- 1Department of Marine Biology, Institute for Biological Sciences, University of Rostock, Rostock, Germany
- 2Department of Human Health, Physical Rehabilitation and Vital Activity, Ternopil V. Hnatiuk National Pedagogical University, Ternopil, Ukraine
- 3Leibniz Institute for Baltic Sea Research, Leibniz ScienceCampus Phosphorus Research Rostock, Warnemünde, Germany
- 4Department of Biological Sciences, Kent State University, Kent, OH, United States
- 5Department of Maritime Systems, Interdisciplinary Faculty, University of Rostock, Rostock, Germany
Fluctuations in the ambient oxygen concentrations represent a major stressor for aerobic organisms causing ATP deficiency during hypoxia and excessive production of reactive oxygen species during reoxygenation. Modulation of the mitochondrial electron transport system activity was proposed as a major mechanism involved in both the mitochondrial injury and adaptive response, but the mechanisms of ETS regulation during hypoxia/reoxygenation (H/R) stress remain poorly understood in hypoxia-tolerant organisms. To address this gap, we focused on the effects of H/R on activities of the mitochondrial Complexes I and IV in hypoxia-tolerant marine bivalves, the blue mussel Mytilus edulis, the Arctic quahog Arctica islandica and the Pacific oyster Crassostrea gigas, exposing them for 1 or 6 days to extreme hypoxia (<0.1% O2) followed by 1 h of reoxygenation. We used a combination of bioinformatics analysis, biochemical and molecular studies to examine the potential role of the reversible protein phosphorylation in regulation of the Complex I and IV activities and in the mitochondrial responses to H/R stress. Our results showed a strong species-specific modulation of two important kinases, the serine/threonine protein kinase A (PKA) and protein kinase C (PKC) by H/R stress in the studied bivalves. The mitochondrial Complexes I and IV emerged as important targets for modulation by H/R stress, mediated in part through reversible phosphorylation by PKA and PKC. The effects of the reversible phosphorylation on the enzyme activities were species- and condition-specific. In mussels and quahogs, phosphorylation by PKA and PKC led to a strong increase in activity of Complexes I and IV. In oysters, Complexes I and IV were insensitive to PKA and PKC activation except after prolonged hypoxia and reoxygenation when elevated sensitivity to PKA and PKC activation indicated a change in the configuration and/or isoform composition of these enzymes. Non-site-specific dephosphorylation strongly suppressed the activity of Complex I and IV in all three studied species. Because in mammals PKA and PKC can have either damaging or protective effects depending on the timing of activation, our findings underscore the need to further study the physiological implications of H/R-induced modulation of the PKA and PKC activity in hypoxia-tolerant organisms.
Introduction
Fluctuations in the ambient oxygen concentrations represent an ultimate stressor for aerobic organisms due to the role of oxygen as an electron acceptor in the mitochondrial electron transport system (ETS). Oxygen deficiency (hypoxia) or the lack of oxygen (anoxia) induce energetic stress in aerobic organisms by limiting substrate availability for the mitochondrial Complex IV (cytochrome c oxidase) which inhibits the ETS activity and ATP generation through oxidative phosphorylation (OXPHOS) (Pamenter, 2014; Sokolova et al., 2019). Paradoxically, return of the oxygen puts additional strain on the aerobic ATP production due to the excessive generation of reactive oxygen species (ROS) which can damage mitochondria, impair restoration of the energy balance and lead to cell injury and death (Hermes-Lima and Zenteno-Savín, 2002; Blomgren et al., 2003; Ham and Raju, 2016). Many aquatic organisms (such as those inhabiting estuaries, shallow coastal habitats, and the intertidal zone of the ocean) experience frequent extreme oxygen fluctuations from anoxia to normoxia or even hyperoxia during diurnal cycles of photosynthesis, tidal emersion-immersion cycles, and seasonal hypoxia that can last from days to weeks (Huggett and Griffiths, 1986; Burnett, 1997; Breitburg et al., 2018; Legrand et al., 2018). These fluctuations are especially impactful for benthic organisms that cannot escape deoxygenated areas and thus depend on physiological adaptations to tolerate hypoxia-reoxygenation (H/R) stress.
Regulation of mitochondrial metabolism is essential for tolerance to H/R stress that requires rapid transitions between inactive and active metabolic states and mitigation of the undesirable side reactions generating ROS. Consistent with this notion, mitochondrial reorganization including modulation of the OXPHOS pathways was shown to play a key role in responses to H/R stress in different groups including mammals, fish, insects, and mollusks (Kim et al., 2011; Ali et al., 2012; Pamenter, 2014; Martos-Sitcha et al., 2017; Devaux et al., 2019; Gerber et al., 2019; Napolitano et al., 2019; Sokolova et al., 2019). In hypoxia-sensitive organisms such as terrestrial mammals, H/R stress commonly leads to suppression of OXPHOS and ETS activity, elevated ROS production, mitochondrial Ca2+ overload and collapse of the mitochondrial membrane potential, culminating in cell death (Kalogeris et al., 2012; Ham and Raju, 2016; Andrienko et al., 2017). In contrast, mitochondria from hypoxia-tolerant organisms (such as intertidal bivalves, fish and freshwater turtles) preserve or upregulate of ETS capacity during H/R stress, reorganize mitochondrial proteome to minimize ROS generation, and rapidly suppress and restore OXPHOS activity depending on the oxygen availability (Buck and Pamenter, 2006; Pamenter, 2014; Haider et al., 2018; Sokolova et al., 2019). These findings point to the central role of the ETS in the regulation that contributes to hypoxia-tolerant mitochondrial phenotype.
In hypoxia-sensitive animals and tissues (such as mammalian heart), Complex I (EC 7.1.1.2 NADH: ubiquinone oxidoreductase) and Complex IV (EC 1.9.3.1 cytochrome c oxidase) play a key role in the mitochondrial responses to hypoxia (Helling et al., 2012; Hüttemann et al., 2012; Fuhrmann and Brüne, 2017) and are involved in hypoxia-reoxygenation-induced pathology (Eismann et al., 2009; Kim et al., 2011; Liu et al., 2014). Complex I is a major target of the H/R-induced mitochondrial injury and a major source of potentially cytotoxic ROS during H/R stress in hypoxia-sensitive species such as terrestrial mammals (Andrienko et al., 2017; Fuhrmann and Brüne, 2017). Mild attenuation of Complex I activity (e.g., using pharmacological interventions) mitigates oxidative damage during H/R stress but a strong H/R-induced loss of Complex I activity limits OXPHOS capacity and exacerbates energy deficiency and tissue damage in mammals (Camara et al., 2011; Fuhrmann and Brüne, 2017; Hernansanz-Agustín et al., 2017). Complex IV is the terminal oxidase of the mitochondrial ETS that uses O2 as the final electron acceptor and plays a key role in oxygen-dependent regulation of the ETS flux (Fukuda et al., 2007). The role of these complexes in the H/R-induced modulation of ETS activity have not been extensively studied in hypoxia-tolerant animals such as marine bivalves (Kurochkin et al., 2008; Sussarellu et al., 2013; Ivanina et al., 2016; Sokolov et al., 2019). To close this gap in our knowledge, we investigated the effects of H/R stress on Complexes I and IV in hypoxia-tolerant marine bivalves and explored the potential role of post-translational modifications through protein kinases A and C in regulation of Complex I and IV activities.
Studies in mammalian models demonstrated an important role of post-translational modification (PTM) of ETS proteins, particularly the Complexes I and IV, in regulating the responses to H/R stress including modulation of the ETS activity, protein-protein and protein-lipid interactions, and ROS production (Hüttemann et al., 2012; Dudek, 2017; Gowthami et al., 2019; Kalpage et al., 2019; Mathers and Staples, 2019). Among different types of PTMs (such as phosphorylation, acetylation, succinylation, or SUMOylation), the reversible protein phosphorylation by protein kinases plays an important and not yet fully deciphered role in regulating the mitochondrial metabolism in different physiological and stress states (Stram and Payne, 2016). PTM by protein kinases provides a rapid, reversible, and energetically inexpensive mechanism to regulate the enzyme activity (Lim et al., 2016; Lucero et al., 2019). Among the mitochondrial protein kinases, serine/threonine protein kinase A (PKA) and protein kinase C (PKC) are recognized as crucial regulators of mitochondrial physiology (Lim et al., 2016). Mitochondrial Complexes I and IV are common substrates for the PKA- and PKC-mediated reversible phosphorylation during different physiological and pathological states, including exposure to H/R stress (Ogbi and Johnson, 2006; Prabu et al., 2006; Dagda and Das Banerjee, 2015; Mathers and Staples, 2019). Phosphorylation of Complex I can activate or suppress its activity depending on the species, tissue, and phosphorylation site (Lucero et al., 2019; Mathers and Staples, 2019). Complex IV is typically suppressed by phosphorylation in mammals, and this suppression plays an important role in hypoxia-induced mitochondrial dysfunction (Acin-Perez et al., 2009; Hüttemann et al., 2012; Srinivasan et al., 2013; Mahapatra et al., 2016). The effects of reversible protein phosphorylation on Complex I and IV activities and the potential role of PKA and PKC in metabolic responses to H/R stress are unknown in hypoxia-tolerant organisms including marine bivalves and require further investigation.
The aim of our study was to determine the effects of hypoxia and reoxygenation on activities of the mitochondrial Complexes I and IV and assess the potential role of the PTM (via reversible protein phosphorylation) in regulating the activity of these enzymes in marine bivalves. As model organisms, we have chosen three species—the blue mussel Mytilus edulis, the Arctic quahog Arctica islandica and the Pacific oyster Crassostrea gigas. These bivalves are common in the temperate coastal zones of the northern hemisphere and are known for their high hypoxia tolerance with the 50% survival times in anoxia (LT50) ranging from >7 days to >30 days, depending on temperature and salinity (Vaquer-Sunyer and Duarte, 2008). To assess the effects of different hypoxia duration such as might be expected during diurnal or seasonal hypoxia in estuaries and shallow coastal zones, the bivalves were exposed for one (short-term) or six (long-term) days to extreme hypoxia (<0.1% O2) followed by 1 h of reoxygenation. We used a combination of bioinformatics analysis, biochemical, and molecular studies to assess the potential role of the post-translational modifications (PTM) by reversible protein phosphorylation in regulation of the Complex I and IV activities and in the mitochondrial responses to H/R stress. We determined the changes in PKA and PKC activities during H/R stress and assessed their implications for the global phosphorylation levels of cellular proteins. The potential phosphorylation sites for different protein kinases on Complexes I and IV were identified, and the phosphorylation states of Complexes I and IV was experimentally manipulated to assess the effects of PKA- and PKC-dependent protein phosphorylation on the activity of these mitochondrial complexes during the H/R stress.
Materials and Methods
Animal Collection and Care
Blue mussels Mytilus edulis were hand-collected from submerged piles near Warnemünde in the Baltic Sea (54°10′49.602′′N, 12°05′21.991′′E). The quahogs Arctica islandica were collected by dredging at ~20–25 m depth offshore of Kühlungsborn in the Baltic Sea. The Pacific oysters Crassostrea gigas were collected in the intertidal zone of the island of List/Sylt in the German Wadden Sea (55°02′54.4′′N 8°27′12.6′′E). All animals were transported within 4 (mussels and quahogs) or 24 (oysters) hours of collection to the University of Rostock. The shells were cleaned from epibionts, and the bivalves were kept in aquaria with aerated artificial sea water (ASW) (Instant Ocean®, Aquarium Systems, Sarrebourg, France) at 15 ± 1°C for at least 4 weeks prior to the experiments. During the preliminary acclimation and exposures, salinity was maintained at 15 ± 1, 20 ± 1, and 30 ± 1 practical salinity units for the mussels, quahogs, and oysters, respectively. These values were within 2–3 salinity units of the respective habitat salinities at the time of collection. Mollusks were fed ad libitum by continuous addition of a commercial algal blend (DT's Live Marine Phytoplankton, CoralSands, Wiesbaden, Germany) to experimental tanks according to the manufacturer's instructions.
H/R Exposures
Bivalves were subjected to hypoxia in air-tight 6 l plastic chambers filled with ASW at the respective acclimation salinity. The water in the chambers was bubbled with nitrogen until oxygen levels dropped below ~0.5% of air saturation. Oxygen content of seawater was measured using a fiber optic oxygen sensor connected to a FireStingO2 Optical Oxygen Meter (PyroScience GmbH, Aachen, Germany). The chamber was submerged in the temperature-controlled aquarium to maintain temperature at 15 ± 0.5°C. The animals were exposed to a short-term (1 day) or long-term (6 days) hypoxia. These time points were chosen based on earlier studies as corresponding to the early onset and the advanced stages of cellular and bioenergetic stress, respectively, in the mussels and oysters (no comparable data are available for quahogs) (Kurochkin et al., 2008; Falfushynska et al., 2020; Haider et al., 2020). For reoxygenation, a subset of mollusks exposed to 1 and 6 days of hypoxia was placed into a fully aerated aquarium for 1 h. One hour reoxygenation was chosen based on an earlier study in oysters showing the strongest change in mitochondrial functions at this time point (compared to the longer recovery periods) (Kurochkin et al., 2008). The mollusks were moved into the aerated aquaria inside their exposure chambers to minimize the handling stress, and the chambers were open to ensure water circulation. Normal oxygen levels (>95% air saturation) were established inside the chambers within <5 min of reoxygenation. Control animals were maintained in normoxia (>95% air saturation) and fed throughout the exposures. Control bivalves were fasted for 24 h prior to sampling. Bivalves exposed to hypoxia were not fed. Our pilot experiments showed that the studied bivalve species close their shells in severe hypoxia such as used in the present study and spontaneously cease filtration and feeding activities. Therefore, no phytoplankton has been added to the hypoxia exposure chambers to prevent possible artifacts due to the excessive growth of anaerobic bacteria on the unremoved algal biomass (Zwaan and Babarro, 2000). The exposures resulted in the following groups: (1) normoxic controls (C); (2) animals exposed to short-term (1 day) hypoxia (H1); (3) animals exposed to 1 h of reoxygenation following short-term (1 day) hypoxia (H1R); (4) animals exposed to long-term (6 days) hypoxia (H6); (5) animals exposed to 1 h of reoxygenation following long-term (6 days) hypoxia (H6R). Each group consisted of 11 animals, 6 of which were used to measure the activities of Complex I and IV in different phosphorylation states, and 5 were used to assess the activities of PKA, PKC, and phosphatases as well as measure the levels of PKA- and PKC-phosphorylated protein residues by immunoblotting. All animals were dissected on ice, and the digestive gland tissue was shock-frozen in the liquid nitrogen and used for further analyses. We have chosen the digestive gland because it is one of the largest and metabolically most active organs in bivalves, sensitive to hypoxia-induced stress (Falfushynska et al., 2020; Haider et al., 2020).
Tissue Homogenization
The digestive gland tissue was homogenized 1:5 w:v using a glass homogenizer in either Protein Kinase Buffer (PKB) (50 mM Tris–HCl, 10 mM 2-mercaptoethanol, 10% v:v glycerol, 25 mM β-glycerophosphate, 50 mM NaF, pH 7.5) or Protein Phosphatase Buffer (PPB) (50 mM Tris–HCl, 10 mM 2-mercaptoethanol, 10% v:v glycerol, 2.5 mM EDTA, 2.5 mM EGTA, pH 7.5) with a few crystals of phenylmethylsulfonyl fluoride (PMSF) added just prior to homogenization. Homogenization was carried out on ice. An aliquot of homogenate prepared with Protein Phosphatase buffer was immediately mixed with 25 mM β-glycerophosphate and 50 mM NaF (final concentrations) to inhibit both protein kinases and phosphatases (stop solution). Homogenates were centrifuged for 20 min at 6,000 g at 4°C, and the supernatant used for enzymatic analyses.
PKA and PKC Kinase Activity Assay
The quantitative Elisa-based PKA (ab139435) and PKC (ab139437) activity assay kits (Abcam, Cambridge, UK) were used to determine of PKA and PKC activity in the digestive gland according to the manufacturer's protocol. For each reaction, 30 μl of the crude tissue homogenate prepared in the PKB Buffer (see “Tissue Homogenization”) was used. The reaction was initiated by ATP, and the absorbance was monitored at 450 nm in the SpectraMax M2 microplate reader (Molecular Devices GmbH, Biberach-an-der-Riß, Germany). Linearity of the assay was checked using the serial dilutions of the samples and compared to the dilutions of the respective standards (purified active PKA and PKC) supplied with the kits. Samples showed good linearity with an average R2 of 0.985 ± 0.022 and the lines that were parallel to the standard curve. Activity of PKA and PKC were expressed in relative units (RU) g−1 fresh mass where 1 RU is equivalent to the activity of 1 ng of the PKA or PKC standard during the 90 min of the reaction.
Total Phosphatase Activity Assay
Total cellular phosphatase activity was determined in digestive gland crude extract of H/R-exposed mollusks by colorimetric assay using p-nitrophenyl phosphate as a substrate (McAvoy and Nairn, 2010). The protein phosphatase activity was calculated from the change in absorbance at 405 nm using a molar extinction coefficient of 1.8 × 104 M−1cm−1. Results were expressed as pmol product min−1 g−1 wet mass.
Enzyme Activity Assays
Mitochondrial Complex I (NADH:ubiquinone oxidoreductase, E.C. EC 1.6.5.3) and IV (cytochrome c oxidase, EC 1.9.3.1) activities were determined using standard spectrophotometric techniques described elsewhere (Birch-Machin and Turnbull, 2001; Ivanina et al., 2008) using homogenates of the digestive gland tissues prepared in the stop solution (to assess the activity of the enzyme in the native phosphorylation state) or after experimental (de-)phosphorylation of the homogenates in PKB or PPB buffers (see “Tissue homogenization” and “In vitro manipulation of the protein phosphorylation state”). The assay was carried out in 200 mM potassium phosphate buffer, pH 7.5 with 5 mM MgCl2 and 0.5 mg ml−1 asolectin by addition of 10 mM NADH, 2mM KCN, and 1.5 mM decylubiquinone as an electron acceptor. Prior to the assay, the homogenates were treated by 1.42 mM lauryl maltoside for 1–2 min to permeabilize the outer mitochondrial membrane. Reactions were started by addition of decylubiquinone and monitored at 340 nm at 25°C. After determining the initial slope corresponding to the total reductase activity of the sample, 4.2 μM rotenone was added to inhibit Complex I. The activity of Complex I was calculated as the rotenone-sensitive reductase activity from the differences in the reaction slopes before and after the rotenone addition using an extinction coefficient of 6.2 l mmole−1 cm−1 for NADH.
Complex IV activity was determined in the assay buffer containing 50 mM sodium phosphate buffer, pH 7.0 with 91 μM reduced cytochrome c. Prior to the assay, the homogenates were treated by 2.8 mM lauryl maltoside for 1-2 min to permeabilize the outer mitochondrial membrane. Reactions were started by addition of aliquot of tissue extract and monitored at 550 nm at 25°C. After the initial reaction slope was determined, the Complex IV was inhibited by 0.3 mM KCN. Complex IV activity was calculated as the difference in the reaction slope before and after KCN addition using the extinction coefficient of 19.6 mM−1·cm−1 for cytochrome c.
Bioinformatic Prediction of Protein Phosphorylation Sites
To test for the potential involvement of different protein kinases in phosphorylation of Complex I and IV proteins, we examined the distribution of predicted phosphorylation sites across all mitochondrially-encoded core subunits of Complexes I and IV in C. gigas, M. edulis, and A. islandica. Seven mitochondrially-encoded Complex I subunits (ND1, ND2, ND3, ND4, ND4L, ND5, and ND6) and three mitochondrially-encoded Complex IV subunits (COX1, COX2, and COX3) were analyzed. NetPhos 3.1 server (http://www.cbs.dtu.dk/services/NetPhos/) was used to predict putative serine, threonine, or tyrosine phosphorylation sites, focusing only on the best prediction for each residue (Blom et al., 1999, 2004). The kinase specific predictions were made for the following 17 kinases: ATM, CKI, CKII, CaM-II, DNAPK, EGFR, GSK3, INSR, PKA, PKB, PKC, PKG, RSK, SRC, cdc2, cdk5, and p38MAPK. Furthermore, the generic kinase phosphorylation sites were predicted. Prediction scores in the range of 0.5–1.0 were considered positive, and putative phosphorylation sites were counted across the proteins. We also considered whether residues on the sequences from different species were homologous using multiple sequence alignment of respective proteins sequences reconstructed in MUSCLE (Edgar, 2004) using MEGA7 (Kumar et al., 2016). For residues that occupied the same alignment position we considered whether they were predicted to be phosphorylated in one, two or all three studied species.
In vitro Manipulation of the Protein Phosphorylation State
To determine the effects of phosphorylation/dephosphorylation on enzymatic activity of Complex I and IV, phosphorylation state of the protein extract was manipulated by cerium (IV) oxide (CeO2) or alkaline phosphatase (AP) (for dephosphorylation), or by stimulating activity of endogenous kinases, protein kinase A (PKA) or protein kinase C (PKC). Crude extracts of the digestive gland tissues (50 μl) prepared either with PKB or PPB buffers (see “Tissue Homogenization”) were mixed with 50 μl (1:1 v:v) of the appropriate incubation buffer solutions (listed below) and incubated at 30°C for 30 min prior to Complex I and IV activity determination. For dephosphorylation with CeO2, the incubation buffer contained 50 mM Tris–HCl, 10% v:v glycerol, 10 mM β-mercaptoethanol, 2.5 mM EDTA, 2.5 mM EGTA, and 20 mM cerium oxide. Cerium (IV) oxide was solubilized in HCl, and the final pH of the incubation buffer was 7.5. CeO2 non-selectively dephosphorylates all accessible serine, threonine, and tyrosine residues regardless of which kinase was responsible for their phosphorylation (Tan et al., 2008). For alkaline phosphatase treatment, the incubation buffer contained 400 U calf intestinal alkaline phosphatase in 50 mM Tris–HCl, 10% v:v glycerol, 10 mM β-mercaptoethanol, 5 mM CaCl2, 5 mM MgCl2, pH 7.5. To stimulate the endogenous protein kinase activities, the following incubation buffers were used: 50 mM Tris–HCl, 10% v:v glycerol, 10 mM β-mercaptoethanol, pH 7.5, 5 mM Mg ATP, 30 mM β- glycerophosphate, and either (1) 1 mM cAMP to stimulate protein kinase A (PKA); or (2) 1.3 mM CaCl2 and 7 μg·ml−1 phorbol-myristate acetate to stimulate protein kinase C (PKC). For control incubations (denoted STOP): the buffer contained 50 mM Tris–HCl, 10% v:v glycerol, 10 mM β-mercaptoethanol, 2.5 mM EDTA, 2.5 mM EGTA and 25 mM β- glycerophosphate, 50 mM NaF, pH 7.5.
Immunoblotting
To test the effects of the experimental manipulations of the phosphorylation status, the homogenates of the digestive gland of M. edulis, A. islandica, or C. virginica treated with CeO2, cAMP, PMA, or STOP solution (as described in the sections “Tissue homogenization” and “In vitro manipulation of the protein phosphorylation status”) were probed with the antibodies specific for the PKA or PKC phosphorylation signatures. Protein concentration in homogenates was measured according to Bradford (Sigma, St. Louis, MO, USA) (Bradford, 1976), and 50 μg of protein per lane was loaded and resolved on 10% denaturing acrylamide gel. Protein was transferred onto Immobilon P membrane (Millipore Corp., Billerica, MA, USA), blocked with 4% bovine serum albumin (BSA) in TBST buffer (20 mM Tris, 150 mM NaCl, 0.1% Tween 20, pH 7.5) and probed with Phospho-(Ser/Thr) PKA Substrate (#9621) or Phospho-(Ser) PKC Substrate (#2261) antibodies (both from Cell Signaling Europe, Frankfurt, Germany) diluted at 1:1,000 in blocking solution. After incubation with the horseradish peroxidase-conjugated goat anti-rabbit IgG secondary antibody (Jackson Immunoresearch Laboratories, West Grove, PA, USA), the signal was developed with Pierce ECL Western Blotting Substrate (Thermo Fisher, Waltham, MA, USA) and detected with an X-ray film. The film was scanned with transmission scanner, and the signal was quantified with GelQuant.NET software provided by biochemlabsolutions.com.
To quantify the total amount of PKA- or PKC-phosphorylated proteins under different oxygen exposure regimes, digestive gland homogenates of M. edulis, A. islandica, or C. virginica treated with the stop solution (to inhibit phosphatases and kinases) were mixed with the lysis buffer (0.1% sodium dodecyl sulfate, 0.1% Triton X-100, 50 mM Tris pH 7.5) supplemented with Proteases and Phosphatases inhibitors cocktail (Sigma, St. Louis, MO, USA) and incubated for 20 min on a shaker. The lysate was sonicated in Sonicator S-4000 (Qsonica, Newtown, CT, USA) at 25% power for 30 s and centrifuged for 4 min at 4°C at 10,000 × g. Protein concentration was measured according to Bradford (Sigma, St. Louis, MO, USA) (Bradford, 1976). For each sample, 15 μg (M. edulis) or 10 μg (A. islandica or C. gigas) of protein were mixed with 200 μl of Tris-buffered saline (TBS) (50 mM Tris, 150 mM NaCl, pH 7.5) and loaded in the slots of MINIFOLD II Microsample filtration manifold (Schleicher & Schuel, Dassel, Germany). The mixture was filtered through Amersham Protran nitrocellulose membrane (GE Healthcare Europe, Freiburg, Germany) by means of vacuum. The membrane was washed in TBS, blocked in 4% BSA in TBST buffer and probed with Phospho-(Ser/Thr) PKA Substrate (#9621) or Phospho-(Ser) PKC Substrate (#2261) antibodies (both from Cell Signaling Europe, Frankfurt, Germany) diluted at 1:1,000 in blocking solution. The signal was visualized with the horseradish peroxidase-conjugated goat anti-rabbit IgG secondary antibody (Jackson Immunoresearch Laboratories, West Grove, PA, USA), developed with Pierce ECL Western Blotting Substrate (Thermo Fisher, Waltham, MA, USA), and detected with an X-ray film. The signal on the film was quantified with GelQuant.NET software provided by biochemlabsolutions.com.
Statistical Analysis
The normality of the data distribution and homogeneity of variances were tested by Kolmogorov-Smirnoff, Shapiro–Wilk, and Levine tests. The data that deviated from the normal distribution were normalized the by Box-Cox transforming method. To test for the effects of the oxygen regime on the studied traits within each species, one-way ANOVA was used with the oxygen regime as a fixed factor with five levels (normoxia, 1 day hypoxia, reoxygenation after 1 day hypoxia, 6 days hypoxia, and reoxygenation after 6 days hypoxia). The effects of the experimental manipulation of the phosphorylation state was tested by one-way ANOVA within each species and oxygen condition using the experimental treatment as a fixed factor with four levels (stop solution corresponding to the native state, dephosphorylation by CeO2 and phosphorylation by activation of PKA or PKC). Tukey's honest significant difference (HSD) test was used to determine which pairs of means are significantly different from each other. Statistical analyses were performed using Statistica v. 12 (StatSoft Inc., Tulsa, OK, USA) and GraphPad Prism v. 8.02 (GraphPad Software Inc., La Jolla, CA, USA) software. The data are presented as means ± standard error of the means (SEM) unless indicated otherwise.
Results
Effects of H/R Stress on Activities of Protein Kinases
In control M. edulis, the activity of PKA and PKC in the digestive gland was high (4,479 ± 295 RU g−1 and 5,510 ± 814 RU g−1, respectively) and did not significantly change during hypoxia and reoxygenation except for a significant decrease of PKC activity after 1 day of hypoxia (Figures 1A,B). In A. islandica, activities of PKA and PKC were lower compared with those of M. edulis under the control conditions (748 ± 155 RU g−1 and 217 ± 31 RU g−1, respectively). Exposure to hypoxia and subsequent reoxygenation led to a strong elevation of the PKA and PKC activities in A. islandica (Figures 1C,D). In C. gigas, PKA and PKC activities under the control conditions were similar to those of A. islandica (828 ± 128 RU g−1 and 187 ± 32 RU g−1, respectively). Short-term (1 day) hypoxia and subsequent reoxygenation had no effect on the activity of PKA and PKC in C. gigas. The long-term (6 days) hypoxia strongly suppressed PKA and PKC activities in the digestive gland of C. gigas, and this suppression was not reversed after 1 h of reoxygenation (Figures 1E,F).
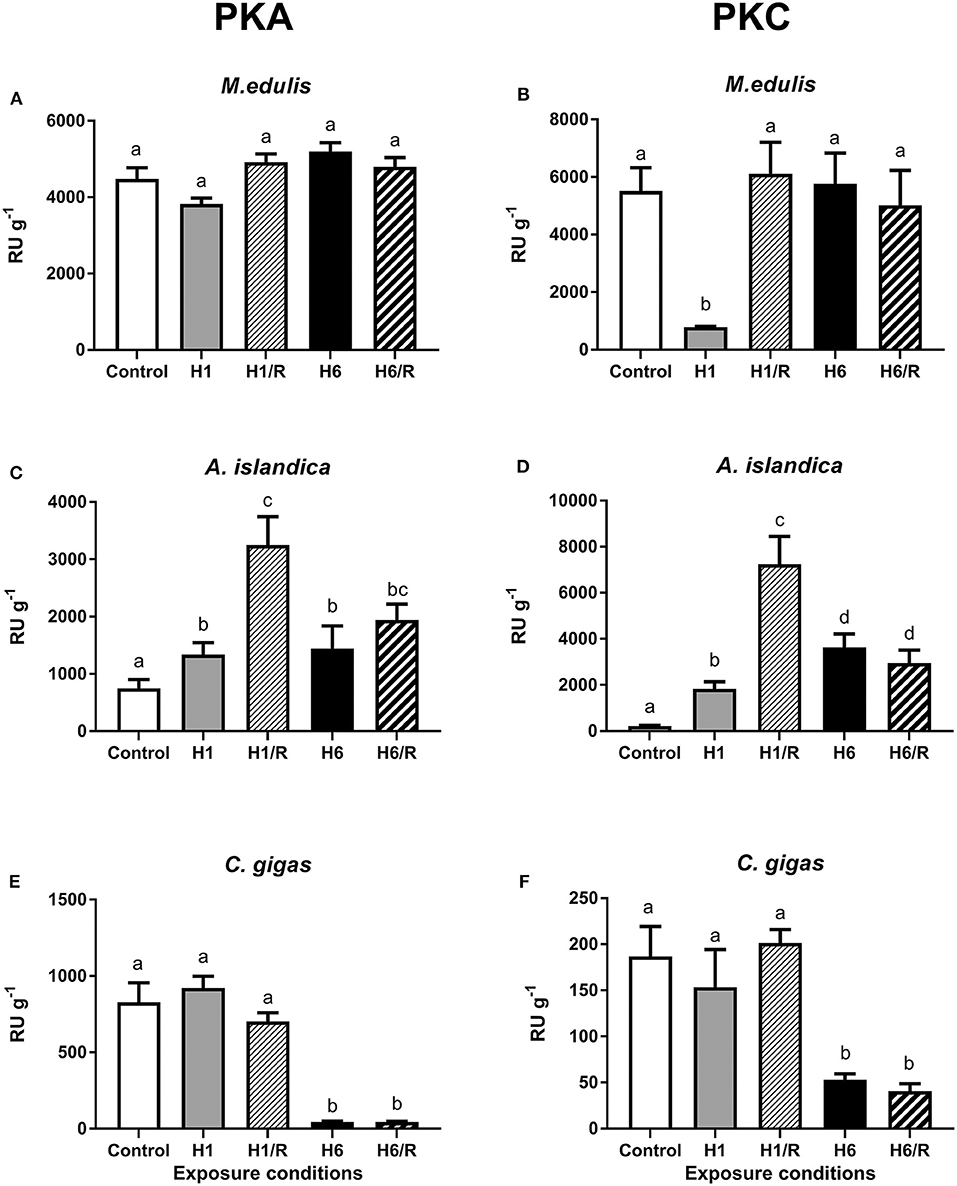
Figure 1. Activities of PKA and PKC in the digestive gland of three species of marine bivalves during the exposure to H/R stress. (A,B) The mussels M. edulis, (C,D) the quahogs A. islandica, and (E,F) the oysters C. gigas. Exposure conditions are control (normoxia), 1 day hypoxia (H1), 1 h reoxygenation following 1 day of hypoxia (H1/R), 6 days of hypoxia (H6), and 1 h reoxygenation following 6 days of hypoxia (H6/R). Activities are given in relative units (RU) per gram wet tissue mass. Columns that do not share a letter represent significantly different values (p < 0.05). The vertical bars represent S.E.M. N = 5.
Effects of H/R Stress on Activities of Protein Phosphatases
The baseline (normoxic) activity levels of protein phosphatases were the lowest in M. edulis and the highest in C. gigas (Figure 2). In M. edulis, H/R stress had no effect on the protein phosphatase activities (Figure 2A). In A. islandica, the activity of the protein phosphatases decreased by ~40% during the short-term (1 day) hypoxia and increased by ~40–70% (compared to the normoxic baseline) during the long-term hypoxia and subsequent reoxygenation (Figure 2B). In C. gigas, a slight (~25–35%) but statistically significant increase in the protein phosphatase activities was observed under all H/R treatment conditions compared with the normoxic controls (Figure 2C).
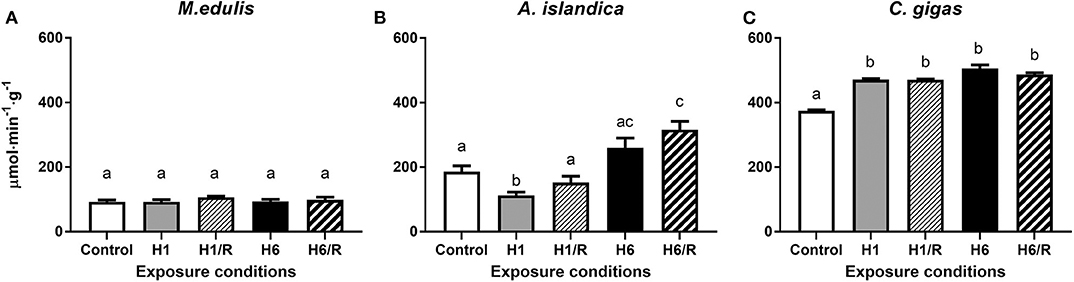
Figure 2. Activities of protein phosphatases in the digestive gland of three species of marine bivalves during the exposure to H/R stress. (A) The mussels M. edulis, (B) the quahogs A. islandica, and (C) the oysters C. gigas. Exposure conditions are control (normoxia), 1 day hypoxia (H1), 1 h reoxygenation following 1 day of hypoxia (H1/R), 6 days of hypoxia (H6), and 1 h reoxygenation following 6 days of hypoxia (H6/R). Activities are expressed in μmol product per minute per gram wet tissue mass. Columns that do not share a letter represent significantly different values (p < 0.05). The vertical bars represent S.E.M. N = 6.
Effects of H/R Stress on the Cellular Levels of Phosphorylated Proteins
The levels of PKA- and PKC-dependent phosphorylation of cellular proteins decreased during hypoxia and reoxygenation in the digestive gland of M. edulis (Figures 3A,B). This trend was significant for the PKC-dependent but not for PKA-dependent phosphorylation (Figures 3A,B). There was also a trend for a stronger decrease in the levels of phosphorylated proteins after the long-term (6 days) compared with the short-term (1 day) hypoxia in M. edulis, albeit this trend was only statistically significant for the PKC-dependent phosphorylation (P < 0.05; Figures 3A,B).
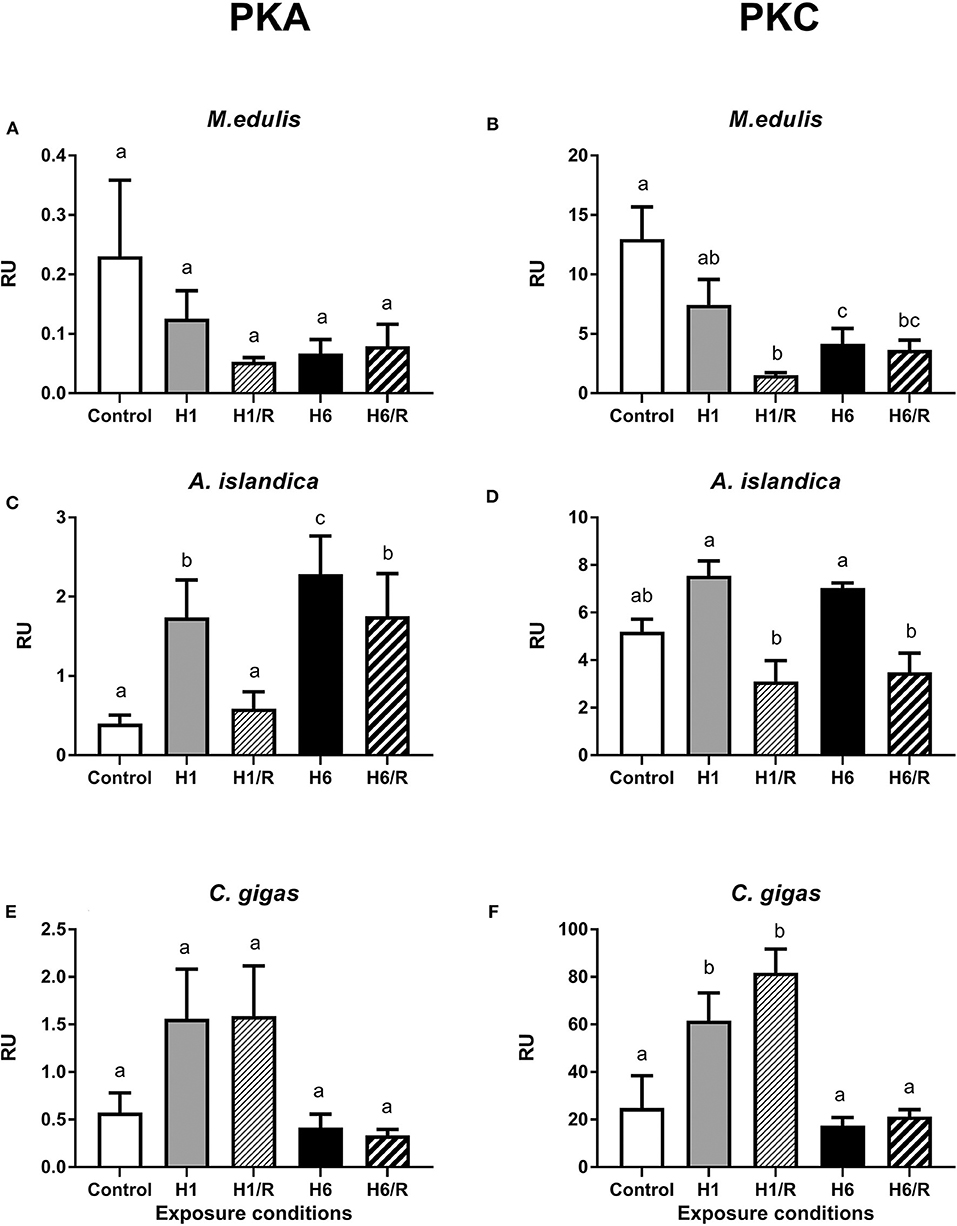
Figure 3. Amount of PKA- and PKC-phosphorylated sites on the digestive gland proteins of three species of marine bivalves during the exposure to H/R stress. (A,B) The mussels M. edulis, (C,D) the quahogs A. islandica, and (E,F) the oysters C. gigas. Exposure conditions are control (normoxia), 1 day hypoxia (H1), 1 h reoxygenation following 1 day of hypoxia (H1/R), 6 days of hypoxia (H6), and 1 h reoxygenation following 6 days of hypoxia (H6/R). RU, relative units. Columns that do not share a letter represent significantly different values (p < 0.05). The vertical bars represent S.E.M. N = 5.
In A. islandica, short-term hypoxia led to an increase of the PKA-dependent phosphorylation levels of the proteins that returned to the baseline after 1 h of recovery (Figure 3C). Long-term (6 days) hypoxia led to a strong increase in the PKA-dependent protein phosphorylation levels, which remained high after 1 h of recovery (Figure 3C). The levels of PKC-dependent protein phosphorylation were higher during hypoxia than during the reoxygenation in A. islandica, albeit neither of the values were significantly different from the normoxic controls (Figure 3D).
In C. gigas, the short-term hypoxia and reoxygenation led to an increase in the PKA- and PKC-dependent phosphorylation levels in the digestive gland proteins albeit this increase was only statistically significant for the PKC-dependent sites (Figures 3E,F). The PKA- and PKC-dependent protein phosphorylation levels in the digestive gland of C. gigas after the long-term hypoxia and reoxygenation were similar to the normoxic baseline (Figures 3E,F).
Activity of Complex I and IV During H/R Stress
In M. edulis, the maximum activity (Vmax) of the Complex I increased after 1 and 6 days of hypoxia and subsequent recovery albeit these trends were not statistically significant (Figure 4A). In A. islandica, the Complex I activity decreased after the short-term (1 day) hypoxia, but returned to the baseline levels after 1 h of reoxygenation (Figure 4B). Long-term (6 days) hypoxia and subsequent reoxygenation had no effect on the Complex I activity in A. islandica. In C. gigas, activity of Complex I decreased after 1 day and 6 days of hypoxia, and remained below the control levels following 1 h reoxygenation after the short-term (but not long-term) hypoxia (Figure 4C).
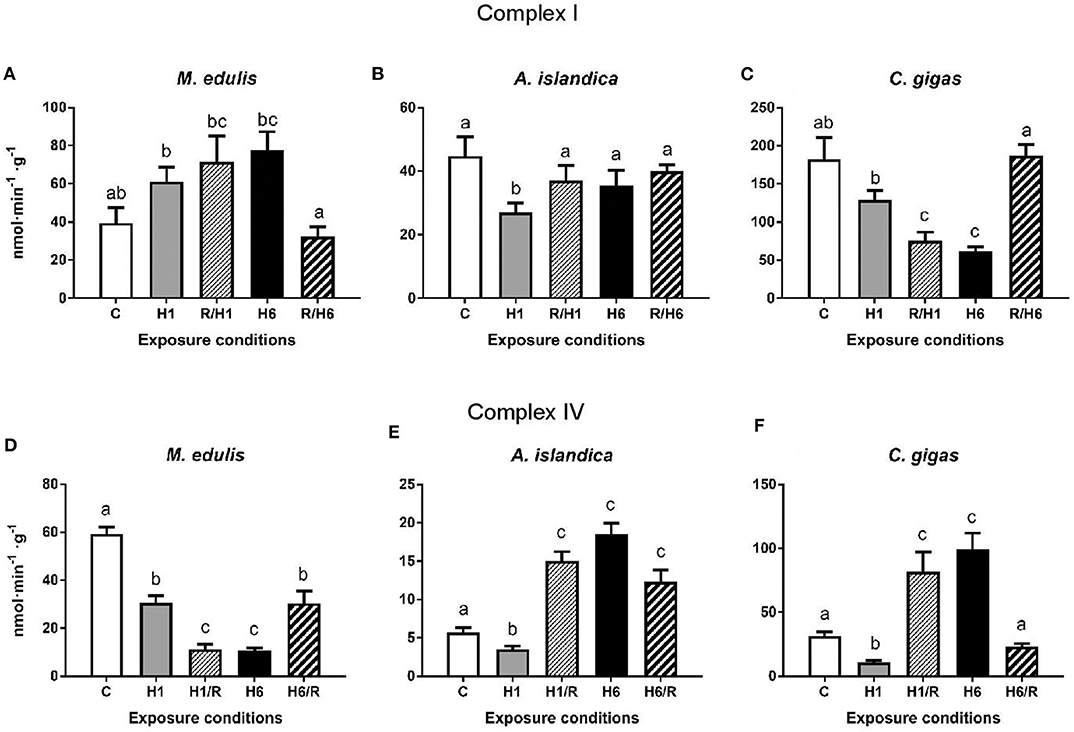
Figure 4. Activities of the mitochondrial Complexes I and IV in the digestive gland of three species of marine bivalves during the exposure to H/R stress. (A,D) The mussels M. edulis, (B,E) the quahogs A. islandica, and (C,F) the oysters C. gigas. (A–C) The activity of mitochondrial Complex I, (D–F) the activity of mitochondrial Complex IV. Enzymes were isolated in the presence of phosphatase and kinase inhibitors to preserve the native phosphorylation state. Exposure conditions are control (normoxia), 1 day hypoxia (H1), 1 h reoxygenation following 1 day of hypoxia (H1/R), 6 days of hypoxia (H6), and 1 h reoxygenation following 6 days of hypoxia (H6/R). Activities are expressed in nmol product per minute per gram wet tissue mass. Columns that do not share a letter represent significantly different values (p < 0.05). The vertical bars represent S.E.M. N = 6.
In M. edulis, exposure to short-term (1 day) or long-term (6 days) hypoxia led to a significant decline of the Complex IV activity that remained below the control levels during post-hypoxic recovery (Figure 4D). In A. islandica and C. gigas, short-term (1 day) hypoxia led to a decrease of the Complex IV activity; however, the Complex IV activity rapidly increased after 1 h of recovery above the respective control (normoxic) levels (Figures 4E,F). Long-term (6 days) hypoxia also led to an increase in the Complex IV activity in A. islandica and C. gigas. After 1 h of recovery following 6 days of hypoxia, the activity of Complex IV remained elevated in A. islandica but returned to the normoxic control level in C. gigas (Figures 4E,F).
Putative Phosphorylation Sites on the Complex I and IV Subunits
In the seven mitochondrially-encoded core Complex I subunits (ND1, ND2, ND3, ND4, ND4L, ND5, and ND6), a total of 505 predicted phosphorylation sites was found across all studied species. Most (470) of these sites were species-specific. Only 24 predicted phosphorylation sites were shared by two out of the three studied species, and 11 sites were shared by all three species (Supplementary Table 1). Similarly, out of 250 predicted phosphorylation sites on the three mitochondrially-encoded Complex IV subunits (COX1, COX 2, and COX3), most (211) sites were unique for each studied species, with 35 sites found in two out of three studied species and 4 phosphorylation sites shared among all three studied species (Supplementary Table 2). The relative fraction of species-specific (unique) phosphorylation sites was higher on the mitochondrially-encoded Complex I subunits compared to the Complex IV subunits (93 vs. 84%, respectively; Table 1).
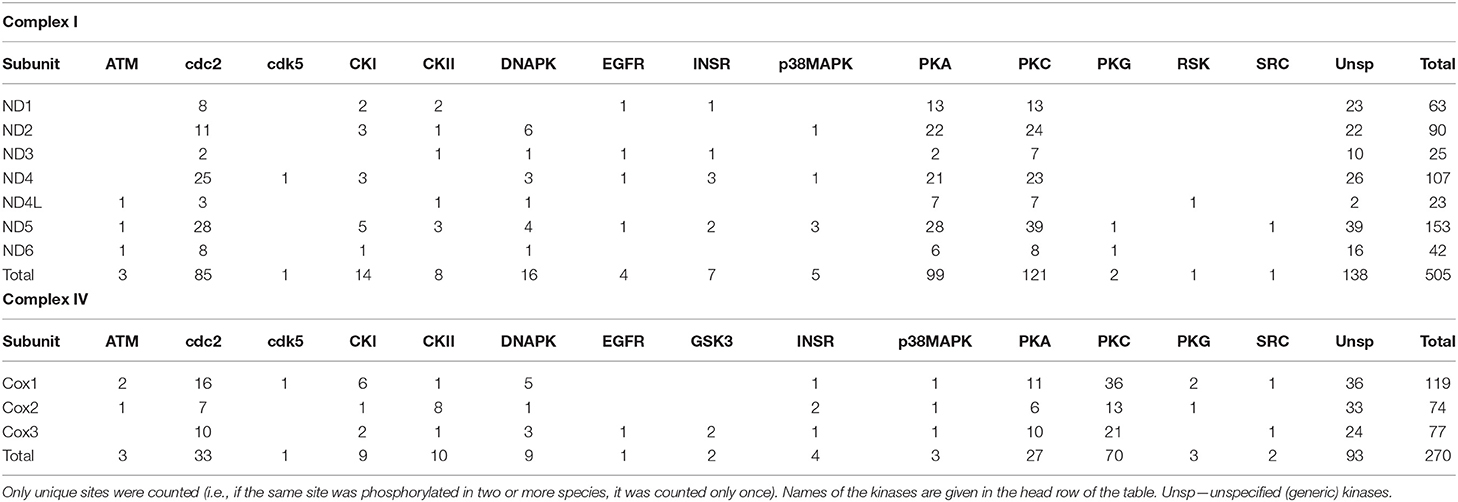
Table 1. The total number of the predicted phosphorylation sites on the core mitochondrially-encoded subunits of Complex I and Complex IV in the three studied species of bivalves.
The amount of the putative phosphorylation sites was unevenly distributed between different studied Complex I and Complex IV subunits, reflecting different sizes of these subunits. Thus, the small subunits of the Complex I (ND4L and ND3) had the least putative phosphorylation sites (23 and 25 across all studied species, respectively), and the largest subunit ND5 had the most (155 across all studied species). Of the Complex IV subunits, COX1, COX 2, and COX3 had 119, 74, and 77 putative phosphorylation sites, respectively.
Predicted phosphorylation sites indicate that protein kinases PKA, PKC, and cdc2 potentially play the greatest role of the PTM of the core subunits of Complexes I and IV of the studied bivalve species. Thus, across all studied species, 99 (27%) sites belonged to PKA, 121 (33%) sites to PKC, and 85 (23%) sites to the cdc2 kinase (disregarding the sites for unspecified generic kinases) (Table 1). The kinases PKA, PKC, and cdc2 accounted, respectively, for 25, 41, and 23% of the phosphorylation sites on Complex I subunits in M. edulis, 31, 25, and 24% in A. islandica, and 23, 37, and 19% in C. gigas (Table 2).
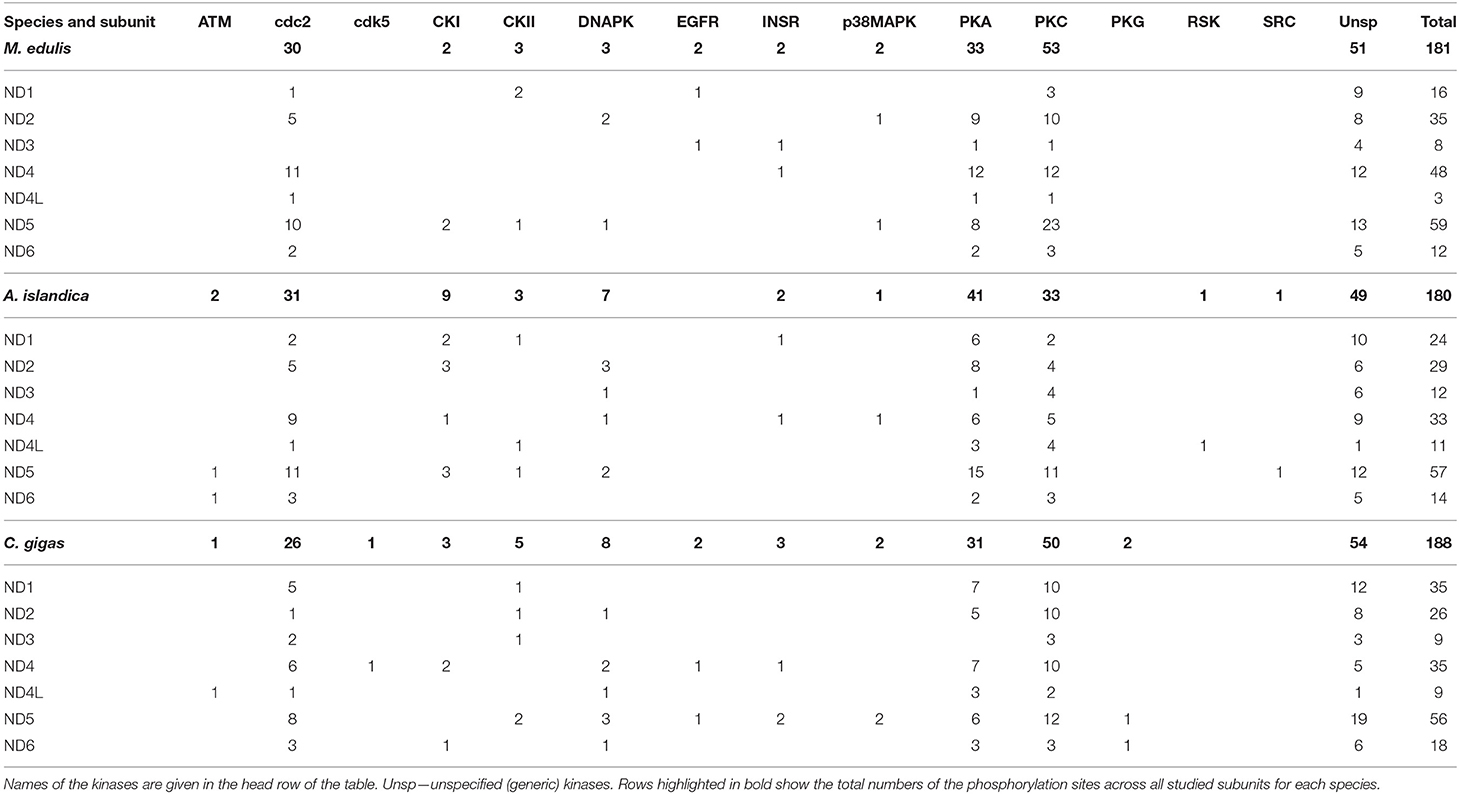
Table 2. Distribution of the predicted phosphorylation sites on the core mitochondrial subunits of Complex I in the three studied species.
Of the 177 kinase-specific phosphorylation sites on the studied Complex IV subunits, 27 (15%) sites belong to PKA, 70 (40%) sites to PKC, and 33 (19%) sites to cdc2 kinase (Table 1). This pattern was similar across all studied species with the percentage of the putative phosphorylation sites for PKA, PKC, and cdc2, respectively: 14, 39, and 21% of in M. edulis, 16, 32, and 19% in A. islandica, and 16, 52, and 14% in C. gigas (Table 3). For the subsequent functional analyses, we focused on the PKA and PKC kinases that play an important role in regulation of Complex I and IV activities, based on the published studies in mammalian models, and jointly account for ~50% of phosphorylation sites on the core subunits of Complexes I and IV of the three studied species of marine bivalves.
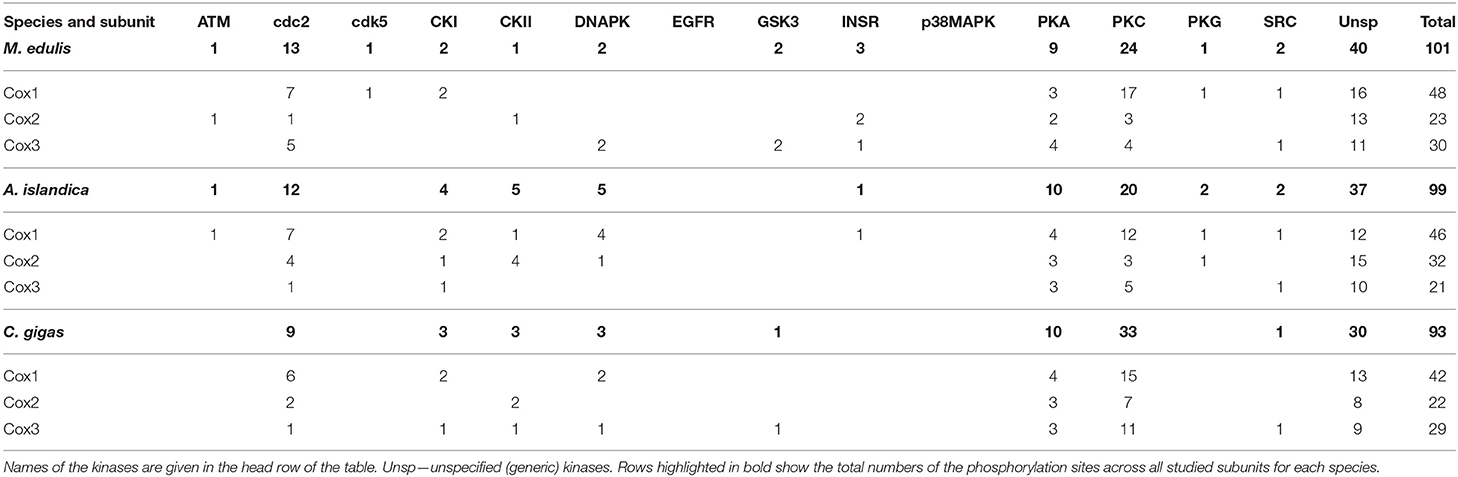
Table 3. Distribution of the predicted phosphorylation sites on the mitochondrial subunits of Complex IV in the three studied species.
Responses of Complex I Activity to the Changes in Phosphorylation Status
In M. edulis, experimental dephosphorylation by CeO2 significantly suppressed the activity of the mitochondrial Complex I (Figure 5A and Supplementary Figure 3). The effects of the stimulation of PKA and PKC activity on the activity of Complex I depended on the experimental treatment of the mussels. In the mussels acclimated under the normoxic conditions or recovering after the long-term (6 days) hypoxia, phosphorylation by PKA led to a considerable increase of the Complex I activity relative to the enzyme in its native phosphorylation state (Supplementary Figure 3). In the mussels exposed to 1 day or 6 days of hypoxia, or in those recovering after the short-term (1 day) hypoxia, no increase in the Complex I activity was observed in response to the PKA treatment (Supplementary Figure 3).
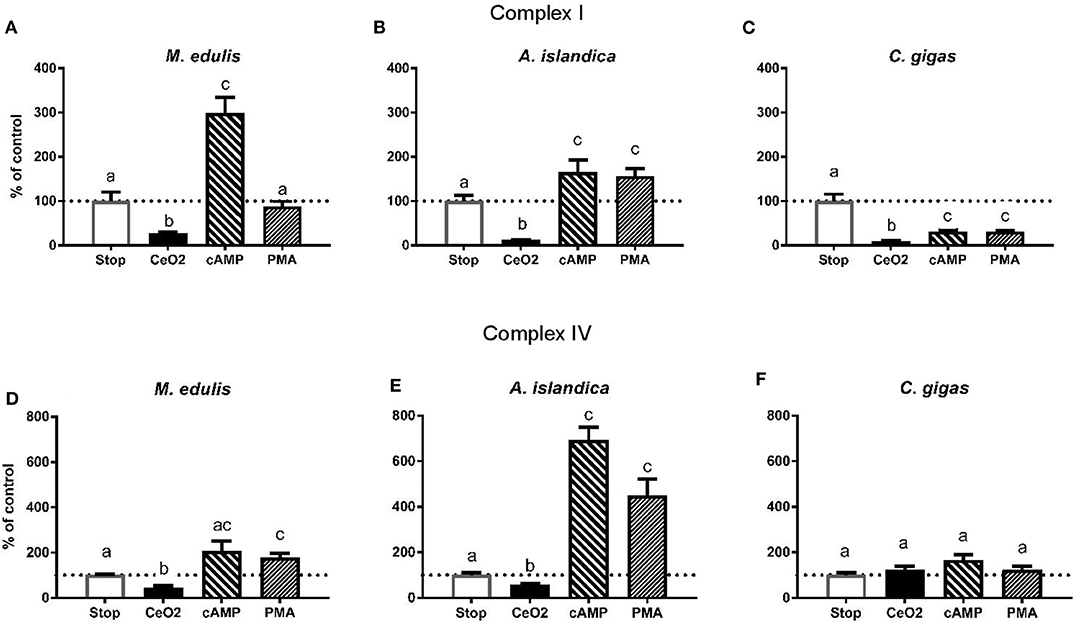
Figure 5. Effects of the experimental manipulation of the phosphorylation state on the activities of the mitochondrial Complexes I and IV in the digestive gland of three species of marine bivalves. (A,D) The mussels M. edulis, (B,E) the quahogs A. islandica, (C,F) the oysters C. gigas. (A–C) The activity of mitochondrial Complex I, (D–F) the activity of mitochondrial Complex IV. Enzymes were isolated from the control (normoxic) animals and treated with CeO2 (to dephosphorylate the enzymes), cAMP or phorbol myristate acetate to stimulate PKA and PKC activity, respectively. Stop solution contained inhibitors of phosphatases and kinases to preserve the native phosphorylation state. Activities are expressed as % of the respective activity in the native phosphorylation state. Columns that do not share a letter represent significantly different values (p < 0.05). The vertical bars represent S.E.M. N = 6.
Stimulation of the PKC had no effect on the Complex I activity in the control mussels, as well as in those exposed to the short-term hypoxia or subsequent recovery. In the mussels exposed to 6 days of hypoxia and subsequent recovery, phosphorylation by PKC led to a suppression of the Complex I activity compared to the respective native phosphorylation state (Supplementary Figure 3). Except for the mussels recovering after 6 days of hypoxia (which showed consistently low Complex I activity regardless of the phosphorylation state), stimulation of the PKA led to the maximum Complex I activity similar in all H/R treatment and normoxic controls (shown by the solid red line on Supplementary Figure 3).
In A. islandica, dephosphorylation by CeO2 led to a suppression of the Complex I activity (Figure 5B and Supplementary Figure 4). Stimulation of the PKA or PKC activity led to an increase in the Complex I activity relative to the native phosphorylation state in all experimental treatments of the quahogs (Supplementary Figure 4). The maximum PKA-stimulated activity of the Complex I in A. islandica was similar under most experimental conditions, except for the animals exposed to 6 days of hypoxia or recovering after 1 day of hypoxia, which showed enhanced stimulation of the Complex I activity by PKA (Supplementary Figure 4).
Dephosphorylation by CeO2 decreased the Complex I activity in C. gigas (Figure 5C and Supplementary Figure 5). Unlike M. edulis and A. islandica, experimental stimulation of PKA and PKC activity led to a decline of the Complex I activity of C. gigas relative to the respective native state of the enzyme. This trend was significant under most experimental conditions except after 6 days of hypoxia and reoxygenation following 1 day of hypoxia, where the native activity of Complex I was low and did not further decrease in response to the experimental (de-)phosphorylation (Supplementary Figure 5).
Of the three studied species, the Complex I of A. islandica showed the largest increase in activity due to phosphorylation by PKA compared to the dephosphorylated state (~12 to 17-fold increase under the most conditions) (Table 4). In M. edulis and C. gigas, the ratio of Complex I activity in the PKA-phosphorylated to dephosphorylated state was ~4 to 12-fold and ~2 to 11-fold, respectively. The PKC-induced increase in Complex I activity (relative to the dephosphorylated state) was lower in all three studied species, ranging between ~1.3 and 5-fold (Table 4).
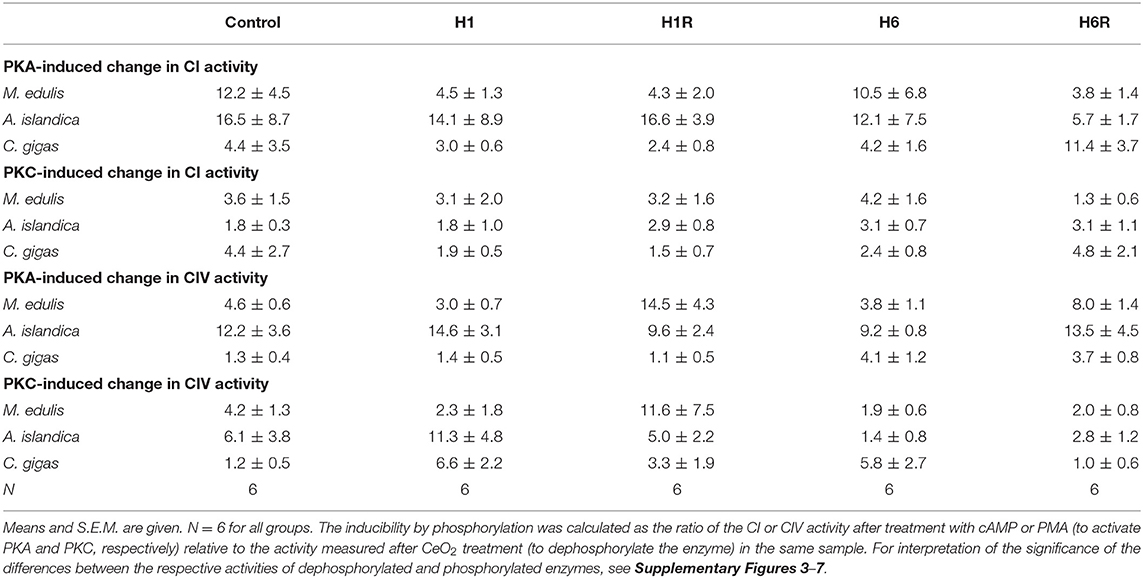
Table 4. Inducibility of CI and CIV activity by PKA and PKC-dependent phosphorylation relative to the dephosphorylated state.
Responses of Complex IV Activity to the Changes in Phosphorylation Status
In M. edulis, dephosphorylation by CeO2 led to a decrease in the Complex IV activity except in the mussels exposed to 6 days of hypoxia, where the low native levels of the Complex IV activity were not affected by CeO2 treatment (Figure 5D and Supplementary Figure 6). Stimulation of PKA increased the Complex IV activity compared to the respective native state except in the mussels exposed to the normoxic condition or 1 day of hypoxia (Supplementary Figure 6). Stimulation of PKC increased the Complex IV activity in the mussels exposed to normoxia or recovery after 1 day of hypoxia (Supplementary Figure 6) but had little or no effect under other experimental conditions.
In A. islandica, dephosphorylation by CeO2 caused mild inhibition of the Complex IV activity (Figure 5E and Supplementary Figure 6). Stimulation of PKA and PKC led to a strong increase in the Complex IV activity in A. islandica in all experimental exposures (Supplementary Figure 7).
In C. gigas, experimental manipulation of the phosphorylation state had little effect on the Complex IV activity under the control (normoxic) conditions (Figure 5F and Supplementary Figure 8). Dephosphorylation by CeO2 suppressed the Complex IV activity in oysters exposed to 6 days of hypoxia or recovering after 1 day of hypoxia compared to the respective native state, but not in other experimental treatments (Supplementary Figure 8). Responses of the Complex IV activity to PKA and PCK stimulation were strongly condition-dependent. Thus, stimulation of the PKA decreased the activity of Complex I in the oysters recovering after 1 days of hypoxia, increased the Complex IV activity in the oysters recovering after 6 days of hypoxia, and had little or no effect under other experimental conditions. Stimulation of the PKC activity led to an increase of the Complex IV activity above the respective native state in the oysters exposed to 1 day of hypoxia but not in other experimental treatments (Supplementary Figure 8).
An increase in the Complex IV activity due to PKA- or PKC-induced phosphorylation (compared with the dephosphorylated state) was higher in A. islandica (~9 to 15-fold and ~3 to 11-fold increase for PKA and PKC, respectively) and M. edulis (~3 to 14-fold and ~2 to 12-fold increase) compared with C. gigas (~1.1 to 4-fold and ~1 to 7-fold increase for PKA and PKC, respectively; Table 4).
Discussion
H/R-Induced Modulation of Kinase and Phosphatase Activities in Marine Bivalves
PKA and PKC are ubiquitous serine/threonine kinases localized in the cytosol and mitochondria of animals (Lim et al., 2016). These enzymes play important roles in regulating cellular metabolism by transducing multiple intracellular signals mediated by cAMP (PKA) or phospholipid hydrolysis (PKC) (Newton, 1997; Taylor et al., 2005). Mollusks are characterized by a broad molecular and functional diversity of PKA and PKC enzymes with demonstrated roles in regulation of the muscle contraction, cytoskeleton, neuronal function and glycolysis (Brooks and Storey, 1997; MacDonald and Storey, 1999; Bardales et al., 2004; Béjar and Villamarín, 2006; Sossin and Abrams, 2009). Our study shows high activities of PKA and PKC in the digestive gland of the three studied bivalve mollusks as well as the presence of PKA- and PKC-phosphorylated residues in multiple cellular proteins.
Earlier studies in humans and model organisms demonstrated an important role of PKA and PKC in responses to H/R stress such as occurs during ischemia-reperfusion (Armstrong, 2004; Sanada et al., 2004; Hüttemann et al., 2012). In hypoxia-sensitive terrestrial mammals, PKA and PKC are activated by hypoxia (Srinivasan et al., 2013; Gozal et al., 2017; Lucia et al., 2020), whereas in a nematode Caenorhabditis elegans, hypoxia suppresses PKA activity (Han et al., 2019). Our present study showed a strong species-specific modulation of the protein kinase activities by H/R stress in marine bivalves ranging from no change (except for a transient decrease in PKC during short-term hypoxia) in M. edulis, an increase during hypoxia and reoxygenation in A. islandica, and a strong suppression after the long-term hypoxia in C. gigas. In mammals, activation of PKA and PKC may have protective or damaging effects during H/R stress depending on the timing of activation (i.e., during preconditioning vs. hypoxic exposure) and the isoform of the protein kinase that becomes activated (Armstrong, 2004; Sanada et al., 2004; Miura et al., 2010; Duquesnes et al., 2011; Yang et al., 2013; Gozal et al., 2017). Physiological implications of the H/R-induced modulation of the PKA and PKC activity are not known in marine bivalves, and further studies are needed to determine the consequences of the modulation of these enzymes for cellular signaling during hypoxia.
The species-specific changes in the protein kinase activity during hypoxia correlated with the changes in the overall levels of the phosphorylated serine/threonine residues in the proteins. In M. edulis, the relatively constant levels of PKA activity were associated with the unchanging amounts of the phosphorylated PKA substrates in the digestive gland (cf. Figures 3A, 4A). In C. gigas and A. islandica, H/R induced increases and decreases in the PKA activity were broadly reflected in the increases and decreases of the PKA-specific phosphorylated residues in the digestive gland (cf. Figures 1C,E, 2C,E). The relationship was less clear for the PKC-mediated phosphorylation. These discrepancies may be due to the changes in the protein phosphatase activities or compartmentalization of PKC in the bivalve tissues. These findings indicate that the kinase-specific protein phosphorylation levels in crude protein extracts can be a useful indicator of the overall tissue activity of PKA but not PKC in the digestive gland of the three studied species of marine bivalves. In contrast, the total phosphatase activity was not correlated with the levels of the phosphorylated protein residues in the digestive gland of the three studies species likely because the total phosphatase activity measured in our study involves multiple phosphatases and not only those specific to PKA- and PKC-phosphorylated residues.
Reversible Phosphorylation as a Regulatory Mechanism for ETS Activity in Bivalves
Bioinformatics analysis discovered a large number (23–153) of the putative phosphorylation sites on the mitochondrially-encoded subunits of Complexes I and IV in the studied marine bivalves. The number of the predicted phosphorylation sites depended on the overall size of the respective subunit, with an average of one phosphorylation site for each 3–5 amino acids. The most frequent phosphorylation sites on the bivalve Complex I mitochondrial subunits corresponded to the recognition sites for the protein kinase A (99 sites) and protein kinase C (121 sites), similar to the human Complex I (Gowthami et al., 2019). The putative PKA and PKC phosphorylation sites were also highly represented (27 and 70 sites, respectively) on the Complex IV subunits of the studied marine bivalves. While a comparable bioinformatics map could not be found for mammals, the important role of PKA-dependent phosphorylation in regulation of the Complex IV activity has been shown experimentally in humans and other mammals (Helling et al., 2012; Castellanos and Lanning, 2019).
High number of the candidate phosphorylation sites in the Complexes I and IV of marine bivalves indicates a considerable potential for this PTM mechanism to regulate ETS activity, even if not all the putative phosphorylation sites undergo reversible phosphorylation in vivo (Chen et al., 2004; Papa et al., 2012; Gowthami et al., 2019). This hypothesis is supported by the results of the experimental manipulation of the enzyme phosphorylation state showing that mitochondrial Complexes I and IV of marine bivalves are sensitive to the regulation by reversible protein phosphorylation. Thus, the Complex I activity was strongly suppressed by the experimental dephosphorylation by CeO2 in all three studied species compared to the enzyme in the native phosphorylation state. This finding is similar to the earlier reports in humans and mammalian models showing deactivation of the Complex I by experimental dephosphorylation or by the site-directed mutagenesis removing the critical phosphorylation residues on Complex I subunits (Ould Amer and Hebert-Chatelain, 2018; Castellanos and Lanning, 2019). Interestingly, unlike humans, rodents, and marine bivalves, dephosphorylation by alkaline phosphatase activated Complex I in the 13-lined ground squirrels (Ictidomys tridecemlineatus) (Mathers and Staples, 2019). This activation by dephosphorylation was found only in the Complex I isolated from torpid (but not from active) squirrels (Mathers and Staples, 2019).
In all three studied species, the activity of Complex I was elevated in the PKA-phosphorylated state albeit the degree of activation was higher in M. edulis and A. islandica (~12 to 16-fold) than in C. gigas (~4-fold). Effects of the PKC-induced phosphorylation on Complex I activity were weaker than PKA and similar in the three studied species (~2 to 4-fold increase compared to the fully dephosphorylated state). These findings are in agreement with the earlier studies in humans and mammalian models that showed the critical importance of serine/threonine phosphorylation on Complex I subunits in the assembly and activation of this enzyme complex (Castellanos and Lanning, 2019). Interestingly, in C. gigas activity of the Complex I was higher in its native state compared to the PKA- and PKC- phosphorylated or dephosphorylated states. This indicates that PTM-dependent activation of this enzyme in oysters might involve other kinases not included in the present study.
Similar to Complex I, dephosphorylation by CeO2 led to a strong decrease in Complex IV activity in M. edulis and A. islandica, while PKA- and PKC-dependent phosphorylation stimulated this complex's activity. The amplitude of the difference in Complex IV activity between the dephosphorylated and PKA- or PKC-phosphorylated state was larger in A. islandica (~12 and 6-fold for PKA and PKC, respectively) than in M. edulis (~4 to 5-fold). In C. gigas, the activity of Complex IV was insensitive to experimental phosphorylation and dephosphorylation under the normoxic conditions. In humans and mammalian models, Complex IV activity is modulated by phosphorylation albeit the effects appear to be site-dependent (Castellanos and Lanning, 2019). Thus, PKA-dependent phosphorylation of serine 58 improves the Complex I activity in the human and mouse cells (Acin-Perez et al., 2011; Castellanos and Lanning, 2019), whereas phosphorylation of tyrosine 304 suppresses Complex I and increases the cellular reliance on glycolysis (Samavati et al., 2008). In mammals, PKA-dependent phosphorylation of the Complex I subunit COX5B also suppresses Complex IV activity during reperfusion (Hüttemann et al., 2012).
It is worth noting that our present study used an in vitro approach that cannot differentiate between different PKA and PKC targets on the bivalve Complexes I and IV and does not permit determining the site-specific effects of phosphorylation on the activity of these enzyme complexes. Furthermore, even though crude tissue extracts used in our study retain all the essential components of the cellular phosphorylation machinery, some auxiliary components (such as the scaffolding proteins that ensure specificity of the phosphorylation sites) might be altered during homogenization (Hüttemann et al., 2012). Despite these caveats, our study shows a strong potential of reversible phosphorylation by PKA and PKC as the PTM mechanism regulating the ETS activity in marine bivalves and demonstrates considerable species-specific sensitivity of Complexes I and IV to these PTMs, with C. gigas enzymes being the least sensitive. Future in vivo studies using targeted phosphoproteomics are required to identify the PKA- and PKC phosphorylation targets in the bivalve ETS complexes and assess the implications of the site-specific protein phosphorylation for the enzyme activities.
Activity of the Mitochondrial Complex I and IV During H/R Stress: A Role for PTM?
Mitochondrial Complexes I and IV are recognized as the key targets for H/R stress involved both in the adaptive response and the stress-induced injury caused by oxygen fluctuations (Kim et al., 2011). Our study showed differential responses of the mitochondrial Complex I to H/R stress in the three studied species of hypoxia-tolerant marine bivalves (Table 5). In A. islandica and C. gigas, both short- and long-term hypoxia led to a suppression of Complex I activity (by ~20–40% and ~30–70% in A. islandica and C. gigas, respectively). These findings agree with an earlier report of a decrease in the oxygen consumption rates of intact mitochondria of C. gigas respiring with Complex I substrates (such as pyruvate, malate, or glutamate) (Sussarellu et al., 2013; Sokolov et al., 2019). Notably, the oxygen consumption of mitochondria respiring with a Complex II substrate (succinate) remained stable or slightly elevated during H/R stress in C. gigas (Kurochkin et al., 2008; Sokolov et al., 2019). This indicates that a decline in the pyruvate- and glutamate-driven mitochondrial respiration in oysters (Sussarellu et al., 2013; Sokolov et al., 2019) reflects inhibition of the Complex I activity (such as found in our present study) rather than suppression of the downstream ETS complex(es). A decrease in the Complex I activity is considered a major adaptive response to H/R stress that suppresses the mitochondrial ROS generation (Fuhrmann and Brüne, 2017). Therefore, the hypoxia-induced suppression of Complex I activity and electron flux capacity might have protective effects in the mitochondria of A. islandica and C. gigas.
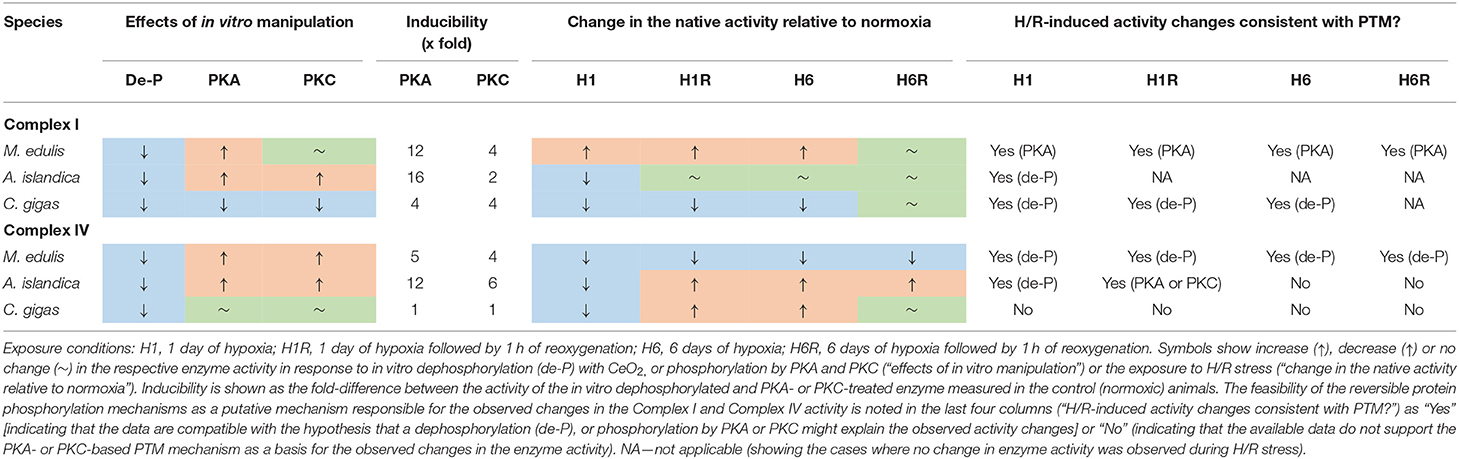
Table 5. Summary of the responses to H/R stress and in vitro manipulation of the protein phosphorylation state on the mitochondrial Complex I and IV of the three studied bivalve species.
In the quahogs A. islandica, Complex I activity remained strongly responsive to PKA and PKC-dependent activation in all experimental H/R treatments (Supplementary Figure 3), so that the activity of Complex I in the native state was intermediate between the fully dephosphorylated and PKA- or PKC-phosphorylated states. This makes it difficult to unequivocally infer the potential role of PKA and PKC in the modulation of the Complex I activity during the H/R stress in the quahogs. Interestingly, during reoxygenation following the short-term hypoxia, the activity of the fully PKA- or PKC-activated Complex I of A. islandica was >2-fold higher than the respective values for the fully activated normoxic enzyme (Supplementary Figure 3C), indicating an increase in the abundance of the Complex I during reoxygenation. In C. gigas, the H/R-induced modulation of the Complex I activity in C. gigas unlikely involved the reversible phosphorylation by PKA and PKC. Either of these protein kinases failed to stimulate the Complex I activity of oysters and, in most experimental exposures, suppressed the Complex I activity below the native state (Supplementary Figure 4). It is conceivable that a decrease in the Complex I activity during hypoxia reflects dephosphorylation of the enzyme, but if this is the case, reactivation of the enzyme during recovery must occur through other protein kinases not investigated in our present study.
Unlike A. islandica and C. gigas, hypoxia led to a modest (~1.6 to 2.0-fold) increase in Complex I activity in M. edulis. This change was statistically not significant due to the large individual variation (P > 0.05), yet an increasing trend of Complex I activity in the mussels was observed both after 1 and 6 days of hypoxia. The blue mussels M. edulis are considered hypoxia-tolerant like many intertidal bivalves (Vaquer-Sunyer and Duarte, 2008). However, the Baltic Sea population of M. edulis used in this study was reportedly less hypoxia tolerant, possibly due to the weakening effects of low salinity (Falfushynska et al., 2020; Haider et al., 2020). Therefore, the lack of the adaptive suppression of Complex I activity during hypoxia is in line with the lower hypoxia tolerance of the mussels compared with the two other studied species of bivalves. The hypoxia-induced increase in the Complex I activity in M. edulis might reflect cAMP-dependent phosphorylation of this complex by PKA. This suggestion is supported by the finding that the activity of Complex I in the hypoxia-exposed mussels is similar in the native and PKA-activated states (Supplementary Figure 2). PKC-dependent phosphorylation is likely not involved in this modulation, since Complex I of the H/R-exposed mussels (like that of the normoxic controls) was insensitive to the activation by PKC.
The change in the overall activity of the mitochondrial Complex IV as well as its responsiveness to the activation by PKA and PKC strongly depended on the duration of hypoxia exposure in the three studies species. In M. edulis, both the short- and long-term hypoxia led to a strong suppression of Complex IV activity by ~2- and 6-fold, respectively. The suppression of Complex IV activity during hypoxia and reoxygenation in mussels likely reflected a decrease in the amount of the potentially active enzyme rather than effects of dephosphorylation, because activation by PKA or PKC did not restore the normal (normoxic) activity of Complex IV (Supplementary Figure 5). Post-hypoxic reoxygenation for an hour failed to restore the normoxic levels of Complex IV activity in the mussels indicating slow mitochondrial recovery.
In A. islandica, short-term hypoxia suppressed the Complex IV activity by ~1.6-fold whereas the long-term hypoxia stimulated the Complex IV activity by ~3-fold. A decrease in the Complex IV activity during the short-term hypoxia in A. islandica can be explained by dephosphorylation of the enzyme, whereas the elevated activity of Complex IV during the long-term hypoxia reflect an increased enzyme amount (Supplementary Figure 6). An increase in the amount of the potentially active enzyme also played a role in the increase of Complex IV activity during post-hypoxic reoxygenation in A. islandica, albeit a contribution of the PKA- or PKC-dependent phosphorylation cannot be excluded.
In C. gigas, the pattern of the hypoxia-induced changes in the Complex IV activity was similar to that observed in A. islandica. Thus, short-term hypoxia suppressed the Complex IV activity of oysters by ~3-fold whereas the long-term hypoxia stimulated the Complex IV activity by ~3-fold. However, the mechanisms underlying these changes appeared to be different in oysters compared to the quahogs. In oysters, the short-term hypoxia and subsequent reoxygenation strongly increased responsiveness of the Complex IV to activation by PKC while PKA failed to activate this enzyme. Suppression of the Complex IV activity after the short-term hypoxia in oysters reflected a decrease in the enzyme abundance, while its recovery during the 1st hour of reoxygenation was consistent with the PKC-dependent activation (Supplementary Figure 7). Similar to A. islandica, activation of Complex IV activity after the long-term hypoxia in oysters was indicative of the elevated amount of the enzyme rather that the kinase-induced activation. Interestingly, the activity of Complex IV returned to the normoxic baseline levels after 1 h of recovery following the long-term hypoxia, but the enzyme had different properties relative to the one found in normoxic animals, as it was highly responsive to PKA activation (Supplementary Figure 7). These data indicate that even though the Complex IV of oysters is insensitive to PKA- and PKC-dependent modulation under the normoxic condition, the H/R-induced changes in the enzyme properties (such as conformation, accessibility of the phosphorylation sites, and/or isoform composition) can strongly affect the regulatory potential of PKA and PKC on the Complex IV activity in this species.
In hypoxia-sensitive terrestrial mammals such as humans and rodents, suppression of the activity of mitochondrial Complex IV during hypoxia (ischemia) or ischemic preconditioning protects the tissue from reoxygenation-induced injury (Yang et al., 2017; Sanderson et al., 2018). Inhibition of the Complex IV activity during hypoxia in mammals is commonly mediated by phosphorylation of serine or threonine residues on several Complex IV subunits (Prabu et al., 2006; Fang et al., 2007) albeit the identity of kinases involved in this response remains controversial (Hüttemann et al., 2012). PKA can also inhibit the Complex IV activity in mammalian liver indirectly by activating downstream kinases that phosphorylate Tyr304 (Hüttemann et al., 2012). Interestingly, activation of the soluble adenylyl cyclase by bicarbonate in mammalian mitochondria results in PKA-dependent phosphorylation of Complex IV that releases allosteric inhibition of Complex IV by ATP and increases its activity (Acin-Perez et al., 2009, 2011). This regulatory pathway is unlikely to be involved in the Complex IV regulation in bivalves as an earlier study showed that bicarbonate inhibits (rather than activates) the ETS activity of marine bivalves via a cAMP-independent mechanism (Haider et al., 2016). Phosphorylation of cytochrome c also plays a role in regulation of the Complex IV activity in mammals (Hüttemann et al., 2012); however, this mechanism is not relevant to our present study because exogenous cytochrome c was used in all enzymatic assays. Suppression of the Complex IV activity such as found in ischemic preconditioning in mammals (Yang et al., 2017; Sanderson et al., 2018) and during the short-term hypoxia in marine bivalves (present study) may play a protective role as it prevents hyperactivation of Complex IV by the mitochondrial Ca2+ overload during reoxygenation and thereby curtails the production of ROS and mitochondrial injury (Hüttemann et al., 2012). Interestingly, the suppression of the Complex IV activity was reversed after the long-term (6 days) hypoxia in the two most tolerant species, A. islandica and C. gigas, indicating that after prolonged hypoxia other protective mechanisms (such as suppression of the Complex I activity) might play more important role.
Conclusions and outlook
Our present study combining bioinformatics, immunohistological, and biochemical approaches provides evidence for the importance of the reversible phosphorylation as a potential regulatory mechanism involved in the cellular response to H/R stress of the hypoxia-tolerant marine bivalves. Activities of the mitochondrial Complexes I and IV emerged as important targets for modulation by H/R stress in marine bivalves, and the observed hypoxia-induced changes in the activities of these enzymes are consistent with the adaptive mitochondrial phenotype earlier described in hypoxia-sensitive terrestrial mammals during ischemic preconditioning that enhances tolerance to H/R stress (Kim et al., 2011; Yang et al., 2017; Sanderson et al., 2018). Stimulation of PKA and PKC led to activation of the Complex I and IV activities in M. edulis and A. islandica regardless of the oxygen exposure conditions, with PKA being a stronger activator. In C. gigas, Complexes I and IV activities were suppressed by PKA- and PKC-induced phosphorylation except under certain experimental conditions (e.g., short-term hypoxia and reoxygenation) where the effects of PKA and PKC on the Complex IV activity were reversed. The species-specific differences in responses to reversible phosphorylation are consistent with the observation that most phosphorylation sites on Complex I and IV subunits are not homologous across different bivalve species. These findings caution against extrapolation of the PTM-dependent regulatory mechanisms identified in one species and/or physiological state to other species and physiological states. Interpretation of the functional implications of the PTM must be supported by the direct measurements of the activities of enzymes in different phosphorylation states for each target species and ideally corroborated by the manipulations of the PTM in the living cells and organisms to assess their effects on the enzyme activities in situ.
Data Availability Statement
The raw data supporting the conclusions of this article will be made available by the authors, without undue reservation, to any qualified researcher. The project metadata can be found in an open access database PANGAEA® (Sokolova et al., 2020).
Author Contributions
HF: conceptualization, data curation, formal analysis, funding acquisition, methodology, investigation, visualization, writing—original draft, and writing—review and editing. ES: data curation, formal analysis, methodology, investigation, visualization, writing—original draft, and writing—review and editing. HP: data curation, formal analysis, methodology, writing—original draft, and writing—review and editing. IS: conceptualization, funding acquisition, methodology, validation, resources, supervision, project administration, writing—original draft, and writing—review and editing. All authors contributed to the article and approved the submitted version.
Funding
This work was in part supported by the German Research Foundation (DFG) project MitoBOX (DFG Award Nos. 415984732 and GZ: SO 1333/5-1) to IS, Alexander von Humboldt Fellowship and MV-2 (MES of Ukraine) to HF, and by the funding line Strategic Networks of the Leibniz Association within the scope of the Leibniz ScienceCampus Phosphorus Research Rostock (www.sciencecampus-rostock.de) to ES and IS. HP was partially supported by Kent State University Brain Health Institute Pilot Award.
Conflict of Interest
The authors declare that the research was conducted in the absence of any commercial or financial relationships that could be construed as a potential conflict of interest.
Acknowledgments
We thank Mathias Wegner (Alfred Wegener Institute Helmholtz Center for Polar and Marine Research, Wadden Sea Station Sylt) for providing oysters for this study.
Supplementary Material
The Supplementary Material for this article can be found online at: https://www.frontiersin.org/articles/10.3389/fmars.2020.00467/full#supplementary-material
References
Acin-Perez, R., Gatti, D. L., Bai, Y., and Manfredi, G. (2011). Protein phosphorylation and prevention of cytochrome oxidase inhibition by ATP: coupled mechanisms of energy metabolism regulation. Cell Metab. 13, 712–719. doi: 10.1016/j.cmet.2011.03.024
Acin-Perez, R., Salazar, E., Kamenetsky, M., Buck, J., Levin, L. R., and Manfredi, G. (2009). Cyclic AMP produced inside mitochondria regulates oxidative phosphorylation. Cell Metab. 9, 265–276. doi: 10.1016/j.cmet.2009.01.012
Ali, S. S., Hsiao, M., Zhao, H. W., Dugan, L. L., Haddad, G. G., and Zhou, D. (2012). Hypoxia-adaptation involves mitochondrial metabolic depression and decreased ROS leakage. PLoS ONE 7:e36801. doi: 10.1371/journal.pone.0036801
Andrienko, T. N., Pasdois, P., Pereira, G. C., Ovens, M. J., and Halestrap, A. P. (2017). The role of succinate and ROS in reperfusion injury: a critical appraisal. J. Mol. Cell. Cardiol. 110, 1–14. doi: 10.1016/j.yjmcc.2017.06.016
Armstrong, S. C. (2004). Protein kinase activation and myocardial ischemia/reperfusion injury. Cardiovasc. Res. 61, 427–436. doi: 10.1016/j.cardiores.2003.09.031
Bardales, J. R., Díaz-Enrich, M. J., Ibarguren, I., and Villamarín, J. A. (2004). Isoforms of cAMP-dependent protein kinase in the bivalve mollusk Mytilus galloprovincialis: activation by cyclic nucleotides and effect of temperature. Arch. Biochem. Biophys. 432, 71–78. doi: 10.1016/j.abb.2004.09.008
Béjar, P., and Villamarín, J. A. (2006). Catalytic subunit of cAMP-dependent protein kinase from a catch muscle of the bivalve mollusk Mytilus galloprovincialis: purification, characterization, and phosphorylation of muscle proteins. Arch. Biochem. Biophys. 450, 133–140. doi: 10.1016/j.abb.2006.02.024
Birch-Machin, M. A., and Turnbull, D. M. (2001). Assaying mitochondrial respiratory complex activity in mitochondria isolated from human cells and tissues. Methods Cell Biol. 65, 97–117. doi: 10.1016/S0091-679X(01)65006-4
Blom, N., Gammeltoft, S., and Brunak, S. (1999). Sequence and structure-based prediction of eukaryotic protein phosphorylation sites. J. Mol. Biol. 294, 1351–1362. doi: 10.1006/jmbi.1999.3310
Blom, N., Sicheritz-Pontén, T., Gupta, R., Gammeltoft, S., and Brunak, S. (2004). Prediction of post-translational glycosylation and phosphorylation of proteins from the amino acid sequence. Proteomics 4, 1633–1649. doi: 10.1002/pmic.200300771
Blomgren, K., Zhu, C., Hallin, U., and Hagberg, H. (2003). Mitochondria and ischemic reperfusion damage in the adult and in the developing brain. Biochem. Biophys. Res. Commun. 304, 551–559. doi: 10.1016/S0006-291X(03)00628-4
Bradford, M. M. (1976). A rapid and sensitive method for the quantitation of microgram quantities of protein utilizing the principle of protein-dye binding. Anal. Chem. 72, 248–254. doi: 10.1016/0003-2697(76)90527-3
Breitburg, D., Levin, L. A., Oschlies, A., Grégoire, M., Chavez, F. P., Conley, D. J., et al. (2018). Declining oxygen in the global ocean and coastal waters. Science 359:eaam7240. doi: 10.1126/science.aam7240
Brooks, S. P. J., and Storey, K. B. (1997). Glycolytic controls in estivation and anoxia: A comparison of metabolic arrest in land and marine molluscs. Comp. Biochem. Physiol. 118A, 1103–1114. doi: 10.1016/S0300-9629(97)00237-5
Buck, L. T., and Pamenter, M. E. (2006). Adaptive responses of vertebrate neurons to anoxia–matching supply to demand. Respir. Physiol. Neurobiol. 154, 226–240. doi: 10.1016/j.resp.2006.03.004
Burnett, L. E. (1997). The challenges of living in hypoxic and hypercapnic aquatic environments. Am. Zool. 37, 633–640. doi: 10.1093/icb/37.6.633
Camara, A., Bienengraeber, M., and Stowe, D. (2011). Mitochondrial approaches to protect against cardiac ischemia and reperfusion injury. Front. Physiol. 2:3. doi: 10.3389/fphys.2011.00013
Castellanos, E., and Lanning, N. J. (2019). Phosphorylation of OXPHOS machinery subunits: functional implications in cell biology and disease. Yale J. Biol. Med. 92, 523–531.
Chen, R., Fearnley, I. M., Peak-Chew, S. Y., and Walker, J. E. (2004). The phosphorylation of subunits of complex I from bovine heart mitochondria. J. Biol. Chem. 279, 26036–26045. doi: 10.1074/jbc.M402710200
Dagda, R. K., and Das Banerjee, T. (2015). Role of protein kinase A in regulating mitochondrial function and neuronal development: implications to neurodegenerative diseases. Rev. Neurosci. 26, 359–370. doi: 10.1515/revneuro-2014-0085
Devaux, J. B. L., Hedges, C. P., Birch, N., Herbert, N., Renshaw, G. M. C., and Hickey, A. J. R. (2019). Acidosis maintains the function of brain mitochondria in hypoxia-tolerant triplefin fish: a strategy to survive acute hypoxic exposure? Front. Physiol. 9:1941. doi: 10.3389/fphys.2018.01941
Dudek, J. (2017). Role of cardiolipin in mitochondrial signaling pathways. Front. Cell Develop. Biol. 5:90. doi: 10.3389/fcell.2017.00090
Duquesnes, N., Lezoualc'h, F., and Crozatier, B. (2011). PKC-delta and PKC-epsilon: foes of the same family or strangers? J. Mol. Cell. Cardiol. 51, 665–673. doi: 10.1016/j.yjmcc.2011.07.013
Edgar, R. C. (2004). MUSCLE: multiple sequence alignment with high accuracy and high throughput. Nucleic Acids Res. 32, 1792–1797. doi: 10.1093/nar/gkh340
Eismann, T., Huber, N., Shin, T., Kuboki, S., Galloway, E., Wyder, M., et al. (2009). Peroxiredoxin-6 protects against mitochondrial dysfunction and liver injury during ischemia-reperfusion in mice. Am. J. Physiol. Gastrointest. Liver Physiol. 296, G266–G274. doi: 10.1152/ajpgi.90583.2008
Falfushynska, H., Piontkivska, H., and Sokolova, I. M. (2020). Effects of intermittent hypoxia on cell survival and inflammatory responses in the intertidal marine bivalves Mytilus edulis and Crassostrea gigas. J. Exp. Biol. 223:jeb217026. doi: 10.1242/jeb.217026
Fang, J.-K., Prabu, S. K., Sepuri, N. B., Raza, H., Anandatheerthavarada, H. K., Galati, D., et al. (2007). Site specific phosphorylation of cytochrome c oxidase subunits I, IVi1 and Vb in rabbit hearts subjected to ischemia/reperfusion. FEBS Lett. 581, 1302–1310. doi: 10.1016/j.febslet.2007.02.042
Fuhrmann, D. C., and Brüne, B. (2017). Mitochondrial composition and function under the control of hypoxia. Redox Biol. 12, 208–215. doi: 10.1016/j.redox.2017.02.012
Fukuda, R., Zhang, H., Kim, J.-W., Shimoda, L., Dang, C. V., and Semenza, G. L. (2007). HIF-1 regulates cytochrome oxidase subunits to optimize efficiency of respiration in hypoxic cells. Cell 129, 111–122. doi: 10.1016/j.cell.2007.01.047
Gerber, L., Clow, K. A., Katan, T., Emam, M., Leeuwis, R. H. J., Parrish, C. C., et al. (2019). Cardiac mitochondrial function, nitric oxide sensitivity and lipid composition following hypoxia acclimation in sablefish. J. Exp. Biol. 222:jeb208074. doi: 10.1242/jeb.208074
Gowthami, N., Sunitha, B., Kumar, M., Keshava Prasad, T. S., Gayathri, N., Padmanabhan, B., et al. (2019). Mapping the protein phosphorylation sites in human mitochondrial complex I (NADH: ubiquinone oxidoreductase): a bioinformatics study with implications for brain aging and neurodegeneration. J. Chem. Neuroanat. 95, 13–28. doi: 10.1016/j.jchemneu.2018.02.004
Gozal, E., Metz, C. J., Dematteis, M., Sachleben, L. R. Jr., Schurr, A., and Rane, M. J. (2017). PKA activity exacerbates hypoxia-induced ROS formation and hypoxic injury in PC-12 cells. Toxicol. Lett. 279, 107–114. doi: 10.1016/j.toxlet.2017.07.895
Haider, F., Falfushynska, H., Ivanina, A. V., and Sokolova, I. M. (2016). Effects of pH and bicarbonate on mitochondrial functions of marine bivalves. Comp. Biochem. Physiol. Part A Mol. Integr. Physiol. 198, 41–50. doi: 10.1016/j.cbpa.2016.03.021
Haider, F., Falfushynska, H. I., Timm, S., and Sokolova, I. M. (2020). Effects of hypoxia and reoxygenation on intermediary metabolite homeostasis of marine bivalves Mytilus edulis and Crassostrea gigas. Compar. Biochem. Physiol. Part A Mol. Integr. Physiol. 242:110657. doi: 10.1016/j.cbpa.2020.110657
Haider, F., Sokolov, E. P., and Sokolova, I. M. (2018). Effects of mechanical disturbance and salinity stress on bioenergetics and burrowing behavior of the soft-shell clam Mya arenaria. J. Exp. Biol. 221:jeb172643. doi: 10.1242/jeb.172643
Ham, P. B. III., and Raju, R. (2016). Mitochondrial function in hypoxic ischemic injury and influence of aging. Prog. Neurobiol. 157, 92–116. doi: 10.1016/j.pneurobio.2016.06.006
Han, J. S., Lee, J. H., Kong, J., Ji, Y., Kim, J., Choe, S. S., et al. (2019). Hypoxia restrains lipid utilization via protein kinase A and adipose triglyceride lipase downregulation through hypoxia-inducible factor. Mol. Cell. Biol. 39:e00390-18. doi: 10.1128/MCB.00390-18
Helling, S., Huttemann, M., Ramzan, R., Kim, S. H., Lee, I., Muller, T., et al. (2012). Multiple phosphorylations of cytochrome c oxidase and their functions. Proteomics 12, 950–959. doi: 10.1002/pmic.201100618
Hermes-Lima, M., and Zenteno-Savín, T. (2002). Animal response to drastic changes in oxygen availability and physiological oxidative stress. Compar. Biochem. Physiol. Part C Toxicol. Pharmacol. 133, 537–556. doi: 10.1016/S1532-0456(02)00080-7
Hernansanz-Agustín, P., Ramos, E., Navarro, E., Parada, E., Sánchez-López, N., Peláez-Aguado, L., et al. (2017). Mitochondrial complex I deactivation is related to superoxide production in acute hypoxia. Redox Biol. 12, 1040–1051. doi: 10.1016/j.redox.2017.04.025
Huggett, J., and Griffiths, C. L. (1986). Some relationships between elevation, physicochemical variables and biota of intertidal rock pools. Mar. Ecol. Prog. Ser. 29, 189–197. doi: 10.3354/meps029189
Hüttemann, M., Helling, S., Sanderson, T. H., Sinkler, C., Samavati, L., Mahapatra, G., et al. (2012). Regulation of mitochondrial respiration and apoptosis through cell signaling: cytochrome c oxidase and cytochrome c in ischemia/reperfusion injury and inflammation. Biochim. Biophys. Acta 1817, 598–609. doi: 10.1016/j.bbabio.2011.07.001
Ivanina, A. V., Habinck, E., and Sokolova, I. M. (2008). Differential sensitivity to cadmium of key mitochondrial enzymes in the eastern oyster, Crassostrea virginica Gmelin (Bivalvia: Ostreidae). Comp. Biochem. Physiol. C Toxicol. Pharmacol. 148, 72–79. doi: 10.1016/j.cbpc.2008.03.009
Ivanina, A. V., Nesmelova, I., Leamy, L., Sokolov, E. P., and Sokolova, I. M. (2016). Intermittent hypoxia leads to functional reorganization of mitochondria and affects cellular bioenergetics in marine molluscs. J. Exp. Biol. 219, 1659–1674. doi: 10.1242/jeb.134700
Kalogeris, T., Baines, C. P., Krenz, M., and Korthuis, R. J. (2012). Cell biology of ischemia/reperfusion injury. Int. Rev. Cell Mol. Biol. 298, 229–317. doi: 10.1016/B978-0-12-394309-5.00006-7
Kalpage, H. A., Bazylianska, V., Recanati, M. A., Fite, A., Liu, J., Wan, J., et al. (2019). Tissue-specific regulation of cytochrome c by post-translational modifications: respiration, the mitochondrial membrane potential, ROS, and apoptosis. FASEB J. 33, 1540–1553. doi: 10.1096/fj.201801417R
Kim, H. K., Thu, V. T., Heo, H. J., Kim, N., and Han, J. (2011). Cardiac proteomic responses to ischemia-reperfusion injury and ischemic preconditioning. Expert Rev. Proteomics 8, 241–261. doi: 10.1586/epr.11.8
Kumar, S., Stecher, G., and Tamura, K. (2016). MEGA7: molecular evolutionary genetics analysis version 7.0 for bigger datasets. Mol. Biol. Evol. 33, 1870–1874. doi: 10.1093/molbev/msw054
Kurochkin, I., Ivanina, A., Eilers, S., and Sokolova, I. (2008). Effects of environmental anoxia and re-oxygenation on mitochondrial function and metabolism of eastern oysters(Crassostrea virginica). Compar. Biochem. Physiol. Part A Mol. Integr. Physiol. 150:S161. doi: 10.1016/j.cbpa.2008.04.420
Legrand, E., Riera, P., Pouliquen, L., Bohner, O., Cariou, T., and Martin, S. (2018). Ecological characterization of intertidal rockpools: seasonal and diurnal monitoring of physico-chemical parameters. Regional Stud. Mar. Sci. 17, 1–10. doi: 10.1016/j.rsma.2017.11.003
Lim, S., Smith, K. R., Lim, S.-T. S., Tian, R., Lu, J., and Tan, M. (2016). Regulation of mitochondrial functions by protein phosphorylation and dephosphorylation. Cell Biosci. 6:25. doi: 10.1186/s13578-016-0089-3
Liu, T., Chen, L., Kim, E., Tran, D., Phinney, B. S., and Knowlton, A. A. (2014). Mitochondrial proteome remodeling in ischemic heart failure. Life Sci. 101, 27–36. doi: 10.1016/j.lfs.2014.02.004
Lucero, M., Suarez, A. E., and Chambers, J. W. (2019). Phosphoregulation on mitochondria: Integration of cell and organelle responses. CNS Neurosci. Ther. 25, 837–858. doi: 10.1111/cns.13141
Lucia, K., Wu, Y., Garcia, J. M., Barlier, A., Buchfelder, M., Saeger, W., et al. (2020). Hypoxia and the hypoxia inducible factor 1α activate protein kinase A by repressing RII beta subunit transcription. Oncogene 39, 3367–3380. doi: 10.1038/s41388-020-1223-6
MacDonald, J. A., and Storey, K. B. (1999). Cyclic AMP-dependent protein kinase: role in anoxia and freezing tolerance of the marine periwinkle Littorina littorea. Mar. Biol. 133, 193–203. doi: 10.1007/s002270050458
Mahapatra, G., Varughese, A., Ji, Q., Lee, I., Liu, J., Vaishnav, A., et al. (2016). Phosphorylation of cytochrome c threonine 28 regulates electron transport chain activity in kidney: implications for AMP kinase. J. Biol. Chem. 292, 64–79. doi: 10.1074/jbc.M116.744664
Martos-Sitcha, J. A., Bermejo-Nogales, A., Calduch-Giner, J. A., and Pérez-Sánchez, J. (2017). Gene expression profiling of whole blood cells supports a more efficient mitochondrial respiration in hypoxia-challenged gilthead sea bream (Sparus aurata). Front. Zool. 14:34. doi: 10.1186/s12983-017-0220-2
Mathers, K. E., and Staples, J. F. (2019). Differential posttranslational modification of mitochondrial enzymes corresponds with metabolic suppression during hibernation. Am. J. Physiol. Regul. Integr. Comp. Physiol. 317, R262–R269. doi: 10.1152/ajpregu.00052.2019
McAvoy, T., and Nairn, A. C. (2010). Serine/threonine protein phosphatase assays. Curr. Protoc. Mol. Biol. Chapter 18, Unit 18.18. doi: 10.1002/0471142727.mb1818s92
Miura, T., Tanno, M., and Sato, T. (2010). Mitochondrial kinase signalling pathways in myocardial protection from ischaemia/reperfusion-induced necrosis. Cardiovasc. Res. 88, 7–15. doi: 10.1093/cvr/cvq206
Napolitano, G., Venditti, P., Fasciolo, G., Esposito, D., Uliano, E., and Agnisola, C. (2019). Acute hypoxia/reoxygenation affects muscle mitochondrial respiration and redox state as well as swimming endurance in zebrafish. J. Comp. Physiol. B Biochem. Syst. Environ. Physiol. 189, 97–108. doi: 10.1007/s00360-018-1198-6
Newton, A. C. (1997). Regulation of protein kinase C. Curr. Opin. Cell Biol. 9, 161–167. doi: 10.1016/S0955-0674(97)80058-0
Ogbi, M., and Johnson, J. A. (2006). Protein kinase Cϵ interacts with cytochrome c oxidase subunit IV and enhances cytochrome c oxidase activity in neonatal cardiac myocyte preconditioning. Biochem. J. 393, 191–199. doi: 10.1042/BJ20050757
Ould Amer, Y., and Hebert-Chatelain, E. (2018). Mitochondrial cAMP-PKA signaling: what do we really know? Biochim. Biophys. Acta 1859, 868–877. doi: 10.1016/j.bbabio.2018.04.005
Pamenter, M. E. (2014). Mitochondria: a multimodal hub of hypoxia tolerance. Can. J. Zool. 92, 569–589. doi: 10.1139/cjz-2013-0247
Papa, S., Rasmo, D. D., Technikova-Dobrova, Z., Panelli, D., Signorile, A., Scacco, S., et al. (2012). Respiratory chain complex I, a main regulatory target of the cAMP/PKA pathway is defective in different human diseases. FEBS Lett. 586, 568–577. doi: 10.1016/j.febslet.2011.09.019
Prabu, S. K., Anandatheerthavarada, H. K., Raza, H., Srinivasan, S., Spear, J. F., and Avadhani, N. G. (2006). Protein kinase A-mediated phosphorylation modulates cytochrome c oxidase function and augments hypoxia and myocardial ischemia-related injury. J. Biol. Chem. 281, 2061–2070. doi: 10.1074/jbc.M507741200
Samavati, L., Lee, I., Mathes, I., Lottspeich, F., and Huttemann, M. (2008). Tumor necrosis factor alpha inhibits oxidative phosphorylation through tyrosine phosphorylation at subunit I of cytochrome c oxidase. J. Biol. Chem. 283, 21134–21144. doi: 10.1074/jbc.M801954200
Sanada, S., Asanuma, H., Tsukamoto, O., Minamino, T., Node, K., Takashima, S., et al. (2004). Protein kinase A as another mediator of ischemic preconditioning independent of protein kinase C. Circulation 110, 51–57. doi: 10.1161/01.CIR.0000133390.12306.C7
Sanderson, T. H., Wider, J. M., Lee, I., Reynolds, C. A., Liu, J., Lepore, B., et al. (2018). Inhibitory modulation of cytochrome c oxidase activity with specific near-infrared light wavelengths attenuates brain ischemia/reperfusion injury. Sci. Rep. 8:3481. doi: 10.1038/s41598-018-21869-x
Sokolov, E. P., Markert, S., Hinzke, T., Hirschfeld, C., Becher, D., Ponsuksili, S., et al. (2019). Effects of hypoxia-reoxygenation stress on mitochondrial proteome and bioenergetics of the hypoxia-tolerant marine bivalve Crassostrea gigas. J. Proteomics 194, 99–111. doi: 10.1016/j.jprot.2018.12.009
Sokolova, I., Falfushynska, H., Sokolov, E., Piontkivska, H. (2020). The effects of hypoxia/reoxygenation stress on activities of mitochondrial complexes I and IV and activities of protein kinases and phosphatases in marine bivalves. PANGAEA. doi: 10.1594/PANGAEA.919121
Sokolova, I. M., Sokolov, E. P., and Haider, F. (2019). Mitochondrial mechanisms underlying tolerance to fluctuating oxygen conditions: lessons from hypoxia-tolerant organisms. Integr. Comp. Biol. 59, 938–952. doi: 10.1093/icb/icz047
Sossin, W. S., and Abrams, T. W. (2009). Evolutionary conservation of the signaling proteins upstream of cyclic AMP-dependent kinase and protein kinase C in gastropod mollusks. Brain Behav. Evol. 74, 191–205. doi: 10.1159/000258666
Srinivasan, S., Spear, J., Chandran, K., Joseph, J., Kalyanaraman, B., and Avadhani, N. G. (2013). Oxidative stress induced mitochondrial protein kinase A mediates cytochrome C oxidase dysfunction. PLoS ONE 8:e77129. doi: 10.1371/journal.pone.0077129
Stram, A. R., and Payne, R. M. (2016). Post-translational modifications in mitochondria: protein signaling in the powerhouse. Cell. Mol. Life Sci. 73, 4063–4073. doi: 10.1007/s00018-016-2280-4
Sussarellu, R., Dudognon, T., Fabioux, C., Soudant, P., Moraga, D., and Kraffe, E. (2013). Rapid mitochondrial adjustments in response to short-term hypoxia and re-oxygenation in the Pacific oyster, Crassostrea gigas. J. Exp. Biol. 216, 1561–1569. doi: 10.1242/jeb.075879
Tan, F., Zhang, Y., Wang, J., Wei, J., Cai, Y., and Qian, X. (2008). An efficient method for dephosphorylation of phosphopeptides by cerium oxide. J. Mass Spectrometry 43, 628–632. doi: 10.1002/jms.1362
Taylor, S. S., Kim, C., Vigil, D., Haste, N. M., Yang, J., Wu, J., et al. (2005). Dynamics of signaling by PKA. Biochim. Biophys. Acta 1754, 25–37. doi: 10.1016/j.bbapap.2005.08.024
Vaquer-Sunyer, R., and Duarte, C. M. (2008). Thresholds of hypoxia for marine biodiversity. Proc. Natl. Acad. Sci. U.S.A. 105, 15452–15457. doi: 10.1073/pnas.0803833105
Yang, C., Talukder, M. A., Varadharaj, S., Velayutham, M., and Zweier, J. L. (2013). Early ischaemic preconditioning requires Akt- and PKA-mediated activation of eNOS via serine1176 phosphorylation. Cardiovasc. Res. 97, 33–43. doi: 10.1093/cvr/cvs287
Yang, Z., Duan, Z., Yu, T., Xu, J., and Liu, L. (2017). Inhibiting cytochrome C oxidase leads to alleviated ischemia reperfusion injury. Korean Circ. J. 47, 193–200. doi: 10.4070/kcj.2016.0137
Keywords: hypoxia, reoxygenation, post-translational modification (PTM), mitochondrial electron transport chain (ETC), Mollusca, respiratory complexes, NADH:ubiquinone oxidoreductase, cytochrome c oxidase
Citation: Falfushynska HI, Sokolov E, Piontkivska H and Sokolova IM (2020) The Role of Reversible Protein Phosphorylation in Regulation of the Mitochondrial Electron Transport System During Hypoxia and Reoxygenation Stress in Marine Bivalves. Front. Mar. Sci. 7:467. doi: 10.3389/fmars.2020.00467
Received: 25 March 2020; Accepted: 26 May 2020;
Published: 02 July 2020.
Edited by:
Silvia Franzellitti, University of Bologna, ItalyReviewed by:
Claudio Agnisola, University of Naples Federico II, ItalyFenglian Xu, Saint Louis University, United States
Copyright © 2020 Falfushynska, Sokolov, Piontkivska and Sokolova. This is an open-access article distributed under the terms of the Creative Commons Attribution License (CC BY). The use, distribution or reproduction in other forums is permitted, provided the original author(s) and the copyright owner(s) are credited and that the original publication in this journal is cited, in accordance with accepted academic practice. No use, distribution or reproduction is permitted which does not comply with these terms.
*Correspondence: Inna M. Sokolova, inna.sokolova@uni-rostock.de
†These authors have contributed equally to this work