- 1School of Agriculture and Environment, The University of Western Australia, Crawley, WA, Australia
- 2Oceans Institute, The University of Western Australia, Crawley, WA, Australia
- 3School of Agriculture and Environment, The University of Western Australia, Albany, WA, Australia
The ingestion of plastic debris has been studied in many marine fish species, although comparisons between species can be difficult due to factors thought to influence ingestion rates, such as habitat preference, feeding behaviours and trophic level. Sardines are found internationally in many coastal environments and represent a potential sentinel species for monitoring and comparing marine plastic exposure rates. We conducted a pilot study, examining the rate of plastic ingestion in 27 commercially caught sardines (Sardinops sagax) from a low populated coastal region of Western Australia. A total of 251 potentially anthropogenic particles were extracted by chemical digestion of the gastrointestinal tract and classified visually. Fibres were the dominant type of material recovered (82.9%), with both yellow (39.8%) and black (32.7%) coloured particles commonly observed. A subset of 64 particles (25.5%), were subject to Fourier transform infrared (FTIR) spectroscopy to identify polymer composition. This chemical characterisation identified seven plastic items (polypropylene, nylon and polyethylene) and a variety of cellulose-based material that was further examined and classified as natural or semi-synthetic. The mean plastic ingestion rate was 0.3 ± 0.4 particles per fish, suggesting Western Australian sardines ingest relatively low concentrations of plastic when compared to international sardine populations examined using similar methodologies. Despite comparatively low concentrations, plastic and semi-synthetic material are still being ingested by sardines from a low populated coastal region demonstrating the ubiquitous nature of the marine debris problem.
Introduction
Globally, over 300 million tonnes of plastic are produced each year, projected to exceed one billion by 2050 (Lusher et al., 2017a). Plastic is manufactured for use across many industries, sought for its low cost, versatility, strength and durability. Plastic items can last longer than their usable life (e.g., single-use plastic bags and straws) and be difficult to recover from waste streams for recycling. Geyer et al. (2017) assessed the amount of plastic waste generated from 1950 to 2015, estimating 9% had been recycled, 12% was incinerated, and 79% had accumulated in landfills or leaked to the natural environment.
There is growing international concern regarding the prevalence and impact of plastic debris in the marine environment (Commonwealth of Australia, 2018; United Nations, 2018). The ubiquitous nature of this pollution is demonstrated through considerable research investigating the open oceans, deep-sea sediments, remote islands, coastal environments and Arctic waters (Browne et al., 2011; Van Cauwenberghe et al., 2013; Eriksen et al., 2014; Lusher et al., 2015; Lavers and Bond, 2017). Contributing sources of plastic debris are widespread and include mismanaged waste, maritime activities (e.g., fishing), stormwater runoff and the discharge of treated wastewater (Vegter et al., 2014; UN Environment, 2018). Jambeck et al. (2015) estimate that between 4.8 and 12.7 million metric tonnes of plastic entered the ocean in 2010. Once in the ocean, the structural properties of plastic allow them to persist for long periods having widespread economic, social and ecological impacts (Beaumont et al., 2019).
Plastic ingestion has been found across many trophic levels in the marine environment including zooplankton (Cole et al., 2013), coral (Hall et al., 2015), fish (Cannon et al., 2016), birds (Roman et al., 2019), and mammals (Provencher et al., 2017). Many studies on plastic ingestion have focused on fish, investigating numerous species from a variety of regions, habitats and trophic levels. The health concerns from plastic ingestion in fish include; a reduced feeding potential (Neves et al., 2015), bioaccumulation (Carbery et al., 2018), the translocation of plastic into other organs (Collard et al., 2017a), and the leaching of harmful chemicals and pollutants from the digestive tract into the fish tissue (Lithner et al., 2011; Gewert et al., 2015). The full effects of these are not understood with many authors emphasising the need for further research (e.g., Cole et al., 2013; Lusher et al., 2017b; Provencher et al., 2017; Smith et al., 2018). As fish represent a key source of protein for humans, plastic ingestion could have potential implications for food security and human health (Thompson et al., 2009; Neves et al., 2015; Rummel et al., 2016; Bessa et al., 2018; Sun et al., 2019).
Plastic has been the focus for many marine debris studies, however, non-plastic anthropogenic material can also be recovered, sometimes in high volumes (Lusher et al., 2013; Remy et al., 2015; Compa et al., 2018). An example is the cellulose-based fibre rayon (also termed “viscose”), manufactured for use in textiles or furnishings. Although the fibre is created using naturally derived material, the manufacturing process requires extensive chemical treatment and can include additives such as flame retardants, ultraviolet absorbers and dyes (Bredereck and Hermanutz, 2008; United Nations Environment Program, 2018). Little is known about the potential release of these additives if manufactured fibres are dispersed into the environment or ingested by aquatic species (Remy et al., 2015; Zhao et al., 2016). Similar to plastic, these manufactured fibres can adsorb ambient pollutants, potentially acting as a vector for pollutants when ingested (Compa et al., 2018). They may also provide a false sense of satiety when ingested, affecting feeding and growth (Kühn et al., 2015). The implications of ingesting non-plastic anthropogenic material are concerning, and although recognised in many studies, non-plastic manufactured fibres are inconsistently reported and classified (Remy et al., 2015; Zhao et al., 2016; Compa et al., 2018; Kroon F.J. et al., 2018).
Due to the wide variety of fish species examined in plastic ingestion studies, comparing research findings, and developing management strategies is difficult. Plastic ingestion in fish can be influenced by species traits such as habitat preferences (Rummel et al., 2016), feeding behaviours (Collard et al., 2017b) and trophic level (e.g., Ferreira et al., 2018). The influence of these factors can be reduced by investigating fish with similar characteristics. “Sardine” is a common name applied to several fish species from the same family found in many coastal regions of the world (Figure 1). As a nearshore epipelagic fish, sardines are likely exposed to anthropogenic sources of marine plastic pollution. Sardines have expected longevity between 7 and 8 years and can reach sexual maturity in 2 years (Silva et al., 2006). They are a highly mobile (Parrish et al., 1989), low trophic level planktivorous fish, potentially ingesting ambient concentrations of small-sized debris through filter-feeding behaviours (Garrido et al., 2008). Sardines form a key component to the marine food web and as one of the highest biomass fisheries in the world (FAO, 2018), are readily available. These factors suggest sardines could be a potential sentinel species for investigating global variations in marine plastic pollution within the nearshore epipelagic zone.
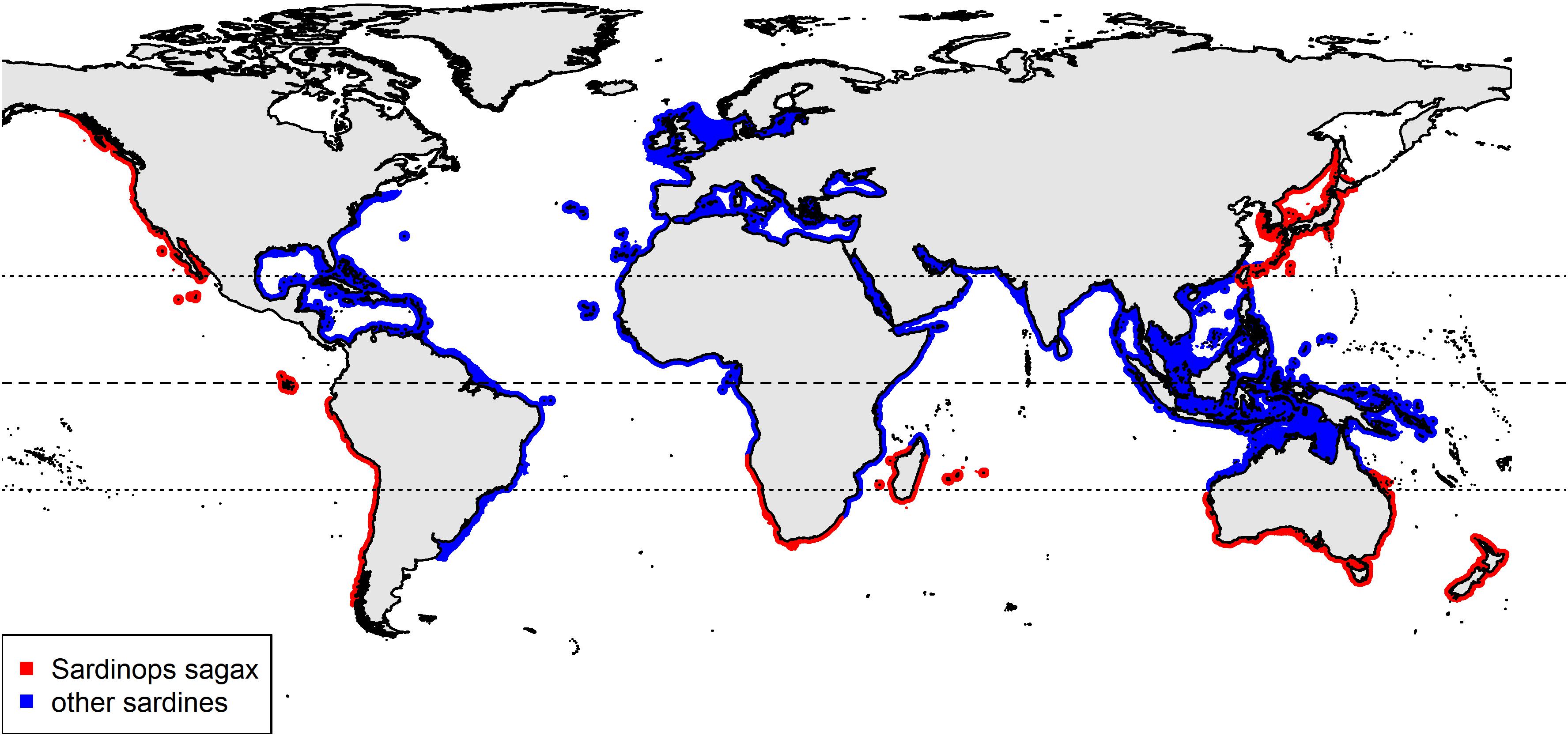
Figure 1. A global distribution of sardines highlighting the range for Sardinops sagax (data sourced from Nature Serve and IUCN, 2020).
In Australia, the sardine Sardinops sagax can be found in the coastal waters of the southern half of the continent (Figure 1). They have extensive migrations, driven by spawning and changing environmental conditions (Fletcher, 1990; Izzo et al., 2012). Sardines are an important food source for Australian marine mammals (e.g., dolphins, seals), birds (e.g., penguins, petrels) and higher trophic level fish species (e.g., southern bluefin tuna, salmon) (Fletcher, 1990; Goldsworthy et al., 2013). Sardines are a valuable economic resource in Australia, with the commercial fishery generating over $27 million in 2016–2017 from approximately 44,000 tonnes of wild-caught sardines (Mobsby, 2018). Most of the catch is used as feedstock for Australian Southern Bluefin Tuna ranching (Commonwealth of Australia, 2009), a fishery worth more than $30 million in 2015–2016 (Patterson et al., 2017). S. Sagax is an important fish species in Australia and research to determine if it is ingesting anthropogenic debris is valuable.
Despite Australia being remote and having a relatively small and widely dispersed population, plastic has been found in many parts of its marine environment (Edyvane et al., 2004; Hardesty et al., 2011; Reisser et al., 2013; Smith et al., 2014; Hajbane and Pattiaratchi, 2017; Kroon F. et al., 2018; Paterson and Dunlop, 2018). Few studies have investigated plastic ingestion in Australian marine fish species with recent reports by Halstead et al. (2018), Kroon F.J. et al. (2018), and Jensen et al. (2019) finding ingestion rates ranged between 0.1 and 2.5 plastic (or plastic containing) particles per fish. Because plastic is used extensively in the manufacture of human-use goods and packaging (GESAMP, 2015), rates of plastic ingestion are expected to be low in Australian waters, particularly in remote regions with low populations.
This study aimed to determine if Australian sardines (S. sagax) caught in a low-populated coastal region were ingesting plastic and where present, examine the polymer type, shape, size and colour. The study would act as a method trial with the findings providing evidence for a previously unassessed area and giving context to existing studies that have measured plastic ingestion in other international sardine populations.
Materials and Methods
Study Area
Twenty-seven commercially caught sardine cadavers were purchased from a fishmonger (Bevans) located in the coastal town of Albany, Western Australia (WA). The fish were stored at −20°C until required for analysis. The sardines were caught in January 2019 from a fishing zone encompassing Frenchman Bay and part of King George Sound. This fishing zone is situated along the southern coast of WA (Figure 2) and has a depth typically less than 50 m. Albany’s Mediterranean climate is characterised by cool, wet winters (June to August) and mild summers (December to February), typically receiving over 750 mm of rainfall each year (Pettit et al., 2015). The regions coastal waters are likely influenced by the Leeuwin Current, which moves warmer tropical water down the west coast of Australia and then eastwards towards Tasmania (Cresswell and Domingues, 2009).
Laboratory Analysis
The laboratory methods used were based on similar studies (Foekema et al., 2013; Dehaut et al., 2016; Bessa et al., 2018; Kumar et al., 2018; Pellini et al., 2018). In summary, the sardines were thawed at room temperature, and the fork length (mm) and weight (g) of each fish was recorded. The gastrointestinal tract of each fish was dissected and weighed, then placed in a 20 ml test tube containing a minimum 5:1 ratio (i.e., five times the gastrointestinal mass) of 10% Potassium Hydroxide (KOH). All test tubes were placed in a water bath for 72 h at 60°C. Tissue digestion was assisted by vortex mixing (Select Vortexer) each test tube for 60 s every 12 h. Digestion was halted at 72 h as samples appeared sufficiently digested.
Following digestion, each solution was individually vacuum filtered through a labelled 22 μm filter paper (WhatmanTM) and rinsed with 50 ml of deionised water. The filter papers were then placed inside clean Petri dishes and oven-dried at 40°C.
A stereo microscope (Olympus SZ60) was used to inspect each filter paper and count the undissolved potential anthropogenic debris. Each particle was initially classified by shape (fibre, fragment or microbead) and colour; and then, where necessary, further distinctions were made based on physical observations (e.g., flat) or approximate size differences (e.g., thin, thick, large, small). The criteria for these particle groups were reviewed throughout the process, confirming visually similar particles were assigned together. All classifications were completed by the same technician to ensure consistency.
Chemical Characterisation
For chemical characterisation, a subset (n = 64) of particles was selected, including at least one item from each visually distinct particle group (n = 22). Overall, the subset represented 25.5% of the isolated debris. From the subset, typical specimens were chosen for microscopy, and particle lengths were measured, then classified in one-millimetre increments up to 5 mm. This measurement indicates the maximum length of a fibre or the broadest cross-section of a fragment or microbead. For chemical characterisation, each particle from the subset was mounted on a metal base plate, and infrared spectroscopy measurements were performed using a Vertex 70 Fourier transform infrared (FTIR) spectrometer (Bruker) and Hyperion 3000 microscope. Infrared spectra were collected in the range of 4000–600 cm–1 using either reflectance or attenuated total reflectance (ATR) operating modes. All spectra were recorded using a resolution of 4 cm–1, and between 100 and 128 scans were performed for each spectrum. Background spectra were obtained by using the same settings but collected without contacting the sample.
To identify particle composition, the generated spectra data were baseline corrected and compared to spectra from the Bio-Rad KnowItAll® IR Spectral Library (Bio-Rad Laboratories, California, United States). Identification was considered sufficient following the visual inspection of characteristic peaks with a minimum 85% match to database examples (Bessa et al., 2018). For illustrative purposes, selective spectral data has been paired with microscope images and presented below for reference. To improve clarity, images have been brightened where necessary, and microscope images and spectral data are presented with enhanced scales.
Particle Classification
Four classification categories were used: “synthetic,” “semi-synthetic,” “natural,” and “unknown.” Synthetic items contained chemically synthesised polymer materials (e.g., polypropylene, nylon). Semi-synthetic material represented those chemically manufactured from natural materials (e.g., rayon, cellulose acetate). Natural particles consisted of undissolved organic debris or naturally derived fibres (e.g., chitin, cotton). Unknown samples were those that generated undistinguishable spectrum that failed to adequately match database references or those that were damaged or lost during analysis.
Cellulose-based fibres can be natural or manufactured (semi-synthetic) and are difficult to classify due to similar visual and spectral characteristics. In this study, semi-synthetic cellulose-based fibres were distinguished from natural fibres, by the absence of a spectral peak at 1105 cm–1 (Comnea-Stancu et al., 2017; Cai et al., 2019).
Data Analyses
The synthetic and semi-synthetic ingestion rates were calculated by the following: the number of classified particles/the number of sardines. Standard deviations were calculated for each ingestion rate and other metrics including fish length and weight. The standard deviations are presented following the respective mean values where necessary.
Contamination Mitigation
Contamination from external sources is a problem for marine plastic research where its presence can misrepresent true pollution levels (Bergmann et al., 2015; Dehaut et al., 2019; GESAMP, 2019). Extensive management protocols were enforced throughout all processing and analysis to minimise potential contamination. These protocols included a 3-day pre-quarantine period for laboratory facilities, restricting laboratory access during processing, wearing cotton clothing and lab coats, cleaning work surfaces, pre-filtering digestion and wash solutions, triplicate rinsing glassware, and avoiding plastic apparatus. Wherever possible, samples and equipment were sealed or covered with aluminium foil to prevent airborne contamination. Three airborne and three procedural blank control samples were used to monitor contamination throughout laboratory analysis. Any items recovered in the control samples were visually characterised and classified as external contamination. Recovered particles that appeared visually similar to the contamination particles were excluded from analyses.
Results
The gastrointestinal tracts from 27 commercially caught WA sardines were examined. The sardines had an average fork length of 146.9 ± 7.1 mm and weight of 36.3 ± 4.9 g.
Visual Classification
Two hundred and fifty-one potential anthropogenic particles were isolated from the sardines and classified into 22 visually distinct particle groups (Table 1 and Supplementary Material). All sardines contained potentially anthropogenic debris, ranging between two and 23 particles per fish. The average ingestion rate was 9.3 ± 5.0 particles per fish (median = 9). Of the 251 recovered particles, 208 were fibres (82.9%), 30 were fragments (12.0%), and 13 were microbeads (5.2%) (Figure 3A). Yellow was the most common colour (39.8%), followed by black (32.7%), translucent (13.6%) and blue (6.8%) (Figure 3B).
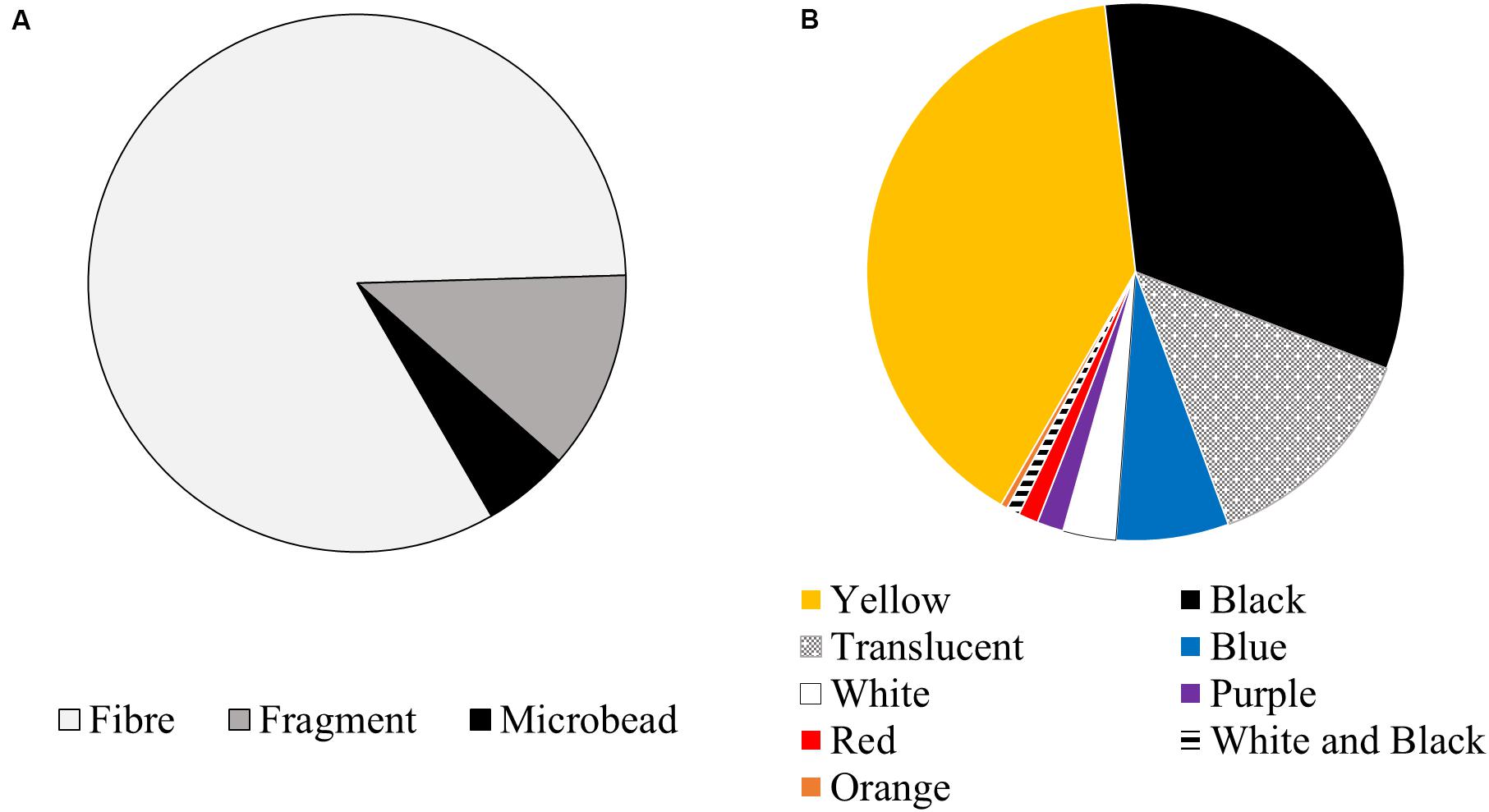
Figure 3. (A) Shape and (B) colour classification of debris recovered from the gastrointestinal tracts of sardines caught in Frenchman Bay, Western Australia in January 2019.
Overall, 46.9% of measured particles were less than 1 mm, and 73.4% were under 2 mm (Figure 4). Four items (6.3%) were larger than 5 mm, including one fibre measuring 18.0 mm in length. The average particle size was 1.6 mm (±2.6 mm). No recovered fragments or microbeads were larger than 3 mm.
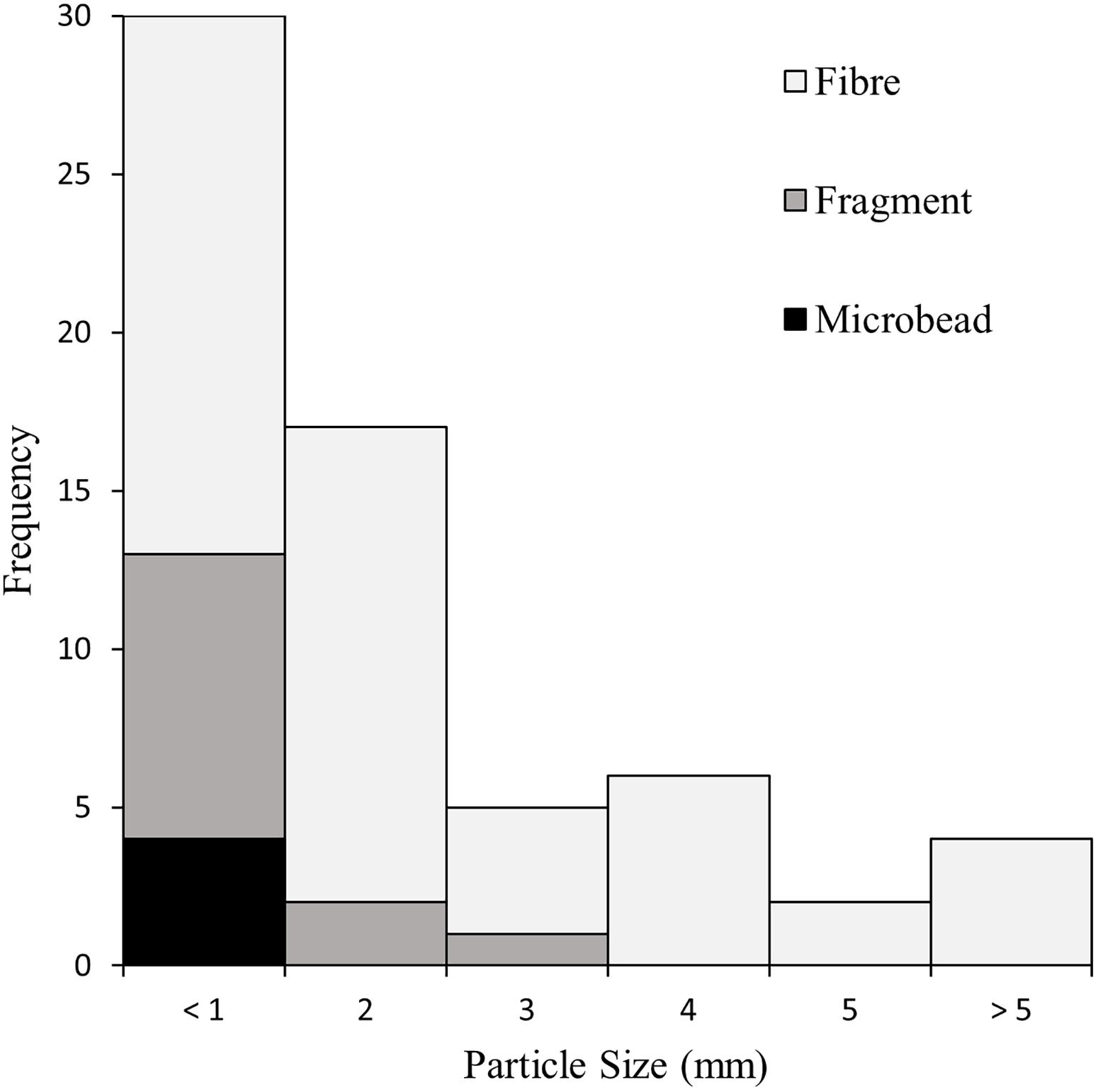
Figure 4. The size distribution of subset particles recovered from the gastrointestinal tracts of sardines caught in Frenchman Bay, Western Australia in January 2019.
Chemical Characterisation
FTIR analysis conducted on the subset particles identified seven (10.9%) as synthetic, 16 (25.0%) as semi-synthetic and 11 (17.2%) as natural. There were 30 unknown items including 17 (26.6%) that failed to generate distinctive spectra and 13 (20.3%) that were unable to be analysed. Synthetic items from the subset included five fibres and two fragments. Three of the fibres were nylon (e.g., Figure 5A), and two were polypropylene (e.g., Figure 5B). One synthetic fragment was identified as polypropylene; the other was polyethylene (Figure 5C). The average size of all measured particles was 1.6 mm (±2.6 mm), however, synthetic particles had an average size of 5.4 mm (±6.1 mm), including a single 18.0 mm polypropylene fibre. The ingestion rate of synthetic particles was 0.3 ± 0.4 per fish with no sardines containing more than one synthetic particle.
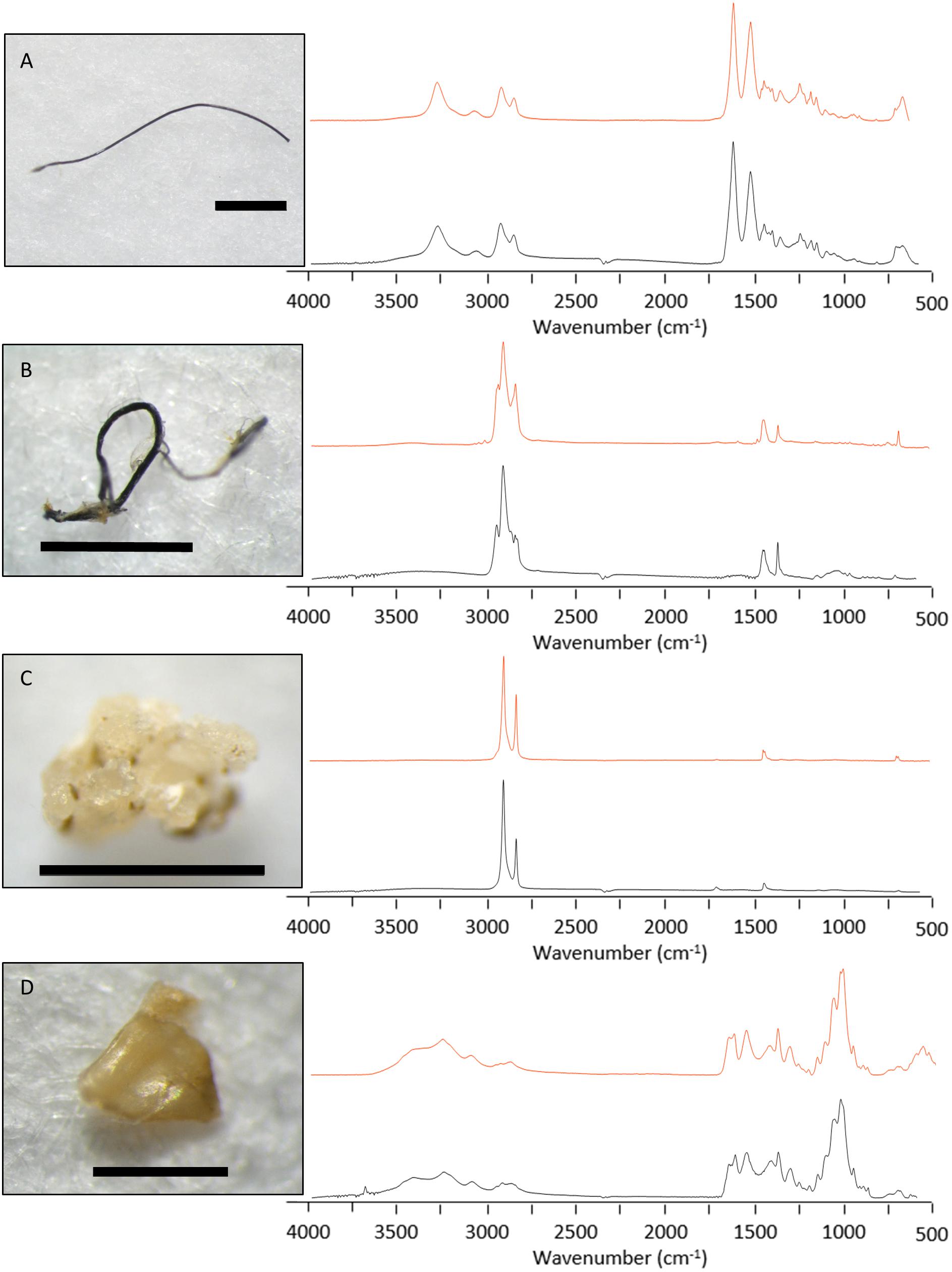
Figure 5. Spectral match information for four particles recovered from the gastrointestinal tract of sardines caught in Frenchman Bay, Western Australia in January 2019. The upper (red) line illustrates Bio-Rad KnowItAll® database reference spectra, with the lower (black) line representing the measured spectra. Spectral analysis identified these particles as (A) nylon, (B) polypropylene, (C) polyethylene, and (D) chitin. Image scale bars represent (A–C) 1 mm and (D) 0.5 mm.
Twenty-seven items were classified as semi-synthetic or natural. Of these, six items were matched with respective database samples and classified accordingly (e.g., chitin, Figure 5D). The remaining 21 were cellulose-based fibres that required further examination of the FTIR spectral data for classification (Comnea-Stancu et al., 2017; Cai et al., 2019). Fibres with a spectral peak at 1105 cm–1 were classified natural, and those without were considered modified, classified as semi-synthetic (Figure 6). Overall, seven fibres and four fragments were classified as natural and 14 fibres and two fragments were classified as semi-synthetic. All microbeads failed to generate distinctive spectra. The resulting semi-synthetic ingestion rate was calculated at 0.6 ± 0.7 particles per fish.
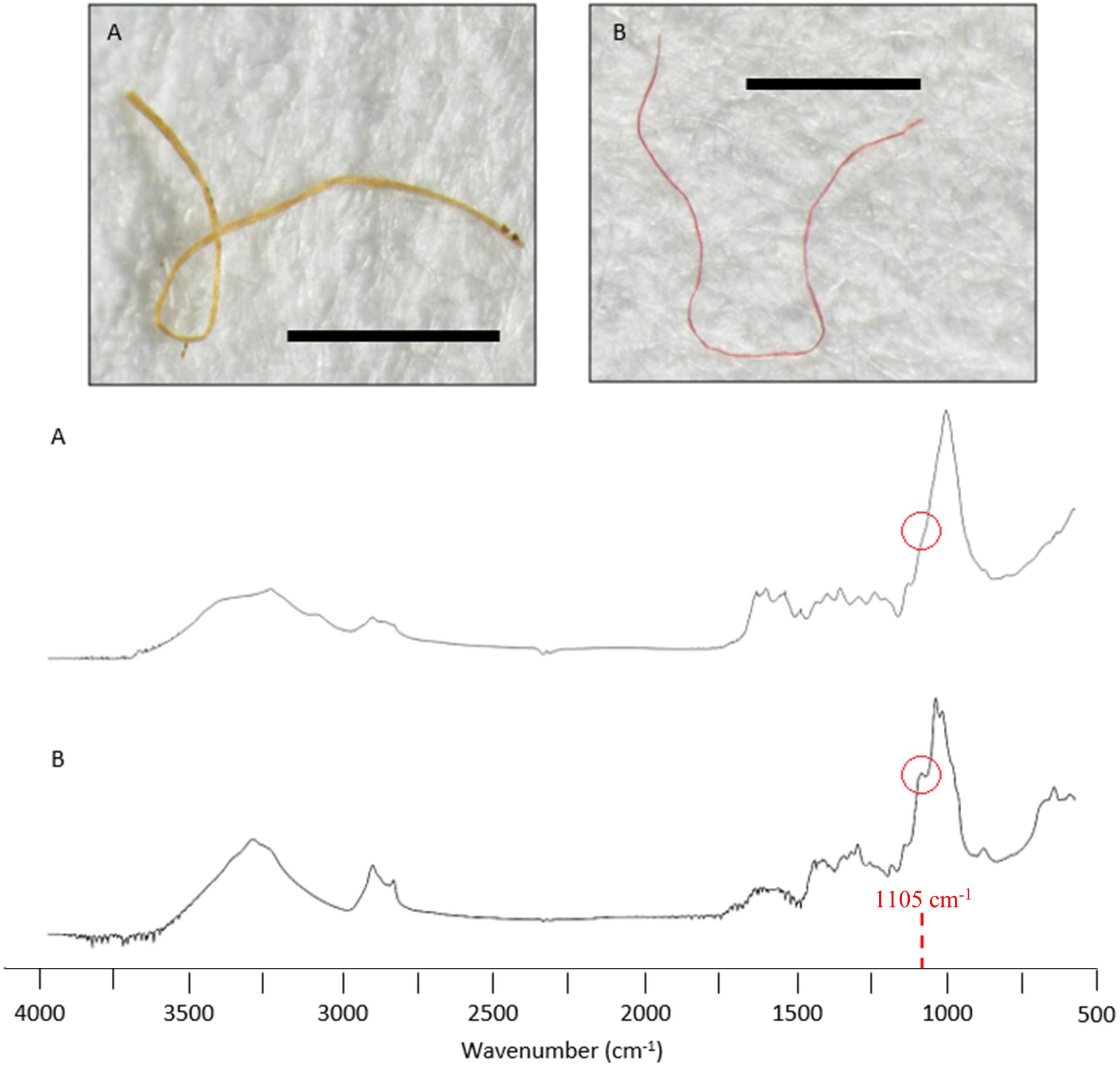
Figure 6. Spectral comparison of two cellulose-based fibres recovered from the gastrointestinal tract of sardines caught in Frenchman Bay, Western Australia in January 2019. Sample (A) was classified semi-synthetic due to the absence of a spectral peak at 1105 cm–1. Sample (B) was classified natural due to the spectral peak at 1105cm–1. Image scalebars represent 1 mm.
Subgroup Extrapolation
A subset of 64 particles (25.5%) was generated by selecting at least one item from each of the 22 visually distinct particle groups. The selected particles were chosen opportunistically, ensuring distinctive particles were analysed where possible. As the subset of particles were not randomly selected, we have not extrapolated the chemical characterisation results to all potential anthropogenic particles as conducted in other studies (e.g., Bessa et al., 2018; Lefebvre et al., 2019). It is, therefore, possible that this study provides a conservative estimate.
Discussion
Western Australian sardines were found to ingest 0.3 ± 0.4 plastic particles per fish, lower than recently reported in three Australian fish species (Kroon F.J. et al., 2018; Jensen et al., 2019) and many other international sardine populations (Table 1). The low ingestion rates could be influenced by various environmental and anthropogenic features in the study region. There are two factors that are considered important in the region, population size and waste management. The sardines were caught in an area that supports a coastal city with a relatively low population of approximately 33,000 people (Australian Bureau of Statistics, 2016), which is expected to correlate with low concentrations of plastic debris (Browne et al., 2011). In addition, the local government authority discharges its treated wastewater to nearby forestry farms (Department of Environment Regulation, 2016) rather than the ocean. This means synthetic fibres collected through the washing of household textile items are not released directly to the marine environment. With the potential of comparatively little input from the terrestrial environment and the migratory nature of this species, the fish in this region are likely exposed to pollution levels consistent with the wider ocean and not local sources. Consideration of local environmental and anthropogenic processes is important when making comparisons between studies, and for applying appropriate regional management actions. Future research guidelines should establish and recommend the disclosure of key environmental and anthropogenic processes thought to influence marine plastic debris; these details can expand on guidelines such as those by Dehaut et al. (2019) and GESAMP (2019), providing greater context to each study.
Various methods have been applied to investigate the occurrence of plastic ingestion in fish, each providing advantages and disadvantages that can have implications on the research outcomes. Recent review studies have examined the current range of techniques applied, identifying some that may miss-report the presence of plastic debris (Hidalgo-Ruz et al., 2012; Lusher et al., 2017b; Miller et al., 2017; Shim et al., 2017; Dehaut et al., 2019). For example; the manual extraction of suspected plastic debris may under-estimate smaller particles or fibres that are difficult to remove from fish organs. Visual classification techniques (e.g., Norén, 2007) potentially over-estimate plastic counts where naturally derived material appears synthetic. Although there is no internationally standardised method of assessing plastic ingestion in fish, current evidence supports the alkaline digestion of the gastrointestinal tract, followed by the chemical characterisation of all, or a subset of, the undigested particles (Kühn et al., 2017; Lusher et al., 2017b; Miller et al., 2017; Dehaut et al., 2019). Quality controls are also recommended to prevent or reduce sample contamination by external material (GESAMP, 2019). Given the methods of assessing plastic ingestion in fish continue to develop, it can be negligent to directly compare new results with existing studies that may have used less rigorous methods. This is demonstrated in Table 1, where studies using visual extraction methods report lower rates of plastic ingestion than those applying digestion protocols, possibly a consequence of the chosen methods.
When classifying ingested debris by particle type (e.g., fragment, fibre, microbead), fibres often account for a major fraction (Table 1). This was observed in Western Australian sardines, where ingested fibres represented 82.9% of all recovered anthropogenic debris (n = 208/251) and 71.4% of recovered plastics (n = 5/7). Plastic fibres can enter the environment through the breakdown of synthetic fishing equipment and the discharge of treated wastewater containing microfibres released from synthetic clothing (Ziajahromi et al., 2017; Montarsolo et al., 2018). To recover and identify plastic fibres from environmental and biotic samples, advanced processing considerations are needed. These include; using appropriate filter apertures to capture small and narrow fibres, restricting airborne fibre contamination, avoiding visual extraction methods, and utilising chemical characterisation methods. Studies using methods that potentially exclude or under-report fibres, may be underestimating plastic concentrations (e.g., Foekema et al., 2013; Cozar et al., 2014; Romeo et al., 2015). Future developments are likely to improve the analytical methods available for small plastic particles (e.g., microfibres, nano-plastics), however, current evidence already demonstrates the ubiquitous nature of synthetic fibres in the marine environment and their importance in comprehensive plastic assessments (Barrows et al., 2018).
In this study, seven plastic fragments composed of either polypropylene, polyethylene or nylon were recovered from the gastrointestinal tracts of Western Australian sardines. These polymer types are commonly found in the marine environment (Andrady, 2011; Hidalgo-Ruz et al., 2012; GESAMP, 2015), and have been ingested by other international sardine populations (Table 1). Polypropylene, polyethylene and nylon are mass-produced for use in the automotive and electronics industry, as well as for packaging and textiles (Geyer et al., 2017; UN Environment, 2018). The widespread use of these polymers and the complex environmental systems make it difficult to identify the potential origin of these particles. Future studies in the region should assess what activities are contributing plastic by examining potential sources such as local stormwater drains and fishing equipment. If ingested, plastics have the potential to impact fish physiology and behaviour as demonstrated in laboratory exposure studies (Rochman et al., 2013; Critchell and Hoogenboom, 2018; Naidoo and Glassom, 2019). Further research is needed to measure the direct effects of ingesting environmentally relevant concentrations of marine plastic debris. Until such information is available, it is difficult to assess the likely health impacts from reported plastic ingestion rates.
Forty-seven per cent (n = 16/34) of successfully identified particles were classified as semi-synthetic, equating to 0.6 ± 0.7 particles per fish. Although semi-synthetic particles are not always quantified, existing studies have found they can constitute 30–58% of recovered debris which is also reflected in this study (Bessa et al., 2018; Kroon F.J. et al., 2018; Jensen et al., 2019). Semi-synthetic fibres (e.g., rayon, cellulose acetate) are manufactured from chemically treated naturally derived material and are used in textiles, cigarette filters and furnishings. These fibres are typically considered harmless and receive less attention than plastic waste, however, like plastic, semi-synthetic fibres can be vectors for adsorbed contaminants in the marine environment (Compa et al., 2018). Their manufacture also involves extensive chemical treatment and can include additives such as flame retardants, ultraviolet absorbers and dyes (Bredereck and Hermanutz, 2008; United Nations Environment Program, 2018). Little is known about the potential release of these contaminants from semi-synthetic material that is released into the environment, however, the physical breakdown is predicted to be quicker than for plastic (Remy et al., 2015; Zhao et al., 2016; Compa et al., 2018). If our interest is in understanding anthropogenic impacts on the environment, limiting the focus to plastic could underestimate total contamination levels. Therefore, when assessing potential contaminant exposure, non-plastic anthropogenic debris and their associated chemical load should also be considered.
It is important to consider feeding behaviours when investigating the ingestion of anthropogenic debris in the marine environment (Mizraji et al., 2017). The sardine is a pelagic species, feeding in the water column of the nearshore coastal environment. Sardines have two feeding mechanisms which it can switch between depending on prey size and density; although filter-feeding is recognised as the primary method (van der Lingen, 1994; Louw et al., 1998). Due to the morphological dimensions of the sardine mouth, particles larger than 1200 μm generally prompt selective particulate-feeding, while smaller particles are often collected by filter-feeding (Louw et al., 1998; Garrido et al., 2008). In this study, 46.9% of the measured potential anthropogenic debris was smaller than 1 mm, potentially a result from non-selective filter-feeding in the region.
Most of the recovered particles were yellow (39.8%), or black (32.7%) in colour and their origin is currently unknown. All yellow particles were fibres had a similar appearance (Figure 6A). Those that were successfully analysed by FTIR were characterised as semi-synthetic, indicating that these particles potentially originate from the same source material. A variety of black fibres, fragments and microbeads were recovered from the sardine GITs. The black items that generated adequate spectral information were classified as a mixture of synthetic, semi-synthetic and natural particles. Future research should conduct greater sampling of the region to assess and identify potential sources of debris.
Through the completion of this pilot study, the authors have identified factors for consideration in similar future studies. The first recommendation is to use a more comprehensive sampling regime that includes a greater number of sardines from multiple regions along the southern coast of Australia. A minimum sample size of 50 is currently recommended by the Joint Group of Experts on Scientific Aspects of Marine Environmental Protection (GESAMP, 2019), although the small sample size used here is not unprecedented (Collard et al., 2017b; Kroon F.J. et al., 2018; Zhu et al., 2019). Examining the biological features of the Australian sardine in more detail (e.g., migration patterns, trophic structures) may assist sampling design and provide greater insight regarding the ingestion of anthropogenic debris in this species. Environmental processes that may influence the concentration of anthropogenic debris, such as rainfall patterns and the transnational movement of seawater need to be included. While consensus on methods for extracting ingested anthropogenic particles is beginning to be achieved, chemical characterisation can still be time-intensive and challenging, particularly when the particles are microscopic (e.g., fibres). Refinement of characterisation procedures is still required. Overall, the processes used within this study were able to provide evidence that plastic is being ingested by Australian sardines, however, additional testing is needed to improve the number of successfully identified particles following chemical characterisation. Future studies should recognise the technical aspects of chemical characterisation are often under-reported in the literature, and researchers should ensure they have adequate capacity to conduct robust assessments.
Conclusion
Plastic ingestion has been studied in many fish species, although inconsistencies in fish characteristics and analytical methods make it difficult to compare findings. Sardines are found in many coastal regions of the world and could potentially be used as a sentinel species, providing some consistency to international plastic ingestion studies. We examined plastic ingestion in sardines from a low populated coastal region of WA, finding lower ingestion rates than reported in several international sardine populations (Table 1). The ingested plastics were predominantly fibres, composed of polypropylene, polyethylene and nylon; polymers that are commonly found in the marine environment. Semi-synthetic anthropogenic particles (e.g., cellulose-based fibres) were also ingested, but at a greater rate than plastics, demonstrating their ability as a potential contamination source.
Overall, this study highlights the ubiquitous nature of plastic debris by providing evidence that sardines from a low-populated region of WA ingest synthetic and semi-synthetic debris. We discuss the influence of fish characteristics, environmental factors and analytical methods, promoting consistency where possible. We comment on the benefits of using sardines as a global sentinel for monitoring marine anthropogenic debris and advocate for further research into their suitability. We also discuss features that can be addressed in future more comprehensive studies, particularly regarding sample size and particle characterisation.
Data Availability Statement
The datasets generated for this study are available on request to the corresponding author.
Ethics Statement
Ethical review and approval was not required for the animal study because the research was conducted on commercially caught fish cadavers.
Author Contributions
TC completed all laboratory and analytical processing. All authors contributed to the article and approved the submitted version.
Conflict of Interest
The authors declare that the research was conducted in the absence of any commercial or financial relationships that could be construed as a potential conflict of interest.
Acknowledgments
We acknowledge the analytical assistance provided by Bobby Pejcic of the Commonwealth Scientific and Industrial Research Organisation and Peta Clode of the Centre for Microscopy, Characterisation and Analysis. We also thank David Tunbridge and Renae Hovey of the University of Western Australia for respective technical support and manuscript reviews.
Supplementary Material
The Supplementary Material for this article can be found online at: https://www.frontiersin.org/articles/10.3389/fmars.2020.00526/full#supplementary-material
TABLE S1 | Criteria for each visually distinct particle group and the respective particle count for each.
References
Andrady, A. L. (2011). Microplastics in the marine environment. Mar. Pollut. Bull. 62, 1596–1605. doi: 10.1016/j.marpolbul.2011.05.030
Australian Bureau of Statistics (2016). 2016 Census QuickStats – Albany. Available online at: https://quickstats.censusdata.abs.gov.au/census_services/getproduct/census/2016/quickstat/5001?opendocument.
Barrows, A. P. W., Cathey, S. E., and Petersen, C. W. (2018). Marine environment microfiber contamination: global patterns and the diversity of microparticle origins. Environ. Pollut. 237, 275–284. doi: 10.1016/j.envpol.2018.02.062
Beaumont, N. J., Aanesen, M., Austen, M. C., Börger, T., Clark, J. R., Cole, M., et al. (2019). Global ecological, social and economic impacts of marine plastic. Mar. Pollut. Bull. 142, 189–195. doi: 10.1016/j.marpolbul.2019.03.022
Bergmann, M., Gutow, L., and Klages, M. (2015). Marine Anthropogenic Litter. Berlin: Springer, doi: 10.1007/978-3-319-16510-3
Bessa, F., Barría, P., Neto, J. M., Frias, J. P. G. L., Otero, V., Sobral, P., et al. (2018). Occurrence of microplastics in commercial fish from a natural estuarine environment. Mar. Pollut. Bull. 128, 575–584. doi: 10.1016/j.marpolbul.2018.01.044
Bredereck, K., and Hermanutz, F. (2008). Man-made cellulosics. Rev. Prog. Color. Relat. Top. 35, 59–75. doi: 10.1111/j.1478-4408.2005.tb00160.x
Browne, M. A., Crump, P., Niven, S. J., Teuten, E., Tonkin, A., Galloway, T., et al. (2011). Accumulation of microplastic on shorelines woldwide: sources and sinks. Environ. Sci. Technol. 45, 9175–9179. doi: 10.1021/es201811s
Cai, H., Du, F., Li, L., Li, B., Li, J., and Shi, H. (2019). A practical approach based on FT-IR spectroscopy for identification of semi-synthetic and natural celluloses in microplastic investigation. Sci. Total Environ. 669, 692–701. doi: 10.1016/j.scitotenv.2019.03.124
Cannon, S. M. E., Lavers, J. L., and Figueiredo, B. (2016). Plastic ingestion by fish in the Southern Hemisphere: a baseline study and review of methods. Mar. Pollut. Bull. 107, 286–291. doi: 10.1016/j.marpolbul.2016.03.057
Carbery, M., O’Connor, W., and Thavamani, P. (2018). Trophic transfer of microplastics and mixed contaminants in the marine food web and implications for human health. Environ. Int. 115, 400–409. doi: 10.1016/j.envint.2018.03.007
Cole, M., Lindeque, P., Fileman, E., Halsband, C., Goodhead, R., Moger, J., et al. (2013). Microplastic ingestion by zooplankton. Environ. Sci. Technol. 47, 6646–6655. doi: 10.1021/es400663f
Collard, F., Gilbert, B., Compère, P., Eppe, G., Das, K., Jauniaux, T., et al. (2017a). Microplastics in livers of european anchovies (Engraulis encrasicolus, L.). Environ. Pollut. 229, 1000–1005. doi: 10.1016/j.envpol.2017.07.089
Collard, F., Gilbert, B., Eppe, G., Roos, L., Compère, P., Das, K., et al. (2017b). Morphology of the filtration apparatus of three planktivorous fishes and relation with ingested anthropogenic particles. Mar. Pollut. Bull. 116, 182–191. doi: 10.1016/j.marpolbul.2016.12.067
Commonwealth of Australia (2009). Assessment of the South Australian Sardine Fishery. Canberra: Commonwealth of Australia.
Commonwealth of Australia (2018). Threat Abatement Plan for the Impacts of Marine Debris on the Vertebrate Wildlife of Australia’s Coasts and Oceans. Canberra: Commonwealth of Australia.
Comnea-Stancu, I. R., Wieland, K., Ramer, G., Schwaighofer, A., and Lendl, B. (2017). On the identification of rayon/viscose as a major fraction of microplastics in the marine environment: discrimination between natural and manmade cellulosic fibers using fourier transform infrared spectroscopy. Appl. Spectrosc. 71, 939–950. doi: 10.1177/0003702816660725
Compa, M., Ventero, A., Iglesias, M., and Deudero, S. (2018). Ingestion of microplastics and natural fibres in Sardina pilchardus (Walbaum, 1792) and Engraulis encrasicolus (Linnaeus, 1758) along the Spanish Mediterranean coast. Mar. Pollut. Bull. 128, 89–96. doi: 10.1016/j.marpolbul.2018.01.009
Cozar, A., Echevarria, F., Gonzalez-Gordillo, J. I., Irigoien, X., Ubeda, B., Hernandez-Leon, S., et al. (2014). Plastic debris in the open ocean. Proc. Natl. Acad. Sci. U.S.A. 111, 10239–10244. doi: 10.1073/pnas.1314705111
Cresswell, G. R., and Domingues, C. M. (2009). The leeuwin current south of western australia. J. R. Soc. West. Aust. 92, 83–100. doi: 10.1071/MF9930285
Critchell, K., and Hoogenboom, M. O. (2018). Effects of microplastic exposure on the body condition and behaviour of planktivorous reef fish (Acanthochromis polyacanthus). PLoS ONE 13:e0193308. doi: 10.4225/28/5a27312c231cc
Dehaut, A., Cassone, A. L., Frère, L., Hermabessiere, L., Himber, C., Rinnert, E., et al. (2016). Microplastics in seafood: benchmark protocol for their extraction and characterization. Environ. Pollut. 215, 223–233. doi: 10.1016/j.envpol.2016.05.018
Dehaut, A., Hermabessiere, L., and Duflos, G. (2019). Current frontiers and recommendations for the study of microplastics in seafood. Trends Anal. Chem. 116, 346–359. doi: 10.1016/j.trac.2018.11.011
Department of Environment Regulation (2016). Albany Wastewater Treatment Plant – Licence L6786/1991/11. Joondalup, WA: Department of Environment Regulation.
Edyvane, K. S., Dalgetty, A., Hone, P. W., Higham, J. S., and Wace, N. M. (2004). Long-term marine litter monitoring in the remote Great Australian Bight, South Australia. Mar. Pollut. Bull. 48, 1060–1075. doi: 10.1016/j.marpolbul.2003.12.012
Eriksen, M., Lebreton, L. C. M., Carson, H. S., Thiel, M., Moore, C. J., Borerro, J. C., et al. (2014). Plastic pollution in the World’s oceans: more than 5 trillion plastic pieces weighing over 250,000 tons Afloat at Sea. PLoS ONE 9:e111913. doi: 10.1371/journal.pone.0111913
FAO (2018). The State of World Fisheries and Aquaculture 2018 – Meeting the Sustainable Development Goals. Rome: FAO.
Ferreira, G. V. B., Barletta, M., Lima, A. R. A., Morley, S. A., Justino, A. K. S., and Costa, M. F. (2018). High intake rates of microplastics in a Western Atlantic predatory fish, and insights of a direct fishery effect. Environ. Pollut. 236, 706–717. doi: 10.1016/j.envpol.2018.01.095
Fletcher, W. (1990). A Synopsis of the Biology and the Exploitation of the Australasian Pilchard Sardinops neopilchardus (Steindachner) Part 1: Biology. East Perth, WA: Fisheries Dept. of Western Australia.
Foekema, E. M., De Gruijter, C., Mergia, M. T., Van Franeker, J. A., Murk, A. J., and Koelmans, A. A. (2013). Plastic in north sea fish. Environ. Sci. Technol. 47, 8818–8824. doi: 10.1021/es400931b
Garrido, S., Ben-Hamadou, R., Oliveira, P. B., Cunha, M. E., Chícharo, M. A., and Van Der Lingen, C. D. (2008). Diet and feeding intensity of sardine Sardina pilchardus: correlation with satellite-derived chlorophyll data. Mar. Ecol. Prog. Ser. 354, 245–256. doi: 10.3354/meps07201
GESAMP (2015). Sources, fate and effects of microplastics in the marine environment: a global assessment. Reports Stud. GESAMP 90:96.
GESAMP (2019). Guidelines for the monitoring and assessment of plastic litter in the ocean. GESAMP Reports Stud. 99:130.
Gewert, B., Plassmann, M. M., and Macleod, M. (2015). Pathways for degradation of plastic polymers floating in the marine environment. Environ. Sci. Process. Impacts 17, 1513–1521. doi: 10.1039/c5em00207a
Geyer, R., Jambeck, J. R., and Law, K. L. (2017). Production, use, and fate of all plastics ever made. Sci. Adv. 3:e1700782. doi: 10.1126/sciadv.1700782
Goldsworthy, S. D., Page, B., Rogers, P. J., Bulman, C., Wiebkin, A., McLeay, L. J., et al. (2013). Trophodynamics of the eastern Great Australian Bight ecosystem: ecological change associated with the growth of Australia’s largest fishery. Ecol. Modell. 255, 38–57. doi: 10.1016/j.ecolmodel.2013.01.006
Güven, O., Gökdaǧ, K., Jovanović, B., and Kideyş, A. E. (2017). Microplastic litter composition of the Turkish territorial waters of the Mediterranean Sea, and its occurrence in the gastrointestinal tract of fish. Environ. Pollut. 223, 286–294. doi: 10.1016/j.envpol.2017.01.025
Hajbane, S., and Pattiaratchi, C. B. (2017). Plastic pollution patterns in offshore, nearshore and estuarine waters: a case study from perth, Western Australia. Front. Mar. Sci. 4:63. doi: 10.3389/fmars.2017.00063
Hall, N. M., Berry, K. L. E., Rintoul, L., and Hoogenboom, M. O. (2015). Microplastic ingestion by scleractinian corals. Mar. Biol. 162, 725–732. doi: 10.1007/s00227-015-2619-7
Halstead, J. E., Smith, J. A., Carter, E. A., Lay, P. A., and Johnston, E. L. (2018). Assessment tools for microplastics and natural fibres ingested by fish in an urbanised estuary. Environ. Pollut. 234, 552–561. doi: 10.1016/j.envpol.2017.11.085
Hardesty, D., Reisser, J., Sharples, R., and Wilcox, C. (2011). “Understanding the types, sources, and at-sea distribution of marine debris in Australian waters,” in Proceedings of the The Fifth International Marine Debris Conference, Honolulu, HI.
Hidalgo-Ruz, V., Gutow, L., Thompson, R. C., and Thiel, M. (2012). Microplastics in the marine environment: a review of the methods used for identification and quantification. Environ. Sci. Technol. 46, 3060–3075. doi: 10.1021/es2031505
Izzo, C., Gillanders, B. M., and Ward, T. M. (2012). “Movement patterns and stock structure of Australian sardine (Sardinops sagax) off South Australia and the East Coast: implications for future stock assessment and management,” in Final Report to the Fisheries Research and Development Corporation. South Australian Research and Development Institute (Aquatic Sciences) (Adelaide: SARDI), 102.
Jambeck, J., Geyer, R., Wilcox, C., Siegler, T. R., Perryman, M., Andrady, A., et al. (2015). Marine pollution. Plastic waste inputs from land into the ocean. Science (80-) 347, 768–771. doi: 10.1126/science.1260352
Jensen, L. H., Motti, C. A., Garm, A. L., Tonin, H., and Kroon, F. (2019). Sources, distribution and fate of microfibres on the Great Barrier Reef, Australia. Sci. Rep. 9:9021. doi: 10.1038/s41598-019-45340-7
Kroon, F. J., Motti, C. E., Jensen, L. H., and Berry, K. L. E. (2018). Classification of marine microdebris: a review and case study on fish from the Great Barrier Reef, Australia. Sci. Rep. 8:16422. doi: 10.1038/s41598-018-34590-6
Kroon, F., Motti, C., Talbot, S., Sobral, P., and Puotinen, M. (2018). A workflow for improving estimates of microplastic contamination in marine waters: a case study from North-Western Australia. Environ. Pollut. 238, 26–38. doi: 10.1016/j.envpol.2018.03.010
Kühn, S., Bravo Rebolledo, E. L., and van Franeker, J. A. (2015). Deleterious Effects of Litter on Marine Life BT – Marine Anthropogenic Litter, eds M. Bergmann, L. Gutow, and M. Klages Cham: Springer International Publishing, 75–116. doi: 10.1007/978-3-319-16510-3_4
Kühn, S., van Werven, B., van Oyen, A., Meijboom, A., Bravo Rebolledo, E. L., and van Franeker, J. A. (2017). The use of potassium hydroxide (KOH) solution as a suitable approach to isolate plastics ingested by marine organisms. Mar. Pollut. Bull. 115, 86–90. doi: 10.1016/j.marpolbul.2016.11.034
Kumar, V. E., Ravikumar, G., and Jeyasanta, K. I. (2018). Occurrence of microplastics in fishes from two landing sites in Tuticorin, South east coast of India. Mar. Pollut. Bull. 135, 889–894. doi: 10.1016/j.marpolbul.2018.08.023
Lavers, J. L., and Bond, A. L. (2017). Exceptional and rapid accumulation of anthropogenic debris on one of the world’s most remote and pristine islands. Proc. Natl. Acad. Sci. U.S.A. 114, 6052–6055. doi: 10.1073/pnas.1619818114
Lefebvre, C., Saraux, C., Heitz, O., Nowaczyk, A., and Bonnet, D. (2019). Microplastics FTIR characterisation and distribution in the water column and digestive tracts of small pelagic fish in the Gulf of Lions. Mar. Pollut. Bull. 142, 510–519. doi: 10.1016/j.marpolbul.2019.03.025
Lithner, D., Larsson, A., and Dave, G. (2011). Environmental and health hazard ranking and assessment of plastic polymers based on chemical composition. Sci. Total Environ. 409, 3309–3324. doi: 10.1016/j.scitotenv.2011.04.038
Louw, G. G., van der Lingen, C. D., and Gibbons, M. J. (1998). Differential feeding by sardine Sardinops Sagax and anchovy Engraulis capensis recruits in mixed shoals. S. Afr. J. Mar. Sci. 19, 227–232. doi: 10.2989/025776198784126647
Lusher, A., Hollman, P., and Mandoza-Hill, J. (2017a). Microplastics in Fisheries and Aquaculture. 127. Available online at: http://www.fao.org/3/a-i7677e.pdf.
Lusher, A., Welden, N., Sobral, P., and Cole, M. (2017b). Sampling, isolating and identifying microplastics ingested by fish and invertebrates. Anal. Methods 9, 1346–1360. doi: 10.1039/c6ay02415g
Lusher, A., McHugh, M., and Thompson, R. (2013). Occurrence of microplastics in the gastrointestinal tract of pelagic and demersal fish from the English Channel. Mar. Pollut. Bull. 67, 94–99. doi: 10.1016/j.marpolbul.2012.11.028
Lusher, A. L., Tirelli, V., O’connor, I., and Officer, R. (2015). Microplastics in arctic polar waters: the first reported values of particles in surface and sub-surface samples. Sci. Rep. 5:14947. doi: 10.1038/srep14947
Miller, M. E., Kroon, F. J., and Motti, C. A. (2017). Recovering microplastics from marine samples: a review of current practices. Mar. Pollut. Bull. 123, 6–18. doi: 10.1016/j.marpolbul.2017.08.058
Mizraji, R., Ahrendt, C., Perez-Venegas, D., Vargas, J., Pulgar, J., Aldana, M., et al. (2017). Is the feeding type related with the content of microplastics in intertidal fish gut? Mar. Pollut. Bull. 116, 498–500. doi: 10.1016/j.marpolbul.2017.01.008
Mobsby, D. (2018). Australian Fisheries and Aquaculture Statistics 2017, Fisheries Research and Development Corporation project 2018-134. Canberra: ABARES, doi: 10.25814/5c07b19d3fec4
Montarsolo, A., Mossotti, R., Patrucco, A., Caringella, R., Zoccola, M., Pozzo, P., et al. (2018). Study on the microplastics release from fishing nets. Eur. Phys. J. Plus 133, 1–13. doi: 10.1140/epjp/i2018-12415-1
Naidoo, T., and Glassom, D. (2019). Decreased growth and survival in small juvenile fish, after chronic exposure to environmentally relevant concentrations of microplastic. Mar. Pollut. Bull. 145, 254–259. doi: 10.1016/j.marpolbul.2019.02.037
Nature Serve and Iucn (2020). Clupeiformes. The IUCN Red List of Threatened Species. Version 2017-3. Available online at: https://www.iucnredlist.org (accessed April 28, 2020).
Neves, D., Sobral, P., Ferreira, J. L., and Pereira, T. (2015). Ingestion of microplastics by commercial fish off the Portuguese coast. Mar. Pollut. Bull. 101, 119–126. doi: 10.1016/j.marpolbul.2015.11.008
Ory, N., Chagnon, C., Felix, F., Fernández, C., Ferreira, J. L., Gallardo, C., et al. (2018). Low prevalence of microplastic contamination in planktivorous fish species from the southeast Pacific Ocean. Mar. Pollut. Bull. 127, 211–216. doi: 10.1016/j.marpolbul.2017.12.016
Parrish, R. H., Grant, W. S., and Serra, R. (1989). The monotypic sardines, sardina and sardinops: their taxonomy, distribution, stock structure, and zoogeography. Can. J. Fish. Aquat. Sci. 46, 2019–2036. doi: 10.1139/f89-251
Paterson, H. L., and Dunlop, J. (2018). Minimal plastic in flesh-footed shearwater Ardenna carneipes burrows at southwestern Australia colonies. Mar. Ornithol. 46:165.
Patterson, H., Noriega, R., Georgeson, L., Larcombe, J., and Curtotti, R. (2017). Fishery Status Reports 2017. Canberra: Australian Bureau of Agricultural and Resource Economics and Sciences.
Pellini, G., Gomiero, A., Fortibuoni, T., Ferrà, C., Grati, F., Tassetti, N., et al. (2018). Characterization of microplastic litter in the gastrointestinal tract of Solea solea from the Adriatic Sea. Environ. Pollut. 234, 943–952. doi: 10.1016/j.envpol.2017.12.038
Pettit, N. E., Naiman, R. J., Fry, J. M., Roberts, J. D., Close, P. G., Pusey, B. J., et al. (2015). Environmental change: prospects for conservation and agriculture in a southwest Australia biodiversity hotspot. Ecol. Soc. 20:10. doi: 10.5751/ES-07727-200310
Provencher, J. F., Bond, A. L., Avery-Gomm, S., Borrelle, S. B., Bravo Rebolledo, E. L., Hammer, S., et al. (2017). Quantifying ingested debris in marine megafauna: a review and recommendations for standardization. Anal. Methods 9, 1454–1469. doi: 10.1039/C6AY02419J
Reisser, J., Shaw, J., Wilcox, C., Hardesty, B. D., Proietti, M., Thums, M., et al. (2013). Marine plastic pollution in waters around Australia: characteristics, concentrations, and pathways. PLoS ONE 8:e80466. doi: 10.1371/journal.pone.0080466
Remy, F., Collard, F., Gilbert, B., Compère, P., Eppe, G., and Lepoint, G. (2015). When microplastic is not plastic: the ingestion of artificial cellulose fibers by macrofauna living in seagrass macrophytodetritus. Environ. Sci. Technol. 49, 11158–11166. doi: 10.1021/acs.est.5b02005
Renzi, M., Specchiulli, A., Blašković, A., Manzo, C., Mancinelli, G., and Cilenti, L. (2018). Marine litter in stomach content of small pelagic fishes from the Adriatic Sea: sardines (Sardina pilchardus) and anchovies (Engraulis encrasicolus). Environ. Sci. Pollut. Res. Int. 26, 2771–2781. doi: 10.1007/s11356-018-3762-8
Rochman, C. M., Hoh, E., Kurobe, T., and Teh, S. J. (2013). Ingested plastic transfers hazardous chemicals to fish and induces hepatic stress. Sci. Rep. 3:3263. doi: 10.1038/srep03263
Roman, L., Paterson, H., Townsend, K. A., Wilcox, C., Hardesty, B. D., and Hindell, M. A. (2019). Size of marine debris items ingested and retained by petrels. Mar. Pollut. Bull. 142, 569–575. doi: 10.1016/j.marpolbul.2019.04.021
Romeo, T., Pietro, B., Pedà, C., Consoli, P., Andaloro, F., and Fossi, M. C. (2015). First evidence of presence of plastic debris in stomach of large pelagic fish in the Mediterranean Sea. Mar. Pollut. Bull. 95, 358–361. doi: 10.1016/j.marpolbul.2015.04.048
Rummel, C. D., Löder, M. G. J., Fricke, N. F., Lang, T., Griebeler, E. M., Janke, M., et al. (2016). Plastic ingestion by pelagic and demersal fish from the North Sea and Baltic Sea. Mar. Pollut. Bull. 102, 134–141. doi: 10.1016/j.marpolbul.2015.11.043
Shim, W. J., Hong, S. H., and Eo, S. E. (2017). Identification methods in microplastic analysis: a review. Anal. Methods 9, 1384–1391. doi: 10.1039/c6ay02558g
Silva, A., Santos, M. B., Caneco, B., Pestana, G., Porteiro, C., Carrera, P., et al. (2006). Temporal and geographic variability of sardine maturity at length in the northeastern Atlantic and the western Mediterranean. ICES J. Mar. Sci. 63, 663–676. doi: 10.1016/j.icesjms.2006.01.005
Smith, M., Love, D. C., Rochman, C. M., and Neff, R. A. (2018). Microplastics in Seafood and the Implications for Human Health. Curr. Environ. Heal. Rep. 5, 375–386. doi: 10.1007/s40572-018-0206-z
Smith, S. D. A., Gillies, C. L., and Shortland-Jones, H. (2014). Patterns of marine debris distribution on the beaches of Rottnest Island, Western Australia. Mar. Pollut. Bull. 88, 188–193. doi: 10.1016/j.marpolbul.2014.09.007
Sun, X., Li, Q., Shi, Y., Zhao, Y., Zheng, S., Liang, J., et al. (2019). Characteristics and retention of microplastics in the digestive tracts of fish from the Yellow Sea. Environ. Pollut. 249, 878–885. doi: 10.1016/j.envpol.2019.01.110
Thompson, R. C., Moore, C. J., vom Saal, F. S., and Swan, S. H. (2009). Plastics, the environment and human health: current consensus and future trends. Philos. Trans. R. Soc. B Biol. Sci. 364, 2153–2166. doi: 10.1098/rstb.2009.0053
UN Environment (2018). Addressing Marine Plastics: A Systemic Approach – Stocktaking report. Notten, P. Nairobi: United Nations Environment Programme.
United Nations (2018). UN Environment 2018 Annual Report. Nairobi:United Nations Environment Programme.
United Nations Environment Program (2018). Exploring the Potential for Adopting Alternative Materials to Reduce Marine Plastic Litter. Nairobi: United Nations Environment Programme. doi: 10.13140/RG.2.2.26075.87849
Van Cauwenberghe, L., Vanreusel, A., Mees, J., and Janssen, C. R. (2013). Microplastic pollution in deep-sea sediments. Environ. Pollut. 182, 495–499. doi: 10.1016/j.envpol.2013.08.013
van der Lingen, C. D. (1994). Effect of particle size and concentration on the feeding behaviour of adult pilchard Sardinops sagax. Mar. Ecol. Prog. Ser. 109, 1–14. doi: 10.3354/dao054001
Vegter, A. C., Barletta, M., Beck, C., Borrero, J., Burton, H., Campbell, M. L., et al. (2014). Global research priorities to mitigate plastic pollution impacts on marine wildlife. Endanger. Species Res. 25, 225–247. doi: 10.3354/esr00623
Zhao, S., Zhu, L., and Li, D. (2016). Microscopic anthropogenic litter in terrestrial birds from Shanghai, China: not only plastics but also natural fibers. Sci. Total Environ. 550, 1110–1115. doi: 10.1016/j.scitotenv.2016.01.112
Zhu, L., Wang, H., Chen, B., Sun, X., Qu, K., and Xia, B. (2019). Microplastic ingestion in deep-sea fish from the South China Sea. Sci. Total Environ. 677, 493–501. doi: 10.1016/j.scitotenv.2019.04.380
Keywords: plastic, ingestion, Sardinops sagax, Western Australia, FTIR, chemical characterisation, sardines
Citation: Crutchett T, Paterson H, Ford BM and Speldewinde P (2020) Plastic Ingestion in Sardines (Sardinops sagax) From Frenchman Bay, Western Australia, Highlights a Problem in a Ubiquitous Fish. Front. Mar. Sci. 7:526. doi: 10.3389/fmars.2020.00526
Received: 24 February 2020; Accepted: 09 June 2020;
Published: 30 June 2020.
Edited by:
Francois Galgani, Institut Français de Recherche pour l’Exploitation de la Mer (IFREMER), FranceReviewed by:
Mario Barletta, Federal University of Pernambuco, BrazilCecilia Martin, King Abdullah University of Science and Technology, Saudi Arabia
Copyright © 2020 Crutchett, Paterson, Ford and Speldewinde. This is an open-access article distributed under the terms of the Creative Commons Attribution License (CC BY). The use, distribution or reproduction in other forums is permitted, provided the original author(s) and the copyright owner(s) are credited and that the original publication in this journal is cited, in accordance with accepted academic practice. No use, distribution or reproduction is permitted which does not comply with these terms.
*Correspondence: Thomas Crutchett, dC5jcnV0Y2hldHRAZ21haWwuY29t