- 1School of Life Sciences, University of Essex, Colchester, United Kingdom
- 2Faculty of Science and Marine Environment, Universiti Malaysia Terengganu, Kuala Terengganu, Malaysia
The movement of sediment and associated microphytobenthos (MPB) between the upper mudflat and salt marsh in a macrotidal estuary was investigated by comparing the variability of benthic chlorophyll a (Chl. a) and suspended Chl. a during flood and ebb spring tides during the 2015 supermoon event. Sampling was carried out for 4 days in August and September. Flood-tide water carried significantly higher amounts of Chl. a from the mudflat transition zone onto the salt marsh compared to the amount of leaving the salt marsh during ebb tides. Suspended solid loads, suspended Chl. a concentrations, and diatom species composition provided evidence that resuspended mudflat sediments containing biofilm material were transferred onto the salt marsh by flood tide. Significant negative correlations between sediment Chl. a concentrations on the upper mudflat transition zone and Chl. a concentrations in flood-tide water indicated biostabilization of sediments by biofilms reducing sediment resuspension. Mean wind speed had a significant positive effect on resuspending Chl. a from the salt marsh sediment surface into the ebb tide (p < 0.001). The amount of Chl. a being resuspended in flood and ebb tidal waters was significantly correlated with MPB biomass on the sediment surface on the mudflat and salt marsh, respectively. Resuspended diatoms over the mudflat during high tide shared a total of 54.3% similar species with diatoms recorded in flood tidal water over the salt marsh. Diatom taxa characteristic of salt marsh assemblages, and some deposited diatom taxa were resuspended and carried off the salt marsh during ebb tide. Resuspension of Chl. a in both flood and ebb waters was significantly controlled by the tidal range (both significant at p < 0.001). During spring tides, there was a net movement of characteristic MPB mudflat diatom taxa and sediment from the adjacent mudflat to the salt marsh, contributing to the accumulation of material within vegetated marshes during summer months.
Introduction
Tidal flats are important coastal and estuarine habitats (Peterson and Peterson, 1979) that play a vital role in ecosystem services. The tidal flats occurring along coastlines are barriers to wave and wind energy (Scholz and Liebezeit, 2012; Leonardi et al., 2015). Tidal flats can be categorized by tide level into supratidal, intertidal, and subtidal zones. The sediment surface of intertidal flats (mudflats) and supratidal flats (salt marsh) are exposed to diurnal or semidiurnal cycles of tides. While mudflats are generally covered by all tides within the neap tide periods in North West Europe, vegetated salt marsh grows above the mean high water neap tide level (Beeftink and Rozema, 1988; de Leeuw et al., 1994) and so is only covered with seawater during spring tides. Upper salt marsh, located in the supratidal area and vegetated with herbs, grasses, and low shrubs (Xin et al., 2013), are only subjected to tidal immersion during the highest spring tides. This results in a greater range of environmental conditions within salt marsh sediments, with higher levels of desiccation, rainfall, temperature, and nutrient delivery through tidal water (Chesman et al., 2006). Salinity ranges of salt marshes are greater than on adjacent mudflats (McKew et al., 2011). Underwood (1997) reported a salinity range between 16 and 210 in salt marsh of the Blackwater Estuary, Essex, while adjacent mudflat salinity ranged between 22 and 39. Periods of tidal cover replenish salt marsh sediments with nutrients and reestablish seawater conditions.
Salt marshes provide a range of ecosystem functions, such as denitrification and nutrient biogeochemistry (Seitzinger, 1973; Ogilvie et al., 1997; Underwood, 1997; Cibic et al., 2007), primary production and carbon storage (Macreadie et al., 2013; Mueller et al., 2019), wave attenuation and coastal protection (Shepard et al., 2011; Yang et al., 2012), food provisioning (Grothues and Able, 2003; Banerjee et al., 2017), and cultural benefits that are important ecosystem services for human society. There has been significant research into how to protect, enhance, and restore salt marshes to preserve these functions in the face of changing environmental pressures on coasts (Reed et al., 2018). Salt marshes can trap and accrete sediment brought in on high tides and grow vertically in the tidal frame, enabling them to keep pace with historic rates of relative sea level rise (Horton and Sawai, 2010). Many salt marshes have a short cliff face between the salt marsh platform and the adjacent mudflat (Reed et al., 2018). Sediment block collapse (slumping) in this transition zone and the dynamics of wave action and the height differences between mudflat, transition zone, and salt marsh can cause lateral erosion of marshes, while providing a source of sediment for vertical growth (Green and Coco, 2014; Ladd et al., 2019). Wave energy, tidal range, flood–ebb tides, and spring-neap tidal cycles are potentially factors that affect the resuspension and deposition of sediment in the tidal flats (French and Spencer, 1993; French et al., 2009; Green, 2011; Reed et al., 2018). The patterns of lateral salt marsh growth or loss and vertical height accretion are therefore dependent on wave climate, local fetch, the supply of sediment to the adjacent mudflats, relative seal level changes, and a range of biological interactions that can stabilize or destabilize sediments (Green and Coco, 2014; Reed et al., 2018).
Microphytobenthic (MPB) biofilms (mixed assemblages of eukaryotic and prokaryotic microorganisms and associated extracellular mucilage) are important agents for biostablization on mudflat and salt marsh sediments, increasing the erosional shear stress needed for sediment to erode (Paterson, 1989; Spears et al., 2008). Microphytobenthos have been attributed as primary colonizers in succession (Coles, 1979) and are important primary producers and biogeochemical agents in coastal sediments. Resuspension of microphytobenthos occurs when erosive forces exceed the shear stress of the sediment bed, and MPB can be carried in the suspended sediment load and can resettle elsewhere in the estuarine system (Koh et al., 2006; Ubertini et al., 2012). MPB are more abundant on unvegetated mudflats than under the vegetation canopy of European salt marshes, but the deposition onto marshes provides a source of new cells, organic material, and sediments. Redzuan (2017) observed coupling between the MPB on the transition zone and the salt marsh in the Colne Estuary, United Kingdom, with higher species similarity between transition and salt marsh MPB assemblages during spring tides than in the neap tides. Some common mudflat MPB species such as Diploneis didyma, Pleurosigma angulatum, Surirella ovata, Gyrosigma scalproides, Gyrosigma wansbeckii, and Nitzschia sigma showed increased relative abundance on salt marsh sediments during spring tide.
The aim of this study was to investigate the transfer of sediment and MPB between the transition zone of mudflats and the adjacent salt marsh surface and the relationships between sediment movement and four abiotic factors: wind speed, tidal range, rainfall, and sun hours. We hypothesized that wind speed and tidal range would increase sediment movement, with lower rainfall and higher sun hours promoting biostabilization and reducing resuspension. In addition to measuring suspended solids and microphytobenthic biomass (Chl. a), we used diatom species composition as a biomarker indicating the source of resuspended sediment. We hypothesized that diatom taxa characteristic of mudflat MPB would be associated with the suspended sediment during flood tide and deposited on the salt marsh. If no resuspension of salt marsh sediment occurred at high tide, then the diatom composition in the ebb-tide water would reflect that of the flood-tide water. However, if salt marsh sediment resuspension did occur, the ebb-tide water would carry a diatom assemblage more typical of a salt marsh sediment diatom flora.
Materials and Methods
Sampling Strategy and Sample Processing
Two sampling surveys, involving four consecutive sampling days during the spring tide across the transition zone on the mudflat and the salt marsh, were carried out in August 2015 (survey 1, 30.08.15 to 02.09.15) and September 2015 (survey 2, 26.09.15 to 29.09.15) (Figure 1). The relatively shallow-water depth over the salt marsh surface even during spring tides can prevent successful deployment of sediment traps to collect any suspended sediment samples. Our study coincided with the “supermoon” event (the closest approaches the moon makes to the earth in its elliptical orbit), peaking on the 28th September 2015, which resulted in increased spring tide ranges (5.3–6.1 m) and greater flooding of the salt marshes in the Colne Estuary, enabling the collection of suspended sediment samples on the salt marsh.
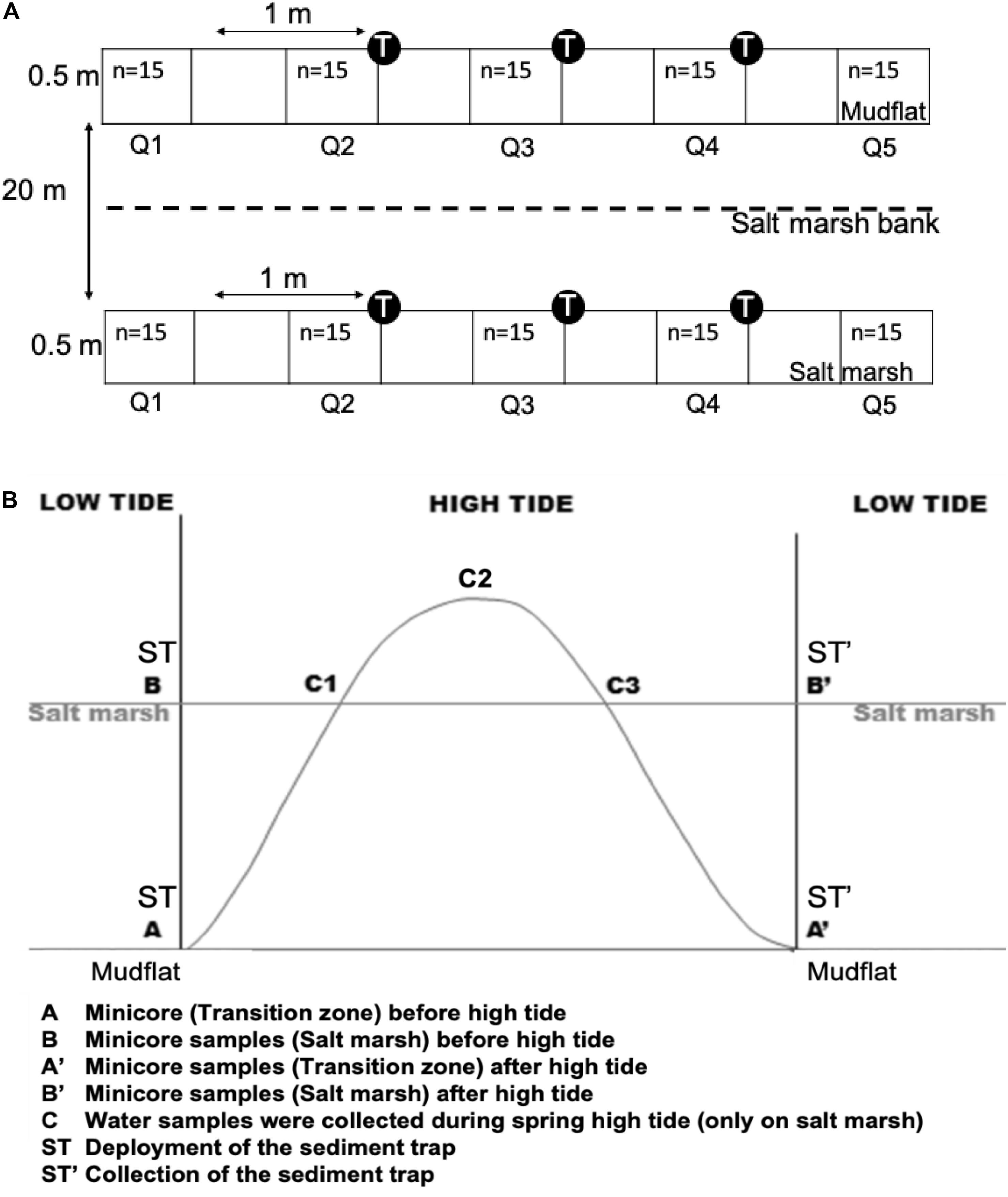
Figure 1. (A) Technical diagram of the plots (0.5 m × 4.5 m) built from five quadrats distanced at 0.5 m each, in which n = 15 samples were collected on mudflat and salt marsh. The n = 15 were pooled in the laboratory to make up triplicate samples for each quadrat, which then made up a sample size of n = 5 for each zone. T and Q, respectively, indicate sediment trap and quadrat; (B) sampling strategy carried out on the transition zone of mud flat (MF) and salt marsh (SM) at Fingringhoe Wick, Colne Estuary, Essex, United Kingdom, in August and September 2015. The sediment trap (ST) (n = 3) was deployed before the high tide and was collected after one period of tidal cover on each sampling day. C1 indicates water sample collection on flood and (C3) ebb tides. The unit of replication, n = 3.
Two sampling plots (0.5 m × 4.5 m, built of five 0.5 m × 0.5 m quadrats distanced at 0.5 m between each other and perpendicularly to the water channel, Figure 1A) were laid out, one on the upper transition zone of the mudflat and one of the salt marsh. The size of the plot was decided after taking into account time to deploy and to collect sediment traps and to obtain sediment samples during each tidal cycle (Figure 1B). Three different sets of data were obtained from the plot on each day of sampling (Supplementary Material A): (1) surface sediment Chl. a concentration (MPB biomass) before and after tidal cover; (2) suspended sediment and associated Chl. a concentrations in sediment traps after high tide; and (3) suspended sediment and Chl. a during flood and ebb tides over the salt marsh surface (see Supplementary Material A). Fifteen sediment surface samples (top 2 mm) were randomly obtained from each quadrat (n = 5) on the mudflat and salt marsh (twice, collected once before and once after one daytime high tide event) (Figure 1B) using minicores (20-ml syringe cut at the end with 2.836 cm2 diameter). Fifteen field replicates (n) were taken within each quadrat, as n = 12 proved to have the least sampling error at this spatial scale (Redzuan, 2017). The samples were immediately transferred into cold–dark containers and were preserved for Chl. a and colloidal carbohydrate (CC) analyses and MPB cell counts. Three sediment traps (inverted 850-mL plastic bottles with the bases removed, attached vertically to canes) were systematically placed along each plot (Figure 1A at a 1-m interval in each zone (Figure 1A). The suspended sediment samples in the traps were collected, labeled, and transferred into 1-liter bottles immediately after the high tide. Triplicate tidal water samples during flood tide (C1) and ebb tide (C3) were collected (only on salt marsh) into 500-ml bottles by hand to investigate the effect of tidal resuspension on water column Chl. a and CC concentrations and cell counts (Figure 1B). All samples were kept in cold and dark containers and returned to the laboratory for processing.
Sample Processing
In the laboratory, sediment samples from the fifteen collected minicores for each quadrat were pooled and mixed thoroughly, after which triplicate subsamples (n = 3) (technical replicates) were freeze dried for Chl. a (Lorenzen, 1967) and CC (Hanlon et al., 2006) analyses. Additionally, another triplicate set of sediment samples was collected and preserved in 0.5% w/v glutaraldehyde. These preserved samples were acid washed (Underwood, 1994) for permanent slide preparation for diatom cell counts.
Sediment trap water (250 ml) and tidal water samples (250 ml) were filtered using 12.5 and 4.5-cm GF/F Whatman filter paper, respectively. Tidal water samples were filtered through the smaller-size filter paper due to low sediment concentration (based on observation). The filtered sediments were freeze dried for Chl. a (Lorenzen, 1967) and CC (Hanlon et al., 2006) analyses. For permanent slide preparation and diatom cell count, a volume of 50 ml water sample from both the suspended and tidal water samples were taken after agitation of the main sample and transferred into plastic 15-ml centrifuge tubes. Samples were preserved with 0.5% w/v glutaraldehyde before going through an acid-washing procedure to make permanent diatom slides following Underwood (1994).
A total of 300 and 250 diatom valves were counted for each of the triplicate sediment surface samples for the mudflat and the salt marsh, respectively. Only 150 and 100 valves were counted for the triplicate suspended and tidal water samples, respectively, due to their low cell density. Each taxon was expressed in relative abundance (%).
Weather-Related Abiotic Factors
Four weather-related abiotic factors, the percentage of cloud cover (%), the tidal range (m), the mean wind speed (m s–1, averaged wind speed data for the 2 days preceding and on sampling day), and the sum of rainfall (mm, sum of rainfall of 2 days pre sampling and on the sampling day), were measured. Data for the cloud cover, sum of rainfall, and wind speed were obtained online on www.worldweatheronline.com/Colchester-weather-histor/Essex/GB.aspx. Tidal range data were obtained from Brightlingsea tide tables1. Cloud cover was chosen to indicate the potential light attenuation and also the weather (cloudy or sunny) at the study site.
Statistical Analyses
T-tests were performed using SPSS 25 to evaluate the variability in both Chl. a (log transformed) and CC (log transformed) concentrations in the top 2-mm sediment surface between before (LT 1) and after (LT 2) tidal immersion on the transition zone and the salt marsh. One-way ANOVA tests were used to investigate the variability of the Chl. a (log transformed) and CC (log transformed) in both the sediment surface and suspended in the water column among sampling days across the sampling surveys. Weather-related abiotic factors were analyzed using one-way ANOVA using day and survey as factors to determine their variability at both factor levels. Daily variability in Chl. a concentrations in both the flood and ebb tides (survey 1, 2) was investigated by using two-way ANOVA. The day and the sampling survey were treated as the factors for the ANOVA analyses; significant variability is reported at significance levels p < 0.05, p < 0.01, and p < 0.001.
Pearson correlation coefficients were used to determine the relationship between the measured variables (Chl. a, CC, suspended sediment Chl. a, and Chl. a in tidal water samples) with the weather-related abiotic factors.
Modified Morisita’s similarity index was used to calculate the percentage similarity between the MPB-diatom species composition on the transition zone and salt marsh sediment surfaces, in sediment traps (on transition zone) and in the flood and ebb-tide samples, using the Multi Variate Statistical Package (MVSP) 3.1 software (Kovach, 1999). Before the analyses, the overall data for each taxon were averaged and log10 n + 1 transformed. NMDS, ANOSIM, and SIMPER analyses were conducted on the diatom species-abundance Bray Curtis dissimilarity matrix, using R version 4.0 with the package vegan (Oksanen et al., 2019; R Core Team, 2020).
Results
Temporal Variability in Microphytobenthic and Suspended Biomass Related to Tides and Weather Factors
Chl. a concentrations in the top 2-mm sediment surface on the transition zone of the mudflat and salt marsh before (LT 1) and after (LT 2) the immersion period showed no significant differences across a flood–ebb cycle (Figures 2A,B). There were significant differences in the sediment CC concentration on the transition zone between the low tides (F1,60 = 235.2, p < 0.001) but no consistent pattern of being higher or lower before and after tidal cover (Figure 2C). The presence of fine particulate organic matter in the salt marsh sediments interfered with the carbohydrate assay, which meant it was not possible to determine accurate CC concentrations in the salt marsh sediments.
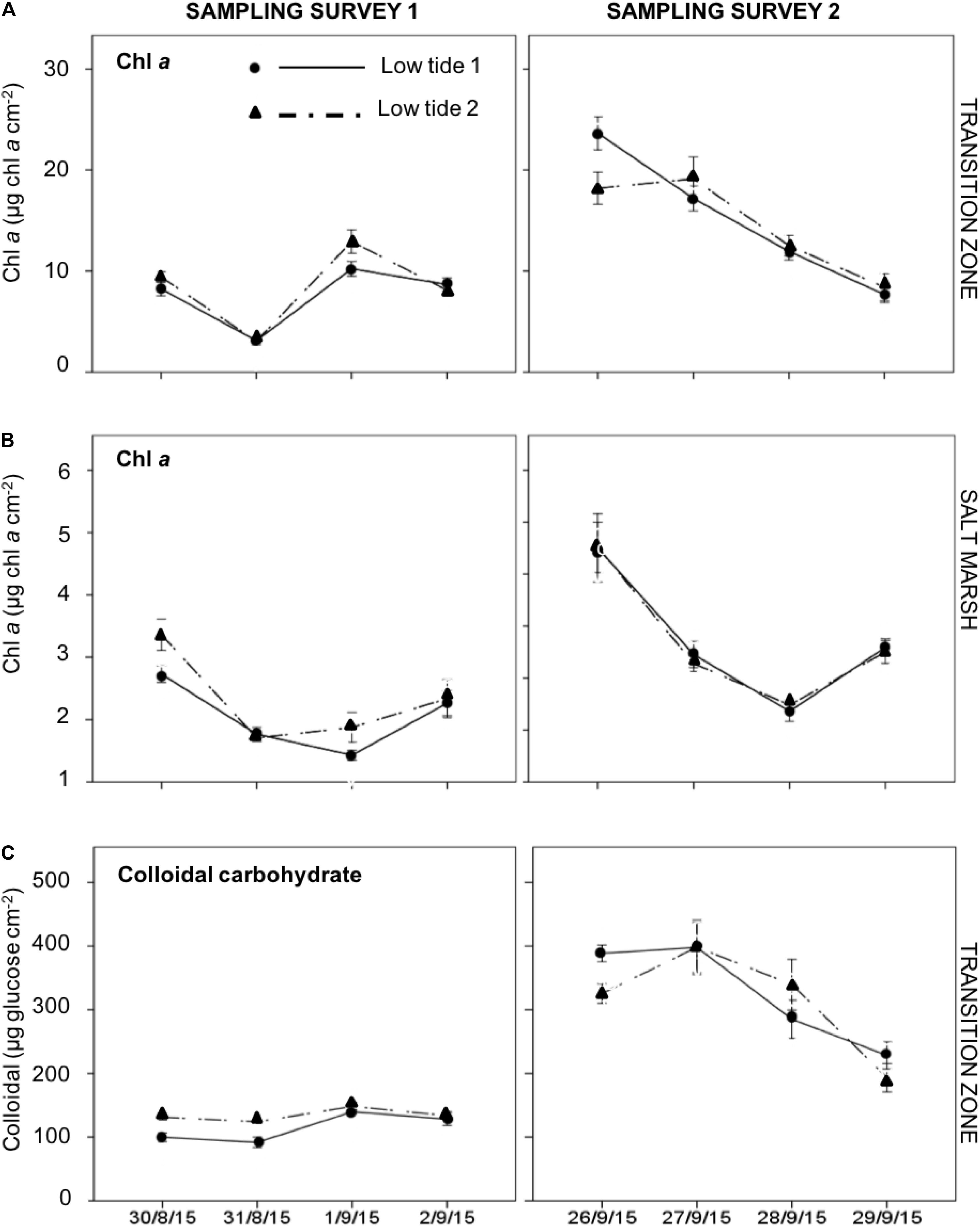
Figure 2. Daily changes in Chl a concentration (μg cm– 2) in the top 2-mm sediment surface on the; (A) transition zone of the mudflat; (B) salt marsh; and (C) colloidal carbohydrate on the transition zone, sampled during low tide 1 (LT1) and low tide 2 (LT2) in sampling survey 1 and survey 2 at Fingringhoe Wick, Colne Estuary, Essex, United Kingdom, in August and September 2015. Values are mean ± SE, n = 5.
Chl. a concentrations in the top 2-mm cm–2 sediment displayed strong temporal variation at daily time scales on both zones in both surveys (Figure 3; survey 1, F3,40 = 80.73, p < 0.001; survey 2, F3,40 = 29.34, p < 0.001) on the transition zone of the mudflat (Figure 3A). There was significantly higher mudflat sediment Chl. a during Survey 2 (overall average 14.8 ± 1.28 μg Chl. a cm–2) than during survey 1 (8.0 ± 0.6 μg Chl. a cm–2) (F1,80 = 26.693, p < 0.001). Chl. a concentrations on the salt marsh in both surveys showed a pattern of decrease followed by an increase after 3 days (Figure 2B; survey 1, F3,40 = 15.965, p < 0.01; survey 2 F3,40 = 38.173, p < 0.001). On the mudflat transition zone, there was a strong positive correlation (r = 0.812, p < 0.001) between the concentrations of Chl. a and CC across sampling days in both surveys (Figure 2 and Supplementary Material B).
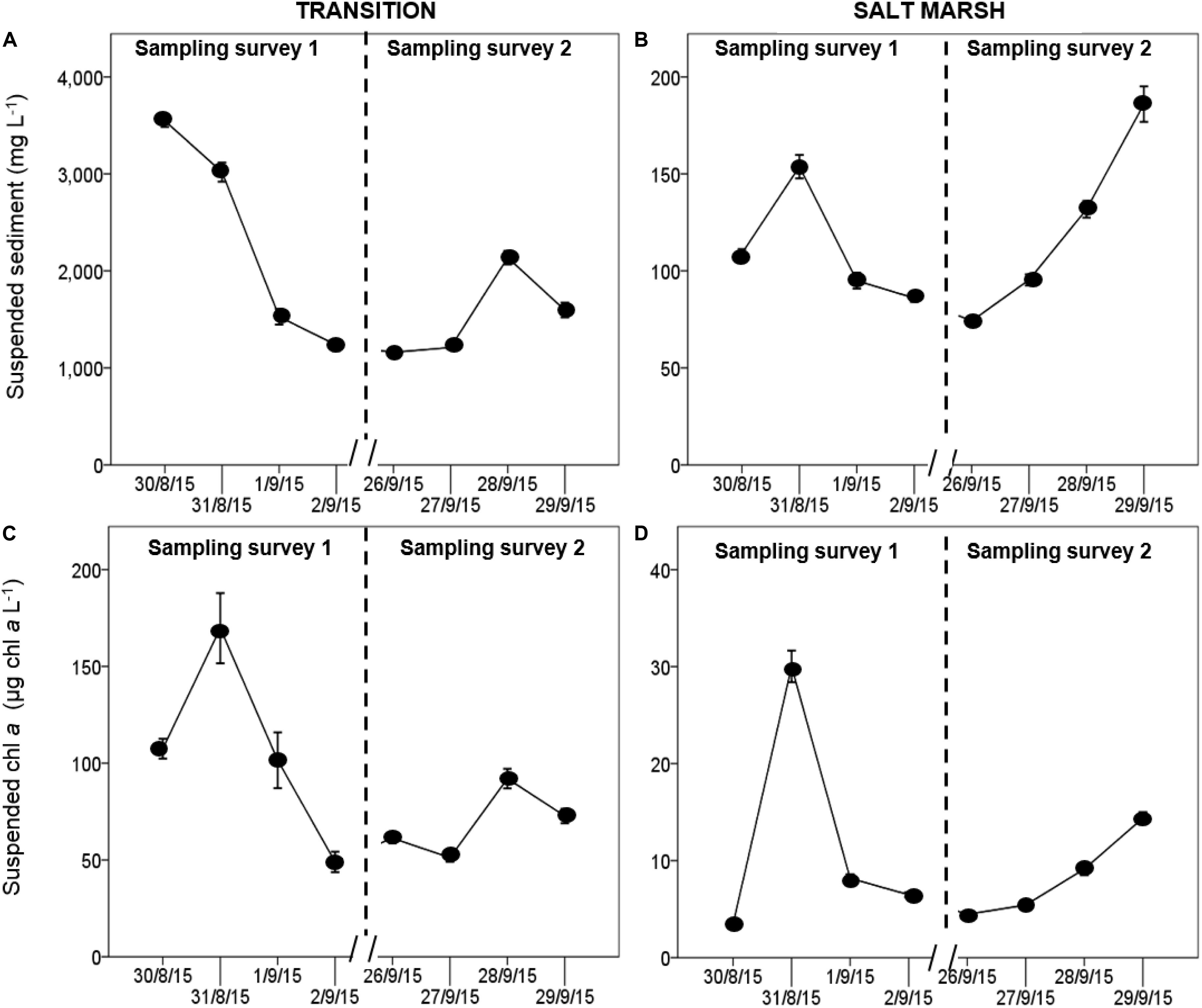
Figure 3. Daily changes in suspended sediment (A,B) and suspended sediment associated Chl. a (C,D) per L of water (obtained by sediment trap) over the transition zone of the mud flat and the salt marsh during two periods of sampling in August and September 2015 at Fingringhoe Wick, Colne Estuary, Essex, United Kingdom Values are mean ± SE, n = 3.
There were significant differences in the weather-related abiotic factors between days and between survey periods (Table 1). The mean sediment Chl. a concentration on the transition zone was significantly negatively correlated with all four abiotic factors, with cloud showing the strongest negative correlation (Table 2) (r value > −0.5). The mean Chl. a concentration on the salt marsh was negatively correlated (r > −0.5) with increasing tidal range (Table 2). Salt marsh Chl. a concentrations were also significantly negatively correlated with averaged wind speed for 3 days (r = −0.172, p < 0.05) and tidal range (r = −0.551, p < 0.001) (Table 2).
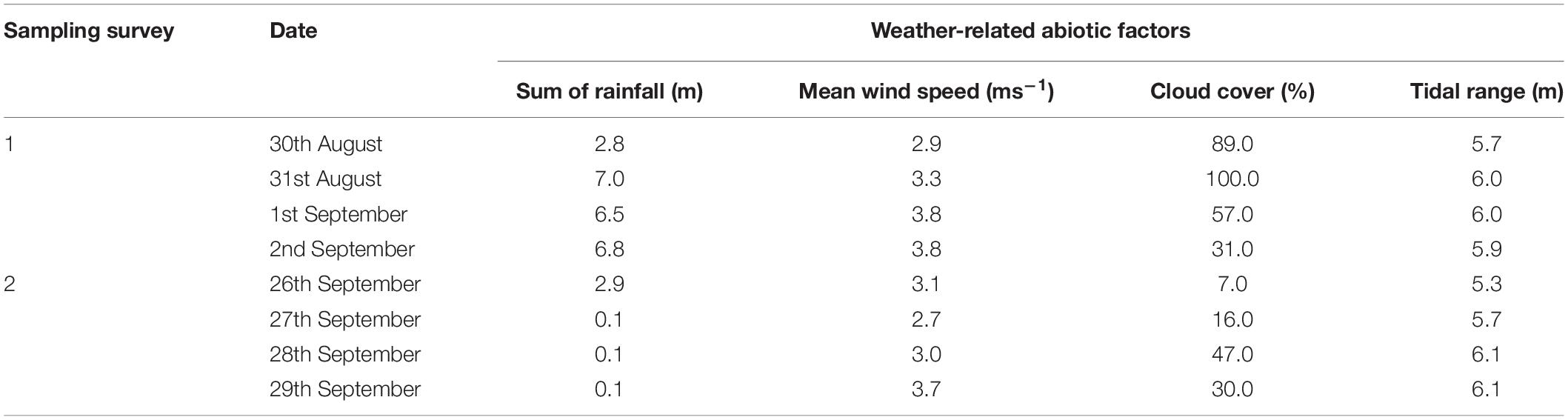
Table 1. Daily changes in the weather-related abiotic factors and tidal range during sampling surveys 1 and 2, at Fingringhoe Wick, Colne Estuary, Essex, United Kingdom, in 2015.

Table 2. Correlations between Chl. a concentration with weather-related abiotic factors and suspended water properties during August and September 2015 on two different intertidal zones at Fingringhoe Wick, Colne Estuary, Essex, United Kingdom.
There were significant negative correlations between sediment Chl. a concentrations and both suspended sediment load and suspended Chl. a in the water column during immersion (Table 2), with the strongest negative correlations on the transition zone and the lowest correlation coefficients for the marsh surface (Table 2). Suspended solid loads were high, exceeding 1,000 mg L–1 over the transition zone of the mudflat and between 100 and 200 mg L–1 on the salt marsh (Figure 3). Suspended Chl. a concentrations showed similar patterns with suspended sediment load (sediment trap data). There was significantly higher suspended sediment on the 30th and the 31st August (post hoc Tukey HSD, p < 0.001) (Figure 3A) and suspended Chl. a (post hoc Tukey HSD, p < 0.001) (Figure 3B) in the water column than other days. Suspended Chl. a concentrations in the water column over the salt marsh were significantly positively correlated with mean wind speed and with tidal range (Supplementary Material C), even if the high values from 31 Aug 2015 were discounted, (i.e., r = 0.40, p < 0.05 (mean wind speed); r = 0.7, p < 0.001 (tidal range).
MPB Coupling Between the Transition Zone (Mudflat) and the Salt Marsh
Chl. a concentrations over the salt marsh were significantly higher in the flood tidal water (23.90 ± 1.43 μg Chl. a L–1) than in the ebb [19.46 ± 0.89 μg Chl. a L–1, F1,48 = 7.105, p < 0.05 (overall data), Figure 4]. Only the Chl. a in the flood tide on the 1st and 2nd Sept 2015 showed lower values than the ebb, which was potentially closely linked to the weather condition (windy and rainy) on these two sampling days. The difference between the flood and ebb-tide concentrations of suspended Chl. a indicates retention of sediment within the salt marsh.
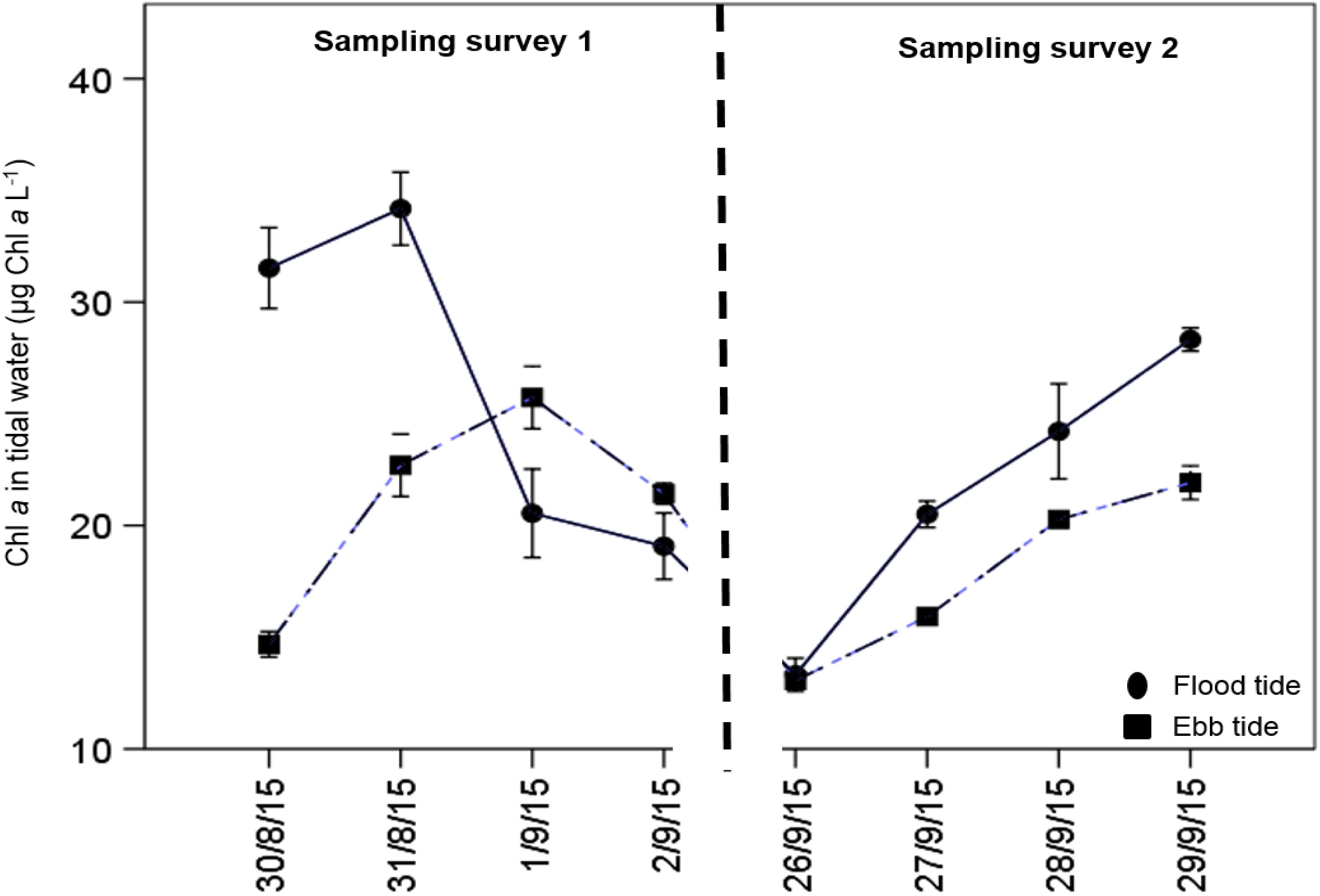
Figure 4. Daily variability in water column Chl. a in flood and ebb tidal water on the same tide over the salt marsh in both surveys 1 and 2 at Fingringhoe Wick, Colne Estuary, Essex, United Kingdom, in August and September 2015. Values are mean ± SE, n of tidal water = 3. The difference between the flood and ebb lines material retained on the marsh over the tidal cycle.
The water column Chl. a concentration in flood-tide water samples on the salt marsh was significantly negatively correlated with the Chl. a concentration on the transition zone of the mud flat sediment surface [r = −0.774, p < 0.001 (Figure 5A)]. The correlation was stronger than the correlation between flood tide Chl. a and Chl. a on the salt marsh (r = −0.410, p < 0.001) (Figure 5B). During ebb tides, there was evidence for the wash away of MPB from the salt marsh. The significantly higher salt marsh sediment Chl. a in survey 2 (2.71 ± 0.15 μg Chl. a cm–2) than in survey 1 (2.18 ± 0.10 μg Chl. a cm–2) was associated with relatively lower Chl. a in the ebb-tide water samples in the survey 2 (17.79 ± 1.07 μg Chl. a L–1) than in survey 1 (21.13 ± 1.29 μg Chl. a L–1). These data revealed the significant negative correlation between ebb-tide Chl. a concentrations and Chl. a in the top 2-mm sediment surface of the salt marsh (Figure 5B). The correlation coefficient was higher than for the correlation during flood tides (Figure 5A). During our period of study, rainfall had no significant effect on water column Chl. a concentrations in either flood- or ebb-tide water over the marsh. Increasing wind speed caused shallow-water mixing on the salt marsh surface (Figure 6A), resulting in increased suspended Chl. a load in ebb tides (Figure 6B). Cloud cover and tidal range were both positively correlated with flood-tide Chl. a concentration, with tidal range also influencing ebb-tide water concentrations (Figures 6C,D).
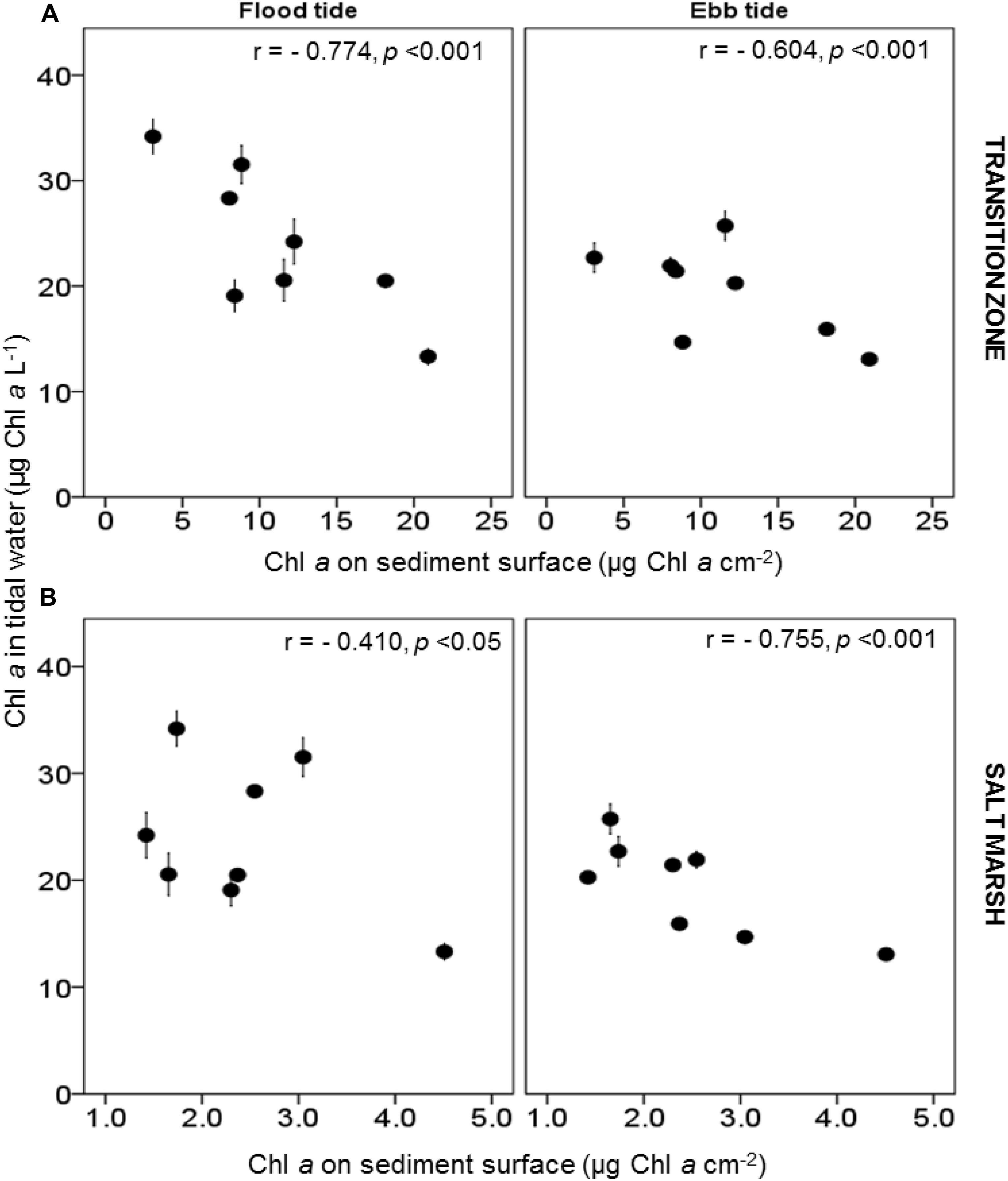
Figure 5. Relationship between Chl. a concentration (μg L–1) in flood and ebb-tide water (mean values ± SE, n = 3) with the Chl. a concentration in the top 2 mm per cm2 sediment surface of the (A) transition zone of the mudflat and; (B) salt marsh at Fingringhoe Wick, Colne Estuary, Essex, United Kingdom, in August and September 2015. Pearson’s correlation coefficients (r) on daily mean of flood and ebb data.
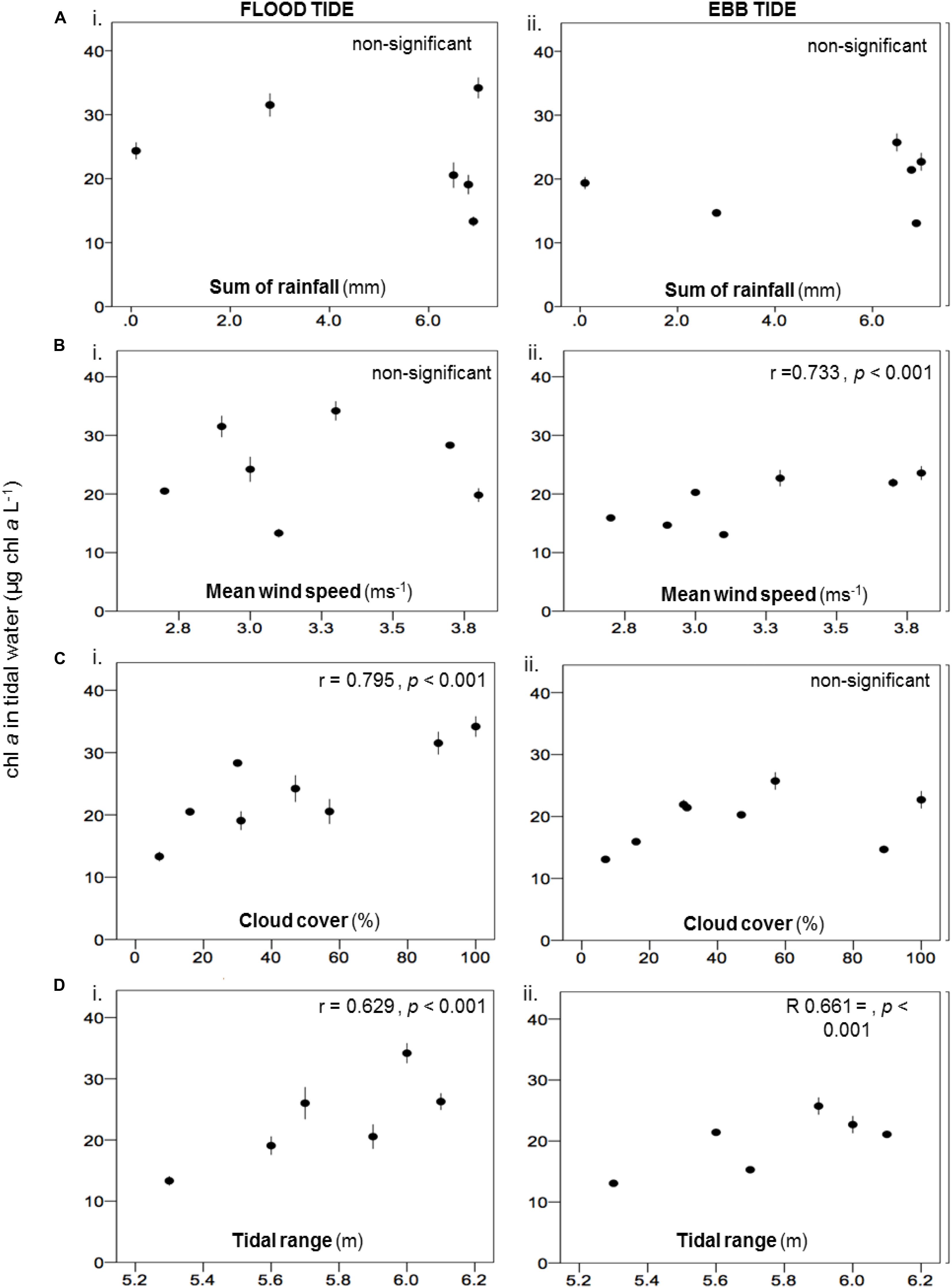
Figure 6. Relationship between water column Chl. a concentration (mean ± SE, n = 3) in (i) flood and (ii) ebb-tide water over the salt marsh with (A) sum of rainfall for 3 days; (B) averaged wind speed for 3 days; (C) percentage of cloud cover; and (D) the tidal range, during spring tides at Fingringhoe Wick, Colne Estuary, Essex, United Kingdom, in August and September 2015.
There were significant differences in the taxonomic composition of the diatom assemblages present on the mudflat and salt marsh and in the sediment traps and flood and ebb-tide waters (Figure 7A, ANOSIM, r = 0.989, p < 0.001). The mudflat flora (64 taxa) was characterized by a number of abundant large Gyrosigma and Pleurosigma species, Diploneis didyma, Synedra, and Nitzschia (SIMPER, p < 0.01 or less, Table 3). Eighteen of the 64 taxa identified on the mudflats were also found in the salt marsh assemblages (Table 3). Characteristic salt marsh taxa (34 taxa) included two Opephora species, Neidium sp., Nitzschia vitrea, and Surirella fastuosa (SIMPER, p < 0.01 or less).
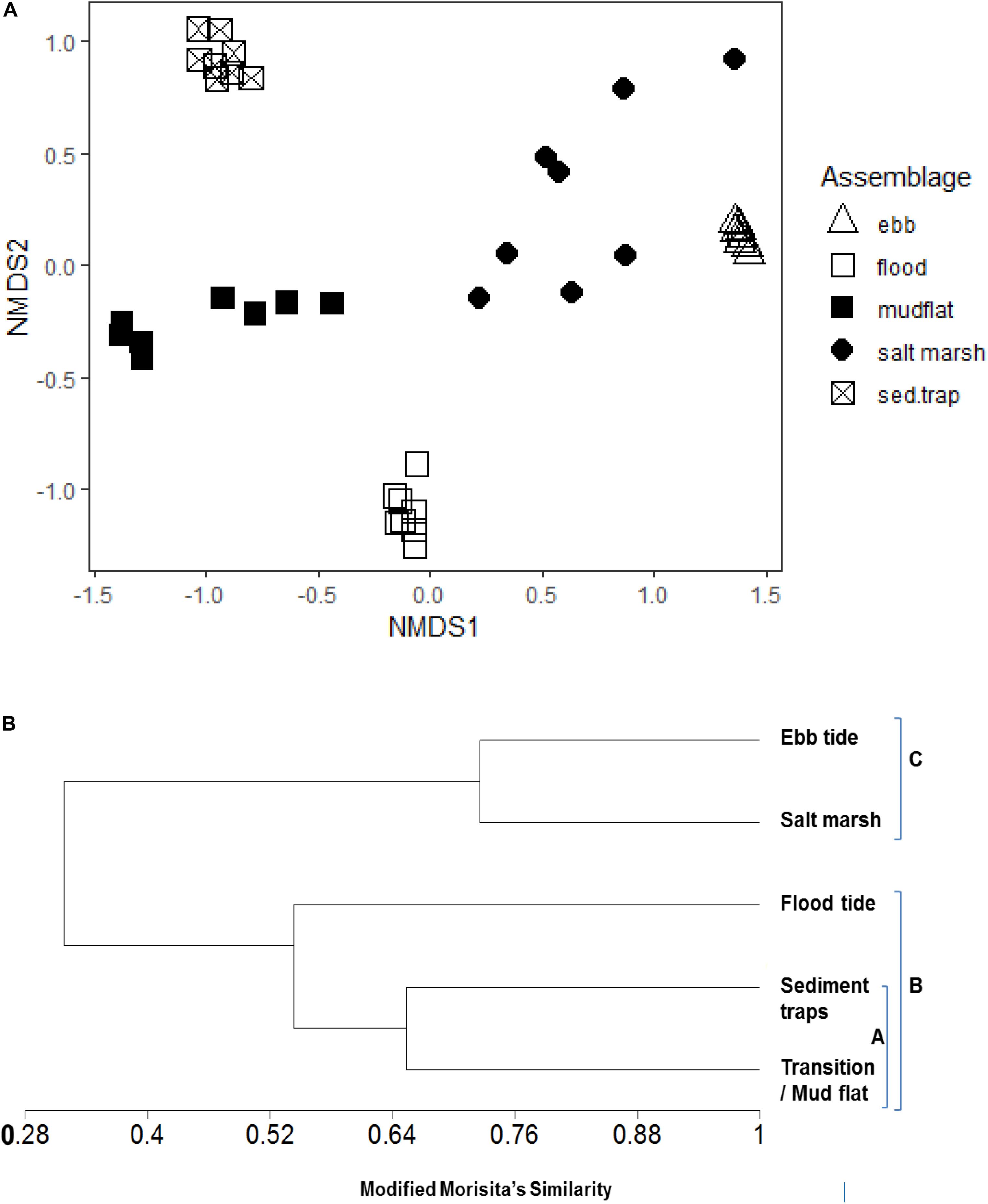
Figure 7. (A) NMDS plot of species composition of diatom assemblages (relative abundance) sampled from ebb-tide water, salt marsh sediment surface, flood-tide water, sediment traps (deployed on transition zone of the mudflat), and transition zone of the mudflat sediment surface samples. (B) Dendrogram showing% similarity (Modified Morista’s) in MPB diatom species composition for the five assemblage types.
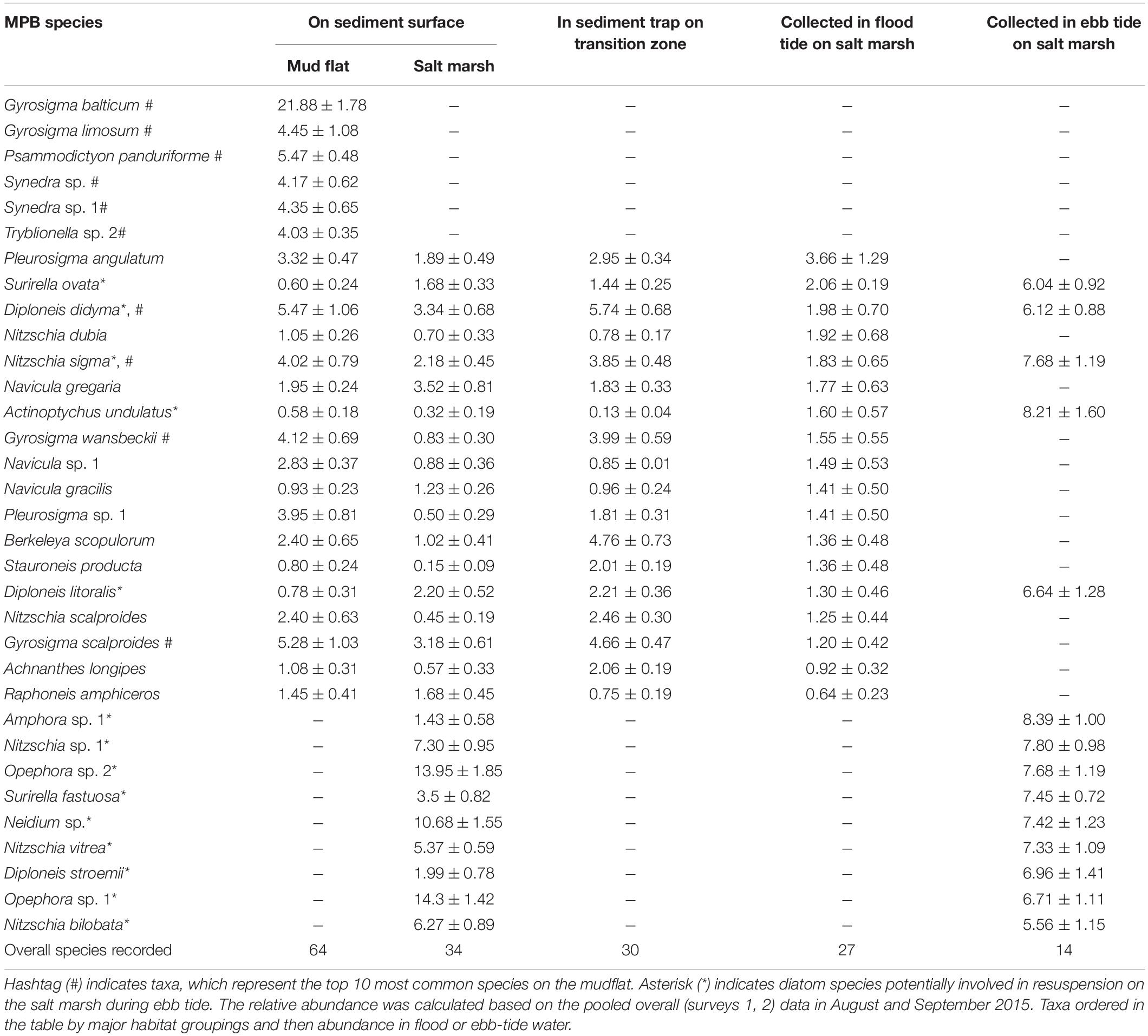
Table 3. Relative abundance ± SE (%) (n = 8) of MPB diatom species present in mudflat and salt marsh sediments and sampled in sediment traps and in flood and ebb-tide water at Fingringhoe Wick, Colne Estuary, Essex, United Kingdom.
There was 65.4% similarity in diatom community composition between the assemblages on the transition zone mudflats and those in sediment trap tide (Figure 7B) and a 54.3% assemblage similarity between the transition zone diatom assemblages (sediment and water) with the diatoms present in flood-tide samples over the salt marsh (Figure 7B). A number of abundant mudflat taxa, notably Gyrosigma balticum, G. limosum, Psammodictyon panduriforme, and the Synedra species were not found in the sediment traps or flood-tide samples or present in the salt marsh (Table 3). All the mudflat taxa present in the flood-tide water samples were also found on the salt marsh. In addition, there were a number of planktonic diatom genera found in the flood-tide samples (Coscinodiscus, Stephanodiscus, Actinoptychus, and Rhizosolenia, data not shown). The ebb-tide water column diatom assemblage (14 taxa) had 72.6% similarity with the species composition on the salt marsh sediment surfaces (Figures 7A,B) and was most dissimilar to the nudflat flora (Figure 7A). Eight taxa were found only in both the salt marsh and the ebb-tide water samples (Table 3). Overall, the species composition of Group B (characterized by MPB originating from the mudflat) shared a total of 31.8% similarity with MPB associated with salt marsh sediments and ebbing tides (Group C) (Figure 7B, Supplementary Material D). Five taxa that were abundant in the ebb-tide samples, Surirella ovata, Diploneis didyma, D. litoralis, Nitzschia sigma, and Actinoptychus undulatus, were also found in varying relative abundances in all the other four sample types (Table 3).
Discussion
This study did not find any significant reduction in MPB Chl. a concentration in the top 2-mm sediment surface before and after high-tide emersion periods during spring tides on both the transition and the salt marsh. Other studies in the Colne estuary have found significant “wash away” from mudflats during tidal cover (Bellinger et al., 2009; Taylor et al., 2013), and modeling shows this can be a significant process in MPB biomass dynamics on mudflats (Savelli et al., 2019). High levels of microspatial variability in MPB biomass (Spilmont et al., 2011; Taylor et al., 2013) may mask individual localized losses on any particular patch of sediment. Our sampling design integrated microspatial patchiness to look at the larger scale of meters. It is clear that some resuspension related to abiotic weather factors (particularly tidal range, wind, and cloud) did occur (Table 2) but that the mass lost was not sufficient to reduce the Chl a stocks remaining in the sediments. Koh et al. (2007) found a significant positive correlation between the daily mean of Chl. a concentration and both the Chl. a concentrations sampled at two different emersion periods of similar sampling day. There is a significant pool of microphytobenthic biomass within sediments, which undergoes micro-migration cycles (Underwood et al., 2005), so loss of some surface biomass may not be detectable against this larger Chl a signal. A strong contributor to reducing wash away is the presence of vertical migratory rhythms of MPB responding to tidal and diel conditions (Smith and Underwood, 1998; Underwood et al., 2005). Downward vertical migration has been reported to reduce the effect of MPB biofilm “wash away” by the tidal current (Fidalgo et al., 2002) and its associated wind (de Jonge and van Beusekom, 1995) and wave (Easley et al., 2005) energies during spring tide immersion (Riggs, 2002). During our period of study, wind speeds were relatively low and not sufficient to produce mass failure of the sediment bed. However, the diatom biomarkers suggest that there was differential removal of taxa under the conditions of our study. A number of large diatom taxa dominant on the mudflats were not found in the suspended sediments. Taxa such as G. balticum live in short mucilage tubes in sediments and are very active and rapid vertical migrators (Jönnson et al., 1994). Gyrosigma balticum also do not remain on the sediment surface throughout periods of tidal exposure (Underwood et al., 2005) but move down before tidal immersion, and other taxa show a similar behavior. Other taxa, such as Synedra and Psammodictyon are large cells and perhaps less likely to be resuspended, especially if they are bound into a mucilage matrix. So though some species avoided resuspension, a wide range of other mudflat taxa were present in the flood water and caught in sediment traps, for example Pleurosigma angulatum, which remains at the mudflat surface throughout periods of tidal exposure (Underwood et al., 2005). The high similarity in assemblage composition indicates that a transfer of biofilm material into the water column with incoming tides did occur.
The ability of MPB biofilms to reduce erosion by increasing the sediment critical erosion threshold has been reported in numerous studies (Paterson, 1989, 1994; Underwood and Paterson, 1993). This biostabilization is due in part to the production of mucilage (colloidal carbohydrates) that forms layers that protect the underlying sediment (Ubertini et al., 2012, 2015), and this is closely related to diatom biomass (Supplementary Material B). We found overall a negative relationship between sediment Chl a and water column Chl a (Table 2), indicating that denser biofilms reduce resuspension. It is also known that under higher light conditions, benthic diatoms will produce excess colloidal carbohydrate, increasing the potential for biostabilization (Perkins et al., 2001). The percentage of cloud cover was also positively correlated with increased suspended Chl. a concentrations in flood-tide samples on the salt marsh (Figures 6Ci,ii) and lower sediment Chl a concentrations. A higher percentage of cloud cover represents lower light conditions that may be partly responsible for lower reduce Chl. a concentrations (Serôdio et al., 2006) and reduced biostabilization (Gerbersdorf et al., 2009; Du et al., 2010) by MPB on the transition zone, facilitating resuspension. Other factors cannot be discounted, such as biofilm age, which can affect biostabilization potential, or varying sediment sizes (Ubertini et al., 2015). In our study, the sediments across the whole site are uniformly fine cohesive sediments, so there were no grain size changes. Other unmeasured biotic and abiotic factors may have influenced our results, demonstrating the many potential interactions influencing biofilm sediment processes in the field.
Lower Chl. a concentrations on the salt marsh sediment surface were associated with higher suspended sediment and suspended Chl. a concentration during immersion in spring tide (Table 2). Salt marsh biofilms are also more mixed in their species composition (Zong and Horton, 1998) and in this study were characterized by non-motile diatom taxa such as Opephora and Actinoptychus. Underwood (1997) reported that only when MPB assemblages consisted of more than 50% epipelic diatom on salt marsh was there a relationship between biomass and colloidal carbohydrate concentrations in the sediment surface. Therefore, the potential for biostabilization by MPB on salt marshes could be related to MPB biomass and species composition. Taxa like Opephora are widely distributed in estuaries across a range of sediment types (Sabbe and Vyverman, 1995), as part of the tychoplankton, and they can be resuspended and carried around with sediment (Underwood, 1994). Salt marsh plants slow water flow and enhance sediment deposition, trapping sediments around their roots and also on their leave surfaces (Mason et al., 2003; Reed et al., 2018; Ladd et al., 2019). This “trapping” of sediment may facilitate the deposition of those mudflat taxa also found in the salt marsh assemblage (Table 3).
Wind speed and tidal range during spring tides were the two abiotic factors that were important in MPB resuspension on the salt marsh. Loss of Chl. a from salt marsh biofilms is promoted by increasing tidal range (Horton et al., 2006). The negative effect of increased tidal range on Chl. a concentrations on the sediment surface is potentially associated with water depth and the wind speed (de Jonge and van Beusekom, 1995; van der Wal et al., 2010; Savelli et al., 2019). Wind speed is positively correlated with wave height at the study site (Redzuan, 2017), so that increases in suspended Chl. a and sediment concentrations on the salt marsh will be a product of water depth and wave energy (Leonardi et al., 2015). The wind speed values during this study were less that those found by de Jonge and van Beusekom (1995) to cause major resuspension, but even lower speeds can resuspend surface cells and sediments loosely associated in a “fluff” layer (Savelli et al., 2019). Such resuspension of biofilm material also weakens the potential for further biostabilization, leading to further resuspension (Ubertini et al., 2015). This chronic resuspension may play an important role in the gradual transfer of material from mudflats to salt marshes (as is clearly indicated by the diatom assemblage composition data), rather than major erosional and resuspension events, which also cause sediments to be lost from salt marshes (Ladd et al., 2019).
As hypothesized, greater tidal range positively increased suspended Chl. a concentrations and had a significant negative correlation with Chl. a concentrations on sediment surfaces of the salt marsh (Table 2). These two correlations further supported the occurrence of opposing influences on the salt marsh at our study site, which potentially caused the lower relative abundance of dominant salt marsh MPB species during spring tides (Redzuan, 2017). Tidal cover is reported to replenish resources such as nutrients on the salt marshes sediment (Blanchard et al., 2001), which may support MPB growth, but is also reported as one of major disturbance to MPB biofilms of the zone (Horton et al., 2006; Le Rouzic, 2012). It is clear that a number of characteristic salt marsh diatom taxa were resuspended and carried off the marsh in the ebb tides, indicating that some resuspension of salt marsh sediments did occur, even under the relatively benign summer weather conditions during this study.
Earlier work conducted in 2013 (Redzuan, 2017) highlighted that the diatom species composition in MPB assemblages on salt marsh, which mostly comprised small, “edaphic,” and epiphytic species during neap tides (Zong and Horton, 1998), was found to shift its assemblage composition toward diatom species common on the transition zone and the mudflat during spring tides. Characteristic mudflat taxa groups such as Pleurosigma, Gyrosigma, large Nitzschia spp., Diploneis, Navicula gregaria, and dominant on European mudflats (Underwood et al., 1998; Ribeiro et al., 2013) were found both in overlying water on the salt marsh and in marsh sediments. Suspended diatom assemblages in the flood-tide samples (sampled on salt marsh) were most similar to the transition zone MPB diatom assemblages (Figure 7), indicating that MPB coupling between the transition zone and the salt marsh occurs during flood tides. Flood-tide tidal currents have been reported by Koh et al. (2006) to cause simultaneous MPB resuspension on the mudflat, appearing to carry MPB biomass from the transition zone onto the salt marsh during the spring tide.
Chl. a concentrations on salt marsh sediment surfaces were negatively correlated with the suspended Chl. a in ebb and flood tides (Figure 6B). Species composition data showed that five out of the six dominant MPB species on the salt marsh sediment surface in neap tide were recorded in the ebb-tide tidal water samples (Table 3). The observation further supported the hypothesis that there was resuspension and possibly wash away of some epipelic, edaphic, and epiphytic salt marsh MPB species during ebb tides. Such taxa (e.g., Opephora) do not form EPS-rich biofilms that provide protection from resuspension or were more oligohaline to freshwater taxa, e.g., Neidium (Licursi et al., 2010). Disturbance during submersion in Spring tides has been shown to decrease in microphytobenthic biomass on the salt marshes (Le Rouzic, 2012).
Conclusion
This study confirmed that transfer of MPB biomass and sediments within a single mudflat and salt marsh tidal flat system can occur over individual tides in the absence of acute erosion events. Resuspension of MPB in both flood and ebb tidal waters strongly connected to spring tide tidal range. Biostabilization was implicated in controlling the amount of MPB resuspension on the mudflat transition zone and was closely linked to the availability of sunlight (based on the cloud cover percentage). Both flood and ebb tides resulted in exchanges of MPB between the zones. Weather-related abiotic factors, cloud cover and wind, were responsible for controlling the MPB exchange. Transfer of mudflat material was confirmed by the presence of particular diatom taxa and high levels of assemblage similarity, both on the flood tide (from the mudflat) and on the ebb tide (from the salt marsh). This present study was only carried out for 8 days in two different sampling surveys and covered non-storm event periods. During this time, a net movement of sediment from the mudflat onto the salt marsh was evident, which would support accretion of the marsh surface. Diatom assemblage analysis can act as a biomarker for that process. Further studies are needed to understand the exchange process of MPB over annual cycles that are influential in maintaining MPB biomass coupling in the estuarine ecosystem.
Data Availability Statement
The datasets generated for this study are available on request to the corresponding author.
Author Contributions
NR and GU designed the study and wrote the manuscript. NR conducted the fieldwork, laboratory, and data analyses. All authors contributed to the article and approved the submitted version.
Funding
NR was funded by the Malaysia Ministry of Higher Education and Universiti Malaysia Terengganu. This work was partly supported by the United Kingdom Natural Environment Research Council, Coastal Biodiversity and Ecosystem Services program (Ref NE/J01561X/1), to GU.
Conflict of Interest
The authors declare that the research was conducted in the absence of any commercial or financial relationships that could be construed as a potential conflict of interest.
Acknowledgments
We acknowledge excellent fieldwork and technical support provided by Mr. J. Green, Mr. R. Smart, and Mrs. T. Cresswell-Maynard.
Supplementary Material
The Supplementary Material for this article can be found online at: https://www.frontiersin.org/articles/10.3389/fmars.2020.00496/full#supplementary-material
Footnotes
References
Banerjee, K., Sappal, S., Ramachandran, P., and Ramachandran, R. (2017). Salt marsh: ecologically important, yet least studied blue carbon ecosystems in India. J. Clim. Chang. 3, 59–72. doi: 10.3233/JCC-170014
Beeftink, W. G., and Rozema, J. (1988). “The nature and functioning of salt marshes,” in Pollution of the North Sea: An Assessment, eds W. Salomons, B. L. Bayne, E. K. Duursma, and U. Förstner (Berlin: Springer), 59–87. doi: 10.1007/978-3-642-73709-1_4
Bellinger, B. J., Underwood, G. J. C., Ziegler, S. E., and Gretz, M. R. (2009). Significance of diatom-derived polymers in carbon flow dynamics within estuarine biofilms determined through isotopic enrichment. Aquat. Microb. Ecol. 55, 169–187. doi: 10.3354/ame01287
Blanchard, F., Guarini, J., Orvain, F., and Sauriau, P. (2001). Dynamic behaviour of benthic microalgal biomass in intertidal mudflats. Mar. Biol. Assoc. U. K. 82, 85–100. doi: 10.1016/s0022-0981(01)00312-4
Chesman, B. S., Burt, G. R., and Langston, W. J. (2006). Characterisation of european marine sites: essex estuaries, European marine site. J. Mar. Biol. Assoc. Spec. Publ. 17, 1–28.
Cibic, T., Blasutto, O., Falconi, C., and Umani, S. F. (2007). Microphytobenthic biomass, species composition and nutrient availability in sublittoral sediments of the gulf of trieste (Northern Adriatic Sea). Estuar. Coast. Shelf Sci. 75, 50–62. doi: 10.1016/j.ecss.2007.01.020
Coles, S. M. (1979). “Benthic microalgal populations on intertidal sediments and their role as precursors to salt marsh development,” in Ecological Processes In Coastal Environments: The First European Symposium Of The British Ecological Society, eds R. L. Jefferies and A. J. Davy (Oxford: Blackwell Scientific), 25–42.
de Jonge, V. N., and van Beusekom, J. E. E. (1995). Wind- and tide-induced resuspension of sediment and microphytobenthos from tidal flats in the Ems estuary. Limnol. Oceanogr. 40, 776–778. doi: 10.4319/lo.1995.40.4.0776
de Leeuw, J., Apon, L. P., Herman, P. M. J., de Munck, W., and Beeftink, W. G. (1994). The response of salt marsh vegetation to tidal reduction caused by the oosterschelde storm-surge barrier. Hydrobiologia 282, 335–353. doi: 10.1007/BF00024640
Du, G. Y., Oak, J.-H., Li, H., and Chung, I.-K. (2010). Effect of light and sediment grain size on the vertical migration of benthic diatoms. Algae 25, 133–140. doi: 10.4490/algae.2010.25.3.133
Easley, J. T., Hymel, S. N., and Plante, C. J. (2005). Temporal patterns of benthic microalgal migration on a semi-protected beach. Estuar. Coast. Shelf Sci. 64, 486–496. doi: 10.1016/j.ecss.2005.03.013
Fidalgo, E., Costa, P., Brotas, V., Cancela, and Da Fonseca, L. (2002). Physical characterisation and microphytobenthos biomass of estuarine and lagoon environments of the Southwest coast of Portugal. Limnetica 21, 69–80.
French, A. J. R., Spencer, T., Murray, A. L., Arnold, N. S., Small, T., and Wetlands, T. (2009). Coastal geostatistical analysis of sediment deposition in two small tidal Wetlands, Norfolk, UK. J. Coast. Res. 11, 308–321.
French, R., and Spencer, T. (1993). Dynamics of sedimentation in a tide-dominated backbarrier salt marsh, Norfolk, UK. Mar. Geol. 110, 315–331. doi: 10.1016/0025-3227(93)90091-9
Gerbersdorf, S. U., Westrich, B., and Paterson, D. M. (2009). Microbial extracellular polymeric substances (EPS) in fresh water sediments. Microb. Ecol. 58, 334–349. doi: 10.1007/s00248-009-9498-8
Green, M., and Coco, G. (2014). Review of wave-driven sediment resuspension and transport in estuaries. Rev. Geophys. 52:437. doi: 10.1002/2013RG000437
Green, M. O. (2011). Very small waves and associated sediment resuspension on an estuarine intertidal flat. Estuar. Coast. Shelf Sci. 93, 449–459. doi: 10.1016/j.ecss.2011.05.021
Grothues, T. M., and Able, K. W. (2003). Response of juvenile fish assemblages in tidal salt marsh creeks treated for phragmites removal. Estuaries 26, 563–573. doi: 10.1007/BF02823731
Hanlon, A. R. M., Bellinger, B., Haynes, K., Xiao, G., Hofmann, T. A., Gretz, M. R., et al. (2006). Dynamics of extracellular polymeric substance (EPS) production and loss in an estuarine, diatom-dominated, microalgal biofilm over a tidal emersion-immersion period. Limnol. Oceanogr. 51, 79–93. doi: 10.4319/lo.2006.51.1.0079
Horton, B. P., Corbett, R., Culver, S. J., Edwards, R. J., and Hillier, C. (2006). Modern saltmarsh diatom distributions of the Outer Banks, North Carolina, and the development of a transfer function for high resolution reconstructions of sea level. Estuar. Coast. Shelf Sci. 69, 381–394. doi: 10.1016/j.ecss.2006.05.007
Horton, B. P., and Sawai, Y. (2010). Diatoms as indicators of former sea levels, earthquakes, tsunamis, and hurricanes. Appl. Environ. Earth Sci. 8, 357–372. doi: 10.1017/CBO9780511763175.020
Jönnson, B., Sundbäck, K., and Nilsson, C. (1994). An upright life-form of an epipelic motile diatoms: on the behaviour of Gyrosigma balticum. Eur. J. Phycol. 29, 11–15. doi: 10.1080/09670269400650421
Koh, C. H., Jong, S. K., Araki, H., Yamanishi, H., Mogi, H., and Koga, K. (2006). Tidal resuspension of microphytobenthic chlorophyll a in a nanaura mudflat, Saga, Ariake Sea, Japan: flood-ebb and spring-neap variations. Mar. Ecol. Prog. Ser. 312, 85–100. doi: 10.3354/meps312085
Koh, C. H., Khim, J. S., Araki, H., Yamanishi, H., and Koga, K. (2007). Within-day and seasonal patterns of microphytobenthos biomass determined by co-measurement of sediment and water column Chlorophylls in the intertidal mudflat of Nanaura, Saga, Ariake Sea, Japan. Estuar. Coast. Shelf Sci. 72, 42–52. doi: 10.1016/j.ecss.2006.10.005
Ladd, C. J. T., Duggan-Edwards, M. F., Bouma, T. J., and Pagès, J. F. (2019). Sediment supply explains long-term and large-scale patterns in salt marsh lateral expansion and erosion. Geophys. Res. Lett. 46:315. doi: 10.1029/2019GL083315
Le Rouzic, B. (2012). Changes in photosynthetic yield (Fv/Fm) responses of salt-marsh microalgal communities along an osmotic gradient (Mont-Saint-Michel Bay, France). Estuar. Coast. Shelf Sci. 115, 326–333. doi: 10.1016/j.ecss.2012.09.012
Leonardi, N., Ganju, N. K., and Fagherazzi, S. (2015). A linear relationship between wave power and erosion determines salt-marsh resilience to violent storms and hurricanes. Proc. Natl. Acad. Sci. U.S.A. 113, 64–68. doi: 10.1073/pnas.1510095112
Licursi, M., Gómez, N., and Donadelli, J. (2010). Ecological optima and tolerances of coastal benthic diatoms in the freshwater-mixohaline zone of the Río de la Plata estuary. Mar. Ecol. Prog. Ser. 418, 105–117. doi: 10.3354/meps08865
Lorenzen, C. J. (1967). Determination of chlorophyll and pheo-pigments: Spectrophotometric equations. Limnol. Oceanogr. 12, 343–346. doi: 10.4319/lo.1967.12.2.0343
Macreadie, P. I., Hughes, A. R., and Kimbro, D. L. (2013). Loss of “Blue Carbon” from coastal salt marshes following habitat disturbance. PLoS One 8:e069244. doi: 10.1371/journal.pone.0069244
Mason, C. F., Underwood, G. J. C., Baker, N. R., Davey, P. A., Davidson, I., Hanlon, A., et al. (2003). The role of herbicides in the erosion of salt marshes in eastern England. Environ. Pollut. 122, 41–49. doi: 10.1016/S0269-7491(02)00284-1
McKew, B. A., Taylor, J. D., McGenity, T. J., and Underwood, G. J. C. (2011). Resistance and resilience of benthic biofilm communities from a temperate saltmarsh to desiccation and rewetting. ISME J. 5, 30–41. doi: 10.1038/ismej.2010.91
Mueller, P., Do, H. T., Jensen, K., and Nolte, S. (2019). Origin of organic carbon in the topsoil of Wadden Sea salt marshes. Mar. Ecol. Prog. Ser. 624, 39–50. doi: 10.3354/meps13009
Ogilvie, B., Nedwell, D. B., Harrison, R. M., Robinson, A., and Sage, A. (1997). High nitrate, muddy estuaries as nitrogen sinks: the nitrogen budget of the River Colne estuary (United Kingdom). Mar. Ecol. Prog. Ser. 150, 217–228. doi: 10.3354/meps150217
Oksanen, J., Blanchet, G., Friendly, M., Kindt, R., Legendre, P., McGlinn, D., et al. (2019). Vegan: Community Ecology Package. R Package Version 2.5-6.
Paterson, D. M. (1994). “Microbiological mediation of sediment structure and behaviour,” in Microbial Mats NATO ASI Series (Series G: Ecological Sciences), Vol. 35, eds L. J. Stal and P. Caumette (Berlin: Springer), 97–109.
Paterson, D. M. (1989). Short-term changes in the erodibility of intertidal cohesive sediments related to the migratory behavior of epipelic diatoms. Limnol. Oceanogr. 34, 223–234. doi: 10.4319/lo.1989.34.1.0223
Perkins, R. G., Underwood, G. J. C., Brotas, V., Snow, G. C., Jesus, B., and Ribeiro, L. (2001). Responses of microphytobenthos to light: primary production and carbohydrate allocation over an emersion period. Mar. Ecol. Prog. Ser. 223, 101–112. doi: 10.3354/meps223101
Peterson, H., and Peterson, N. M. (1979). The Ecology of Intertidal Flats of North Carolina: A Community Profile. Slidell, LA: United States Fish and Wildlife Service, Office of Biological Services, 73.
R Core Team (2020). R: A Language And Environment For Statistical Computing. Vienna: R Foundation for Statistical Computing.
Redzuan, N. S. (2017). Microphytobenthos (MPB) Biomass Variability And Sediment-Water Column Exchanges On An Intertidal Flat: Influence Of Weather-Related Abiotic Factors Across Neap-Spring-Neap Tidal Cycle. Ph. D. thesis, University of Essex, Colchester.
Reed, D., van Wesenbeeck, B., Herman, P. M. J., and Meselhe, E. (2018). Tidal flat-wetland systems as flood defenses: understanding biogeomorphic controls. Estuar. Coast. Shelf Sci. 213, 269–282. doi: 10.1016/j.ecss.2018.08.017
Ribeiro, L., Brotas, V., Rincé, Y., and Jesus, B. (2013). Structure and diversity of intertidal benthic diatom assemblages in contrasting shores: a case study from the Tagus estuary. J. Phycol. 49, 258–270. doi: 10.1111/jpy.12031
Riggs, S. R. (2002). “Life at the edge of North Carolina’s coastal system: the geo- logic controls,” in Life at the Edge of the Sea: Essays on North Carolina’s Coast and Coastal Culture, eds C. Beal and C. Prioli (Wilmington, NC: Coastal Carolina Press), 63–95.
Sabbe, K., and Vyverman, W. (1995). Taxonomy, morphology and ecology of some widespread representatives of the diatom genus opephora. Eur. J. Phycol. 30, 235–249. doi: 10.1080/09670269500651011
Savelli, R., Bertin, X., Orvain, F., Gernez, P., Dale, A., Coulombier, T., et al. (2019). Impact of chronic and massive resuspension mechanisms on the microphytobenthos dynamics in a temperate intertidal mudflat. JGR Biogeosci. 124, 3752–3777. doi: 10.1029/2019JG005369
Scholz, B., and Liebezeit, G. (2012). Microphytobenthic dynamics in a Wadden Sea intertidal flat – Part II: seasonal and spatial variability of non-diatom community components in relation to abiotic parameters. Eur. J. Phycol. 47, 120–137. doi: 10.1080/09670262.2012.665251
Seitzinger, S. P. (1973). Denitrification in freshwater and coastal marine ecosystems: ecological and geochemical significance. Limnol. Oceanogr. 33, 702–724. doi: 10.4319/lo.1988.33.4part2.0702
Serôdio, J., Coelho, H., Vieira, S., and Cruz, S. (2006). Microphytobenthos vertical migratory photoresponse as characterised by light-response curves of surface biomass. Estuar. Coast. Shelf Sci. 68, 547–556. doi: 10.1016/j.ecss.2006.03.005
Shepard, C. C., Crain, C. M., and Beck, M. W. (2011). The protective role of coastal marshes: a systematic review and meta-analysis. PLoS One 6:e027374. doi: 10.1371/journal.pone.0027374
Smith, D. J., and Underwood, G. J. C. (1998). Exopolymer production by intertidal epipelic diatoms. Limnol. Oceanogr. 43, 1578–1591. doi: 10.4319/lo.1998.43.7.1578
Spears, B. M., Saunders, J. E., Davidson, I., and Paterson, D. M. (2008). Microalgal sediment biostabilisation along a salinity gradient in the Eden Estuary, Scotland: unravelling a paradox. Mar. Freshw. Res. 59, 313–321. doi: 10.1071/MF07164
Spilmont, N., Seuront, L., Meziane, T., and Welsh, D. T. (2011). There’s more to the picture than meets the eye: sampling microphytobenthos in a heterogeneous environment. Estuar. Coast. Shelf Sci. 95, 470–476. doi: 10.1016/j.ecss.2011.10.021
Taylor, J. D., McKew, B. A., Kuhl, A., McGenity, T. J., and Underwood, G. J. C. (2013). Microphytobenthic extracellular polymeric substances (EPS) in intertidal sediments fuel both generalist and specialist EPS-degrading bacteria. Limnol. Oceanogr. 58, 1463–1480. doi: 10.4319/lo.2013.58.4.1463
Ubertini, M., Lefebvre, S., Gangnery, A., Grangeré, K., Le Gendre, R., and Orvain, F. (2012). Spatial variability of benthic-pelagic coupling in an estuary ecosystem: consequences for microphytobenthos resuspension phenomenon. PLoS One 7:e044155. doi: 10.1371/journal.pone.0044155
Ubertini, M., Lefebvre, S., Rakotomalala, C., and Orvain, F. (2015). Impact of sediment grain-size and biofilm age on microphytobenthos resuspension. J. Exp. Mar. Biol. Ecol. 467, 52–64. doi: 10.1016/j.jembe.2015.02.007
Underwood, G. J. C. (1994). Seasonal and spatial variation n epipelic diatom assemblages in the Severn estuary. Diatom Res. 9, 451–472. doi: 10.1080/0269249x.1994.9705319
Underwood, G. J. C. (1997). Microalgal colonization in a saltmarsh restoration scheme. Estuar. Coast. Shelf Sci. 44, 471–481. doi: 10.1006/ecss.1996.0138
Underwood, G. J. C., Hanlon, A. R. M., Oxborough, K., and Baker, N. R. (2005). Patterns in microphytobenthic primary productivity: species-specific variation in migratory rhythms and photosynthetic efficiency in mixed-species biofilms. Am. Soc. Limnol. Oceanogr. 50, 755–767. doi: 10.4319/lo.2005.50.3.0755
Underwood, G. J. C., and Paterson, D. M. (1993). Recovery of intertidal benthic diatoms after biocide treatment and associated sediment dynamics. J. Marine Biol. Assoc. UK. 73, 25–45. doi: 10.1017/S002531540003263X
Underwood, G. J. C., Phillips, J., and Saunders, K. (1998). Distribution of estuarine benthic diatom species along salinity and nutrient gradients. Eur. J. Phycol. 33, 173–183. doi: 10.1080/09670269810001736673
van der Wal, D., Wielemaker-van den Dool, A., and Herman, P. M. J. (2010). Spatial synchrony in intertidal benthic algal biomass in temperate coastal and estuarine ecosystems. Ecosystems 13, 338–351. doi: 10.1007/s10021-010-9322-9
Xin, P., Li, L., and Barry, D. A. (2013). Tidal influence on soil conditions in an intertidal creek-marsh system. Water Resour. Res. 49, 137–150. doi: 10.1029/2012WR012290
Yang, S. L., Shi, B. W., Bouma, T. J., Ysebaert, T., and Luo, X. X. (2012). Wave attenuation at a salt marsh margin: a case study of an exposed coast on the yangtze estuary. Estuar. Coast. 35, 169–182. doi: 10.1007/s12237-011-9424-4
Keywords: microphytobenthos, diatom species, suspended sediment, suspended Chl. a, flood tide, ebb tide
Citation: Redzuan NS and Underwood GJC (2020) Movement of Microphytobenthos and Sediment Between Mudflats and Salt Marsh During Spring Tides. Front. Mar. Sci. 7:496. doi: 10.3389/fmars.2020.00496
Received: 30 November 2019; Accepted: 03 June 2020;
Published: 07 July 2020.
Edited by:
David M. Paterson, University of St Andrews, United KingdomReviewed by:
Clarisse Mallet, UMR 6023 Laboratoire Microorganismes Génome et Environnement (LMGE), FranceLourenço Soares Ribeiro, Center for Marine and Environmental Sciences (MARE), Portugal
Copyright © 2020 Redzuan and Underwood. This is an open-access article distributed under the terms of the Creative Commons Attribution License (CC BY). The use, distribution or reproduction in other forums is permitted, provided the original author(s) and the copyright owner(s) are credited and that the original publication in this journal is cited, in accordance with accepted academic practice. No use, distribution or reproduction is permitted which does not comply with these terms.
*Correspondence: Nurul S. Redzuan, nurulshahida@umt.edu.my