticipated to decrease in all seasons into the future.
An increasingly intense Arctic hydrological cycle will result in increased precipitation and evaporation, which peak in late autumn and winter (when evaporation peaks; Bintanja and Selten, 2014), making the future AO warmer, wetter, and cloudier (e.g., Vavrus et al., 2012). Along with a general increase in annual precipitation by the end of the twenty-first century, the largest increases are anticipated in the fall and winter months (Oct-March; e.g., Kattsov et al., 2007; Vihma et al., 2016). Furthermore, a diminished sea ice cover permits more atmosphere-ocean exchange throughout the year, but particularly in the winter. Increased evaporation and low cloud cover create a positive feedback mechanism that contributes to further warming of the surface ocean in the shoulder seasons (Huang et al., 2019), and with more evaporation, local precipitation becomes more important (Bintanja and Selten, 2014). River discharge is also anticipated to increase (e.g., Nummelin et al., 2016), with the most pronounced increases along the coasts of central and eastern Siberia and Alaska, north of 70°N (Bring et al., 2017). Seasonality in pan-Arctic river discharge is anticipated to continue following recent trends shifting to earlier peak flows and overall increased discharge, most notably in the fall and winter (Holmes et al., 2018; Ahmed et al., 2020). For example, mean model projections for the Lena and Mackenzie rivers show the most dramatic increases in discharge in April and May, whereas peak summer flows in June-July-August are predicted to reduce only marginally, shifting the seasonal distribution considerably earlier by the end of the twenty-first century (Gelfan et al., 2017). It is important to note that these projections consider river inputs only, glacial inputs are more complicated as they consist of a combination of solid ice discharge and surface runoff (derived from surface mass balance), which is driven by atmospheric processes, ocean processes, and albedo feedbacks among other things. On the Greenland Ice Sheet, solid ice discharge is becoming less prominent as glaciers retreat and thin, while surface mass balance is becoming increasing important, making up ∼60% of total discharge in recent decades (Shepherd et al., 2020). Similar to rivers, it is likely that the glacial runoff season will continue to lengthen on either side of the peak melt discharge, as it has been increasing at a rate of 2 days per year since 1972, with a total increase of 70 days (Mernild et al., 2011). Locally this will act to maintain shallow stratification of the fjords for a larger portion of the growing season.
Sea ice volume is also predicted to continue to decrease substantially, coupled with decreased ice formation in winter and longer open water seasons, shifting and dampening the seasonal fluctuations between solid and liquid FW storage in the surface AO. Recent predictions further indicate ice-free summer conditions are possible as early as mid-century (e.g., Stroeve and Notz, 2018; SIMIP Community, 2020). As discussed above, this reduced temporal extent of sea ice cover may continue to change bloom phenology, whereby blooms may occur even earlier (e.g., Kahru et al., 2011) causing a mismatch with zooplankton predators, or further increase the incidence of fall blooms (e.g., Ardyna et al., 2014) due to enhanced wind mixing with later sea ice formation.
The shifting seasonality patterns of FW inputs will also impact the CaCO3 saturation state of seawater (Ω) in the surface AO. Projections of continued increases in atmospheric CO2 will drive further decreases in surface ocean Ω (Zhang et al., 2020). However, the ocean’s response is not straightforward, and is seasonally dependent. Increased stratification due to a reduced, or absent, F/M cycle will reduce the seasonal dilution of the surface AO, which acts to lower Ω; while increased warming in the upper ocean and enhanced primary productivity act to increase Ω (Bates and Mathis, 2009; Zhang et al., 2020). The CaCO3 saturation state of the surface ocean thus follows the seasonal cycle of FW inputs, responding in parallel to a fresher future AO. Dilution of the surface ocean from increasingly earlier river inputs will extend the seasonal contribution of low-Ω waters, potentially beginning as early as March (Figure 7). As river inputs progressively shift their seasonality to earlier spring flows, sea ice melt dilution will add further to reducing Ω and increasing stratification in summer, while delayed freeze-up will stabilize the surface ocean even later into the fall. This expanded window of the seasonal input of low-Ω waters to the surface AO may now cover 2/3rds of the year. Continued FW stratification of the surface ocean will maintain surface water interaction with the atmosphere longer, contributing to increased CO2 uptake across the air-sea interface until equilibrium is reached. Stratification will also restrict nutrient replenishment, limiting PP and weakening the CO2 sink associated with organic matter export. Both of these responses to increased stratification will contribute to lower surface ocean Ω. Increased FW stratification, however, also contributes to a warmer surface ocean, thus seasonally increasing Ω, and potentially turning the AO into a CO2 source, until fall cooling begins and Ω (and pCO2) are again lowered. In a future perennially ice-free ocean, lacking a F/M cycle, the seasonal cooling of the surface ocean in winter would further reduce Ω; however, strong mixing by late fall and winter storms could successfully bring more low-Ω PW into the surface, preconditioning for an even lower Ω-state the following spring.
Potential Future States of the Upper Arctic Ocean Under a Changing Freshwater System
The evolution of our understanding of the upper AO over the last several decades has established that there is no “average” Arctic Ocean, but that regionality and seasonality set the physical and geochemical constraints upon which biological communities develop. First, FW plays a primary role in the regulation of upper ocean circulation and mixing processes. Second, the impacts of FW on geochemistry are dependent on the source, location, and timing of FW inputs, and as such, the characteristics of FW typically used to define it from a physical perspective (e.g., S < 34.8) are inadequate to distinguish its biogeochemical properties and importance in setting the geochemical state of the upper ocean. Third, the effects of FW on biological systems (Table 2) can be described as a combination of the indirect physical effects (e.g., changing light environment from sea ice and/or turbid inputs, stratification-mixing, upwelling at shelf breaks and glacial fronts) and direct geochemical effects (e.g., nutrient and carbon addition-dilution from FW runoff, sediment addition, changing inorganic carbon chemistry; Table 2), which are also dependent on FW source, regionality, and seasonality. As such, the physical, geochemical, and biological processes of the upper AO are intrinsically linked to FW fluxes through the AO, across global to local scales, and are at the mercy of their complex and changing state.
We now find ourselves standing at a precipice, where the AO FW system is trending away from its previous state and changing more rapidly than parameterization-based models can predict (e.g., Stroeve et al., 2007, 2012a; Wang and Overland, 2012; Box et al., 2019). Non-linear system responses add to this uncertainty (i.e., Lenton, 2012). This begs the question, “what if a future state of the AO FW system is one that does not include the seasonal presence of sea ice?” The FW burden from ice melt that is recycled annually in the central basins has approximately doubled since the 1980s, and this FW loading will persist into mid-century as we enter an ice-free summer state (section “Changing State of Freshwater in the Arctic Ocean,” Table 1; e.g., Stroeve and Notz, 2018; SIMIP Community, 2020). A future AO, experiencing another ∼40% more sea ice melt during the summer biological growing season will continue to impact physical, geochemical, and biological systems of the upper AO, undoubtedly with magnifying consequences for stratification and warming (as described in section “Impacts of Continued Freshening of the Arctic Ocean on Physics, Geochemistry, and Biota”). Yet, the trajectory of melt water increase cannot continue unabated into the future. Continued sea ice loss, in particular in the ice-recycling stronghold of the Beaufort Gyre, has the potential to alter the balance of the atmosphere-ocean coupling that constrains liquid and solid FW storage within this surface convergent system (cf. Proshutinsky et al., 2009). It should also be noted that the atmospheric Beaufort High and oceanic Beaufort Gyre are the only coupled anticyclonic systems (with enhanced FW storage) in the Northern (beta) Ocean (Carmack et al., 2015c), and that relaxation of this coupling will alter spreading pathways within the AO and may release excess FW into the subarctic North Atlantic, thus affecting meridional overturning (Proshutinsky et al., 2015). In a future, warmer and fresher AO, seasonal processes may no longer shunt FW through the sea ice freeze-melt cycle; leaving a perennially ice free AO without this significant seasonal redistribution of FW in the upper ocean. This scenario, once thought improbable, may already be beginning along the AO fringes of inflow shelves, where unprecedented winter sea ice loss has been observed in the Bering (Stabeno and Bell, 2019) and Barents (Schlichtholz, 2019) seas, and conceivably could expand into the Nansen Basin (cf. Aagaard and Carmack, 1994). Furthermore, modeling studies warn that a seasonal ice-free state is all but assured by the end of the twenty-first century (e.g., Jahn, 2018; Stroeve and Notz, 2018; Thackeray and Hall, 2019; SIMIP Community, 2020), and that perennially ice-free scenarios could follow closely behind (e.g., Bathiany et al., 2016). Such scenarios could contribute to a set of environmental conditions not observed since the Eocene and Pliocene (Burke et al., 2018).
In this uncertain future, many questions arise as to the stability of a new paradigm and its impact on the regionality and seasonality of FW in the upper AO. Questions about regional impacts include impacts of a redistribution of carbon and nutrients within the RCD and shelf seas, for example, without the restriction of the rigid-ice zone separating landfast and pack ice, would more nutrients and carbon be permitted to spread across shelf seas in winter, fueling an earlier PP pulse in spring? Would heterotrophic processes increase as more terrestrial organic material (and more sediment export) is available to be broken down? Within the Atlantic inflow and interior shelves and EB, were “atlantification” dominates (Polyakov et al., 2020), questions may surround changing productivity in a less stratified and stronger mixing environment, for example, could new hot spots of productivity emerge if upwelling is enhanced, bringing more nutrients to the surface in some shelf regions? Within the sea ice stronghold of the Beaufort Gyre of the AB, questions surrounding the persistence of stratification would dominate, for example, would the Beaufort Gyre intensify without the damping of its sea ice lid, retaining more FW within the gyre and further stratifying (suppressing nutrients inputs to the ML)? or will it relax, releasing more FW out of the AO, and reducing overall stratification in the AB (redeeming nutrient inputs to the ML)? And ultimately, questions surrounding communication of FW between the deep basins would arise, for example, would more FW get imported into the AB from the EB without sea ice or would the lack of ice export via the transpolar drift effectively cut off this component of FW exchanges between the two basins?
While the impacts of an absent freeze-melt cycle would manifest in the physical, geochemical, and biological processes across all AO hydromorphological domains (Figure 6), seasonal impacts are particularly uncertain for geochemical and biological systems. For example, over the sunlit spring-summer, would light limitation cease as long as insolation conditions are met? Or would the persistent salt-stratifying layer be so thick that it would physically separate phytoplankton from access to light by deepening the nutricline out of the summer photic zone, rendering these basins unproductive and heterotrophic? What is the consequence of altering fall and winter convective processes associated with sea ice formation on the shelf seas, is stirring from wind mixing and cooling sufficient to penetrate into the permanent FW stratification and supply nutrients to the upper waters in the absence of brine rejection? And critically, in an perennially ice-free system, what becomes of the seasonal refugia for sea ice associated species?
Recent Arctic Ocean change has been swift and future trajectories remain uncertain. A challenge remains to reconcile the pace of change with observable system descriptors; here, recognition of identifiable mileposts is useful, as we can look back at those we have already passed to gauge where we are going. For example, in 2012, the September sea ice minimum dropped to half of the historical average (1979–1990; National Snow and Ice Data Center, 2020) exposing much of the pan-Arctic shelf to wind forcing, enhancing shelf-break upwelling, and adding an excess seasonal FW load greater than that of annual river inputs, if the ice was, on average, 1.4 m thick (e.g., Kwok and Rothrock, 2009). The relatively long observational record from the Beaufort Gyre (BG) in the Amerasian Basin shows that surface ocean geochemistry, regional FW storage, and biological community composition have also passed key mileposts. In 2007, this region was the first deep basin to reach undersaturated conditions for aragonite at the surface, a condition that has persisted since (Zhang et al., 2020). In 2008, the FW content of the BG plateaued, after increasing steadily over the previous five years, and has risen only modestly to its peak in 2016 (Krishfield et al., 2014; Proshutinsky et al., 2019). As BG FW content rose over this early period (2003–2008), the abundance of picoplankton relative to nanoplankton in the upper ocean increased (Li et al., 2009), as smaller cell size classes became favored in an increasingly stratified and nutrient-depleted surface ocean, a transition with significant consequences for the biological pump. In subsequent years (2009–2012), as the trend toward increasing stratification and decreasing nutrient flux in the BG flattened, this index of community composition dropped and instead displayed greater interannual variability (Li et al., 2013), longer time series will bear out if these observations characterize a new steady state. As with these past mileposts, we anticipate future markers to emerge, frequently without warning, and ignoring the usual boundaries of regional domains and seasonal patterns. For example, one may anticipate inflow shelves that flicker toward seasonally, then perennially ice-free states; the arrival of peak river discharge to interior shelves months or more earlier; and the complete loss of ice cover from the central Arctic basins, which would then no longer export sea ice to the North Atlantic and sites of deep water formation. Such changes have the potential to completely transform AO ecosystems in their wake (e.g., Moore et al., 2018; Huntington et al., 2020).
In summary, the Arctic Ocean presents as vast and intractably complex; with a global influence not really warranted by its relatively small volume. The pace of change of the Anthropocene adds further challenge to deep understanding, and inherent non-linearity fully guarantees that the system will confound and surprise (Holling, 1973). We struggle to incorporate interdisciplinary science, but, as put by Beer (1980), “Interdisciplinary science often consists of individual disciplinarians standing in a circle, holding hands for comfort, while the problem of interest slips through the middle.” Faced with this challenge, we have attempted here to address the intractable by providing a unifying constraint based on freshwater, by defining regional domains based on a small but distinct number of hydro-geomorphological properties, and by recognizing that as much as any place on Earth, seasonality and its baggage of phenology governs process and network interactions. Within this more holistic, systems-level framework, we assert that rigorous reductionist experiments can be proposed and carried out, that the dots on our Arctic map will be connected by roads and rivers, and that perhaps we disciplinarians will more clearly see the problems of interest.
Data Availability Statement
Publicly available datasets were analyzed in this study. These data are available from Codispoti et al. (2011), NODC Accession 0072133.
Author Contributions
EC conceived of the initial idea. All authors participated in writing the manuscript.
Funding
This paper was based on ideas prepared for and discussed at an international workshop on pan-Arctic marine systems in Motovun Croatia, organized by P. Wassmann and supported by funding from Arctic SIZE (http://site.uit.no/arcticsize/). KB was supported with funding from Fisheries and Oceans Canada and the Natural Sciences and Engineering Research Council of Canada. JH was supported by the European Commission H2020 programme under the Marie Skłodowska-Curie Actions (GrIS-Melt: grant no. 752325) and the H2020 project INTAROS (grant no. 727890).
Conflict of Interest
The authors declare that the research was conducted in the absence of any commercial or financial relationships that could be construed as a potential conflict of interest.
Acknowledgments
We would like to thank Paul Wassmann and all the participants of the pan-Arctic marine system workshop in Motovun Croatia for invigorating and insightful discussions that helped to crystalize the ideas presented in this manuscript. We would also like to thank Patricia Kimber for turning the ideas of this paper into visual art. We thank Knut Aagaard for his reading of the manuscript, and his suggestion that we recognize mileposts in the progression of change. We also thank the two reviewers for their constructive comments which helped to improve the manuscript.
References
Aagaard, K., and Carmack, E. C. (1989). The role of sea ice and other fresh water in the Arctic circulation. J. Geophys. Res. 94, 14485–14498. doi: 10.1029/JC094iC10p14485
Aagaard, K., and Carmack, E. C. (1994). “The Arctic Ocean and climate: a perspective,” in The Polar Oceans and Their Role in Shaping the Global Environment, Geophysical Monograph 85, eds J. Johannessen, R. D. Muench, J. E. Overland (Washington, DC: American Geophysical Union), 4–20.
Aagaard, K., Coachman, L. K., and Carmack, E. C. (1981). On the pycnocline of the Arctic Ocean. Deep Sea Res. 28, 529–545.
Ahmed, R., Prowse, T., Dibike, Y., Bonsal, B., and O’Neil, H. (2020). Recent trends in freshwater influx to the arctic ocean from four major Arctic-Draining Rivers. Water 12:1189. doi: 10.3390/w12041189
Alkire, M. B., Morison, J., and Andersen, R. (2015). Variability in the meteoric water, sea-ice melt, and Pacific water contributions to the central Arctic Ocean, 2000–2014. J. Geophys. Res. Oceans 120, 1573–1598. doi: 10.1002/2014JC010023
Alkire, M. B., Morison, J., Schweiger, A., Zhang, J., Steele, M., Peralta-Ferriz, C., et al. (2017). A meteoric water budget for the Arctic Ocean. J. Geophys. Res. Oceans 122, 10020–10041. doi: 10.1002/2017JC012807
Alkire, M. B., Rember, R., and Polyakov, I. (2019). Discrepancy in the identification of the Atlantic/Pacific front in the central Arctic Ocean: NO versus nutrient relationships. Geophys. Res. Lett. 46, 3843–3852. doi: 10.1029/2018gl081837
AMAP (2017). Snow, Water, Ice and Permafrost in the Arctic (SWIPA) 2017. Oslo: Arctic Monitoring and Assessment Programme (AMAP), 269.
AMAP (2018). AMAP Assessment 2018: Arctic Ocean Acidification. Tromsø, Norway: Arctic Monitoring and Assessment Programme (AMAP), 187.
Ardyna, M., Babin, M., Gosselin, M., Devred, E., Rainville, L., and Tremblay, J.-É. (2014). Recent Arctic Ocean sea ice loss triggers novel fall phytoplankton blooms. Geophys. Res. Lett. 41, 6207–6212. doi: 10.1002/2014GL061047
Ardyna, M., Gosselin, M., Michel, C., Poulin, M., and Tremblay, J.-É. (2011). Environmental forcing of phytoplankton community structure and function in the Canadian High Arctic: contrasting oligotrophic and eutrophic regions. Mar. Ecol. Prog. Ser. 442, 37–57. doi: 10.3354/meps09378
Arendt, K. E., Dutz, J., Jonasdottir, S. H., Jung-Madsen, J., Friis Moller, E., and Nielsen, T. G. (2011). Effects of suspended sediments on copepods feeding in a glacial influenced sub-Arctic fjord. J. Plankton Res. 33, 1526–1537. doi: 10.1093/plankt/fbr054
Arrigo, K. R., van Dijken, G., and Pabi, S. (2008). Impact of a shrinking Arctic ice cover on marine primary production. Geophys. Res. Lett. 35:L19603.
Arrigo, K. R., and van Dijken, G. L. (2015). Continued increases in Arctic Ocean primary production. Prog. Oceanogr. 136, 60–70. doi: 10.1016/j.pocean.2015.05.002
Azetsu-Scott, K., Petrie, B., Yeats, P., and Lee, C. (2012). Composition and fluxes of freshwater through Davis Strait using multiple chemical tracers. J. Geophys. Res. 117:C12011. doi: 10.1029/2012JC008172
Bacon, S., Aksenov, Y., Fawcett, S., and Madec, G. (2015). Arctic mass, freshwater and heat fluxes: methods and modelled seasonal variability. Philos. Trans. R. Soc. A 373:20140169. doi: 10.1098/rsta.2014.0169
Barnhart, K. R., Overeem, I., and Anderson, R. S. (2014). The effect of changing sea ice on the physical vulnerability of Arctic coasts. Cryosphere 8, 1777–1799. doi: 10.5194/tc-8-1777-2014
Bates, N. R., and Mathis, J. T. (2009). The Arctic Ocean marine carbon cycle: evaluation of air-sea CO2 exchanges, ocean acidification impacts and potential feedbacks. Biogeosciences 6, 2433–2459. doi: 10.5194/bg-6-2433-2009
Bates, N. R., Moran, S. B., Hansell, D. A., and Mathis, J. T. (2006). An increasing CO2 sink in the Arctic Ocean due to sea-ice loss. Geophys. Res. Lett. 33:L23609. doi: 10.1029/2006GL027028
Bathiany, S., Notz, D., Mauritsen, T., Raedel, G., and Brovkin, V. (2016). On the potential for abrupt Arctic winter sea ice loss. J. Climate 29, 2703–2719. doi: 10.1175/jcli-d-15-0466.1
Beer, S. (1980). “Introduction to autopoiesis,” in Autopoirsis and Cognition: The Realization of the Living, eds H. R. Maturana and F. J. Varela (Boston, MA: Reidel), 63–72.
Bergeron, M., and Tremblay, J.-É. (2014). Shifts in biological productivity inferred from nutrient drawdown in the southern Beaufort Sea (2003-2011) and the northern Baffin Bay (1997-2011), Canadian Arctic. Geophys. Res. Lett. 41, 3979–3987. doi: 10.1002/2014gl059649
Bhatia, M. P., Kujawinski, E. B., Das, S. B., Breier, C. F., Henderson, P. B., and Charette, M. A. (2013). Greenland meltwater as a significant and potentially bioavailable source of iron to the ocean. Nat. Geosci. 6, 274–278. doi: 10.1038/ngeo1746
Bintanja, R., and Selten, F. M. (2014). Future increases in Arctic precipitation linked to local evaporation and sea-ice retreat. Nature 509, 479–482. doi: 10.1038/nature13259
Bluhm, B. A., Kosobokoba, K. N., and Carmack, E. C. (2015). A tale of two basins: an integrated physical and biological perspective of the deep Arctic Ocean. Prog. Oceanogr. 139, 89–121. doi: 10.1016/j.pocean.2015.07.011
Box, J. E., Colgan, W. T., Christensen, T. R., Schmidt, N. M., Lund, M., Parmentier, F.-J., et al. (2019). Key indicators of Arctic climate change: 1971–2017. Environ. Res. Lett. 14:045010. doi: 10.1088/1748-9326/aafc1b
Bring, A., Fedorova, I., Dibike, Y., Hinzman, L., Mård, J., Mernild, S. H., et al. (2016). Arctic terrestrial hydrology: a synthesis of processes, regional effects, and research challenges. J. Geophys. Res. Biogeosci. 121, 621–649. doi: 10.1002/2015JG003131
Bring, A., Shiklomanov, A., and Lammers, R. B. (2017). Pan-Arctic river discharge: Prioritizing monitoring of future. Earths Future 5, 72–92. doi: 10.1002/2016EF000434
Brown, K. A., Miller, L. A., Mundy, C. J., Papakyriakou, T., Francois, R., Gosselin, R., et al. (2015). Inorganic carbon system dynamics in landfast Arctic sea ice during the early-melt period. J. Geophys. Res. Oceans 120, 3542–3566. doi: 10.1002/2014JC010620
Brown, Z. W., Casciotti, K. L., Pickart, R. S., Swift, J. H., and Arrigo, K. R. (2015). Aspects of the marine nitrogen cycle of the Chukchi Sea and Canada Basin. Deep Sea Res. II 118, 73–87. doi: 10.1016/j.dsr2.2015.02.009
Burke, K. D., Williams, J. W., Chandler, M. A., Haywood, A. M., Lunt, D. J., and Otto-Bliesner, B. L. (2018). Pliocene and Eocene provide best analogs for near-future climates. Proc. Natl. Acad. Sci. U.S.A. 115, 13288–13293. doi: 10.1073/pnas.1809600115
Cai, W.-J., Chen, L., Chen, B., Gao, Z., Lee, S. H., Chen, J., et al. (2010). Decrease in the CO2 uptake capacity in an ice-free Arctic Ocean basin. Science 329, 556–559. doi: 10.1126/science.1189338
Carmack, E. C. (2000). “The Arctic Ocean’s Freshwater Budget: sources, storage and export,” in The Freshwater Budget of the Arctic Ocean, eds E. L. Lewis, E. P. Jones, P. Lemke, T. D. Prowse, and P. Wadhams (Netherlands: Kluwer Academic Publishers), 91–126.
Carmack, E. C. (2007). The alpha/beta ocean distinction: a perspective on freshwater fluxes, convection, nutrients and productivity in high-latitude seas. Deep Sea Res. Part II Top. Stud. Oceanogr. 54, 2578–2598. doi: 10.1016/j.dsr2.2007.08.018
Carmack, E. C., and McLaughlin, F. A. (2011). Towards Recognition of physical and geochemical change in subarctic and arctic seas. Prog. Oceanogr. 90, 90–104. doi: 10.1016/j.pocean.2011.02.007
Carmack, E. C., McLaughlin, F. A., Yamamoto-Kawai, M., Itoh, M., Shimada, K., Krishfield, R., et al. (2008). “Freshwater storage in the Northern Ocean and the special role of the Beaufort Gyre,” in Arctic–Subarctic Ocean Fluxes, Defining the Role of the Northern Seas in Climate, eds R. R. Dickson, J. Meincke, and P. Phines (Dordrecht: Springer), 145–169. doi: 10.1007/978-1-4020-6774-7_8
Carmack, E. C., and Wassmann, P. (2006). Food webs and physical–biological coupling on pan-Arctic shelves: Unifying concepts and comprehensive perspectives. Prog. Oceanogr. 71, 446–477. doi: 10.1016/j.pocean.2006.10.004
Carmack, E., Polyakov, I., Padman, L., Fer, I., Hunke, E., Hutchings, J., et al. (2015a). Towards quantifying the increasing role of oceanic heat flux in sea ice loss in the new Arctic. Bull. Am. Meteorol. Soc. 96, 2079–2105. doi: 10.1175/BAMS-D-13-00177.1
Carmack, E. C., Winsor, P., and Williams, W. (2015b). The contiguous panarctic Riverine Coastal Domain: a unifying concept. Prog. Oceanogr. 139, 13–23. doi: 10.1016/j.pocean.2015.07.014
Carmack, E. C., Yamamoto-Kawai, M., Haine, T. W. N., Bacon, S., Bluhm, B. A., Lique, C., et al. (2015c). Freshwater and its role in the Arctic Marine System: sources, disposition, storage, export, and physical and biogeochemical consequences in the Arctic and global oceans. J. Geophys. Res. Biogeosci. 121, 675–717. doi: 10.1002/2015JG003140
Carton, J. A., Ding, Y., and Arrigo, K. R. (2015). The seasonal cycle of the Arctic Ocean under climate change. Geophys. Res. Lett. 42, 7681–7686. doi: 10.1002/2015GL064514
Chierici, M., and Fransson, A. (2009). Calcium carbonate saturation in the surface water of the Arctic Ocean: undersaturation in freshwater influenced shelves. Biogeosciences 6, 2421–2432.
Codispoti, L. A., Kelly, V., Thessen, A., Matrai, P., Suttles, S., Hill, V., et al. (2013). Synthesis of primary production in the Arctic Ocean: III. Nitrate and phosphate based estimates of net community production. Prog. Oceanogr. 110, 126–150. doi: 10.1016/j.pocean.2012.11.006
Codispoti, L. A., and the University of Maryland Center for Environmental Science Horn Point Laboratory (2011). Product Database Composed of Physical and Nutrient Profile Data Collected in the Arctic Ocean and Adjacent Seas from 1928 to 2008 (NODC Accession 0072133). Version 1.1. National Oceanographic Data Center, NOAA, Dataset. (accessed November 10, 2017).
Coello-Camba, A., Agustí, S., Vaque, D., Holding, J. M., Arrieta, J. M., Wassmann, P., et al. (2014a). Experimental assessment of temperature thresholds for Arctic Phytoplankton communities. Estuaries Coasts 38, 873–885. doi: 10.1007/s12237-014-9849-7
Coello-Camba, A., Agusti, S., Holding, J., Arrieta, J. M., and Duarte, C. M. (2014b). Interactive effect of temperature and CO2 increase in Arctic phytoplankton. Front. Mar. Sci. 1:49. doi: 10.3389/fmars.2014.00049
Cooper, L. W., McClelland, J. W., Holmes, R. M., Raymond, P. A., Gibson, J. J., Guay, K., et al. (2008). Flow-weighted values of runoff tracers (δ18O, DOC, Ba, alkalinity) from the six largest Arctic rivers. Geophys. Res. Lett. 35:L18606. doi: 10.1029/2008GL035007
Cooper, L. W., Whitledge, T. E., Grebmeier, J. M., and Weingartner, T. (1997). The nutrient, salinity, and stable oxygen isotope composition of Bering and Chukchi Seas waters in and near the Bering Strait. J. Geophys. Res. 102, 12563–12573. doi: 10.1029/97jc00015
Coupel, P., Jin, H. Y., Joo, M., Horner, R., Bouvet, H. A., Sicre, M. A., et al. (2012). Phytoplankton distribution in unusually low sea ice cover over the Pacific Arctic. Biogeosciences 9, 4835–4850. doi: 10.5194/bg-9-4835-2012
Coupel, P., Ruiz-Pino, D., Sicre, M. A., Chen, J. F., Lee, S. H., Schiffrine, N., et al. (2015). The impact of freshening on phytoplankton production in the Pacific Arctic Ocean. Prog. Oceanogr. 131, 113–125. doi: 10.1016/j.pocean.2014.12.003
Danielson, S. L., Ahkinga, O., Ashjian, C., Basyuk, E., Cooper, L. E., Eisner, L., et al. (2020). Manifestation and consequences of warming and altered heat fluxes over the Bering and Chukchi Sea continental shelves. Deep Sea Res. II. doi: 10.1016/j.dsr2.2020.104781
Dean, K. G., Stringer, W. J., Ahlnas, K., Searcy, C., and Weingartner, T. (1994). The influence of river discharge on the thawing of sea ice, Mackenzie River Delta: albedo and temperature analyses. Polar Res. 13, 83–94. doi: 10.3402/polar.v13i1.6683
Dodd, P. A., Rabe, B., Hansen, E., Falck, E., Mackensen, A., Rohling, E., et al. (2012). The freshwater composition of the Fram Strait outflow derived from a decade of tracer measurements. J. Geophys. Res. 117:C11005. doi: 10.1029/2012JC008011
Drost, H. E., Lo, M., Carmack, E. C., and Farrell, A. P. (2016). Acclimation potential of Arctic cod (Boreogadus saida) from the rapidly warming Arctic Ocean. J. Exp. Biol. 219, 3114–3125. doi: 10.1242/jeb.140194
Dumas, J., Carmack, E. C., and Melling, H. (2005). Climate change impacts on the Beaufort shelf landfast ice. Cold Reg. Sci. Tech. 42, 41–51. doi: 10.1016/j.coldregions.2004.12.001
Dutkiewicz, S., Morris, J. J., Follows, M. J., Scott, J., Levitan, O., Dyhrman, S. T., et al. (2015). Impact of ocean acidification on the structure of future phytoplankton communities. Nat. Clim. Change 5, 1002–1006. doi: 10.1038/nclimate2722
Eicken, H., Gradinger, R., Gaylord, A., Mahoney, A., Rigor, I., and Melling, H. (2005). Sediment transport by sea ice in the Chukchi and Beaufort Seas: Increasing importance due to changing ice conditions? Deep-Sea Res. II 52, 3281–3302. doi: 10.1016/j.dsr2.2005.10.006
Else, B. G. T., Galley, R. J., Lansard, B., Barber, D. G., Brown, K., Miller, L. A., et al. (2013). Further observations of a decreasing atmospheric CO2 uptake capacity in the Canada Basin (Arctic Ocean) due to sea ice loss. Geophys. Res. Lett. 40, 1132–1137. doi: 10.1002/grl.50268
Engel, A., Borchard, C., Piontek, J., Schulz, K. G., Riebesell, U., and Bellerby, R. (2013). CO2 increases 14C primary production in an Arctic plankton community. Biogeosciences 10, 1291–1308. doi: 10.5194/bg-10-1291-2013
Fabry, V. J., McClintock, J. B., Mathis, J. T., and Grebmeier, J. (2009). Ocean acidification at high latitudes: the bellwether. Oceanography 22, 160–171. doi: 10.5670/oceanog.2009.105
Falkenberg, L. J., Jelmert, A., Mark, F. C., Rost, B., Schulz, K. G., and Thor, P. (2018). “Biological responses to ocean acidification,” in Proceedings of the AMAP Assessment 2018: Arctic Ocean Acidification, (Tromsø: Arctic Monitoring and Assessment Programme (AMAP)), 15–40.
Farmer, D. M. (1975). Penetrative convection in the absence of mean shear. Quart. J. R.. Meteorol. Soc. 101, 869–891. doi: 10.1002/qj.49710143011
Feely, R. A., Sabine, C. L., Lee, K., Berelson, W., Kleypas, J., Fabry, V. J., et al. (2004). Impact of anthropogenic CO2 on the CaCO3 system in the oceans. Science 305, 362–366. doi: 10.1126/science.1097329
Fransson, A., Chierici, M., Nomura, D., Granskog, M., Kristiansen, S., Martma, T., et al. (2015). Effect of glacial drainage water on the CO2 system and ocean acidification state in an Arctic tidewater-glacier fjord during two contrasting years. J. Geophys. Res. Oceans 120, 2413–2429. doi: 10.1002/2014jc010320
Frey, K. E., Perovich, D. K., and Light, B. (2011). The spatial distribution of solar radiation under a melting Arctic sea ice cover. Geophys. Res. Let. 38:L22501. doi: 10.1029/2011GL049421
Friis Møller, E., and Nielsen, T. G. (2019). Borealization of Arctic zooplankton—smaller and less fat zooplankton species in Disko Bay, Western Greenland. Limnol. Oceanog. 65, 1175–1188. doi: 10.1002/lno.11380
Fujiwara, A., Hirawake, T., Suzuki, K., Imai, I., and Saitoh, S. I. (2014). Timing of sea ice retreat can alter phytoplankton community structure in the western Arctic Ocean. Biogeosciences 11, 1705–1716. doi: 10.5194/bg-11-1705-2014
Gelfan, A., Gustafsson, D., Motovilov, Y., Arheimer, B., Kalugin, A., Krlenko, I., et al. (2017). Climate change impact on the water regime of two great Arctic rivers: modeling and uncertainty issues. Clim. Change 141, 499–515. doi: 10.1007/s10584-016-1710-5
Granger, J., Sigman, D. M., Gagnon, J., Tremblay, J. É., and Mucci, A. (2018). On the properties of the Arctic halocline and deep water masses of the Canada Basin from nitrate isotope ratios. J. Geophys. Res. Oceans 123, 5443–5458. doi: 10.1029/2018JC014110
Grebmeier, J. M., Moore, S. E., Overland, J. E., Frey, K. E., and Gradinger, R. (2010). Biological Response to Recent Pacific Arctic Sea Ice Retreats. EOS Trans. Am. Geophys. Union 91, 161–168.
Haine, T. W. N., Curry, B., Gerdes, R., Hansen, E., Karcher, M., Lee, C., et al. (2015). Arctic freshwater export: status, mechanisms, and prospects. Glob. Planet. Change 125, 13–35. doi: 10.1016/j.gloplacha.2014.11.013
Halbach, L., Vihtakari, M., Duarte, P., Everett, A., Granskog, M. A., Hop, H., et al. (2019). Tidewater glaciers and bedrock characteristics control the phytoplankton growth environment in a Fjord in the Arctic. Front. Mar. Sci. 6:254. doi: 10.3389/fmars.2019.00254
Hein, M., and Sand-Jensen, K. (1997). CO2 increases oceanic primary production. Nature 388, 526–527. doi: 10.1038/41457
Hendriks, I. E., Duarte, C. M., and Álvarez, M. (2010). Vulnerability of marine biodiversity to ocean acidification: a meta-analysis. Estuar. Coast. Shelf Sci. 86, 157–164. doi: 10.1016/j.ecss.2009.11.022
Holding, J. M., Duarte, C. M., Arrieta, J., Vaquer-Sunyer, R., Coello-Camba, A., Wassmann, P., et al. (2013). Experimentally determined temperature thresholds for Arctic plankton community metabolism. Biogeosciences 10, 357–370. doi: 10.5194/bg-10-357-2013
Holding, J. M., Duarte, C. M., Sanz-Martín, M., Mesa, E., Arrieta, J. M., Chierici, M., et al. (2015). Temperature dependence of CO2-enhanced primary production in the European Arctic Ocean. Nat. Clim. Chang. 5, 1079–1082. doi: 10.1038/nclimate2768
Holding, J. M., Markager, S., Juul-Pedersen, T., Paulsen, M. L., Møller, E. F., Meire, L., et al. (2019). Seasonal and spatial patterns of primary production in a high-latitude fjord affected by Greenland Ice Sheet run-off. Biogeosciences 16, 3777–3792. doi: 10.5194/bg-16-3777-2019
Holland, M. M., Finnis, J., Barrett, A. P., and Serreze, M. C. (2007). Projected changes in Arctic Ocean freshwater budgets. J. Geophys. Res. 112:G04S55. doi: 10.1029/2006JG000354
Holling, C. S. (1973). Resilience and the stability of ecological systems. Annu. Rev. Ecol. Syst. 4, 1–23. doi: 10.1146/annurev.es.04.110173.000245
Holmes, R. M., Coe, M. T., Fiske, G. J., Gurtovaya, T., McClelland, J. W., Shiklomanov, A. I., et al. (2013). “Climate change impacts on the hydrology and biogeochemistry of Arctic Rivers,” in Climatic Change and Global Warming of Inland Waters: Impacts and Mitigation for Ecosystems and Societies (First, eds C. R. Goldman, M. Kumagai, and R. D. Robarts (Hoboken, NJ: John Wiley & Sons), 1–26. doi: 10.1002/9781118470596.ch1
Holmes, R. M., McClelland, J. W., Peterson, B. J., Shiklomanov, I. A., Shiklomanov, A. I., Zhulidov, A. V., et al. (2002). A circumpolar perspective on fluvial sediment flux to the Arctic Ocean. Glob. Biogeochem. Cycles 16:1098. doi: 10.1029/2001GB001849
Holmes, R. M., McClelland, J. W., Peterson, B. J., Tank, S. E., Bulygina, E., Eglinton, T. I., et al. (2012). Seasonal and annual fluxes of nutrients and organic matter from large rivers to the Arctic Ocean and Surrounding Seas. Estuaries Coasts 35, 369–382. doi: 10.1007/s12237-011-9386-6
Holmes, R. M., Shiklomanov, A. I., Suslova, A., Tretiakov, M., McClelland, J. W., Spencer, R. G. M., et al. (2018). River Discharge [in Arctic Report Card 2018]. Available online at: https://arctic.noaa.gov/Report-Card/Report-Card-2018/ArtMID/7878/ArticleID/786/River-Discharge (accessed December 1, 2019).
Holmes, R. M., Shiklomanov, A. I., Tank, S. E., McCelland, J. W., and Tretiakov, M. (2015). River Discharge [in Arctic Report Card 2015]. Available online at: https://www.arctic.noaa.gov/Report-Card (accessed June 5, 2018).
Hood, E., Battin, T. J., Fellman, J., O’Neel, S., and Spencer, R. G. M. (2015). Storage and release of organic carbon from glaciers and ice sheets. Nat. Geosci. 8, 91–96. doi: 10.1038/ngeo2331
Hood, E., and Scott, D. (2008). Riverine organic matter and nutrients in southeast Alaska affected by glacial coverage. Nat. Geosci. 1, 583–587. doi: 10.1038/ngeo280
Hoppe, C. J. M., Wolf, K. K. E., Schuback, N., Tortell, P. D., and Rost, B. (2018). Compensation of ocean acidification effects in Arctic phytoplankton assemblages. Nat. Clim. Chang. 8, 529–533. doi: 10.1038/s41558-018-0142-9
Hopwood, M. J., Carroll, D., Dunse, T., Hodson, A., Holding, J. M., Iriarte, J. L., et al. (2020). How does glacier discharge affect marine biogeochemistry and primary production in the Arctic? Cryosphere 14, 1347–1383. doi: 10.5194/tc-14-1347-2020
Hopwood, M. J., Carroll, D., Browning, T. J., Meire, L., Mortensen, J., Krisch, S., et al. (2018). Non-linear response of summertime marine productivity to increased meltwater discharge around Greenland. Nat. Commun. 9:3256. doi: 10.1038/s41467-018-05488-8
Huang, Y., Dong, X., Bailey, D. A., Holland, M. M., Xi, B., DuVivier, A. K., et al. (2019). Thicker clouds and accelerated Arctic Sea ice decline: the atmosphere sea ice interactions in spring. Geophys. Res. Lett. 46, 6980–6989. doi: 10.1029/2019GL082791
Huntington, H. P., Danielson, S. L., Wiese, F. K., Baker, M., Boveng, P., Citta, J. J., et al. (2020). Evidence suggests potential transformation of the Pacific Arctic ecosystem is underway. Nat. Clim. Change 10, 342–348. doi: 10.1038/s41558-020-0695-2
Hutchison, Z. L., Hendrick, V. J., Burrows, M. T., Wilson, B., and Last, K. S. (2016). Buried Alive: the Behavioural Response of the Mussels, Modiolus modiolus and Mytilus edulis to Sudden Burial by Sediment A. Davies [ed.]. PLoS One 11:e0151471. doi: 10.1371/journal.pone.0151471
Jackson, J. M., Carmack, E. C., McLaughlin, F. A., Allen, S. E., and Ingram, R. G. (2010). Identification, characterization and change of the nearsurface temperature maximum in the Canada Basin, 1993–2008. J. Geophys. Res. 115:C05021. doi: 10.1029/2009JC005265
Jahn, A. (2018). Reduced probability of ice-free summers for 1.5°C compared to 2°C warming. Nat. Clim. Change 8, 409–413. doi: 10.1038/s41558-018-0127-8
Ji, R., Jin, M., and Varpe, Ø. (2013). Sea ice phenology and timing of primary production pulses in the Arctic Ocean. Glob. Change Biol. 19, 734–741. doi: 10.1111/gcb.12074
Jones, E. P., Anderson, L. G., Jutterstrom, S., Mintrop, L., and Swift, J. H. (2008). Pacific freshwater, river water and sea ice meltwater across Arctic Ocean basins: Results from the 2005 Beringia Expedition. J. Geophys. Res. 113:C08012. doi: 10.1029/2007JC004124
Jutterström, S., and Anderson, L. (2010). Uptake of CO2 by the Arctic Ocean in a changing climate. Mar. Chem. 122, 96–104. doi: 10.1016/j.marchem.2010.07.002
Juul-Pedersen, T., Arendt, K. E., Mortensen, J., Blicher, M. E., Søgaard, D. H., and Rysgaard, S. (2015). Seasonal and interannual phytoplankton production in a sub-Arctic tidewater outlet glacier fjord, SW Greenland. Mar. Ecol. Prog. Ser. 524, 27–38. doi: 10.3354/meps11174
Kahru, M., Brotas, V., Manzano-Sarabia, M., and Mitchell, B. G. (2011). Are phytoplankton blooms occurring earlier in the Arctic? Global Change Bio. 17, 1733–1739. doi: 10.1111/j.1365-2486.2010.02312.x
Kattsov, V. M., Walsh, J. E., Chapman, W. L., Govorkova, V. A., Pavlova, T. V., and Zhang, X. (2007). Simulation and Projection of Arctic Freshwater Budget Components by the IPCC AR4 Global Climate Models. J. Hydrometeorol. 8, 571–589. doi: 10.1175/jhm575.1
Krishfield, R. A., Proshutinsky, A., Tateyama, K., Williams, W. J., Carmack, E. C., McLaughlin, F. A., et al. (2014). Deterioration of perennial sea ice in the Beaufort Gyre from 2003 to 2012 and impact on the oceanic freshwater cycle. J. Geophys. Res. 119, 1271–1305. doi: 10.1002/2013JC008999
Krumpen, T., Belter, H. J., Boetius, A., Damm, E., Haas, C., Hendricks, S., et al. (2019). Arctic warming interrupts the Transpolar Drift and affects long-range transport of sea ice and ice rafted matter. Sci. Rep. 9:5459. doi: 10.1038/s41598-019-41456-y
Kwok, R., Cunningham, G. F., Wensnahan, M., Rigor, I., Zwally, H. J., and Yi, D. (2009). Thinning and volume loss of Arctic sea ice: 2003–2008. J. Geophys. Res. 114:C07005. doi: 10.1029/2009JC005312
Kwok, R., and Rothrock, D. A. (2009). Decline in Arctic sea ice thickness from submarine and ICESat records: 1958 – 2008. Geophys. Res. Lett. 36:L15501. doi: 10.1029/2009GL039035
Lantuit, H., Overduin, P. P., and Wetterich, S. (2013). Recent progress regarding permafrost coasts, permafrost and periglac. Processes 24, 120–130. doi: 10.1002/ppp.1777
Lawson, E. C., Bhatia, M. P., Wadham, J. L., and Kujawinski, E. B. (2014). Continuous summer export of nitrogen-rich organic matter from the greenland ice sheet inferred by ultrahigh resolution mass spectrometry. Environ. Sci. Technol. 48, 14248–14257. doi: 10.1021/es501732h
Le Fouest, V., Babin, M., and Tremblay, J. É. (2013). The fate of riverine nutrients on Arctic shelves. Biogeosciences 10, 3661–3677. doi: 10.5194/bg-10-3661-2013
Le Fouest, V., Matsuoka, A., Manizza, M., Shernetsky, M., Tremblay, B., and Babin, M. (2018). Towards an assessment of riverine dissolved organic carbon in surface waters of the western Arctic Ocean based on remote sensing and biogeochemical modeling. Biogeosciences 15, 1335–1346. doi: 10.5194/bg-15-1335-2018
Le Fouest, V., Manizza, M., Tremblay, B., and Babin, M. (2015). Modelling the impact of riverine DON removal by marine bacterioplankton on primary production in the Arctic Ocean. Biogeosciences 12, 3385–3402. doi: 10.5194/bg-12-3385-2015
Lenton, T. M. (2012). Arctic climate tipping points. Ambio 41, 10–22. doi: 10.1007/s13280-011-0221-x
Li, W. K. W., Carmack, E. C., McLaughlin, F. A., Nelson, R. J., and Williams, W. J. (2013). Space-for-time substitution in predicting the state of picoplankton and nanoplankton in a changing Arctic Ocean. J. Geophys. Res. Oceans 118, 5750–5759. doi: 10.1002/jgrc.20417
Li, W. K. W., McLaughlin, F. A., Lovejoy, C., and Carmack, E. C. (2009). Smallest algae thrive as the Arctic Ocean freshens. Science 326:539. doi: 10.1126/science.1179798
Liljedahl, A. K., Gädeke, A., O’Neel, S., Gatesman, T. A., and Douglas, T. A. (2017). Glacierized headwater streams as aquifer recharge corridors, subarctic Alaska. Geophys. Res. Lett. 44, 6876–6885. doi: 10.1002/2017GL073834
Loeng, H. (1991). Features of the physical oceanographic conditions of the Barents Sea. Polar Res. 10, 5–18. doi: 10.3402/polar.v10i1.6723
Loeng, H., Brander, K., Carmack, E., Denisenko, S., Drinkwater, K., Hansen, B., et al. (2005). “Arctic Climate Change, Marine Systems, Ch. 9,” in Arctic Climate Impact Assessment, eds C. Symon, L. Arris and B. Heal (Cambridge: Cambridge University Press), 1020.
MacGilchrist, G. A., Naveira Garabato, A. C., Tsubouchi, T., Bacon, S., Torres-Valdes, S., and Azetsu-Scott, K. (2014). The Arctic Ocean Carbon sink. Deep Sea Res. I 86, 39–55. doi: 10.1016/j.dsr.2014.01.002
Manizza, M., Follows, M. J., Dutkiewicz, S., Menemenlis, D., Hill, C. N., and Key, R. M. (2013). Changes in the Arctic Ocean CO2 sink (1996–2007): a regional model analysis. Glob. Biogeochem. Cycles 27, 1108–1118. doi: 10.1002/2012GB004491
Mastrandrea, M. D., Mach, K. J., Plattner, G.-K., Edenhofer, O., Stocker, T. F., Field, C. B., et al. (2011). The IPCC AR5 guidance note on consistent treatment of uncertainties: a common approach across the working groups. Clim. Change 108:675. doi: 10.1007/s10584-011-0178-6
Mathis, J. T., Cross, J. N., and Bates, N. R. (2011). Coupling primary production and terrestrial runoff to ocean acidification and carbonate mineral suppression in the eastern Bering Sea. J. Geophys. Res. 116:C02030. doi: 10.1029/2010JC006453
Mathis, J. T., Cross, J. N., Evans, W., and Doney, S. C. (2015). Ocean acidification in the surface waters of the Pacific-Arctic boundary regions. Oceanography 28, 122–135. doi: 10.5670/oceanog.2015.36
Mathis, J. T., Pickart, R. S., Byrne, R. H., McNeil, C. L., Moore, G. W. K., Juranek, L. W., et al. (2012). Storm-induced upwelling of high pCO2 waters onto the continental shelf of the western Arctic Ocean and implications for carbonate mineral saturation states. Geophys. Res. Lett. 39:L07606. doi: 10.1029/2012GL051574
McClelland, J. W., Holmes, R. M., Dunton, K. H., and Macdonald, R. W. (2012). The Arctic Ocean Estuary. Estuaries Coasts 35, 353–368. doi: 10.1007/s12237-010-9357-3
McClelland, J. W., Holmes, R. M., Peterson, B. J., Raymond, P. A., Striegl, R. G., Zhulidov, A. V., et al. (2016). Particulate organic carbon and nitrogen export from major Arctic rivers. Glob. Biogeochem. Cycles 30, 629–643. doi: 10.1002/2015GB005351
McLaughlin, F. A., and Carmack, E. C. (2010). Nutricline deepening in the Canada Basin, 2003–2009. Geophys. Res. Lett 37:L24602. doi: 10.1029/2010GL045459
Meire, L., Meire, P., Struyf, E., Krawczyk, D. W., Arendt, K. E., Yde, J. C., et al. (2016). High export of dissoved silica from the Greenland Ice Sheet. Geophys. Res. Lett. 43, 9173–9182. doi: 10.1002/2016gl070191
Meire, L., Mortensen, J., Meire, P., Juul-Pedersen, T., Sejr, M. K., Rysgaard, S., et al. (2017). Marine-terminating glaciers sustain high productivity in Greenland fjords. Glob. Chang. Biol. 12, 5344–5357. doi: 10.1111/gcb.13801
Mernild, S. H., Mote, T. L., and Liston, G. E. (2011). Greenland ice sheet surface melt extent and trends: 1960–2010. J. Glaciol. 57, 621–628. doi: 10.3189/002214311797409712
Miller, L. A., Papakyriakou, T. N., Collins, R. E., Deming, J. W., Ehn, J. K., Macdonald, R. W., et al. (2011). Carbon dynamics in sea ice: a winter flux time series. J. Geophys. Res. 116:C02028. doi: 10.1029/2009JC006058
Moore, S. E., Stabeno, P. J., Grebmeier, J. M., and Okkonen, S. R. (2018). The Arctic Marine Pulses Model: linking annual oceanographic processes to contiguous ecological domains in the Pacific Arctic. Deep Sea Res. Part II Top. Stud. Oceanogr. 152, 8–21. doi: 10.1016/j.dsr2.2016.10.011
Murray, C., Markager, S., Stedmon, C. A., Juul-Pedersen, T., Sejr, M. K., and Bruhn, A. (2015). The influence of glacial melt water on bio-optical properties in two contrasting Greenlandic fjords. Estuar. Coast. Shelf. Sci. 163, 72–83. doi: 10.1016/j.ecss.2015.05.041
National Snow and Ice Data Center (2018). 1981-2010 Median Arctic Sea Ice Extent and Interdecile Range from the ChArtic Interactive Sea Ice Graph. Available online at: https://nsidc.org/arcticseaicenews/charctic-interactive-sea-ice-graph/ (accessed June, 2018).
National Snow and Ice Data Center (2020). Sea Ice Extent observations from 2009-2019 from the ChArtic Interactive Sea Ice Graph. Available online at: https://nsidc.org/arcticseaicenews/charctic-interactive-sea-ice-graph/ (accessed April, 2020).
Neeley, A. R., Harris, L. A., and Frey, K. E. (2018). Unraveling phytoplankton community dynamics in the Northern Chukchi Sea Under Sea-Ice-Covered and Sea-Ice-Free Conditions. Geophys. Res. Lett. 45, 7663–7671. doi: 10.1029/2018gl077684
Neukermans, G., Oziel, L., and Babin, M. (2018). Increased intrusion of warming Atlantic water leads to rapid expansion of temperate phytoplankton in the Arctic. Glob. Chang. Biol. 24, 2545–2553. doi: 10.1111/gcb.14075
Nghiem, S. V., Hall, D. K., Rigor, I. G., Li, P., and Neumann, G. (2014). Effects of Mackenzie River discharge and bathymetry on sea ice in the Beaufort Sea. Geophys. Res. Lett. 41, 873–879. doi: 10.1002/2013GL058956
Nishino, S., Kawaguchi, Y., Inoue, J., Yamamoto-Kawai, M., Aoyama, M., Harada, N., et al. (2020). Do strong winds impact water mass, nutrient, and phytoplankton distributions in the ice-free Canada Basin in the fall? J.Geophys. Res. Oceans 125:e2019JC015428. doi: 10.1029/2019JC015428
Nummelin, A., Ilicak, M., Li, C., and Smedsrud, L. H. (2016). Consequences of future increased Arctic runoff on Arctic Ocean stratification, circulation, and sea ice cover. J. Geophys. Res. Oceans 121, 617–637. doi: 10.1002/2015JC011156
Nürnberg, D., Wollenburg, I., Dethleff, D., Eicken, H., Kassens, H., Letzig, T., et al. (1994). Sediments in Arctic sea ice: implications for entrainment, transport and release. Mar. Geol. 119, 185–214. doi: 10.1016/0025-3227(94)90181-3
O’Connor, M. I., Holding, J. M., Kappel, C. V., Duarte, C. M., Brander, K., Brown, C. J., et al. (2015). Strengthening confidence in climate change impact science. Glob. Ecol. Biogeogr. 24, 64–76. doi: 10.1111/geb.12218
Oshima, K., and Yamazaki, K. (2017). Atmospheric hydrological cycles in the Arctic and Antarctic during the past four decades. Czech Polar Rep. 7, 169–180. doi: 10.5817/CPR2017-2-17
Overeem, I., Anderson, R. S., Wobus, C. W., Clow, G. D., Urban, F. E., and Matell, N. (2011). Sea ice loss enhances wave action at the Arctic coast. Geophys. Res. Lett. 38:L17503. doi: 10.1029/2011GL048681
Overeem, I., Hudson, B. D., Syvitski, J. P. M., Mikkelsen, A. B., Hasholt, B., van den Broeke, M. R., et al. (2017). Substantial export of suspended sediment to the global oceans from glacial erosion in Greenland. Nat. Geosci. 1, 859–863. doi: 10.1038/ngeo3046
Overeem, I., and Syvitski, J. P. M. (2010). Shifting discharge peaks in Arctic rivers, 1977–2007. Geografiska Ann. Ser. A Phys. Ann. Geogr. 92, 285–296. doi: 10.1111/j.1468-0459.2010.00395.x
Oziel, L., Baudena, A., Ardyna, M., Massicotte, P., Randelhoff, A., Sallee, J.-B., et al. (2020). Faster Atlantic currents drive poleward expansion of temperate phytoplankton in the Arctic Ocean. Nat. Commun. 11:1705. doi: 10.1038/s41467-020-15485-5
Pabi, S., van Dijken, G. L., and Arrigo, K. R. (2008). Primary production in the Arctic Ocean, 1998 – 2006. J. Geophys. Res. 113, 1–22. doi: 10.1029/2007JC004578
Paulsen, M. L., Nielsen, S. E. B., Muller, O., Friis Møller, E., Stedmon, C. A., Juul-Pedersen, T., et al. (2017). Carbon bioavailability in a high Arctic fjord influenced by glacial meltwater, NE Greenland. Front. Mar. Sci. 4:176. doi: 10.3389/fmars.2017.00176
Paulson, M. L., and Robson, B. A. (2019). “Glaciers and Land-to-Ocean Flux of Carbon,” in Encyclopedia of Water: Science, Technology, and Society, ed. P. A. Maurice (Hoboken, NJ: John Wiley & Sons, Inc).
Pemberton, P., and Nilsson, J. (2015). The response of the central Arctic Ocean stratification to freshwater perturbations. J. Geophys. Res. Oceans 121, 792–817. doi: 10.1002/2015JC011003
Peralta-Ferriz, C., and Woodgate, R. A. (2015). Seasonal and interannual variability of pan-Arctic surface mixed layer properties from 1979 to 2012 from hydrographic data, and the dominance of stratification for multiyear mixed layer depth shoaling. Prog. Oceanogr. 134, 19–53. doi: 10.1016/j.pocean.2014.12.005
Peterson, B. J., Holmes, R. M., McClelland, J. W., Vorosmarty, C. J., Lammers, R. B., Shiklomanov, A. I., et al. (2002). Increasing river discharge to the Arctic Ocean. Science 298, 2171–2173. doi: 10.1126/science.1077445
Petrenko, D., Pozdnyakov, D., Johannessen, J., Counillon, F., and Sychov, V. (2013). Satellite-derived multi-year trend in primary production in the Arctic Ocean. Int. J. Remote Sens. 34, 3903–3937. doi: 10.1080/01431161.2012.762698
Polyakov, I. V., Alkire, M. B., Bluhm, B. A., Brown, K. A., Carmack, E. C., Chierici, M., et al., (2020). Borealization of the Arctic Ocean in response to anomalous advection from sub-arctic seas. Front. Mar. Sci. 7:491. doi: 10.3389/fmars.2020.00491
Polyakov, I. V., Pnyushkov, A. V., Alkire, M. B., Ashik, I. M., Baumann, T. M., Carmack, E. C., et al. (2017). Greater role for Atlantic inflows on sea-ice loss in the Eurasian Basin of the Arctic Ocean. Science 356, 285–291. doi: 10.1126/science.aai8204
Polyakov, I. V., Pnyushkov, A. V., and Carmack, E. C. (2018). Strength of the Arctic Halocline as an indicator of Climate Change. Environ. Res. Lett. 13:125008. doi: 10.1088/1748-9326/aaec1e
Polyakov, I. V., Pnyushkov, A. V., Rember, R., Padman, L., Carmack, E. C., and Jackson, J. M. (2013). Winter convection transports Atlantic water heat to the surface layer in the eastern Arctic Ocean. J. Phys. Oceanogr. 43, 2142–2155. doi: 10.1175/jpo-d-12-0169.1
Proshutinsky, A., Dukhovskoy, D., Timmermans, M.-L., Krishfield, R., and Bamber, J. (2015). Arctic circulation regimes. Philos. Trans. R. Soc. A 373:20140160. doi: 10.1098/rsta.2014.0160
Proshutinsky, A., Krishfield, R., Timmermans, M.-L., Toole, J., Carmack, E. C., McLaughlin, F., et al. (2009). Beaufort Gyre Fresh Water Reservoir: state and variability from observations. J. Geophys. Res. 114:C00A10. doi: 10.1029/2008JC005104
Proshutinsky, A., Krishfield, R., Toole, J. M., Timmermans, M.-L., Williams, W., Zimmermann, S., et al. (2019). Analysis of the Beaufort Gyre freshwater content in 2003–2018. J. Geophys. Res. Oceans 124, 9658–9689. doi: 10.1029/2019JC015281
Prowse, T., Bring, A., Mård, J., and Carmack, E. (2015a). Arctic Freshwater Synthesis: introduction. J. Geophys. Res. Biogeosci. 120, 2121–2131. doi: 10.1002/2015JG003127
Prowse, T., Bring, A., Mård, J., Carmack, E., Holland, M., Instanes, A., et al. (2015b). Arctic freshwater synthesis: summary of key emerging issues. J. Geophys. Res. Biogeosci. 120, 1887–1893. doi: 10.1002/2015JG003128
Rabe, B., Karcher, M., Kauker, F., Schauer, U., Toole, J. M., Krishfield, R. A., et al. (2014). Arctic Ocean basin liquid freshwater storage trend 1992–2012. Geophys. Res. Lett. 41, 961–968. doi: 10.1002/2013GL058121
Randelhoff, A., Holding, J., Janout, M., Sejr, M. K., Babin, M., Tremblay, J.-É., et al. (2020). Pan-Arctic Ocean primary production constrained by turbulent nitrate fluxes. Front. Mar. Sci. 7:150. doi: 10.3389/fmars.2020.00150
Randelhoff, A., and Sundfjord, A. (2018). Short commentary on marine productivity at Arctic shelf breaks: upwelling, advection and vertical mixing. Ocean Sci. 14, 293–300. doi: 10.5194/os-14-293-2018
Reisdorph, S. C., and Mathis, J. T. (2015). Assessing net community production in a glaciated Alaskan fjord. Biogeosciences 12, 5185–5198. doi: 10.5194/bg-12-5185-2015
Rudels, B., Anderson, L. G., and Jones, E. P. (1996). Formation and evolution of the surface mixed layer and halocline of the Arctic Ocean. J. Geophys. Res. 101, 8807–8821. doi: 10.1029/96JC00143
Rysgaard, S., Bendtsen, J., Delille, B., Dieckmann, G. S., Glud, R. N., Kennedy, H., et al. (2011). Sea ice contribution to the air-sea CO2 exchange in the Arctic and Southern Oceans. Tellus Ser. B 63, 823–830. doi: 10.1111/j.1600-0889.2011.00571.x
Rysgaard, S., Nielsen, T. G., and Hansen, B. W. (1999). Seasonal variation in nutrients, pelagic primary production and grazing in a high-Arctic coastal marine ecosystem, Young Sound, Northeast Greenland. Mar. Ecol. Prog. Ser. 179, 13–25. doi: 10.3354/meps179013
Schauer, U., and Losch, M. (2019). Freshwater in the Ocean is Not a Useful Parameter in Climate Research. J. Phys. Ocean 49, 2309–2321. doi: 10.1175/jpo-d-19-0102.1
Schlichtholz, P. (2019). Subsurface ocean flywheel of coupled climate variability in the Barents Sea hotspot of global warming. Sci. Rep. 9:13692. doi: 10.1038/s41598-019-49965-6
Schulz, K. G., Bach, L. T., Bellerby, R. G. J., Bermudez, R., Budenbender, J., Boxhammer, T., et al. (2017). Phytoplankton blooms at increasing levels of atmospheric carbon dioxide: experimental evidence for negative effects on prymnesiophytes and positive on small picoeukaryotes. Front. Mar. Sci. 4:64. doi: 10.3389/fmars.2017.00064
Serreze, M. C., Barrett, A. P., Slater, A. G., Woodgate, R. A., Aagaard, K., Lammers, R. B., et al. (2006). The large-scale freshwater cycle of the Arctic. J. Geophys. Res. 111:C11010. doi: 10.1029/2005JC003424
Shepherd, A., Ivins, E. R., Rignot, E., Smith, B., van den Broeke, M., Velicogna, I., et al. (2020). Mass balance of the Greenland Ice Sheet from 1992 to 2018. Nature 579, 233–239. doi: 10.1038/s41586-019-1855-2
Shu, Q., Qiao, F., Song, Z., Zhao, J., and Li, X. (2018). Projected freshening of the Arctic Ocean in the 21st century. J. Geophys. Res. Oceans 123, 9232–9244. doi: 10.1029/2018JC014036
SIMIP Community (2020). Arctic sea ice in CMIP6. Geophys. Res. Lett. 47:e2019GL086749. doi: 10.1029/2019GL086749
Sipler, R. E., Kellogg, C. T. E., Connelly, T. L., Roberts, Q. N., Yager, P. L., and Bronk, D. A. (2017). Microbial community response to terrestrially derived dissolved organic matter in the coastal arctic. Front. Microbiol. 8:1018. doi: 10.3389/fmicb.2017.01018
Slagstad, D., Wassmann, P. F. J., and Ellingsen, I. (2015). Physical constraints and productivity in the future Arctic Ocean. Front. Mar. Sci. 2:85. doi: 10.3389/fmars.2015.00085
Søreide, J. E., Leu, E., Berge, J., Graeve, M., and Falk-Petersen, S. (2010). Timing of blooms, algal food quality and Calanus glacialis reproduction and growth in a changing Arctic. Glob. Change Biol. 16, 3154–3163. doi: 10.1111/j.1365-2486.2010.02175.x
Stabeno, P. J., and Bell, S. W. (2019). Extreme Conditions in the Bering Sea (2017–2018): record-Breaking Low Sea-Ice Extent. Geophys. Res. Lett. 46, 8952–8959. doi: 10.1029/2019gl083816
Steele, M., and Boyd, T. (1998). Retreat of the cold halocline layer in the Arctic Ocean. J. Geophys. Res. 103, 10419-10435. doi: 10.1029/98JC00580
Steiner, N. S., Sou, T., Deal, C., Jackson, J. M., Jin, M., Popova, E., et al. (2016). The future of the subsurface chlorophyll-a maximum in the Canada Basin—A model intercomparison. J. Geophys. Res. Oceans 121, 387–409. doi: 10.1002/2015jc011232
Stigebrandt, A. (1985). On the hydrographic and ice conditions in the Northern North Atlantic during different phases of the glaciation cycle. Paleogeogr. Paleoclimatol. Paleoecol. 50, 302–321. doi: 10.1016/0031-0182(85)90074-4
Stroeve, J. C., Holland, M. M., Meier, W., Scambos, T., and Serreze, M. (2007). Arctic sea ice decline: faster than forecast. Geophys. Res. Lett. 34:L09501. doi: 10.1029/2007GL029703
Stroeve, J. C., Kattsov, V., Barrett, A. P., Serreze, M. C., Pavlova, T., Holland, M. M., et al. (2012a). Trends in Arctic sea ice extent from CMIP5, CMIP3 and observations. Geophys. Res. Lett. 39:L16502. doi: 10.1029/2012GL052676
Stroeve, J. C., and Notz, D. (2018). Changing state of Arctic sea ice across all seasons. Environ. Res. Lett. 13:103001. doi: 10.1088/1748-9326/aade56
Stroeve, J. C., Serreze, M. C., Holland, M. M., Kay, J. E., Masklanik, J., and Barrett, A. P. (2012b). The Arctic’s rapidly shrinking sea ice cover: a research synthesis. Clim. Change 110, 1005–1027.
Tank, S. E., Raymond, P. A., Striegl, R. G., McClelland, J. W., Holmes, R. M., Fiske, G. J., et al. (2012a). A land-to-ocean perspective on the magnitude, source and implication of DIC flux from major Arctic rivers to the Arctic Ocean. Glob. Biogeochem. Cycles 26:GB4018. doi: 10.1029/2011GB004192
Tank, S. E., Manizza, M., Holmes, R. M., McClelland, J. W., and Peterson, B. J. (2012b). The processing and impact of dissolved Riverine nitrogen in the Arctic Ocean. Estuaries Coasts 35, 401–415. doi: 10.1007/s12237-011-9417-3
Terhaar, J., Orr, J. C., Ethe, C., Regnier, P., and Bopp, L. (2019). Simulated Arctic Ocean Response to Doubling of Riverine Carbon and Nutrient Delivery. Glob. Biogeochem. Cycles 33, 1048–1070. doi: 10.1029/2019gb006200
Thackeray, C. W., and Hall, A. (2019). An emergent constraint on future Arctic sea-ice albedo feedback. Nat. Clim. Change 9, 972–978. doi: 10.1038/s41558-019-0619-1
Thrush, S., Hewitt, J., Cummings, V., Ellis, J., Hatton, C., Lohrer, A., et al. (2004). Muddy waters: elevating sediment input to coastal and estuarine habitats. Front. Ecol. Environ. 2, 299–306. doi: 10.1890/1540-9295(2004)002%5B0299:mwesit%5D2.0.co;2
Toole, J. M., Timmermans, M. L., Perovich, D. K., Krishfield, R. A., Proshutinsky, A., and Richter-Menge, J. A. (2010). Influences of the ocean surface mixed layer and thermohaline stratification on Arctic Sea ice in the central Canada Basin. J. Geophys. Res. 115:C10018. doi: 10.1029/2009JC005660
Torres-Valdes, S., Tsubouchi, T., Bacon, S., Naveira-Garabato, A. C., Sanders, R., McLaughlin, F. A., et al. (2013). Export of nutrients from the Arctic Ocean. J. Geophys. Res 118, 1625–1644. doi: 10.1002/jgrc.20063
Tremblay, G., Belzile, C., Gosselin, M., Poulin, M., Roy, S., and Tremblay, J.-É. (2009). Late summer phytoplankton distribution along a 3500 km transect in Canadian Arctic waters: strong numerical dominance by picoeukaryotes. Aquat. Microb. Ecol. 54, 55–70. doi: 10.3354/ame01257
Tremblay, J.-É., Anderson, L. G., Matrai, P., Coupel, P., Belanger, S., Michel, C., et al. (2015). Global and regional drivers of nutrient supply, primary production and CO2 drawdown in the changing Arctic Ocean. Prog. Oceanogr. 139, 171–196. doi: 10.1016/j.pocean.2015.08.009
Tremblay, J.-É., Bélanger, S., Barber, D. G., Asplin, M., Martin, J., Darnis, G., et al. (2011). Climate forcing multiplies biological productivity in the coastal Arctic Ocean. Geophys. Res. Lett. 38, 2–6. doi: 10.1029/2011GL048825
Tsubouchi, T., Bacon, S., Naveira-Garabato, A. C., Aksenov, Y., Laxon, S. W., Fahrbach, E., et al. (2012). The Arctic Ocean in summer: a quasi-synoptic inverse estimate of boundary fluxes and water mass transformation. J. Geophys. Res. 117:C01024. doi: 10.1029/2011JC007174
Vancoppenolle, M., Bopp, L., Madec, G., Dunne, J., Ilyina, T., Halloran, P. R., et al. (2013). Future Arctic Ocean primary productivity from CMIP5 simulations: uncertain outcome, but consistent mechanisms. Glob. Biogeochem. Cycles 27, 605–619. doi: 10.1002/gbc.20055
Vaquer-Sunyer, R., Duarte, C. M., Santiago, R., Wassmann, P., and Reigstad, M. (2010). Experimental evaluation of planktonic respiration response to warming in the European Arctic Sector. Polar Biol. 33, 1661–1671. doi: 10.1007/s00300-010-0788-x
Vaqué, D., Lara, E., Arrieta, J. M., Holding, J., Sá, E. L., Hendriks, I. E., et al. (2019). Warming and CO2 enhance Arctic heterotrophic microbial activity. Front. Microbiol. 10:494. doi: 10.3389/fmicb.2019.00494
Vavrus, S. J., Holland, M. M., Jahn, A., Bailey, D., and Blazey, B. A. (2012). Twenty-first-century Arctic climate change in CCSM4. J. Clim. 25, 2696–2710. doi: 10.1175/jcli-d-11-00220.1
Vihma, T., Screen, J., Tjernström, M., Newton, B., Zhang, X., Popova, V., et al. (2016). The atmospheric role in the Arctic water cycle: a review on processes, past and future changes, and their impacts. J. Geophys. Res. Biogeosci. 121, 586–620. doi: 10.1002/2015JG003132
Vonk, J. E., Mann, P. J., Davydov, S., Davydova, A., Spencer, R. G. M., Schade, J., et al. (2013). High biolability of ancient permafrost carbon upon thaw. Geophys. Res. Lett. 40, 2689–2693. doi: 10.1002/grl.50348
Wadham, J. L., Hawkings, J. R., Tarasov, L., Gregoire, L. J., Spencer, R. G. M., Gutjahr, M., et al. (2019). Ice sheets matter for the global carbon cycle. Nat. Commun. 10:3567. doi: 10.1038/s41467-019-11394-4
Wang, M., and Overland, J. E. (2012). A sea ice free summer Arctic within 30 years: an update from CMIP5 models. Geophys. Res. Lett. 39:L18501. doi: 10.1029/2012GL052868
Wang, Q., Wekerle, C., Danilov, S., Sidorenko, D., Koldunov, N., Sein, D., et al. (2019). Recent sea ice decline did not significantly increase the total liquid freshwater content of the Arctic Ocean. Am. Meteorol. Soc. 32, 15–32. doi: 10.1175/JCLI-D-18-0237.1
Wassmann, P., Bauerfeind, E., Fortier, M., Fukuchi, M., Hargrave, B., Moran, B., et al. (eds) (2004). “Particulate organic carbon flux to the Arctic Ocean sea floor,” in The Organic Carbon Cycle in the Arctic Ocean, eds R. Stein, and R. W. MacDonald (Berlin: Springer-Verlag).
Wassmann, P., and Reigstad, M. (2011). Future Arctic Ocean seasonal ice zones and implications for pelagic-benthic coupling. Oceanography 24, 220–231. doi: 10.5670/oceanog.2011.74
White, J. R., and Dagg, M. J. (1989). Effects of suspended sediments on egg production of the calanoid copepod Acartia tonsa. Mar. Biol. 102, 315–319. doi: 10.1007/bf00428483
Wiktor, J., Weslawski, J. M., Wieczorek, P., Zajackowski, M., and Okolodkov, Y. B. (1998). Phytoplankton and suspensions in relation to the freshwater in Arctic coastal marine ecosystems. Pol. Polar Res. 19, 219–234.
Wlodarska-Kowalczuk, M., and Pearson, T. H. (2004). Soft-bottom macrobenthic faunal associations and factors affecting species distributions in an Arctic glacial fjord (Kongsfjord, Spitsbergen). Polar Biol. 27, 155–167. doi: 10.1007/s00300-003-0568-y
Woodgate, R. (2018). Increases in the Pacific inflow to the Arctic from 1990 to 2015, and insights into seasonal trends and driving mechanisms from year-round Bering Strait mooring data. Prog. Oceanogr. 160, 124–154. doi: 10.1016/j.pocean.2017.12.007
Woodgate, R. A., Aagaard, K., and Weingartner, T. J. (2005). Monthly temperature, salinity, and transport variability of the Bering Strait through flow. Geophys. Res. Lett. 32:L04601. doi: 10.1029/2004GL021880
Woodgate, R. A., Weingartner, T., and Lindsay, R. (2010). The 2007 Bering Strait oceanic heat flux and anomalous Arctic sea-ice retreat. Geophys. Res. Lett. 37:L01602. doi: 10.1029/2009GL041621
Woosley, R. J., and Millero, F. J. (2020). Freshening of the western Arctic negates anthropogenic carbon uptake potential. Limonl. Oceanogr. doi: 10.1002/lno.11421
Yamamoto-Kawai, M., Carmack, E. C., McLaughlin, F. A., and Falkner, K. (2010). Oxygen isotope ratio, barium and salinity in waters around the North American coast from the Pacific to the Atlantic: implications for freshwater sources to the Arctic throughflow. J. Mar. Res. 68, 97–117. doi: 10.1357/002224010793078988
Yamamoto-Kawai, M., McLaughlin, F. A., and Carmack, E. C. (2013). Ocean acidification in the three oceans surrounding northern North America. J. Geophys. Res. Oceans 118, 6274–6284. doi: 10.1002/2013JC009157
Yamamoto-Kawai, M., McLaughlin, F. A., Carmack, E. C., Nishino, S., and Shimada, K. (2008). Freshwater budget of the Canada Basin, Arctic Ocean, from salinity, δ18O, and nutrients. J. Geophys. Res. 113:C01007. doi: 10.1029/2006JC003858
Yamamoto-Kawai, M., McLaughlin, F. A., Carmack, E. C., Nishino, S., Shimada, K., and Kurita, N. (2009). Surface freshening of the Canada Basin, 2003–2007: river runoff versus sea ice meltwater. J. Geophys. Res. 114:C00A05. doi: 10.1029/2008JC005000
Yang, D. (1999). An Improved Precipitation Climatology For The Arctic Ocean. Geophys. Res. Lett. 26, 1625–1628. doi: 10.1029/1999gl900311
Yun, M. S., Whitledge, T. E., Stockwell, D., Son, S. H., Lee, J. H., Park, J. W., et al. (2016). Primary production in the Chukchi Sea with potential effects of freshwater content. Biogeosciences 13, 737–749. doi: 10.5194/bg-13-737-2016
Zhang, J., Spitz, Y. H., Steele, M., Ashjian, C., Campbell, R., Berline, L., et al. (2010). Modeling the impact of declining sea ice on the Arctic marine planktonic ecosystem. J. Geophys. Res. 115:C10015. doi: 10.1029/2009JC005387
Keywords: Arctic Ocean, freshwater, freshening, primary production, stratification, physics, geochemistry, biology
Citation: Brown KA, Holding JM and Carmack EC (2020) Understanding Regional and Seasonal Variability Is Key to Gaining a Pan-Arctic Perspective on Arctic Ocean Freshening. Front. Mar. Sci. 7:606. doi: 10.3389/fmars.2020.00606
Received: 12 December 2019; Accepted: 30 June 2020;
Published: 14 August 2020.
Edited by:
Markus A. Janout, Alfred Wegener Institute Helmholtz Centre for Polar and Marine Research (AWI), GermanyReviewed by:
Lee W. Cooper, University of Maryland Center for Environmental Science (UMCES), United StatesManfredi Manizza, University of California, San Diego, United States
Copyright © 2020 Brown, Holding and Carmack. This is an open-access article distributed under the terms of the Creative Commons Attribution License (CC BY). The use, distribution or reproduction in other forums is permitted, provided the original author(s) and the copyright owner(s) are credited and that the original publication in this journal is cited, in accordance with accepted academic practice. No use, distribution or reproduction is permitted which does not comply with these terms.
*Correspondence: Kristina A. Brown, S3Jpc3RpbmEuQnJvd25AZGZvLW1wby5nYy5jYQ==