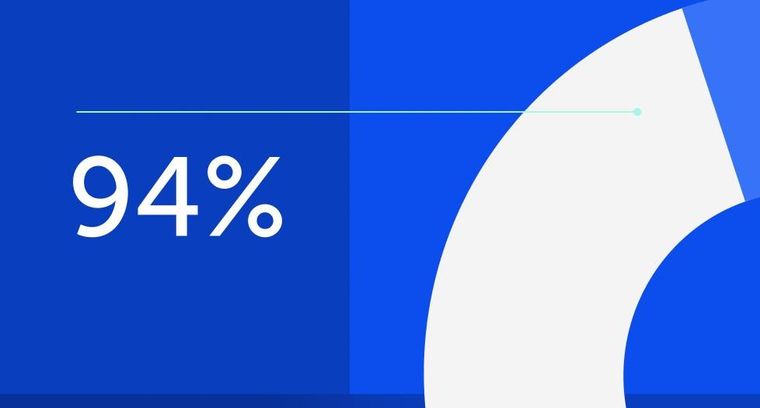
94% of researchers rate our articles as excellent or good
Learn more about the work of our research integrity team to safeguard the quality of each article we publish.
Find out more
ORIGINAL RESEARCH article
Front. Mar. Sci., 26 May 2020
Sec. Marine Ecosystem Ecology
Volume 7 - 2020 | https://doi.org/10.3389/fmars.2020.00320
This article is part of the Research TopicStudying the Biology of Aquatic Animals through Calcified StructuresView all 21 articles
The application and utility of otolith chemistry continues to expand despite an incomplete understanding of the mechanisms that regulate elemental incorporation. An unresolved question is what role individual factors such as growth play in regulating elemental incorporation. Disentangling growth variation from thermal effects is particularly challenging in fishes yet integral to understanding the mechanisms of incorporation and interpreting patterns of variation in the field. Juvenile Pacific cod (Gadus macrocephalus) were maintained in a controlled laboratory setting to evaluate the relative importance of growth rate, ration, and temperature on otolith elemental incorporation. Fish were held at four temperatures (2, 5, 9, 13°C) and fed daily to apparent satiation. An additional treatment included fish that were held at 9°C and fed a reduced ration (1% body mass d−1). Fish were maintained for variable duration (40–147 d), depending on ration and temperature, to ensure adequate otolith growth for analysis. Water samples for chemical analysis were collected to determine elemental partition coefficients (DMe). Overall, mean growth rates ranged from −0.09 to 1.52% d−1. For the 9°C fish, there was a clear ration effect on DMn (2.6X higher at high ration) and DSr (1.5X higher at low ration), a small effect for DMg (1.1X higher at high ration), and no effect for DBa. For high ration fish, there was a positive effect of temperature on DMn and DMg, due solely to differences associated with the 2°C treatment, and no effect on DSr and DBa. Correlations between growth and DMe within temperature treatments were variable, but for DMn and DSr the directionality mirrored the ration effect with positive correlations for DMn and negative correlations for DSr. Overall, the observed ration effects were greater than any growth rate effect, indicating that the effect of ration is due to more than growth variation.
Elemental analysis of accretionary hard tissues in aquatic animals is now widely used in a variety of disciplines, such as aquaculture (Yamada and Mulligan, 1982; Gibson-Reinemer et al., 2009) and climatology (Wurster and Patterson, 2001; Schloesser et al., 2009). While incomplete, our mechanistic understanding of the factors regulating elemental incorporation into hard structures, such as fish scales and otoliths, mollusk shells, and coral skeletons, has advanced through controlled experiments on (1) abiotic crystals, such as calcite and aragonite (Gaetani and Cohen, 2006; Lopez et al., 2009); (2) animal tissues that grow in direct contact with the water, such as coral skeletons and bivalve shells (Mitsuguchi et al., 2003; Holcomb et al., 2009; Marchitto et al., 2018); and (3) animal tissues that grow internally with multiple interfaces for the discrimination, or partitioning, of elements, such as teleost otoliths (Elsdon and Gillanders, 2003; Miller, 2009, 2011). For otolith elemental incorporation, physical characteristics, such as water elemental concentrations, temperature, salinity, and pH (Bath et al., 2000; Elsdon and Gillanders, 2004; DiMaria et al., 2010), as well as biological characteristics, such as growth, diet, and reproductive state (Buckel et al., 2004; Walther et al., 2010; Sturrock et al., 2015), have all been identified as factors that can influence elemental incorporation.
For many ecological applications, it is assumed that the dominant mechanisms regulating elemental incorporation are related to physical water characteristics with minor or minimal effects of biological traits, such as diet or growth. While there are laboratory experiments and reviews that highlight some of these potentially confounding factors (Sturrock et al., 2014; Izzo et al., 2018), many field studies do not explicitly address them, and the occurrence of strong biotic effects has the potential to confound interpretation of field observations. Direct effects of growth and metabolic variation are of interest because, if dominant, such effects could result in substantial variation among individuals residing in the same locations or water masses, which would violate one of the most common assumptions. Thus, it is important to determine the relative importance of abiotic (or “kinetic”) and biotic (or “vital”) effects on elemental incorporation in general and of growth variation specifically.
There is some evidence for growth rate effects on otolith elemental incorporation (Sadovy and Severin, 1994; Walther et al., 2010; Stanley et al., 2015); however, most studies are confounded by temperature. Growth of ectotherms covaries with temperature and relatively few studies have reported growth effects on otolith elemental incorporation within comparable thermal environments (Martin et al., 2004; Martin and Thorrold, 2005; DiMaria et al., 2010; Walther et al., 2010; Stanley et al., 2015). Overall, variable effects of growth are reported, with positive, negative, and no effects on incorporation reported for the most commonly examined elements: magnesium (Mg), manganese (Mn), strontium (Sr), and barium (Ba) (Tables 1, 2). These findings are further complicated because there are multiple opportunities for partitioning, i.e., at the gills, in the blood and endolymph, and during crystal formation. However, in studies that attempted to account for growth variation by limiting comparisons to individuals in similar temperature treatments, the results are more consistent with primarily positive or no growth effects on the incorporation of Mg and Mn and negative or no effects on the incorporation of Sr and Ba (Table 2). Therefore, growth and temperature could have independent, differential, additive, or multiplicative effects across these interfaces, which could vary across species and in relation to the species' thermal range.
Table 1. Laboratory studies that examined temperature and/or growth effects on otolith elemental incorporation of immature marine or euryhaline fish species.
Table 2. Review of studies that examined temperature and growth effects on otolith elemental incorporation of immature marine or euryhaline fish species.
To separate temperature and growth effects on otolith elemental incorporation, we manipulated both temperature and ration in a controlled laboratory experiment on juvenile Pacific cod (Gadus macrocephalus). By maintaining fish at realistic temperatures that can be experienced during their first year of life (2, 5, 9, 13°C) and manipulating ration within the 9°C treatments, which reflects the species' optimal temperature for growth (Hurst et al., 2010), we independently examined the effects of temperature and growth on elemental incorporation.
Age-0 Pacific cod were collected within a Kodiak Island juvenile nursery using a 36-m beach seine. Fish were maintained for at least 48 h at the Alaska Fisheries Science Center (AFSC) Kodiak Laboratory in ambient seawater prior to shipment to the AFSC Laboratory in Newport, Oregon. Fish were shipped overnight in insulated containers filled with seawater and oxygen. Prior to use in laboratory experiments, fish were maintained in 1-m diameter round tanks with flow-through seawater maintained at 8–10°C. During this acclimation period fish were fed thawed krill and a gelatinized combination of squid, krill, herring, commercial fish food, amino acid supplements, and vitamins three times per week.
After 2 months of laboratory acclimation, 225 fish were randomly assigned to 15 tanks at densities of 15 fish per tank. Experimental tanks were 1 m in diameter and filled to a depth of 55 cm. After 5 d, tank temperatures were adjusted to treatment temperatures at a rate of 1°C per day. Three tanks were assigned to the 2, 5, and 13°C treatments and six tanks were assigned to the 9°C treatment. Lights were maintained on a 12:12 h light:dark photoperiod throughout the experiment. Tanks were checked twice daily for mortalities, which were removed, weighed, and measured. During the tank and temperature acclimation period, fish were fed three times per week.
Following 2 weeks of temperature acclimation, all fish in the experiment were measured and experimental feeding conditions initiated (experiment day 0). Initial mean sizes of the fish were 95.7 mm SL (± 10.1 SD) and 9.2 g wet weight (± 2.7 SD). Three of the 9°C tanks were assigned to a restricted ration treatment with all other tanks being fed thawed krill (Euphausia pacifica) to apparent satiation once per day. The restricted ration treatment was offered food at a rate of 1% wet mass per day, with the ration adjusted following fish measurements. This ration level was slightly above the estimated “maintenance ration” for juvenile Pacific cod and expected to support low levels of somatic growth. All fish in the 9 and 13°C treatments were measured every 2 weeks and fish in the 2 and 5°C treatments were measured every 3 weeks. Due to the expected differences in growth rates, lower temperature and low-ration treatments were extended to allow time for enough otolith deposition for chemical analyses. Therefore, the sampling interval and experimental duration varied among the treatments (Table 3). At the end of the experiment, all fish were euthanized with 250 mg/L of tricaine methanesulfonate (MS-222) buffered to a pH of 7.0 with sodium bicarbonate [American Veterinary Medical Association (AVMA), 2007] and frozen prior to dissection and extraction of otoliths.
Somatic growth rates were determined individually for each fish in the experiment. In lieu of individual marking of the fish, we used the size disparity among fish in each tank as an indicator of identity, assuming maintenance of relative size ranks throughout (Hurst et al., 2012). Weight-specific growth rate (SGR, % body mass d−1) of each fish was calculated by regression of ln-transformed wet mass against sampling date. An indicator of body condition, the hepatosomatic index (HSI), was also calculated (wet liver mass/body mass · 100). Fish size at the end of the experiment, SGR, and the HSI were compared across all five treatments (2, 5, 9_low, 9_high, and 13°C) with one-way Analysis of Variance (ANOVA) and Tukey HSD post-hoc comparisons using R version 3.6.0. Data were log-transformed to satisfy parametric assumptions.
All tanks in the experiment shared the same water source. Coastal oceanic water was pumped from Yaquina Bay during flooding tides into a 3 million L reservoir. Water was pumped from the reservoir through a sand filter to the fish holding facilities where it was heated or chilled to achieve the target temperatures. Given that the water source was the same for all tanks, water samples were collected haphazardly from two of the 15 tanks every 10–14 d for elemental analysis. Water samples were filtered (0.25 μm) and acidified (< 2 pH) with ultrapure HNO3 (ULTREX, J.T. Baker). Dissolved elemental concentrations were measured using a Leeman-Teledyne inductively coupled plasma optical emission spectrometer (ICP-OES) (Mg at 279.1 nm, Ca at 317.9 nm, Mn at 259.4 nm, Sr at 421.5 nm, and Ba at 455.4 nm). Filtered, acidified samples were diluted 150x for the determination of Mg, Ca, and Sr and 20x for Mn and Ba. Matrix-matched standards were created using SPEX Certiprep Group® certified reference materials, National Institute of Standards and Technology (NIST) liquid standard (1643e), and a sodium chloride (NaCl) solution. Matrix-matched NIST standards and HNO3 blanks were used to evaluate accuracy. Measured Mg, Ca, Mn, Sr, and Ba concentrations were within 6, 2, 4, 1, and 6%, respectively, of certified values. Repeated measurements of the same standards indicated that precision was within 5% for all elements (n = 5). Elemental concentrations are presented in ppm (Mg, Ca, Sr) or ppb (Mn, Ba) or in mmol mol−1 (Mg:Ca, Sr:Ca) or μmol mol−1 (Mn:Ca, Ba:Ca). We used paired t-tests to compare the two separate tank water samples collected on the same days. If there were no differences in water elemental concentrations between tank samples, then the water Me:Ca ratios for the two samples were averaged and used for comparison with otolith chemistry. Elemental ratios were ln-transformed to normalize data distributions and homogenize the variance.
Juveniles were weighed (to 0.01 mg) and measured (standard length SL, to 1.0 mm), and both sagittae were removed using standard methods to minimize contamination. The left otolith was mounted on a glass slide using thermoplastic resin and polished to expose the core using 3MTM tri-mite WetordryTM paper (240–1,200 grit) and diamond lapping film. Polished otoliths were imaged at 400× magnification using a Leica DM1000 compound microscope and Micropublisher camera. The mean otolith deposition in each temperature-ration treatment was determined by measuring the total otolith deposition during the experiment based on increment counts. For most fish, particularly in the low-ration treatments, the otoliths displayed a prominent check that aligned with the initiation of the experiment (Figure 1). Daily increments (Narimatsu et al., 2007) within the final 30 μm, which represented the otolith material that was ablated to estimate elemental composition, were measured using ImagePro®. Otoliths were interpreted at least twice with at least 90% agreement for estimates included in subsequent analyses. The duration of the experiment varied across treatments with the 2°C fish maintained for 147 d; the 5°C fish for 84 d; the 9°C low-ration fish for 56 d; and the 9 °C high-ration and 13 °C fish for 40 d. For the 2°C treatment otolith deposition = 198 μm (27 SD); 5°C = 129 μm (16 SD); 9°C low-ration = 81 μm (6 SD); 9°C high-ration = 70 μm (10 SD); and 13°C = 91 μm (12 SD). Individual increment widths during the final 30 μm of otolith deposition experiment were averaged and log-transformed to meet parametric assumptions prior to analysis. Variation across treatments was examined with a one-way ANOVA and Tukey HSD post-hoc tests for pairwise comparisons using R version 3.6.0.
Figure 1. Image of otolith from the low-ration treatment (top image) with apparent stress check (at 80 μm) and ablation scar (30 μm) indicated. Otolith length plotted against fish standard length (left graph) and fish mass (right graph) at the end of the experiment are also included, with the low- and high-ration fish indicated.
The left sagittae were also used for elemental analysis. Otolith thin sections were cleaned ultrasonically in NANOpure® water (18 MΩ·cm), dried in a Class 100 clean bench, and mounted randomly onto clean glass slides. To remove any surface contamination, each otolith was pre-ablated along a transect (500 μm in length) parallel to the outer otolith edge in the anterior-dorsal quadrant; the laser was set at a pulse rate of 2 Hz with a 100-μm spot moving at 100 μm s−1. To collect otolith elemental data, the laser was set at a pulse rate of 7 Hz with a spot of 30 μm moving at 2 μm s−1. Given that a minimum average otolith deposition during the experiment was 70 μm (±10 μm SD), the 30-μm spot size only sampled material deposited during the experiment.
Otolith analyte count data were normalized by 43Ca to adjust for variability in instrument sensitivity and the amount of ablated material and converted to elemental ratios based on measurements of the NIST 612 standard. Elemental ratios are presented in mmol mol−1 (Mg, Sr) or μmol mol−1 (Mn, Ba). Precision (%RSD) was determined based on repeated measurements of NIST 612 glass slides (24Mg:43Ca = 6.0%, 55Mn:43Ca = 3.3%, 86Sr:3Ca = 2.6%, 138Ba:43Ca = 3.7%). Accuracy was measured using repeated analysis of USGS calcium carbonate standards MACS-1 and MACS-3 (mean ± SD for Mg:Ca = 114% ± 6.9, Mn:Ca = 99.4% ± 2.5, Sr:Ca = 102% ± 3.8, Ba:Ca = 113% ± 5.5). To describe elemental composition of otolith carbonate deposited during the experiment, Me:Ca values across 250–350 μm of each transect were averaged for each otolith.
There are various points at which discrimination, or partitioning, of elements can occur within teleosts, including at the water-gill interface, incorporation into blood plasma and endolymph, and during crystallization (Campana, 1999). Therefore, comparison of partition coefficients is common (Morse and Bender, 1990) and allows for a more appropriate comparison of otolith elemental incorporation, particularly given the variable experimental duration across treatments in this study. Partition coefficients (DMe) were calculated using the following equation:
To evaluate temperature effects on DMe, we used a one-way ANOVA with temperature as a fixed factor and tank as the level of replication for all high-ration fish. To evaluate the effect of ration on DMe, we also used a one-way ANOVA with ration as the fixed factor for the 9°C fish only. Data were log-transformed (Sr:Ca, Ba:Ca) or square root transformed (Mg:Ca, Mn:Ca) to meet parametric assumptions. In order to further evaluate the potential effects of growth, we determined Pearson correlation coefficients between SGR and DMe and between otolith increment width and DMe for all fish within the same temperature and ration treatment. Analyses were completed using R version 3.6.0.
Finally, we compiled available literature information on growth and temperature effects on otolith elemental incorporation in order to separate results that are potentially confounded by a temperature x growth interaction from those results that examined, or accounted for in some manner, growth variation. We limited this review to controlled laboratory studies that examined the otolith incorporation of one or more of the most commonly used elements (Mg, Mn, Sr, or Ba). We further limited this analysis to studies on larval or juvenile marine and euryhaline fish in order to avoid potential confounding factors associated with large differences in salinity or maturity.
This experiment was conducted in NOAA's Alaska Fisheries Science Center Laboratory in Newport, Oregon. This research was carried out in accordance with all applicable institutional and national guidelines at the time that the study was conducted; all work followed American Fisheries Society policies on the Guidelines for Use of Fishes in Research (https://fisheries.org/docs/policy_useoffishes.pdf) and AVMA (American Veterinary Medical Association) Guidelines on Euthanasia (https://olaw.nih.gov/sites/default/files/Euthanasia2007.pdf). Fish were collected under permit CF-09-081 from the Alaska Department of Fish and Game. There was no formal ethics review of this study because NOAA National Marine Fisheries Service does not have an Institutional Animal Care and Use Committee (IACUC) or an ethics approval processes for research on fishes and, at the time of this study (2009–2010), OSU's IACUC did not provide review of research that was completed in US federal facilities.
There were very few mortalities during the experiment (one fish in the 5°C and two fish in 9°C low-ration treatment); these were removed from the tank and excluded from all analyses. Due to a handling error, fish from two tanks were irretrievably lost (Tanks five and seven). One additional tank (15) was removed due to consistently high values for otolith Mg:Ca (>6 SD greater than the overall tank mean) and Mn:Ca (4 SD greater than the tank mean). We identified no reason for these values and determined removal of the tank was the best option.
For the remaining 12 tanks, fish metrics (SL, SGR, HSI, and otolith increment width) were compared across all treatments (2, 5, 9 low-ration, 9 high-ration, and 13°C). Tank mean SL ranged from 91.3 to 131.5 mm, and there were differences among treatments (F4, 7 = 12.84, P = 0.002) (Table 4 and Table S2). Mean length of the 9°C, low-ration fish was less than the 2, 5, and 13°C fish (pairwise P < 0.045). Mean SGR ranged from −0.09 g d−1 to 1.52% g d−1 and also varied with treatment (F4, 7 = 263.1, P < 0.0001) (Figure 2). Mean SGR consistently increased with temperature except for the 9°C, low-ration fish, which grew more slowly than the 2°C fish. The low-ration fish also displayed the most variable growth (−0.31 to 0.31). However, only two low-ration fish displayed a negative SGR or even a value <0.1 g d−1, which indicates that the ration, while low, was effectively a maintenance ration. Pairwise contrasts demonstrated that SGR differed among all treatments (pairwise P < 0.002 for all except the 9 and 13°C comparison, P = 0.012). Mean HSI ranged from 1.68 to 4.78 and varied with treatment (F4, 7 = 13.85, P = 0.002). The differences were due to consistently low HSI values in the low-ration, 9°C treatment compared to the four other treatments (P < 0.027) (Figure S1). Mean otolith increment width also increased with temperature, although differences among treatments were relatively small (Figure 2). Overall, there was an effect of treatment on otolith increment width (F4, 7 = 4.86, P = 0.034) with the smallest increments observed in the 9°C low-ration and the largest observed in the 13°C treatment (Figure 2). However, pairwise comparisons indicated that the differences were primarily due to smaller increment widths in the 2°C (P = 0.044), 5°C (P = 0.056), and 9°C low-ration (P = 0.035) compared with the 13°C treatments (Table 4). The otolith length was positively related to fish length and mass, and the low-ration fish had larger otoliths at size than the high-ration fish (Figure 1). Tank mean otolith increment width and SGR were positively correlated (r = 0.69; P = 0.012).
Table 4. Tank number, water temperature (T), ration, and mean (SD) fish size at end of the experiment (standard length, SL) and specific growth rate (SGR) and otolith growth rate during the experiment.
Figure 2. Median, first, and third quartiles and outlying points for specific growth rate (% body mass d−1) and otolith increment width (μm) during the experiment for the 2, 5, 9_low, 9_high, and 13°C treatments.
Elemental concentrations between the two tank water samples collected on the same day were similar (paired t-test, n = 14, P > 0.30). Therefore, the mean of the two samples taken on each of 14 sampling days was used to describe water elemental concentrations during the experiment (Table S1) and values were then averaged for each temperature treatment according to the duration of each treatment (Table 3). There was some seasonal variation observed. Therefore, otolith Me:Ca values are presented (Table 5) but all statistical analyses were based on DMe, which accounts for variation in water chemistry (Figure 3).
Table 5. Tank mean (standard deviation) for otolith elemental concentrations (Me:Ca) and partition coefficients (DMe) during the experiment.
Figure 3. Median, first, and third quartiles and outlying points for otolith Me:Ca (upper four graphs) and DMe (lower four graphs) for the 2, 5, 9_low ration (9L), 9_high ration (9H), and 13°C treatments.
The effect of temperature on partition coefficients, which was evaluated using only high- ration fish, varied across elements with positive effects on DMn and DMg, inconclusive effects on DSr, and no effect on DBa (Figure 3; Table S2). For DMn, there was a positive effect of temperature (F3,5 = 32.73, P = 0.001) where the 2°C treatment was lower than the 5°C (P = 0.003), 9°C (P < 0.001), and 13°C (P = 0.005) treatments. For DMg, there was also a positive effect of temperature (F3,5 = 8.54, P = 0.021) where the 2°C treatment was lower than the 5°C (P = 0.028) and 13°C (P = 0.022) treatments. For DSr, there was a minor association with temperature (F3,5 = 4.09, P = 0.082) due to slightly higher values in the 2°C treatment than in the 5°C treatment (P = 0.080). There was no effect of temperature on DBa (F3,5 = 1.31, P = 0.368). Overall, any observed temperature effects on otolith elemental incorporation were due to the 2°C treatment (Figure S2).
There was a strong, positive effect of ration on the partitioning of Mn (F1,3 = 380.9, P = 0.0003) and negative effect on the partitioning of Sr (F1,3 = 111.9, P = 0.002) (Figure 3; Table S2). There was a small, positive effect of ration on partitioning for Mg (F1,3 = 10.21, P = 0.05) and no effect for Ba (F1,3 = 1.31, P = 0.368). Overall, DMn was 2.6X greater and DMg was 1.1X in high-ration compared with low-ration fish. In contrast, DSr was 1.5X greater in low-ration compared with high-ration fish.
We also determined the Pearson correlation coefficients between SGR and DMe and increment width and DMe within the temperature treatments to further evaluate the potential effects of growth rate (Table 6). There were no wholly consistent patterns observed for any partition coefficients at all temperatures (Figure 4) and no P-values were smaller than 0.05 after conservative adjustments for multiple comparisons. However, the directionality of the correlations between SGR and DMe were similar to that of the ration effect for DMn, positive for four of the five correlations and for DSr, negative for four of the five correlations. For DMg, three of the five correlations were negative, which is opposite the observed, relatively small ration effect. The pattern of correlations between mean otolith increment width and DMe were similar to SGR. The coefficient of variation for otolith increment width was greater than for SGR, except for the low ration treatment in which variation in SGR was very high (Table 6). The patterns between DMe and HSI were similar to SGR and otolith growth (Figure S1).
Table 6. Correlation coefficients between DMe and specific growth rate (SGR, % body mass per day) and partition coefficients (DMe) for fish within the same treatments.
Figure 4. Scatter plot of DMe and specific growth rate (% body mass d−1) for all fish in the 2, 5, 9_low ration (9L), 9_high ration (9H), and 13°C treatments (top four graphs) and for DMe and mean otolith increment width (bottom four graphs).
The observed ration effects were consistently greater than the observed temperature effects. The ration effects are also greater than any observed effects due to growth variation within temperature treatments. For example, the mean difference in SGR between the 2 and 13°C treatments (1.09% g d−1) was similar to the difference between the low- and high-ration 9°C treatments (1.20% g d−1). In contrast, the mean difference in DMn between the 2 and 13°C fish (1.3X) was half of the observed difference between the low- and high-ration fish (2.6X). Additionally, mean DSr was similar in the 2 and 13°C fish (0.244 and 0.229, respectively) whereas DSr values for the low-ration 9°C fish were, on average, 1.5X greater than the high-ration 9°C fish, despite the similar growth rate variation. Similarly, the HSI was, on average, 1.2X greater in the 13°C treatments compared with the 2°C treatments but was 2.8X greater in fish from the high-ration vs. low-ration 9°C treatments. Thus, the difference in condition, as measured by the HSI, reflects the variation in DMe better than somatic or otolith growth.
We identified 21 studies in our literature review of growth and temperature effects on otolith elemental incorporation, which was limited to laboratory studies on larval and juvenile marine and euryhaline fish. Only 10 of these studies explicitly attempted to account for growth vs. temperature effects and only one other study directly manipulated growth through ration (Walther et al., 2010). An important consideration is that most other studies used a correlative approach to examine growth effects within or across temperature treatments (Tables 1,2).
For magnesium, the most commonly reported finding was no effect or a positive effect of temperature when comparisons did not explicitly account for growth (Gauldie, 1996; DiMaria et al., 2010; Miller, 2011; Stanley et al., 2015). When growth was examined at least somewhat independently, no effect of growth was most common (four studies) with one report of positive and one of negative growth effects on Mg incorporation. Marohn et al. (2011) observed a positive effect of growth for the European eel (Anguilla anguilla) but only at 19°C whereas Martin and Thorrold (2005) observed a negative growth effect for juvenile spot (Leiostomus xanthurus).
For manganese, there is evidence for positive temperature effects in two species (Marohn et al., 2011; Stanley et al., 2015), no effects in three species (Elsdon and Gillanders, 2002; Martin and Wuenschel, 2006; Miller, 2009) and negative effects for one species (Miller, 2009) although these observations are all potentially confounded by growth variation. However, when attempts to isolate growth effects were made, there are only reports of positive or no effect of growth on elemental incorporation.
For strontium, there are numerous studies with findings of positive, negative, or no effects of temperature; however, the majority of those are also confounded by potential growth effects (Table 2). For studies that controlled ration or examined body condition or growth within comparable temperatures, only negative (five of eight studies) or no effects (three of eight) of growth are reported. For example, DiMaria et al. (2010) examined temperature and growth effect on DSr of larval Pacific cod and observed a negative effect of temperature across temperature treatments, an observation that was confounded by temperature-induced growth variation. Within temperature treatments, there was evidence for a growth effect on DSr only within the 5°C treatment.
For barium, variable effects of temperature on incorporation, including positive, negative, and no effects, have been reported although most of these are also potentially confounded by growth (Table 2). However, similar to Sr, for studies that manipulated ration or examined growth or condition at comparable temperatures, there were no (six of nine studies) or negative (three of nine studies) effects of growth on otolith incorporation reported.
The use of otolith chemistry to address ecological questions, such as identification of natal origins or estimation of mixing among groups of individuals, and environmental challenges, such as reconstruction of water temperature or salinity, usually requires the implicit assumption that individual variation in growth has minimal effect on the elemental signatures. While there are some indications that this assumption is not wholly supported in immature (Walther et al., 2010; Marohn et al., 2011; Stanley et al., 2015) or mature (Kalish, 1991; Sadovy and Severin, 1994; Sturrock et al., 2014) fishes, independent evaluations are scarce due to the inherent covariation of temperature and growth in fishes. While we observed a relatively large effect of ration on the incorporation of Mn (+) and Sr (–), there was a relatively small effect for Mg (+), and no effect for Ba. Temperature effects were only observed for DMn and DMg and only associated with the 2°C treatments. Furthermore, the data were inconclusive regarding the effect of growth rate within temperature treatments although the observed directionality of the potential growth effects were similar to the ration effect for Mn and for Sr but not for Mg.
The proposed mechanisms by which trace elements become associated with an otolith include: substitution for calcium; trapped in interstitial spaces within the crystal; passive or active association with the organic component; or incorporation as a biochemical co-factor (Campana, 1999; Doubleday et al., 2014; Thomas et al., 2017). Determining definitively which of these processes occurs is challenging. However, several studies have examined elemental concentrations throughout the pathway leading to crystallization, i.e., in the blood, endolymph, and otolith (Kalish, 1991; Payan et al., 1999; Melancon et al., 2009; Sturrock et al., 2014, 2015), while other studies focused on determining which elements were associated with the organic, proteinaceous component of the endolymph and otolith (Miller et al., 2006; Izzo et al., 2016).
Recently, Thomas et al. (2017) used size-exclusion chromatography with inductively coupled plasma mass spectrometry to determine if elements within the endolymph were associated with proteins, present only as free ions, or both. They propose that elements occurring only as free ions (or within the “salt” fraction) are the most likely to be substituted for calcium and therefore reflect environmental variation whereas elements found only associated with proteins (within the “proteinaceous” fraction) would be more likely to be associated with the organic component and to reflect physiological variation. Elements found in both fractions could reflect both environmental and physiological process, thereby complicating interpretation of otolith concentrations. Magnesium and manganese were found only in the salt fraction whereas calcium, strontium, and barium were all found in both the proteinaceous and salt fractions (Thomas et al., 2017). The substitution of other ions for calcium in aragonite appears to be dependent on size with some authors suggesting that those with larger ionic radii than calcium (Sr and Ba) are more likely to substitute (Thomas et al., 2017) whereas others indicate those with smaller ionic radii (Mg) are energetically favored as substitutes (Menadakis et al., 2008). Magnesium has a smaller ionic radius than calcium and could also be trapped within the growing otolith matrix. Thomas et al. (2017) propose that their observation of Mn only in the salt fraction of the endolymph, combined with its potential to form a dimer with an ionic radius similar to calcium, is an indication that Mn otolith incorporation could occur through substitution for calcium. However, several studies have reported Mn associated with otolith proteins (Miller et al., 2006; Izzo et al., 2016; Thomas and Swearer, 2019). Despite the progress made on understanding the mechanisms of otolith elemental incorporation, uncertainty regarding how various factors, such as temperature or growth, influence those mechanisms remains high.
Seasonal variation in plasma or endolymph chemistry has been reported in adult fish (Kalish, 1991; Sturrock et al., 2014, 2015). However, there is less information on seasonality for immature, marine or euryhaline fish. The experimental phase of our study ranged from 40 to 147 d, and the difference in duration between the low- and high-ration 9°C fish, which showed the largest treatment effect, was 16 d. Therefore, it is unlikely the changes due to seasonality could explain our observations although it is not out of the realm of possibility for the temperature effect observed for the 2°C treatment. For mature, female bearded rock cod (Pseudophycis barbatus), Kalish (1991) observed that protein, Sr, and Sr:Ca levels in the endolymph, the calcifying fluid in which the otolith grows, all reached their lowest levels in March, which was also when the otolith Sr:Ca reached its nadir. Kalish (1991) concluded that these seasonal patterns were most likely related to changes in protein concentrations and composition within the plasma and endolymph that were associated with reproduction. He noted that changes in endolymph composition associated with gonad development cannot explain otolith Sr:Ca changes in immature fish but postulated that stress could change the protein complement and ultimately affect the relative proportions of calcium and strontium in the endolymph.
Given that the largest incorporation effects in this study were associated with ration rather than growth or temperature, could the pattern be explained by a stress response? We did not assess stress directly, and therefore cannot objectively determine if the low-ration fish were stressed. As noted, stress could alter the protein quantity and composition and ultimately affect the relative proportions of calcium and strontium in the endolymph. Kalish (1989) and Townsend et al. (1992) reported higher otolith Sr:Ca in presumably stressed immature fish with relatively low condition indices compared with unstressed fish that had higher condition indices. Stress in fishes can lead to increased production of hormones, such as corstisol and other glucocorticoids, which can increase permeability of gill and intestinal membranes potentially altering ion transport (Wendelaar Bonga, 1997). If such alterations occurred, they could affect endolymph chemistry and otolith composition. However, studies that examined endolymph chemistry of fish that were stressed through starvation (Payan et al., 1998) or through Cl2-stress (Payan et al., 2004b) report no change in Ca, sodium (Na), or potassium (K) concentrations within the endolymph. Furthermore, a notable change in endolymph Ca concentrations, which are regulated through active transport and ion exchange, could be expected to similarly affect other elements that can substitute for Ca. However, we observed a negative effect of ration only for DSr. Alternatively, if the low-ration fish experienced this type of stress response that led to increases in Sr concentrations while there was a greater level of ion regulation for Ca, it is plausible that the observed increase in DSr was due to increases in strontium concentrations within the endolymph rather than declines in calcium (Payan et al., 2002, 2004b). Additionally, as noted, the concentration of proteins within the endolymph can vary (Mugiya, 1987; Payan et al., 1999; Edeyer et al., 2000), which could also influence the incorporation of protein-associated elements. If there was a decline in protein concentration within the endolymph associated with the low-ration treatment, that could potentially result in a decline in the incorporation of manganese, which is reported to be associated with matrix proteins (Miller et al., 2006; Izzo et al., 2016; Thomas and Swearer, 2019), in the low-ration fish as we observed.
Overall, we identified modest effects of temperature only for DMn and DMg. These relatively small incorporation effects associated with temperature were due entirely to differences in the 2°C treatment and thus appear unlikely to be solely the result of growth variation. For DMn, we observed a moderate, positive effect of temperature (1.3X) and the relationships between DMn and somatic and otolith growth were inconclusive. As noted earlier, for the few other studies that attempted to separate growth and temperature effects, there are only reports of positive or no relationships between DMn and growth (Martin and Thorrold, 2005; Miller, 2009; Marohn et al., 2011; Stanley et al., 2015). For DMg, we observed a small, positive effect of temperature, a relatively small effect of ration, and no consistent effect of growth on DMg. In fact, two low-ration fish with relatively high DMg exhibited the fastest and one of the slowest growth rates in the entire study (DMg = 0.77 and 0.74 and SGR = 0.01 and 0.32% g d−1, respectively). For DSr and DBa there was no effect of temperature even though growth rates varied across treatments. Overall, these differences in elemental incorporation associated with ration or temperature do not appear to simply reflect growth variation.
The observed growth variation across treatments was greatest in terms of change in mass, followed by length, with the smallest differences observed for otolith increment width. While this could initially appear incongruous, there are several plausible explanations for the smaller growth effects within the otoliths. Studies report continued otolith deposition during periods of starvation (Campana, 1983; Mosegaard and Titus, 1987) whereas others report continued otolith deposition with a decrease in the frequency of increment formation (Jones and Brothers, 1987; Zhang and Runham, 1992). However, Jones and Brothers (1987) determined that, for striped bass (Morone saxatilis), this apparent reduction was likely due to a limited ability to detect increments using light microscopy as daily increments were successfully identified using scanning electron microscopy. Additionally, studies demonstrate a positive relationship between metabolic rate and otolith deposition independent of somatic growth rate (Mosegaard and Titus, 1987; Wright, 1991). In this experiment, the low-ration fish were maintained at 9°C. Therefore, it is plausible that these low-ration fish maintained a relatively high metabolic rate in comparison with the 2 and 5°C fish, which could influence their otolith deposition. Additionally, the process of otolith growth and the deposition of incremental and discontinuous zones is thought to be under circadian control, thus maintained under periods of reduced food (Mugiya, 1984, 1987; Payan et al., 2004a; Thomas and Swearer, 2019). Another observation that indicates otolith deposition occurred in all of our treatments is that the otoliths for these low ration fish were larger than the otoliths of fish of comparable body size in the other treatments, which indicates continued otolith deposition with no or minimal growth.
Our DMn values were relatively high (2.1–6.2), and exceeded estimates reported from other laboratory studies. Martin and Wuenschel (2006) reported DMn values just over 1.0 for juvenile gray snapper Lutjanus griseus and Miller (2009) documented DMn values from 0.01 to 0.41 for juvenile black rockfish (Sebastes melanops) across a wide range of water concentrations (4.6–118.9 μM M−1). However, Dorval et al. (2007) collected water and juvenile spotted sea trout (Cynoscion nebulosus) in Chesapeake Bay and also reported relatively high estimates of DMn, ranging from 7.7 to 32.8. Their otolith Mn:Ca values (21–40 μM M−1) were similar to or greater than our observations for Pacific cod (12–26.7 μM M−1) with lower or similar water Mn:Ca levels. This relatively large range of DMn values in wholly marine species indicates that multiple mechanisms could be influencing otolith incorporation of manganese.
An additional consideration with otolith incorporation of Mn is recent work that indicates Mn uptake is related to dissolved oxygen levels, with hypoxia-induced increases in Mn+2 due to favorable redox conditions (Limburg et al., 2011, 2015; Limburg and Casini, 2018). Although our dissolved oxygen levels were not constantly monitored in our experiment, values were typically >7 mg L−1 and the maximum fish density was <0.75 g L−1 with uneaten food biomass <2% of the total fish biomass in each tank. Additionally, there was no evidence in the water samples for elevated Mn in the high-ration treatment. There is also evidence for positive relationships between otolith Mn:Ca and growth: Turner and Limburg (2015) observed positive relationships between otolith growth and otolith Mn:Ca in blueblack (Alosa aestivalis) and river herring (A. pseudoharengus). Across an ~2.5 to 4x increase in otolith growth, they observed increases in otolith Mn:Ca comparable to or greater than our observed ration effect. Therefore, although multiple lines of evidence support a positive effect of growth on otolith incorporation of Mn, it is not yet entirely clear how growth and temperature, and potentially other factors, interact to regulate this element in otoliths.
Effects of ration, condition, or growth on otolith elemental incorporation have been reported previously. Izzo et al. (2015) isotopically spiked water and evaluated the effects of variable salinity and temperature on otolith elemental incorporation in barramundi (Lates calcarifer) and also determined if there were shifts in the percent contribution of food vs. water across treatments. Using a mixed-model approach, they found that temperature and indices of body condition (Fulton's K for Sr:Ca) or growth (RNA:DNA for Ba:Ca) combined were the best predictors of otolith chemistry. In their experiment, they observed higher DMe at warmer temperatures (30°C) but fish at the lower temperature (26°C) were in better condition, which indicates negative effects of condition and positive effects of temperature on otolith incorporation of Sr and Ba. They also observed a reduction in the percent contribution of water to otolith Sr:Ca and Ba:Ca (and an increase in the food contribution) at the lower temperature, which was the treatment with the higher condition fish. The observation of a negative effect of ration and condition on DSr is similar to our results as well as those of Walther et al. (2010), who reported a negative effect of ration on DSr and DBa in juvenile spotted chromis (Acanthochromis polycanthus) and observed negative relationships between fish growth and DSr and DBa. Therefore, for the few studies that manipulated or evaluated the effects of ration, there are consistently negative effects of increased ration, higher condition, or elevated growth on DSr and some consistency (two out of three studies) for DBa. Combined with our observations of positive effects of ration on DMn, these findings indicate that there may be stress-, condition-, or nutrition-mediated effects on otolith elemental incorporation, at least for some elements.
An important question is whether or not it is reasonable to expect a general pattern for growth effects on elemental incorporation to emerge if enough species are studied. Given the structural differences in calcium carbonate morphology (trigonal, orthorhombic, and hexagonal) across crystal types (calcite, aragonite, vaterite) and the observations of variable growth effects across crystal types (De Choudens-Sánchez and González, 2009), comparisons with abiotic aragonite, which is the common morphology of sagittae, are the most relevant. Experimental manipulations of abiotic aragonite have demonstrated that incorporation of alkaline earth cations (Mg+2, Ca+2, Sr+2, and Ba+2) have an inverse relationship with temperature (Gaetani and Cohen, 2006). Furthermore, aragonite precipitation rate also influenced elemental incorporation rates with positive effects for Mg:Ca and negative effects for Sr:Ca and Ba:Ca, and models that reconstruct temperature based on Me:Ca in coral aragonite were improved by inclusion of these precipitation rate effects (Gaetani et al., 2011). Therefore, it does not seem unreasonable to expect some consistent patterns to emerge in regard to growth rate effects on otolith elemental incorporation.
Given the collective evidence currently available, there is support for ration, growth, or condition effects on otolith elemental incorporation, at least for Mn, Sr, and Ba. Therefore, there are some clear recommendations. Otoliths are growth structures that, in most cases, require extensive preparation prior to elemental analysis. Despite the effort involved in preparing otoliths for chemical analysis, which could also be used to generate estimates of otolith and somatic growth, many studies do not report any estimates of somatic or otolith growth and compare fish across relatively large ranges of size, ages and growth rates [but see (Darnaude et al., 2014; Bouchoucha et al., 2018)]. Our understanding of the factors influencing otolith elemental incorporation would be enhanced by the inclusion of somatic and/or otolith growth rates and consideration of body condition, whenever feasible, in both laboratory and field studies. This type of information could advance the understanding of factors influencing otolith chemistry. Additionally, other approaches to manipulate growth rates and condition, such as by manipulating activity levels, could help further disentangle the related aspects of temperature, growth, condition, and feeding rates and advance the understanding and utility of a powerful methodology in fisheries and ecological science.
The datasets generated for this study are available on request to the corresponding author.
Ethical review and approval was not required for the animal study because This experiment was conducted in NOAA's Alaska Fisheries Science Center Laboratory in Newport, Oregon. This research was carried out in accordance with all applicable institutional and national guidelines at the time that the study was conducted; all work followed American Fisheries Society policies on the Guidelines for Use of Fishes in Research (https://fisheries.org/docs/policy_useoffishes.pdf) and AVMA (American Veterinary Medical Association) Guidelines on Euthanasia (https://olaw.nih.gov/sites/default/files/Euthanasia2007.pdf). Fish were collected under permit CF-09-081 from the Alaska Department of Fish and Game. There was no formal ethics review of this study because NOAA National Marine Fisheries Service does not have an Institutional Animal Care and Use Committee (IACUC) or an ethics approval processes for research on fishes and, at the time of this study (2009–2010), OSU's IACUC did not provide review of research that was completed in US federal facilities.
JM and TH conceived of and executed the research, analyzed the data, and wrote the manuscript. TH led the laboratory experiment. JM completed the otolith and water analyses.
Experiment was supported North Pacific Research Board grant no. R0816.
Reference to trade names does not imply endorsement by the National Marine Fisheries Service. The findings in this paper are those of the authors an do not necessarily reflect the views of the National Marine Fisheries Service.
The authors declare that the research was conducted in the absence of any commercial or financial relationships that could be construed as a potential conflict of interest.
The laboratory experiment was performed with support from M. Ottmar, M. Spencer, S. Haines, and R. DiMaria. Thomas Murphy provided assistance with otolith preparation. We appreciate the comments of three reviewers on an earlier version of this manuscript.
The Supplementary Material for this article can be found online at: https://www.frontiersin.org/articles/10.3389/fmars.2020.00320/full#supplementary-material
Figure S1. Scatter plot of DMe and Hepatic Somatic Index (HSI) for all fish in the 2, 5, 9_low ration (9L), 9_high ration (9H), and 13°C treatments.
Figure S2. Median, first, and third quartiles and outlying points for otolith Me:Ca (upper four graphs) and DMe (lower four graphs) for each tank. Axis labels indicate the temperature followed by the tank number (e.g., 2.1 = 2°C treatment, tank 1).
Table S1. Water chemistry during experiment. Values represent the mean based on samples collected from two tanks on each day. For Ba:Ca and Mn:Ca, all standard deviations were less than 0.005. In order to calculate partition coefficients (DMe), water values were averaged based on duration of experiment within each treatment. Values were averaged from 10 Dec 09 to 09 Jan 10 for the 9 and 13°C full ration treatments, from 10 Dec 09 to 01 Feb 10 for the 9_low °C restricted ration treatment, from 10 Dec 09 to 24 Feb 10 for the 5°C treatment, and from 10 Dec 09 to 25 Apr 10 for the 2°C treatment. “All” = 2, 5, 9_low, 9_high, and 13°C treatments.
Table S2. Results of the one-way Analyses of Variance completed to evaluate the effect of treatment (A) on fish length, specific growth rate, otolith increment width, and hepatosomatic index and the effect of temperature (B) or ration (C) on partition coefficients (DMe).
American Veterinary Medical Association (AVMA) (2007). Guidelines on Euthanasia. Available online at: https://olaw.nih.gov/sites/default/files/Euthanasia2007.pdf (accessed December 15, 2019).
Barnes, T. C., and Gillanders, B. M. (2013). Combined effects of extrinsic and intrinsic factors on otolith chemistry: implications for environmental reconstructions. Can. J. Fish. Aquat. Sci. 70, 1159–1166. doi: 10.1139/cjfas-2012-0442
Bath, G. E., Thorrold, S. R., Jones, C. M., Campana, S. E., McLaren, J. W., and Lam, J. W. H. (2000). Strontium and barium uptake in aragonitic otoliths of marine fish. Geochim. Cosmochim. Acta 64, 1705–1714. doi: 10.1016/S0016-7037(99)00419-6
Bouchoucha, M., Brach-Papa, C., Gonzalez, J.-L., Lenfant, P., and Darnaude, A. M. (2018). Growth, condition and metal concentration in juveniles of two Diplodus species in ports. Mar. Poll. Bull. 126, 31–42. doi: 10.1016/j.marpolbul.2017.10.086
Buckel, J. A., Sharack, B. L., and Zdanowicz, V. S. (2004). Effect of diet on otolith composition in Pomatomus saltatrix, an estuarine piscivore. J. Fish Biol. 64, 1469–1484. doi: 10.1111/j.0022-1112.2004.00393.x
Campana, S. E. (1983). Feeding periodicity and the production of daily growth increments in the otoliths of steelhead trout (Salmo gairdneri) and starry flounder (Platichthys stellatus). Can. J. Zool. 61, 1591–1597. doi: 10.1139/z83-214
Campana, S. E. (1999). Chemistry and composition of fish otoliths: pathways, mechanisms, and applications. Mar. Ecol. Prog. Ser. 188, 263–297. doi: 10.3354/meps188263
Chesney, E. J., McKee, B. M., Blanchard, T., and Chan, L. C. (1998). Chemistry of otoliths from juvenile menhaden Brevoortia patronus: evaluating strontium, strontium:calcium and strontium isotope ratios as environmental indicators. Mar. Ecol. Prog. Ser. 171, 261–273. doi: 10.3354/meps171261
Darnaude, A. M., Sturrock, A., Trueman, C. N., Mouillot, D. E. I. M. F., Campana, S. E., et al. (2014). Listening in on the past: what can otolith delta O-18 values really tell us about the environmental history of fishes? PLOS ONE 9:e114951. doi: 10.1371/journal.pone.0108539
De Choudens-Sánchez, V., and González, L. A. (2009). Calcite and aragonite precipitation under Controlled instantaneous supersaturation: elucidating the role of CaCO3 saturation state and Mg/Ca ratio on calcium carbonate polymorphism. J. Sediment. Res. 79, 363–376. doi: 10.2110/jsr.2009.043
DiMaria, R. A., Miller, J. A., and Hurst, T. P. (2010). Temperature and growth effects on otolith elemental chemistry of larval Pacific cod, Gadus macrocephalus. Environ. Biol. Fish. 89, 453–462. doi: 10.1007/s10641-010-9665-2
Dorval, E., Jones, C. M., Hannigan, R., and van Montfrans, J. (2007). Relating otolith chemistry to surface water chemistry in a coastal plain estuary. Can. J. Fish. Aquat. Sci. 64, 411–424. doi: 10.1139/f07-015
Doubleday, Z. A., Harris, H. H., Izzo, C., and Gillanders, B. M. (2014). Strontium randomly substituting for calcium in fish otolith aragonite. Anal. Chem. 86, 865–869. doi: 10.1021/ac4034278
Edeyer, A., de Pontual, H., Payan, P., Troadec, H., Severe, A., and Mayer-Gostan, N. (2000). Daily variations of the saccular endolymph and plasma compositions in the turbot Psetta maxima: relationship with the diurnal rhythm in otolith formation. Mar. Ecol. Prog. Ser. 192, 287–294. doi: 10.3354/meps192287
Elsdon, T. S., and Gillanders, B. M. (2002). Interactive effects of temperature and salinity on otolith chemistry: challenges for determining environmental histories of fish. Can. J. Fish. Aquat. Sci. 59, 1796–1808. doi: 10.1139/f02-154
Elsdon, T. S., and Gillanders, B. M. (2003). Relationship between water and otolith elemental concentrations in juvenile black bream Acanthopagrus butcheri. Mar. Ecol. Prog. Ser. 260, 263–272. doi: 10.3354/meps260263
Elsdon, T. S., and Gillanders, B. M. (2004). Fish otolith chemistry influenced by exposure to multiple environmental variables. J. Exp. Mar. Biol. Ecol. 313, 269–284. doi: 10.1016/j.jembe.2004.08.010
Gaetani, G. A., and Cohen, A. L. (2006). Element partitioning during precipitation of aragonite from seawater: a framework for understanding paleoproxies. Geochim. Cosmochim. Acta 70, 4617–4634. doi: 10.1016/j.gca.2006.07.008
Gaetani, G. A., Cohen, A. L., Wang, Z., and Crusius, J. (2011). Rayleigh-based, multi-element coral thermometry: A biomineralization approach to developing climate proxies. Geochim. Cosmochim. Acta 75, 1920–1932. doi: 10.1016/j.gca.2011.01.010
Gallahar, N. K., and Kingsford, M. J. (1996). Factors influencing Sr/Ca ratios in otoliths of Girella elevata: An experimental investigation. J. Fish. Biol. 48, 174–186. doi: 10.1111/j.1095-8649.1996.tb01111.x
Gauldie, R. W. (1996). Effects of temperature and vaterite replacement on the chemistry of metal ions in the otoliths of Oncorhynchus tshawytscha. Can. J. Fish. Aquat. Sci. 53, 2015–2026. doi: 10.1139/cjfas-53-9-2015
Gibson-Reinemer, D. K., Johnson, B. M., Martinez, P. J., Winkelman, D. L., Koenig, A. E., and Woodhead, J. D. (2009). Elemental signatures in otoliths of hatchery rainbow trout (Oncorhynchus mykiss): distinctiveness and utility for detecting origins and movement. Can. J. Fish. Aquat. Sci. 66, 513–524. doi: 10.1139/F09-015
Hoff, G. R., and Fuiman, L. A. (1995). Environmentally induced variation in elemental composition of red drum (Sciaeops ocellatus) otoliths. Bull. Mar. Sci. 56, 578–591.
Holcomb, M., Cohen, A. L., Gabitov, R. I., and Hutter, J. L. (2009). Compositional and morphological features of aragonite precipitated experimentally from seawater and biogenically by corals. Geochim. Cosmochim. Acta 73, 4166–4179. doi: 10.1016/j.gca.2009.04.015
Hurst, T. P., Laurel, B. J., and Ciannelli, L. (2010). Ontogenetic patterns and temperature-dependent growth rates in early life stages of Pacific cod (Gadus macrocephalus). Fish Bull NOAA 108, 382–392.
Hurst, T. P., Munch, S.B., and Lavelle, K. (2012). Thermal reaction norms for growth vary among cohorts of Pacific cod (Gadus macrocephalus). Mar. Biol. 159, 2173–2183. doi: 10.1007/s00227-012-2003-9
Izzo, C., Doubleday, Z. A., and Gillanders, B. M. (2016). Where do elements bind within the otoliths of fish? Mar. Freshw. Res. 67, 1072–1076. doi: 10.1071/MF15064
Izzo, C., Doubleday, Z. A., Woodcock, S. H., and Gillanders, B. M. (2015). Contribution of water chemistry and fish condition to otolith chemistry: comparisons across salinity environments. J. Fish Biol. 86, 1680–1698. doi: 10.1111/jfb.12672
Izzo, C., Reis-Santos, P., and Gillanders, B. M. (2018). Otolith chemistry does not just reflect environmental conditions: a meta-analytic evaluation. Fish Fisher. 19, 441–454. doi: 10.1111/faf.12264
Jones, C., and Brothers, E. B. (1987). Validation of the otolith increment aging technique for striped bass, Morone saxatilis, larvae reared under suboptimal feeding conditions. Fish. Bull. 85, 171–178.
Kalish, J. M. (1989). Otolith microchemistry: validation of the effects of physiology, age, and environment on otolith composition. J. Exp.Mar. Biol.Ecol. 132, 151–178.
Kalish, J. M. (1991). Determinants of otolith chemistry: seasonal variation in the composition of blood plasma, endolymph and otolith of the beared rock cod Pseudophycis barbatus. Mar. Ecol. Prog. Ser. 74, 137–159. doi: 10.3354/meps074137
Limburg, K. E., and Casini, M. (2018). Effect of marine hypoxia on Baltic Sea cod Gadus morhua: Evidence from otolith chemical proxies. Front. Mar. Sci. 5:482. doi: 10.3389/fmars.2018.00482
Limburg, K. E., Olson, C., Walther, Y., Dale, D., Slomp, C. P., and Høie, H. (2011). Tracking Baltic hypoxia and cod migration over millennia with natural tags. Proc. Natl. Acad. Sci. U.S.A. 108, E177–E182. doi: 10.1073/pnas.1100684108
Limburg, K. E., Walther, B. D., Lu, Z., Jackman, G., Mohan, J., Walther, Y., et al. (2015). In search of the dead zone: Use of otoliths for tracking fish exposure to hypoxia. J. Mar. Sys. 141, 167–178. doi: 10.1016/j.jmarsys.2014.02.014
Lopez, O., Zuddas, P., and Faivre, D. (2009). The influence of temperature and seawater composition on calcite crystal growth mechanisms and kinetics: Implications for Mg incorporation in calcite lattice. Geochim. Cosmochim. Acta 73, 337–347. doi: 10.1016/j.gca.2008.10.022
Marchitto, T. M., Bryan, S. P., Doss, W., McCulloch, M. T., and Montagna, P. (2018). A simple biomineralization model to explain Li, Mg, and Sr incorporation into aragonitic foraminifera and corals. Earth Planet. Sc. Lett. 481, 20–29. doi: 10.1016/j.epsl.2017.10.022
Marohn, L., Hilge, V., Zumholz, K., Klügel, A., Anders, H., and Hanel, R. (2011). Temperature dependency of element incorporation into European eel (Anguilla anguilla) otoliths. Anal. Bioanal. Chem. 399, 2175–2184. doi: 10.1007/s00216-010-4412-2
Martin, G. B., and Thorrold, S. R. (2005). Temperature and salinity effects on magnesium, manganese, and barium incorporation in otoliths of larval and early juvenile spot Leiostomus xanthurus. Mar. Ecol. Prog. Ser. 293, 223–232. doi: 10.3354/meps293223
Martin, G. B., Thorrold, S. R., and Jones, C. M. (2004). Temperature and salinity effects on strontium incorporation in otoliths of larval spot (Leiostomus xanthurus). Can. J. Fish. Aquat. Sci. 61, 34–42. doi: 10.1139/f03-143
Martin, G. B., and Wuenschel, M. J. (2006). Effect of temperature and salinity on otolith element incorporation in juvenile gray snapper Lutjanus griseus. Mar. Ecol. Prog. Ser. 324, 229–239. doi: 10.3354/meps324229
Melancon, S., Fryer, B. J., and Markham, J. L. (2009). Chemical analysis of endolymph and the growing otolith: Fractionation of metals in freshwater fish species. Environ. Toxic. Chem. 28, 1279–1287. doi: 10.1897/08-358.1
Menadakis, M., Maroulis, G., and Koutsoukos, P. G. (2008). Incorporation of Mg2+, Sr2+, Ba2+ and Zn2+ into aragonite and comparison with calcite. J.Math. Chem. 46:484. doi: 10.1007/s10910-008-9490-4
Miller, J. A. (2009). The effects of temperature and water concentration on the otolith incorporation of barium and manganese in black rockfish Sebastes melanops. J. Fish. Biol. 75, 39–60. doi: 10.1111/j.1095-8649.2009.02262.x
Miller, J. A. (2011). Effects of water temperature and barium concentration on otolith composition along a salinity gradient: implications for migratory history. J. Exp. Mar. Biol. Ecol. 405, 42–52. doi: 10.1016/j.jembe.2011.05.017
Miller, M. B., Clough, A. M., Batson, J. N., and Vachet, R. W. (2006). Transition metal binding to cod otolith proteins. J. Exp. Mar. Biol. Ecol. 329, 135–143. doi: 10.1016/j.jembe.2005.08.016
Mitsuguchi, T., Matsumoto, E., and Uchida, T. (2003). Mg/Ca and Sr/Ca ratios of Porites coral skeleton: Evaluation of the effect of skeletal growth rate. Coral Reefs 22, 381–388. doi: 10.1007/s00338-003-0326-1
Morse, J. W., and Bender, M. L. (1990). Partition coefficients in calcite: examination of factors influencing the validity of experimental results and their application to natural systems. Chem. Geol. 82, 265–277. doi: 10.1016/0009-2541(90)90085-L
Mosegaard, H., and Titus, R. (1987). “Daily growth rates of otoliths in yolk sac fry of two salmonid species at five different temperatures,” in Proceedings of the Fifth Conference of European Ichthyologists, eds S. O. Kallender and B. Fernholm. Stockholm: Swedish Museum of Natural History, 221–227.
Mugiya, Y. (1984). Diurnal rhythm in otolith formation in the rainbow trout Salmo gairdneri: seasonal reversal of the rhythm in relation to plasma calcium concentration. Comp. Biochem. Physiol. 78A, 289–293. doi: 10.1016/0300-9629(84)90149-X
Mugiya, Y. (1987). Phase difference between calcification and organic matrix formation in the diurnal growth of otoliths in the Rainbow trout, Salmo gairdneri. Fish. Bull. 85, 395–401.
Narimatsu, Y., Hattori, T., Ueda, Y., Matsuzaka, H., and Shiogaki, M. (2007). Somatic growth and otolith microstructure of larval and juvenile Pacific cod Gadus macrocephalus. Fish. Sci. 73:1257–1264. doi: 10.1111/j.1444-2906.2007.01463.x
Payan, P., Borelli, G., Boeuf, G., and Mayer-Gostan, N. (1998). Relationship between otolith and somatic growth: consequence of starvation on acid-base balance in plasma and endolymph in the rainbow trout Oncorhynchus mykiss. Fish Physiol. Biochem. 19, 35–41. doi: 10.1023/A:1016064813517
Payan, P., Borelli, G., Priouzeau, F., De Pontual, H., Bœuf, G., and Mayer-Gostan, N. (2002). Otolith growth in trout Oncorhynchus mykiss: supply of Ca2+ and Sr2+ to the saccular endolymph. J. Exp. Biol. 205, 2687–2695.
Payan, P., De Pontual, H., Bœuf, G., and Mayer-Gostan, N. (2004a). Endolymph chemistry and otolith growth in fish. Gen. Palaeont. 3, 535–547. doi: 10.1016/j.crpv.2004.07.013
Payan, P., Edeyer, A., De Pontual, H., Borelli, G., Boeuf, G., and Mayer-Gostan, N. (1999). Chemical composition of saccular endolymph and otolith in fish inner ear: lack of spatial uniformity. Am. J. Physiol. 277, 123–131. doi: 10.1152/ajpregu.1999.277.1.R123
Payan, P., Pontual, H. D., Edeyer, A., Borelli, G., Boeuf, G., and Mayer-Gostan, N. (2004b). Effects of stress on plasma homeostasis, endolymph chemistry, and check formation during otolith growth in rainbow trout (Oncorhynchus mykiss). Can. J. Fish. Aquat. Sci. 61, 1247–1255. doi: 10.1139/f04-059
Radtke, R. L. (1989). Strontium-calcium concentration ratios in fish otoliths as environmental indicators. Comp. Biochem. Physiol. A 92, 189–193. doi: 10.1016/0300-9629(89)90151-5
Reis-Santos, P., Tanner, S. E., Elsdon, T. S., Cabral, H. N., and Gillanders, B. M. (2013). Effects of temperature, salinity and water composition on otolith elemental incorporation of Dicentrarchus labrax. J. Exp. Mar. Biol. Ecol. 446, 245–252. doi: 10.1016/j.jembe.2013.05.027
Sadovy, Y., and Severin, K. P. (1994). Elemental patterns in Red Hind (Epinephelus guttatus) otoliths from Bermuda and Puerto Rico reflect growth rate, not temperature. Can. J. Fish. Aquat. Sci. 51, 133–141. doi: 10.1139/f94-015
Schloesser, R. W., Rooker, J. R., Louchuoarn, P., Neilson, J. D., and Secor, D. H. (2009). Interdecadal variation in seawater delta C-13 and delta O-18 recorded in fish otoliths. Limnol. Oceanogr. 54, 1665–1668. doi: 10.4319/lo.2009.54.5.1665
Stanley, R. R. E., Bradbury, I. R., DiBacco, C., Snelgrove, P. V. R., Thorrold, S. R., and Killen, S. S. (2015). Environmentally mediated trends in otolith composition of juvenile Atlantic cod (Gadus morhua). ICES J. Mar. Sci. 72, 2350–2363. doi: 10.1093/icesjms/fsv070
Sturrock, A. M., Hunter, E., Milton, J. A., Johnson, R. C., Waring, C. P., Trueman, C. N., et al. (2015). Quantifying physiological influences on otolith microchemistry. Methods Ecol. Evol. 6, 806–816. doi: 10.1111/2041-210X.12381
Sturrock, A. M., Trueman, C. N., Milton, J. A., Waring, C. P., Cooper, M. J., and Hunter, E. (2014). Physiological influences can outweigh environmental signals in otolith microchemistry research. Mar. Ecol. Prog. Ser. 500, 245–264. doi: 10.3354/meps10699
Thomas, O. R. B., Ganio, K., Roberts, B. R., and Swearer, S. E. (2017). Trace element protein interactions in endolymph from the inner ear of fish: implications for environmental reconstructions using fish otolith chemistry. Metallomics 9, 239–249. doi: 10.1039/C6MT00189K
Thomas, O. R. B., and Swearer, S. E. (2019). Otolith biochemistry—A review. Rev. Fish. Sci. Aquacul. 27, 458–489. doi: 10.1080/23308249.2019.1627285
Townsend, D. W., Radtke, R. L., Corwin, S., and Libby, D. A. (1992). Strontium:calcium ratios in juvenile Atlantic herring Clupea harengus L. otoliths as a function of water temperature. J. Exp. Mar. Biol.Ecol. 160, 131–140. doi: 10.1016/0022-0981(92)90115-Q
Turner, S. M., and Limburg, K. E. (2015). Does daily growth affect the rate of manganese uptake in juvenile River Herring otoliths? Trans. Amer. Fish. Soc. 144, 873–881. doi: 10.1080/00028487.2015.1059888
Walsh, C. T., and Gillanders, B. M. (2018). Extrinsic factors affecting otolith chemistry – implications for interpreting migration patterns in a diadromous fish. Environ. Biol. Fish. 101, 905–916. doi: 10.1007/s10641-018-0746-y
Walther, B., Kingsford, M. J., O'Callaghan, M. D., and McCulloch, M. T. (2010). Interactive effects of ontogeny, food ration and temperature on elemental incorporation in otoliths of a coral reef fish. Environ. Biol. Fish. 89, 441–451. doi: 10.1007/s10641-010-9661-6
Webb, S. D., Woodcock, S. H., and Gillanders, B. M. (2012). Sources of otolith barium and strontium in estuarine fish and the influence of salinity and temperature. Mar. Ecol. Prog. Ser. 453, 189–199. doi: 10.3354/meps09653
Wendelaar Bonga, S. E. (1997). The stress response in fish. Physiol. Rev. 77, 591–625. doi: 10.1152/physrev.1997.77.3.591
Wright, P. J. (1991). The influence of metabolic rate on otolith increment width in Atlantic salmon parr, Salmo salar L. J. Fish Biol. 38, 929–933. doi: 10.1111/j.1095-8649.1991.tb03632.x
Wurster, C. M., and Patterson, W. P. (2001). Late Holocene climate change for the eastern interior United States: Evidence from high-resolution δ18O values of sagittal otoliths. Palaeogeogr. Palaeoclimatol. 170, 81–100. doi: 10.1016/S0031-0182(01)00229-2
Yamada, S. B., and Mulligan, T. J. (1982). Strontium marking of hatchery reared coho salmon Oncorhynchus kisutch identification of adults. J. Fish Biol. 20, 5–10. doi: 10.1111/j.1095-8649.1982.tb03889.x
Keywords: partition co-efficients, manganese, strontium, barium, magnesium, Pacific cod
Citation: Miller JA and Hurst TP (2020) Growth Rate, Ration, and Temperature Effects on Otolith Elemental Incorporation. Front. Mar. Sci. 7:320. doi: 10.3389/fmars.2020.00320
Received: 16 December 2019; Accepted: 20 April 2020;
Published: 26 May 2020.
Edited by:
Benjamin D. Walther, Texas A&M University Corpus Christi, United StatesReviewed by:
Susanne Eva Tanner, University of Lisbon, PortugalCopyright © 2020 Miller and Hurst. This is an open-access article distributed under the terms of the Creative Commons Attribution License (CC BY). The use, distribution or reproduction in other forums is permitted, provided the original author(s) and the copyright owner(s) are credited and that the original publication in this journal is cited, in accordance with accepted academic practice. No use, distribution or reproduction is permitted which does not comply with these terms.
*Correspondence: Jessica A. Miller, amVzc2ljYS5taWxsZXJAb3JlZ29uc3RhdGUuZWR1
Disclaimer: All claims expressed in this article are solely those of the authors and do not necessarily represent those of their affiliated organizations, or those of the publisher, the editors and the reviewers. Any product that may be evaluated in this article or claim that may be made by its manufacturer is not guaranteed or endorsed by the publisher.
Research integrity at Frontiers
Learn more about the work of our research integrity team to safeguard the quality of each article we publish.