- 1Earth and Life Institute (ELI), UCLouvain, Louvain-la-Neuve, Belgium
- 2Cooperative Institute for Marine and Atmospheric Studies (CIMAS), University of Miami, Miami, FL, United States
- 3Atlantic Oceanographic and Meteorological Laboratory (AOML), NOAA, Miami, FL, United States
- 4Halmos College of Natural Sciences and Oceanography, Nova Southeastern University, Dania Beach, FL, United States
- 5Institute of Mechanics, Materials and Civil Engineering (IMMC), UCLouvain, Louvain-la-Neuve, Belgium
Connectivity between coral reefs is critical to ensure their resilience and persistence against disturbances. It is driven by ocean currents, which often have very complex patterns within reef systems. Only biophysical models that simulate both the fine-scale details of ocean currents and the life-history traits of larvae transported by these currents can help to estimate connectivity in large reef systems. Here we use the unstructured-mesh coastal ocean model SLIM that locally achieves a spatial resolution of ~100 m, 10 times finer than existing models, over the entire Florida Reef Tract (FRT). It allows us to simulate larval dispersal between the ~1,000 reefs composing the FRT. By using different connectivity measures and clustering methods, we have identified two major connectivity pathways, one originating on the westernmost end of the outer shelf and the other originating on the inner shelf, North of the Lower Keys. We introduce new connectivity indicators, based on the PageRank algorithm, to show that protection efforts should be focused on the most upstream reefs of each pathway, while reefs best suited for restoration are more evenly spread between the Lower and Upper Keys. We identify one particular reef, North of Vaca Key, that is a major stepping stone in the connectivity network. Our results are the first reef-scale connectivity estimates for the entire FRT. Such fine-scale information can provide knowledge-based decision support to allocate conservation and restoration resources optimally.
1. Introduction
Coral reefs are one of the most important and biologically diverse ecosystems on Earth. Scleractinian corals, also known as stony corals, build the reefs by excreting calcium carbonate skeletons. Their skeletons provide habitat, shelter, nursery areas, and food for over 9 million species of animals and plants (Knowlton, 2001). Reefs provide essential ecosystem services, such as protection of coastlines from strong wave action, and are a very important source of food and income (fisheries, tourism) for coastal communities (Moberg and Folke, 1999).
Over the past few decades, coral reefs have experienced a system-wide decline (Hoegh-Guldberg et al., 2017) with the percent cover of live coral decreasing globally (Gardner et al., 2003; Pandolfi et al., 2003; Bruno and Selig, 2007). It is estimated that 30% of the reefs have already been severely degraded and 60% will be lost by 2030 (Hughes et al., 2003). This decline is due to global anthropogenic stressors, such as ocean warming which causes recurrent and more intense bleaching events and disease outbreaks (Connell et al., 1997; Bruno et al., 2003; De'ath et al., 2012; Bruno and Valdivia, 2016; Hughes et al., 2018), and local anthropogenic stressors, such as pollution (Brown, 1987), physical destruction of reefs for coastal development (Erftemeijer et al., 2012), increased sedimentation (Jordan et al., 2010), nutrient run-off (Hughes et al., 2003; Sheppard et al., 2009), and overharvesting (particularly of herbivorous species which control algae growth, Jackson et al., 2001; Bellwood et al., 2004). As a result, many coral-dominated reefs have undergone regime shifts to become macroalgae-dominated (Mumby et al., 2006; Cheal et al., 2010; Graham et al., 2015). Regime shifts result in profound changes to the structure of reefs, impacting all reef associated species and thus compromising the socio-economic revenues of fishing and tourism industries for local communities.
The recovery of corals requires the inversion of mortality of adult colonies, maintenance (or increase) of existent genotypic diversity and promotion of effective sexual recruitment, i.e., establishment of larval-derived recruits and their survival to sexual maturity (Ritson-Williams et al., 2009). These are highly dependent on curtailing global carbon emissions, but local actions can facilitate recovery. The local actions necessary to allow coral populations to naturally recover can be divided in two approaches: passive (reduction of local stressors) and active (human intervention to increase reproductive potential). The passive approach includes prohibiting or limiting the collection of wild specimens, establishing marine protected areas, and regulating boat anchoring, scuba, and the discharge of pollutants, nutrients, and sediment. These actions tend to be effective in the long term (Selig and Bruno, 2010; Harrison et al., 2012), but are highly dependent on levels of protection and respective compliance (Arias et al., 2015). The active approach, i.e., restoration, consists of increasing local coral cover by growing corals on land or in offshore nurseries and then outplanting the coral fragments on the reef. This approach is more expensive, requires more man-power, and thus is only feasible in a relatively smaller spatial scale; however, it can achieve positive results faster (Miller et al., 2016). The recovery of most reefs will require a thoughtful combination of both approaches.
The impact of both protection and restoration approaches is further increased by trying to maximize their effect on the entire system. Areas that should be prioritized for protection include reefs that act as important larval sources, i.e., which disperse larvae and repopulate a significantly large number of reefs and thus contribute the most to the resilience of the system, as well as reefs that are more susceptible to disturbance, i.e., which are isolated. Similarly, for reef restoration, corals should be strategically outplanted in areas where the currents facilitate the dispersal of embryos/larvae produced in the outplant site to a greater number of surrounding reefs, and therefore contribute to the replenishment of the ecosystem beyond that site. To date, outplant sites are solely selected based on their local conditions, such as suitable light and depth, presence of wild colonies, absence of predators, and/or low abundance of macroalgae. A site's potential to serve as a larval source and therefore contribute to increase the resilience of the entire system is still overlooked during the site selection process because the larval dispersal and connectivity patterns in most reefs is unknown or poorly understood at a spatial scale relevant to management interventions.
Estimating larval dispersal and demographic connectivity cannot be done empirically. Moreover, measuring genetic similarity is not sufficient as this only infers the flow of genes between populations, which might be very different from the flow of individuals required to maintain a population (Cowen and Sponaugle, 2009). Experimentally-calibrated numerical models that simulate both the ocean currents and larval biology can provide a realistic picture of larval dispersal and connectivity, but accurately modeling water circulation at the spatial scales that affect larval dispersal remains a key challenge. For instance, small-scale flow features such as recirculation eddies around reefs and islands strongly influence larval dispersal as they increase local retention and hence reduce connectivity (Wolanski, 1994; Burgess et al., 2007; Figueiredo et al., 2013). This requires an ocean model that can explicitly simulate flow features down to the scale of individual reefs and reef passages, which is of the order of 100–1,000 m in dense reef systems. Today, very few regional ocean models achieve such a spatial resolution, because of the computational resources required and the difficulty to approximate the complex topography of coral reef systems with traditional numerical methods. Unstructured-mesh ocean models offer a potential solution to the latter problems by locally increasing the model resolution close to reefs and islands (Lambrechts et al., 2008; Thomas et al., 2014, 2015). This approach focuses the computational resources where they are most needed. High resolution bio-physical dispersal models can guide passive and active conservation plans by helping to identify priority protection areas, i.e., areas either more vulnerable to disturbance because of their isolation, or with a greater capacity to recolonize other reefs through sexual recruitment to boost the overall connectivity and maximize resilience.
The Florida Reef Tract (FRT) spans over 580 km from the Dry Tortugas National Park west of the Florida Keys to the St. Lucie Inlet in Martin County, constituting the third largest barrier reef in the world (Finkl and Andrews, 2008). These reefs have a fauna and species richness typical of Caribbean reefs, including more than 40 species of stony corals (Banks et al., 2008). The bouldering Montastraea cavernosa is the dominant reef builder (Banks et al., 2008; Jackson et al., 2014). The northern half-section of the FRT is a relict, early Holocene reef framework and indurated sand ridges which are no longer accreting, while the southern half-section is composed of chain of limestone islands (Keys), fossilized (lithified) remnants of ancient coral reefs and sand bars (Hoffmeister and Multer, 1968; Shinn, 1988; Lidz and Shinn, 1991). This is the only area in the continental U.S. where active carbonate deposition is occurring on a large scale (Enos, 1977; Shinn et al., 1989).
The FRT is located along the northern side of the Straits of Florida that connect the Gulf of Mexico and the North Atlantic Ocean. The large-scale ocean circulation of that region is dominated by the Florida Current (FC), which is an intense western boundary current that continues the Loop Current inside the Gulf of Mexico and, downstream, forms the Gulf Stream. The FC is characterized by spatial variability and meandering, which are associated with the presence of cyclonic eddies between the core of the current and the FRT (Lee et al., 1995; Fratantoni et al., 1998; Kourafalou and Kang, 2012). The typical periods for these meandering and cyclonic eddies are about 30–70 days in the Lower Keys near the entrance of the Straits of Florida (Lee et al., 1995), and shorter (2–21 days) in the Upper Keys (Lee and Mayer, 1977). These eddies provide connectivity pathways along the FRT (Limouzy-Paris et al., 1997; Sponaugle et al., 2005; D'Alessandro et al., 2007), while they also promote productivity and larval survival (Shulzitski et al., 2015, 2016). On the northernmost section of the FRT, these meanders and eddies cause cold-water upwelling that limits the northern range of tropical species (Walker and Gilliam, 2013). On the upper part of the shelf along the FRT, the circulation, and thus the larval transport, is largely influenced by winds (Lee et al., 2001; D'Alessandro et al., 2007), with typical periods of a 2–14 days associated with weather variations (Lee and Smith, 2002). The dynamics on the upper part of the shelf is also influenced by diurnal and semi-diurnal tidal processes (Lee et al., 2001; Lee and Smith, 2002; D'Alessandro et al., 2007).
Over the past four decades, the FRT has suffered a severe decline, with significant shifts in the coral community and decreases in abundance due to increased frequency of bleaching events caused by warming (Kuffner et al., 2015), higher levels of pollution (including sewage, sedimentation, eutrophication, and heavy metals), and increased prevalence of disease outbreaks (Williams and Miller, 2012). For example, the once dominant Acropora species have suffered a >97% decline in the abundance throughout their ranges in the 1970s and 1980s (Southeast Regional Office, 2015). The coral cover in the FRT has dropped from 40 to 60% in 1975 to <7% in the Florida Keys (Jackson et al., 2014) and <3% in the northern section (Walton et al., 2018) homogenizing the FRT coral communities (Burman et al., 2012). The recovery of these reefs requires curtailing local and global stressors. To conserve these coral reefs and speed up recovery, it is urgent to protect and/or restore the areas which (could) contribute the most with larvae to the rest of reef. To do so, the larval dispersal and connectivity patterns along the FRT need to be known. The existing hydrodynamic models for this region do not accurately represent the small scale circulation in shallower areas, and have a sub-reef-scale resolution. The best resolution currently available, to our knowledge, is ~900 m with the FKEYS-HYCOM model that has been developed for the Florida Keys region (Kourafalou and Kang, 2012; Sponaugle et al., 2012; Vaz et al., 2016).
The objective of this study is to estimate larval dispersal and connectivity of M. cavernosa in the FRT by developing an experimentally-calibrated bio-physical dispersal model. With a resolution of about 100m, this model can represent cross-shore transport more realistically than has been done before, in particular the high-frequency wind-driven and tide-related dynamical processes on the upper shelf along the FRT, and hence provide better estimates of larval exchanges between the inner and outer shelves. We can thus identify connectivity pathways that would be ignored by coarser models. By taking the 2010 spawning event as a test case, we show how areas of greater interest to protect or to restore, and areas most vulnerable to disturbance, can be identified. Ultimately, our model could provide policy makers and managers with quantitative information on the most effective management actions that could be undertaken to increase coral recruitment success.
2. Methods
2.1. Larval Dispersal and Connectivity Modeling
Marine connectivity can be estimated in many different ways. These include empirical approaches based on genetic analysis or micro-chemical fingerprinting, and physical approaches based on numerical models. Only the latter can be used to estimate demographic connectivity throughout the entire FRT system.
The first step to model larval dispersal and connectivity is to be able to simulate ocean currents. An ocean model should provide a realistic large-scale circulation while also resolving small-scale flow features down to the scale of individual reefs. In this study, we use the unstructured-mesh depth-integrated coastal ocean model SLIM1 to simulate ocean currents over an area that includes the FRT but also the Florida Strait and part of the Gulf of Mexico (Figure 1). By using an unstructured mesh, we can increase the model resolution only over the FRT and hence concentrate computational resources where they are most needed. SLIM, being a depth-averaged model, is well-suited to shallow-water flows. On the shelf break and in deeper areas, it might however not be able to model more complex processes such as mesoscale eddies that are observed along the southern flank of the FRT and the thermally-driven FC. This issue has been partly circumvented by relaxing SLIM's velocity toward the depth-averaged velocity obtained with the HYbrid Coordinate Ocean Model (HYCOM, Chassignet et al., 2007) implemented over the Gulf of Mexico, when the water depth exceeds a certain threshold. Details of the model formulation and validation are provided in the Appendix.
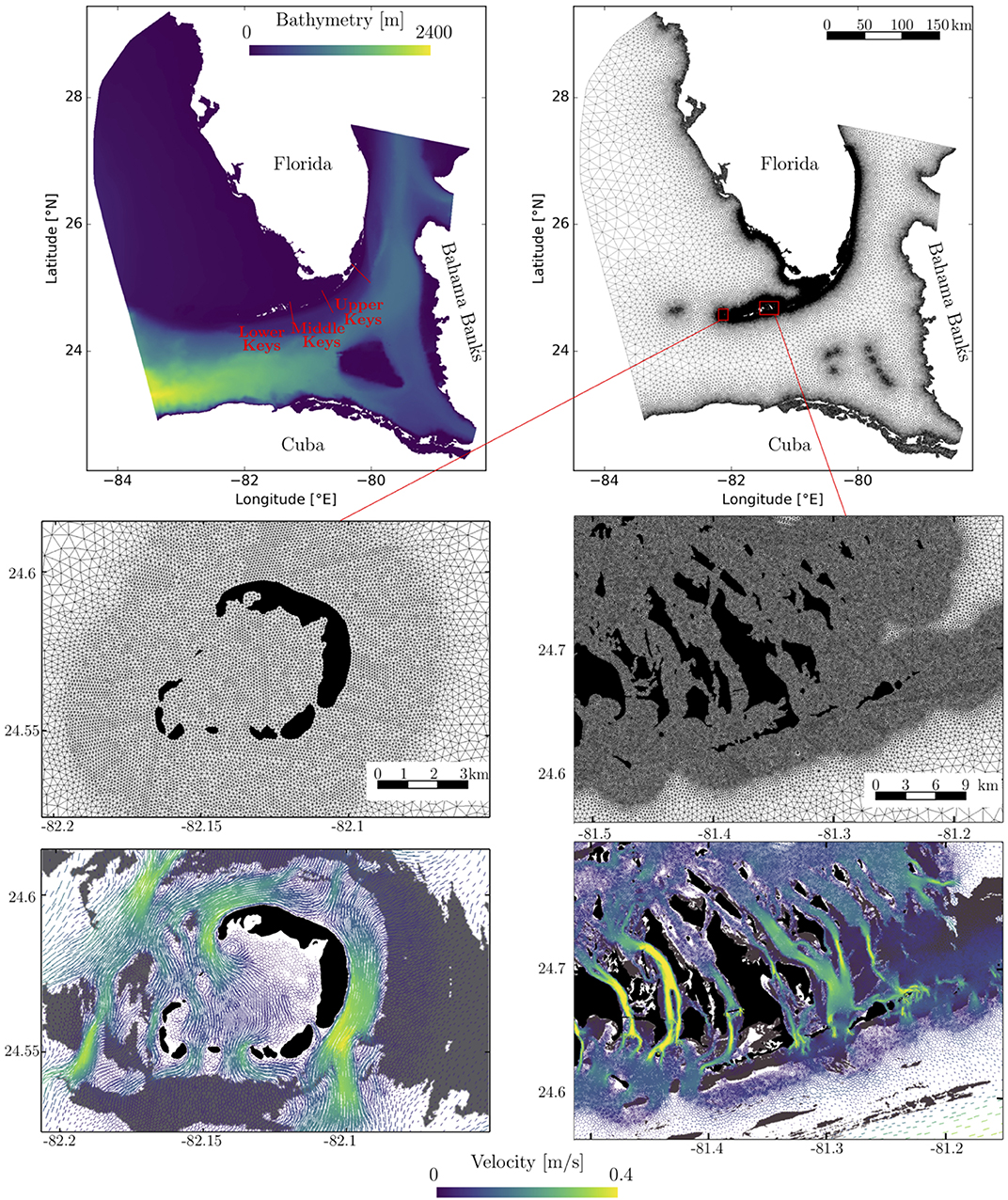
Figure 1. (Top) Model computational domain with the bathymetry and location of the Lower, Middle, and Upper Keys (left), and the unstructured mesh (right). The mesh contains about 106 elements and the resolution varies between about 100 m and 15 km. Close-up views of the mesh (Middle) and snapshots of the currents on September, 27 2010 at 22:00 (Bottom), for the Marquesas Keys (left) and the Lower Keys (right). This illustrates the benefits of unstructured meshes to represent the fine-scale details of the topography and hence simulate currents down to the scale of individual reefs (shown in gray) and islands (shown in black).
The mesh resolution depends only on the distance to the coast but we distinguish between the coastlines along the FRT where we impose a maximum resolution of 100 m and the other coastlines along which the maximum resolution is 900 m. The mesh has been generated with the open-source mesh generator GMSH (Geuzaine and Remacle, 2009) and is shown in Figure 1. It has about 106 elements. The coarsest elements, far away from the FRT, have a size of about 15 km. An illustration of ocean currents simulated on that mesh are shown in Figure 1. It shows how a 100 m spatial resolution allows us to simulate fine-scale details of the flow, such as recirculation eddies and currents within the dense reef system in the Lower Keys that consist of many individual reefs with narrow passages in between.
The simulated currents can then be used to model coral larval dispersal throughout the FRT. In this study, we only consider one coral species, M. cavernosa, which is widely distributed through most reef environments in the FRT. During 2005–2010, it was among the 5 most abundant species in terms of number of colonies (Lirman et al., 2011). However, colonies being big, the life tissue area of M. cavernosa is significantly larger than other coral species (Walton et al., 2018). In the northern third of the FRT, it was one of the most abundant reef building corals that has recently declined because of the on-going Stony Coral Tissue Loss disease (Walton et al., 2018) and is now the focus of future restoration efforts (Page et al., 2018). In the Southern portion of the FRT, it is responsible for most of the abundance, although it also recently declined because of whole colony mortality associated with the on-going coral disease outbreak. Walton et al. (2018) report a 45% colony loss between 2015 and 2016. Spawning for this species occurs just after the full moon of August.
We study larval dispersal only for the year 2010. During that year, HYCOM has been heavily used and validated following the Deepwater Horizon oil spill (Mezić et al., 2010; Le Hénaff et al., 2012; Valentine et al., 2012). The SLIM simulation begins on August 24, 2010 at 00:00, the day of the full moon. All larvae are released at this moment, which is in agreement with field observations (Jordan, 2018). While spawning occurs over a couple of hours during the night, the model results are not very sensitive to the precise timing of spawning and its duration, as larvae spend a few hours over the reefs before being flushed away. This is due to rather weak currents on the reef flat. We then simulate larval dispersal until September 29, 2010 at 00:00. To simulate the physical processes driving larval dispersal, we use a depth-averaged Lagrangian particle tracker (Dimou and Adams, 1993; Spagnol et al., 2002). The deterministic advection term is modeled with a 4th-order Runge-Kutta and the stochastic diffusion term is modeled as a Wiener process whose diffusivity is calculated using Okubo (1971)'s formula. A number of connectivity and dispersal studies have already been performed by coupling SLIM with this Lagrangian model (Thomas et al., 2014, 2015; Critchell and Lambrechts, 2016; Grech et al., 2016, 2018).
Biological processes representing the larvae life history traits are then included in the particle tracker. These include mortality, competence and settlement. Mortality is represented as a fixed probability of dying each day, which has been extracted from the survival proportion curve of Kuba (2016). It yields a mortality rate of 0.067 day−1, which means that larvae have a life expectancy of about 15 days. Competence represents the ability of a larva to settle onto a reef. After 3.8 days, M. cavernosa larvae start acquiring competence at a rate of 6.4% day−1 (Kuba, 2016). Finally, we assume that once a larva is competent, it settles on the first reef it travels over. This is of course a simplification of reality but the lack of empirical data does not enable us to formulate a more elaborate description.
After setting up the model, the next step is to seed virtual larvae on all the reefs composing the FRT. To do so, a map of the location of the different reefs of Florida has been used. We use the layer giving the areas where coral reefs and hardbottom are found from the Unified Florida Reef Tract Map (FWRI, 2016). This generates 990 reefs in total. Some assumptions have been made for the release of larvae. First of all, it is assumed that the areas corresponding to coral reefs and hardbottom are entirely covered with corals. However, sometimes corals cover only a small fraction of the area and the quantity of larvae released is therefore overestimated. Likewise, some of the areas identified as coral reefs can be covered by seagrass or algae hence limiting the amount of larvae that can settle. Moreover, we have assumed that M. cavernosa always covers the entire area of each reef. This is surely far from reality. However, to remove these assumptions, we would need for each structure, the proportion of the area covered by corals and, more specifically, the proportion covered by M. cavernosa. Because of the lack of information, we have chosen to keep these assumptions. Our model therefore provides potential connections between reefs as would happen if each reef had the same coral density and enough room to accommodate all settlers. As a second step, realized (or actual) connectivity could be estimated by using coral cover and/or habitat quality to modulate potential connectivity according to the state of the source and destinations reefs, however ongoing disease and other impacts will likely confound this approach. For the spawning of larvae, we have released on every reef a concentration of particles of 1,600 larvae/km2 and a minimum number of particles on a single habitat reef of 400. This is well above the particle density threshold suggested by Monroy et al. (2017) to achieve connectivity results insensitive to the number of particles released. With these parameters, about 7.1 × 106 particles are released over the entire domain at initial time.
2.2. Potential Connectivity Measures
The output of the Lagrangian particle tracker is a potential connectivity matrix whose entries are denoted Cij. The matrix rows correspond to the source reefs and the columns correspond to the destination reefs. Hence Cij represents the number of virtual larvae originating from reef i that have settled on reef j. The ~ 103 reefs in the FRT yield a connectivity matrix with ~ 106 entries. While the majority of these entries are zero, it remains challenging to extract useful information from such a large matrix. The connectivity matrix can be more easily handled by interpreting it as a large graph. Graph nodes will be all the reefs of the FRT. Node i will be connected to node j if the corresponding entry in the connectivity matrix (Cij) is non-zero. The strength of a connection corresponds to the number of larvae traveling from one node to the other. It is important to note that connections are directional, which means that a connection from node i to node j does not imply that there is a connection from node j to node i. This simply reflects the fact that the connectivity matrix is not symmetric.
Many different measures can be derived from the connectivity graph by means of graph-theory algorithms (see for instance Minor and Urban, 2007; Rayfield et al., 2011; Kool et al., 2013; Dubois et al., 2016). Table 1 summarizes the connectivity measures that we will use in this study to highlight the most important nodes and the connectivity pathways. These measures are useful to identify which reefs are the most self-persistent, the most isolated, the best importers, the best exporters, or the main stepping stones in the graph. However, from a management and conservation perspective, it is also interesting to have indicators directly showing which reefs are the most in need of protection and which reefs would be best suited for restoration projects. This of course requires defining the need of protection of a reef and its suitability for restoration projects. Daigle et al. (2020) and Kininmonth et al. (2019) have shown that the best strategy for achieving metapopulation persistence was to protect sites based on their outgoing PageRank index. Here we go one step further and argue that among stronger exporters, we should focus protection efforts on those that are the most fragile (i.e., the weak importers). These would be the reefs that significantly increase the resilience of the system but are not very resilient themselves. In terms of restoration practices, we suggest that the best suited reefs are the ones that both receive and supply many larvae. This way, outplanted reefs would be good sources and, at the same time, be sufficiently resilient to persist. This is in agreement with the conclusions of Hastings and Botsford (2006) who noted that only patches that truly both receive and contribute larvae to the entire system play a role in the sustainability of the system.
By using the incoming and outgoing PageRank measures (denoted πin and πout, resp.), we can define protection and restoration indices as follows:
• The PageRank protection index is the difference between πout and πin normalized by their sum:
The protection index ranges between −1 and +1. Values close to +1 correspond to good sources that are not well supplied while values close to −1 correspond to reefs that receive many larvae but do not provide many. Therefore, reefs having values close to +1 should be protected in priority.
• The PageRank restoration index πout × in is the multiplication of πout and πin:
Reefs with the highest restoration index values are both good sources and good sinks. They are thus the most suitable sites for outplanted corals.
Beyond “single reef” measures, we also identify reef clusters. Clustering can help us simplify the graph by highlighting groups of reefs strongly connected to each other and weakly connected to reefs outside their group. From an ecological perspective, strongly connected reefs are likely to be home of similar species. In this study, we consider two different clustering methods : the strongly connected components (SCC) method and the modularity optimization (MO) method.
The SCC method does not focus on the strength of the connections but simply on the presence of bi-directional connections (Tarjan, 1972). Two nodes belong to the same SCC (in other words to the same cluster) if there is a path connecting them in each direction. It can potentially be satisfied through multistep connections. SCCs will therefore group reefs that interact bi-directionally and thus have a higher genetic mixing among them. Hastings and Botsford (2006) have shown that population persistence depended on the existence of multi-generational connectivity pathways that eventually form a closed loop. The SCC method hence identifies clusters of reefs that belong to such a persistence loop. Since the life-history traits of M. cavernosa are similar to a number of other coral species (such as Orbicella faveolata and Orbicella franksi that are also important reef builders, and also potentially Diploria strigosa and Dendrogyra cylindrus), we can expect similar species living on these reefs.
The MO method does not require bidirectional paths between nodes belonging to the same community. Here we consider a particular MO algorithm that compares the strength of connections within the communities to a user-defined connection strength parameter ζ. The communities are then derived such that (1) the average connection strength between the reefs within the community is greater than ζ and (2) the average connection strength between any two reefs in different communities is less than ζ. This approach allows us to detect reef communities at different spatial scales. If we use a low value of ζ, the connectivity between any two communities will be small (i.e., we have nearly impermeable community boundaries), but the internal connectivity may also be low and the size of the communities will tend to be large. If instead we use a higher value of ζ, the connectivity between any two communities may be higher, but the internal connectivity will also be higher and communities will tend to be smaller and boundaries more permeable. The ζ parameter is therefore an indicator of how much inter- and intra-community connectivity there is.
The advantage of this method is that it requires only directional connections and there is no need of a closed loop. This is particularly useful for studying marine connectivity since the ocean currents often induce a strong directionality in the connectivity patterns. Furthermore, it explicitly takes the strength of the connections into account. However, the value of the parameter ζ is arbitrary. Different values of ζ will yield different community structures. As ζ increases, the algorithm produces more communities containing fewer reefs. However, for some ranges of values of ζ, the community structure can be stable. Large plateaux therefore correspond to pertinent partitions. The optimization procedure that yields the community structure is carried out using a modified Louvain community detection algorithm (Blondel et al., 2008) following the approach described in Traag et al. (2011). More details on the application of this method to marine connectivity can be found in Thomas et al. (2014).
3. Results
The graph corresponding to the connectivity matrix derived for M. cavernosa following the 2010 spawning event shows that larval transport is directed north-eastward (Figure 2). While the most intense transport seems to occur along the outer shelf, larvae can also be transported from the inner shelf to the outer shelf, either directly through the dense reef system in the Lower Keys or through the Middle Keys where some reefs appear to be major intergenerational stepping-stones. The graph also shows that large-distance connectivity, from the Marquesas and Lower Keys up to the northernmost reefs in the FRT, is possible.
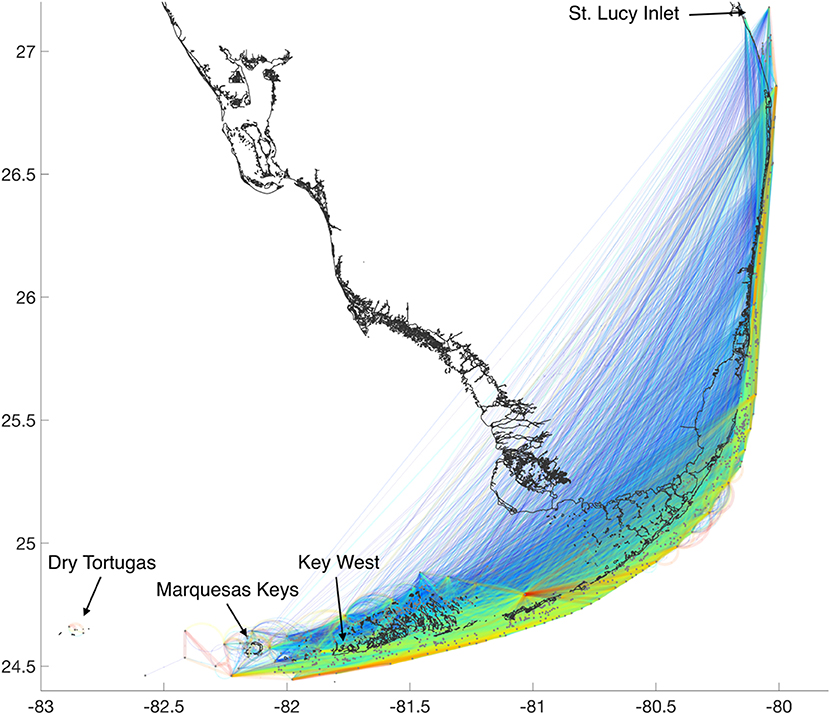
Figure 2. The connectivity graph highlights the exchange of coral larvae between all the reefs of the FRT. The color code, from blue to red, indicates the strength of connections (i.e., the number of larvae exchanged). The graph suggests that connectivity can occur over the entire length of the FRT as reefs around Key West and the Marquesas Keys are connected to reefs offshore of St. Lucy Inlet. The Dry Tortugas however appear to be isolated from the rest of the FRT.
Self recruitment can reach 100% for the most upstream reefs, which are located either at the westernmost end of the FRT or North of the Lower Keys (Figure 3A). In 2010, this is for instance the case for the reefs in the Dry Tortugas, which receive very little larvae from other reefs and also hardly keep the larvae they produce. Some reefs inside the Lower Keys reef system and in the inner-shelf part of the Upper Keys are also quite isolated by topographical barriers that restrict larval transport. These reefs are most at risk as they could hardly recover following a disturbance that would strongly reduce their coral cover. Local retention can exceed 25% inside the Lower Keys reef system (Figure 3B). In such areas where reefs are closely aggregated, the self-seeding potential is high because the reef system directs the mean circulation around it and hence traps larvae inside. This phenomenon is sometimes called the “sticky water effect” (Wolanski, 1994). Both local retention and self recruitment are very small along the outer shelf region since the FC tends to transport larvae faraway.
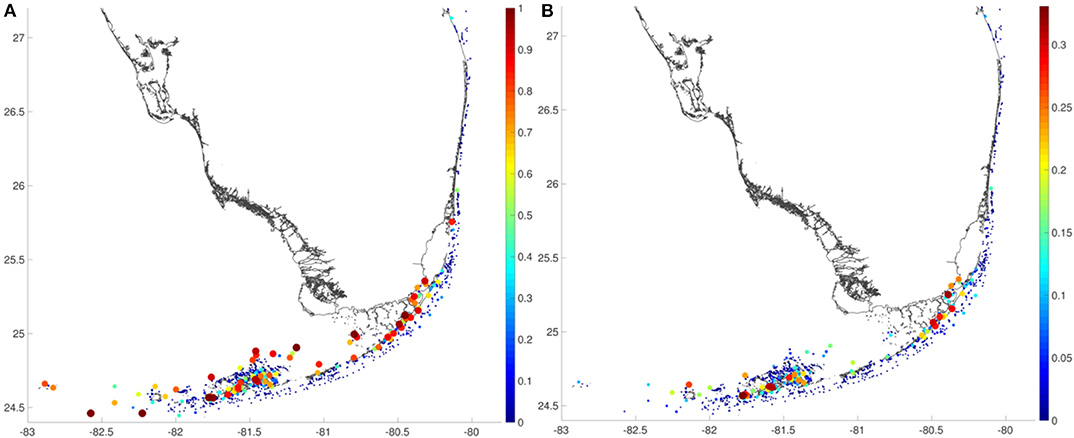
Figure 3. (A) Self recruitment and (B) local retention on the whole FRT. Low self recruitment (and hence high isolation) are observed for the most upstream reefs and inside areas where topographical barriers can prevent larval transport. High local retention is observed inside the Lower Keys where small-scale flow features keep larvae near their source reef.
Areas with a high reef density have a high proportion of larvae that settle but these larvae tend to settle close to the source reefs (Figure 4). This is particularly the case for reefs located on the inner shelf for which larvae will settle <20 km from the source reef. On the outer shelf, the reef system is less dense and currents are stronger. Larvae can then be transported further away, and can travel over distances of the order of 100 km before they settle. The proportion of larvae that can eventually settle on a reef is then also smaller. The model highlights clear differences between inner- and outer-shelf reefs. The outer shelf area in the Upper Keys is characterized by a much smaller connectivity length. This appears to be due to stronger tidal currents in that area that keep larvae closer to the source reefs. The Dry Tortugas again appear to be isolated from the rest of the FRT as only a small amount of larvae that were released there eventually settles. This is due to a wind-driven northward current in the model simulation that transports larvae away from the source reefs.
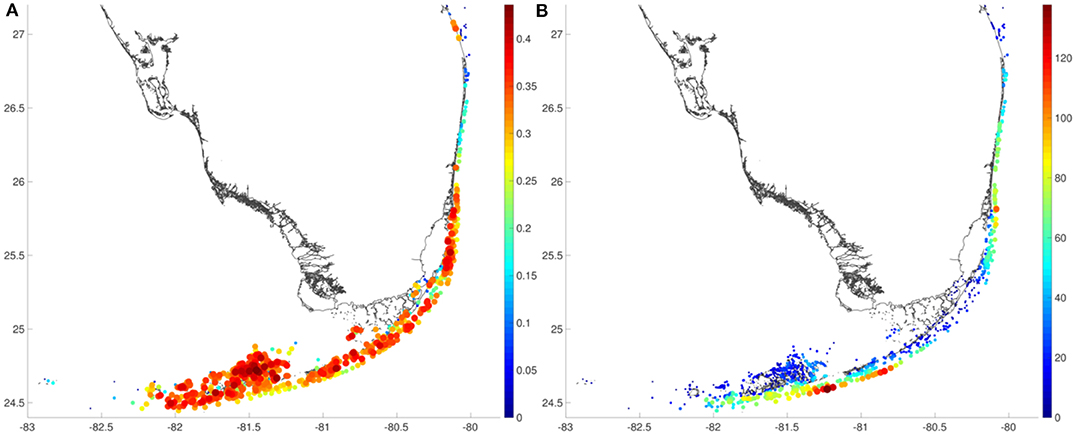
Figure 4. (A) The proportion of larvae that settle and (B) the weighted connectivity length (WCL, in km) show that larvae originating from the outer shelf are transported further away but are also less likely to settle than larvae from the inner shelf. Inside dense reef matrices, larvae are more likely to settle and tend to reach reefs close to their source reef.
Reefs with the largest incoming PageRank are all located on the outer shelf, in the northern part of the reef tract (Figure 5). These are the reefs with the best larval supply as they receive many larvae from reefs that are also well supplied. Most larvae eventually end up in the outer shelf area, where the FC acts as a conveyor belt that transports larvae northeastward. On the other hand, the reefs with the largest outgoing PageRank are located upstream of the two major connectivity pathways: one starts on the westernmost end of the outer shelf; the other starts on the inner shelf, North of the Lower Keys. These two groups of reefs have the most important impact on the rest of the FRT. They provide a large number of larvae to many reefs, which in turn also provide larvae to many other reefs. They are thus the most useful reefs. Figures illustrating the subgraphs associated with a sink and a source hotspot are provided online as Supplementary Material. The incoming/outgoing connections reveal the upstream/downstream footprints of a reef and hence highlight the group of reefs on which it depends and which it supports.
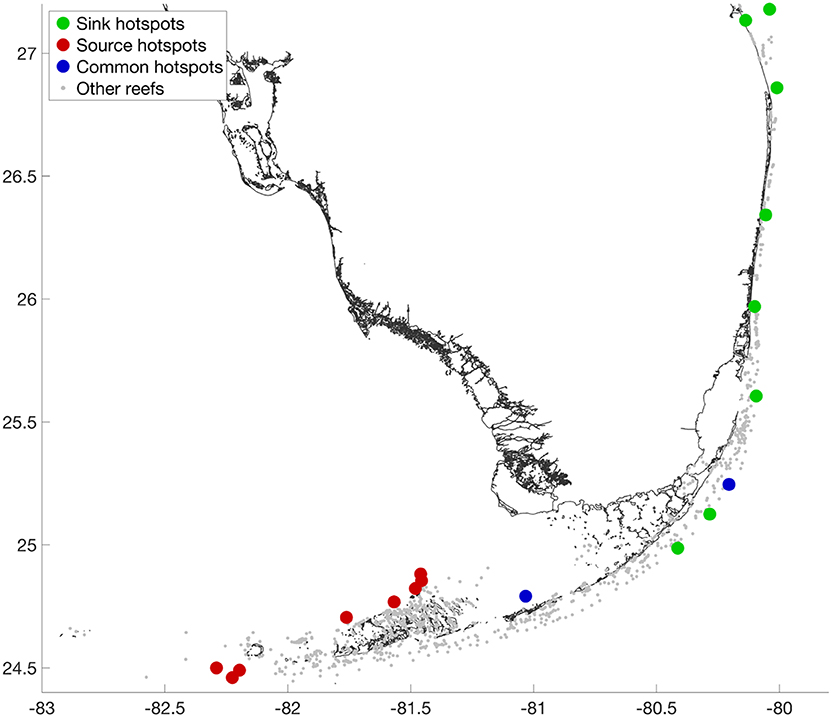
Figure 5. Top 1% source and sink reefs obtained with the PageRank algorithm. The top sink reefs are all located downstream, on the outer shelf. These reefs have the most robust supply of larvae and should be among the most resilient reefs. The top source reefs are located upstream of the two main connectivity pathways. These are the reefs that are most useful to the rest of the system.
The top 1% protection hotspots are located in the same areas as the source hotspots (Figure 6). However, it is not exactly the same reefs within these areas that have the largest values for both indices. The protection index also takes into account the supply of larvae. It highlights the reefs that are at the same time useful (high output) and fragile (low input). Since it is based on the PageRank, it is a non-local measure that takes the entire graph topology into account. The restoration index highlights the reefs that are both good sources and good sinks. These reefs are shared between the inner shelf area of the Lower and Middle Keys and the outer shelf area in the Upper Keys. Figures illustrating the subgraphs associated with a protection and a restoration hotspot are provided online as Supplementary Material. Protection hotspots are characterized by a large downstream and a small upstream footprint. Restoration hotspots combine large downstream and upstream footprints.
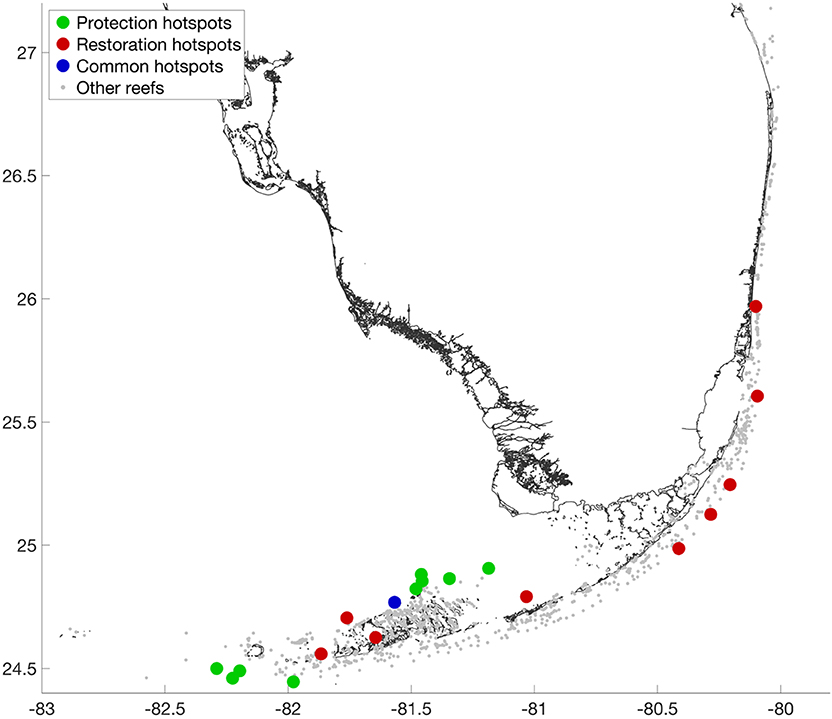
Figure 6. Top 1% reefs most in need of protection and best suited to restoration projects. Reefs that need protection are located on the same areas as the best source reefs. Reefs best suited to restoration are more spread in the FRT as both inner- and outer-shelf reefs can be suitable.
Betweenness measures the degree to which a reef serves as a stepping stone to multigenerational connectivity. Larvae released on the most upstream reefs will eventually arrive to downstream reefs preferably through those hubs. We can clearly distinguish two regions where the betweenness hotspots are located: the Lower Keys and the Upper Keys (Figure 7A). In the former, the dense reefs system yields a large amount of mixing and hence have a densely-connected sub-graph. The latter acts as a transit zone between the Lower Keys and reefs North of Biscayne Bay. Between those two regions, there is a reef located North from Vaca Key (that we will call Vaca reef), which has by far the largest betweenness value of all reefs in the FRT. It receives many larvae from the upstream reefs but it also provides many larvae to downstream reefs (Figure 7B). It appears to be a central stepping stone within the connectivity graph as a very large number of connections and larvae arrive to that node and leave from it. Among the 990 reefs composing the FRT, about 660 reefs are connected to the Vaca reef. Hence two thirds of all the reefs in the FRT either provide or receive larvae to/from the Vaca reef.
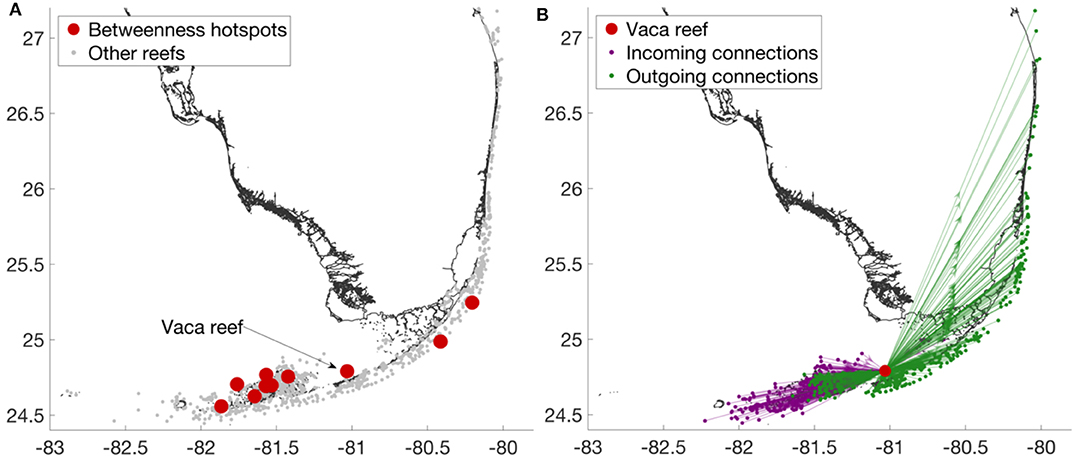
Figure 7. (A) The top 1% reefs with the largest betweenness value. Among these hotspots, the reef with the largest betweenness value is the Vaca reef. (B) Close-up view on the subgraph associated with the Vaca reef. This subgraph highlights the importance of the Vaca reef as a major hub in the connectivity graph. About 2/3 of all the reefs in the FRT are connected to the Vaca reef.
When considering only connections that are demographically significant, i.e., with weights larger than 0.1% of the total amount of larvae released over the source reef, we can identify two major SCCs (Figure 8A). The first one regroups the majority of the reefs in the Lower Keys, where the dense reef system favors small-distance bidirectional connectivity. The other SCC is located in the Upper Keys. It is interesting to note that this cluster contains reefs that are both on the outer and inner shelves. They are connected together thanks to the model fine mesh resolution that allows us to explicitly represent the narrow passages through the topographical barrier that separates both areas. The MO algorithm requires only one-directional connections within a community. When using a resolution parameter ζ = 2 × 10−5, we obtain a stable community structure that includes most of the reefs in the FRT (Figure 8B). The Dry Tortugas are not part of that large community. The Lower Keys appear to be well connected to the outer-shelf region and directly/indirectly provide larvae to the most upstream reefs. The outer shelf area can be seen as a conveyor belt that transports larvae all the way from the Lower Keys to northernmost reefs in the FRT.
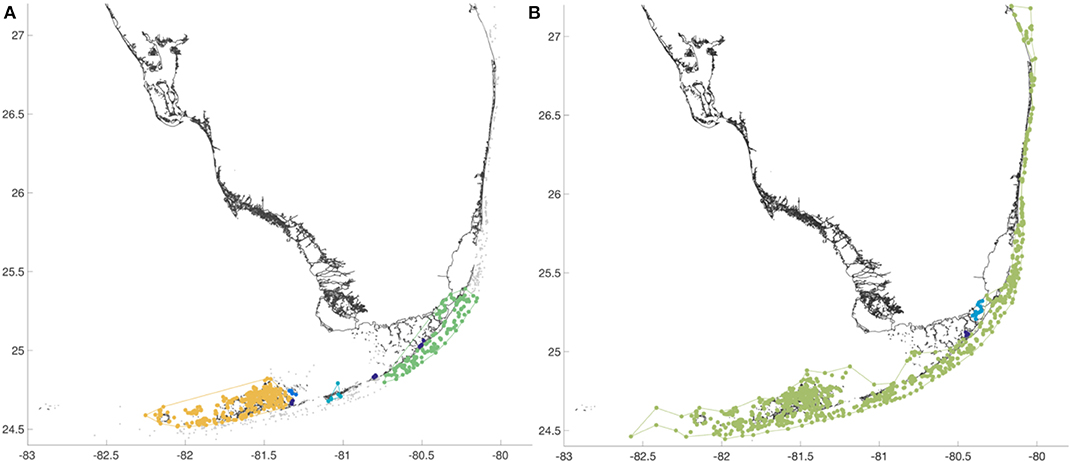
Figure 8. (A) When taking only demographically significant connections into account, the SCC clustering method keeps two main groups of reefs in the Lower and Upper Keys. (B) The community partitioning obtained with the MO method for a resolution parameter ζ = 2 × 10−5 shows one major community that encompasses almost all the FRT. The existence of such a large community shows that the FC acts as a conveyor belts that connects almost all the reefs in Keys to the reefs along the outer shelf.
4. Discussion and Conclusions
By using a high-resolution ocean model, we have been able to model the fine-scale details of coral connectivity pathways throughout the FRT. Our results for the 2010 spawning event suggest that there are two major connectivity pathways originating from the Lower Keys area (Figure 9). One pathway starts on the outer shelf, South of the Marquesas Keys, and is driven by the FC that acts as a major conveyor belt. The other pathway originates North of the Lower Keys and connects to the outer shelf either by passing through the dense reef system composing the Lower Keys, or through the Middle Keys. The areas from where these two pathways originate contain the reefs that should be protected in priority. Restoration hotspots are more evenly spread between the Lower and Upper Keys. Along the connectivity pathways, the Vaca reef appears to be a major stepping stone between the Lower Keys and the northern part of the FRT.
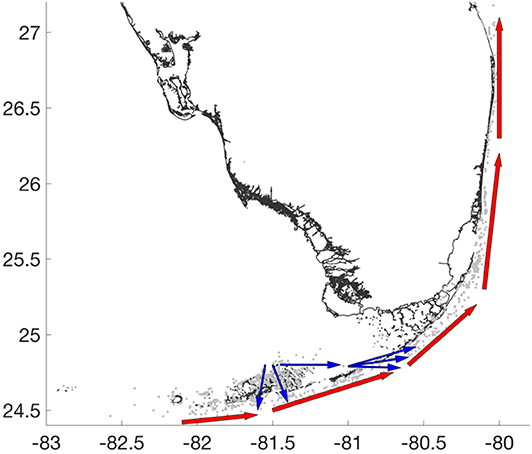
Figure 9. Illustration of the two main connectivity pathways identified by our model. The outershelf pathway (red arrows) acts as a conveyor belt driven by the FC that connects most of the reefs from the South to the North. The Lower Keys pathway (blue arrows) connects the inner shelf reefs to the outer shelf by passing through the Lower and Middle Keys.
The model shows that larval transport is very different in the inner and outer shelf areas. The former is characterized by a higher local retention, a smaller weighted connectivity length and a higher proportion of larvae that settle locally because of the higher sticky water effect. By using a high resolution ocean model, we can explicitly represent recirculation eddies near reefs and islands that will further retain larvae. Hence, by increasing the model resolution, local retention is increased and long-distance connectivity is reduced. As discussed by Briton et al. (2018), high local retention is directly associated with the self-persistence of the local population. These reefs are therefore expected to sustain themselves and be quite resilient. This may partly explain why the reefs in the Keys historically are the most diverse and have the best coral coverage (Burman et al., 2012) as opposed to the north of the reef tract. However, other factors like proximity to population centers and the latitudinal shift into a more temperate climate regime are also major factors (Walker, 2012; Walker and Gilliam, 2013).
While most larvae from the Lower Keys stay in that area, a fraction can reach the outer shelf. Reefs on the outer shelf are under the influence of stronger currents. Local retention is hence lower, whilst weighted connectivity length and proportion lost are higher. Larvae released there will indeed be quickly taken away by the FC and transported over longer distances, although the mesoscale circulation is able to retain larvae and thus temper this long-distance export (Sponaugle et al., 2005, 2012; D'Alessandro et al., 2007; Vaz et al., 2016). If we look at the FRT as a road network, the outer shelf area would be the main highway and the inner shelf would be composed of many smaller roads that eventually connect to this highway. While sometimes overlooked, the inner shelf is thus an important component of the FRT. It further appears to have higher coral diversity, which leads to greater reef complexity and thus habitat for fish and lobsters, both important recreational fisheries.
The community structure obtained with the modularity optimization algorithm supports that the FC acts like a major conveyor belt that connects almost all the reefs in the FRT in one direction. Most of the reefs in the Lower Keys belong to this large community and form the initial pool of larvae that will feed the rest of the system. Such a community structure is quite different from the one obtained by Thomas et al. (2014) for the Great Barrier Reef, where several large and mostly isolated communities could be identified. The SCC analysis further supports that the Lower Keys form a well-mixed area where a high genetic homogeneity is expected. This is supported by the genetic connectivity measures of Serrano et al. (2014) who show a high level of mixing for M. cavernosa in the Keys. It also shows the existence of a large community of reefs including most reefs of the Upper Keys. This SCC exists thanks to the tidal currents that move larvae back and forth, and hence produce a bidirectional connectivity pattern. Without such an important tidal flow, the residual FC would transport larvae to the North and that community would vanish. Both community structures suggest the vulnerability of the Dry Tortugas reefs since they were isolated from the rest of the system for the 2010 spawning event. The few reefs in this region must be able to provide themselves in larvae to survive but local retention is low in that area. However, it should be noted that we do not consider the potential transport of larvae from areas outside of the FRT. Holstein et al. (2014) have indeed shown that larvae can be transported from Yucatan and Cuba to the Lower Keys.
From a conservation perspective, our results for the 2010 spawning event suggest that protection effort should be focused on the reefs located upstream of the two connectivity pathways identified above. These reefs have a long-ranging influence. They not only directly provide larvae to neighboring reefs, but they are the starting point of connectivity pathways that extend up the northern end of the FRT. Being the most upstream reefs they are particularly fragile as they do not receive many larvae. These reefs are all located in the Florida Keys National Marine Sanctuary, which was established in 1990 and protects more than 2,900 square nautical miles of Florida Keys coastal and ocean waters. Within this marine sanctuary, the conservation hotspot reefs are either included in or very close to the Key West and Great White Heron National Wildlife Refuges. However, the level of protection in those areas is probably not sufficient for reefs to recover (Toth et al., 2014). The protections for no anchoring and no fishing are not countering all the other aspects that are not controlled (water temperature, disease, turbidity, nutrients).
According to our simulations, reefs best suited to restoration projects are more evenly spread between the Lower and Upper Keys. The model shows these reefs are both good sources and good sinks of larvae, which is supported by their high betweenness value. Since most of the reefs in the Lower and Upper Keys are included in a SCC, we can expect that outplanting corals on the reefs identified in those areas will have a strong effect on the local environment. One reef that stands out both for the restoration and betweenness measures is the Vaca Reef. This reef is very well-located between the Lower and Upper Key. We could assume that its great restoration value is due to its large size but normalizing the connectivity matrix by the number of larvae seeded does not change the results. However, it should be noted that the database used to generate the reef map indicates that only a fraction of the Vaca reef (from 0 to 10%) is effectively covered by corals. The amount of larvae released on this reef might therefore be overestimated. An improvement of the model would be to take into account the fraction of coral cover in the release and settling processes. This information is however still very incomplete in the database and we have thus chosen not to take it into account. We have therefore focused this study on potential connectivity.
As with any modeling study, it is important to understand the assumptions on which the model is based. Here, we have used a 2D barotropic ocean model, which is well suited to shallow regions but does not include all the physical processes that are driving the larger scale ocean circulation. It has therefore been coupled with the 3D HYCOM model as soon as the water depth is >50 m. This allows us to indirectly represent baroclinic phenomena, such as mesoscale eddies. However, the coupling might not be strong enough to correctly represent these eddies along the outer shelf, where they can influence larval transport (Limouzy-Paris et al., 1997; Sponaugle et al., 2005; D'Alessandro et al., 2007). Mesoscale eddies are known to increase local retention and, by misrepresenting them, SLIM might overestimate the WCL. On the other hand, the coupling uses the depth-averaged barotropic currents from HYCOM, which will be smaller than the near-surface currents that transport most of the larvae. As a result, SLIM might also underestimate the WCL along the shelf break.
Another limitation of our approach is that we only simulate larval dispersal for one spawning season and for one particular species. The interannual and interspecific variability is thus ignored. Considering other years and other species is currently being investigated with this model configuration. Properly taking into account the interannual connectivity variations requires to run dispersal simulations over multiple years. Recent studies in the Coral Triangle (Thompson et al., 2018) and in the north-west Mediterranean Sea (Hidalgo et al., 2019) suggest that at least 20 spawning events should be simulated to achieve robust estimates. This is not yet possible here considering the heavy computation cost of the SLIM set-up over the Florida Keys. Finally, the lack of sufficiently accurate coral cover and habitat quality information led us to compute only potential connectivity, which is obtained by assuming spatially-homogeneous larval production and post-settlement mortality rates.
Despite these assumptions, we believe that our model provides unprecedented perspectives on the role and impact of the small-scale circulation on the connectivity over the entire FRT. With a resolution 10 times higher than existing models, it can explicitly resolve complex topographical features such as inlets, channels and small islands. The resulting currents have much richer dynamics that yields better estimates of larval dispersal. That allowed us to identify connectivity pathways that are ignored by coarser models. In particular, we highlighted the importance of larval cross-shore transport, from the inner shelf to the outer shelf. By measuring a site's potential as a larval source and sink, our model can provide new insight to conservation and restoration strategies that are currently mostly based on empirical data. After checking for anthropogenic stress, it could help reef managers to distinguish between sites that locally appear equally good and hence provide much-needed quantitative decision support.
Data Availability Statement
The datasets generated for this study are available on request to the corresponding author.
Author Contributions
EH, ML, and JF conceptualized the study and designed the modeling experiments. CF and AS-A run the simulations and analyzed the results. AK, JF, and BW collected the biological and GIS data. JL, VV, and DV developed the model. All authors contributed to the writing of the manuscript.
Funding
This paper is a result of research funded by the National Oceanic and Atmospheric Administration's RESTORE Act Science Program under award NA15NOS4510226 to the University of Miami. ML was supported under the auspices of the Cooperative Institute for Marine and Atmospheric Studies (CIMAS), a cooperative institute of the University of Miami and NOAA, cooperative agreement NA10OAR4320143. This work was also supported by NOAA/AOML.
Conflict of Interest
The authors declare that the research was conducted in the absence of any commercial or financial relationships that could be construed as a potential conflict of interest.
Acknowledgments
Computational resources were provided by the Consortium des Équipements de Calcul Intensif (CÉCI), funded by the F.R.S.-FNRS under Grant No. 2.5020.11. This study was presented at the 3rd IMARCO and at the 11th IMUM conferences.
Supplementary Material
The Supplementary Material for this article can be found online at: https://www.frontiersin.org/articles/10.3389/fmars.2020.00312/full#supplementary-material
Footnotes
References
Arias, A., Cinner, J. E., Jones, R. E., and Pressey, R. L. (2015). Levels and drivers of fishers' compliance with marine protected areas. Ecol. Soc. 20, 1–14. doi: 10.5751/ES-07999-200419
Banks, K. W., Riegl, B. M., Richards, V. P., Walker, B. K., Helmle, K. P., Jordan, L. K., et al. (2008). “The reef tract of continental Southeast Florida (Miami-Dade, Broward and Palm Beach counties, USA),” in Coral Reefs of the USA, eds B. M. Riegl and R. E. Dodge (Dordrecht: Springer), 175–220. doi: 10.1007/978-1-4020-6847-8_5
Bellwood, D. R., Hughes, T. P., Folke, C., and Nyström, M. (2004). Confronting the coral reef crisis. Nature 429:827. doi: 10.1038/nature02691
Blondel, V. D., Guillaume, J., Lambiotte, R., and Lefebvre, E. (2008). Fast unfolding of communities in large networks. J. Stat. Mech. 10:P10008. doi: 10.1088/1742-5468/2008/10/P10008
Botsford, L. W., White, J. W., Coffroth, M.- A., Paris, C. B., Planes, S., Shearer, T. L., et al. (2009). Connectivity and resilience of coral reef metapopulations in marine protected areas: matching empirical efforts to predictive needs. Coral Reefs 28, 327–337. doi: 10.1007/s00338-009-0466-z
Brandes, U. (2001). A faster algorithm for betweenness centrality. J. Math. Sociol. 25, 163–177. doi: 10.1080/0022250X.2001.9990249
Briton, F., Cortese, D., Duhaut, T., and Guizien, K. (2018). High-resolution modelling of ocean circulation can reveal retention spots important for biodiversity conservation. Aquat. Conserv. 28, 882–893. doi: 10.1002/aqc.2901
Brown, B. (1987). Heavy metals pollution on coral reefs. Human Impacts on Coral Reefs: Facts and Recommendations. Antenne de Tahiti Muséum EPHE, Moorea, pages 119-134.
Bruno, J. F., Petes, L. E., Drew Harvell, C., and Hettinger, A. (2003). Nutrient enrichment can increase the severity of coral diseases. Ecol. Lett. 6, 1056–1061. doi: 10.1046/j.1461-0248.2003.00544.x
Bruno, J. F., and Selig, E. R. (2007). Regional decline of coral cover in the Indo-Pacific: timing, extent, and subregional comparisons. PLoS ONE 2:e711. doi: 10.1371/journal.pone.0000711
Bruno, J. F., and Valdivia, A. (2016). Coral reef degradation is not correlated with local human population density. Sci. Rep. 6:29778. doi: 10.1038/srep29778
Burgess, S. C., Kingsford, M. J., and Black, K. P. (2007). Influence of tidal eddies and wind on the distribution of presettlement fishes around One Tree Island, Great Barrier Reef. Mar. Ecol. Prog. Ser. 341, 233–242. doi: 10.3354/meps341233
Burman, S. G., Aronson, R. B., and van Woesik, R. (2012). Biotic homogenization of coral assemblages along the Florida Reef Tract. Mar. Ecol. Prog. Ser. 467, 89–96. doi: 10.3354/meps09950
Chassignet, E. P., Hurlburt, H. E., Smedstad, O. M., Halliwell, G. R., Hogan, P. J., Wallcraft, A. J., et al. (2007). The HYCOM (HYbrid Coordinate Ocean Model) data assimilative system. J. Mar. Syst. 65, 60–83. doi: 10.1016/j.jmarsys.2005.09.016
Cheal, A., MacNeil, M. A., Cripps, E., Emslie, M., Jonker, M., Schaffelke, B., et al. (2010). Coral-macroalgal phase shifts or reef resilience: links with diversity and functional roles of herbivorous fishes on the Great Barrier Reef. Coral Reefs 29, 1005–1015. doi: 10.1007/s00338-010-0661-y
Connell, J. H., Hughes, T. P., and Wallace, C. C. (1997). A 30-year study of coral abundance, recruitment, and disturbance at several scales in space and time. Ecol. Monogr. 67, 461–488. doi: 10.1890/0012-9615(1997)067[0461:AYSOCA]2.0.CO;2
Costa, A., Petrenko, A. A., Guizien, K., and Doglioli, A. M. (2017). On the calculation of betweenness centrality in marine connectivity studies using transfer probabilities. PLoS ONE 12:e0189021. doi: 10.1371/journal.pone.0189021
Cowen, R. K., and Sponaugle, S. (2009). Larval dispersal and marine population connectivity. Annu. Rev. Mar. Sci. 1, 443–466. doi: 10.1146/annurev.marine.010908.163757
Critchell, K., and Lambrechts, J. (2016). Modelling accumulation of marine plastics in the coastal zone; what are the dominant physical processes? Estuar. Coast. Shelf Sci. 171, 111–122. doi: 10.1016/j.ecss.2016.01.036
Daigle, R. M., Metaxas, A., Balbar, A., McGowan, J., Treml, E. A., Kuempel, C. D., et al. (2020). Operationalizing ecological connectivity in spatial conservation planning with Marxan Connect. Methods Ecol. Evol. 11, 570–579. doi: 10.1111/2041-210X.13349
D'Alessandro, E., Sponaugle, S., and Lee, T. (2007). Patterns and processes of larval fish supply to the coral reefs of the upper Florida keys. Mar. Ecol. Prog. Ser. 331, 85–100. doi: 10.3354/meps331085
De'ath, G., Fabricius, K. E., Sweatman, H., and Puotinen, M. (2012). The 27-year decline of coral cover on the Great Barrier Reef and its causes. Proc. Natl. Acad. Sci. U.S.A. 109, 17995–17999. doi: 10.1073/pnas.1208909109
Dimou, K., and Adams, E. (1993). A Random-walk, particle tracking model for well-mixed estuaries and coastal waters. Estuar. Coast. Shelf Sci. 37, 99–110. doi: 10.1006/ecss.1993.1044
Dubois, M., Rossi, V., Ser-Giacomi, E., Arnaud-Haond, S., Lopez, C., and Hernandez-Garcia, E. (2016). Linking basin-scale connectivity, oceanography and population dynamics for the conservation and management of marine ecosystems. Glob. Ecol. Biogeogr. 25, 503–515. doi: 10.1111/geb.12431
Enos, P. (1977). Quaternary sedimentation in South Florida, Part I, Holocene sediment accumulations of the South Florida shelf margin. Geol. Soc. Am. 147:198. doi: 10.1130/MEM147-p1
Erftemeijer, P. L., Riegl, B., Hoeksema, B. W., and Todd, P. A. (2012). Environmental impacts of dredging and other sediment disturbances on corals: a review. Mar. Pollut. Bull. 64, 1737–1765. doi: 10.1016/j.marpolbul.2012.05.008
Figueiredo, J., Baird, A. H., and Connolly, S. R. (2013). Synthesizing larval competence dynamics and reef-scale retention reveals a high potential for self-recruitment in corals. Ecology 94, 650–659. doi: 10.1890/12-0767.1
Finkl, C. W., and Andrews, J. L. (2008). Shelf geomorphology along the southeast Florida atlantic continental platform: Barrier coral reefs, nearshore bedrock, and morphosedimentary features. J. Coast. Res. 244, 823–849. doi: 10.2112/08A-0001.1
Fratantoni, P. S., Lee, T. N., Podesta, G. P., and Muller-Karger, F. (1998). The influence of Loop Current perturbations on the formation and evolution of Tortugas eddies in the southern Straits of Florida. J. Geophys. Res. 103, 24759–24779. doi: 10.1029/98JC02147
Gardner, T. A., Côté, I. M., Gill, J. A., Grant, A., and Watkinson, A. R. (2003). Long-term region-wide declines in Caribbean corals. Science 301, 958–960. doi: 10.1126/science.1086050
Geuzaine, C., and Remacle, J.-F. (2009). GMSH: A 3-D finite element mesh generator with built-in pre- and post-processing facilities. Int. J. Numer. Methods Eng. 156.6, 1297–1309. doi: 10.1002/nme.2579
Graham, N. A., Jennings, S., MacNeil, M. A., Mouillot, D., and Wilson, S. K. (2015). Predicting climate-driven regime shifts versus rebound potential in coral reefs. Nature 518, 94–97. doi: 10.1038/nature14140
Grech, A., Hanert, E., McKenzie, L., Rasheed, M., Thomas, C., Tol, S., et al. (2018). Cumulative effects of multiple disturbance events on seagrass connectivity. Glob. Change Biol. 24, 3093–3104. doi: 10.1111/gcb.14127
Grech, A., Wolter, J., Coles, R., McKenzie, L., Rasheed, M., Thomas, C., et al. (2016). Spatial patterns of seagrass dispersal and settlement. Divers. Distrib. 22, 1150–1162. doi: 10.1111/ddi.12479
Harrison, H. B., Williamson, D. H., Evans, R. D., Almany, G. R., Thorrold, S. R., Russ, G. R., et al. (2012). Larval export from marine reserves and the recruitment benefit for fish and fisheries. Curr. Biol. 22, 1023–1028. doi: 10.1016/j.cub.2012.04.008
Hastings, A., and Botsford, L. W. (2006). Persistence of spatial populations depends on returning home. Proc. Natl. Acad. Sci. U.S.A. 103, 6067–6072. doi: 10.1073/pnas.0506651103
Hidalgo, M., Rossi, V., Monroy, P., Ser-Giacomi, E., Hernández-García, E., Guijarro, B., et al. (2019). Accounting for ocean connectivity and hydroclimate in fish recruitment fluctuations within transboundary metapopulations. Ecol. Appl. 29:e01913. doi: 10.1002/eap.1913
Hoegh-Guldberg, O., Poloczanska, E. S., Skirving, W., and Dove, S. (2017). Coral reef ecosystems under climate change and ocean acidification. Front. Mar. Sci. 4:158. doi: 10.3389/fmars.2017.00158
Hoffmeister, J., and Multer, H. (1968). Geology and origin of the Florida Keys. Geol. Soc. Am. Bull. 79, 1487–1502. doi: 10.1130/0016-7606(1968)79[1487:GAOOTF]2.0.CO;2
Holstein, D. M., Paris, C. B., and Mumby, P. J. (2014). Consistency and inconsistency in multispecies population network dynamics of coral reef ecosystem. Mar. Ecol. Prog. Ser. 499, 1–18. doi: 10.3354/meps10647
Hughes, T. P., Anderson, K. D., Connolly, S. R., Heron, S. F., Kerry, J. T., Lough, J. M., et al. (2018). Spatial and temporal patterns of mass bleaching of corals in the anthropocene. Science 359, 80–83. doi: 10.1126/science.aan8048
Hughes, T. P., Baird, A. H., Bellwood, D. R., Card, M., Connolly, S. R., Folke, C., et al. (2003). Climate change, human impacts, and the resilience of coral reefs. Science 301, 929–933. doi: 10.1126/science.1085046
Jackson, J., Donovan, M., Cramer, K., and Lam, V. (2014). Status and Trends of Caribbean Coral Reefs. Gland: Global Coral Reef Monitoring Network; IUCN.
Jackson, J. B., Kirby, M. X., Berger, W. H., Bjorndal, K. A., Botsford, L. W., Bourque, B. J., et al. (2001). Historical overfishing and the recent collapse of coastal ecosystems. Science 293, 629–637. doi: 10.1126/science.1059199
Jordan, A. (2018). Patterns in Caribbean coral spawning [Master's thesis]. Halmos College of Natural Sciences and Oceanography; Nova Southeastern University, Fort Lauderdale, FL, United States.
Jordan, L., Banks, K., Fisher, L. E., Walker, B. K., and Gilliam, D. S. (2010). Elevated sedimentation on coral reefs adjacent to a beach nourishment project. Mar. Pollut. Bull. 60, 261–271. doi: 10.1016/j.marpolbul.2009.08.032
Kininmonth, S., Weeks, R., Abesamis, R. A., Bernardo, L. P. C., Beger, M., Treml, E. A., et al. (2019). Strategies in scheduling marine protected area establishment in a network system. Ecol. Appl. 29:e01820. doi: 10.1002/eap.1820
Knowlton, N. (2001). The future of coral reefs. Proc. Natl. Acad. Sci. U.S.A. 98, 5419–5425. doi: 10.1073/pnas.091092998
Kool, J. T., Moilanen, A., and Treml, E. A. (2013). Population connectivity: recent advances and new perspectives. Landsc. Ecol. 28, 165–185. doi: 10.1007/s10980-012-9819-z
Kourafalou, V. H., and Kang, H. (2012). Florida Current meandering and evolution of cyclonic eddies along the Florida Keys Reef Tract: are they interconnected? J. Geophys. Res. 117:C05028. doi: 10.1029/2011JC007383
Kuba, A. (2016). Transgenerational effects of thermal stress impacts on and beyond coral reproduction [Master's thesis]. Halmos College of Natural Sciences and Oceanography; Nova Southeastern University, Fort Lauderdale, FL, United States.
Kuffner, I. B., Lidz, B. H., Hudson, J. H., and Anderson, J. S. (2015). A century of ocean warming on Florida Keys coral reefs: historic in situ observations. Estuar. Coasts 38, 1085–1096. doi: 10.1007/s12237-014-9875-5
Lambrechts, J., Hanert, E., Deleersnijder, E., Bernard, P.-E., Legat, V., Remacle, J.-F., et al. (2008). A multi-scale model of the hydrodynamics of the whole Great Barrier Reef. Estuar. Coast. Shelf Sci. 79, 143–151. doi: 10.1016/j.ecss.2008.03.016
Le Hénaff, M., Kourafalou, V. H., Paris, C. B., Helgers, J., Aman, Z. M., Hogan, P. J., et al. (2012). Surface evolution of the Deepwater Horizon oil spill patch: combined effects of circulation and wind-induced drift. Environ. Sci. Technol. 46, 7267–7273. doi: 10.1021/es301570w
Lee, T. N., Johns, E., Wilson, D., and Smith, N. (2001). “Transport processes linking South Florida coastal ecosystems,” in The Everglades, Florida Bay, and Coral Reefs of the Florida Keys: An Ecosystem Sourcebook, ed J. Porter (Boca Raton, FL: CRC Press), 309–343. doi: 10.1201/9781420039412-15
Lee, T. N., Leaman, K., Williams, E., Berger, T., and Atkinson, L. (1995). Florida Current meanders and gyre formation in the southern Straits of Florida. J. Geophys. Res. 100, 8607–8620. doi: 10.1029/94JC02795
Lee, T. N., and Mayer, D. A. (1977). Low-frequency current variability and spin-off Southeast Florida. J. Mar. Res. 35, 193–220.
Lee, T. N., and Smith, N. (2002). Volume transport variability through the Florida keys tidal channels. Continent. Shelf Res. 22, 1361–1377. doi: 10.1016/S0278-4343(02)00003-1
Lidz, B. H., and Shinn, E. A. (1991). Paleoshorelines, reefs, and a rising sea: South Florida, USA. J. Coast. Res. 7, 203–229.
Limouzy-Paris, C. B., Graber, H. C., Jones, D. L., Röpke, A. W., and Richards, W. J. (1997). Translocation of larval coral reef fishes via sub-mesoscale spin-off eddies from the Florida Current. Bull. Mar. Sci. 60, 966–983.
Lirman, D., Schopmeyer, S., Manzello, D., Gramer, L. J., Precht, W. F., Muller-Karger, F., et al. (2011). Severe 2010 cold-water event caused unprecedented mortality to corals of the Florida Reef Tract and reversed previous survivorship patterns. PLoS ONE 6:e23047. doi: 10.1371/journal.pone.0023047
Mezić, I., Loire, S., Fonoberov, V. A., and Hogan, P. (2010). A new mixing diagnostic and Gulf oil spill movement. Science 330, 486–489. doi: 10.1126/science.1194607
Miller, M. W., Kerr, K., and Williams, D. E. (2016). Reef-scale trends in Florida acropora spp. abundance and the effects of population enhancement. PeerJ 4:e2523. doi: 10.7717/peerj.2523
Minor, E. S., and Urban, D. L. (2007). Graph theory as a proxy for spatially explicit population models in conservation planning. Ecol. Appl. 17, 1771–1782. doi: 10.1890/06-1073.1
Moberg, F., and Folke, C. (1999). Ecological goods and services of coral reef ecosystems. Ecol. Econ. 29, 215–233. doi: 10.1016/S0921-8009(99)00009-9
Monroy, P., Rossi, V., Ser-Giacomi, E., Löpez, C., and Hernández-García, E. (2017). Sensitivity and robustness of larval connectivity diagnostics obtained from Lagrangian flow networks. ICES J. Mar. Sci. 74, 1763–1779. doi: 10.1093/icesjms/fsw235
Mumby, P. J., Dahlgren, C. P., Harborne, A. R., Kappel, C. V., Micheli, F., Brumbaugh, D. R., et al. (2006). Fishing, trophic cascades, and the process of grazing on coral reefs. Science 311, 98–101. doi: 10.1126/science.1121129
Okubo, A. (1971). Oceanic diffusion diagrams. Deep Sea Res. 18, 789–802. doi: 10.1016/0011-7471(71)90046-5
Page, C. A., Muller, E. M., and Vaughan, D. E. (2018). Microfragmenting for the successful restoration of slow growing massive corals. Ecol. Eng. 123, 86–94. doi: 10.1016/j.ecoleng.2018.08.017
Page, L., Brin, S., Motwani, R., and Winograd, T. (1999). The PageRank Citation Ranking: Bringing Order to the Web. Technical report, Stanford InfoLab.
Pandolfi, J. M., Bradbury, R. H., Sala, E., Hughes, T. P., Bjorndal, K. A., Cooke, R. G., et al. (2003). Global trajectories of the long-term decline of coral reef ecosystems. Science 301, 955–958. doi: 10.1126/science.1085706
Rayfield, B., Fortin, M.-J., and Fall, A. (2011). Connectivity for conservation: a framework to classify network measures. Ecology 92, 847–858. doi: 10.1890/09-2190.1
Ritson-Williams, R., Arnold, S. N., Fogarty, N. D., Steneck, R. S., Vermeij, M. J., and Paul, V. J. (2009). New perspectives on ecological mechanisms affecting coral recruitment on reefs. Smithson. Contrib. Mar. Sci. 38:437. doi: 10.5479/si.01960768.38.437
Selig, E. R., and Bruno, J. F. (2010). A global analysis of the effectiveness of marine protected areas in preventing coral loss. PLoS ONE 5:e9278. doi: 10.1371/journal.pone.0009278
Serrano, X., Baums, I., O'Reilly, K., Smith, T., Jones, R., Shearer, T., et al. (2014). Geographic differences in vertical connectivity in the Caribbean coral Montastraea cavernosa despite high levels of horizontal connectivity at shallow depths. Mol. Ecol. 23, 4226–4240. doi: 10.1111/mec.12861
Sheppard, C., Davy, S., Pilling, G., and Graham, N. (2009). The Biology of Coral Reefs. Oxford: Oxford University Press. doi: 10.1093/acprof:oso/9780198566359.001.0001
Shinn, E. A., Steinen, R. P., Lidz, B. H., and Swart, P. K. (1989). Whitings, a sedimentologic dilemma: perspectives. J. Sediment. Res. 59. doi: 10.1306/212F8F3A-2B24-11D7-8648000102C1865D
Shulzitski, K., Sponaugle, S., Hauff, M., Walter, K., D'Alessandro, E. K., and Cowen, R. K. (2015). Close encounters with eddies: oceanographic features increase growth of larval reef fishes during their journey to the reef. Biol. Lett. 11:20140746. doi: 10.1098/rsbl.2014.0746
Shulzitski, K., Sponaugle, S., Hauff, M., Walter, K. D., and Cowen, R. K. (2016). Encounter with mesoscale eddies enhances survival to settlement in larval coral reef fishes. Proc. Natl. Acad. Sci. U.S.A. 113, 6928–6933. doi: 10.1073/pnas.1601606113
Southeast Regional Office, P. R. D. (2015). Recovery Plan for Elkhorn (Acropora palmata) and Staghorn (A. cervicornis) Corals. Available online at: http://data.nodc.noaa.gov/coris/library/NOAA/CRCP/project/2160/final_acropora_recovery_plan.pdf (accessed March 20, 2020).
Spagnol, S., Wolanski, E., Deleersnijder, E., Brinkman, R., McAllister, F., Cushman-Roisin, B., et al. (2002). An error frequently made in the evaluation of advective transport in two-dimensional Lagrangian models of advection-diffusion in coral reef waters. Mar. Ecol. Prog. Ser. 235, 299–302. doi: 10.3354/meps235299
Sponaugle, S., Lee, T., Kourafalou, V., and Pinkard, D. (2005). Florida Current frontal eddies and the settlement of coral reef fishes. Limnol. Oceanogr. 50, 1033–1048. doi: 10.4319/lo.2005.50.4.1033
Sponaugle, S., Paris, C., Walter, K., Kourafalou, V., and D'Alessandro, E. (2012). Observed and modeled larval settlement of a reef fish to the Florida Keys. Mar. Ecol. Prog. Ser. 453, 201–212. doi: 10.3354/meps09641
Tarjan, R. E. (1972). Depth-first search and linear graph algorithms. SIAM J. Comput. 1, 146–160. doi: 10.1137/0201010
Thomas, C.J., Bridge, T.C.L., Figueiredo, J., Deleersnijder, E., and Hanert, E. (2015). Connectivity between submerged and near-sea-surface coral reefs: can submerged reef populations act as refuges? Divers. Distrib. 21, 1254–1266. doi: 10.1111/ddi.12360
Thomas, C.J., Lambrechts, J., Wolanski, E., Traag, V.A., Blondel, V.D., Deleersnijder, E., et al. (2014). Numerical modelling and graph theory tools to study ecological connectivity in the Great Barrier Reef. Ecol. Modell. 272, 160–174. doi: 10.1016/j.ecolmodel.2013.10.002
Thompson, D., Kleypas, J., Castruccio, F., Curchitser, E., Pinsky, M., Jönsson, B., et al. (2018). Variability in oceanographic barriers to coral larval dispersal: do currents shape biodiversity? Prog. Oceanogr. 165, 110–122. doi: 10.1016/j.pocean.2018.05.007
Toth, L., Van Woesik, R., Murdoch, T., Smith, S., Ogden, J., Precht, W., et al. (2014). Do no-take reserves benefit Florida's corals? 14 years of change and stasis in the Florida Keys National Marine Sanctuary. Coral Reefs 33, 565–577. doi: 10.1007/s00338-014-1158-x
Traag, V. A., Van Dooren, P., and Nesterov, Y. (2011). Narrow scope for resolution-limit-free community detection. Phys. Rev. E 84:016114. doi: 10.1103/PhysRevE.84.016114
Valentine, D. L., Mezić, I., Maćešic, S., Črnjarić-Žic, N., Ivić, S., Hogan, P. J., et al. (2012). Dynamic autoinoculation and the microbial ecology of a deep water hydrocarbon irruption. Proc. Natl. Acad. Sci. U.S.A. 109, 20286–20291. doi: 10.1073/pnas.1108820109
Vaz, A. C., Paris, C. B., Olascoaga, M. J., Kourafalou, V. H., Kang, H., and Reed, J. K. (2016). The perfect storm: match-mismatch of bio-physical events drives larval reef fish connectivity between Pulley Ridge mesophotic reef and the Florida Keys. Continent. Shelf Res. 125, 136–146. doi: 10.1016/j.csr.2016.06.012
Walker, B. K. (2012). Spatial analyses of benthic habitats to define coral reef ecosystem regions and potential biogeographic boundaries along a latitudinal gradient. PLoS ONE 7:e30466. doi: 10.1371/journal.pone.0030466
Walker, B. K., and Gilliam, D. S. (2013). Determining the extent and characterizing coral reef habitats of the northern latitudes of the Florida Reef Tract (Martin County). PLoS ONE 8:e80439. doi: 10.1371/journal.pone.0080439
Walton, C., Hayes, N. K., and Gilliam, D. S. (2018). Impacts of a regional, multi-year, multi-species coral disease outbreak in southeast Florida. Front. Mar. Sci. 5:323. doi: 10.3389/fmars.2018.00323
Williams, D., and Miller, M. (2012). Attributing mortality among drivers of population decline in acropora palmata in the Florida Keys (USA). Coral Reefs 31, 369–382. doi: 10.1007/s00338-011-0847-y
Keywords: Florida reef tract, coral connectivity, biophysical modeling, PageRank, community detection, reef management
Citation: Frys C, Saint-Amand A, Le Hénaff M, Figueiredo J, Kuba A, Walker B, Lambrechts J, Vallaeys V, Vincent D and Hanert E (2020) Fine-Scale Coral Connectivity Pathways in the Florida Reef Tract: Implications for Conservation and Restoration. Front. Mar. Sci. 7:312. doi: 10.3389/fmars.2020.00312
Received: 27 December 2019; Accepted: 16 April 2020;
Published: 07 May 2020.
Edited by:
Linda Wegley Kelly, San Diego State University, United StatesReviewed by:
Raven Delaney Blakeway, Texas A&M University at Galveston, United StatesThiago Mendes, Federal University of Sǎo Paulo, Brazil
Copyright © 2020 Frys, Saint-Amand, Le Hénaff, Figueiredo, Kuba, Walker, Lambrechts, Vallaeys, Vincent and Hanert. This is an open-access article distributed under the terms of the Creative Commons Attribution License (CC BY). The use, distribution or reproduction in other forums is permitted, provided the original author(s) and the copyright owner(s) are credited and that the original publication in this journal is cited, in accordance with accepted academic practice. No use, distribution or reproduction is permitted which does not comply with these terms.
*Correspondence: Emmanuel Hanert, ZW1tYW51ZWwuaGFuZXJ0JiN4MDAwNDA7dWNsb3V2YWluLmJl
†These authors have contributed equally to this work