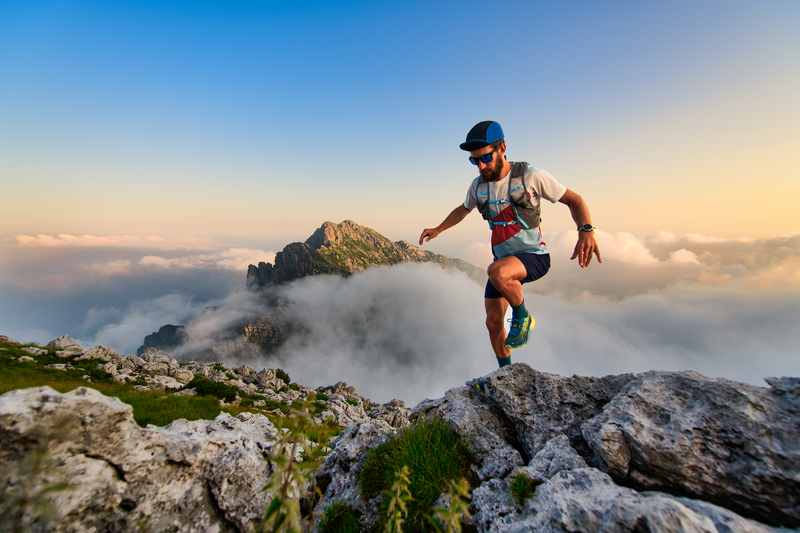
94% of researchers rate our articles as excellent or good
Learn more about the work of our research integrity team to safeguard the quality of each article we publish.
Find out more
ORIGINAL RESEARCH article
Front. Mar. Sci. , 23 June 2020
Sec. Marine Biogeochemistry
Volume 7 - 2020 | https://doi.org/10.3389/fmars.2020.00478
Many regions of Antarctica are classified as high nutrient low chlorophyll (HNLC) areas. In these, iron availability is limiting primary productivity and subsequent carbon export. Domoic acid (DA) has previously been detected in the Southern Ocean and suggested to act as a ligand that facilitates iron assimilation for Pseudo-nitzschia spp., species that contribute to Antarctic diatom blooms. An incubation experiment using the Antarctic species Pseudo-nitzschia subcurvata was performed in Antarctic seawater at low and high iron concentrations. Dissolved DA was added to one set of each of the two treatments. This was done to verify whether DA positively affects the growth of the non-toxic species Pseudo-nitzschia subcurvata and increases its cellular iron content, particularly under low iron conditions. We hypothesize that (i) DA is taken up under low iron conditions (ii) that more iron is taken up if DA is available and (iii) that the growth rate increases in the presence of DA. We showed that P. subcurvata did not take up any added DA, even under low iron conditions. Additionally and contrary to our hypothesis, the cells were not positively influenced by the addition of dissolved DA in terms of growth rate, cellular iron and carbon content. Hence, there was no significant difference in iron content between the different treatments. However, the cellular copper content increased under low iron conditions when DA was added. This study suggests that dissolved DA in naturally occurring concentrations does not increase bioavailability of iron to P. subcurvata and that only species producing DA might benefit from it.
Marine and terrestrial primary production, similarly, contribute to global net primary production (Field et al., 1998). Phytoplankton fix marine carbon (Falkowski, 1994), a process which also requires nitrogen and phosphorous (Redfield, 1958), as well as trace elements such as iron, manganese, zinc, copper, cadmium, cobalt, and molybdenum (Quigg et al., 2003). Iron is a particularly important trace element as it is involved in many metabolic pathways including respiration and photosynthesis (Geider and La Roche, 1994). Many areas of the Southern Ocean (SO) are rich in macronutrients, but harbor low primary productivity making them so-called high nutrient low chlorophyll (HNLC) areas (Allanson et al., 1981). One of the most important limiting factors for phytoplankton growth in the SO is iron availability (Martin et al., 1990; De Baar et al., 1995). Important sources of iron to the SO are upwelling of iron rich deep water (Blain et al., 2007), iron rich sediments (Martin et al., 1990), atmospheric dust deposition (Martin, 1990), hydrothermal vents (Tagliabue et al., 2014), and icebergs (Smith et al., 2007). However, iron bioavailability critically depends on its chemical speciation and resulting solubility (Boye et al., 2001). The speciation of iron can control phytoplankton community composition (Trimborn et al., 2017) with some phytoplankton species possessing more efficient mechanisms to adapt to low iron concentrations than others (Meyerink et al., 2017; Bender et al., 2018).
Iron limitation can lead to a reduction of photosynthetic pigments (chlorosis) in phytoplankton (Greene et al., 1991, 1992; Geider and La Roche, 1994). Such a decrease in the cellular light harvesting pigment (fucoxanthin, chlorophyll a and c2) contents, usually observed for iron-limited Antarctic diatoms (i.e., van Leeuwe and Stefels, 1998; Koch and Trimborn, 2019), can lead to a decrease in light absorption (Van Oijen et al., 2004). The de-epoxidation of diadinoxanthin to diatoxanthin is part of a protection mechanism against photoinhibition (Olaizola et al., 1994). These xanthophyll cycle pigments usually increase in phytoplankton under low iron conditions (Geider et al., 1993; Petrou et al., 2014; Trimborn et al., 2019).
Domoic acid (DA) is primarily produced by different species of the diatom Pseudo-nitzschia (Bates et al., 1989) and is well-known for its neurotoxicity, causing amnesic shellfish poisoning (Quilliam et al., 1989). While Pseudo-nitzschia species have a cosmopolitan distribution (Hasle, 2002; Trainer et al., 2012), not all species are capable of DA production (Silver et al., 2010; Lelong et al., 2012) and the amounts of DA produced can differ greatly. Depending on the species, blooms and different environmental conditions, the particulate DA (pDA) content can vary by eight orders of magnitude, between 2.5 × 10–9 pmol cell–1 (for a cell density of 6 × 108 cells mL–1 in cultures Cerino et al., 2005) to 0.25 pmol cell–1 (for a cell density of 49 cells mL–1 in field samples Trainer et al., 2000). pDA quantified in the SO was 2.7 10–3 pmol cell–1 and thus two orders of magnitude lower than this high value of 0.25 pmol cell–1. During the same study, dissolved DA (dDA) in the range of 6–707 pmol L–1 was measured (Silver et al., 2010). In a recent study, dDA up to 20 pmol L–1 was found in the SO (Geuer et al., 2019). The amount of DA released by cells can differ greatly (between 11% and in rare cases up to 83% of total DA) (Wang et al., 2007; Delegrange et al., 2018). The amount of dDA released depends on a variety of factors such as inter- and intraspecific differences as well as environmental conditions (Hagström et al., 2007). Iron and copper deficiency can also increase the release of dDA (Maldonado et al., 2002; Wells et al., 2005).
Although the primary ecological function of DA is still not well understood, various effects of the substance on its environment have been described. The diatom species Pseudo-nitzschia seriata increased DA production when exposed to exudates of predatory copepods (Tammilehto et al., 2015). Copepod escape response level decreased after ingestion of toxic Pseudo-nitzschia cells suggesting a role of DA as defense mechanism (Harðardóttir et al., 2018). Phosphate limitation also stimulated DA production (Lema et al., 2017). Furthermore, DA has been suggested to act as a ligand, binding both iron and copper (Rue and Bruland, 2001). Increased DA production under iron limitation and an even higher production under copper stress coupled to observations of increased DA release into the surrounding medium support such a role (Maldonado et al., 2002). DA was also suggested to act as a copper ligand as part of an efficient iron uptake system (Wells et al., 2005). Iron in connection with DA production is likely to improve competitiveness of Pseudo-nitzschia spp. over other diatoms (Prince et al., 2013). Generally, Pseudo-nitzschia spp. are known to adapt to low iron environments by modifying their cellular iron content (Marchetti et al., 2006) as well as their cell morphology by increased surface to volume (A:V) ratios (Marchetti and Harrison, 2007). Due to its ability to adapt to low iron environments, various Pseudo-nitzschia spp. can be found in open ocean HNLC regions (Marchetti et al., 2008, 2015). Furthermore, many Pseudo-nitzschia spp. are among the best adapted species to low iron concentrations within the SO (Smetacek et al., 2004; Marchetti et al., 2006; Hoppe et al., 2013; Russo et al., 2015). In the species P. granii, which is associated with open ocean HNLC areas, genes were discovered, which encode iron storage and iron concentration proteins, showing that HNLC Pseudo-nitzschia spp. possess mechanisms for an efficient iron uptake (Marchetti et al., 2017).
At least seven different Pseudo-nitzschia spp. (P. heimii, P. lineola, P. turgidula, P. prolongatoides, P. turgiduloides, P. subcurvata, and P. antarctica) occur in the Weddell Sea and contribute to the diatom diversity (Kang and Fryxell, 1993; Almandoz et al., 2008; Lelong et al., 2012). P. lineola and P. turgidula are cosmopolitan species, while P. prolongatoides, P. turgiduloides, and P. subcurvata are endemic to the SO (Hasle and Syvertsen, 1997; Bates et al., 2018). Of the species found within Antarctica, DA production has been verified for P. turgidula and P. lineola (Rhodes et al., 1996; Silver et al., 2010). DA production in endemic Pseudo-nitzschia spp. of Antarctica, however, has barely been described (Kegel et al., 2013; Bates et al., 2018). P. subcurvata are reported to not produce DA (Fryxell et al., 1991), while the species P. prolongatoides and P. antarctica have not yet been tested for DA production (Lelong et al., 2012; Bates et al., 2018). Generally, the connection between DA, iron and Antarctic Pseudo-nitzschia species has been rarely studied (Bates et al., 2018), even though Pseudo-nitzschia are important contributors to local blooms under HNLC conditions.
In this study, we used the Antarctic species Pseudo-nitzschia subcurvata, which does not produce DA, to test whether this species benefits from the addition of dDA. We hypothesize that (i) the growth rates of P. subcurvata in low iron conditions potentially increase, if DA is available, (ii) that DA is taken up by the cells, and that (iii) the intracellular iron content increases in presence of dDA.
The experiment was conducted under trace metal clean conditions according to GEOTRACES guidelines (Cutter et al., 2017). All equipment was soaked for 1 week in a 1% aqueous detergent solution (Citranox®, Alconox, Sigma-Aldrich, Germany), rinsed thoroughly with ultrapure water (UPW, Milli-Q®, Millipore A 10 system, Merck KGaA, Germany), and then placed in a hydrochloric acid (HCl) bath (1 mol L–1, HPLC grade, Merck KGaA) for another week. Then, the items were rinsed with UPW again, dried in a clean bench (Class 100, Opta, Germany), and stored in two polyethylene (PE) bags until use.
The diatom Pseudo-nitzschia subcurvata, identified by scanning electron microscopy (SEM) (Almandoz et al., 2008) was isolated at 49° S, 02° E during ANT-XXI/4 in April 2004 by Philipp Assmy and used in previous studies (Trimborn et al., 2013, 2014). Monocultures of P. subcurvata were kept for more than 2 years in stock cultures in iron-deplete (0.7 nmol L–1) and -replete (1.7 nmol L–1) natural Antarctic seawater medium. In the main experiment, iron replete treatments (+Fe and +FeDA) were additionally spiked with iron [Fe(III), ICP-MS standard, TraceCERT®, Fluka, Germany] to obtain a final iron concentration of 1.7 nmol L–1. Iron deplete treatments (Control, +DA; 0.7 nmol L–1) received no additional iron. Before the start of the main experiment, P. subcurvata was pre-acclimated for 2 weeks in dilute batch cultures in four different treatments (Table 1). To the Control treatment, no additions of iron and DA were made (Table 1). The iron replete treatments +Fe and +FeDA received additional iron (Table 1). dDA was added to the treatments +DA and +FeDA (Table 1).
Triplicate polycarbonate (PC) bottles were filled with 4.2 L of sterile filtered (0.2 μm, acid-cleaned Sartobran capsule, Sartorius, Germany) naturally low iron Antarctic seawater (0.7 nmol L–1 iron, collected at 59° 61’ S, 148° 64’ W in January 2017) and spiked with macronutrients (100 μmol L–1 NO3–, 6.25 μmol L–1 PO43, and 100 μmol L–1 Si–) and vitamins (30 nmol L–1 B1, 23 nmol L–1 B7, and 0.228 nmol L–1 B12) (F/2R medium; Guillard and Ryther, 1962).
In order to remove trace metals, all macronutrients and vitamins were passed through a chelex column (Chelex® 100, Merck KGaA). Since the experiment was performed in natural, trace metal low Antarctic seawater, zinc (0.16 nmol L–1), copper (0.08 nmol L–1), cobalt (0.09 nmol L–1), molybdenum (0.05 nmol L–1), and manganese (1.9 nmol L–1) were also added to maintain the ratio of the original f/2 recipe to prevent potential limiting effects of the trace metals other than iron. These trace metals were added in concentrations typical for Antarctic open ocean waters (Sañudo-Wilhelmy et al., 2002; Grotti et al., 2005; Hopkinson et al., 2007; Trimborn et al., 2015). No ethylenediaminetetraacetic acid (EDTA) was added to avoid changing natural trace metal interactions of seawater (Gerringa et al., 2000). Treatments with DA addition were adjusted to a final concentration of 3500 pmol L–1 (Table 1) assuming an 11% release of dDA (Wang et al., 2007) from the particulate phase of a Pseudo-nitzschia bloom event with high pDA amounts (Smith et al., 2018). The final concentration of dDA used in this experiment exceeded previously measured dDA concentrations of the SO by fivefold (Silver et al., 2010). To account for potential chemical changes of the treatments not related to P. subcurvata, abiotic control bottles (filtered culture medium without P. subcurvata cells) were incubated alongside the main experiment in duplicate being exposed for the same time to the same four different experimental conditions. The abiotic controls were axenic since the culture media and all additions (nutrients, vitamins, and trace metals) were filter sterilized prior to use for the experiments.
To avoid carry-over from previous culture conditions, the cultures were kept in pre-acclimation (2 weeks) prior to the experiment. During this preacclimation phase as well as during the main experiment (duration of 6–7 days), cultures were maintained at 2°C under a light:dark cycle of 16:8 h in front of light emitting diodes (LEDs, SolarStinger SunStrip Daylight, Econlux, Germany) at 100 μmol photons m–2 s–1 at color temperature of 8100 K. All treatments were harvested during exponential growth of the cells when a density of 0.6–1.2 × 105 cells mL–1 was reached after 6 up to 7 days, with no pH shifts occurring (Table 2). All treatments were tested for the presence of bacterial DNA using polymerase chain reaction (PCR) with universal bacterial primers and subsequent agarose gel electrophoresis. The treatments were not axenic. However, under visual microscopic observations only very few bacterial cells were observed.
Table 2. P. subcurvata grown under absence/addition of dissolved iron (Fe) and domoic acid (DA), respectively.
To prevent pH drifts in the cultures, the pH was monitored at least every second day at the growth temperature using a pH-Meter (827, Metrohm AG, Germany), which was calibrated (3-point calibration) with buffers certified from the National Institute of Standards and Technology before use. Upon harvest of the experiments, samples for dissolved inorganic carbon (DIC) were filtered through cartridges (0.2 μm, Thermo Fisher Scientific, Germany) into 5 mL air-tight borosilicate bottles with no headspace and stored at 4°C until analysis. Quantification was performed via colorimetric analysis on an autoanalyzer (QuAAtro, SEAL Analytical GmbH, Germany). At the final time point when the cells were harvested (6–7 days), salinity was determined for every sample as well.
For the determination of total dissolved metal concentrations (Mn, Fe, Co, Cu, Zn), 100 mL of seawater was filtered under a laminar flow bench (Class 100, Opta). All used labware was cleaned as described in Dick et al. (2008). Prior to analysis, all seawater samples were acidified to pH 1.7 with sub-boiled HNO3 (distilled 65% HNO3, pro analysis, Merck KGaA) and irradiated for 1.5 h using a UV power supply system (7830) and photochemical lamp (7825) from ArcGlass to provide total dissolved concentrations of Cu (Biller and Bruland, 2012). Fe and Cu concentrations in seawater samples were analyzed via external calibration using a SeaFAST system (Elemental Scientific, Germany) coupled to an Element2 mass spectrometer (Thermo Fisher Scientific). Standards for external calibration were prepared from Antarctic seawater and spiked with commercially available inductively coupled plasma mass spectrometry (ICP-MS) single element standards (SCP Science; 1000 mg L–1). The SeaFAST system eliminates matrix components, such as Na, Mg, and Cl from the seawater and preconcentrates the samples by a factor 40. This procedure reduces possible interferences by the matrix and enables analyzing expected low concentrations of elements of interest. The Nass-7 reference material (Natural Research Council Canada) was used to validate the quality of the analysis of trace elements in seawater at the beginning and end of a batch run. Since the element concentrations of the reference material are much higher than the concentrations expected in our seawater samples, the reference material was analyzed in a 1:10 dilution. The analysis of the Nass-7 reference material (n = 10) showed good results for Fe and Cu, for which average and standard deviation were in range of the certified material. Recovery rates were Fe (99.7%) and Cu (93.2%).
For dDA quantification, 1000 mL of sample were filtered via a combusted filter (glass microfiber filters, GF/F, ∼0.7 μm, Whatman GmbH, Germany) at the end of the experiment. The aqueous phase was acidified to pH 2 and stored at 4°C for a maximum of 2 days. The samples were then processed via solid-phase extraction. Cartridges (PPL, 200 mg, Agilent Technologies GmbH, Germany) were conditioned with 6 mL of methanol (100%, LiChrosolv®, HPLC grade, Merck KGaA) and 6 mL of acidified UPW (pH2, acidified with HCl, Suprapur®, Merck KGaA). Subsequently, the samples were loaded onto the cartridge. After loading, the cartridges were washed by filling them three times with acidified UPW before drying them with clean N2. Two additions of 500 μL methanol were used to elute into weighed combusted screw top vials (Agilent Technologies GmbH). To determine the exact volume of the extract, the filled vials were weighed again and the actual volume of methanol extract as well as the enrichment factor of each sample was calculated. The samples were then stored at −20°C until further analysis.
Corresponding filters for pDA analysis were stored in centrifuge tubes (FalconTM, Thermo Fisher Scientific, Germany) at −20°C until further processing. For pDA extraction, the filters were thawed and transferred into microcentrifuge tubes (Eppendorf, Germany) filled with 0.9 g of ceramic beads. 1 mL of methanol was added to each sample, which was shaken at 6500 rpm for 45 s (MagNA Lyser, Roche, Switzerland). Subsequently, the samples were centrifuged for 15 min at 18,111 × g (Centrifuge 5424 R, Eppendorf). In two steps, the supernatant was transferred onto a filter unit insert (0.45 μm, Durapore®, Merck KGaA) on a micro tube (Sarstedt, Germany), centrifuged for 30 s at 18,111 × g, transferred into combusted screw top vials (Agilent Technologies GmbH) and stored at −20°C until analysis. A process blank underwent the same procedure starting with filtration of 1000 mL of UPW.
Both dDA and pDA were analyzed via reversed phase ultra high performance liquid chromatography coupled to a triple quadrupole mass spectrometer (RP-UPLC-MS/MS, ACQUITY UPLC with Xevo TQ-XS, Waters) with electrospray ionization in positive mode. Total run time was 4.5 min at a flow rate of 0.6 mL min–1 with an aqueous formate buffer as mobile phase A, (pH 5.8, 20 mM ammonium formate, Riedel-de Haën, formic acid, Merck KGaA) and acetonitrile (LiChrosolv®, Merck KGaA) as mobile phase B. From 0 to 3.8 min, a gradient from 1 to 99% B was run, followed by isocratic conditions for 0.2 min. In a 0.3 min linear gradient the initial conditions were restored and the column equilibrated for another 0.2 min. Separation was performed on a C18 column with pre-column (2.1 × 50 mm, 1.7 μm, ACQUITY, Waters GmbH with BEH C18, 1.7 μm, VanGuardTM, Waters GmbH, Germany) at 35°C. DA was detected with mass transitions m/z 312 > 266 and 312 > 193. For dDA quantification the calibration curve was prepared in the extract of one of the abiotic treatments without DA addition to account for potential matrix effects. The calibration curve for pDA quantification was prepared in UPW. To determine limit of detection (LOD) and limit of quantification (LOQ), a calibration curve with DA concentrations of 0.8, 1.6, 3.2, 16.1, 32.1, 160.1 nmol L–1 was measured 5 times. The calibration curves were prepared in an abiotic extract of the respective matrix and the limits calculated as described previously (Geuer et al., 2019). For dDA quantification, LOD was 9 pmol L–1 and LOQ was 28 pmol L–1 in water, accounting for an enrichment factor of 1000. LOD for pDA in medium was 2.1 pmol L–1 and LOQ was 6.3 pmol L–1 normalized to the biomass in 1 L filtered medium.
Right after sampling, determination of cell density was performed at least every second day using a Multisizer 3 Particle Counter® (Beckman Coulter, Germany). Additionally, another set of subsamples was taken and fixed with 10% acid Lugol’s solution and stored in the dark at 2°C. To determine start and end point cell densities, cells were counted in Utermöhl chambers (Hydrobios, Germany) under an inverted microscope (Axio Observer D1, Zeiss, Germany) according to the method described by Utermöhl (1958). To this end, samples were allowed to settle for 24 h before counting at least 400 cells in stripes. Growth rates were calculated from cell densities derived by light microscopy using the following formula:
where μ (d–1) is the growth rate, Nt0 and Ntend are the initial and final cell densities, respectively, and t is the time between the sampled time points of the experiment (e.g., Furnas, 1990).
To determine cell sizes, Lugol fixed samples from the end point of each sample were used. The length and width of 25 cells per sample were measured using the program AxioVision (Release 4.8.2, Carl Zeiss Microscopy GmbH, Germany) from pictures that were previously taken using a camera connected to the inverted microscope (Axio Observer, Carl Zeiss Microscopy GmbH) at a magnification of 640×. The cell height was assumed to be half the cell width, since due to their elliptical shape the cells were only visible in valve view under the microscope. From these dimensions, cellular biovolume and cell surface and subsequently cellular surface to volume ratios (A:V) were calculated using the formula for prism on parallelogram-base according to Hillebrand et al. (1999).
Particulate organic matter was sampled at the end of the experiment. For the analyses of particulate organic carbon (POC) and particulate organic nitrogen (PON), 300 mL of sample were filtered through a precombusted (15 h, 500°C) filter (GF/F, 0.7 μm, Whatman GmbH). The filters were stored in combusted (15 h, 500°C) glass petri dishes at −20°C. All POC samples were taken in duplicate. Between the two filtrations, a blank filter was put onto the filtration unit and pressure was applied for a few seconds. Prior to POC analysis, the samples were dried for 12 h at 60°C. After drying, the filters were acidified with 200 μL of 0.2 N HCl (reagent grade, Sigma-Aldrich). The filters were again dried for 12 h at 60°C. The dry filters were folded under clean air, packed into tin cups and stored at room temperature and a humidity of 32% until quantification. Analysis was performed on an Elemental Analyzer (Euro EA – CHNSO, HEKAtech GmbH, Germany). Combusted filter blanks were subtracted from the obtained yields. For cellular quotas, POC and PON were normalized to filtered volume and cell volume. For POC production, the cell-volume-normalized POC content was multiplied by the growth rate.
For pigment content, 200 mL of sample were filtered (GF/F, ∼0.7 μm, Whatman GmbH). The filters were flash frozen in liquid nitrogen and subsequently stored at −80°C. Before analysis, a 24 h dark extraction at 4°C in 90% acetone was performed. After centrifugation (5 min, 4°C, 18,111 × g) and filtration (0.45 μm, Nalgene, Thermo Fisher Scientific) pigment concentrations were determined by RP high performance liquid chromatography (HPLC). The LaChrom Elite® HPLC system was equipped with a chilled autosampler (L-2200) connected to a DAD detector (L-2450, both VWR Hitachi International GmbH, Germany). For data analysis, the software EZChrom Elite (Ver. 3.1.3.; Agilent Technologies) was used. Separation was performed on a Spherisorb ODS-2 column (5 μm particle size, 25 cm × 4.6 mm, Waters) guarded by a guard cartridge (LiChrospher 100-RP-18, Merck KGaA). A gradient was run as described in Wright (1991). Peaks of the pigments chlorophyll a and c2, fucoxanthin, diadinoxanthin, diatoxanthin and β-carotene were identified and quantified against known standard concentrations (DHI Lab Products, Denmark).
The cellular metal content was determined by filtering 500 mL over trace metal clean filters (0.2 μm, PC, Whatman GmbH). The filters were stored in clean reaction tubes (Eppendorf). For intracellular iron content, 500 mL were filtered over trace metal clean filters again. After filtration, the filters were rinsed with oxalic acid to remove cell surface bound metals (Hassler and Schoemann, 2009). After rinsing, the filters were stored in clean reaction tubes. Before analysis, the filters were digested in 5 mL HNO3 (distilled 65%, p.a. Merck, United States) for 16 h at 180°C with 0.5 mL Hydrofluoric acid (HF, 40%, Suprapure®, Merck KGaA) (Twining and Baines, 2013). Subsequently, 0.5 mL UPW was added. By evaporation under a glass hood at 140°C, the cell extract was reduced to 0.5 mL. After the addition of 0.2 mL HNO3 (distilled 65%, Merck, United States) and moving the solution to a clean vial, 10 μL Rh (1 mg L–1) was added as internal standard. The sample was filled up to 10 mL with UPW and analyzed on an ICP-MS (Attom, Nu Instruments, United Kingdom). As reference samples to ensure low background trace metals and the quality of digestion, acid (5 mL 65% distilled HNO3, 0.5 mL HF), two filter blanks and BCR-414 (Plankton reference material, Sigma-Aldrich) were processed and analyzed. BCR-414 recovery rates were Mn (87.1%), Fe (93.1%), Co (81.2%), Cu (81.5%), and Zn (85.1%). Intracellular iron quotas were normalized per cell volume.
To test normality of the data the Shapiro-Wilk test was applied. Normally distributed data was tested for statistical differences in means using analysis of variance (ANOVA). For non-normally distributed data Wilcoxon signed-rank test and Wilcoxon rank-sum test were used. Correlations between variables were tested using a correlation matrix based on Person correlation. The significance level for all tests was chosen as α = 0.05 and significance was assumed when p ≤ 0.05. Statistical tests were performed using the programming language R in the software application RStudio®.
The salinity of all treatments during the experiment was 33.8 ± 0.2 (n = 12). DIC and pH did not show any significant differences between the treatments (p > 0.05, Table 2). dDA was below detection in non-amended controls but could be almost fully recovered in the different incubations of P. subcurvata, to which DA was added (Table 2), with a recovery rate for dDA of 92 ± 2% for treatment +DA and 89 ± 4% for treatment +FeDA. Recovery rates did not differ significantly between these treatments (p > 0.05). pDA was only quantified in the treatments with dDA addition. pDA levels were very low and only above detection in two bottles of +DA and in one bottle of the +FeDA treatment (Table 2). The approximate amount of pDA in these samples was 3.4 × 10–8 pmol cell–1. This pDA amount accounted on average for 0.10 ± 0.02% of the initially added DA.
Growth rates differed significantly between low iron and +Fe treatments. Treatments Control and +DA showed significantly lower growth rates (p < 0.01, for both: 0.44 ± 0.01 d–1) compared to the +FeDA and +Fe treatments (0.53 ± 0.02 and 0.53 ± 0.01 d–1; respectively, Table 3). Significant differences in A:V quotients were observed between all treatments (p < 0.01, Table 3). The +DA treatment showed the highest A:V quotient (2.36 ± 0.01 μm–1), while it was lowest in the +Fe treatment (1.99 ± 0.03). A:V quotient was significantly higher for low compared to high iron treatments (p < 0.01). POC production in the +DA treatment was lowest (p < 0.05, 6.8 ± 0.5 fmol μm–3 d–1, Table 3) while highest POC production was found in the +FeDA treatment (p < 0.05, 12.0 ± 1.2 fmol μm–3 d–1). The POC content was highest in the +FeDA (23.2 ± 2.1 fmol μm–3), followed by the +Fe treatment (18.9 ± 0.7 fmol μm–3). In the Control and +DA treatment a significantly lower POC was observed (p > 0.05, 17.5 ± 0.6, 15.6 ± 1.4 fmol μm–3, respectively, Table 3). Compared to all other treatments, +DA showed the highest C:N ratio (p < 0.05, 6.5 ± 0.2 mol mol–1). There was no statistical difference between the other treatments (p > 0.05). The PON content in +DA was significantly lower than in the other treatments (p < 0.05, 2.4 ± 0.2 fmol μm–3), while it was highest in +FeDA (p < 0.01, 3.9 ± 0.2 fmol μm–3, Table 3). Cellular iron contents did not differ significantly between the treatments (p > 0.05). The +Fe treatment showed the highest average iron content of 1.09 ± 0.61 amol μm–3. In contrast, the cellular copper content was highest in +DA and differed significantly from the other treatments (p < 0.05). Standard deviations for iron to carbon (Fe:C) ratios were high, particularly for treatment +Fe, which did not differ significantly from any other treatment (57.0 ± 30.4 μmol mol–1). Lowest Fe:C ratios were found in the Control and +FeDA treatment (23.1 ± 5.1 and 19.2 ± 4.7 μmol mol–1, respectively), a significantly higher Fe:C ratio was found in treatment +DA (p < 0.05, 50.2 ± 12.7 μmol mol–1, Table 3).
Table 3. Growth rates, surface to volume quotients (A:V) and particulate organic carbon (POC) production rates and elemental composition of the cells: cell-volume-normalized particulate organic carbon and nitrogen (POC, PON) contents, molar carbon to nitrogen ratios (C:N), cell-volume-normalized iron and copper contents and molar iron to carbon (Fe:C) ratios for the four different treatments.
The concentration of the pigments diatoxanthin and β–carotene did not differ significantly between the treatments (p > 0.05, Table 4). Diatoxanthin could not be quantified in Control and +DA treatments and was low in +Fe treatments. For pigments chlorophyll a and c2, fucoxanthin and diadionoxanthin, both low iron treatments showed significantly lower concentrations than the +Fe treatments (p < 0.05). Both chlorophyll c2 and fucoxanthin were highest in the +FeDA treatment (p < 0.05).
Table 4. Cellular pigment content in P. subcurvata grown in the four different treatments, normalized to cell volume.
To determine whether dDA facilitates iron uptake for Pseudo-nitzschia spp. from HNLC regions can be useful to further elucidate the ecological role of DA (Marchetti et al., 2008). This study furthers our understanding of whether DA plays a role in iron acquisition by P. subcurvata.
dDA was exclusively quantified in the treatments, to which it was added (+DA, +FeDA; Table 2). This outcome was expected as DA production by P. subcurvata has not been reported in another strain identified by SEM (Fryxell et al., 1991). In both +DA treatments, even though the recovery rate of dDA was 91 ± 3%, the amount of DA measured in the cells accounted for approximately 0.1%. Thus, the 10% loss of dDA cannot be explained by cellular uptake. Potential reasons for the observed loss of dDA are adsorption and photodegradation. DA adsorbs to natural clays in a comparatively low amount compared with other common non-polar organic substances. Furthermore, the adsorption is enhanced if metal ions are present (Burns and Ferry, 2007). In an experiment investigating the production of DA and chemical cues, a PC membrane was used (Tammilehto et al., 2015). The authors suspected the PC membrane to hold back only lipophilic components. Bottles used for incubation in this experiment were also made from PC. The hydrophilic DA should thus not adsorb onto the surface of the treatment bottles in large amounts. DA is photochemically degraded in natural seawater if subjected to radiation (Bouillon et al., 2006; Fisher et al., 2006). The photodegradation rate usually decreases with decreasing radiation energy and is strongest at wavelengths in the UV range (280–400 nm) with a maximum at 330 nm (Bouillon et al., 2006). Photodegradation still occurs at higher wavelengths and is promoted if Fe(III) ions and DOM are present (Fisher et al., 2006). In fact, all our treatments were illuminated 16 h per day with 100 μmol photons m–2 s–1 at 8100 K in this experiment, covering the range of wavelengths representative for daylight, but being out of the UV range in which photodegradation is most efficient. Moreover, light in this range would not have passed the walls of the PC bottles used, since they are nearly opaque to these wavelengths. Thus, it is likely that only a slight loss of dDA by photodegradation occurred in this experiment. Since the treatments were not axenic, only few bacteria were present and determined by PCR and light microscopy, bacterial degradation may also have removed some of the dDA (Hagström et al., 2007).
Iron concentrations in the medium without iron addition were 0.66 ± 0.08 nmol L–1 and 0.75 ± 0.08 nmol L–1, respectively (Table 1). For both treatments without iron addition, the growth rate and POC production was significantly lower than for iron enriched treatments, while the A:V quotient was higher, indicating that the cells were physiologically iron limited (Maldonado et al., 2002; Marchetti and Harrison, 2007; Zhu et al., 2016). This is supported by light harvesting pigments (fucoxanthin, chlorophyll a and c2, Table 4) being significantly lower in the low iron treatments (Koch and Trimborn, 2019; Koch et al., 2019).
Low iron treatments (Control and +DA) showed a significantly lower growth rate than high iron treatments (+Fe and +FeDA, Table 3). Under iron deficiency and copper stress, growth rates of temperate Pseudo-nitzschia species can decrease by as much as 50% (Maldonado et al., 2002). We observed a 20% lower growth rate for both low iron treatments compared to the +Fe treatments (Table 3). In a previous temperature experiment with P. subcurvata the growth rate at 2°C was 0.6 d–1 under ample supply of iron (Zhu et al., 2017) and thus similar to our +Fe treatments. In agreement with our results, another experiment by Zhu et al. (2016), reported a 20% difference in growth rate of P. subcurvata at 0°C between low and high iron conditions. Cell numbers of Pseudo-nitzschia have been reported to increase when dDA was added to the medium (Trick et al., 2010) and an increased growth rate was previously observed upon the addition of dDA (Wells et al., 2005). Similarly, Prince et al. (2013) showed increased growth of P. delicatissima under iron replete conditions when dDA was added to the culture (Prince et al., 2013). In this study we observed no increase in growth rate when dDA was added even at low iron conditions, findings contrary to our initial hypothesis. They are, however, consistent with observations for DA producing Pseudo-nitzschia species. Even though an increased release of dDA in iron limited treatments was observed, exponential phase growth rates remained lower for these treatments than for iron sufficient treatments (Maldonado et al., 2002; Sobrinho et al., 2017).
A:V quotients were higher for the low iron treatments compared to the +Fe treatments. Enhanced A:V quotients are beneficial for cells since they help increase nutrient uptake, release of waste products and heat as well as uptake and loss of other compounds (Lewis, 1976). Surprisingly, the A:V quotient was highest in the +DA treatment, suggesting enhanced iron limitation stress for this treatment, because increasing A:V quotients under low iron environments is a common adaptation strategy of phytoplankton since it facilitates the uptake of trace metals (Raven and Kübler, 2002; Koch and Trimborn, 2019). The increase in A:V quotients was previously reported for Pseudo-nitzschia spp. grown under low iron conditions and hypothesized to facilitate iron uptake (Marchetti and Harrison, 2007). In this study, the average difference in A:V quotients between low and high iron treatments was 13%, which is in the range of what was previously observed for other species (9–40%, Marchetti and Harrison, 2007) and indicative of an acclimation to low iron conditions.
In our experiment, POC production was lowest in the low iron treatments (Table 3). This is in line with previous studies, which showed generally a reduction of POC under iron deficiency in various phytoplankton species (e.g., Hutchins et al., 1999; Hoppe et al., 2013; Koch et al., 2019; Trimborn et al., 2019), the same as in temperate Pseudo-nitzschia species (Marchetti and Harrison, 2007), but also for the Antarctic P. subcurvata (Zhu et al., 2016). From our results it seemed that when iron was available in sufficient amounts, the addition of dDA was more beneficial than when it was depleted, which was contrary to our hypothesis. Hence, cellular POC production and POC and PON contents were significantly higher in the +FeDA treatment relative to the other treatments. Furthermore, the intracellular contents of the light harvesting pigments fucoxanthin and chlorophyll c2 were also significantly higher in this treatment. This might hint toward a better light utilization, which translated into a higher POC production (Table 3).
Except for the +DA treatment, no changes in C:N ratios were observed (Table 3), with values being consistent with other temperate oceanic Pseudo-nitzschia spp. (Marchetti and Harrison, 2007). Similarly, the C:N ratio of P. subcurvata remained constant in response to different iron availabilities (Zhu et al., 2016) as well as temperature and pCO2 (Zhu et al., 2017). Only for P. pseudodelicatissima, an increase of the C:N ratio with decreasing iron availability was reported (Sugie and Yoshimura, 2013). The authors suggested a rapid decrease in N relative to C assimilation under iron limitation. For our experiment, this was only observed in the +DA treatment, in which PON was lowest while POC remained similar in all treatments. Nitrogen assimilation can be limited by iron availability, since iron is essential for enzymes that reduce nitrate and nitrite (Morel et al., 1991; Milligan and Harrison, 2000). Milligan and Harrison (2000) suggested that during low iron availability the ability to process photons becomes limited within the photosynthetic electron transport chain, impacting also nitrite reduction as limiting step in nitrogen assimilation, which is a common phenomenon in iron limited phytoplankton (Hutchins et al., 1999). Nitrate reductase reduction was previously observed for low iron conditions, resulting also in a reduced nitrate assimilation capacity, which can be compensated by recycling proteins (Koch et al., 2019). Thus, an increase of C:N ratios may be triggered by iron limitation but often no changes are observed (Milligan and Harrison, 2000; Price, 2005; Koch et al., 2019). From the significantly higher C:N ratio in our +DA treatment, it appears that the combination of low iron availability and DA addition might have caused higher iron stress and affected nitrogen metabolism relative to the low iron treatment alone, which contradicts the hypothesis that dDA addition is beneficial to iron deplete cells.
Photosynthetic pigment contents in phytoplankton usually are reduced when iron is limiting, a process called chlorosis (Greene et al., 1991, 1992; Geider and La Roche, 1994). Chlorophyll a content was reduced in both low iron treatments (Table 4). The synthesis of chlorophyll a is negatively impacted by iron deficiency, which decreases its cellular content. An associated decrease in growth rate and carbon fixation was also observed in this and other experiments (Davey and Geider, 2001). P. subcurvata decreased their light harvesting pigments fucoxanthin, chlorophyll a and c2 under iron limitation, which was previously observed in the Antarctic diatom Phaeocystis antarctica (Van Leeuwe and Stefels, 2007; Koch et al., 2019). Pigments of the xanthophyll cycle (diadinoxanthin und diatoxanthin) have been shown to decrease under iron limitation in the Antarctic diatom Chaetoceros brevis, while β–carotene content was not affected by iron limitation (Van Oijen et al., 2004). In our experiment, only the diadinoxanthin content was significantly lower in the low iron treatments. Diatoxanthin was not detected in these treatments, implying no increase in de-epoxidation of diadinoxanthin and thus no higher investment in photoprotective mechanisms (Olaizola et al., 1994; Van Oijen et al., 2004).
The amount of DA in the cellular fraction of the treatments containing DA was below LOQ, which was contrary to the hypothesis of dDA being taken up by the cells. This was not surprising, since so far, only in the toxic species P. multistriata amino-acid transporters were found in silico, which were similar to kainoids transporters. These might be involved in the transport of DA in- or outside the cell (Di Dato et al., 2015). P. subcurvata, to our knowledge, does not possess such a transporter. Furthermore, DA not necessarily increases iron uptake by out-competing other ligands but by increasing bioavailability due to increased iron exchange among ligands and cell surface (Albrecht-Gary and Crumbliss, 1998; Maldonado et al., 2002). Rue and Bruland (2001) discovered that the strength, with which DA binds to iron and copper in combination with its concentration relative to other naturally occurring ligands does have the potential to affect their chemical speciation and thus their bioavailability and detoxification. Moreover, dDA was suggested to increase copper uptake under iron limitation, which in turn could enable the synthesis of a copper containing high affinity iron uptake system (Wells et al., 2005). In our experiment, DA was added at ecologically relevant concentrations previously reported for Pseudo-nitzschia blooms (Wang et al., 2007; Smith et al., 2018). The amount of DA present in the medium was thus high enough to act as a ligand, also for non-producing species (Maldonado et al., 2002). Furthermore, iron was added as Fe(III), which was shown to bind well to DA (Bates et al., 2001; Rue and Bruland, 2001) and was also used in previous experiments investigating DA and iron uptake (Maldonado et al., 2002; Wells et al., 2005). Still, the cellular iron content did not differ significantly between the treatments (Table 3), although Fe contents in the +DA and +Fe treatments were higher. The differences were, however, obscured by a high standard deviation between the replicates of the same treatments. However, the Fe:C ratio was significantly higher in +DA compared to the Control treatment. This might be related to the high iron content in this treatment. Treatments with iron addition did not show higher Fe:C ratios. The Fe:C in +FeDA was similar to the Control treatment, while in +Fe, the standard deviation within the triplicate was high, which was presumably related to the high standard deviation of the iron content. In contrast, other experiments using Pseudo-nitzschia describe Fe:C ratios usually higher by one to two orders of magnitude in high iron compared to low iron treatments (Maldonado et al., 2002; Marchetti et al., 2006). For P. subcurvata, iron limited Fe:C ratios of 25 μmol mol–1 (0°C) to 50 μmol mol–1 (4°C) were reported, while iron replete Fe:C ratios were higher (∼70 and 210 μmol mol–1, respectively) (Zhu et al., 2016). Fe:C ratios in our experiment were comparable to their iron limited Fe:C ratios. Surprisingly, in our experiment, iron addition did not result in elevated iron content and Fe:C ratios. Since Pseudo-nitzschia spp. generally adapt well to low iron concentrations (Marchetti et al., 2006; Sobrinho et al., 2017), the limiting effect of iron might be relatively low in this experiment, resulting in changes less prominent. With regard to DA addition, elevated Fe:C was observable in the +DA treatment but not in the +FeDA treatment, which showed equally low values as in the Control treatment. In another experiment under iron limitation, an increased iron uptake rate was observed when DA was added to present concentrations of FeEDTA (Maldonado et al., 2002). The authors, however, observed the same for iron replete treatments, even though the effect was lower. Thus, from our results it remains unclear whether dDA addition improves cellular iron acquisition.
The cellular copper content was significantly higher in the +DA treatment (Table 3). Rue and Bruland (2001) proved that relative to natural copper-binding organic ligands rather low concentrations of dDA are required to compete with other ligands for free copper ions. Since more copper than iron ions usually are available for complexation in natural seawater, complex formation between DA and copper is likely, even if DA has a higher theoretical complexation capacity for iron (Ladizinsky, 2003). In a study where P. multiseries was grown in low and high copper containing media (19.6 and 396 nmol L–1, respectively) both dDA and pDA were lower in the low copper treatment (Fuentes and Wikfors, 2013). In contrast Ladizinsky (2003) and Wells et al. (2005) observed a DA production increase and high dDA release when copper was deficient. In our experiment, the average copper concentration was 1.5 ± 0.2 nmol L–1 and thus not limiting (Lelong et al., 2013). An elevated copper uptake has been previously observed in iron limited oceanic diatoms (Peers et al., 2005; Koch and Trimborn, 2019). Copper is required for iron acquisition by diatoms (Peers et al., 2005). It was also previously shown, that copper is essential for several Pseudo-nitzschia species to adapt to iron limiting environments (Wells et al., 2005; Lelong et al., 2013). This could explain that cellular copper was only elevated in the +DA treatment and not in the +FeDA treatment, since the iron replete treatments had lower copper requirements. Wells et al. (2005) suggested a high affinity iron uptake system in some Pseudo-nitzschia species involving copper uptake with the help of dDA. Iron complexed by siderophores would thus be accessed. Further tests of this hypothesis confirmed copper requirements in iron limited P. delicatissima, but in their experiment no production of DA was observed (Lelong et al., 2013). The authors interpreted that either their strain did not possess such an iron uptake system or not enough siderophores bound to iron were present in their experiment. The P. subcurvata used in this experiment also did not produce DA, but our results indicate that copper might be useful for iron limited cells in that they better access it when dDA was added to the medium. However, it remains uncertain if the elevated copper content was involved in obtaining the higher Fe:C ratio in the +DA treatment in comparison with the Control treatment.
Furthermore, no advantages for cells in treatment +DA were observed compared to the Control treatment in terms of growth, morphology and element ratios. Instead, treatment +DA showed increased A:V quotients, decreased POC production and higher cellular iron and copper values, indicating that cells of this treatment were in an even higher need for iron. Pseudo-nitzschia spp. are known to adapt better to low iron concentrations compared to other oceanic diatoms (Marchetti et al., 2006; Hoppe et al., 2013; Russo et al., 2015; Sobrinho et al., 2017). It was suggested, that Pseudo-nitzschia may cope with low iron conditions by using an efficient iron uptake system (Wells et al., 2005) and storing excess iron (Marchetti et al., 2006). Consistent with this hypothesis, in the species P. granii, genes for an iron independent photosynthetic and a putative iron transport system were expressed under low iron concentrations (Cohen et al., 2018). The results of our experiment indicate a relatively good adaptation of cells to the low iron concentration in the low iron treatments.
It is important to keep in mind that the biological function of DA as ligand for Pseudo-nitzschia is still not fully understood (Sobrinho et al., 2017). An increased uptake of iron was previously observed in a non-producing strain that took up more iron when DA was added (Maldonado et al., 2002). The authors suggested that dDA might increase the rate of exchange of iron among ligands in solution and the cell surface and might help with iron uptake even if the respective species might not possess the proposed uptake mechanisms (Albrecht-Gary and Crumbliss, 1998; Maldonado et al., 2002). In our experiments, no strong ligands were added to the treatments but natural seawater was used that might have contained other ligands and dDA. However, the amount of dDA added in the experiment of Maldonado et al. (2002) was approximately three orders of magnitude higher than in our experiment. Lelong et al. (2013) postulated that if DA is used as an iron uptake system, it might not occur in every Pseudo-nitzschia species, which might also apply to P. subcurvata used in this experiment. To further elucidate the role of DA as ligand, the mechanism of DA production in combination with iron uptake mechanisms should further be assessed. For the non-DA-producing species P. subcurvata, however, DA does not appear to play an important role as ligand increasing iron bioavailability in naturally occurring concentrations.
P. subcurvata was well adapted to deal with low iron conditions and the cells did not draw down the DA added, as only very small amounts were found in the cells and >90% remained as dDA in the media. The availability of dDA did not result in any physiological advantages for P. subcurvata under low iron conditions, showing that using DA does not seem to be a strategy to directly acquire more iron when it is scarce. In addition, while the cellular iron content did not increase the cellular copper content increased in the +DA treatment, possibly due to the added DA. Further investigations would, however, be required to investigate whether copper might be involved in subsequently increasing iron uptake. This study highlights that if dDA is produced and released as a ligand, it might only be advantageous to Pseudo-nitzschia and Nitzschia species, which are also capable of producing and taking up DA or that dDA would be required in higher concentrations than naturally occurring in the SO. Thus, it would be of interest to compare these results to other HNLC species capable of producing DA. This study confirmed that there still is considerable need for research in terms of the ecological and physiological role of DA, especially when iron is scarce.
The original contributions presented in the study are included in the article/supplementary material, further inquiries can be directed to the corresponding author/s.
ST, BPK, JG, BK, FK, and TB designed this study. JG and TB conducted the experiment. TB, JG, and FK acquired the data. JG, ST, FK, BK, and BPK analyzed and interpreted the data. JG wrote the manuscript with contributions and critical feedback from all co-authors. All authors contributed to the article and approved the submitted version.
JG, BK, and BPK were funded by the strategy fund of the Alfred Wegener Institute Helmholtz Centre for Polar and Marine Research in the framework of the project “Inorganics in organics: Chemical and biological controls on micronutrient and carbon fluxes in the polar ocean” (Koch; IP76010001). ST, TB, and FK were funded by the Helmholtz Association (HGF Young Investigators Group EcoTrace, VH-NG-901). FK was furthermore supported by the Deutsche Forschungsgemeinschaft (SPP1158, KO5563/1-1“ViTMeD”).
The authors declare that the research was conducted in the absence of any commercial or financial relationships that could be construed as a potential conflict of interest.
We would like to express our sincere thanks to C. Völkner for quantification of metals and advice for sample preparation and thank D. Wilhelms-Dick for performing pretests for the experiment. Furthermore we would like to thank J. Tebben and W. Geibert for advice and lively discussions during planning this study as well as the reviewers and editor for their valuable comments. We sincerely thank B. Meyer-Schlosser for the pigment analysis and K.-U. Ludwichowski for nutrient analysis. M. Camoying and F. Pausch are thanked for their valuable help in the laboratory and M. Camoying for fruitful discussions about the results.
Albrecht-Gary, A.-M., and Crumbliss, A. L. (1998). “Coordination chemistry of siderophores: thermodynamics and kinetics of iron chelation and release,” in Metal Ions in Biological Systems, eds A. Sigel and H. Sigel (New York, NY: Marcel Dekker, Inc), 239–327. doi: 10.1002/cber.19971301203
Allanson, B. R., Hart, R. C., and Lutjeharms, J. R. E. (1981). Observations on the nutrients, chlorophyll and primary production of the Southern Ocean south of Africa. S. Afr. J. Antarct. Res. 1, 3–14.
Almandoz, G. O., Ferreyra, G. A., Schloss, I. R., Dogliotti, A. I., Rupolo, V., Paparazzo, F. E., et al. (2008). Distribution and ecology of Pseudo-nitzschia species (Bacillariophyceae) in surface waters of the Weddell Sea (Antarctica). Polar Biol. 31, 429–442. doi: 10.1007/s00300-007-0369-9
Bates, S. S., Bird, C. J., de Freitas, A. S. W., Foxall, R., Gilgan, M., Hanic, L. A., et al. (1989). Pennate Diatom Nitzschia pungens as the Primary Source of Domoic Acid, a Toxin in Shellfish from Eastern Prince Edward Island, Canada. Can. J. Fish. Aquat. Sci. 46, 1203–1215. doi: 10.1139/f89-156
Bates, S. S., Hubbard, K. A., Lundholm, N., Montresor, M., and Leaw, C. P. (2018). Pseudo-nitzschia, Nitzschia, and domoic acid: new research since 2011. Harmful Algae 79, 3–43. doi: 10.1016/j.hal.2018.06.001
Bates, S. S., Léger, C., Satchwell, M., and Boyer, G. L. (2001). “The effects of iron on domoic acid production by Pseudo-nitzschia multiseries,” in Harmful Algal Blooms (2000), eds G. A. Hallegraeff, S. I. Blackburn, C. J. Bolch, and R. J. Lewis (Paris: IUCN), 320–323.
Bender, S. J., Moran, D. M., Mcilvin, M. R., Zheng, H., Mccrow, J. P., Badger, J., et al. (2018). Colony formation in Phaeocystis antarctica: connecting molecular mechanisms with iron biogeochemistry. Biogeosciences 15, 4923–4942. doi: 10.5194/bg-15-4923-2018
Biller, D. V., and Bruland, K. W. (2012). Analysis of Mn, Fe, Co, Ni, Cu, Zn, Cd, and Pb in seawater using the Nobias-chelate PA1 resin and magnetic sector inductively coupled plasma mass spectrometry (ICP-MS). Mar. Chem. 13, 12–20. doi: 10.1016/j.marchem.2011.12.001
Blain, S., Quéguiner, B., Armand, L., Belviso, S., Bombled, B., Bopp, L., et al. (2007). Effect of natural iron fertilization on carbon sequestration in the Southern Ocean. Nature 446, 1070–1074. doi: 10.1038/nature05700
Bouillon, R.-C., Knierim, T. L., Kieber, R. J., Skrabal, S. A., and Wright, J. L. C. (2006). Photodegradation of the algal toxin domoic acid in natural water matrices. Limnol. Oceanogr. 51, 321–330. doi: 10.4319/lo.2006.51.1.0321
Boye, M., Van Den Berg, C. M. G., De Jong, J. T. M., Leach, H., Croot, P., and De Baar, H. J. W. (2001). Organic complexation of iron in the Southern Ocean. Deep. Res. Part I Oceanogr. Res. Pap. 48, 1477–1497. doi: 10.1016/S0967-0637(00)00099-6
Burns, J. M., and Ferry, J. L. (2007). Adsorption of domoic acid to marine sediments and clays. J. Environ. Monit. 9, 1373–1377. doi: 10.1039/b713101a
Cerino, F., Orsini, L., Sarno, D., Dell’Aversano, C., Tartaglione, L., and Zingone, A. (2005). The alternation of different morphotypes in the seasonal cycle of the toxic diatom Pseudo-nitzschia galaxiae. Harmful Algae 4, 33–48. doi: 10.1016/j.hal.2003.10.005
Cohen, N. R., Gong, W., Moran, D. M., McIlvin, M. R., Saito, M. A., and Marchetti, A. (2018). Transcriptomic and proteomic responses of the oceanic diatom Pseudo-nitzschia granii to iron limitation. Environ. Microbiol. 20, 3109–3126. doi: 10.1111/1462-2920.14386
Cutter, G., Casciotti, K., Croot, P., Geibert, W., Heimbürger, L.-E., Lohan, M., et al. (2017). Sampling and Sample-handling Protocols for GEOTRACES Cruises. Version 3. Toulouse: GEOTRACES International Project Office, doi: 10.25607/OBP-2
Davey, M., and Geider, R. J. (2001). Impact of iron limitation on the photosynthetic apparatus of the diatom Chaetoceros muelleri (Bacillariophyceae). J. Phycol. 37, 987–1000. doi: 10.1046/j.1529-8817.2001.99169.x
De Baar, H. J. W., de Jong, J. T. M., Bakker, D. C. E., Löscher, B. M., Veth, C., Bathmann, U., et al. (1995). Importance of iron for plankton blooms and carbon dioxide drawdown in the Southern Ocean. Nature 373, 412–415. doi: 10.1038/373412a0
Delegrange, A., Lefebvre, A., Gohin, F., Courcot, L., and Vincent, D. (2018). Pseudo-nitzschia sp. diversity and seasonality in the southern North Sea, domoic acid levels and associated phytoplankton communities. Estuar. Coast. Shelf Sci. 214, 194–206. doi: 10.1016/j.ecss.2018.09.030
Di Dato, V., Musacchia, F., Petrosino, G., Patil, S., Montresor, M., Sanges, R., et al. (2015). Transcriptome sequencing of three Pseudo-nitzschia species reveals comparable gene sets and the presence of Nitric Oxide Synthase genes in diatoms. Sci. Rep. 5, 1–14. doi: 10.1038/srep12329
Dick, D., Wegner, A., Gabrielli, P., Ruth, U., Barbante, C., and Kriews, M. (2008). Rare earth elements determined in Antarctic ice by inductively coupled plasma-time of flight, quadrupole and sector field-mass spectrometry: an inter-comparison study. Anal. Chim. Acta 621, 140–147. doi: 10.1016/j.aca.2008.05.026
Falkowski, P. G. (1994). The role of phytoplankton photosynthesis in global biogeochemical cycles. Photosynth. Res. 39, 235–258. doi: 10.1007/BF00014586
Field, C. B., Behrenfeld, M. J., Randerson, J. T., and Falkowski, P. (1998). Primary production of the biosphere: integrating terrestrial and oceanic components. Science 281, 237–240. doi: 10.1126/science.281.5374.237
Fisher, J. M., Reese, J. G., Pellechia, P. J., Moeller, P. L., and Ferry, J. L. (2006). Role of Fe(III), phosphate, dissolved organic matter, and nitrate during the photodegradation of domoic acid in the marine environment. Environ. Sci. Technol. 40, 2200–2205. doi: 10.1021/es051443b
Fryxell, G. A., Garza, S. A., and Roelke, D. L. (1991). Auxospore formation in an Antarctic clone of Nitzschia subcurvata hasle. Diatom Res. 6, 235–245. doi: 10.1080/0269249X.1991.9705169
Fuentes, M. S., and Wikfors, G. H. (2013). Control of domoic acid toxin expression in Pseudo-nitzschia multiseries by copper and silica: relevance to mussel aquaculture in New England (USA). Mar. Environ. Res. 83, 23–28. doi: 10.1016/j.marenvres.2012.10.005
Furnas, M. J. (1990). In situ growth rates of marine phytoplankton: approaches to measurement, community and species growth rates. J. Plankton Res. 12, 1117–1151. doi: 10.1093/plankt/12.6.1117
Geider, R. J., and La Roche, J. (1994). The role of iron in phytoplankton photosynthesis, and the potential for iron-limitation of primary productivity in the sea. Photosynth. Res. 39, 274–301. doi: 10.1007/BF00014588
Geider, R. J., La Roche, J., Greene, R. M., and Olaizola, M. (1993). Response of the photosynthetic apparatus of Phaeodactylum Tricornutum (Bacillariophyceae) to nitrate, phosphate, or iron Starvation. J. Phycol. 29, 755–766. doi: 10.1111/j.0022-3646.1993.00755.x
Gerringa, L. J. A., de Baar, H. J. W., and Timmermans, K. R. (2000). A comparison of iron limitation of phytoplankton in natural oceanic waters and laboratory media conditioned with EDTA. Mar. Chem. 68, 335–346. doi: 10.1016/S0304-4203(99)00092-4
Geuer, J. K., Krock, B., Leefmann, T., and Koch, B. P. (2019). Quantification, extractability and stability of dissolved domoic acid within marine dissolved organic matter. Mar. Chem. 215:103669. doi: 10.1016/j.marchem.2019.103669
Greene, R. M., Geider, R. J., and Falkowski, P. G. (1991). Effect of iron limitation on photosynthesis in a marine diatom. Limnol. Oceanogr. 36, 1772–1782. doi: 10.4319/lo.1991.36.8.1772
Greene, R. M., Geider, R. J., Kolber, Z., and Falkowski, P. G. (1992). Iron-induced changes in light harvesting and photochemical energy conversion processes in eukaryotic marine algae. Plant Physiol. 100, 565–575. doi: 10.1104/pp.100.2.565
Grotti, M., Soggia, F., Ianni, C., and Frache, R. (2005). Trace metals distributions in coastal sea ice of Terra Nova Bay, Ross Sea, Antarctica. Antarct. Sci. 17, 289–300. doi: 10.1017/S0954102005002695
Guillard, R., and Ryther, J. (1962). Studies of marine planctonic diatoms I. Cyclotella nana Hustedt and Detonula confervacea (Cleve) Gran. Can. J. Microbiol. 8, 229–239. doi: 10.1139/m62-029
Hagström, J. A., Granéli, E., Maneiro, I., Barreiro, A., Petermann, A., and Svensen, C. (2007). Release and degradation of amnesic shellfish poison from decaying Pseudo-nitzschia multiseries in presence of bacteria and organic matter. Harmful Algae 6, 175–188. doi: 10.1016/j.hal.2006.08.002
Harðardóttir, S., Krock, B., Wohlrab, S., John, U., Gissel, T., and Lundholm, N. (2018). Can domoic acid affect escape response in copepods? Harmful Algae 79, 50–52. doi: 10.1016/j.hal.2018.08.009
Hasle, G. R. (2002). Are most of the domoic acid-producing species of the diatom genus Pseudo-nitzschia cosmopolites? Harmful Algae 1, 137–146. doi: 10.1016/S1568-9883(02)00014-8
Hasle, G. R., and Syvertsen, E. E. (1997). “Marine diatoms,” in Identifying Marine Phytoplankton, ed. C. R. Thomas (San Diego, CA: Academic Press), 5–385. doi: 10.1016/b978-012693015-3/50005-x
Hassler, C. S., and Schoemann, V. (2009). Discriminating between intra- and extracellular metals using chemical extractions: an update on the case of iron. Limnol. Oceanogr. Methods 7, 479–489. doi: 10.4319/lom.2009.7.479
Hillebrand, H., Dürselen, C. D., Kirschtel, D., Pollingher, U., and Zohary, T. (1999). Biovolume calculation for pelagic and benthic microalgae. J. Phycol. 35, 403–424. doi: 10.1046/j.1529-8817.1999.3520403.x
Hopkinson, B. M., Mitchell, B. G., Reynolds, R. A., Wang, H., Selph, K. E., Measures, C. I., et al. (2007). Iron limitation across chlorophyll gradients in the southern Drake Passage: phytoplankton responses to iron addition and photosynthetic indicators of iron stress. Limnol. Oceanogr. 52, 2540–2554. doi: 10.4319/lo.2007.52.6.2540
Hoppe, C. J. M., Hassler, C. S., Payne, C. D., Tortell, P. D., Rost, B. R., and Trimborn, S. (2013). Iron limitation modulates ocean acidification effects on Southern Ocean phytoplankton communities. PLoS One 8:e79890. doi: 10.1371/journal.pone.0079890
Hutchins, D. A., Franck, V. M., Brzezinski, M. A., and Bruland, K. W. (1999). Inducing phytoplankton iron limitation in iron-replete coastal waters with a strong chelating ligand. Limnol. Oceanogr. 44, 1009–1018. doi: 10.4319/lo.1999.44.4.1009
Kang, S.-H., and Fryxell, G. A. (1993). Phytoplankton in the Weddell Sea, Antarctica: composition, abundance and distribution in water-column assemblages of the marginal ice-edge zone during austral autumn. Mar. Biol. 348, 335–348. doi: 10.1007/bf00350024
Kegel, J. U., Del Amo, Y., and Medlin, L. K. (2013). Introduction to project MIDTAL: Its methods and samples from Arcachon Bay. France. Environ. Sci. Pollut. Res. 20, 6690–6704. doi: 10.1007/s11356-012-1299-9
Koch, F., Beszteri, S., Harms, L., and Trimborn, S. (2019). The impacts of iron limitation and ocean acidification on the cellular stoichiometry, photophysiology, and transcriptome of Phaeocystis antarctica. Limnol. Oceanogr. 64, 357–375. doi: 10.1002/lno.11045
Koch, F., and Trimborn, S. (2019). Limitation by Fe, Zn, Co, and B12 results in similar physiological responses in two antarctic phytoplankton species. Front. Mar. Sci. 6:514. doi: 10.3389/fmars.2019.00514
Ladizinsky, N. (2003). The influences of dissolved copper on the production of domoic acid by Pseudo-nitzschia species in Monterey Bay, California: Laboratory Experiments and Field Observations. Long Beach: California State University.
Lelong, A., Bucciarelli, E., Hégaret, H., and Soudant, P. (2013). Iron and copper limitations differently affect growth rates and photosynthetic and physiological parameters of the marine diatom Pseudo-nitzschia delicatissima. Limnol. Oceanogr. 58, 613–623. doi: 10.4319/lo.2013.58.2.0613
Lelong, A., Hégaret, H., Soudant, P., and Bates, S. S. (2012). Pseudo-nitzschia (Bacillariophyceae) species, domoic acid and amnesic shellfish poisoning: revisiting previous paradigms. Phycologia 51, 168–216. doi: 10.2216/11-37
Lema, K. A., Latimier, M., Nézan, É, Fauchot, J., and Le Gac, M. (2017). Inter and intra-specific growth and domoic acid production in relation to nutrient ratios and concentrations in Pseudo-nitzschia: phosphate an important factor. Harmful Algae 64, 11–19. doi: 10.1016/j.hal.2017.03.001
Lewis, W. (1976). Surface/volume ratio: implications for phytoplankton morphology. Science 192, 885–887. doi: 10.1126/science.192.4242.885
Maldonado, M. T., Hughes, M. P., Rue, E. L., and Wells, M. L. (2002). The effect of Fe and Cu on growth and domoic acid production by Pseudo-nitzschia multiseries and Pseudo-nitzschia australis. Limnol. Oceanogr. 47, 515–526. doi: 10.4319/lo.2002.47.2.0515
Marchetti, A., Catlett, D., Hopkinson, B. M., Ellis, K., and Cassar, N. (2015). Marine diatom proteorhodopsins and their potential role in coping with low iron availability. ISME J. 9, 2745–2748. doi: 10.1038/ismej.2015.74
Marchetti, A., and Harrison, P. J. (2007). Coupled changes in the cell morphology and the elemental C, N, and Si composition of the pennate diatom Pseudo-nitzschia due to iron deficiency. Limnol. Oceanogr. 52, 2270–2284. doi: 10.4319/lo.2007.52.5.2270
Marchetti, A., Lundholm, N., Kotaki, Y., Hubbard, K., Harrison, P. J., and Virginia Armbrust, E. (2008). Identification and assessment of domoic acid production in oceanic Pseudo-nitzschia (Bacillariophyceae) from iron-limited waters in the northeast subarctic Pacific. J. Phycol. 44, 650–661. doi: 10.1111/j.1529-8817.2008.00526.x
Marchetti, A., Maldonado, M. T., Lane, E. S., and Harrison, P. J. (2006). Iron requirements of the pennate diatom Pseudo-nitzschia: comparison of oceanic (high-nitrate, low-chlorophyll waters) and coastal species. Limnol. Oceanogr. 51, 2092–2101. doi: 10.4319/lo.2006.51.5.2092
Marchetti, A., Moreno, C. M., Cohen, N. R., Oleinikov, I., deLong, K., Twining, B. S., et al. (2017). Development of a molecular-based index for assessing iron status in bloom-forming pennate diatoms. J. Phycol. 53, 820–832. doi: 10.1111/jpy.12539
Martin, J. H. (1990). Glacial-interglacial CO2 change: the Iron Hypothesis. Paleoceanography 5, 1–13. doi: 10.1029/PA005i001p00001
Martin, J. H., Gordon, R. M., and Fitzwater, S. E. (1990). Iron in Antarctic waters. Nature 345, 156–158. doi: 10.1038/345156a0
Meyerink, S. W., Ellwood, M. J., Maher, W. A., Dean Price, G., and Strzepek, R. F. (2017). Effects of iron limitation on silicon uptake kinetics and elemental stoichiometry in two Southern Ocean diatoms, Eucampia antarctica and Proboscia inermis, and the temperate diatom Thalassiosira pseudonana. Limnol. Oceanogr. 62, 2445–2462. doi: 10.1002/lno.10578
Milligan, A. J., and Harrison, P. J. (2000). Effects of non-steady-state iron limitation on nitrogen assimilatory enzymes in the marine diatom Thalassiosira weissflogii (Bacillariophyceae). J. Phycol. 36, 78–86. doi: 10.1046/j.1529-8817.2000.99013.x
Morel, F. M. M. M., Hudson, R. J. M., and Price, N. M. (1991). Limitation of productivity by trace metals in the sea. Limnol. Oceanogr. 36, 1742–1755. doi: 10.4319/lo.1991.36.8.1742
Olaizola, M., La Roche, J., Kolber, Z., and Falkowski, P. G. (1994). Non-photochemical fluorescence quenching and the diadinoxanthin cycle in a marine diatom. Photosynth. Res. 41, 357–370. doi: 10.1007/BF00019413
Peers, G., Quesnel, S. A., and Price, N. M. (2005). Copper requirements for iron acquisition and growth of coastal and oceanic diatoms. Limnol. Oceanogr. 50, 1149–1158. doi: 10.4319/lo.2005.50.4.1149
Petrou, K., Trimborn, S., Rost, B., Ralph, P. J., and Hassler, C. S. (2014). The impact of iron limitation on the physiology of the Antarctic diatom Chaetoceros simplex. Mar. Biol. 161, 925–937. doi: 10.1007/s00227-014-2392-z
Price, N. M. (2005). The elemental stoichiometry and composition of an iron-limited diatom. Limnol. Oceanogr. 50, 1159–1171. doi: 10.4319/lo.2005.50.4.1159
Prince, E., Irmer, F., and Pohnert, G. (2013). Domoic Acid Improves the Competitive Ability of Pseudo-nitzschia delicatissima against the Diatom Skeletonema marinoi. Mar. Drugs 11, 2398–2412. doi: 10.3390/md11072398
Quigg, A., Finkel, Z. V., Irwin, A. J., Rosenthal, Y., Ho, T.-Y., Reinfelder, J. R., et al. (2003). The evolutionary inheritance of elemental stoichiometry in marine phytoplankton. Nature 425, 291–294. doi: 10.1038/nature01953
Quilliam, M. A., Sim, P. G., McCulloch, A. W., and McCinnes, A. G. (1989). High-performance liquid chromatography of domoic acid, a marine neurotoxin, with application to shellfish and plankton. Int. J. Environ. Anal. Chem. 36, 139–154. doi: 10.1080/03067318908026867
Raven, J. A., and Kübler, J. E. (2002). New light on the scaling of metabolic rate with the size of algae. J. Phycol. 38, 11–16. doi: 10.1046/j.1529-8817.2002.01125.x
Redfield, A. C. (1958). The biological control of chemical factors in the environment. Am. Sci. 46, 205–221.
Rhodes, L. L., White, D., Shyre, M., and Atkinson, M. (1996). “Pseudo-nitzschia species isolated from New Zealand coastal waters: domoic acid production in vitro and links with shellfish toxicity,” in Harmful and Toxic Algal Blooms, eds T. Yasumoto, Y. Oshima, and M. Fukuyo (Paris: Intergovernmental Oceanographic Commission of UNESCO), 155–158.
Rue, E., and Bruland, K. (2001). Domoic acid binds iron and copper: a possible role for the toxin produced by the marine diatom Pseudo-nitzschia. Mar. Chem. 76, 127–134. doi: 10.1016/S0304-4203(01)00053-6
Russo, A. D. A. P. G., de Souza, M. S., Mendes, C. R. B., Jesus, B., Tavano, V. M., and Garcia, C. A. E. (2015). Photophysiological effects of Fe concentration gradients on diatom-dominated phytoplankton assemblages in the Antarctic Peninsula region. J. Exp. Mar. Bio. Ecol. 466, 49–58. doi: 10.1016/j.jembe.2015.02.002
Sañudo-Wilhelmy, S. A., Olsen, K. A., Scelfo, J. M., Foster, T. D., and Flegal, A. R. (2002). Trace metal distributions off the Antarctic Peninsula in the Weddell Sea. Mar. Chem. 77, 157–170. doi: 10.1016/S0304-4203(01)00084-6
Silver, M. W., Bargu, S., Coale, S. L., Benitez-Nelson, C. R., Garcia, A. C., Roberts, K. J., et al. (2010). Toxic diatoms and domoic acid in natural and iron enriched waters of the oceanic Pacific. Proc. Natl. Acad. Sci. U.S.A. 107, 20762–20767. doi: 10.1073/pnas.1006968107
Smetacek, V., Assmy, P., and Henjes, J. (2004). The role of grazing in structuring Southern Ocean pelagic ecosystems and biogeochemical cycles. Antarct. Sci. 16, 541–558. doi: 10.1017/S0954102004002317
Smith, J., Gellene, A. G., Hubbard, K. A., Bowers, H. A., Kudela, R. M., Hayashi, K., et al. (2018). Pseudo-nitzschia species composition varies concurrently with domoic acid concentrations during two different bloom events in the Southern California Bight. J. Plankton Res. 40, 1–17. doi: 10.1093/plankt/fbx069
Smith, K. L., Robison, B. H., Helly, J. J., Kaufmann, R. S., Ruhl, H. A., Shaw, T. J., et al. (2007). Free-drifting icebergs: hot spots of chemical and biological enrichment in the Weddell Sea. Science 317, 478–482. doi: 10.1126/science.1142834
Sobrinho, B. F., De Camargo, L. M., Sandrini-Neto, L., Kleemann, C. R., Da Costa Machado, E., and Mafra, L. L. (2017). Growth, toxin production and allelopathic effects of Pseudo-Nitzschia multiseries under Iron-enriched conditions. Mar. Drugs 15:331. doi: 10.3390/md15100331
Sugie, K., and Yoshimura, T. (2013). Effects of pCO2 and iron on the elemental composition and cell geometry of the marine diatom Pseudo-nitzschia pseudodelicatissima (Bacillariophyceae). J. Phycol. 49, 475–488. doi: 10.1111/jpy.12054
Tagliabue, A., Aumont, O., and Bopp, L. (2014). The impact of different external sources of iron on the global carbon cycle. Geophys. Res. Lett. 41, 920–926. doi: 10.1002/2013GL059059
Tammilehto, A., Nielsen, T. G., Krock, B., Møller, E. F., and Lundholm, N. (2015). Induction of domoic acid production in the toxic diatom Pseudo-nitzschia seriata by calanoid copepods. Aquat. Toxicol. 159, 52–61. doi: 10.1016/j.aquatox.2014.11.026
Trainer, V. L., Adams, N. G., Bill, B. D., Stehr, C. M., Wekell, J. C., Moeller, P., et al. (2000). Domoic acid production near California coastal upwelling zones, June (1998). Limnol Ocean. 45, 1818–1833. doi: 10.4319/lo.2000.45.8.1818
Trainer, V. L., Bates, S. S., Lundholm, N., Thessen, A. E., Cochlan, W. P., Adams, N. G., et al. (2012). Pseudo-nitzschia physiological ecology, phylogeny, toxicity, monitoring and impacts on ecosystem health. Harmful Algae 14, 271–300. doi: 10.1016/j.hal.2011.10.025
Trick, C. G., Bill, B. D., Cochlan, W. P., Wells, M. L., Trainer, V. L., and Pickell, L. D. (2010). Iron enrichment stimulates toxic diatom production in high-nitrate, low-chlorophyll areas. Proc. Natl. Acad. Sci. U.S.A. 107, 5887–5892. doi: 10.1073/pnas.0910579107
Trimborn, S., Brenneis, T., Hoppe, C. J. M., Laglera, L. M., Norman, L., Santos-Echeandía, J., et al. (2017). Iron sources alter the response of Southern Ocean phytoplankton to ocean acidification. Mar. Ecol. Prog. Ser. 578, 35–50. doi: 10.3354/meps12250
Trimborn, S., Brenneis, T., Sweet, E., and Rost, B. (2013). Sensitivity of Antarctic phytoplankton species to ocean acidification: growth, carbon acquisition, and species interaction. Limnol. Oceanogr. 58, 997–1007. doi: 10.4319/lo.2013.58.3.0997
Trimborn, S., Hoppe, C. J. M., Taylor, B. B., Bracher, A., and Hassler, C. (2015). Physiological characteristics of open ocean and coastal phytoplankton communities of Western Antarctic Peninsula and Drake Passage waters. Deep. Res. Part I Oceanogr. Res. Pap. 98, 115–124. doi: 10.1016/j.dsr.2014.12.010
Trimborn, S., Thoms, S., Bischof, K., and Beszteri, S. (2019). Susceptibility of two Southern Ocean phytoplankton key species to iron limitation and high light. Front. Mar. Sci. 6:167. doi: 10.3389/fmars.2019.00167
Trimborn, S., Thoms, S., Petrou, K., Kranz, S. A., and Rost, B. (2014). Photophysiological responses of Southern Ocean phytoplankton to changes in CO2 concentrations: short-term versus acclimation effects. J. Exp. Mar. Bio. Ecol. 451, 44–54. doi: 10.1016/j.jembe.2013.11.001
Twining, B. S., and Baines, S. B. (2013). The Trace Metal Composition of Marine Phytoplankton. Ann. Rev. Mar. Sci. 5, 191–215. doi: 10.1146/annurev-marine-121211-172322
Utermöhl, H. (1958). Zur Vervollkommnung der quantitativen Phytoplankton-Methodik. Int. Vereinigung Theor. und Angew. Limnol. Mitteilungen 9, 1–38. doi: 10.1080/05384680.1958.11904091
van Leeuwe, M. A., and Stefels, J. (1998). Effects of iron and light stress on the biochemical composition of Antarctic Phaeocystis sp. (Prymnesiophyceae). II. pigment composition. J. Phycol. 34, 496–503. doi: 10.1046/j.1529-8817.1998.340496.x
Van Leeuwe, M. A., and Stefels, J. (2007). Photosynthetic responses in Phaeocystis antarctica towards varying light and iron conditions. Biogeochemistry 83, 61–70. doi: 10.1007/978-1-4020-6214-8_6
Van Oijen, T., Van Leeuwe, M. A., Gieskes, W. W. C., and De Baar, H. J. W. (2004). Effects of iron limitation on photosynthesis and carbohydrate metabolism in the Antarctic diatom Chaetoceros brevis (Bacillariophyceae). Eur. J. Phycol. 39, 161–171. doi: 10.1080/0967026042000202127
Wang, Z., King, K. L., Ramsdell, J. S., and Doucette, G. J. (2007). Determination of domoic acid in seawater and phytoplankton by liquid chromatography-tandem mass spectrometry. J. Chromatogr. A 1163, 169–176. doi: 10.1016/j.chroma.2007.06.054
Wells, M. L., Trick, C. G., Cochlan, W. P., Hughes, M. P., and Trainer, V. L. (2005). Domoic acid: the synergy of iron, copper, and the toxicity of diatoms. Limnol. Oceanogr. 50, 1908–1917. doi: 10.4319/lo.2005.50.6.1908
Wright, S. W. (1991). Improved HPLC method for the analysis of chlorophylls and carotenoids from marine phytoplankton. Mar. Ecol. Prog. Ser. 77, 183–196. doi: 10.3354/meps077183
Zhu, Z., Qu, P., Gale, J., Fu, F., and Hutchins, D. A. (2017). Individual and interactive effects of warming and CO2 on Pseudo-nitzschia subcurvata and Phaeocystis antarctica, two dominant phytoplankton from the Ross Sea. Antarctica. Biogeosciences 14, 5281–5295. doi: 10.5194/bg-14-5281-2017
Keywords: Antarctica, copper, incubation experiment, diatoms (Bacillariophyceae), phytoplankton, toxin, high nutrient low chlorophyll regions
Citation: Geuer JK, Trimborn S, Koch F, Brenneis T, Krock B and Koch BP (2020) Dissolved Domoic Acid Does Not Improve Growth Rates and Iron Content in Iron-Stressed Pseudo-Nitzschia subcurvata. Front. Mar. Sci. 7:478. doi: 10.3389/fmars.2020.00478
Received: 06 February 2020; Accepted: 28 May 2020;
Published: 23 June 2020.
Edited by:
Michael William Lomas, Bigelow Laboratory for Ocean Sciences, United StatesReviewed by:
David Allen Hutchins, University of Southern California, United StatesCopyright © 2020 Geuer, Trimborn, Koch, Brenneis, Krock and Koch. This is an open-access article distributed under the terms of the Creative Commons Attribution License (CC BY). The use, distribution or reproduction in other forums is permitted, provided the original author(s) and the copyright owner(s) are credited and that the original publication in this journal is cited, in accordance with accepted academic practice. No use, distribution or reproduction is permitted which does not comply with these terms.
*Correspondence: Jana K. Geuer, amFuYS5nZXVlckBhd2kuZGU=
Disclaimer: All claims expressed in this article are solely those of the authors and do not necessarily represent those of their affiliated organizations, or those of the publisher, the editors and the reviewers. Any product that may be evaluated in this article or claim that may be made by its manufacturer is not guaranteed or endorsed by the publisher.
Research integrity at Frontiers
Learn more about the work of our research integrity team to safeguard the quality of each article we publish.