- Pacific Islands Fisheries Science Center, NOAA Inouye Regional Center, Honolulu, HI, United States
Environmental conditions of deep-sea corals were monitored with instruments placed in and adjacent to three Hawaiian deep-sea coral patches dominated by gorgonian octocorals and zoanthid gold coral. Temperature, backscatter, and flow differed among and within the patches and highlighted distinctions in distribution of focal taxa (Hemicorallium laauense, Pleurocorallium secundum, Narella spp., Acanella dispar, Kulamanamana haumeaae). Two of the patches (Barbers Pt., Makapu‘u Pt.) had more than double the sustained mean flow of the third patch (Keahole Pt.), where backscatter levels of the passing water mass showed scattering strengths a third higher, suggesting greater food supply in the water at the Keahole Pt. patch. Further, spectral analysis of flow speed and direction suggests that flow at the first two high-flow sites (Barbers Pt., Makapu‘u Pt.) are dominated by semi-diurnal tidal forcing (flow changing 4x daily, direction 2x daily), while Keahole Pt. patch shows a distinct pattern more typical of diurnal forcing. Of the focus taxa, the two coralliids occupied a similar temperature range but differed in dominance between sites along a flow/scatter gradient, with the “red” coral, H. laauense, found at the site with low flow (0.5–4.9 cm/s) and higher scatter (−28 dB) and the “pink” coral, P. secundum, seen at the patch with higher sustained flow (12.6–18.4 cm/s) and lower backscatter (−43 dB). Narella spp. spanned a 10°C temperature range but were found more frequently at sites with the highest mean flow (18.4–21.7 cm/s). The final two corals, the parasitic zoanthid “gold” coral, K. haumeaae, and its most common host, bamboo coral, A. dispar, were found at all three sites over a wide temperature range with flow ranging from 2.8 to 18.9 cm/s. The number of gold colonies was negatively correlated with flow even though that relationship was not apparent for the bamboo coral. These patterns were considered in relation to what is known about the life history of deep-sea corals and how they might influence community settlement, growth, and diversity.
Introduction
Little is understood about the patchy nature of deep-sea coral communities and the current flow fields upon which they depend. Typically, depth and its correlate temperature are the first variables used to describe the boundaries of a taxon’s potential distribution (Dolan et al., 2008; Parrish et al., 2017). Then to further constrain its range, conspicuous features in the bathymetry (e.g., summits and ridges) are identified that might change flow and provide some premium conditions for settlement and growth (Genin et al., 1986, 1992; Tracey et al., 2011; Tong et al., 2012). This approach has been used with mixed success in Hawai‘i to find locations of commercially sought “precious corals” (Pleurocorallium secundum, Hemicorallium laauense, Acanella dispar, Kulamanamana haumeaae) that were harvested to obtain raw materials for the jewelry trade (Grigg, 1993). Even at sites where precious corals have been surveyed, there have been notable differences in depth ranges between patches of these corals (Parrish, 2007), and the fauna distribution can vary considerably over relatively small spatial scales within the same patch (Long and Baco, 2013). The Hawaiian precious coral fishery is currently dormant, but there is still a need to predict the location of coral ecosystems in order to protect them from impacts of fishing activities (Roberts, 2002; Clark and Koslow, 2007; Clark et al., 2016; Baco et al., 2019) cable laying, alternate energy (Bauer et al., 2016), and deep-sea mining (Hein et al., 2013). The most efficient and promising approaches are modeling efforts that use large-scale data sets of coral presence in fishery bycatch and combine seafloor bathymetry with oceanographic models to project the probability of deep-sea coral occurrence (Bryan and Metaxas, 2007; Dolan et al., 2008; Tracey et al., 2011; Guinotte and Davies, 2014; Anderson et al., 2016a,b; Bauer et al., 2016; Rowden et al., 2017; Rooper et al., 2018; Georgian et al., 2019).
A fundamental challenge to all deep-sea coral community work is determining what defines good habitat for deep-sea corals. For Hawaiian corals, which grow as solitary colonies, good habitat is indicated by their presence in higher densities. Exploration surveys of the deep-sea show corals have a very wide distribution (Bohnenstiehl et al., 2018; Cantwell et al., 2018), and their presence is often identified from encountering a single colony, especially for some species of black corals and gorgonian octocorals. One coral colony does indeed indicate the habitat is minimally suitable for that taxon, but perhaps not ideal for higher density (and greater diversity) communities that we refer to as a coral garden or a patch (Bullimore et al., 2013). Inherently, a patch is a conspicuous change from the background density, and for the main Hawaiian Islands, which are located south of the productive waters of the North Pacific Basin (Polovina et al., 2001), the background is an impoverished condition where the seafloor is often a barren tract with few to no coral colonies. As a frame of reference to describe the typical density of a patch, we can look at the standardized scoring of visual transect data from submersible and remote operated vehicle (ROV) surveys submitted in regional reports to NOAA’s Deep-Sea Coral Research and Technology Program. They define high-density communities “as having at least 3000 combined coral and sponge counts per kilometer” (NOAA National Database for Deep-Sea Corals Sponges, 2019). Given the illuminated view area of the camera on the survey vehicles (extending ∼7 m ahead for an area of ∼50 square meters), this roughly corresponds to a high-density patch averaging more than 21 colonies within the camera’s field of view.
Measuring environmental conditions at patches, especially those dominated by a particular coral taxon, is one strategy to identify good habitat conditions for that taxon. Comparing the conditions across the patch from high to low colony density could show an environmental gradient. Assuming consistent depth and substrate, we would expect the patch to extend to where flow conditions permit growth. At the center of the patch where density is highest and corals thrive, flow should be different than at the edge of the patch where the ability to sustain coral growth seems to end. Conditions at the center of these patches must be stable through time, persisting long enough for the slow-growing deep-sea corals to colonize, grow, and reproduce. Studies of deep-sea corals indicate this process of growth and development can take decades for primnoids and coralliids (Andrews et al., 2002; Roark et al., 2006; Matsumoto, 2007), more than a century for isidids (Roark et al., 2005; Tracey et al., 2007; Andrews et al., 2009; Prouty et al., 2017), and millennia for proteinaceous corals like K. haumeaae (Druffel et al., 1995; Parrish and Roark, 2009; Roark et al., 2009). For this reason, the presence of a dense coral patch is a reliable indicator that the site is a consistently high quality habitat for coral colonization and growth.
There are few opportunities to consider current flow from the perspective of the coral colony. Usually, we use large scale characterizations of regional hydrodynamic models that are sometimes augmented by individual submersible or ROV dive observations (Bohnenstiehl et al., 2018; Cairns, 2018; Cantwell et al., 2018; Auscavitch et al., 2020). Site-specific process studies tend to increase the resolution with more detailed measurements using moorings or landers deployed in the general vicinity of corals (Rogers et al., 2007; De Clippele et al., 2018). In this study, we go a step further and use submersibles to place and recover multiple instruments in and adjacent to known coral patches, composed primarily of primnoids, coralliids, isidids, and zoanthids, for 6 or more months looking for patterns in temperature, flow, tide, and scatter strength of water moving through the coral colonies.
Materials And Methods
Coral Patch Study Sites
We looked at three Hawaiian deep-sea coral patches between 300 and 450 m depth, all growing on fossil carbonate substrate. The patches were selected because they were dominated by the primary targets of the precious coral fishery, including the two Coralliidae: “pink” (P. secundum) and “red” coral (H. laauense), the “bamboo” coral (A. dispar), and the “gold” coral (K. haumeaae) formerly referred to as Gerardia sp. (Sinniger et al., 2013). The gold coral is a zoanthid parasite that colonizes other species of deep coral but primarily the A. dispar bamboo colonies (Parrish, 2015). Finally, a primnoid group “Narella spp.” was included to provide a range of “focal” coral taxa known to occur in sufficient numbers to permit multiple independent observations of their immediate environment (Parrish et al., 2017). The locations of the patches’ high density centers (27–56 mean colonies in the vehicle’s field-of-view) and edges without colonies had been identified in prior survey work (Grigg, 1993, 2002; Parrish, 2007; Long and Baco, 2013). The bathymetry differed between the three sites and was expected to be a primary influence on observed flow conditions. The smallest and largest patches were on the southern shelf of the island of O‘ahu and included the Barbers Pt. patch on the summit of a small pinnacle and the Makapu‘u Pt. patch on a large, even tract of hardpan bottom sloped at an eight percent grade. Keahole Pt., the third patch, grew on the western edge of the shelf of the island of Hawai‘i, where the bottom steeply dropped off to deep water (>1000 m) to the south (Figure 1).
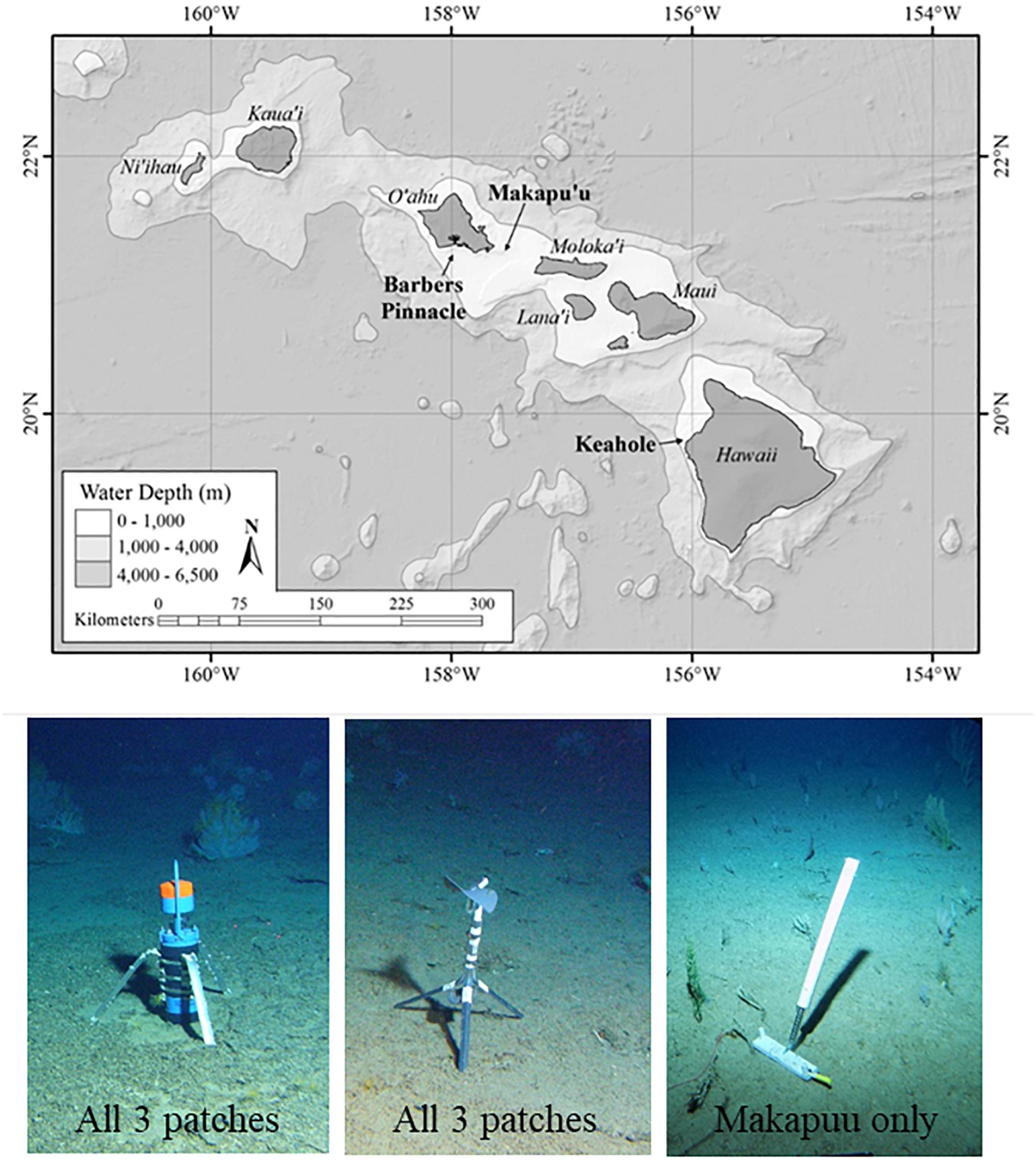
Figure 1. Map of the main Hawaiian Islands showing the locations of the three coral patches whose environment was monitored with instruments, two on the south, and south-east platform base of O‘ahu and one on the edge of the west Hawai‘i shelf. Photos indicate the types of instruments used: acoustic current meter (left), flowmeter (center), tiltmeter (right).
Environmental and Coral Colony Observations
Two approaches were used to characterize the environment at each coral patch. First, a single acoustic current meter was used to record and compare a suite of variables at the center of each patch where conditions for deep coral colonization and growth were best, and second, multiple mechanical flowmeters were used to measure the variability in flow intensity and spectra from the center of the patch out to the edge of the patch. The instruments were placed and recovered using the submersibles Pisces V and Pisces IV as well as the ROV Deep Discoverer (D2). All instruments stood 50 cm above the bottom and recorded environmental conditions hourly. The height of the instruments fell within the range in average colony height for the coral types considered; Narella spp. and Coralliidae (∼30 cm), bamboo and gold coral (∼100 cm) (Figure 1 instrument photos). The acoustic current meter at the center of each patch (Aannderaa SEAGUARD RCM) recorded current direction, flow rate, temperature, and backscatter. To look for change in flow intensity and temperature across the coral patch, at least three flowmeters (General Oceanics digital low velocity flow meter 2030R6 fitted with a data logger) were deployed out to the edge of the patch at a consistent depth. The first at the patch center, the second midway to the edge of the patch, and the third at or just beyond the edge of the patch. At Makapu‘u Pt. patch, tiltmeters (Lowell Instruments TCM-3) were added to sample across contours, starting deeper and passing over the contour with the flowmeters. Tiltmeter placement roughly followed the subtle crest in the bottom, extending to the shallow extreme of the patch. Deployment and recovery of instruments was opportunistic, largely dependent on piggybacking sub dives onto a larger research effort being conducted in the region. Particularly for the large Makapu‘u Pt. patch, multiple dives over a number of years were used to deploy and recover the gear. Consequently, the duration of sampling varied with the time the instrument was left in the water and its battery life. The acoustic current meters recorded data between 6 and 7 months for the Barbers Pt. and Makapu‘u Pt. patches. Ship time was unavailable to recover the Keahole Pt. instruments for 5 years, so the instrument recorded for 33 months until it ran out of battery (Table 1). In addition to the focal taxa, all sessile taxa observed within a 5-m radius of each instrument were counted and identified to the lowest level. The size and directional orientation of the colonies were noted for the focal taxa.
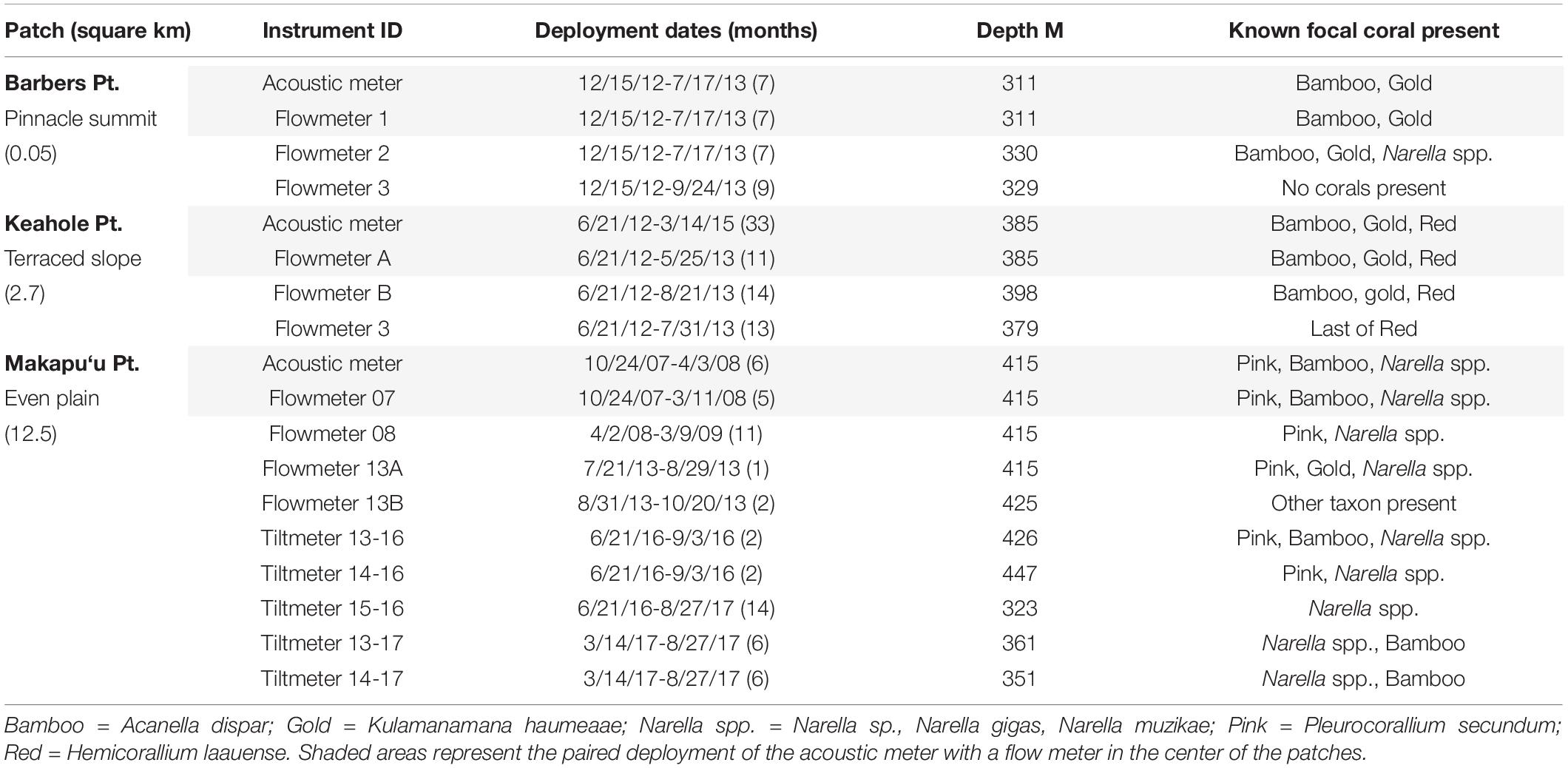
Table 1. List of instruments types and deployment dates in the three coral patches indicating the area, depth, and the focal corals present.
Analysis
Plots of the data (current direction, flow intensity, temporal spectra, and backscatter) from the acoustic current meters at the center of the patches were compared. Only instruments that were deployed for more than a year could be evaluated for seasonal effects of primary flow direction and intensity of flow. The 15 mechanical instruments (flow and tiltmeters) measured within-patch variability of mean flow and temporal spectra from center out to the edge of the patch. The range of means for all instruments was plotted and considered in relation to the number of colonies of the focal corals among the patches to look for patterns in temperature and flow. All statistical tests were carried out in IBM SPSS v24. Statistical significance was defined when tests returned an alpha level P < 0.05 (Siegel and Castellan, 1988).
Results
Environment Among the Three Coral Patches
Depth, temperature, and current flow varied among the three deep coral patches. Most similar in temperature were the Makapu‘u Pt. (8.4°C, 0.70 SD at 415 m) and Keahole Pt. patches (8.2°C, 0.40 SD at 385 m) whereas the shallower Barbers Pt. patch was 2° warmer (10.2°C, 1.05 SD at 311 m). The range of flow rates of the three patches grouped differently with Makapu‘u Pt. (13.6 cm/s, 7.8 SD) and Barbers Pt. (12.7, 7.4 SD) exhibiting twice the flow rate observed at the Keahole Pt. patch (4.6 cm/s, 4.0 SD) (Figure 2). The dominant current direction differed among the three patches with the peak flow at Makapu‘u Pt. alternating between north and south, Barbers Pt. alternated south and east-northeast, and the terraced slope at Keahole Pt. was subject to pulses of flow to the north (Figure 3, left column; Figure 4, top row).
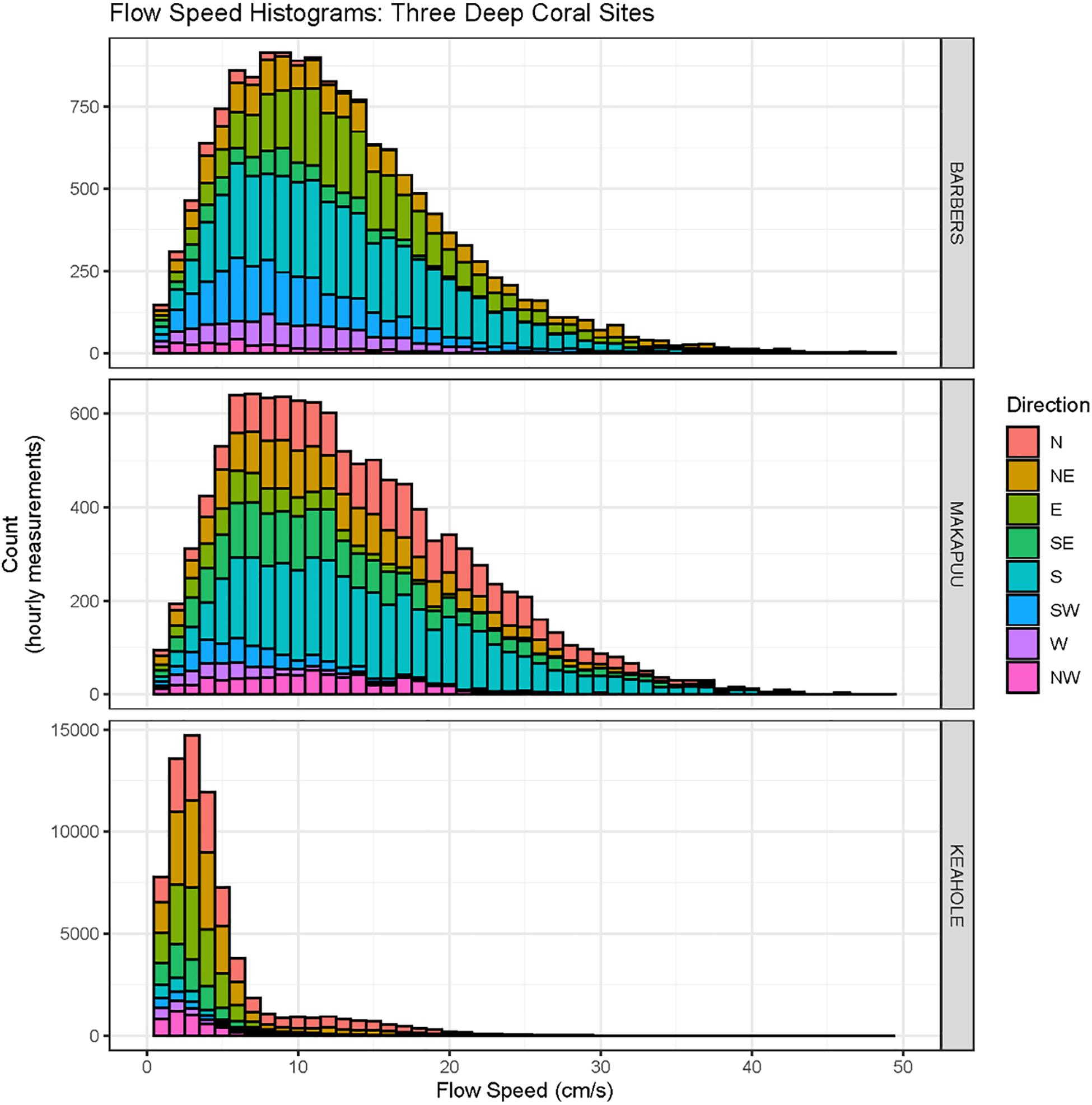
Figure 2. Flow characteristics documented by the acoustic current meter at the center of the three patches where coral density was highest. Top two panels show the distribution of flow rates by direction at the two O‘ahu sites having the strongest flow (Barbers Pt. and Makapu‘u Pt.). The bottom panel shows lower flow levels in the Keahole Pt. patch.
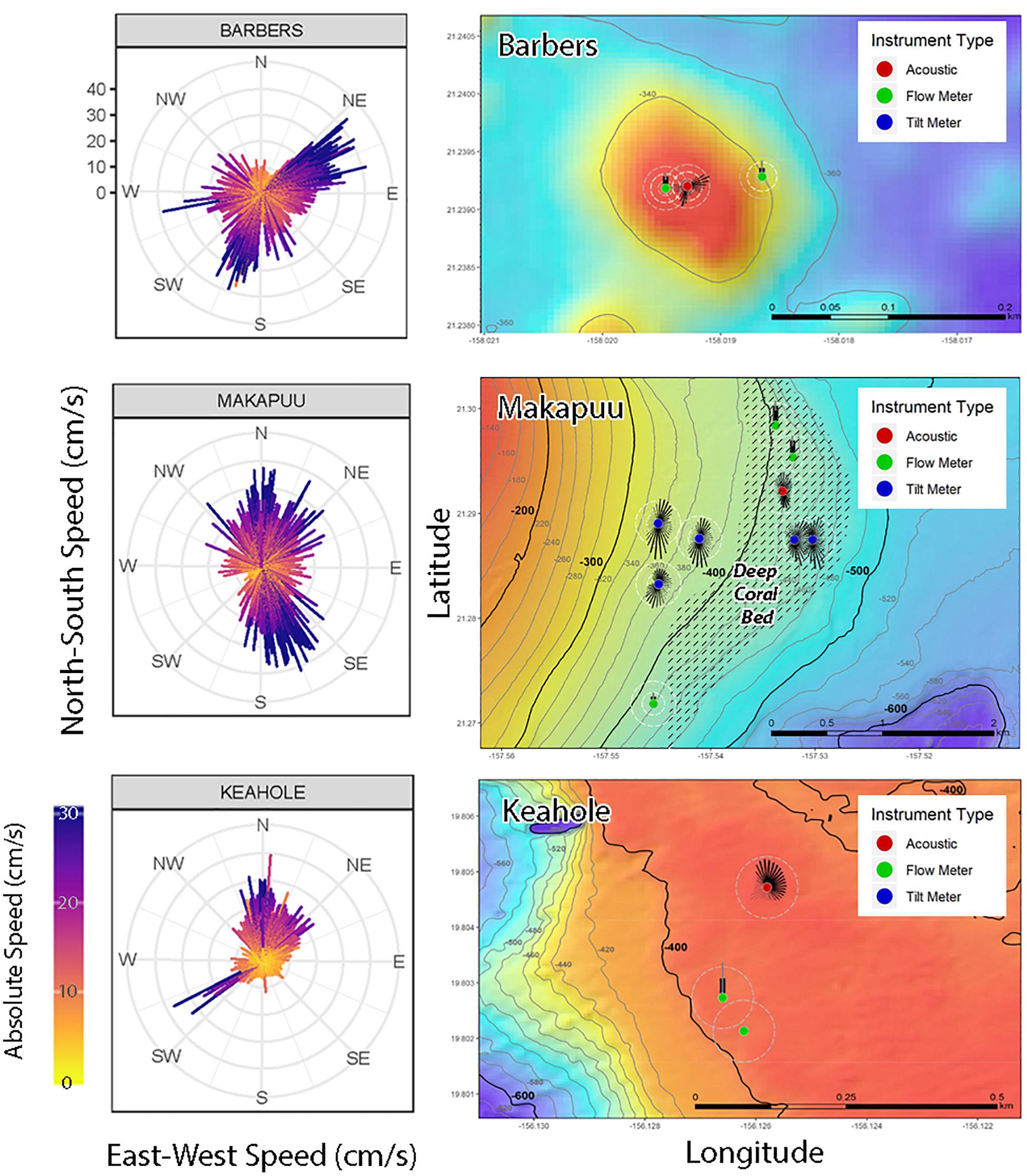
Figure 3. Plots of the dominant direction of current flow at the three coral patches. The left column shows the flow rose from the acoustic current meter placed at the center of each patch. The right column has maps of the patch topography with flow roses for all the instruments placed to show the within-patch flow gradient. Red dot is the acoustic current meter, blue dots are tiltmeters, and green dots are flowmeters. As the flowmeters only record flow intensity, a single vector representing mean intensity was set to point north, similar to the instruments that recorded direction. Dash shading on the Makapu‘u Pt. patch map indicates the area of the coralliid deep coral bed of the precious coral fishery.
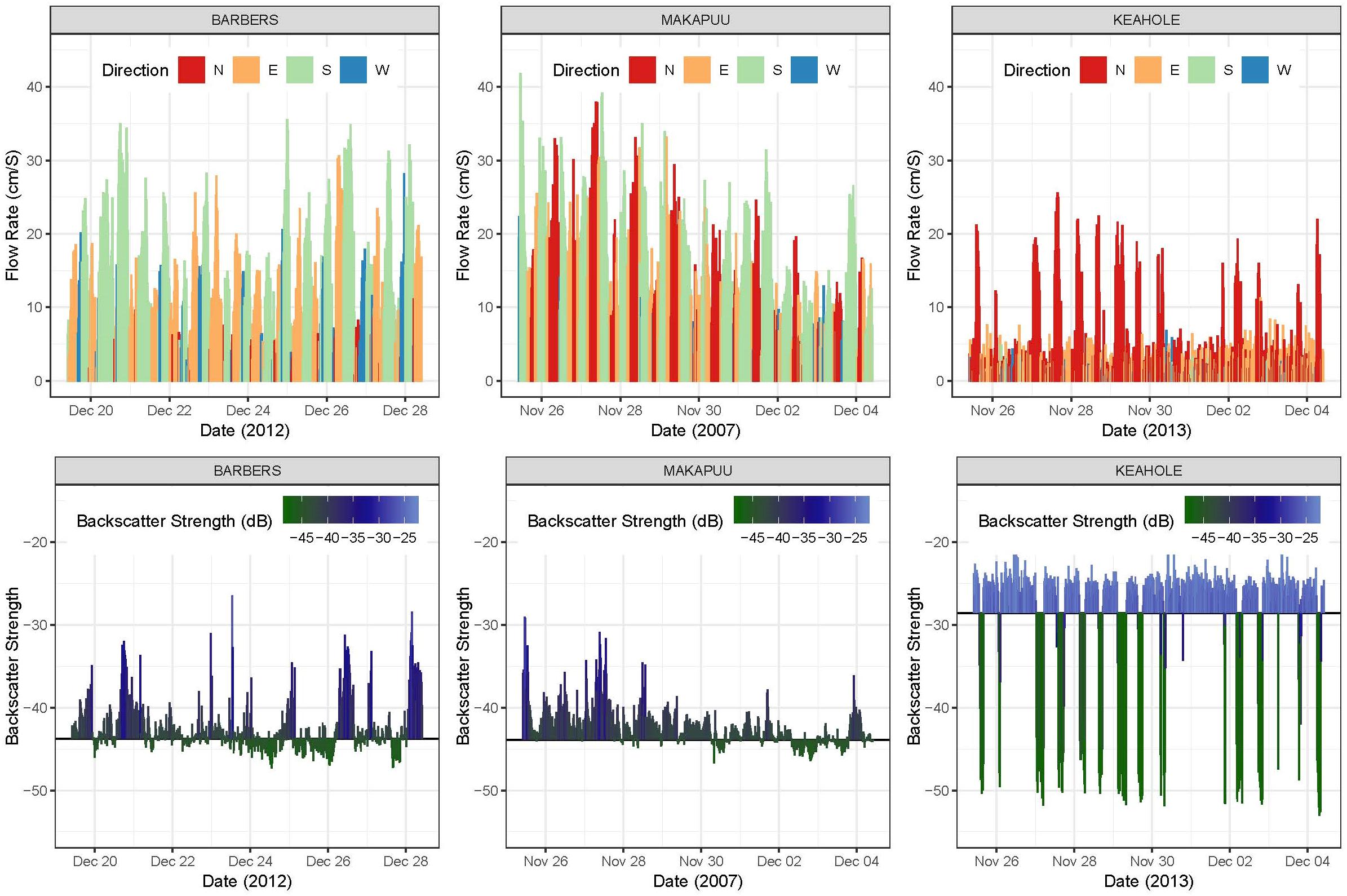
Figure 4. Representative current flow (top row) and backscatter data (bottom row) for a 10-day period showing the Keahole Pt. patch having a stronger average scattering signal than the other two coral patches. Also evident at Keahole Pt. is a drop in the scattering strength correlated to pulses of northern flow.
Comparison of acoustic backscatter data revealed regional differences in the water mass among the patches. Mean scattering values for the low flow Keahole Pt. patch was a third greater (−28.5 dB), indicating more suspended materials (e.g., zooplankton), than the Barbers Pt. and Makapu‘u Pt. patches (−43.7 to −43.9 dB). Also at Keahole Pt., there was a pronounced pattern of the tidally driven pulses of northerly flow reducing the scatter strength to a level similar to that of Barbers Pt. and Makapu‘u Pt. until the flow pulse abated (Figure 4). During these flow pulses, the temperature dropped suggesting an introduction of cooler water (r = −0.210, p < 0.001).
Similarly, patches at Makapu‘u Pt. and Barbers Pt. shared roughly the same patterns of spectral density in flow speed and direction, with the highest peak occurring in a manner suggestive of semi-diurnal tidal forcing with spring-neap patterning (speed: 4x daily, twice monthly; direction: 2x daily; Figure 5). Spectral patterns at Keahole Pt. again showed different forcing, with peaks in flow speed occurring almost exclusively at twice daily, without a spring-neap peak, and more variability in the peaks present in flow direction (Figure 5).
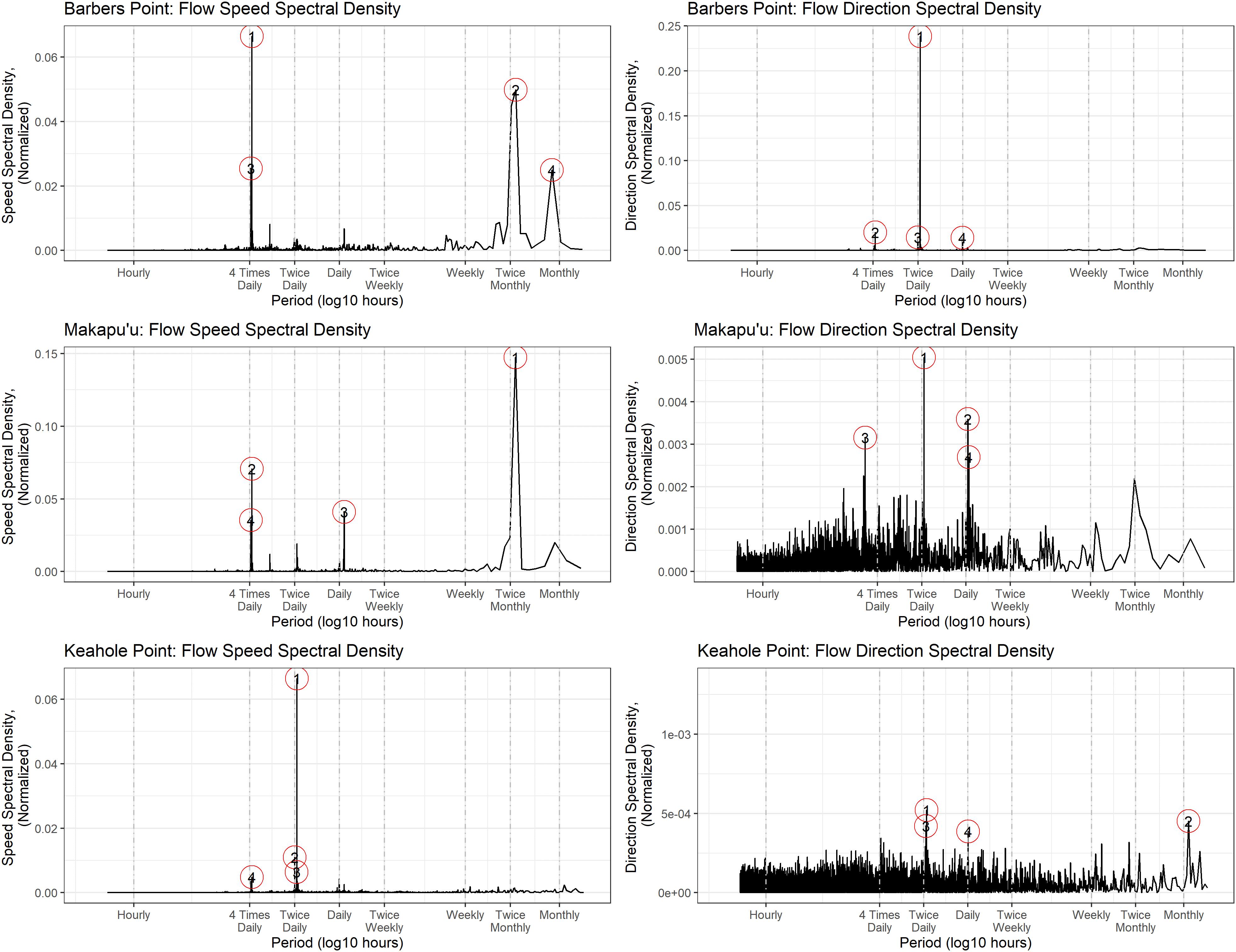
Figure 5. Left column: Temporal plots of spectral density of flow speed for the three coral patches showing peak changes just under twice daily, consistent with tidal influences. Right column: Tidal spectral effects on current direction for the three patches on a weekly scale.
Within Patch Variability
The data from the array of flow and tiltmeters (n = 15) showed the within-patch flow intensity as a gradient. There was good agreement of the three flowmeters paired with the acoustic current meters (R2 = 0.35–0.63, P < 0.001) and was best at the patch with the lowest flow rate (Keahole Pt.). The means and standard deviations of the flow for the 15 flowmeters and tiltmeters showed the Barbers Pt. patch clustered in the middle between the high flow measurements at the Makapu‘u Pt. patch and the lowest at the Keahole Pt. patch. The lowest flow rates for each patch were recorded by the flowmeters placed at the edge of the patch (Figure 6). The edge site at both Barbers Pt. (Barbers F3 instrument) and Makapu‘u Pt. (Makapu‘u F13B instrument) had flow rates (7.9–8.4 cm/s) well above the highest values seen at any of the Keahole Pt. sites (0.54–4.9 cm/s) where the scattering strength in the passing water masses was strongest. It is not known how the current direction shifts at different points across the patch except for the data from the Makapu‘u Pt. tiltmeters. They showed some change in directional emphasis across the wide area of the patch, but the peak flow events were still largely consistent with the north-south pattern reported by the acoustic current meter at the center of the patch (Figure 3 right column). Pooling the directional data (tiltmeter and acoustic current meter) from all three patches (n = 8 instruments), a partial correlation controlling for instrument indicated variability in current direction was positively correlated with flow rate (r = 0.556, p < 0.001).
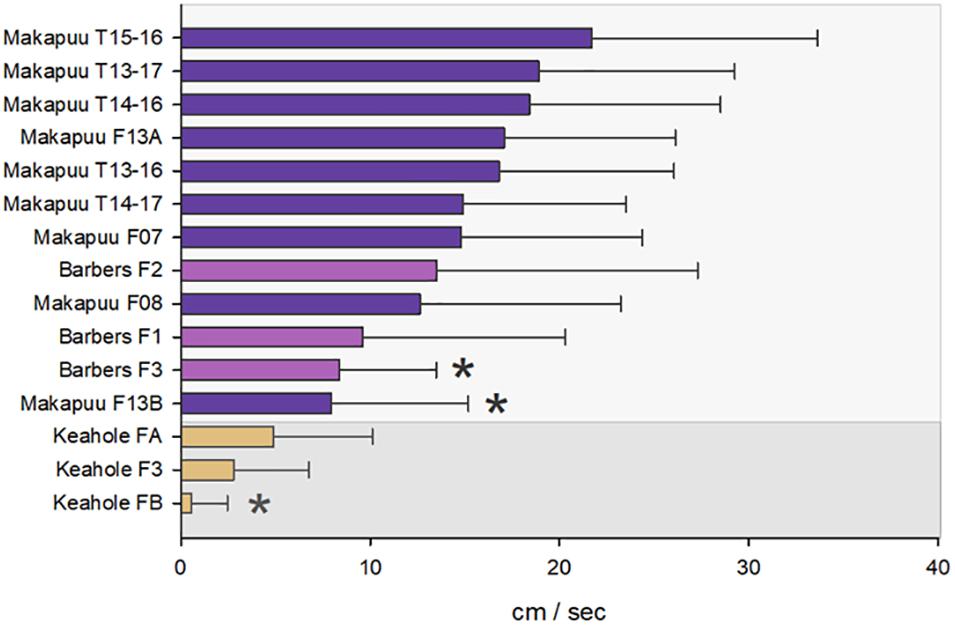
Figure 6. The mean and standard deviation of the 15 flow and tiltmeters placed in the three patches with Makapu‘u Pt. values clustered at the top, Barbers Pt. in the middle, and Keahole Pt. at the bottom. The asterisks indicate an instrument at the edge of the coral patch. The darker shaded background represents the greater scatter strength (−24 dB) of the Keahole Pt. water mass over the other sites (−42 dB).
The observed patterns in flow persisted among the instruments at a patch even though the length of their deployments varied (1–14 months). Two of the coral patches (Keahole Pt. and Makapu‘u Pt.) had instruments that monitored direction and flow rate year-round and the variation seen in the monthly means remained proportional to the overall flow rate of the patch. At the low flow site of Keahole Pt., the range of variability was narrow with flow speed changing 1.36 cm/s peaking in the winter months (ANOVA df = 3 F = 52.5 p < 0.001) with no significant change in flow direction (4.9°). The highest flow site, Makapu‘u Pt., had greater range of flow 8.72 cm/s (fall months being the weakest) and a wider span of 20.8° for changing flow direction (in summer) (ANOVA df = 3 F = 20.4 p < 0.001). These changes (likely seasonal) occur at a scale moderate enough that monitoring a site for a single month can still effectively represent the key differences in the site-specific flow intensity and tidal cycle.
Taxon-Specific Observations
A total of 19 sessile taxa were identified near the 15 flow instruments (Table 2) and not surprisingly, the diversity increased with the size of the coral patch. The taxa were mostly gorgonian corals but did include a stony coral (Equchipsammia sp.) and sea pens (Halipteris sp.). Narella spp. were the most abundant of the corals seen near instruments, closely followed by colonies of pink coral, P. secundum. Both corals colonized areas at the high end of the flow spectrum, primarily at Makapu‘u Pt. Bamboo coral (A. dispar) and gold coral (K. haumeaae) were present at all three patches growing in the widest range of flow rates whereas the red coral, H. laauense, was at the low end of the flow range and exclusively observed at Keahole Pt.
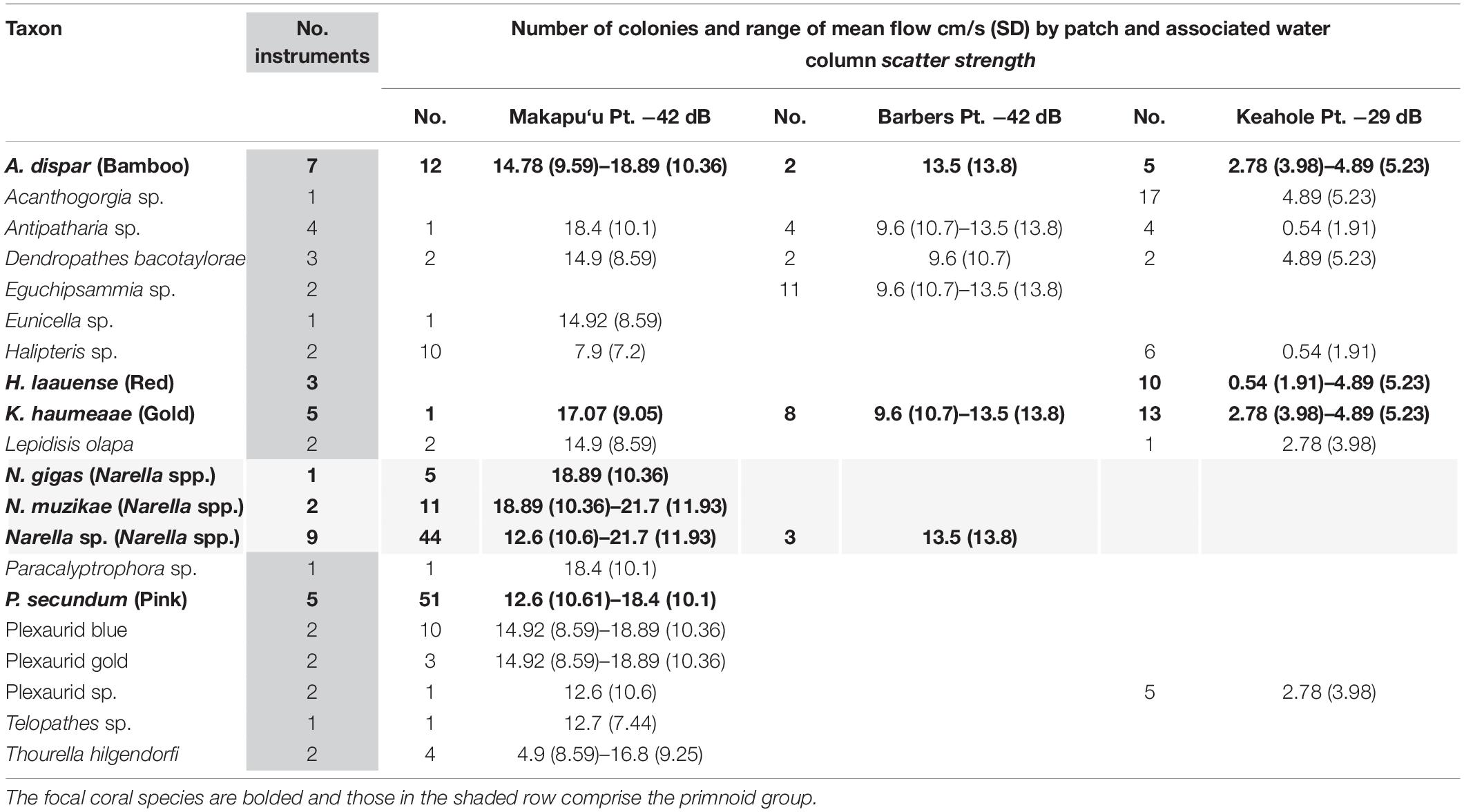
Table 2. Breakdown of all sessile taxa seen within a 5-m radius of placed instruments including number of instruments, number of colonies, and the range of mean flow measured at each of the three patches with the scattering strength indicated in the column labels.
Plotting the mean and standard deviation for both flow and temperature from the individual instruments showed the two coralliids occupied opposite extremes in the range of observed flow with little overlap, even though they showed complete overlap in temperature, occurring along the cooler side of the temperature gradient (Figure 7 top). Colony orientation also differed with all the Makapu‘u Pt. pink coral colonies facing the same direction to exploit the dominant north to south tidal flow while the Keahole Pt. red corals had a more haphazard orientation.
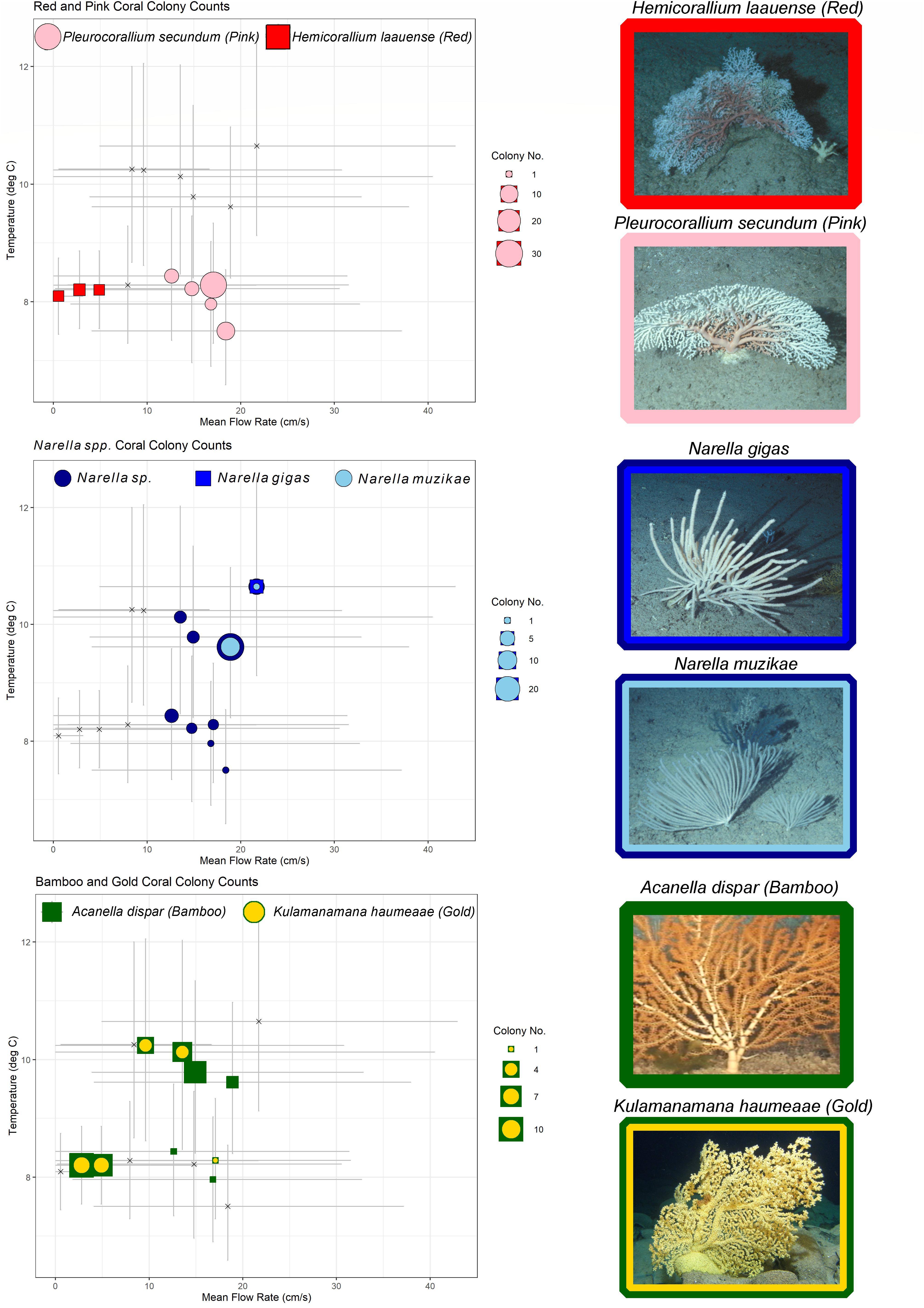
Figure 7. Mean and standard deviation of temperature and flow for the flow and tiltmeter instruments by focal coral taxon. Top row shows red and pink colonies at the same temperature but different flow rates. Middle row shows Narella spp. colonies at all temperatures but clustered at the high extreme of flow. Bottom row shows bamboo colonies at all temperatures and flows and the parasitic gold coral colonizing the bamboo host colonies most at lower flow sites. Photos courtesy of Hawaii Undersea Research Laboratory.
The density of Narella spp. colonies was greatest at the shallowest end of Makapu‘u Pt. patch and thinned as it extended deeper, overlapping with the area of pink coral colonies below 361 m. In this area, the flow was strong (12.6–18.9 cm/s) and continued to increase as depth decreased to a high of 21.7 cm/s at 323 m where Narella gigas and Narella muzikae appeared (Figure 7 middle). Across this depth range, the temperature increased from 8.4 to 10.6°C, and based on one tiltmeter observation, may have been a limiting factor that prevented the edge of pink coral patch from spreading shallower. The instrument placed at 351 m documented higher temperature (9.7°C) and the same mean flow rate (14.9 cm/s) as at the center of the pink coral area (415 m). However, there were no pink coral colonies and the number of Narella spp. colonies were few (n = 3) compared to the adjacent instrument sites with higher flow (12–35 colonies), suggesting that the site at 351 m may have been too warm for pink corals, and the flow rate too slow for Narella spp. patch growth.
Only the bamboo and gold coral colonies were present at all three patches. Gold coral is a parasite that is known worldwide to colonize and subsume isidids, sea fans (Druffel et al., 1995; Roark et al., 2006), and black coral (Pers. Comm. Di Tracey 3/2020). Because in Hawai‘i, the bamboo coral (A. dispar) is a primary host (Parrish, 2015), both gold and bamboo were plotted together in the bottom panel of Figure 7. In the plot, the gold colonies are over printed on the bamboo colonies to represent temperature and flow conditions that were suitable for both the colonization by the bamboo host and the subsequent colonization by the parasitic gold coral. There was a wide spread in both temperature and flow for the bamboo coral host. Gold coral was seen to colonize bamboo coral at all temperatures but primarily at locations with lower flow rates. This observation is supported by the number of gold coral colonies observed in the patches being negatively correlated with flow (r = −0.960, p < 0.005) even though no similar relationship was seen for the bamboo host (r = −0.014, p = 0.488).
Discussion
Regional Effects
Location plays an important role in comparison of flow among the three coral patches. The Makapu‘u Pt. and Barbers Pt. patches are both more exposed to flow moving between and around the islands as the trade winds push the ocean from east to west at this latitude. Makapu‘u Pt., located in the channel between O‘ahu and Moloka‘i, feels the flow moving to the south as it moves around O‘ahu. Barbers Pt. is further in the lee of O‘ahu about equidistant between the Kauai channel and the Moloka‘i channel so may be influenced by multiple flow directions. In contrast, the Keahole Pt. patch is in the protected lee of the 90-mile coastline of Hawai‘i Island and its two 4-km-tall volcanos that create a place of low flow and deep water upwelling (Calil et al., 2008). The bathymetry of each site was seen to shape the flow and the growth of the associated benthic community, as was reported in prior findings (Genin et al., 1986; Etnoyer et al., 2010; Tracey et al., 2011). The uniform hardpan bottom at Makapu‘u Pt. caused the flow to peak at its subtle cross-contour ridge. The flow at Barbers Pt. pinnacle changed depending on the summit’s exposure to the prevailing current, providing sides with high flow supporting corals and sides of low flow without corals. Finally, Keahole Pt. patch was exposed to consistent low flow with periodic pulses of northern flow from the adjacent deep water source to the south.
The temperature data from the present work confirm results from prior temperature monitoring in Hawaiian deep coral patches, suggesting that the environment remains stable through time. Specifically, the data at the Makapu‘u Pt. coral patch were consistent with years of prior monitoring (2001–2008) at the site (Parrish and Roark, 2009). The same monitoring study identified regional differences in other Hawaiian deep-sea coral patches (French Frigate Shoals 352 m: 10.9°C ± 0.66, Cross Seamount 392 m: 8.74°C ± 0.50), but showed the within-site inter-annual patterns (5 or more years) were consistent. In the present study, the longest monitoring was 2.75 years at the Keahole Pt. patch and temporal patterns for both temperature and flow persisted through successive years. For the flow instruments that monitored a complete year, some limited seasonal change was seen but not to a degree that would obscure the signal of the between-patch differences.
Most of the observed temporal change was tidal in nature, with some distinctions between sites in domination of a semi-diurnal vs. diurnal pattern (i.e., Barbers/Makapu‘u Pts. vs. Keahole Pt.). Tide has been reported as the primary driver of enhanced seafloor flow (Matsuyama et al., 1993; Musgrave et al., 2016) with semidiurnal (M2 period) tides documented as the dominant signal across the Hawaiian Ridge (Merrifield et al., 2001; Merrifield and Holloway, 2002; Rudnick et al., 2003). Some variability in the relative extent of tidal influence was apparent among the coral patches (Figure 5).
Patch Formation
This study looks at the environmental conditions for a deep-sea coral patch without information about the role of larval supply and settlement success. The corals’ reproductive life history (e.g., localized recruitment or long planktonic dispersal) and the distance from the source colonies are likely to be important factors in the formation of a coral patch (Richmond, 1988; Mercier and Hamel, 2011). However, given the longevity of deep-sea corals (decades to millennia; Prouty et al., 2017) it seems unlikely there would be premium areas with excellent environmental conditions for deep-seal corals that have yet to be colonized unless the site is out of reach of the drifting planula. The norm is more likely to be an abundance of habitat marginally suitable for colonization by deep-sea coral that is yet undiscovered. We know sites with higher flow tend to host more coral density and diversity, but it is also possible there are sites where flow rates are too high for colonization. In Table 2, we reported flow rates for all taxa encountered, but many (e.g., Lepidisis olapa, plexaurids, Antipatharia) did not occur in numbers sufficient to be considered a patch, even though patches of these corals have been encountered elsewhere by the lead author. Other species were seen as a patch at the Makapu‘u Pt. site (e.g., Acanthogorgia sp., Halipteris sp.), but overlapped with too few instruments to make comparisons. Typically, these other taxa comprise a sparse mixed assemblage dispersed throughout the patches of the focal corals we chose to study. Generally, a patch tends to have a dominant coral species. Surveys of six coral patches throughout the Hawaiian Archipelago found that two were mostly pink coral, three mostly red coral, and one mostly gold coral (Parrish, 2007). Seems likely that when the habitat conditions are ideal for a particular taxon, years of sustained growth spurs successive continued recruitment until the assemblage becomes increasingly monotypic.
At the colony level, if the depth, temperature, and planktonic load of the water are suitable, then any observed differences among sites are likely due to the localized flow dynamics associated with the bottom topography of the area. For deep-sea corals to thrive, the conditions must achieve balance between having flow rates fast enough to deliver suspended food for viable coral growth, yet slow enough so as not to prevent settlement or exert drag that exceeds the strength of the colony’s holdfast. Increased drag on a colony will influence the height to which the colony can grow, reproduce, and form a patch at that site. As we compared the flow around the coral colonies, we also considered how flow and the associated drag matches their skeletal morphology and life history.
Narella spp.
Our observations of high numbers of Narella spp. at Makapu‘u Pt. match findings by Long and Baco (2013) who identified depth-related patterns in the coral assemblage and the dominance of primnoids at the site. The range of observed mean flow for Narella spp. was narrow (12–21.7 cm/s) despite pooling the observations for the two known species, N. gigas and N. muzikae, and the undescribed species. The patches of Narella spp. and the pink coral colonies overlapped like a Venn diagram. As depth increased, flow rates diminished and the number of Narella spp. dropped to sparse levels (2–4 colonies), but colonies did persist down to the center of the deeper pink coral patch. This trend suggests that the presence of Narella spp. is more affected by flow than depth or temperature. Standing roughly 40 cm in height, the morphology of the Narella spp. colonies is either whirl or fan-like in shape with pliable branches of fleshy polyps (Figure 7 photos). This morphology is well suited to bend and accommodate the prevailing north-south alternating flow at Makapu‘u Pt. The Barbers Pt. patch, tiny compared to the Makapu‘u Pt. patch, had sites comparable in depth, temperature, and flow rate, and did have a few Narella spp. colonies, whereas none were seen at the lower flow Keahole Pt. patch. A search of the Hawai‘i Undersea Research Laboratory animal database for Narella spp. identified none documented for the 66 annotated submersible dives at the Keahole Pt. site. There is no age and growth work for Narella spp. in Hawai‘i, but other primnoids in the NE Pacific are reported to live between a hundred and a thousand years and are reliant on surface particulate organic matter (Andrews et al., 2002; Prouty et al., 2017).
Coralliids (Pink and Red Corals)
The patch dominance of the two coralliids, pink at Makapu‘u Pt., and red at Keahole Pt., appear to be related to very different flow preferences even though the colonies are similar in size (Grigg, 1988), have crustose fan morphologies, have similar reproductive strategies (Grigg, 1993; Waller and Baco, 2007), and were observed at the same temperature. Conditions at Makapu‘u Pt. appear to be premium for pink corals with recruitment and mortality in steady state, leaving other areas recruitment limited (Grigg, 1993). The pink colonies have robust stems and thick branches all oriented in the same northern direction, suggesting that at this flow intensity the tidal spectra is important to this taxon. Colonies of pink corals have been identified in small numbers at Keahole Pt., Cross Seamount, and one sizable “refuge” patch between Nihoa and Necker Island in the Northwestern Hawaiian Islands (Grigg, 1993; Parrish, 2007; Parrish and Baco, 2007). Finally, the depth boundary we saw for the shallow edge of the Makapu‘u Pt. pink coral patch confirms findings from earlier survey work by Long and Baco (2013). Neither their survey nor ours encountered red coral colonies at Makapu‘u Pt., but prior sample collection for genetic studies have verified its presence at the site (Baco and Shank, 2005). It is possible our surveys overlooked them or did not extend deep enough to reach the suitable flow range to encounter them.
The low flow rates seen at Keahole Pt. may be the preferred conditions for red coral. Baco and Shank (2005) were able to collect red coral to describe the genetic population structure across six sites in the lower Hawaiian archipelago (including Makapu‘u Pt.), but did not find it at the “refuge” patch where pink coral was the dominant taxon. Red coral skeletal morphology is frail with a thinner base and delicate branches that easily break when handled, so this coral may do poorly in sites of extreme flow where forces of drag would topple the colony before it could attain maturity. It is also possible that slower flow rates are optimal feeding conditions for this coral, as has been shown by feeding experiments on other suspension feeding corals (Purser et al., 2010; Orejas et al., 2016). Unlike the consistent orientation of pink corals at Makapu‘u Pt., the colonies of red coral were observed to grow in a haphazard orientation at multiple sites across the archipelago (Parrish, 2007) suggesting that red coral is suited to exploit low-flow water conditions. The distribution of polyps does differ between the two taxa: red coral polyps are distributed evenly along the branches, whereas pink coral polyps are clustered at the ends of the branch tips (Bayer, 1956).
Bamboo and Gold Corals
Bamboo coral and the parasitic gold coral are the most adaptable of the focal corals in this study. They were present at all three patches and spanned the widest range of temperature, flow, tidal spectra, and water mass productivity. Both corals grow to more than three times the height of the other focus corals, allowing them to exploit more of the passing water mass (Parrish and Baco, 2007), and are reported to rely on surface particulate organic material (Roark et al., 2006; Prouty et al., 2017) which may contribute to their adaptability and slow growth. As such, the intensity and spectra of the flow seem less important to these two corals.
The bamboo host colony, which can take a century to attain its full size (Roark et al., 2005), exhibited no obvious patterns in relation to flow rate or temperature. Its common name refers to its hybrid morphology; a planar frame composed of carbonate segments linked by protein nodes that provides more rigidity so it can extend and exploit suspended materials higher in the water column, but still more flexible, allowing it to bend as needed to accommodate periods of intense flow without breaking.
In contrast, more colonies of gold coral were found growing in lower flow environments. An established bamboo coral colony is the preferred host for gold coral (Parrish, 2015), which means the minimum flow thresholds of bamboo and gold corals are the same. The cluster of gold corals at lower flow speeds is likely an artifact of the zoanthid being a larger fleshy polyp that builds a stiff continuous protein frame (Sinniger et al., 2013) that as it grows needs to withstand the risk of toppling with increasing drag from the current. A gold coral can take millennia to reach full size (Parrish and Roark, 2009; Roark et al., 2009) so, in high flow environments, the coral is likely to topple and wash away early on at a smaller size. At sites with low flow, the gold colony can attain full size (∼2 m) and if it does eventually topple from drag, its large size and weight will anchor it so that it will either adapt and begin to grow upward, perpendicularly from the seafloor, or if its new prone position makes flow inadequate, the skeleton will take hundreds of years to decay away (Parrish, 2015).
This illustrates the important role flow plays in the growth, reproduction, and subsequent recruitment that makes up the community of a coral patch. It could be that high flow environments are a place where the bamboo coral population can grow with some refuge from colonization by the parasitic gold coral, which once settled, subsumes the host rapidly as part of the “midas” phase of its life history (Parrish, 2015). Patches of bamboo colonies, free of gold, have been seen relatively close to sites that are homogeneous patches of gold corals. It is likely that sites dominated by gold coral are the result of lower flow that (1) allows them to persist and grow to full size and (2) retains the planula, enhancing localized recruitment of available host colonies within the patch (Sammarco and Andrews, 1988; Todd, 1998). In a high flow patch, the full-size mature gold colonies are limited by the intense flow rate, and if reproductively viable colonies do grow, the planula are at risk of being blown out of the patch before a suitable settlement site can be found (Kinlan and Gaines, 2003; Hilario et al., 2015).
Flow and Scatter Strength of Water Mass
The organic component suspended in a passing water mass influences the necessary flow rate to sustain suspension feeding corals (Emery, 2001). This is best illustrated by patterns in bamboo and gold corals because they occurred at all three patches but were absent from each patches’ edge site. At the edge sites of Barbers Pt. (Figure 6. instrument F3 = 8.3 cm/s) and Makapu‘u Pt. (instrument F13B = 7.9 cm/s), the flow rates were much higher than all the sites at Keahole Pt. (0.54–4.89 cm/s), including those that had bamboo and gold colonies. However, the 1/3 greater backscatter strength at Keahole Pt. (−29 vs. −42 dB) indicates that more food is carried by passing water and able to sustain coral colonies at a lower delivery rate. If a high scatter strength were present at the edge sites of the Barbers Pt. and Makapu‘u Pt. patches, it would likely make those flow rates viable for coral growth and the edge of the coral patches would still be expanding.
The reason for the higher backscatter at Keahole Pt. is unclear, but it is the only patch with a backscatter pattern that suggests it receives a tidal injection of nutrient rich biogenic water. Similar tidal patterns have been documented in other deep-sea coral studies (Hanz et al., 2019) and would explain the correlation between the tidal pulse of the northern current and the temporary reduction in scatter strength. The nutrient rich water comes from the south, where the water column is more than 1000 m deep and is likely part of the documented cyclonic eddy that pumps upwelling in the lee of Hawai‘i Island (Bienfang and Szyper, 1981; Calil et al., 2008; Jia et al., 2011). Daily monitoring at a depth of 674 m (∼10 km from the Keahole Pt. coral patch) shows dissolved nitrates and phosphates seven to eight times greater than that found in the mixed layer (NELHA National Energy Laboratory Hawaii Authority, 2015). As this water is forced shallower, it enhances the production to the bathypelagic and slope benthic communities (Bidigare et al., 2003). The inter-mixing of the deep chlorophyll maximum with the rising inorganic nutrients creates recurring localized patches of biologically enhanced seascape (Seki et al., 2002). The greater observed backscatter at Keahole Pt. patch indicates more zooplankton (Iida et al., 1996) and thus more food for corals (Orejas et al., 2003; Carlier et al., 2009; Naumann et al., 2011). With this, the corals at the Keahole Pt. patch can thrive at a flow rate that is insufficient for the Makapu‘u Pt. and Barbers Pt. patches.
It is possible that conditions of high productivity water and low flow are ideal to form full patches of red coral. Its delicate skeletal structure seems adapted to a low flow that would require a productive water mass. Recent work surveying the deep-sea coral communities of the west Hawai‘i slope south of the Keahole Pt. patch has identified numerous patches of red coral as an early colonizing taxon with densities varying with the age of the lava flows they colonize (Putts et al., 2019). Red corals are also found on Cross Seamount (∼100 nm) to the east (Grigg, 2002) in the drift path of eddies that form in the island’s lee (Seki et al., 2001; Yoshida et al., 2010). Other red coral patches, some sizable, have been identified in the Northwestern Hawaiian Islands (Grigg, 2002; Parrish, 2007; Waller and Baco, 2007) where ocean productivity increases with latitude. The largest red coral patches have been reported from the archipelago’s northernmost extreme where they were commercially dredged by foreign fishing fleets in the 1970s (Grigg, 1993). Recent work visually surveying the impacted sites after decades of protection has documented some recovery of these coral patches (Baco et al., 2019). More environmental observations are needed from other coral patches to build on the preliminary findings seen in this work.
Coral Community Effects
It is not known to what degree colonization by more and different coral species changes the flow permeability of the patch canopy (Rubol et al., 2018) and influences how the community develops. This friction factor should be especially important for cold-water coral species elsewhere in the world that form intertwined reef frameworks (Davis et al., 2017). For the solitary individual colonies addressed in this study, any drag effects on measured flow are likely secondary to bathymetric influences. However, modifications to the flow field from increasing density and diversity of solitary colonies may contribute to long term changes in the settlement, growth, and succession of the patch. Seben et al. (1997) showed that with greater number and spacing of branches in coral colonies flow is reshaped and changes the success of particle capture. As of yet, the only attempt to look at these types of cumulative effects for corals have been experimental efforts measuring flow change in constructed patches using colony skeletons in laboratory flumes (Mienis et al., 2019).
There are also other influences that might shape the coral community. Field surveys have documented greater biodiversity of fish, crustaceans, and invertebrates in more developed coral communities that provide a greater range of structure (Baco, 2007; Parrish and Baco, 2007; Buhl-Mortensen et al., 2010). Crinoids have been seen covering colonies of pink corals at the Makapu‘u Pt. site (Parrish and Baco, 2007), likely increasing effects of drag on colony growth. Colony damage can also occur from swimming predators that strike the colony while hunting for prey sheltering in the coral branches (Parrish et al., 2002; Putts et al., 2019). In addition, a number of coral associated species are coral predators. For example, cidarid urchins and sea stars graze on the tissue of bamboo coral colonies leaving bare areas of skeleton (Mah et al., 2010). This can be severe, denuding the colony so it is lost, or partial predation that predisposes a coral to being colonized by the parasitic gold coral and introduces a new colony morphology to the spot. Future efforts examining the relationship between flow and deep-sea coral may also want to consider interactions with other species in the community that could influence coral colonization and succession.
Flow vs. Temperature Dependence
While much about the distribution of deep-sea coral remains elusive, here we show that the patterns of both flow speed and food availability meaningfully impact the distribution of the corals we observed. In each coral cluster, we examine (Figure 7)—red vs. pink, bamboo, and gold, and the Narella spp. cluster—depth and temperature apparently play a less significant role in structuring habitat than does flow and food availability. Further study with an increasingly comprehensive set of environmental parameters will build toward a more comprehensive description of suitable habitat, but based on the results presented here, habitat modeling exercises should not ignore the important impacts of flow on the distribution of deep-sea corals.
Data Availability Statement
The datasets generated for this study are available on request to the corresponding author.
Author Contributions
FP designed the study, obtained the financial support, conducted the field work, assembled the database, assisted with analysis and graphics, and wrote the first draft of the manuscript. TO led the data analysis, prepared the figures, contributed to the writing and revision of the manuscript text for submission, and filed all the associated metadata, database, and code in a publicly accessible repository.
Funding
This work was supported with opportunistic funding from the Hawai‘i Undersea Research Laboratory (HURL), the Deep-Sea Coral Research and Technology Program (DSCRTP), and the Office of Ocean Exploration and Research (OER).
Conflict of Interest
The authors declare that the research was conducted in the absence of any commercial or financial relationships that could be construed as a potential conflict of interest.
Acknowledgments
We are indebted to the pilots and support staff of the submersibles Pisces IV, Pisces V, and the Deep Discover ROV who were committed to placing and recovering the instruments needed to describe the flow-scape of these three deep-sea coral patches. Helpful support and reviews were provided by T. Acoba, M. Putts, V. Moriwake, A. Tengberg, and C. Kelley.
References
Anderson, O., Guinotte, J., Rowden, A., Clark, M., Mormede, S., Davies, A., et al. (2016a). Field validation of habitat suitability models for vulnerable marine ecosystems in the South Pacific Ocean: implications for the use of broad-scale models in fisheries management. Ocean Coast. Manag. 120, 110–126.
Anderson, O., Guinotte, J., Rowden, A., Tracey, D., Mackay, K., and Clark, M. (2016b). Habitat suitability models for predicting the occurrence of vulnerable marine ecosystem indicator taxa to inform deep-sea fisheries management in the South Pacific Ocean. Fish. Res. 211, 256–274.
Andrews, A. H., Cordes, E. E., Mahoney, M. M., Munk, K., Coale, K. H., Cailliet, G. M., et al. (2002). Age, growth and radiometric age validation of a deep-sea, habitat-forming gorgonian (Primnoa resedaeformis) from the Gulf of Alaska. Hydrobiologia 471, 101–110.
Andrews, A. H., Stone, R. P., Lundstrom, C. C., and DeVogelaere, A. P. (2009). Growth rate and age determination of bamboo corals from the northeastern Pacific Ocean using refined 210Pb dating. Mar. Ecol. Prog. Ser. 397, 173–185.
Auscavitch, S. R., Deere, M. C., Keller, A. G., Rotjan, R. D., Shank, T. M., and Cordes, E. E. (2020). Oceanographic drivers of deep-sea coral species distribution and community assembly on seamounts, islands, atolls, and reefs within the Phoenix Islands Protected Area. Front. Mar. Sci. 7:42. doi: 10.3389/fmars.2020.00042
Baco, A. R. (2007). Exploration for deep-sea corals on North Pacific Seamounts and Islands. Oceanography 20, 108–117.
Baco, A. R., Roark, E. B., and Morgan, N. B. (2019). Amid fields of rubble, scars, and lost gear, signs of recovery observed on seamounts on 30- to 40-year time scales. Sci. Adv. 5;eaaw4513. doi: 10.1126/sciadv.aaw4513
Baco, A. R., and Shank, T. (2005). “Population genetic structure of the Hawaiian precious coral Corallium lauuense (Octocorallia: Coralliidae) using microsatellites,” in Cold-Water Corals Coral and Ecosystems, eds A. Freiwald, and J. M. Roberts (Berlin: Springer-Verlag), 663–678.
Bauer, L., Poti, M., Costa, B., Wagner, D., Parrish, F., Donovan, M., et al. (2016). “Benthic habitats and corals,” in Marine Biogeographic Assessment of the Main Hawaiian Islands. Bureau of Ocean Energy Management and National Oceanic and Atmospheric Administration. OCS Study BOEM 2016-035 and NOAA Tech Memorandum NOS NCCOS 214, eds B. M. Costa, and M. S. Kendall (Contributor: Center for Coastal Environmental Health and Biomolecular Research), 57–136.
Bayer, F. M. (1956). Descriptions and redescriptions of the Hawaiian octocorals collected by the U.S. fish commission steamer “albatross” (2. Gorgonacea: Scleraxonia). Pac. Sci. 10, 67–95.
Bidigare, R. R., Benitez-Nelson, C., Leonard, C. L., Quay, P. D., Parsons, M. L., Foley, D. G., et al. (2003). Influences of a cyclonic eddy on microheterotroph biomass and carbon export in the lee of Hawaii. Geophys. Res. Lett. 30:1318. doi: 10.1029/2002GL016393
Bienfang, P. K., and Szyper, J. P. (1981). Phytoplankton dynamics in the subtropical Pacific Ocean off Hawaii. Deep Sea Res. Part A Oceanogr. Res. Pap. 28, 981–1000.
Bohnenstiehl, D. R., France, S. C., Cantwell, K., and White, M. (2018). Mountains in the deep: exploration of the seamounts of the central Pacific Basin. Oceanography 31, 78–79.
Bryan, T. L., and Metaxas, A. (2007). Predicting suitable habitat for deep-water gorgonian corals on the Atlantic and Pacific continental margins of North America. Mar. Ecol. Prog. Ser. 330, 113–126.
Buhl-Mortensen, L., Vanreusel, A., Gooday, A. J., Levin, L. A., Priede, I. G., and Buhl-Mortensen, P. (2010). Biological structures as a source of habitat heterogeneity and biodiversity on the deep ocean margins. Mar. Ecol. 31, 21–50.
Bullimore, R. D., Foster, N. L., and Howell, K. L. (2013). Coral-characterized benthic assemblages of the deep Northeast Atlantic: defining “Coral Gardins” to support future habitat mapping efforts. ICES J. Mar. Sci. 70, 511–522. doi: 10.1093/icesjms/fss195
Cairns, S. D. (2018). Primnoidae (Cnidaria: Octocorallia: Calcaxonia) of the Okeanos explorer expeditions (CAPSTONE) to the central Pacific. Zootaxa 4532, 1–43. doi: 10.11646/zootaxa.4532.1.1
Calil, P. H. R., Richards, K. L., Jia, Y., and Bidigare, R. R. (2008). Eddy activity in the lee of the Hawaiian Islands. Deep Sea Res. II Top. Stud. Oceanogr. 55, 1179–1194.
Cantwell, K., Elliott, K., and Kennedy, B. R. C. (2018). Deepwater exploration of the pacific remote Islands marine national monument and central pacific basin. Oceanography 31, 74–75.
Carlier, A., Le Guilloux, E., Olu, K., Sarrazin, J., Mastrototaro, F., Taviani, M., et al. (2009). Trophic relationships in a deep Mediterranean cold-water coral bank (Santa Maria di Leuca Ionian Sea). Mar. Ecol. Prog. Ser. 397, 125–137.
Clark, M., Althaus, F., Schlacher, T., Williams, A., Bowden, D., and Rowden, A. (2016). The impacts of deep-sea fisheries on benthic communities: a review. ICES J. Mar. Sci. 73, 51–69. doi: 10.1371/journal.pone.0022588
Clark, M. R., and Koslow, J. A. (2007). Impacts of fisheries on seamounts. Seamounts Ecol. Fish. Conserv. 12, 413–441.
Davis, J. S., Guillaumont, B., Tempera, F., Vertino, A., Beuck, L., Olafsdottir, S. H., et al. (2017). A new classification scheme of European cold-water corals habitats: implications for ecosystem-based management of the deep-sea. Deep Sea Res. II Top. Stud. Oceanogr. 145, 102–109.
De Clippele, L. H., Huvenne, V. A. I., Orejas, C., Lundalv, T., Fox, A., Hennige, S. J., et al. (2018). The effect of local hydrodynamics on the spatial extent and morphology of cold-water coral habitats at Tisler Reef, Norway. Coral Reefs 37, 253–266. doi: 10.1007/s00338-017-1653-y
Dolan, M. F., Grehan, A. J., Guinan, J. C., and Brown, C. (2008). Modelling the local distribution of cold-water corals in relation to bathymetric variables: adding spatial context to deep-sea video data. Deep Sea Res. I Oceanogr. Res. Pap. 55, 1564–1579.
Druffel, E. R. M., Griffin, S., Witter, A., Nelson, E., Southon, J., Kashgarian, M., et al. (1995). Gerardia: bristlecone pine of the deep-sea? Geochim. Cosmochim. Acta 59, 5031–5036.
Etnoyer, P. J., Wood, J., and Shirley, T. C. (2010). How large is the seamount biome? Oceanography 23, 206–209.
Genin, A., Dayton, P. K., Lonsdale, P. F., and Spiess, F. N. (1986). Corals on seamount peaks provide evidence of current acceleration over deep-sea topography. Nature 322, 59–61.
Genin, A., Paull, C. K., and Dillon, W. P. (1992). Anomalous abundances of deep-sea fauna on a rocky bottom exposed to strong currents. Deep Sea Res. A Oceanogr. Res. Pap. 39, 293–302.
Georgian, S. E., Anderson, O. F., and Rowden, A. A. (2019). Ensemble habitat suitability modeling of vulnerable marine ecosystems in the seas around New Zealand. Deep Sea Res. Part 1 Oceanogr. Res. Pap. 115, 265–292.
Grigg, R. W. (1988). Recruitment limitation of a deep benthic hard-bottom octocoral population in the Hawaiian Islands. Mar. Ecol. Prog. Ser. 45, 121–126.
Grigg, R. W. (1993). Precious coral fisheries of Hawaii and the US pacific Islands. Mar. Fish. Rev. 55, 50–60.
Grigg, R. W. (2002). Precious corals in Hawaii: discovery of a new bed and revised management measures for existing beds. Mar. Fish. Rev. 64, 13–20.
Guinotte, J. M., and Davies, A. (2014). Predicted deep-sea coral habitat suitability for the U.S. west coast. PLoS One 9:e93918. doi: 10.1371/journal.pone.0093918
Hanz, U., Wienberg, C., Hebbein, D., Duineveld, G., Lavaleye, M., Juva, K., et al. (2019). Environmental factors influencing cold-water corals ecosystems in the oxygen minimum zones on the Angolan and Namibian margins. J. Biogeosci. 16, 4337–4356.
Hein, J. R., Mizell, K., Koschinsky, A., and Conrad, T. A. (2013). Deep-ocean mineral deposits as a source of critical metals for high- and green-technology applications: comparison with land-based resources. Ore Geol. Rev. 51, 1–14. doi: 10.1016/j.oregeorev.2012.12.001
Hilario, A., Metaxas, A., Gaudron, S. M., Howell, K. L., Mercier, A., Mestre, N. C., et al. (2015). Estimating dispersal distance in the deep-sea: challenges and applications to marine reserves. Front. Mar. Sci. 2:6. doi: 10.3389/fmars.2015.00006
Iida, K., Mukai, T., and Hwang, D. (1996). Relationship between acoustic backscattering strength and density of zooplankton in the sound-scattering layer ICES J. Mar. Sci. 53, 507–512.
Jia, Y., Calil, P., Chassignet, E., Metzger, E., Potemra, J., Richards, K., et al. (2011). Generation of mesoscale eddies in the lee of the Hawaiian Islands. J. Geophys. Res. Oceans 116:C11009.
Kinlan, B. P., and Gaines, S. D. (2003). Propagule dispersal in marine and terrestrial environments: a community perspective. Ecology 84, 2007–2020.
Long, D. J., and Baco, A. R. (2013). Rapid change with depth in megabenthic structure-forming communities of the Makapu‘u deep-sea coral bed. Deep Sea Res. II Top. Stud. Oceanogr. 99, 158–168.
Mah, C., Nizinski, M., and Lundsten, L. (2010). Phylogenetic revision of the Hippasterinae (Goniasteridae: Asteroidea): systematics of deep-sea corallivores including one new genus and 3 new species. Zool. J. Linn. Soc. 160, 266–301.
Matsumoto, A. K. (2007). Effects of low water temperature on growth and magnesium carbonate concentration in the cold-water gorgonian Primnoa pacifica. Bull. Mar. Sci. 81, 423–435.
Matsuyama, M., Ohta, S., Hibiya, T., and Yamada, H. (1993). Strong tidal currents observed near the bottom in the Suruga trough, central Japan. J. Oceanogr. 49, 683–696.
Mercier, A., and Hamel, J.-F. (2011). Contrasting reproductive strategies in three deep-sea octocorals from eastern Canada: priminoa resedaeformis, Keratoisis ornate, and Anthomastus grandiflorus. Coral Reefs 30, 337–350.
Merrifield, M. A., and Holloway, P. E. (2002). Model estimates of M2 internal tide energetics at the Hawaiian ridge. J. Geophys. Res. 107, 1–12.
Merrifield, M. A., Holloway, P. E., and Shaun Johnston, T. M. (2001). The generation of internal tides at the Hawaiian Ridge. Geophys. Res. Lett. 28, 559–562.
Mienis, F., Bouma, T. J., Witbaard, R., Van Oevelen, D., and Duineveld, G. C. A. (2019). Experimental assessment of the effects of coldwater coral patches on water flow. Mar. Ecol. Prog. Ser. 609, 101–117.
Musgrave, R. C., Pinkel, R., MacKinnon, J. A., Mazloff, M. R., and Young, W. R. (2016). Stratified tidal flow over a tall ridge above and below the turning latitude. J. Fluid Mech. 793, 933–957.
Naumann, M. S., Orejas, C., Wild, C., and Ferrier-Pages, C. (2011). First evidence for zooplankton feeding sustaining key physiological processes in a scleractinian cold-water coral. J. Exp. Biol. 214, 3570–3576. doi: 10.1242/jeb.061390
NELHA National Energy Laboratory Hawaii Authority, (2015). Pipeline Data Table and Graphs Appendix C-DSW40-1 NELHA Pipeline Data Table and Graphs. Available at: http://nelha.hawaii.gov/wp-content/uploads/2014/01/APPENDIX_C_Pipeline_2015.pdf (accessed November 15, 2019).
NOAA National Database for Deep-Sea Corals Sponges, (2019). NOAA Deep-Sea Coral Research and Technology Program. Available at: https://deepseacoraldata.noaa.gov/ (accessed April 3, 2020).
Orejas, C., Gili, J., and Arntz, W. (2003). Role of small-plankton communities in the diet of the two Antarctic octocorals (Primnoisis antarctica and Primnoella sp.). Mar. Ecol. Prog. Ser. 250, 105–116.
Orejas, C., Gori, A., Rad-Menendez, C., Last, K. S., Davies, A. J., Beveridge, C. M., et al. (2016). The effect of flow speed and food size on the capture efficiency and feeding behavior of the cold-water coral Lophelia pertusa. J. Exp. Mar. Biol. 481, 34–40.
Parrish, F. A. (2007). Density and habitat of three deep-sea corals in the lower Hawaiian chain. Bull. Mar. Sci. 81, 185–194.
Parrish, F. A. (2015). Settlement, colonization, and succession patterns of gold coral Kulamanamana haumeaae in Hawaiian deep coral assemblages. Mar. Ecol. Prog. Ser. 533, 135–147.
Parrish, F. A., Abernathy, K., Marshall, G. J., and Buhleier, B. M. (2002). Hawaiian monk seals (Monachus schauinslandi) foraging in deep-water coral beds. Mar. Mamm. Sci. 18, 244–258.
Parrish, F. A., and Baco, A. R. (2007). “State of deep coral ecosystems in the U.S. Pacific Islands Region: Hawaii and the U.S. Pacific Territories,” in The State of Deep Coral Ecosystems of the United States, eds S. E. Lumsden, T. F. Hourigan, A. W. Bruckner, and G. Dorr (Silver Spring, MD: NOAA Technical Memorandum), 155–194.
Parrish, F. A., Baco-Taylor, A. R., Kelley, C., Cairns, S. D., and Hourigan, T. F. (2017). Deep-Sea Coral Taxa in the Hawaiian Archipelago and other U.S. Pacific Islands: Depth and Geographical Distribution. Available at: https://deepseacoraldata.noaa.gov/ (accessed January 6, 2020).
Parrish, F. A., and Roark, E. B. (2009). Growth validation of gold coral Gerardia sp. in the Hawaiian archipelago. Mar. Ecol. Prog. Ser. 397, 163–172.
Polovina, J. J., Howell, E., Kobayashi, D. R., and Seki, M. P. (2001). The transition zone chlorophyll front. A dynamic global feature defining migration and forage habitat for marine resources. Prog. Oceanogr. 49, 299–308.
Prouty, N. G., Roark, E. B., Andrews, A. H., Robinson, L. F., Hill, T., Sherwood, O., et al. (2017). “Age, growth rates, and paleoclimate studies in deep-sea corals of the united states,” in The State of Deep-Sea Coral and Sponge Ecosystems of the United States, eds T. F. Hourigan, P. J. Etnoyer, and S. D. Cairns (Silver Spring, MD: NOAA Technical Memorandum).
Purser, A., Larsson, A. I., Thomsen, L., and van Oevelen, D. (2010). The influence of flow velocity and food concentration on Lophelia pertusa (Scleractinia) zooplankton capture rates. J. Exp. Mar. Biol. Ecol. 395, 55–62.
Putts, M. R., Parrish, F. A., Trusdell, F. A., and Kahng, S. (2019). Structure and development of Hawaiian deep-water coral communities on Mauna Loa lava flows. Mar. Ecol. Prog. Ser. 630, 69–82.
Richmond, R. H. (1988). “Competency and dispersal potential of planula larvae of a spawning versus a brooding coral,” in Proceedings of the 6th International Coral Reef Symposium Vol. 2, (Townsville), 827–831.
Roark, E. B., Guilderson, T. P., Dunbar, R. B., Fallon, S. J., Shester, G. S., and Mucciarone, D. A. (2009). Extreme longevity in proteinaceous deep-sea corals. Proc. Natl. Acad. Sci. U.S.A. 106, 5204–5208. doi: 10.1073/pnas.0810875106
Roark, E. B., Guilderson, T. P., Dunbar, R. B., and Ingram, B. L. (2006). Radiocarbon based ages and growth rates: Hawaiian deep-sea corals. Mar. Ecol. Prog. Ser. 327, 1–14.
Roark, E. B., Guilderson, T. P., Flood-Page, S., Dunbar, R. B., Ingram, B. L., Fallon, S. J., et al. (2005). Radiocarbon-based ages and growth rates of bamboo corals from the Gulf of Alaska. Geophys. Res. Lett. 32:L04606.
Roberts, C. M. (2002). Deep impact: the rising toll of fishing in the deep-sea. Trends Ecol. Evol. 17, 242–245.
Rogers, A. D., Baco, A., Griffiths, H., and Hall-Spencer, J. M. (2007). “Corals on Seamounts,” in Seamounts: Ecology Fisheries and Conservation, Blackwell Fisheries and Aquatic Resources Series. Hoboken, NJ: Blackwell Scientific, 141–169.
Rooper, C. N., Wilborn, R., Goddard, P., Williams, K., Towler, R., and Holf, G. R. (2018). Validation of deep-sea coral and sponge distribution models in the Aleutian Islands, Alaska. ICES J. Mar. Sci. 75, 199–209. doi: 10.1093/icesjms/fsx087
Rowden, A., Anderson, O. F., Georgian, S. E., Bowden, D. A., Clark, M. R., Pallentin, A., et al. (2017). High-resolution habitat suitability models for the conservation and management of vulnerable marine ecosystems on the Louisville Seamount Chain, South Pacific Ocean. Front. Mar. Sci. 4:335. doi: 10.3389/fmars.2017.00335
Rubol, S., Ling, B., and Battiato, I. (2018). Universal scaling-law for flow resistance over canopies with complex morphology. Sci. Rep. 8:4430. doi: 10.1038/s41598-018-22346-1
Rudnick, D. L., Boyd, T. J., Brainard, R. E., Carter, G. S., Gary, D., Gregg, M. C., et al. (2003). From tides to mixing along the Hawaiian ridge. Science 301, 355–357. doi: 10.1126/science.1085837
Sammarco, P. W., and Andrews, J. C. (1988). Localized dispersal and recruitment in the great barrier reef corals: the Helix experiment. Science 239, 1422–1424. doi: 10.1126/science.239.4846.1422
Seben, K. P., Witting, J., and Helmuth, B. (1997). Effects of water flow and branch spacing on particle capture by the reef coral Madracis mirabilis (Duchassaing and Michelotti). J. Exp. Mar. Bio. Ecol. 211, 1–28.
Seki, M. P., Lumpkin, R., and Flament, P. (2002). Hawaii cyclonic eddies and blue marlin catches: the case study of the 1995 Hawaiian international billfish tournament. J. Oceanogr. 58, 739–745.
Seki, M. P., Polovina, J. J., Brainard, R. E., Bidigare, R. R., Leonard, C. L., and Foley, D. G. (2001). GOES thermal imagery in Hawaiian waters. Geophys. Res. Lett. 28, 1583–1586.
Siegel, S., and Castellan, N. J. (1988). Nonparametric Statistics for the Behavioral Sciences, 2nd Edn. New York, NY: McGraw-Hill Inc, 399.
Sinniger, F., Ocana, O. V., and Baco, A. R. (2013). Diversity of zoanthids (Anthozoa: Hexacorallia) on Hawaiian seamounts: description of the Hawaiian gold coral and additional zoanthids. PLoS One 8:e52607. doi: 10.1371/journal.pone.0052607
Todd, C. D. (1998). Larval supply and recruitment of benthic invertebrates: do larvae always disperse as much as we believe? Hydrobiologia 375/376, 1–21.
Tong, R., Purser, A., Unnithan, V., and Guinan, J. (2012). Multivariate statistical analysis of distribution of deep-water gorgonian corals in relation to seabed topography on the Norwegian margin. PLoS One 7:e43534. doi: 10.1371/journal.pone.0043534
Tracey, D., Rowden, A., Mackay, K., and Compton, T. (2011). Habitat-forming cold-water corals show affinity for seamounts in the New Zealand region. Mar. Ecol. Prog. Ser. 430, 1–22.
Tracey, D. M., Neil, H., Marriott, P., Andrews, A. H., Calliet, G. M., and Sanchez, J. A. (2007). Age and growth of two genera of deep-sea bamboo corals (family Isididae) in New Zealand waters. Bull. Mar. Sci. 81, 393–408.
Waller, R. G., and Baco, A. R. (2007). Reproductive morphology of three species of deepwater precious corals from the Hawaiian Archipelago; Gerardia sp., Corallium secundum, and Corallium lauuense. Bull. Mar. Sci. 81, 533–542.
Keywords: flow, backscatter, ocean environment, deep-sea coral habitat, Primnoidae, Coralliidae, Isididae, Zoanthidae
Citation: Parrish FA and Oliver TA (2020) Comparative Observations of Current Flow, Tidal Spectra, and Scattering Strength in and Around Hawaiian Deep-Sea Coral Patches. Front. Mar. Sci. 7:310. doi: 10.3389/fmars.2020.00310
Received: 08 January 2020; Accepted: 16 April 2020;
Published: 15 May 2020.
Edited by:
Juan Armando Sanchez, University of Los Andes, Colombia, ColombiaReviewed by:
Les Watling, University of Hawai‘i at Mānoa, United StatesDianne Margaret Tracey, National Institute of Water and Atmospheric Research (NIWA), New Zealand
Copyright © 2020 Parrish and Oliver. This is an open-access article distributed under the terms of the Creative Commons Attribution License (CC BY). The use, distribution or reproduction in other forums is permitted, provided the original author(s) and the copyright owner(s) are credited and that the original publication in this journal is cited, in accordance with accepted academic practice. No use, distribution or reproduction is permitted which does not comply with these terms.
*Correspondence: Frank A. Parrish, RnJhbmsuUGFycmlzaEBub2FhLmdvdg==