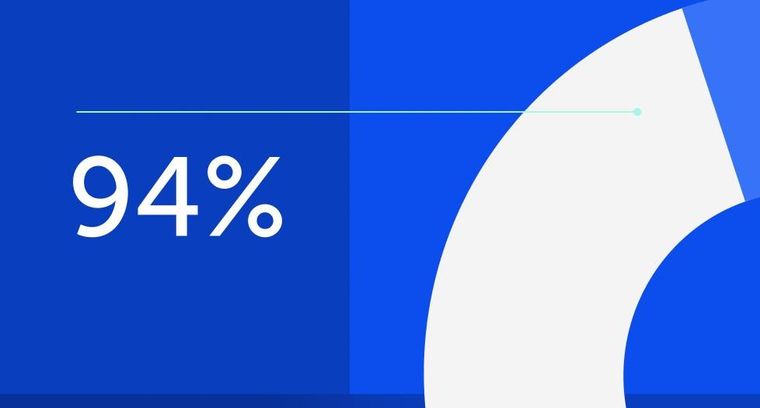
94% of researchers rate our articles as excellent or good
Learn more about the work of our research integrity team to safeguard the quality of each article we publish.
Find out more
SYSTEMATIC REVIEW article
Front. Mar. Sci., 26 May 2020
Sec. Marine Ecosystem Ecology
Volume 7 - 2020 | https://doi.org/10.3389/fmars.2020.00300
This article is part of the Research TopicSeagrasses under Times of ChangeView all 19 articles
Halophila stipulacea is a small tropical seagrass, native to the Red Sea, Persian Gulf, and the Indian Ocean. It invaded the Mediterranean Sea 150 years ago as a Lessepsian migrant, but so far has remained in insulated, small populations across this basin. Surprisingly, in 2002 it was reported in the Caribbean Sea, where within less than two decades it spread to most of the Caribbean Island nations and reaching the South American continent. Unlike its invasion of Mediterranean, in the Caribbean H. stipulacea creates large, continuous populations in many areas. Reports from the Caribbean demonstrated the invasiveness of H. stipulacea by showing that it displaces local Caribbean seagrass species. The motivation for this review comes from the necessity to unify the existing knowledge on several aspects of this species in its native and invasive habitats, identify knowledge gaps and develop a critical strategy to understand its invasive capacity and implement an effective monitoring and conservation plan to mitigate its potential spread outside its native ranges. We systematically reviewed 164 studies related to H. stipulacea to create the “Halophila stipulacea database.” This allowed us to evaluate the current biological, ecological, physiological, biochemical, and molecular knowledge of H. stipulacea in its native and invasive ranges. Here we (i) discuss the possible environmental conditions and plant mechanisms involved in its invasiveness, (ii) assess the impact of H. stipulacea on native seagrasses and ecosystem functions in the invaded regions, (iii) predict the ability of this species to invade European and transoceanic coastal waters, (iv) identify knowledge gaps that should be addressed to better understand the biology and ecology of this species both in its native and non-native habitats, which would improve our ability to predict H. stipulacea's potential to expand into new areas in the future. Considering the predicted climate change scenarios and exponential human pressures on coastal areas, we stress the need for coordinated global monitoring and mapping efforts that will record changes in H. stipulacea and its associated communities over time, across its native, invasive and prospective distributional ranges. This will require the involvement of biologists, ecologists, economists, modelers, managers, and local stakeholders.
Seagrass meadows represent one of the most valuable ecosystems on Earth, with an estimated value of $ 2.8 106 yr−1 km−2 (Costanza et al., 2014). As “ecosystem engineers,” they provide crucial ecological services, including sequestering and storing “blue” carbon from the atmosphere and oceans, nutrient cycling, sediment stabilization, and formation of essential habitats for economically important marine species (Bloomfield and Gillanders, 2005; Orth et al., 2006; Fourqurean et al., 2012). Loss of seagrasses, recorded worldwide, entails the loss of primary productivity, the devastation of associated biological communities, reduction of local fishing grounds, and increased coastal erosion (Orth et al., 2006). Runoff of nutrients and sediments that reduce water quality and light penetration, increases in water temperatures, alongside longer, and more frequent heatwaves, have been identified as major threats to seagrass meadows (Waycott et al., 2009; Marbà and Duarte, 2010; Jordà et al., 2012; Oliver et al., 2018; Savva et al., 2018). Particularly for Mediterranean and Caribbean marine ecosystems, a new threat to native seagrass species could be the ongoing expansion of the invasive seagrass H. stipulacea (Buckley and Csergo, 2017).
Halophila stipulacea (Forsskål and Niebuhr) Ascherson (order Alismatales, family Hydrocharitaceae) is a dioecious, small tropical seagrass (Figures 1, 2), native to the Red Sea, the Persian Gulf and Indian Ocean (Den, 1970; Spalding et al., 2003; Mejia et al., 2016). H. stipulacea has become an invader in two major biogeographic areas: (i) the eastern and southern Mediterranean (Lipkin, 1975a,b; Gambi et al., 2009; Sghaier et al., 2011), and (ii) the eastern Caribbean island nations (Willette and Ambrose, 2012; Vera et al., 2014; Scheibling et al., 2018; Figure 4).
Figure 1. General features of the tropical seagrass Halophila stipulacea. Shown are typical (A,B) shallow (3–6 m) and (C,D) deeper (20–50 m) meadows growing in the native habitat of the northern tip of the GoA (Eilat, Israel), where H. stipulacea grows in extensive meadows or within neighboring local coral reefs. Shown are also examples of plants growing in the invasive habitat of the Mediterranean (E,F) where H. stipulacea plants grow intermixed with native Mediterranean temperate seagrasses such as Cymodocea nodosa and Posidonia oceanica (Dream café site, Limassol, Cyprus). Photos were taken by Gidon Winters (A–C,F), Yoni Sharon (D) and Yuval Sapir (E). All photos in this figure have been obtained with permission from the original copyright holders.
Figure 2. Characteristic morphology of the tropical seagrass Halophila stipulacea. (A) Shown are rhizomes (smooth with long internodes and leaf scars at stem base), roots (covered by small hairs, could be sand-or gravel-binding), and shoots (each carrying two linear leaf blades that contain mid and branched veins). (B-D) Flowers and fruits of H. stipulacea: shown are mature male (B) and female (C) flowers, alongside seeds within a cut fruit (D). Magnification (x10) showing that leaf margin is serrated (E) and minute trichomes may be present on one side of the leaf surface (F). Photos were taken by Gidon Winters (A,E,F) and Hung Manh Nguyen (B–D). (C) and (D) were adapted from Nguyen et al., 2018. All photos in this figure have been obtained with permission from the original copyright holders.
There is a clear difference between these two invasions. In the Mediterranean, many invasion sites were recorded over the last 150 years (Figure 4A), but the areas occupied by H. stipulacea in each site, have remained small and highly restricted. In contrast, in the Caribbean, the invader has occupied vast areas and has done so rapidly (Figure 4B).
Studies from the Caribbean have demonstrated the invasiveness of H. stipulacea by showing that it physically displaces native Caribbean seagrass species (e.g., Syringodium filiforme, Halophila decipiens, and Halodule wrightii), entailing changes in the Caribbean's seagrass landscapes.
Like many non-native species that have become highly invasive in the Mediterranean over the past decades (Rilov and Galil, 2009; Zenetos et al., 2012), there is a possibility that the non-native H. stipulacea might become increasingly invasive in the Mediterranean. There are initial indications of this already occurring in some sites within the Mediterranean, where the native Cymodocea nodosa has been replaced by the invasive H. stipulacea (Sghaier et al., 2014), hinting that the dynamics of this invasion in this region may be changing.
The ongoing “tropicalization” of the Mediterranean basin, with its waters becoming saltier and warmer (Bianchi and Morri, 2003; Borghini et al., 2014), accompanied by the recent doubling of the Suez Canal (Galil et al., 2015), may cause less favorable conditions for native seagrasses.
Despite the capability of Posidonia oceanica plants to acclimate to temperature changes (e.g., Marín-Guirao et al., 2017, 2019), it has been predicted that even under a relatively mild greenhouse-gas emissions scenario, the endemic P. oceanica will face functional extinction by the middle of this century (Jordà et al., 2012). As the conditions may be worsening for native Mediterranean seagrasses (Jordà et al., 2012), they may become more favorable to tropical seagrass species. Indeed, the potential threat posed by this rapidly spreading plant is serious and has resulted in the inclusion of H. stipulacea in the “100 Worst Invasive Alien Species in the Mediterranean” (Lowe et al., 2000).
This review presents the current biological, ecological, physiological, biochemical, and molecular knowledge of H. stipulacea from both its native and invaded ranges. This combined knowledge allowed us to (i) discuss the possible environmental conditions and plant mechanisms involved in the two different invasions, (ii) assess the impact of H. stipulacea on native seagrasses and ecosystem functions in the invaded regions, and (iii) predict the ability of this species to invade European and transoceanic Atlantic coastal waters.
For this review, the search words “Halophila stipulacea” were entered into Google Scholar (accessed 14/03/2017-01/10/2019). The selected studies were all available online, in English, and a very few in Italian (due to the relatively abundant number of studies on H. stipulacea in the Mediterranean Sea in Italian waters). Downloaded studies were from published peer-reviewed journals, proceedings of scientific symposiums, published books, one M.Sc. thesis, and in rare occasions technical reports from academic institutions that were published as reports but not as scientific papers. Studies had to be easily downloadable (as opposed to publications on Google scholar that were not accessible via several platforms). Studies had to be focused specifically on H. stipulacea (general studies that just mentioned H. stipulacea by the way, were excluded) and usually included the species name in the title. All articles reviewed concerned geographical distribution, invasiveness, ecological, physiological, and biotic and abiotic interactions studies of the species itself. In addition, to account for older references that may not have been available through the literature search, the reference lists of each article was also checked and added to the database if considered to be relevant. We also updated the database with our own unpublished articles. Resulting articles were downloaded into Endnote to create a “H. stipulacea database” containing a total of 164 studies (Table S1; Figure S1).
Studies were categorized into regions in which studies were performed: Red Sea (R), Mediterranean Sea (M), Indian Ocean (I), Arabian Gulf (A) or the Caribbean Sea (C). Within each region, each study was assigned a reference label. Labels were spatially displayed using QGIS (https://qgis.org/) on maps pinpointing the precise/approximate location described in each study (447 sites; Table S1, Figures 3, 4, Supplementary Material S1). For uniformity, coordinates were converted into decimal degrees (D.ddd) in World Geographic System 84 coordinates (WGS84). Published studies with only a general location (e.g., the coast of Bahrain; Naser, 2014) were discriminated from those with precise localization (Table S1, Figures 3, 4).
Figure 3. Geographic distribution of published studies on Halophila stipulacea from its native habitat (black color). Shown are known records for the Indian Ocean (A), Red Sea (B), and Persian Gulf [(C); numbers for each site refer to the appropriate source (Table S1)]. Original coordinates were converted into decimal degrees (D.ddd) in World Geographic System 84 coordinates (WGS84).
Figure 4. Geographic distribution of published studies on Halophila stipulacea from its historical and more recent invaded habitats (red color) in the Mediterranean (A) and Caribbean (B) Seas. Numbers for each site refer to the appropriate source (Table S1). Labels refer to the year of the first report of H. stipulacea by location (Table S1). Original coordinates were converted into decimal degrees (D.ddd) in World Geographic System 84 coordinates (WGS84).
Studies within the “H. stipulacea database” (Table S1) were evaluated and classified according to their region, publication period (Figure 5A), and the general topic of study (study category 1): physiology, ecology, distribution, links with humans, or other (Table S1, Figure 5B). Studies were further assigned into more specific subcategories (study category 2; Table S1), such as sexual reproduction, grazing, mapping, etc. For this, a word cloud was generated using www.wordart.com (wordart.com/create; accessed 13/12/2019) to graphically display the diversity and frequency of the specific topics of research (study category 2) of the entire “H. stipulacea database” (Table S1). The wordlist is provided in Table S1.
Figure 5. (A) Number of publications per decade and region (based on Table S1). (B) Number of publications according to the paper's general field of study (category 1—physiology, ecology, distribution, links with humans, or other), per region.
To quantitatively assess the envelope of environmental conditions in which H. stipulacea exists in native and invasive regions, publications were searched for information associated with the abiotic conditions in described study sites—minimal/maximal depths, salinity, sediment characteristics, irradiance, minimal/maximal sea surface temperatures. Plant-related parameters including H. stipulacea's horizontal growth rates, leaf production rates, per cent cover, and characteristics of sexual reproduction, were collated and compared across all regions (Table 1, Supplementary Material S1).
Table 1. Summary of main environmental and ecological parameters published for Halophila stipulacea across its entire distribution (see Table S1 for full details).
The distribution of published studies on H. stipulacea from its native habitat (Figure 3) reveals that most studies were concentrated in just a few points in each biogeographic region. For example, in the 2,250 km long Red Sea, known for vast areas of H. stipulacea, most studies originate from the northern tip of the Gulf of Aqaba (GoA; Figure 3B). Studies from the Arabian Gulf stem only from the southern part of the Gulf, with not even one published study from Iranian shoreline (some 1,000 km long; Figure 3B). In the vast Indian Ocean (Figure 3A), all published studies originate from Tanzania, Kenya, or southern India.
The distribution of published studies on H. stipulacea from its historical invaded habitat in the Mediterranean Sea (Figure 4A) reveals a large number of studies, most of which are reported from the northeastern corner of this basin (Cyprus, Greece, and Turkey; Lipkin et al., 2003), with the most western record coming from Tunisia (Sghaier et al., 2011, 2014). Together with recent reports from Sicily (Gambi et al., 2018), these western locations potentially confirm the beginning of a westward migration of local populations in a rapidly warming Mediterranean Sea (Georgiou et al., 2016). The fact that H. stipulacea was recorded along nearly all shores of the eastern Mediterranean basin, but not along the Israeli and most of Libya's shorelines, is probably due to different reasons. In Libya, given the species' distribution in the surrounding nearby areas (Sghaier et al., 2011), we suspect that the absence of H. stipulacea in most of Libya is probably due to the lack of extensive monitoring data and underreporting (Badalamenti et al., 2011) rather than true absence. For the Israeli Mediterranean shoreline, the absence of H. stipulacea might be related to the fact that along this ~190 km there are no natural shallow protected bays that would allow the development of seagrasses.
The distribution of published studies on H. stipulacea from its new invaded range in the Caribbean (Figure 4B) demonstrates that H. stipulacea has expanded there rapidly; starting from its first finding in Grenada in 2002 it has expanded fast, both northwards and westwards (reviewed by Willette et al., 2014; discussed below).
The summary of published studies (Table S1) highlights continuous research efforts (spanning over more than 40 years) on different aspects of H. stipulacea, both in its native Red Sea habitat (33% of studies) and in its historical invaded area in the Mediterranean Sea (35% of studies; Figure 5A). While H. stipulacea is also native to the Indian Ocean and Arabian Gulf (Figure 4), our review points to the relatively few studies, and thus a large gap of knowledge, in these two regions (Figure 5A). This is of particular concern in the Arabian Gulf which accounts for only 4% of the studies on H. stipulacea. On the other hand, the results confirm the growing research interests in the Caribbean (14% of H. stipulacea studies), where, in less than two decades, there are as many publications as in the much larger native habitat in the Indian Ocean (14% of total studies).
Categorizing the H. stipulacea data set (Table S1) into regions and according to their main area of research (category 1; Figure 5B), revealed that, across all regions, studies on distribution and ecology of H. stipulacea were numerous. However, the Arabian Gulf and the Caribbean regions lack studies on the physiology, links with humans and genetics/eco chemistry (“other” study category), in comparison with the diversity of H. stipulacea studies from the Red and Mediterranean Seas. These results indicate an “evolution” of topics of study, starting with distribution record, and with time, including other topics as ecology, physiology, and eventually links with humans (Figure 5B).
The generated word cloud (Figure 6) shows that across the entire “H. stipulacea database” (164 published studies; Table S1), the most frequent areas of research are ecology, habitat surveys and physiology. This word cloud identifies specific gaps of knowledge with relatively few studies on associated fish and epibiotic communities, ecosystem services, and on conservation and management of H. stipulacea, highlighting necessary future attention in these fields.
Figure 6. Word cloud showing the diversity of the specific topics of research on Halophila stipulacea (category 2 in Table S1, Figure 5B). The size of each word indicates the relative frequency of the research topic.
Summarizing the main environmental and ecological parameters published for H. stipulacea across its native and non-native ranges (Table 1) demonstrates that H. stipulacea grows in a wide range of depths (1–70 m), salinities (24–70 PSU), temperatures (17–42°C) and substrates (Table 1). It is interesting to observe how in its non-native habitats, sexual reproduction is less frequent. This summary also highlights some gaps of knowledge of its ecology in the Arabian Gulf and the Indian Ocean, despite these regions being much of H. stipulacea's native habitat.
Researchers have been debating on what characteristics make alien species successful invaders (see e.g., Williamson and Fitter, 1996; Kolar and Lodge, 2001; Sol et al., 2012). In principle, the most basic attributes include high reproductive capacity (sexual and/or asexual), wide phenotypic plasticity, high dispersal ability (e.g., extended planktonic duration) and strong competitive ability. Furthermore, the receiving environment should, in theory, exhibit “invadable” characteristics such as elevated level of disturbance for the native species, availability of empty niches, low level of biotic resistance, and high availability of resources (Olyarnik et al., 2009).
The uniformity of conditions in the receiving environment is also important. The variability of environmental conditions in the Mediterranean and Caribbean Seas are different. The Mediterranean exhibits a wider range of temperature and salinity values from the south-eastern Levant corner characterized by salty, warm, and fast-warming waters (Rilov, 2016; Ozer et al., 2017) to the coolest and less salty parts of the Adriatic Sea (Russo et al., 2012). Conversely, the conditions are much more uniform in the Caribbean and Red Seas, which apparently are ideal for the growth and spread of H. stipulacea (Georgiou et al., 2016).
The invasion history (timeline) and distributional spread of H. stipulacea in the Mediterranean and Caribbean Seas (Figure 4) show contrasting patterns. In the Mediterranean, H. stipulacea's invasion followed the opening of the Suez Canal in 1869, with the first meadow reported only 25 years later in Rhodes (Fritsch, 1895; Table S1), making it one of the first successful Lessepsian migrants (Lipkin, 1975a,b). By 1926, well-established meadows were reported from Rhodes (Issel, 1928; Figure 4A). After that, it was recorded along the coasts of Greece, Egypt, Malta, Cyprus and Lebanon (Den Hartog, 1970; Lipkin, 1975a,b; Van der Velde and Den Hartog, 1992), followed by a northward expansion into Turkey and Albania (Alpinar, 1987; Kashta and Pizzuto, 1995) and a westward expansion toward Malta and the Ionian coast of Sicily (Lanfranco, 1970; Biliotti and Abdelahad, 1990). The first report of H. stipulacea in the western Mediterranean was in 1995 off Vulcano (Sicily; Acunto et al., 1995), followed by reports from the southern coast of Italy, Libya, and Tunisia (Gambi et al., 2009; Sghaier et al., 2011, 2014). By now H. stipulacea has spread throughout most of the eastern and southern Mediterranean Sea (Lipkin, 1975a,b; Procaccini et al., 1999b; Gambi et al., 2009, 2018; Sghaier et al., 2011, 2014; Nguyen et al., 2018; Figure 4A). Based on these records, the spread of the invasive H. stipulacea in the Mediterranean can be considered old, slow, and highly punctuated in space. The species spread rate across the Mediterranean Sea over these 120 years is roughly 12 km yr−1 (Georgiou et al., 2016) which is very low compared to the 300 km yr−1 expansion of other invasive macrophytes in this region (Lyons and Scheibling, 2009; Mineur et al., 2015).
Within the Mediterranean, sightings have mostly been limited to locations near ports and marinas. Meadows sizes have been from relatively minute (e.g., 16 m2; Gambi et al., 2009) to large (e.g., 0.2 ha; Sghaier et al., 2014). These invasion dynamics suggest that the main vectors for the introduction and further spread were shipping activities (it was first recorded in a port and all subsequent locations were also ports and marinas). Although H. stipulacea was categorized as one of the worst invasive species in the Mediterranean (Lowe et al., 2000; Streftaris and Zenetos, 2006), these observations suggest, in fact, a relatively limited “invasion success” in this region, as can also be inferred from the limited number of studies reporting competitive displacement of native seagrasses by the alien H. stipulacea (Williams, 2007; Tsiamis et al., 2010). However, reports on competitive displacement do exist. Sghaier et al. (2014) showed that a large (0.2 ha) patch of H. stipulacea in Cap Monastir Marina (eastern Tunisian coast) grew to cover more than 2.2 ha in only 4 years, and, in the process, displaced 50% of the native Cymodocea nodosa. It is also possible that this transition is not driven by competitive exclusion, but by natural (or human-driven) reduction of the native which freed areas for colonization by the alien seagrass.
It has been observed that H. stipulacea populations in the Mediterranean are ephemeral (Chiquillo et al., 2018), with meadows shrinking in winter, and expanding in summer (Nguyen et al., 2020a; Procaccini, pers. comm.). Concordantly, the Mediterranean temperatures fluctuate rapidly between 14°C in the winter and 29°C in the summer. Similarly, growth rates vary from 0.5 cm d−1 during the summer and dropping to a minimum of 0.06 cm d−1 in the winter (Georgiou et al., 2016).
However, water temperatures in the Mediterranean Sea do not seem to be a limiting factor for this species' survival and expansion, as growth rates in the Mediterranean are overall greater (0.35–0.5 cm d−1; Georgiou et al., 2016), than in its native Red Sea (maximum growth rate 0.206 cm d−1; Wahbeh, 1984). These differences may be related to other intrinsic properties that may act to control the spread and growth of H. stipulacea in the Mediterranean (Shaltout and Omstedt, 2014).
The introduction of H. stipulacea into the Caribbean is believed to have been unintentionally caused mainly by recreational vessels traveling from the Mediterranean to the Caribbean (Ruiz and Ballantine, 2004). In contrast to the invasion history and distributional spread of H. stipulacea in the Mediterranean (Figure 4A), the Caribbean invasion by H. stipulacea (Figure 4B) is young (<17 years) and rapid.
H. stipulacea has been in the Caribbean for at least 17 years. This seagrass was first reported growing as a 30 m2 monospecific bed in bare sand in Flamingo Bay, Grenada, in 2002 (Ruiz and Ballantine, 2004). Five years later it was recorded 350 km to the north on Dominica, covering an area of 22 ha (Willette and Ambrose, 2009). Since then, reports on H. stipulacea found on other eastern Caribbean islands and along the Venezuelan coastline have been published almost yearly (Vera et al., 2014; Willette et al., 2014; Ruiz et al., 2017; Figure 4B).
The regional spread of H. stipulacea in the Caribbean is likely due to a combination of storm-induced redistribution, inter-island vessel transit, and near-shore fishing activities (Willette and Ambrose, 2012; Willette et al., 2014). Fragments of H. stipulacea were shown to survive for days in the water column, settle, and take root (Willette et al., 2020). Smulders et al. (2017) showed all H. stipulacea fragments tethered above the sediment rooted within 10 days. On average, these fragments added 0.9 new shoots d−1 (Smulders et al., 2017), approximately twice the rate reported for H. stipulacea in the Mediterranean Sea (Georgiou et al., 2016).
Halophila stipulacea fragments are released during the removal of wooden and metal fish traps commonly used by fishermen in the eastern Caribbean. Fish trap removal from H. stipulacea beds generated fragments 72% of the time, with each trap creating on average 11 fragments consisting of multiple shoots (Willette and Ambrose, 2012). Furthermore, these traps are often moved across bays and are not regularly cleaned from fouling organisms, including seagrass fragments (Willette, pers. observations), thus facilitating local dissemination of H. stipulacea.
In the Caribbean, H. stipulacea grows at depths between 0.2 and 32 m (Maréchal et al., 2013; van Tussenbroek et al., 2016) and is often reported in harbors and ports but is also found in bays and along open coastlines (Willette et al., 2014). The seagrass has been reported to grow on a range of substrates, including sand, mud, and coral rubble (Steiner et al., 2010; Willette et al., 2014). Much of the Caribbean landscape that H. stipulacea has expanded into consists of bare sand, including sand “halos” and the margins of coral reefs, where other seagrasses usually do not grow (Steiner and Willette, 2015a). These sand “halos” and coral reef margins are maintained by the grazing activities of reef-associated invertebrates and fish (Randall, 1965; Valentine and Heck, 2005).
The loss of the sand “halos” and colonization of H. stipulacea in these areas suggest that H. stipulacea's growth rate is faster than local grazers can consume and, that it is able to utilize the available bare sand as its niche habitat (Steiner and Willette, 2015a). Indeed, in the Caribbean H. stipulacea has a rapid and wide range of lateral rhizome expansion rates, ranging between 0.5–6.7 cm d−1 (Willette and Ambrose, 2009; Willette et al., 2020; Table 1). Failure of reef-associated organisms to maintain these sand “halos” may also indicate lower herbivory pressure on H. stipulacea compared to other native Caribbean seagrass species (e.g., S. filiforme; Muthukrishnan et al., in review).
In the Caribbean, H. stipulacea is typically reported growing in monospecific beds or as understory in the much taller S. filiforme and Thalassia testudinum but also described growing in mixed meadows with H. decipiens (Willette et al., 2014). van Tussenbroek et al. (2016) observed that H. stipulacea grew at different densities depending on the nitrogen (N) content of its substrate—low shoot densities in substrates with low N content (<7%), and dense, thick mats in areas with high N content (>11%).
In the Caribbean, as the density of H. stipulacea increases, it sends out lateral rhizomes that grow between shoots of the native S. filiforme, enfolding and eventually (within months) displacing it by monopolizing its space (Willette and Ambrose, 2012; Steiner and Willette, 2015b). Over a 5-year period in Dominica, Steiner and Willette (2015b) documented a dramatic gain in seagrass cover, from 316 ha to 773 ha, attributing this increase to the expansion of H. stipulacea beds. They found that S. filiforme's distribution decreased by 150 ha, while in parallel the cover of H. stipulacea increased by 649 ha, mainly through colonization of bare sand and notably by physically displacing beds of S. filiforme. Likewise, H. stipulacea replaced H. wrightii at depths >4 m, while H. decipiens was entirely eliminated from the surveyed sites. Similarly, Smulders et al. (2017) used fixed location surveys and observed a significant decrease in T. testudinum cover, from 53 to 33%, and a significant increase in H. stipulacea from 6 to 20% in Lac Bay, Bonaire between 2011 and 2015. S. filiforme maintained a consistently low coverage over this period. Shifts illustrating a decrease in native seagrass coverage and the concurrent increase in H. stipulacea have also been quantified or anecdotally reported from Carriacou, Martinique, St. Thomas, St. John, and Curaçao (Maréchal et al., 2013; Willette et al., 2014; Scheibling et al., 2018; Engelen, pers. observation). These studies indicate H. stipulacea's contribution toward the transformation of the Caribbean seagrass species composition, leading to a major change in the Caribbean's seagrass landscape (Steiner and Willette, 2015b).
Compared with the relatively old (<120 years) and limited “invasion success” in the Mediterranean (discussed above), the fast and far-reaching spread in the Caribbean, along with the competitive exclusion of several native species (Steiner and Willette, 2015b), suggest a highly “successful” invasion by H. stipulacea (Ruiz et al., 2017). Understanding the differences between these two invasions is crucial for being able to predict the potential mechanism of H. stipulacea's success in its new invaded habitats.
The vegetative and some of the reproductive morphology of H. stipulacea from its native habitats have been described before (Lipkin, 1975c; El Shaffai, 2016; Nguyen et al., 2018). Key morphological features of H. stipulacea from its native habitats include short stems, each carrying two leaves, linear leaf blades (>6 cm long and 0.8–1.0 cm wide) that contain a clear mid vein with branched cross veins (Figure 2A). The leaf margin is serrated and minute trichomes may be present on one side of the leaf surface (Figure 2E). H. stipulacea's rhizome is smooth with long internodes (1–4 cm) and is covered by leaf scars at the stem base (El Shaffai, 2016). Roots are usually shallow and are covered by small hairs and, depending on the substrate, they could be sand- or gravel-binding (Den Hartog, 1970; Kuo and McComb, 1989). Structurally, H. stipulacea has not been reported to differ between non-native and native ranges.
Halophila stipulacea reproduces both sexually (through seeds) and asexually (i.e., fragmentation or vegetative rhizome growth) in its native range (Malm, 2006; Nguyen et al., 2018). While the importance of sexual recruitment in seagrass populations is assumed to be generally low (Rasheed, 1999), small seagrass species such as H. stipulacea are thought to be more sexually fecund than larger seagrass species (Kenworthy, 2000; Malm, 2006). In terms of sexual reproduction, H. stipulacea belongs to a rare group of plants (only ~5% of angiosperms; Charlesworth, 2002) that are dioecious, meaning that there is a separation between male and female individuals.
Morphologically, male (staminate) and female (pistillate) flowers are both inconspicuous (Ackerman, 2000). The almost translucent perianth consists of three tepal lobes (Lipkin, 1975c; Chiquillo et al., 2018; Figure 2B) that, for female flowers, are fused into a 6 mm perianth-tube enclosing an inferior ovary (Kaul, 1968), three carpels, three styles, and three stigmas (Simpson, 1989; Figure 2C). The perianth of male flowers encloses a sessile stamen and three dark-colored anthers (Lipkin, 1975c; Pettitt, 1981; Figure 2B). Male flowers release trinucleate pollen in four mucilaginous strands (Pettitt, 1981) which may fertilize female flowers to form seed capsules (4–6 mm) containing 0.2 mm diameter seeds (Figure 2D). Ripe seed capsules (Figure 2D) detach from the mother plant (Figure 2C) and float on the water surface for some hours before seeds are dispersed, the latter of which do not float (Malm, 2006).
Sexual reproduction seems to vary across its native and non-native ranges. In the former, the flowering season lasts 4–5 months (May-Sep), with flowering events producing both staminate (male) and pistillate (female) flowers (Den Hartog, 1972; Lipkin, 1975c; Malm, 2006; Figures 2C–E). In the northern Red Sea where this species is native, flowering occurs annually (Malm, 2006; Nguyen et al., 2018) and the female/male sex ratio (F/M) is strongly biased toward female flowers (Malm, 2006). In the Mediterranean, the flowering of H. stipulacea is much less common. Flowers in that region were first reported by Politis in Greece in 1926, while fruits were first reported from Cyprus in 1967 (Lipkin, 1975a), ~73 years after the Lessepsian migration. Typically, only male flowers were observed in the Mediterranean region (Procaccini et al., 1999a; Gambi et al., 2009) and it was assumed that (a) clonal propagation might be the dominant reproductive mode in the Mediterranean Sea (Procaccini et al., 1999a; Chiquillo et al., 2018), and/or that (b) the introduction of H. stipulacea into the Mediterranean was of male genotypes only, which spread clonally; or alternatively, that (c) female flowers were unable to develop under the Mediterranean environmental conditions (Gambi et al., 2009). However, in 2012, Gerakaris and Tsiamis (2015) reported on the presence of mature seed capsules (female plants) in the Chios (Greece). More recently, Nguyen et al. (2018) found adjacent female and male flowers in the Mediterranean (Cyprus) and confirmed that sexual reproduction was indeed taking place; Nguyen et al. (2018) reported that sex ratios, however, were male-dominated in the invaded sites as opposed to the female-dominated native habitats.
In the northern GoA, where H. stipulacea is the native dominant seagrass species, reproduction starts in May and ends by the beginning of September (with <5% of plants flowering by mid-September Malm, 2006; Nguyen et al., 2018). Although we don't have any data on the exact beginning of the reproductive season in the Mediterranean (highlighting another knowledge gap), it seems that it lasts much longer into the autumn, with Nguyen et al. (2018) showing that some 23% of H. stipulacea invasive plants in Cyprus, were still reproducing in Mid-October.
Flowering in the Caribbean appears to be even less common than in the Mediterranean, although the lack of reports on H. stipulacea female flowers and fruits could be a consequence of past limited survey efforts. The first flowering report in the Caribbean found only male flowers occurring in Venezuela (Vera et al., 2014), 12 years after H. stipulacea was initially observed in this region (Ruiz and Ballantine, 2004). The fact that since then, reports on flowering of invasive H. stipulacea in the Caribbean have only found male flowers (e.g., Chiquillo et al., 2018) suggests that introductions of H. stipulacea in this region have, so far, included only male plants of this dioecious seagrass, or that local conditions are somehow preventing the appearance/survival of female flowers. If female flowers were to be found in invasive Caribbean populations, this may have important implications for the future dispersal, survival, and maintenance of invasive populations in this region.
In its native range in the Indian Ocean and the Red Sea (where it was originally described; Forsskål and Niebuhr, 1775; Lipkin, 1975b), H. stipulacea is one of the most widespread seagrasses (Wahbeh, 1984; Price and Coles, 1992). In the Arabian Gulf, it co-occurs with the fast-growing Halodule uninervis and Halophila ovalis (Phillips et al., 2002; Campbell et al., 2015c). Recent records from the central and southern Red Sea have shown that, in some sites (e.g., Umluj, Jazan), it forms mixed meadows with H. uninervis, H. ovalis, Syringodium isoetifolium, Thalassodendron ciliatum, and Thalassia hemprichii (Qurban et al., 2019). On the other hand, H. stipulacea in the northern GoA (northern Red Sea) usually forms mono-specific meadows (Al-Rousan et al., 2011) both in shallow and deep environments (1–50 m depth; Sharon et al., 2011b; Winters et al., 2017), although even here it has been reported to mix with H. uninervis (Al-Rousan et al., 2011).
Along the Indian and eastern African coasts, H. stipulacea is markedly less documented (Jagtap, 1991; De Troch et al., 2001; Kamermans et al., 2002). In Madagascar and Kenya, its existence is rare and poorly documented, but it was reported at depths beyond all other local seagrass species (De Troch et al., 2001). Reproductive female and male flowers were observed off the Kenyan coasts (Pettitt, 1981) but, altogether, the presence of H. stipulacea seems to be scarcer there than in the Red Sea.
Although the general morphological features of H. stipulacea have been described before (e.g., Den Hartog, 1970; see also Figure 2), in its native areas, it displays high morphological and biochemical plasticity in response to temporal and spatial environmental gradients. For example, in the GoA H. stipulacea produced new leaves at intervals of 5–12 days depending on the season, resulting in an estimated leaf turnover of 64.8 days (Wahbeh, 1984). Studies in the GoA pointed out significant variability in leaf density and biometry, with a lower number of leaves and larger leaf area in winter relative to the number of leaves and leaf area in summer (Beca-Carretero et al., 2020). There was a marked increase in leaf descriptors such as length, width, and leaf area with depth, which would allow for better light capturing at depth (i.e., Lipkin, 1979; Rotini et al., 2017). Also, internode lengths varied from 11.2 cm in deeper areas (30 m) compared to 6.8 cm at intermediate depth (17 m) (Schwarz and Hellblom, 2002). Similar observations have been widely documented for other seagrass species (Short and Duarte, 2001; Olesen et al., 2015).
Accompanying these structural leaf changes, biochemical variations with depth were also observed, with higher photosynthetic pigment concentrations (chlorophyll a, chlorophyll b, carotenoids) recorded at deeper areas, allowing to optimize light-capturing at dim irradiances (Lee et al., 2007; Rotini et al., 2017). Indications of biochemical plasticity also included temporal and spatial adjustments of H. stipulacea's leaf phenol content, with significant reductions at increasing depth/reduced light (Mejia et al., 2016; Rotini et al., 2017). A significantly higher phenol content was also found in winter than in summer months (Beca-Carretero et al., 2020), suggesting that this species is better protected from herbivory during certain seasons.
Interestingly, total fatty acid (TFA) content and composition were found to vary at different depths (6–21 m; Beca-Carretero et al., 2019). There was a high capacity to accumulate significantly more TFAs from shallow [6 m depth, 1.2% of dry weight (DW)] to deep areas (21 m, 1.6% of DW). These differences were mainly related to the synthesis of polyunsaturated fatty acids (PUFAs), which promote the fluidity of the chloroplast membranes, as well as electron transport in the photosystems, thus improving optimal photosynthetic responses.
Carbon (C) content in H. stipulacea from the GoA varied from 18 to 37% for leaves and 25.5–34.4% of DW for rhizomes/roots; while the N content ranged from 0.8 to 1.7% in leaves and 0.31–1.62% of DW in roots/rhizomes (Wahbeh, 1984; Schwarz and Hellblom, 2002; Beca-Carretero et al., 2020). Overall, the C content remained rather stable over seasons and depths, whereas the N content changed significantly across seasons and depths (Beca-Carretero et al., 2020). The low levels of nitrogen observed in H. stipulacea in native areas (<1.8% of DW) indicated a marked nutrient limitation in those marine environments (Duarte, 1992). Lipid accumulation in leaves (5.1–16.7% of DW) and rhizomes (27.2–3.4% of DW) varied significantly from season to season, with higher levels observed in spring (Wahbeh, 1984).
Working in the GoA, Beca-Carretero et al. (2019) recently assessed the total content of fatty acids (TFA) in H. stipulacea, and showed that the TFA content in H. stipulacea leaves (1.4 ± 0.2 mg g−1 DW) was comparable to seagrass species at similar latitudes (Nichols and Johns, 1985; Hanson et al., 2010). However, further analysis revealed an unusually high content of PUFA (66.0% of TFA), more similar to seagrass species inhabiting higher latitudes, and thus colder regions (e.g., 64.0% of TFA in Zostera noltii) than tropical or subtropical species, including H. ovalis (48% of TFA) (Viso et al., 1993; Hanson et al., 2010; Beca-Carretero et al., 2018). Lipid composition of the thylakoid membrane partially determine the thermal tolerance of primary producers (i.e., Nishida and Murata, 1996), consequently, this physiological characteristic of H. stipulacea might partially explain its capacity to survive to winter temperatures (~14–16°C) in the Mediterranean Sea.
H. stipulacea features a unique way of adapting to various irradiances by its ability to perform the so-called chloroplast clumping. This phenomenon was first described by Drew (1979); he observed that leaves of H. stipulacea from high-irradiance intertidal southern Sinai (Red Sea) became pale during midday, and then turned darker green from dusk until the following morning. Microscopy (performed in the field) revealed that the paleness of the leaves was due to clumping of chloroplasts to one part of the cytoplasm of each epidermal (i.e., photosynthesizing) cell.
Chloroplast clumping (Sharon and Beer, 2008; Figures 7A–D) could be induced by growing H. stipulacea in high midday irradiance of 450 μmol photons m−2 s−1 (ca. ¼ of full sunlight), whereas no such clumping occurred in a shaded midday irradiance of 150 μmol photons m−2 s−1 (Sharon and Beer, 2008). The chloroplast clumping resulted in leaf optical changes, with a decrease in absorbance and an increase in transmittance, causing a decrease in the absorption factor (AF) of the leaves from ~0.6 to ~0.3 (Sharon and Beer, 2008; Figure 7E). This has implications on photosynthetic measurements by pulse-amplitude modulated (PAM) fluorometry since electron transport rates (ETR) are a direct function of AF (Beer et al., 1998, 2014). Another important consequence of such chloroplast clumping is that it provides H. stipulacea the potential to adapt to a large spatial and temporal variation in irradiances, e.g., along depth gradients, seasons, and localities (as well as diurnally). This was demonstrated when H. stipulacea ramets in the GoA were transplanted from shallow (8 m with ~400 μmol photons m−2 s−1 during midday) to deeper (33 m, ~35 μmol photons m−2 s−1 during midday at the low-light season) areas, and vice versa, along a continuous meadow, and the photosynthetic properties of the leaves were followed for 2 weeks using in situ PAM fluorometry. It was found that both maximal photosynthetic rates at light saturation of photosynthesis (Pmax) and the onset of saturating light (Ik) acclimated in the transplanted plants within 1 week to values similar to the control plants (i.e., plants that grew naturally at the corresponding depths and were moved within the same depth; Sharon et al., 2009). Similarly, chlorophyll levels in the leaves of the transplanted ramets closely reflected values from the control plants. Thus, plants were able to photo-acclimate rapidly to both increased and decreased irradiances.
Figure 7. Chloroplast clumping in Halophila stipulacea. Microscope pictures (A,B) and whole-leaf pictures (C,D) of H. stipulacea leaves growing in low and high light environments. The chloroplasts (residing mainly in the epidermis) are diffused throughout the cells' cytoplasm at low irradiance (A,C), thus leaves appear dark green. In contrast, the chloroplast clump together (B,D) in shallow high-light growing plants, thus leaves appear more transparent. The chlorophyll content per leaf area is the same in (A–D), but the difference in color is due to clumping of the chloroplasts, entailing less light-absorption and more photoprotection (from Beer et al., 2014). (E) Daily changes in absorption factor (AF, n = 10) of H. stipulacea grown on a sunlit water table under shading nets at ~150 (open circles) and ~450 (closed circles) μmol photons m−2 s−1 during midday. Significant differences (p < 0.01, one-way ANOVA) along the day are indicated with different letters (adapted from Sharon and Beer, 2008; Beer et al., 2014). Photos taken by Yoni Sharon (A–D). All photos in this figure have been obtained with permission from the original copyright holders.
In a follow-up study, chloroplast clumping in H. stipulacea plants also occurred in response to high UV irradiance (Sharon et al., 2011a). Hence, it seems that chloroplast clumping protects the leaves' photosynthetic machinery by shielding one another from potentially harmful irradiances, including UV light. While some chloroplasts in the periphery of the clump “sacrifice” themselves and become photodamaged, most chloroplasts benefit from the clumping mechanism thus allowing the species to survive in shallow intertidal and high-light exposed waters. Conversely, the intracellular spreading of chloroplasts in the leaf surface of deep-water seagrasses allows for maximum light capture in the light-limited environment.
While the clumping phenomena are rare and have so far not been documented in other seagrasses, there are few reports on its existence in the terrestrial plant literature (e.g., Kondo et al., 2004; Yang et al., 2011).
An additional feature that could support photosynthesis and growth in dim-light environments (e.g., in deep waters) is the apparent ability to change photosystem II (PSII) to photosystem I (PSI) ratios under extremely low irradiances. At the ~50 m depth limit of H. stipulacea in the northern Red Sea, the PSII:(PSII + PSI) ratio was ~0.4 compared to the ~0.6 for H. stipulacea in shallow environments (Sharon et al., 2011b). This is an apparent adaptation to both the low light (~100 μmol photons m−2 s−1 at midday in summer) and blue-shifted irradiance spectrum prevailing at these depths.
So, what are the light requirements for maintenance of positive net photosynthetic rates to sustain growth in H. stipulacea? Being rooted, with a considerable part of their biomass underground, seagrasses, in general, have a higher light requirement than both phytoplankton (0.1–1% of surface light) and macroalgae (1–2% of surface light), with the dogma for a “typical” seagrass surface irradiance requirement is ~10% (Duarte, 1991). However, given that H. stipulacea is thin-leaved (a large proportion of the leaf consists of only two layers of photosynthesizing epidermal cells) and the root/shoot ratio is low, this seagrass may need much less light. A good estimate is ~5% of surface light (i.e., ~100 μmol photons m−2 s−1) as derived from the irradiance measured at its ~50 m depth limit in the northern Red Sea on a sunny summer's day (Sharon et al., 2011b).
The photosynthetic traits that were described here for H. stipulacea are unique among seagrasses (although they might be shared with other Halophila species, e.g., H. ovalis; Beer et al., 2002; Phandee and Buapet, 2018). These traits, together with an efficient Ci-acquisition system, undoubtedly play a role in H. stipulacea's adaptability to various environments and its apparent rapid acclimation to changing conditions. This might be one reason for its invasiveness into habitats where it was recently introduced. What we do not know is how these photosynthetic abilities may influence its competitiveness with other seagrasses and marine macrophytes. Pursuing research into the degree by which the special photosynthetic traits of H. stipulacea contribute toward invasiveness is thus recommended (e.g., can blocking the chloroplast clumping in H. stipulacea influence its competitiveness with other seagrasses? Yang et al., 2011).
Halophila stipulacea is not only highly adaptable to various irradiances, but also features very efficient inorganic carbon (Ci) acquisition mechanisms. These carbon concentrating mechanisms (CCM) consist of either a bicarbonate () transporter localized within the outer membranes of the photosynthesizing cells, or a carbonic anhydrase (CA)-catalyzed extracellular enzyme (within the cell wall) for the conversion of to CO2; both are assisted by proton pumps acting outwards from the cells (Beer et al., 2002). Since is the major Ci source in seawater, either of these mechanisms (or both together) may confer high photosynthetic rates to H. stipulacea.
It has been suggested that the invasiveness of H. stipulacea might be attributed to it being highly adaptive to a wide range of abiotic conditions, including light intensities (Sharon et al., 2009, 2011b), water temperatures (Angel et al., 1995; Georgiou et al., 2016) and salinities (Por, 1971, reviewed by Gambi et al., 2009; Oscar et al., 2018).
Halophila stipulacea is known as a euryhaline species because of its wide range of salinity tolerance (Den Hartog, 1970; Por, 1971; Oscar et al., 2018). Salinity is a major environmental component that can influence the growth, function, structure and distribution of seagrasses (Montague and Ley, 1993; Salo et al., 2014). Although it is assumed that the first establishments of H. stipulacea in the Mediterranean were directly from ships (Lipkin, 1975b), tolerance to the hypersaline waters of the Suez enabled this euryhaline seagrass species to become very abundant in the canal (Fox, 1926; Aleem, 1979; Gab-Alla, 2001) and in the same way, also made it possible to thrive in the less saline waters of the Mediterranean (Lipkin, 1975b). Changing conditions, such as the ongoing increase in water temperature and salinity associated with the tropicalization of the Mediterranean Sea (Bianchi and Morri, 2003; Borghini et al., 2014) can potentially restructure seagrass communities, where species with lower salinity and temperature tolerance range can possibly disappear (Zieman et al., 1999; Rudnick et al., 2005).
The only known study investigating salinity tolerance of H. stipulacea at the cellular level has shown that the epidermal concentrations of Na+ and Cl− were lower than in the surrounding seawater, indicating the existence of some ion exclusion mechanisms (Beer et al., 1980). Additionally, this study also showed that carbon-fixing enzymes were able to function in the presence of intra-cellular salt concentrations in vitro, which is an important adaptive mechanism to salinity variations. Detailed experiments exploring the thresholds of H. stipulacea's hyper- and hypo-salinity tolerance need to be conducted and combined with niche models in order to predict if salinity is a limiting factor for the spread of this species (see for example Oscar et al., 2018; Gamliel et al., 2020).
Differences in other abiotic factors among the various geographic basins of H. stipulacea do not seem strong enough to justify the observed differences in the growth rates and the occurrence of sexual reproduction (Table 1). For example, SSTs differ greatly between the Mediterranean and the Red Sea, while irradiance is relatively similar. However, SSTs and irradiance in the Red Sea and the Caribbean are relatively similar. Alternatively, the differences in the occurrence of sexual reproduction may be related to the dynamics of the different introductions (the Mediterranean and Caribbean Seas).
Experimentally, Georgiou et al. (2016) showed that H. stipulacea from Cyprus is functional at most Mediterranean temperatures (from 10 to 30°C). While Georgiou et al. (2016) did not test the functionality of H. stipulacea beyond 30°C, it was expected to thrive within the warming waters of the western and northern Mediterranean (Georgiou et al., 2016). Based on experiments with plants from one of its invaded locations in the eastern Mediterranean (Limassol, Cyprus), Georgiou et al. (2016) suggested that summer maxima in the Levant are indeed beyond the optimal conditions for growth.
In a recent experimental study (Nguyen et al., 2020b), native (Eilat, northern GoA), and invasive (Limassol, Cyprus, eastern Mediterranean Sea) H. stipulacea populations were subjected to a 2-week heatwave (29 and 32°C) in a controlled microcosm experiment. While invasive plants remained largely unaffected after the heatwave, native plants experienced reduced fitness and biochemical and photo-physiological parameters. These results not only point out the differences in the thermal tolerance among populations but also suggest a rapid adaptation (or a previous selection, as happens in ballast waters) by the invasive population to the ongoing warming of the Mediterranean Sea. This indicates that high temperatures in the Levant may not be a limiting factor for the presence of the alien seagrass in the region, although longer exposure might be more detrimental for this population.
In terms of substrate, H. stipulacea can grow in different sediment types, ranging from fine sand/mud to coarse gravel-sand, and even in patches between coral heads (Jacobs and Dicks, 1985; Angel et al., 1995; Mejia et al., 2016; Figure 4). In disturbed areas, such as the oil-polluted waters of Saudi Arabia, H. stipulacea was the most abundant amongst other seagrass species, highlighting its capacity to survive in contaminated and unfavorable environments (Kenworthy et al., 1993).
In terms of nutrient uptake, a recent study in the GoA reported a limited capacity of H. stipulacea for nitrate uptake, but high capacity and efficiency for ammonium, a trait common to other seagrass species (Cardini et al., 2018). Noticeably, this species exhibited an unusual high capability for N uptake under N-limited environmental conditions, potentially due to a high capacity for N2 fixation and ammonium production of its associated diazotrophic epiphytes. This may represent an asset for H. stipulacea when interacting and competing for resources with other seagrass species (Cardini et al., 2018). In its invaded ranges, H. stipulacea displayed also a limited capacity to use nitrate, which may restrict growth and survival in areas where the availability of ammonium, the preferred nitrogen source of the species, becomes infrequent or non-existent (Alexandre et al., 2014). On the other hand, the equal capacity and efficiency of leaves and roots for ammonium uptake may contribute to the dispersion of the species in sites where nutrients are available both in the water and sediment.
Seagrasses and seagrass meadows are in general considered one of the most productive and complex systems on a worldwide scale (Den Hartog, 1970). H. stipulacea beds support a wide diversity of algal and animal communities in both their native and invaded ranges (De Troch et al., 2001, 2003; Tsirika and Haritonidis, 2005; Di Martino et al., 2007; Willette and Ambrose, 2012; Scheibling et al., 2018). In its native range in the Indian Ocean, studies of seagrass-associated fauna and flora are scarce (Aleem, 1979; De Troch et al., 2001, 2003; section Associations of H. stipulacea With Other Organisms in Its Native Ranges), whereas such studies in its invaded ranges in the Caribbean and the Mediterranean are more abundant (see sections Associations of H. stipulacea With Other Organisms in the Mediterranean and Association of H. stipulacea With Other Organisms in the Caribbean Sea).
Seagrasses host highly diversified microbial communities (Bagwell et al., 2002; Hamisi et al., 2009; Cúcio et al., 2016; Mejia et al., 2016; Rotini et al., 2017) that are known to form a singular entity or holobiont, in line with what has been suggested for corals (Rosenberg et al., 2007). In the “holobiont” framework, the associated microbial communities may influence the health, performance and resilience of the hosts (Taylor et al., 2007; Zilber-Rosenberg and Rosenberg, 2008; Rout et al., 2013; Coats and Rumpho, 2014; Singh and Reddy, 2014). Likewise, the host condition may shape the structure and the diversity of the microbial communities (Meron et al., 2011; Campbell et al., 2015a,b; Marzinelli et al., 2015; Martin et al., 2018). Unfortunately, microbiome research in seagrasses is still at its infant stage, far less than microbial studies in sponges and corals. The great metabolic variability of microbes, made available to host plants, calls for further studies aimed at investigating plant-microbes interactions and their functional outcomes, including ecological resilience and invasive capacity.
In its native range, H. stipulacea leaves and rhizomes were found to be almost devoid of epibionts across different seasons (Aleem, 1979). Macroalgae like Turbinaria spp. and Caulerpa spp. were found to occasionally co-occur with H. stipulacea in the Red Sea (Jacobs and Dicks, 1985). Among the marine fauna associated with H. stipulacea meadows, De Troch et al. (2001, 2003) reported high diversity of harpacticoid copepods off the coast of Kenya where a deep and mixed bed of H. stipulacea and S. isoetifolium exists.
Very few studies have been conducted on H. stipulacea-associated fish communities, however, a fish feeding experiment at the Kenyan coast showed higher feeding preference for pioneering, short-lived, species such as C. rotundata, S. isoetifolium, and H. stipulacea over “climax,” long-lived, species such as Enhalus acoroides and Thalassodendron ciliatum (Mariani and Alcoverro, 1999). In the GoA, our knowledge of fish associated with local H. stipulacea meadows is limited to the study of Khalaf et al. (2012) that did not find any H. stipulacea-dedicated fish species.
In terms of mega grazers, dugongs have been sighted in the Red Sea (Egyptian and Saudi Arabian coast; Preen, 1989), grazing heavily on H. stipulacea meadows (e.g., in Abu Dabab and Marsa Alam, Egypt). Studies have shown that dugongs prefer “pioneer” seagrasses (Preen and Marsh, 1995), especially those of the genera Halophila and Halodule. While it seems important to quantify these dugong-Halophila interactions, its frequency in most of the native habitat of H. stipulacea meadows is unknown, and reports are anecdotal. This represents an important gap of knowledge in the regions where H. stipulacea is native.
Other studies in the GoA focused on the interactions between local H. stipulacea and invertebrates. The collector urchins (Tripneustes gatilla) were found to graze heavily on H. stipulacea (Hulings and Kirkman, 1982), while Operculina ammonoides was the dominant epiphytic foraminifera on leaves (Oron et al., 2014). In the northern GoA,next generation sequencing (NGS) studies on H. stipulacea-associated microbial communities showed differences across sites and plant compartments (aboveground compartment, i.e., leaves; belowground compartment, i.e., roots and rhizomes), providing an “environmental fingerprint.” In addition to these differences, these studies also found the existence of a “core microbiome” consisting of bacteria that were always present, shared across sites, and independent of the depth or location (Mejia et al., 2016; Rotini et al., 2017). This hints toward the existence of a functional relationship between H. stipulacea and these shared microbes, as in the framework of the “holobiont theory” (Rosenberg et al., 2007; Zilber-Rosenberg and Rosenberg, 2008). When comparing H. stipulacea at different sites (Mejia et al., 2016), this “core microbiome” was composed of the phyla Proteobacteria and Planctomycetes, representing more than 70% of the Operational Taxonomic Units (OTUs) shared on both leaves and roots/rhizomes. Within this phylum, Alphaproteobacteria, Gammaproteobacteria, and Deltaproteobacteria were the most abundant classes: on the leaves, Alphaproteobacteria was the dominant class across all stations (68% of the community), while on the roots/rhizomes no single dominant class was found. Nevertheless, along the gradient, all the sites had a higher number of unique OTUs (i.e., “environmental fingerprint” bacteria), than shared ones, with only 7% of the OTUs shared among different meadows (i.e., “core microbiome”). The microbial diversity in H. stipulacea may contribute to its adaptiveness and may aid its colonization and expansion into new territories. This could be particularly important considering that rhizosphere-associated microbial communities are known to persist on the roots and rhizome segments established in new environments (Coats and Rumpho, 2014; Cúcio et al., 2016).
Surprisingly, regarding the associations of H. stipulacea with other organisms, we seem to know much more from studies in its invaded habitats compared with studies in its native habitats. In the Mediterranean, numerous species of macroalgae associated with H. stipulacea have been reported (Alongi et al., 1993; Rindi et al., 1999; Di Martino et al., 2006), with up to 30 species (mostly Rhodophyta) found in meadows of the Catania harbor (Alongi et al., 1993). The presence of the epiphytic rhodophyte Chondria pygmaea in the Mediterranean is noteworthy (Garbary and Vandermeulen, 1990), raising the possibility of co-migration with its host, H. stipulacea, from the Red Sea (Cormaci et al., 1992). Di Martino et al. (2006) studied temporal variations in the algal assemblage within an H. stipulacea meadow in Syracuse, eastern Sicily, where 110 species, mostly epiphytic Rhodophyta, were found. Nevertheless, Rindi et al. (1999) stated that, in comparison with other Mediterranean seagrass meadows, H. stipulacea has a qualitatively and quantitatively poor epiphytic flora, with the distinct absence of encrusting coralline algae. The fast turnover rate of H. stipulacea leaves was hypothesized to be the main reason for this scarcity (Rindi et al., 1999).
Cancemi et al. (1994) and Acunto et al. (1997) described the animal communities associated with H. stipulacea in eastern Sicily, Italy. Mollusca, Amphipoda, and Decapoda were the most abundant groups in Taormina, Province of Messina (Cancemi et al., 1994), while Polychaeta, Crustacea, and Mollusca were the dominant macrozoobenthos at Vulcano island (the Aeolian Islands, Sicily; Acunto et al., 1997). The fish assemblages associated with H. stipulacea were mainly characterized by the presence of sparids, labrids, and benthic gobiids (Di Martino et al., 2007). Gambi et al. (2009) also observed a school of Sarpa salpa in a small patch of H. stipulacea at 5 m depth but found no signs of direct grazing on its leaves. It is likely that the observed fish grazed on the leaf epiphytes or upon the small macroalgae interspersed between the H. stipulacea shoots. In general, fish assemblages associated with H. stipulacea meadows in the Mediterranean were related to the stable structure of the meadow throughout the year and not with shoot density (Di Martino et al., 2007).
Despite the invasiveness of H. stipulacea in various parts of the world and the established role of the associated microbes, there is hardly any information on H. stipulacea microbiomes in its invaded range. Two recent, yet unpublished, studies in the eastern Mediterranean Sea (Limassol, Cyprus; Conte et al., unpublished), highlighted the influence of the environment on the epiphytic microbial community structure but, at the same time, the capability of H. stipulacea to host a diverse microbial community that may contribute to its invasiveness.
In the Caribbean, H. stipulacea has been reported growing with a range of native and non-native Caribbean marine organisms. Native Chlorophyta algal species, namely Caulerpa spp., Penicillus pyriformis, Penicullus sp., Udotea cyathiformis, and Ulva intestinalis, have all been found growing with H. stipulacea (Steiner and Willette, 2010; Maréchal et al., 2013; Willette et al., 2014). Additionally, Parvocaulis exiguus, an Indo-Pacific green alga potentially introduced by ships, was collected in H. stipulacea beds in St. Eustatius (Maréchal et al., 2013; Steiner and Willette, 2015b). Mats of unidentified cyanobacteria and dinoflagellates have also been reported growing on top of H. stipulacea beds, yet the cause or impact of these mats is unknown (Maréchal et al., 2013; Steiner and Willette, 2015b).
Epifaunal invertebrates occurring on the blades of H. stipulacea in the Caribbean include representative ascidians, annelids, crustaceans, molluscs, and nematodes (Ortea et al., 2012; Willette and Ambrose, 2012; Scheibling et al., 2018). Larger sessile and benthic invertebrates have also been recorded within H. stipulacea beds, including native Strombus gigas (Gastropoda), Pinna carnea (Bivalvia), Astichopus multifidus (Holothuroidea), Oreaster reticularis (Asteroidea) as well as other ascidians, crustaceans, and echinoderms (Willette et al., 2014; Scheibling et al., 2018). The seagrass-grazing urchin Tripneustes ventricosus is often found in H. stipulacea beds (Willette et al., 2014); however, densities of this sea urchin are less than half of that found on native T. testudinum (Scheibling et al., 2018). The dense growth form of H. stipulacea beds does seem to benefit the feeding strategy of O. reticularis, a native Caribbean Sea star whose populations have been decimated elsewhere in the region due to seagrass loss (Scheibling et al., 2018). Ferry et al. (2017) reported the presence of the Indo-West Pacific crab Charybdis hellerii in the island of Martinique, where more than 90% of the specimens found were exclusively on dense beds of H. stipulacea (roughly 0.37 crabs m−2). The absence of C. hellerii on bare sand, coral, and mixed beds of seagrass was attributed to the presence of predators on native substrates. Thus, H. stipulacea may provide a refuge for this introduced crab to thrive in the Caribbean.
Seagrass beds form essential fish habitats in the Caribbean, serving as nurseries for juvenile fish and shelter and foraging grounds for larger fish (Nagelkerken et al., 2001). Thus, the impact of H. stipulacea on native fish is of particular ecological and resource management interest. Using local fish trap methods, Willette and Ambrose (2012), reported significantly larger average fish sizes, and slightly higher fish abundance and species richness on non-native H. stipulacea compared with native S. filiforme beds. This difference in fish abundance and species richness can be attributed to the significantly higher fish prey abundance (namely crustaceans) on H. stipulacea. Juvenile fish, however, were twice more abundant on native S. filiforme than on H. stipulacea, which in part, could be attributed to the latter's much shorter canopy height and thus lower sheltering provision (Willette and Ambrose, 2012). Olinger et al. (2017) conducted an intensive field study focusing on juvenile fish abundances in meadows of H. stipulacea and native seagrasses along St. Thomas (U.S. Virgin Islands). Overall, fish diversity was higher among native seagrasses and over sand than on H. stipulacea in the bays examined. Nocturnal carnivores, however, showed higher abundance in H. stipulacea meadows in contrast to the low abundance of diurnal carnivores and herbivores in the same area, indicating different habitat preferences for different trophic species (Olinger et al., 2017).
Working in Lac Bay, Bonaire, Caribbean Netherlands, Becking et al. (2014a) found that fish abundance was almost half in H. stipulacea meadows compared with that measured in meadows dominated by the native T. testudiunum, in addition to significant differences in the composition of fish species assemblage between the two meadows—Pomacentridae, Mullidae, and Sphyraenidae were present in T. testudinum meadows but absent in the invaded meadows. Becking et al. (2014a) estimated that future expansion and/or persistence of H. stipulacea could possibly result in a diminished nursery function of certain fish species in Lac Bay.
Lastly, southern stingrays, Dasyatis americana, and the sharptail snake-eel Myrichthys breviceps have been reported foraging among H. stipulacea beds (Willette et al., 2014; Scheibling et al., 2018), as has the green turtle Chelonia mydas (Becking et al., 2014b; Christianen et al., 2019). Yet, studies on the interactions between H. stipulacea and these marine megafaunas are limited, hence warrant further examination.
Available information on the H. stipulacea's microbiome in the Caribbean has shown that across bays of Curaçao island there were large distinctions between the below and above ground H. stipulacea compartments and that microbial communities within roots and rhizomes (i.e., the below ground compartment) also differed from the microbial communities found in local sediments (Stuij, 2018). This distinction suggests that H. stipulacea selects and cultures specific microbial communities within its roots and rhizomes. Microbial communities associated with H. stipulacea across five bays in Curaçao did not show strong spatial differentiation, in contrast to the site differentiation demonstrated in the northern GoA (Mejia et al., 2016). In Curaçao, where microbial communities were compared among different seagrass species, the microbial communities associated with H. stipulacea were highly diverse and specific to H. stipulacea but differentiation between below- and above-ground tissue-associated microbiomes was the smallest of the three seagrasses investigated (Stuij, 2018). Sulfur and nitrogen cycling bacterial OTUs were abundant and widespread for all seagrasses including H. stipulacea, suggesting a strong shared functionality among host species-specific microbiomes. Despite, or because, of its recent arrival in the Caribbean, the only study available on microbial communities associated with H. stipulacea seems to suggest that H. stipulacea microbial communities perhaps did not suffer a bottleneck effect, and its high diversity and species specificity may contribute to H. stipulacea's proliferating potential. Clearly much more research in this area is required, preferably combining descriptive and experimental approaches covering micro to global scales.
The ability of H. stipulacea to establish itself first in the Mediterranean and later in the Caribbean makes it an attractive model species for reconstructing its potentially complex history of introductions and studying tolerance and resilience to different environmental conditions at the molecular level (Sakai et al., 2001; Lee, 2002; Davey et al., 2016).
First genetic diversity studies of H. stipulacea employed sequence data of single DNA regions or multi-locus markers that do not allow a precise estimation of population genetic parameters. Ruggiero and Procaccini (2004) found no differentiation in the ITS rDNA regions between H. stipulacea from the Red Sea (native) and Mediterranean (invasive) populations, suggesting that H. stipulacea populations in the Mediterranean originated from the Red Sea (Ruggiero and Procaccini, 2004). This type of molecular marker, however, could not infer whether the introduction occurred once or at multiple times. Interestingly, the same study found a high degree of intra-individual variability in the ITS region, suggesting a high rate of sexual recombination and a slow rate of concerted evolution in the genotypes analyzed. Recent results on the caryology of Mediterranean and the Red Sea individuals of H. stipulacea exclude the existence of polyploidy as a possible cause for the observed intra-individual variability (Gargiulo et al., 2018). Conversely, Varela-Álvarez et al. (2011) found no ITS intra-individual nucleotide diversity in Turkey. The first extensive population recorded in the western Mediterranean basin (i.e., Vulcano Island, Sicily, Italy) has been analyzed by means of randomly amplified polymorphic DNA (RAPD) markers, and high genetic diversity was found together with a clear genetic distinction between shallow and deep stands of the same population (Procaccini et al., 1999a).
The use of more polymorphic and reliable markers would allow addressing ecological questions related to the reproductive and spreading mode as well as track, with more precision, the origin of the invasions.
Seagrasses belong to four/five different families in the subclass Alismatidae (Les et al., 1997). H. stipulacea belongs to the family Hydrocharitaceae, that evolved together with the other major clades 40–78 Mya (Olsen et al., 2016). Seagrass species belonging to different families have different genome size. The genome size of H. stipulacea has been assessed in samples collected from Eilat, northern GoA and it was 12.26 picogram in size (~5.9 Gb; Gargiulo et al., 2018). The value is 2, 6 and 30 times higher than the genome size of P. oceanica, Z. muelleri (~900 Mbp) and Z. marina (~202.3 Mbp), respectively (Procaccini pers. comm. for P. oceanica; Cavallini et al., 1995).
Z. muelleri and Z. marina (Zosteraceaae) are the only two seagrass species for which the complete genome is available at the moment (Lee et al., 2016; Olsen et al., 2016). Although this could represent a potential source of information to scan for the presence of genes that can relate to the H. stipulacea plasticity and invasiveness, the phylogenetic distance between Hydrocharitaceae and Zosteraceaae strongly reduces the power of such analysis. The availability of the H. stipulacea genome would represent an imperative step toward explaining its invasiveness and plasticity.
Understanding the response of H. stipulacea to changes in abiotic factors will facilitate our prediction of the further expansion of this species. One way of comprehending ecological traits is to combine phenotypic and physiological assessments with transcriptomic and their equivalent metabolic pathways (Exadactylos, 2015). With the emergence of molecular profiling and “omics” techniques in seagrass biology (Procaccini et al., 2007; Mazzuca et al., 2013; Davey et al., 2016), the ability to investigate plant responses to biotic and abiotic factors has become more feasible. Recent studies have focused on the response to light, increased water temperature, salinity, and high CO2 levels at the transcriptomic and proteomic levels. These studies have revealed new insights into mechanisms applied by seagrasses to survive under various abiotic stresses (Franssen et al., 2012; Kong et al., 2014; Piro et al., 2015a,b; Kumar et al., 2017; Marín-Guirao et al., 2017; Procaccini et al., 2017). While most of these studies have been performed on the temperate seagrass species Z. marina, P. oceanica, C. nodosa, Z. muelleri, and Z. noltii, there are no reports of any of such study on the tropical H. stipulacea (see Nguyen et al., 2020a).
Although metabolomics is not so much explored in seagrasses (see Gu et al., 2012; Hasler-Sheetal et al., 2016), it holds great potential in combination with transcriptomics and proteomics, in understanding responses to biotic/abiotic stress (Buapet, 2017). Reprogramming of the metabolome under various stresses such as heat (Gu et al., 2012), anoxia (Hasler-Sheetal et al., 2015) and light (Hasler-Sheetal et al., 2016) in seagrasses like Z. marina and Z. noltii were observed. Identifying and studying the regulation of primary and secondary metabolites in H. stipulacea will provide essential insights into the adaptive mechanisms of this seagrass to changing abiotic conditions, significantly increasing our ability to predict the further expansion of this species.
Epigenetic variation is often an important prerequisite and has also been known to facilitate the survival of invasive species in new environments (Schrey et al., 2012; Richards et al., 2017). The extent and the form of such epigenetic plasticity can be an advantage of invasive species over indigenous species (Stachowicz et al., 2002; Chown et al., 2007; Kleynhans et al., 2014).
An interesting area to look into is the shifting in methylation patterns in DNA, i.e., epigenetic variation in response to biotic and abiotic factors (Ardura et al., 2017). Several studies have recently begun to link phenotypic plasticity with changing methylation patterns both in animals and plants (Kardong, 2003; Bossdorf et al., 2008; Zhang et al., 2013) with recent work also on seagrasses (Jueterbock et al., 2019; Ruocco et al., 2019). Genomic tools like whole-genome bisulfite sequencing and ChIP-Seq (to study histone modification) might help to further explain the invasive capability of H. stipulacea as has already been shown in studies of other invasive species, such as in populations of marine invertebrate (Ardura et al., 2017) and insect pests (Jones et al., 2018).
In summary, a combination of metabolomics, proteomics, transcriptomics, and epigenomic studies, in combination with physiological, biochemical, and other more classic indicators (Roca et al., 2016), could provide a holistic view of how H. stipulacea responds to abiotic and biotic stress and in turn help our understanding of this seagrass' invasive capabilities.
Given the widespread of H. stipulacea in its invaded ranges, it is important to understand its potential for future range expansions. This can be done using species distribution models (SDMs) that typically correlate species occurrences with environmental layers (Guisan and Thuiller, 2005; Elith and Leathwick, 2009; Kearney and Porter, 2009). These models are developed using the knowledge on the current distribution of the studied species (the realized niche) which can be potentially projected in space or time to forecast the species distributions within the invaded ranges within a time frame (Fitzpatrick and Hargrove, 2009; Gallien et al., 2010). Applying SDMs for H. stipulacea (Gamliel et al., 2020) using mean annual bottom temperature and net primary productivity as environmental predictors revealed some interesting patterns (Figure 8). The main result was the striking differences in the predicted suitability of the Mediterranean Sea to support H. stipulacea when the model is based on the native (Figure 8A) vs. invaded (Figure 8B) range occurrences. When using the native range occurrences only, the Mediterranean Sea climate appears to be very marginal for this species. However, when using the invaded Mediterranean range occurrences, the entire Mediterranean seems to provide an adequate climate for H. stipulacea. Thus, the climatic niche as estimated from the native range does not represent the full physiological potential of this species (Parravicini et al., 2015). Only after the invasion, when the species may be enjoying reduced biological constraints (the “biotic release” hypotheses) is the full climatic affinity of the species exposed. This means deducing the climatic constraints on H. stipulacea invasion using native range SDMs may severely underestimate invasion potential. Indeed, SDMs work best when the species-realized niches are representative of their fundamental niche, shaped by the underlying physiological constraints. However, if the fundamental and realized niches diverge, correlative SDMs may be far less accurate in predicting the future distribution of the species (Elith et al., 2010; Parravicini et al., 2015).
Figure 8. Halophila stipulacea distribution models based on MaxEnt showing relative habitat suitability based solely on (A) native Indo-Pacific and (B) invasive Mediterranean occurrences. Predicted habitat suitability of H. stipulacea in the Mediterranean Sea under current environmental conditions (left; C,E,G) and forecasted under future (2100) environment (right; D,F,H). Models include either regular SDMs (C,D) or combined physiology-SDMs (E,F). Lower panels (G,H) show the difference between the physiology-SDMs (E,F) and the regular SDMs (C,D). Blue colors (G,H) indicate regions where physiology-SDMs predict high occurrence probability than regular SDMs. Figures adapted from Gamliel et al. (2020).
One way to overcome this limitation is to directly model the fundamental niche, e.g., using physiology. Physiological models take into account the biological mechanism behind the species distribution, and thus can be used more confidently for forecasting the response into novel environmental conditions such as in the invaded ranges (Kearney et al., 2010; Cheaib et al., 2012). The simplest form of these models utilizes a physiological threshold, such as temperature, to predict species future distributions. However, complex physiological models require substantial data on the relationship between the specific environmental conditions and species performance (Buckley et al., 2011; Cheaib et al., 2012).
A promising direction is to combine physiological estimates of species performance and correlative SDMs (Woodin et al., 2013; Martínez et al., 2015; Talluto et al., 2016). Such models may provide more robust forecasts of species distributions in novel climates. Gamliel et al. (2020) used a recently proposed a Bayesian approach that combines SDMs with physiological data (sensu Talluto et al., 2016) to forecast the distribution of H. stipulacea. The physiological data included H. stipulacea‘s change in leaf area at different temperatures (Georgiou et al., 2016), which was used to calculate a temperature response curve. This data was then used as a prior for the coefficients relating environmental predictor to species occurrences within an SDM. RCP (Representative Concentration Pathway) scenarios were used to make predictions for 2100, based on the CCSM45 (Community Climate System Model 4), HadGEM2-ES (Hadley Centre Global Environmental Model 2), and MIROC55 (Interdisciplinary Research on Climate 5) climatic models. Surprisingly, the incorporation of the physiological data did not change the present and predicted future (2100) distribution of this species within the Mediterranean (Figure 8). This likely reflects the wide temperature tolerance of the species (Georgiou et al., 2016). In contrast, the hybridization of SDMs with reproductive window phenology of the invasive seaweed Sargassum muticum did strongly affect the distribution projections of the species under future climate change scenarios.
The results of this modeling exercise suggest that to accurately predict the potential for range expansion of H. stipulacea, as well as its response to climate change, it may be necessary to move beyond both correlative SDMs and simple combination of SMDs with physiology. For example, model performance may be enhanced by using more sophisticated models that incorporate physiological data for other environmental variables beyond temperature (e.g., salinity, turbidity, etc.) as well as phenological information. Conventional SDMs may also be improved by careful selection of occurrences, background data and predictors (Mainali et al., 2015). Further improvements may be achieved with including data on dispersal ability, reproductive features (e.g., reproductive periods, reproductive timing; Chefaoui et al., 2019) and biotic interactions such as competition, predation or facilitation, which are also likely to impact future distributions (Gilman et al., 2010; Kissling et al., 2012; Zarnetske et al., 2012; Wisz et al., 2013; Record et al., 2018).
The motivation for this review comes from the risk of H. stipulacea becoming invasive worldwide. This is a risk that has not been identified so far—at least in terms of research efforts and funding priorities. This species has high plasticity, characteristics typical of an r-strategist species and thus has the potential to become an invader in a wide range of environmental conditions. Indeed, with the recent doubling of the Suez Canal (Galil et al., 2015, 2017) and the ongoing tropicalization and warming of the Mediterranean (Bianchi and Morri, 2003; Borghini et al., 2014), a process that is happening even faster in the eastern Mediterranean (Ozer et al., 2017), H. stipulacea could potentially become more prevalent in these waters in the coming years. This is even more probable considering that conditions in the Mediterranean Sea are becoming less favorable for its temperate, native, seagrass species (Jordà et al., 2012; Chefaoui et al., 2018; Marín-Guirao et al., 2018; Savva et al., 2018) and more welcoming of tropical species (Sghaier et al., 2014; Georgiou et al., 2016; Gerakaris et al., 2020). The traits that make H. stipulacea amenable to invasiveness include rapid horizontal growth and leaf turnover rates (Wahbeh, 1984; Willette and Ambrose, 2012), tolerance to a wide range of environmental conditions, including salinity (Oscar et al., 2018), light (Sharon et al., 2009, 2011a,b), and temperatures (Georgiou et al., 2016). This species has the ability to grow from very small fragments (Willette et al., 2020). It is capable of maintaining high-density meadows in both high and relatively low nutrient levels (Beca-Carretero et al., 2020) in different types of sediments (from soft mud to the nutrient-limited carbonate sediments). It has physiological plasticity (e.g., an efficient Ci-acquisition system, changes in leaf area, chloroplast clumping), and, probably, is capable of interacting with many different microbial species.
At least for the Caribbean, it seems that the invasive H. stipulacea with its short leaves and relatively smaller roots, cannot replace all the traits and services provided by the native Caribbean seagrass species that it displaces (e.g., wave attenuation and protection from storms, habitat complexity and use of meadows as fish nurseries), potentially entailing changes to the economic and social benefits that seagrasses provide in this region (reviewed by Viana et al., 2019).
The aim of this review was to evaluate the existing knowledge on the biological, ecological, physiological, biochemical, and molecular traits of H. stipulacea in its native and invaded habitats. This framework allowed us to (i) compare traits and environmental conditions across basins, (ii) discuss the possible environmental conditions and plant mechanisms involved in its invasion, (iii) assess the impact of H. stipulacea on native seagrasses and ecosystem functioning in the invaded regions, and (iv) predict the ability of this species to invade European and transoceanic coastal waters.
This review has also allowed us to identify several knowledge gaps, highlighted throughout the text, that need to be addressed in the future: The ecological interactions between H. stipulacea meadows and grazers (e.g., from small species up to dugongs) have been investigated mostly in the Caribbean, but we know very little about fish associated with H. stipulacea meadows in the Red and Mediterranean Seas. We know little about the functional role of the associated microbiome—do they contribute to the invasive success of their host? We lack data on the genetic diversity and connectivity of H. stipulacea populations. For these knowledge gaps, developing “omic” tools would be of particular relevance. We lack data on thermal tolerance of H. stipulacea populations (that could be collected from mesocosm experiments, modeling or in situ long term data) and how these compare with other neighboring seagrass or other species (e.g., corals and sponges). For this, the use of new technologies and innovative approaches (e.g., mesocosm common garden experiments, isotopic, biochemical, ecological, and molecular markers) will be mandatory. We need better niche models, accurate SDMs or climate envelope distribution models—these could help in predicting future expansions of H. stipulacea's distributions (e.g., what regions and ecological niches are likely to be invaded?) and the impacts of such changes (can we even control such expansions?). The word cloud highlights that we know little about ecosystem services directly associated with H. stipulacea in both native and invaded habitats. We need to compare reproductive seasons (timing, duration, female/male ratios) among different sites in both native and invaded ranges.
Our review identifies regions for which we have even larger knowledge gaps—we know very little about populations of H. stipulacea in many parts of its native range. Similarly, we lack studies on seasonal changes in Mediterranean populations, where quantitative data dealing temporal changes of H. stipulacea don't exist. Finally, we conclude that a coordinated mapping of H. stipulacea and permanent monitoring efforts are needed across native and invaded distribution areas. The issues at stake entail the involvement of biologists, ecologists, modelers, managers, and local stakeholders. In the current scenarios of climate change and exponential human pressure on coastal areas, long-term monitoring is needed to record changes in H. stipulacea over time with associated communities to contextualize current observations in native (Red Sea), invaded (Mediterranean and Caribbean Seas), and prospective distributional ranges.
From the perspective of future management efforts in regions where H. stipulacea might become invasive, we do not believe it would be possible to remove newly discovered plants—unless on a very small scale (<10 m2). Due to its rapid clonal growth, prolonged survival as fragments, and its ability to regrow from small fragments (Smulders et al., 2017; Willette et al., 2020), we need to take into account that if H. stipulacea plants are pulled out, tiny fragments can survive and settle in other places. Perhaps, more efforts should be placed on prevention of loss of native seagrasses. We know that when native seagrass disappears, H. stipulacea can rapidly colonize the available area (especially in the Caribbean). But if native seagrass is still there, this probably is not that easy for H. stipulacea. Indeed, this was demonstrated by a study by Steiner and Willette (2015b) in Dominica, where so long as S. filiforme had a cover of <45% it was able to resist invasion by H. stipulacea (S. filiforme “strongholds”), but if S. filiforme was below this, there was space for H. stipulacea to come in and S. filiforme eventually was displaced (Steiner and Willette, 2015b). The comparison of the invasiveness of H. stipulacea in protected vs. unprotected MPAs has yet to be done, but the mere ban of fishing in seagrass meadows located in Caribbean island nations might help slowing invasiveness of H. stipulacea since it has been shown that wooden and metal fish traps commonly used by fishermen in the eastern Caribbean facilitate local dissemination of H. stipulacea (Willette and Ambrose, 2012; discussed above). While protecting native seagrasses from global warming is difficult, they can be protected from local stressors such as physical damage (e.g., anchoring), and more importantly from eutrophication. While setting up marine protected areas (MPAs) has become a fundamental strategy in marine conservation, their effectiveness on seagrass meadows has been relatively less studied (reviewed by Alonso Aller et al., 2017). Seagrass MPAs in tropical areas were shown to increase the temporal stability of seagrass-associated fish communities, which in turn enhanced herbivory followed by enhanced seagrass growth rates (Alonso Aller, 2018). However, MPAs were not able to protect seagrasses from land-use effects, highlighting the importance of coupling seagrass conservation with land-based management. Indeed, both Björk et al. (2008) and Waycott et al. (2009) have identified nutrient inputs as the number one threat to seagrass ecosystems worldwide. Thus, in parallel to mapping and monitoring changes in areas where H. stipulacea meadows have already invaded, it might be more important, in areas where this species has not yet completely overtaken native seagrasses, to apply improved land-based management strategies that would reduce potential eutrophication and prevent loss of water quality, stressors that would enhance such invasiveness.
All datasets generated for this study are included in the article/Supplementary Material.
GW and GR initiated the Euro Marine workshop that kicked off this review. GW led the writing and editing helped by IV. TA-G, BV, and BM led the review of published studies (Table 1, Table S1). RS-T prepared the maps. All authors contributed to writing sections: SB (responses to light, carbon sources), PB-C (biochemistry), LM, AE, and AR (microbial studies), MO (tolerance to salinity), GR (invasiveness, H. stipulacea as an invader), JB and IG (modelling), AA (nutrient uptake), AA and GP (H. stipulacea in the Mediterranean), DW, AE, and KC (H. stipulacea in the Caribbean), MO and GP (developing omic tools). SB, DW, IV, and AR helped with editing final versions.
The workshop that begun this review was supported by Euro Marine, the Inter-University Institute in Eilat (IUI), Israel Oceanographic and Limnological Research (ILOR), The Israel Nature and Parks Authority (INPA), and the ADSSC (the Dead Sea-Arava Science Center). IV was awarded a postdoctoral fellowship of the Leibniz-DAAD postdoctoral programme (Germany) and Xunta de Galicia (Consellería de Cultura, Educación e Ordenación Universitaria). AE and AA were individually supported by FCT in the form of SFRH/BPD/107878/2015 and UIDB/04326/2020 within CEECINST/00114/2018, and SFRH/BPD/91629/2012, respectively.
The authors declare that the research was conducted in the absence of any commercial or financial relationships that could be construed as a potential conflict of interest.
This review was initiated at the EuroMarine (European marine research network) foresight workshop entitled Developing an integrated framework for studying H. stipulacea, the world's first globally invasive marine angiosperm (Seagrass) held in Eilat in July 2016. The workshop included some 25 participants that worked on different aspects of H. stipulacea, in both its native and invasive habitats. We thank the Inter-University Institute in Eilat (IUI) for hosting this meeting. Finally, we thank ongoing support to the ADSSC by the Israeli Ministry for Science and Technology (MOST).
The Supplementary Material for this article can be found online at: https://www.frontiersin.org/articles/10.3389/fmars.2020.00300/full#supplementary-material
Ackerman, J. D. (2000). Abiotic pollen and pollination: ecological, functional, and evolutionary perspectives. Plant Syst. Evol. 222, 167–185. doi: 10.1007/BF00984101
Acunto, S., Maltagliati, F., Rindi, F., Rossi, F., and Cinelli, F. (1995). Osservazioni su una prateria di Halophila stipulacea (Forsskål and Niebuhr) aschers. (Hydrocharitaceae) nel mar Tirreno Meridionale. Atti Soc. Tosc. Sci. Nat. Mem. Ser. B 102, 19–22.
Acunto, S., Maltagliati, F., Rindi, F., Rossi, F., Cinelli, F., and Lardicci, C. (1997). “Indagine su una prateria di Halophila stipulacea (Forssk.) aschers. (Hydrocharitaceae) dell'Isola di Vulcano,” in Atti 12 Congresso dell'Associazione Italiana di Oceanologia e Limnologia, ed M. Piccazzo (Genoa: AIOL), 51–60.
Aleem, A. A. (1979). A contribution to the study of seagrasses along the Red Sea coast of Saudi Arabia. Aquat. Bot. 7, 71–78. doi: 10.1016/0304-3770(79)90009-3
Aleem, A. A. (1980). Contributions to the study of the marine algae of the Red Sea. IV-algae and seagrasses inhabiting the Suez Canal. Bull. Fac. Sci. King Abdul Aziz. Univ. 4, 31–89.
Aleem, A. A. (1984). The Suez Canal as a habitat and pathway for marine algae and seagrasses. Deep Sea Res. A. Oceanograph. Res. Pap. 31, 901–918. doi: 10.1016/0198-0149(84)90047-5
Alexandre, A., Georgiou, D., and Santos, R. (2014). Inorganic nitrogen acquisition by the tropical seagrass Halophila stipulacea. Mar. Ecol. 35, 387–394. doi: 10.1111/maec.12128
Alongi, G., Cormaci, M., and Pizzuto, F. (1993). La macroflora epifita delle foglie di Halophila stipulacea (Forssk.) aschers. del Porto di Catania. Biol. Mar. Mediterr. 1, 287–288.
Alonso Aller, E. (2018). Effects of marine protected areas on tropical seagrass ecosystems (Ph.D. thesis). Department of Ecology, Environment and Plant Sciences, Stockholm University, Stockholm, Sweden, 54. Available online at: http://urn.kb.se/resolve?urn=urn:nbn:se:su:diva-154966 (accessed September 14, 2019).
Alonso Aller, E., Jiddawi, N. S., and Eklöf, J. S. (2017). Marine protected areas increase temporal stability of community structure, but not density or diversity, of tropical seagrass fish communities. PLoS ONE 12:e0183999. doi: 10.1371/journal.pone.0183999
Alpinar, K. (1987). A new record for Turkish flora Halophila stipulacea. Istanbul Universitesi Eczacilik Fakultesi Mecmuasi 23, 83–84.
Al-Rousan, S., Al-Horani, F., Eid, E., and Khalaf, M. (2011). Assessment of seagrass communities along the Jordanian coast of the Gulf of Aqaba, Red Sea. Mar. Biol. Res. 7, 93–99. doi: 10.1080/17451001003660319
Al-Salaymeh, A. (2006). Modelling of global daily solar radiation on horizontal surfaces for Amman city. J. Eng. Res. 11, 49–56.
Al-Sayed, R. (2013). “Status of renewable energy in Jordan,” in 1st International Conference and Exhibition on the Applications of Information Technology to Renewable Energy Processes and Systems, IEEE (Amman), 66–72. doi: 10.1109/IT-DREPS.2013.6588153
Angel, D. L., Eden, N., and Susel, L. (1995). “The influence of environmental variables on Halophila stipulacea growth,” in Improving the Knowledge Base in Modern Aquaculture, eds H. Rosenthal, B. Moav, and H. Gordin (Ghent: European Aquaculture Society Special Publication), 103–128.
Anton, A., Hendriks, I. E., Marbà, N., Krause-Jensen, D., Garcias-Bonet, N., and Duarte, C. M. (2018). Iron deficiency in seagrasses and macroalgae in the Red Sea is unrelated to latitude and physiological performance. Front. Mar. Sci. 5:74. doi: 10.3389/fmars.2018.00074
Ardura, A., Zaiko, A., Moran, P., Planes, S., and Garcia-Vazquez, E. (2017). Epigenetic signatures of invasive status in populations of marine invertebrates. Nat. Sci. Rep. 7:42193. doi: 10.1038/srep42193
Badalamenti, F., Ben Amer, I., Dupuy de la Grandrive, R., Foulquie, M., Milazzo, M., and Sghaier, Y. (2011). “Scientific field survey report for the development of Marine Protected Areas in Libya,” in Part of the UNEP/MAP-RAC/SPA Project to Develop the Inventorying, Mapping and Monitoring of Posidonia meadows in four Mediterranean Countries: Algeria, Libya, Tunisia, and Turkey. Available online at: www.rac-spa.org/sites/default/files/doc_medmpanet/final_docs_libya/29_field_survey_ain_al_ghazala_bomba_libya.pdf
Bagwell, C. E., Rocque, J. R., Smith, G. W., Polson, S. W., Friez, M. J., Longshore, J. W., et al. (2002). Molecular diversity of diazotrophs in oligotrophic tropical seagrass bed communities. FEMS Microbiol. Ecol. 39, 113–119. doi: 10.1111/j.1574-6941.2002.tb00912.x
Beca-Carretero, P., Guihéneuf, F., Marín-Guirao, L., Bernardeau-Esteller, J., García-Muñoz Stengel, D. B., and Ruiz, J. M. (2018). Effects of an experimental heat wave on fatty acid composition in two Mediterranean seagrass species. Mar. Pollut. Bull. 134, 27–37. doi: 10.1016/j.marpolbul.2017.12.057
Beca-Carretero, P., Guihéneuf, F., Winters, G., and Stengel, D. B. (2019). Depth-induced adjustment of fatty acid and pigment composition suggests high biochemical plasticity in the tropical seagrass Halophila stipulacea. Mar. Ecol. Prog. Ser. 608, 105–117. doi: 10.3354/meps12816
Beca-Carretero, P., Rotini, A., Mejia, A. Y., Migliore, L., Vizzini, S., and Winters, G. (2020). Halophila stipulacea descriptors in the native area (Red Sea): a baseline for future comparisons with native and non-native populations. Mar. Environ. Res. 153:104828. doi: 10.1016/j.marenvres.2019.104828
Becking, L., Bussel, T., Debrot, A., and Christianen, M. (2014b). First record of a caribbean Green Turtle (Chelonia mydas) grazing on invasive seagrass (Halophila stipulacea). Caribb. J. Sci. 48, 162–163. doi: 10.18475/cjos.v48i3.a05
Becking, L. E., van Bussel, T., Engel, M. S., Christianen, M. J. A., and Debrot, A. O. (2014a). Proximate Response of Fish, Conch, and Sea Turtles to the Presence of the Invasive Seagrass Halophila Stipulacea in Bonaire. Report number C118/14, IMARES Wageningen UR, 35.
Beer, S., Björk, M., and Beardall, M. (2014). Photosynthesis in the Marine Environment. New Jersey, NJ: Wiley Blackwell, 224.
Beer, S., Björk, M., Hellblom, F., and Axelsson, L. (2002). Inorganic carbon utilization in marine angiosperms (seagrasses). Funct. Plant Biol. 29, 349–354. doi: 10.1071/PP01185
Beer, S., Shomer-Ilan, A., and Waisel, Y. (1980). Carbon metabolism in seagrasses: II. patterns of photosynthetic CO2 incorporation. J. Exp. Bot. 31, 1019–1026. doi: 10.1093/jxb/31.4.1019
Beer, S., Vilenkin, B., Weil, A., Veste, M., Susel, L., and Eshel, A. (1998). Measuring photosynthetic rates in seagrasses by Pulse Amplitude Modulated (PAM) fluorometry. Mar. Ecol. Prog. Ser. 174, 293–300. doi: 10.3354/meps174293
Bianchi, C. N., and Morri, C. (2003). Global sea warming and “tropicalization” of the Mediterranean sea: biogeographic and ecological aspects. Biogeographia 24, 319–329. doi: 10.21426/B6110129
Biliotti, M., and Abdelahad, N. (1990). Halophila stipulacea (Forssk.) aschers. (Hydrocharitaceae): espèce nouvelle pour l'Italie. Posidonia Newsletter 3, 23–26.
Björk, M., Short, F., Mcleod, E., and Beer, S. (2008). “Managing seagrasses for resilience to climate change,” in IUCN Resilience Science Group Working Paper Series - No 3 (Gland: IUCN), 56.
Bloomfield, A. L., and Gillanders, B. M. (2005). Fish and invertebrate assemblages in seagrass, mangrove, saltmarsh, and nonvegetated habitats. Estuaries 28, 63–77. doi: 10.1007/BF02732754
Borghini, M., Bryden, H., Schroeder, K., Sparnocchia, S., and Vetrano, A. (2014). The Mediterranean is becoming saltier. Ocean Sci. 10, 693–700. doi: 10.5194/os-10-693-2014
Bossdorf, O., Richards, C., and Pigliucci, M. (2008). Epigenetics for ecologists. Ecol. Lett. 11, 106–115.
Buapet, P. (2017). “Photobiology of seagrasses: a systems biology perspective,” in Systems Biology of Marine Ecosystems, eds M. Kumar, and P. J. Ralph (Cham: Springer), 133–165. doi: 10.1007/978-3-319-62094-7_7
Buckley, L. B., Waaser, S. A., MacLean, H. J., and Fox, R. (2011). Does including physiology improve species distribution model predictions of responses to recent climate change? Ecology 92, 2214–2221. doi: 10.1890/11-0066.1
Buckley, Y. M., and Csergo, A. M. (2017). Predicting invasion winners and losers under climate change. Proc. Natl. Acad. Sci. U.S.A. 114, 4040–4041. doi: 10.1073/pnas.1703510114
Campbell, A. H., Marzinelli, E. M., Gelber, J., and Steinberg, P. D. (2015a). Spatial variability of microbial assemblages associated with a dominant habitat-forming seaweed. Front. Microbiol. 6:230. doi: 10.3389/fmicb.2015.00230
Campbell, A. M., Fleisher, J., Sinigalliano, C., White, J. R., and Lopez, J. V. (2015b). Dynamics of marine bacterial community diversity of the coastal waters of the reefs, inlets, and wastewater outfalls of southeast Florida. Microbiol. Open 4, 390–408. doi: 10.1002/mbo3.245
Campbell, J. E., Lacey, E. A., Decker, R. A., Crooks, S., and Fourqurean, J. W. (2015c). Carbon storage in seagrass beds of Abu Dhabi, United Arab Emirates. Estuar. Coasts 38, 242–251. doi: 10.1007/s12237-014-9802-9
Cancemi, G., Terlizzi, A., Scipione, M., and Mazzella, L. (1994). Il prato ad Halophila stipulacea (Forssk.) aschers. di G. Naxos (Sicilia): caratteristiche della planta e del popolamento a fauna vagile. Biol. Mar. Mediterr. 1, 401–402.
Cardini, U., van Hoytema, N., Bednarz, V. N., Al-Rshaidat, M. M. D., and Wild, C. (2018). N2 fixation and primary productivity in a red sea Halophila stipulacea meadow exposed to seasonality. Limnol. Oceanogr. 63, 786–798. doi: 10.1002/lno.10669
Cavallini, A., Natali, L., Giordani, T., Polizzi, E., Balestri, E., Cinelli, F., et al. (1995). Cytophotometric and biochemical characterization of Posidonia oceanica L. (potamogetonaceae) genome. Caryologia 48, 201–209. doi: 10.1080/00087114.1995.10797330
Charlesworth, D. (2002). Plant sex determination and sex chromosomes. Heredity 88, 94–101. doi: 10.1038/sj.hdy.6800016
Cheaib, A., Badeau, V., Boe, J., Chuine, I., Delire, C., Dufrêne, E., et al. (2012). Climate change impacts on tree ranges: model intercomparison facilitates understanding and quantification of uncertainty. Ecol. Lett. 15, 533–544. doi: 10.1111/j.1461-0248.2012.01764.x
Chefaoui, R. M., Duarte, C. M., and Serrão, E. A. (2018). Dramatic loss of seagrass habitat under projected climate change in the Mediterranean Sea. Glob. Change Biol. 24, 4919–4928. doi: 10.1111/gcb.14401
Chefaoui, R. M., Serebryakova, A., Engelen, A. H., Viard, F., and Serrão, E. A. (2019). Integrating reproductive phenology in ecological niche models changed the predicted future ranges of a marine invader. Divers. Distrib. 25, 688–700. doi: 10.1111/ddi.12910
Chiquillo, K. L., Barber, P. H., and Willette, D. A. (2018). Fruits and flowers of the invasive seagrass Halophila stipulacea in the Caribbean sea. Bot. Mar.62, 109–112. doi: 10.1515/bot-2018-0052
Chown, S. L., Slabber, S., McGeoch, M. A., Janion, C., and Leinaas, H. P. (2007). Phenotypic plasticity mediates climate change responses among invasive and indigenous arthropods. Proc. R. Soc. B 274, 2531–2537. doi: 10.1098/rspb.2007.0772
Christianen, M. J. A., Smulders, F. O. H., Engel, M. S., Nava, M. I., Willis, S., Debrot, A. O., et al. (2019). Megaherbivores may impact expansion of invasive seagrass in the Caribbean. J. Ecol. 107, 45–57. doi: 10.1111/1365-2745.13021
Coats, V. C., and Rumpho, M. E. (2014). The rhizosphere microbiota of plant invaders: an overview of recent advances in the microbiomics of invasive plants. Front. Microbiol. 5:368. doi: 10.3389/fmicb.2014.00368
Coppejans, E., Beeckman, H., and De Wit, M. (1992). The seagrass and associated macroalgal vegetation of Gazi Bay (Kenya). Hydrobiologia 247, 59–75. doi: 10.1007/BF00008205
Cormaci, M., Furnari, G., Alongi, G., Dinaro, R., and Pizzuto, F. (1992). On the occurrence in Sicily of three florideophyceae new to the Mediterranean sea. Bot. Mar. 35, 447–450. doi: 10.1515/botm.1992.35.5.447
Costanza, R., de Groot, R., Sutton, P., van der Ploeg, S., Anderson, S. J., Kubiszewski, I., et al. (2014). Changes in the global value of ecosystem services. Glob. Environ. Chang. 26, 152–158. doi: 10.1016/j.gloenvcha.2014.04.002
Cúcio, C., Engelen, A. H., Costa, R., and Muyzer, G. (2016). Rhizosphere microbiomes of european seagrasses are selected by the plant, but are not species specific. Front. Microbiol. 7:440. doi: 10.3389/fmicb.2016.00440
Davey, P. A., Pernice, M., Sablok, G., Larkum, A., Lee, H. T., Golicz, A., et al. (2016). The emergence of molecular profiling and omics techniques in seagrass biology; furthering our understanding of seagrasses. Funct. Integr. Genomics 16, 465–480. doi: 10.1007/s10142-016-0501-4
De Troch, M., Fiers, F., and Vincx, M. (2001). Alpha and beta diversity of harpacticoid copepods in a tropical seagrass bed: the relation between diversity and species1 range size distribution. Mar. Ecol. Prog. Ser. 215, 225–236. doi: 10.3354/meps215225
De Troch, M., Fiers, F., and Vincx, M. (2003). Niche segregation and habitat specialization of harpacticoid copepods in a tropical seagrass bed. Mar. Biol. 142, 345–355. doi: 10.1007/s00227-002-0958-7
Debrot, A., Hylkema, A., Vogelaar, W., Meesters, H., Engel, M., Leon, R., et al. (2012). Baseline Surveys of Lac Bay Benthic and Fish Communities, Bonaire. Wageningen: IMARES, 1–52. Available online at: https://library.wur.nl/WebQuery/wurpubs/433547 (accessed October 30, 2019).
Den Hartog, C. (1970). The Sea-Grasses of the World. Amsterdam; London: North-Holland Publishing Company, 276.
Den Hartog, C. (1972). Range extension of Halophila stipulacea (hydrocharitaceae) in the Mediterranean. Blumea Biodivers. Evol. Biogeograp. Plants 20, 154–156.
Den, H. C. (1970). The sea-grasses of the world. Verh. Kon. Ned. Akad. Wet. Afd. Natuurkunde 59:275.
Di Martino, V., Stancanelli, B., and Molinari, A. (2007). Fish community associated with Halophila stipulacea meadow in the Mediterranean Sea. Cybium Int. J. Ichthyol. 31, 451–458.
Di Martino, V., Blundo, M. C., and Tita, G. (2006). The Mediterranean introduced seagrass Halophila stipulacea in eastern Sicily (Italy): temporal variations of the associated algal assemblage. Vie et Milieu 56, 223–230.
Drew, E. A. (1979). Physiological aspects of primary production in seagrasses. Aquat. Bot. 7, 139–150. doi: 10.1016/0304-3770(79)90018-4
Duarte, C. M. (1991). Seagrass depth limits. Aquat. Bot. 40, 363–377. doi: 10.1016/0304-3770(91)90081-F
Duarte, C. M. (1992). Nutrient concentration of aquatic plants: patterns across species. Limnol. Oceanograp. 37, 882–889. doi: 10.4319/lo.1992.37.4.0882
El Shaffai, A. (2016). “Field guide to seagrasses of the Red sea,” in International Union for the Conservation of Nature, eds A. Rouphael, and A. Abdulla (Gland: IUCN and Courbevoie: Total Foundation), 56. Available online at: https://www.iucn.org/content/field-guide-seagrasses-red-sea-0 (accessed September 14, 2019).
Elith, J., Kearney, M., and Phillips, S. (2010). The art of modelling range-shifting species. Methods Ecol. Evol. 1, 330–342. doi: 10.1111/j.2041-210X.2010.00036.x
Elith, J., and Leathwick, J. R. (2009). Species distribution models: ecological explanation and prediction across space and time. Annu. Rev. Ecol. Evol. Syst. 40, 677–697. doi: 10.1146/annurev.ecolsys.110308.120159
Exadactylos, A. (2015). “Molecular approach of seagrasses response related to tolerance acquisition to abiotic stress,” in Molecular Approaches to Genetic Diversity, eds M. Caliskan, H. Kavakh, G. C. and B. Ozcan. (London: IntechOpen), 75–91. doi: 10.5772/59425
Ferry, R., Buske, Y., Poupin, J., and Smith-Ravin, J. (2017). First record of the invasive swimming crab Charybdis hellerii (A. Milne Edwards, 1867) (Crustacea, Portunidae) off Martinique, French Lesser Antilles. Bioinvasions Rec. 6, 239–247. doi: 10.3391/bir.2017.6.3.09
Fitzpatrick, M., and Hargrove, W. (2009). The projection of species distribution models and the problem of non-analog climate. Biodivers. Conserv. 18, 2255–2261. doi: 10.1007/s10531-009-9584-8
Forsskål, P., and Niebuhr, C. (1775). Flora Aegyptiaco-Arabica : sive descriptiones plantarum quas per Aegyptum inferiorem et Arabiam felicem. Hauniae: Ex officina Mlleri.
Fourqurean, J. W., Duarte, C. M., Kennedy, H., Marbà, N., Holmer, M., Mateo, M. A., et al. (2012). Seagrass ecosystems as a globally significant carbon stock. Nat. Geosci. 5, 505–509. doi: 10.1038/ngeo1477
Fox, H. M. (1926). Cambridge expedition to the Suez Canal, 1924. Trans. Zool. Soc. Lond. 22, 1–64. doi: 10.1111/j.1096-3642.1926.tb00320.x
Franssen, S., Gu, J., Bergmann, N., Winters, G., Klostermeier, U., Rosenstiel, P., et al. (2012). Transcriptomic resilience to global warming in the seagrass Zostera marina, a marine foundation species. Proc. Nat. Acad. Sci. U.S.A. 108, 19276–19281. doi: 10.1073/pnas.1107680108
Fritsch, C. (1895). Uber die auffindung einer marinen hydrocharidee im mittelmeer. Verhandlungen der Zoologisch Botanischen Gesamten Wien 45, 104–106.
Gab-Alla, A. A. (2001). Ecological status of the seagrass community in Sharm El-Moyia Bay (Gulf of Aqaba, Red Sea) after oil pollution in 1999. J. King Abdul. Univ. Mar. Sci. 12, 231–239. doi: 10.4197/mar.12-1.17
Galil, B., Marchini, A., Occhipinti-Ambrogi, A., and Ojaveer, H. (2017). The enlargement of the Suez Canal—erythraean introductions and management challenges. Manag.Biol. Invasion 8, 141–152. doi: 10.3391/mbi.2017.8.2.02
Galil, B. S., Boero, F., Campbell, M. L., Carlton, J. T., Cook, E., Fraschetti, S., et al. (2015). ‘Double trouble': the expansion of the Suez Canal and marine bioinvasions in the Mediterranean Sea. Biol. Invasions 17, 973–976. doi: 10.1007/s10530-014-0778-y
Gallien, L., Münkemüller, T., Albert, C., Boulangeat, I., and Thuiller, W. (2010). Predicting potential distributions of invasive species: where to go from here? Divers. Distrib. 16, 331–342. doi: 10.1111/j.1472-4642.2010.00652.x
Gambi, M. C., Barbieri, F., and Bianchi, C. N. (2009). New record of the alien seagrass Halophila stipulacea (Hydrocharitaceae) in the western Mediterranean: a further clue to changing Mediterranean sea biogeography. Mar. Biodivers. Rec. 2:e84. doi: 10.1017/S175526720900058X
Gambi, M. C., Gaglioti, M., and Barbieri, F. (2018). Sometimes they come back: the re-colonization of the alien seagrass Halophila stipulacea (Forsskål and Niebuhr) Ascherson, 1867 (Hydrocharitaceae) in the Palinuro Harbor (Tyrrhenian Sea, Italy). Bioinvasions Rec. 7, 215–221. doi: 10.3391/bir.2018.7.3.01
Gamliel, I., Buba, Y., Guy-Haim, T., Garval, T., Willette, D., Rilov, G., et al. (2020). Incorporating physiology into species distribution models moderates the projected impact of warming on selected Mediterranean marine species. Ecography. 43, 1–17. doi: 10.1111/ecog.04423
Garbary, D., and Vandermeulen, H. (1990). Chondria pygmaea sp. nov. (Rhodomelaceae, Rhodophyta) from the Gulf of Aquaba, Red sea. Bot. Mar. 33, 311–318. doi: 10.1515/botm.1990.33.4.311
Gargiulo, G. M., Vilardo, I., Cambrea, G., Gemelli, F., and Crosca, A. (2018). Karyomorphology and DNA quantification in the marine angiosperm Halophila stipulacea (Forsskål and Niebuhr) Ascherson from Mediterranean and Red seas. Aquat. Bot. 148, 1–9. doi: 10.1016/j.aquabot.2018.04.001
Georgiou, D., Alexandre, A., Luis, J., and Santos, R. (2016). Temperature is not a limiting factor for the expansion of Halophila stipulacea throughout the Mediterranean sea. Mar. Ecol. Prog. Ser. 544, 159–167. doi: 10.3354/meps11582
Gerakaris, V., Lardi, P-L., and Issaris, Y. (2020). First record of the tropical seagrass species Halophila decipiens ostenfeld in the Mediterranean sea. Aquat. Bot. 160:103151. doi: 10.1016/j.aquabot.2019.103151
Gerakaris, V., and Tsiamis, K. (2015). Sexual reproduction of the Lessepsian seagrass Halophila stipulacea in the Mediterranean sea. Bot. Mar.58, 51–53. doi: 10.1515/bot-2014-0091
Gilman, S. E., Urban, M. C., Tewksbury, J., Gilchrist, G. W., and Holt, R. D. (2010). A framework for community interactions under climate change. Trends Ecol. Evol. 25, 325–331. doi: 10.1016/j.tree.2010.03.002
Gu, J., Weber, K., Klemp, E., Winters, G., Franssen, S. U., Wienpahl, I., et al. (2012). Identifying core features of adaptive metabolic mechanisms for chronic heat stress attenuation contributing to systems robustness. Integr. Biol. 4, 480–493. doi: 10.1039/c2ib00109h
Guisan, A., and Thuiller, W. (2005). Predicting species distribution: offering more than simple habitat models. Ecol. Lett. 8, 993–1009. doi: 10.1111/j.1461-0248.2005.00792.x
Hamisi, M., Lyimo, T., Muruke, M. H. S., and Bergman, B. (2009). Nitrogen fixation by epiphytic and epibenthic diazotrophs associated with seagrass meadows along the Tanzanian coast, Western Indian ocean. Aquat. Microb. Ecol. 57, 33–42. doi: 10.3354/ame01323
Hanson, C., Hyndes, G., and Wang, S. (2010). Differentiation of benthic marine primary producers using stable isotopes and fatty acids: implications to food web studies. Aquat. Bot. 93, 114–122. doi: 10.1016/j.aquabot.2010.04.004
Hasler-Sheetal, H., Castorani, M., Glud, R., Canfield, D., and Holmer, M. (2016). Metabolomics reveals cryptic interactive effects of species interactions and environmental stress on nitrogen and sulfur metabolism in seagrass. Environ. Sci. Technol. 50, 11602–11609. doi: 10.1021/acs.est.6b04647
Hasler-Sheetal, H., Fragner, L., Holmer, M., and Weckwerth, W. (2015). Diurnal effects of anoxia on the metabolome of the seagrass Zostera marina. Metabolomics 11, 1208–1218. doi: 10.1007/s11306-015-0776-9
Hulings, N. C., and Kirkman, H. (1982). Further observations and data on seagrasses along the Jordanian and Saudi Arabian coasts of the Gulf of Aqaba. Tethys 10, 218–220.
Issel, R. (1928). Cenni sui risultati ottenuti dalla missione zoologica nel dodecaneso 1926 per quanto concerne la fauna e la flora marine, con alcune osservazioni generali. Arch. Zool. Ital. 12, 259–271.
Jacobs, R., and Dicks, B. (1985). Seagrasses in the Zeit Bay area and at Ras Gharib (Egyptian Red sea coast). Aquat. Bot. 23, 137–147. doi: 10.1016/0304-3770(85)90061-0
Jagtap, T. (1991). Distribution of seagrasses along the Indian coast. Aquat. Bot. 40, 379–386. doi: 10.1016/0304-3770(91)90082-G
Jones, C. M., Lim, K. S., Chapman, J. W., and Bass, C. (2018). Genome-wide characterization of DNA methylation in an invasive lepidopteran pest, the cotton bollworm Helicoverpa armigera. G3 (Bethesda). 8, 779–787. doi: 10.1534/g3.117.1112
Jordà, G., Marbà, N., and Duarte, C. M. (2012). Mediterranean seagrass vulnerable to regional climate warming. Nat. Clim. Chang. 2, 821–824. doi: 10.1038/nclimate1533
Jueterbock, A., Boström, C., James, A. C., Olsen, J., Kopp, M., Dhanasiri, A., et al. (2019). Methylation variation promotes phenotypic diversity and evolutionary potential in a millenium-old clonal seagrass meadow. bioRxiv 787754. doi: 10.1101/787754
Kamermans, P., Hamminga, M. A., Tack, J. F., Mateo, M., Marb,à, N., Mtolera, M. S. P., et al. (2002). Groundwater effects on diversity and abundance of lagoonal seagrasses in Kenya and on Zanzibar Island (East Africa). Mar. Ecol. Prog. Ser. 231, 75–83. doi: 10.3354/meps231075
Kardong, K. (2003). Epigenomics: the new science of functional and evolutionary morphology. Anim. Biol. 53, 225–243. doi: 10.1163/157075603322539435
Kashta, L., and Pizzuto, F. (1995). Sulla presenza di Halophila stipulacea (Forsskål and Niebuhr) Ascherson (Hydrocharitales, Hydrocharitaceae) nelle coste dell'Albania. Boll. Acc. Gioenia. Sci. Nat. 28, 161–166.
Katsanevakis, S. (2011). Rapid assessment of the marine alien megabiota in the shallow coastal waters of the Greek islands, Paros and Antiparos, Aegean sea. Aquat. Invasions 6, 133–137. doi: 10.3391/ai.2011.6.S1.030
Kaul, R. B. (1968). Floral development and vascularature in hydrocleis (butomaceae). Am. J. Bot. 55, 236–242. doi: 10.1002/j.1537-2197.1968.tb06966.x
Kearney, M., and Porter, W. (2009). Mechanistic niche modelling: combining physiological and spatial data to predict species' ranges. Ecol. Lett. 12, 334–350. doi: 10.1111/j.1461-0248.2008.01277.x
Kearney, M. R., Wintle, B. A., and Porter, W. P. (2010). Correlative and mechanistic models of species distribution provide congruent forecasts under climate change. Conserv. Lett. 3, 203–213. doi: 10.1111/j.1755-263X.2010.00097.x
Kenworthy, W. J. (2000). The role of sexual reproduction in maintaining populations of Halophila decipiens: implications for the biodiversity and conservation of tropical seagrass ecosystems. Pac. Conserv. Biol. 5, 265–268. doi: 10.1071/PC000260
Kenworthy, W. J., Durako, M. J., Fatemy, S. M. R., Valavi, H., and Thayer, G. W. (1993). Ecology of seagrasses in northeastern Saudi Arabia one year after the Gulf war oil spill. Mar. Pollut. Bull. 27, 213–222. doi: 10.1016/0025-326X(93)90027-H
Khalaf, M., Al-Rousan, S., and Al-Horani, F. (2012). Fish assemblages in seagrass habitat along the Jordanian coast of the Gulf of Aqaba. Nat. Sci. 4, 517–525. doi: 10.4236/ns.2012.48069
Kissling, W. D., Dormann, C. F., Groeneveld, J., Hickler, T., Kuhn, I., McInerny, G. J., et al. (2012). Towards novel approaches to modelling biotic interactions in multispecies assemblages at large spatial extents. J. Biogeograp. 39, 2163–2178. doi: 10.1111/j.1365-2699.2011.02663.x
Kleynhans, E., Mitchell, K. A., Conlong, D. E., and Terblanche, J. S. (2014). Evolved variation in cold tolerance among populations of Eldana saccharina (Lepidoptera: Pyralidae) in South Africa. J. Evol. Biol. 27, 1149–1159. doi: 10.1111/jeb.12390
Kolar, C. S., and Lodge, D. M. (2001). Progress in invasion biology: predicting invaders. Trends Ecol. Evol. 16, 199–204. doi: 10.1016/S0169-5347(01)02101-2
Kondo, A., Kaikawa, J., Funaguma, T., and Ueno, O. (2004). Clumping and dispersal of chloroplasts in succulent plants. Planta 219, 500–506. doi: 10.1007/s00425-004-1252-3
Kong, F., Li, H., Sun, P., Zhou, Y., and Mao, Y. (2014). De novo assembly and characterization of the transcriptome of seagrass Zostera marina using illumina paired-end sequencing. PLoS ONE 9:e112245. doi: 10.1371/journal.pone.0112245
Kumar, M., Padula, M. P., Davey, P., Pernice, M., Jiang, Z., Sablok, G., et al. (2017). Proteome analysis reveals extensive light stress-response reprogramming in the seagrass Zostera muelleri (alismatales, zosteraceae) metabolism. Front. Plant Sci. 7:2023. doi: 10.3389/fpls.2016.02023
Kuo, J., and McComb, A. (1989). “Seagrass taxonomy, structure and development,” in A treatise on the biology of seagrasses with special reference to the Australian region, eds A. Larkum, A. McComb and S. Shephard (Amsterdam: Elsevier Science Pub.), 6–73.
Lanfranco, E. (1970). The occurrence of Halophila stipulacea (Forsskål and Niebuhr) Ascherson (family: Hydrocharitaceae) in maltese waters. Maltese Nat. 1, 16–17.
Lee, C. E. (2002). Evolutionary genetics of invasive species. Trends Ecol. Evol. 17, 386–391. doi: 10.1016/S0169-5347(02)02554-5
Lee, H., Golicz, A. A., Bayer, P. E., Jiao, Y., Tang, H., Paterson, A. H., et al. (2016). The genome of a southern hemisphere seagrass species (Zostera muelleri). Plant Physiol. 172, 272–283. doi: 10.1104/pp.16.00868
Lee, K-S., Park, S. R., and Kim, Y. K. (2007). Effects of irradiance, temperature, and nutrients on growth dynamics of seagrasses: a review. J. Exp. Mar. Biol. Ecol. 350, 144–175. doi: 10.1016/j.jembe.2007.06.016
Les, D. H., Cleland, M. A., and Waycott, M. (1997). Phylogenetic studies in alismatidae, II: evolution of marine angiosperms (seagrasses) and hydrophily. Syst. Bot. 22, 443–463. doi: 10.2307/2419820
Lipkin, Y. (1975a). Halophila stipulacea in cyprus and rhodes, 1967–1970. Aqua. Bot. 1, 309–320. doi: 10.1016/0304-3770(75)90029-7
Lipkin, Y. (1975b). Halophila stipulacea, a review of a successful immigration. Aquat. Bot. 1, 203–215. doi: 10.1016/0304-3770(75)90023-6
Lipkin, Y. (1979). Quantitative aspects of seagrass communities, particularly of those dominated by Halophila stipulacea, in Sinai (Northern Red sea). Aquat. Bot. 7, 119–128. doi: 10.1016/0304-3770(79)90016-0
Lipkin, Y., Beer, S., and Zakai, D. (2003). “The Eastern Mediterranean and Red Sea,” in World Atlas of Seagrasses, eds. E. P. Green and F. T. Short. (Berkeley, California: University of California Press), 65–73.
Lowe, S., Browne, M., Boudjelas, S., and De Poorter, M. (2000). 100 of the World's Worst Invasive Alien Species: A Selection from the Global Invasive Species Database. Invasive Species Specialist Group SSC IUCN, 12.
Lyons, D., and Scheibling, R. (2009). Range expansion by invasive marine algae: rates and patterns of spread at a regional scale. Divers. Distrib. 15, 762–775. doi: 10.1111/j.1472-4642.2009.00580.x
Mahalingam, R., and Gopinath, K. (1987). Ecological conservation of seagrass beds in the Gulf of Mannar, India. Environ. Conserv. 14, 265–268. doi: 10.1017/S0376892900016507
Mainali, K. P., Warren, D. L., Dhileepan, K., McConnachie, A., Strathie, L., Hassan, G., et al. (2015). Projecting future expansion of invasive species: comparing and improving methodologies for species distribution modeling. Glob. Chang. Biol. 21, 4464–4480. doi: 10.1111/gcb.13038
Malea, P. (1994). Seasonal variation and local distribution of metals in the seagrass Halophila stipulacea (Forsk.) aschers. in the Antikyra Gulf, Greece. Environ. Pollut. 85, 77–85. doi: 10.1016/0269-7491(94)90240-2
Malm, T. (2006). Reproduction and recruitment of the seagrass Halophila stipulacea. Aquat. Bot. 85, 345–349. doi: 10.1016/j.aquabot.2006.05.008
Marbà, N., and Duarte, C. M. (2010). Mediterranean warming triggers seagrass (Posidonia oceanica) shoot mortality. Glob. Chang. Biol. 16, 2366–2375. doi: 10.1111/j.1365-2486.2009.02130.x
Marbà, N., Hemminga, M. A., Mateo, M. Á., Duarte, C. M., Mass, Y. E., Terrados, J., et al. (2002). Carbon and nitrogen translocation between seagrass ramets. Mar. Ecol. Prog. Ser. 226, 287–300. doi: 10.3354/meps226287
Maréchal, J-P., Meesters, E. H., Vedie, F., and Hellio, C. (2013). Occurrence of the alien seagrass Halophila stipulacea in martinique (French West Indies). Mar. Biodivers. Rec. 6:e127. doi: 10.1017/S1755267213000961
Mariani, S., and Alcoverro, T. (1999). A multiple-choice feeding-preference experiment utilising seagrasses with a natural population of herbivorous fishes. Mar. Ecol. Prog. Ser. 189, 295–299. doi: 10.3354/meps189295
Marín-Guirao, L., Bernardeau-Esteller, J., García-Muñoz, R., Ramos, A., Ontoria, Y., Romero, J., et al. (2018). Carbon economy of Mediterranean seagrasses in response to thermal stress. Mar. Pollut. Bull. 135, 617–629. doi: 10.1016/j.marpolbul.2018.07.050
Marín-Guirao, L., Entrambasaguas, L., Dattolo, E., Ruiz, J. M., and Procaccini, G. (2017). Molecular mechanisms behind the physiological resistance to intense transient warming in an iconic marine plant. Front. Plant Sci. 8:1142. doi: 10.3389/fpls.2017.01142
Marín-Guirao, L., Entrambasaguas, L., Ruiz, J. M., and and Procaccini, G. (2019). Heat-stress induced flowering can be a potential adaptive response to ocean warming for the iconic seagrass Posidonia oceanica. Mol. Ecol. 28, 2486-2501. doi: 10.1111/mec.15089
Martin, B. C., Gleeson, D., Statton, J., Siebers, A. R., Grierson, P., Ryan, M. H., et al. (2018). Low light availability alters root exudation and reduces putative beneficial microorganisms in seagrass roots. Front. Microbiol. 8:2667. doi: 10.3389/fmicb.2017.02667
Martínez, B., Arenas, F., Trilla, A., Viejo, R. M., and Carreño, F. (2015). Combining physiological threshold knowledge to species distribution models is key to improving forecasts of the future niche for macroalgae. Glob. Change Biol. 21, 1422–1433. doi: 10.1111/gcb.12655
Marzinelli, E. M., Campbell, A. H., Zozaya Valdes, E., Vergés, A., Nielsen, S., Wernberg, T., et al. (2015). Continental-scale variation in seaweed host-associated bacterial communities is a function of host condition, not geography. Environ. Microbiol. 17, 4078–4088. doi: 10.1111/1462-2920.12972
Mazzuca, S., Bjork, M., Beer, S., Felisberto, P., Gobert, S., Procaccini, G., et al. (2013). Establishing research strategies, methodologies and technologies to link genomics and proteomics to seagrass productivity, community metabolism, and ecosystem carbon fluxes. Front. Plant Sci. 4:38. doi: 10.3389/fpls.2013.00038
Mejia, A. Y., Rotini, A., Lacasella, F., Bookman, R., Thaller, M. C., Shem-Tov, R., et al. (2016). Assessing the ecological status of seagrasses using morphology, biochemical descriptors and microbial community analyses. A study in Halophila stipulacea (Forsk.) Aschers meadows in the Northern Red sea. Ecol. Indic. 60, 1150–1163. doi: 10.1016/j.ecolind.2015.09.014
Meron, D., Atias, E., Kruh, L. I., Elifantz, H., Minz, D., Fine, M., et al. (2011). The impact of reduced pH on the microbial community of the coral Acropora eurystoma. ISME J. 5, 51–60. doi: 10.1038/ismej.2010.102
Milchakova, N., Phillips, R., and Ryabogina, V. (2005). New data on the locations of seagrass species in the Indian Ocean. Atoll. Res. Bull. 537, 177–188. doi: 10.5479/si.00775630.537.177
Mineur, F., Arenas, F., Assis, J., Davies, A., Engelen, A., Fernandes, F., et al. (2015). European seaweeds under pressure: consequences for communities and ecosystem functioning. J. Sea Res. 98, 91–108. doi: 10.1016/j.seares.2014.11.004
Montague, C. L., and Ley, J. A. (1993). A possible effect of salinity fluctuation on abundance of benthic vegetation and associated fauna in northeastern Florida Bay. Estuaries 16, 703–717. doi: 10.2307/1352429
Muthukrishnan, R., Willette, D. A., Chiquillo, K. L., Cross, C., Fong, P., Kelley, T, et al. (in review). Little giants: an invasive seagrass alters ecosystem functioning relative to native foundation species. Mar. Biol.
Nagelkerken, I., Kleijnen, S., Klop, T., Van Den Brand, R., de La Moriniere, E. C., and Van der Velde, G. (2001). Dependence of Caribbean reef fishes on mangroves and seagrass beds as nursery habitats: a comparison of fish faunas between bays with and without mangroves/seagrass beds. Mar. Ecol. Prog. Ser. 214, 225–235. doi: 10.3354/meps214225
Naser, H. (2014). “Marine ecosystem diversity in the Arabian Gulf: threats and conservation,” in Biodiversity - The Dynamic Balance of the Planet, ed O. Grillo (IntechOpen), 297–328. doi: 10.5772/57425
Nguyen, H. M., Kleitou, P., Kletou, D., Sapir, Y., and Winters, G. (2018). Differences in flowering sex ratios between native and invasive populations of the seagrass Halophila stipulacea. Bot. Mar. 61, 337–342. doi: 10.1515/bot-2018-0015
Nguyen, H. M., Savva, I., Kleitou, P., Kletou, D., Lima, F., Sapir, Y., et al. (2020a). Seasonal dynamics of native and invasive Halophila stipulacea populations. Aquat. Bot. 162:103205. doi: 10.1016/j.aquabot.2020.103205
Nguyen, H. M., Yadav, N. S., Barak, S., Lima, F. P., Sapir, Y., and Winters, G. (2020b). Responses of invasive and native populations of the seagrass Halophila stipulacea to simulated climate change. Front. Mar. Sci. 6:812. doi: 10.3389/fmars.2019.00812
Nichols, P. D., and Johns, R. (1985). Lipids of the tropical seagrass Thallassia hemprichii. Phytochemistry 24, 81–84. doi: 10.1016/S0031-9422(00)80811-0
Nishida, I., and Murata, N. (1996). Chilling sensitivity in plants and cyanobacteria: the crucial contribution of membrane lipids. Annu. Rev. Plant Physiol. Plant Mol. Biol. 47, 541–568. doi: 10.1146/annurev.arplant.47.1.541
Olesen, B., Krause-Jensen, D., Marbà, N., and Christensen, P. B. (2015). Eelgrass Zostera marina in subarctic Greenland: dense meadows with slow biomass turnover in cold waters. Mar. Ecol. Prog. Ser. 518, 107–121. doi: 10.3354/meps11087
Olinger, L. K., Heidmann, S. L., Durdall, A. N., Howe, C., Ramseyer, T., Thomas, S. G., et al. (2017). Altered juvenile fish communities associated with invasive Halophila stipulacea seagrass habitats in the US Virgin islands. PLoS ONE 12:e0188386. doi: 10.1371/journal.pone.0188386
Oliver, E., Donat, M., Burrows, M., Moore, P., Smale, D., Alexander, L., et al. (2018). Longer and more frequent marine heatwaves over the past century. Nat. Commun. 9:1324. doi: 10.1038/s41467-018-03732-9
Olsen, J. L., Rouzé, P., Verhelst, B., Lin, Y-C., Bayer, T., Collen, J., et al. (2016). The genome of the seagrass Zostera marina reveals angiosperm adaptation to the sea. Nature 530, 331–348. doi: 10.1038/nature16548
Olyarnik, S. V., Bracken, M. E., Byrnes, J. E., Hughes, A. R., Hultgren, K. M., and Stachowicz, J. J. (2009). “Ecological factors affecting community invasibility,” in Biological Invasions in Marine Ecosystems, eds G. Rilov and J. Crooks (Berlin; Heidelberg: Springer), 215–238. doi: 10.1007/978-3-540-79236-9_12
Oron, S., Angel, D., Goodman-Tchernov, B., Merkado, G., Kiflawi, M., and Abramovich, S. (2014). Benthic foraminiferal response to the removal of aquaculture fish cages in the Gulf of Aqaba-Eilat, Red sea. Mar. Micropaleontol. 107, 8–17. doi: 10.1016/j.marmicro.2014.01.003
Ortea, J., Espinosa, J., Caballer, M., and Buske, Y. (2012). Initial inventory of the sea slugs (opisthobranchia and sacoglossa) from the expedition KARUBENTHOS, held in May 2012 in Guadaloupe (Lesser Antilles, Caribbean sea). Rev. Acad. Canar. Cienc. 24, 153–182.
Orth, R. J., Carruthers, T. J., Dennison, W. C., Duarte, C. M., Fourqurean, J. W., Heck, K. L., et al. (2006). A global crisis for seagrass ecosystems. Bioscience 56, 987–996. doi: 10.1641/0006-3568(2006)56[987:AGCFSE]2.0.CO;2
Oscar, M. A., Barak, S., and Winters, G. (2018). The tropical invasive seagrass, Halophila stipulacea, has a superior ability to tolerate dynamic changes in salinity levels compared to its freshwater relative, Vallisneria americana. Front. Plant Sci. 9:950. doi: 10.3389/fpls.2018.00950
Ozer, T., Gertman, I., Kress, N., Silverman, J., and Herut, B. (2017). Interannual thermohaline 1979–2014 and nutrient 2002–2014 dynamics in the Levantine surface and intermediate water masses, SE Mediterranean sea. Glob. Planet. Chang. 151, 60–67. doi: 10.1016/j.gloplacha.2016.04.001
Parravicini, V., Azzurro, E., Kulbicki, M., and Belmaker, J. (2015). Niche shift can impair the ability to predict invasion risk in the marine realm: an illustration using Mediterranean fish invaders. Ecol. Lett. 18, 246–253. doi: 10.1111/ele.12401
Pereg, L., Lipkin, Y., and Sar, N. (1994). Different niches of the Halophila stipulacea seagrass bed harbor distinct populations of nitrogen fixing bacteria. Mar. Biol. 119, 327–333. doi: 10.1007/BF00347529
Pettitt, J. (1981). Reproduction in seagrasses: pollen development in Thalassia hemprichii, Halophila stipulacea and Thalassodendron ciliatum. Ann. Bot. 48, 609–622. doi: 10.1093/oxfordjournals.aob.a086169
Phandee, S., and Buapet, P. (2018). Photosynthetic and antioxidant responses of the tropical intertidal seagrasses Halophila ovalis and Thalassia hemprichii to moderate and high irradiances. Bot. Mar. 61, 247–256. doi: 10.1515/bot-2017-0084
Phillips, R. C., Loughland, R., and Ashraf, Y. (2002). Seagrasses of Abu Dhabi, United Arab Emirates, Arabian Gulf: A review. Tribulus ENHG 15, 21–24.
Piro, A., Marín-Guirao, L., Serra, I. A., Spadafora, A., Sandoval-Gil, J. M., Bernardeau-Esteller, J., et al. (2015a). The modulation of leaf metabolism plays a role in salt tolerance of Cymodocea nodosa exposed to hypersaline stress in mesocosms. Front. Plant Sci. 6:464. doi: 10.3389/fpls.2015.00464
Piro, A., Serra, I. A., Spadafora, A., Cardilio, M., Bianco, L., Perrotta, G., et al. (2015b). Purification of intact chloroplasts from marine plant Posidonia oceanica suitable for organelle proteomics. Proteomics 15, 4159–4174. doi: 10.1002/pmic.201500246
Por, F. D. (1971). One hundred years of Suez Canal—a century of Lessepsian migration: retrospect and viewpoints. Syst. Zool. 20, 138–159. doi: 10.2307/2412054
Preen, A. (1989). The Status and Conservation of Dugongs in the Arabian Region. Saudi Arabia, Meteorological and Environmental Protection Administration (MEPA), MEPA Coastal and Marine Management Series, Report No. 10, 200.
Preen, A., and Marsh, H. (1995). Response of dugongs to large-scale loss of seagrass from Hervey Bay, Queensland Australia. Wildlife Res. 22, 507–519. doi: 10.1071/WR9950507
Price, A. R., and Coles, S. L. (1992). Aspects of seagrass ecology along the western Arabian Gulf coast. Hydrobiologia 234, 129–141. doi: 10.1007/BF00014245
Procaccini, G., Acunto, S., Famà, P., and Maltagliati, F. (1999a). Structural, morphological and genetic variability in Halophila stipulacea (Hydrocharitaceae) populations in the western Mediterranean. Mar. Biol. 135, 181–189. doi: 10.1007/s002270050615
Procaccini, G., Mazzella, L., Alberte, R. S., and Les, D. H. (1999b). Chloroplast tRNA Leu (UAA) intron sequences provide phylogenetic resolution of seagrass relationships. Aquat. Bot. 62, 269–283. doi: 10.1016/S0304-3770(98)00099-0
Procaccini, G., Olsen, J. L., and Reusch, T. B. (2007). Contribution of genetics and genomics to seagrass biology and conservation. J. Exp. Mar. Biol. Ecol. 350, 234–259. doi: 10.1016/j.jembe.2007.05.035
Procaccini, G., Ruocco, M., Marín-Guirao, L., Dattolo, E., Brunet, C., D'Esposito, D., et al. (2017). Depth-specific fluctuations of gene expression and protein abundance modulate the photophysiology in the seagrass Posidonia oceanica. Sci. Rep. 7:42890. doi: 10.1038/srep42890
Qurban, M. A. B., Karuppasamy, M., Krishnakumar, P. K., Garcias-Bonet, N., and Duarte, C. M. (2019). “Seagrass distribution, composition and abundance along the Saudi Arabian coast of Red sea,” in Oceanographic and Biological Aspects of the Red Sea, eds N. Rasul and I. Stewart (Cham: Springer Nature), 367–385. doi: 10.1007/978-3-319-99417-8_20
Randall, J. E. (1965). Grazing effect on sea grasses by herbivorous reef fishes in the West Indies. Ecology 46, 255–260. doi: 10.2307/1936328
Rasheed, M. A. (1999). Recovery of experimentally created gaps within a tropical Zostera capricorni (aschers.) seagrass meadow, Queensland Australia. J. Exp. Mar. Biol. Ecol. 235, 183–200. doi: 10.1016/S0022-0981(98)00158-0
Record, S., Strecker, A., Tuanmu, M-N., Beaudrot, L., Zarnetske, P., Belmaker, J., et al. (2018). Does scale matter? a systematic review of incorporating biological realism when predicting changes in species distributions. PLoS ONE 13:e0194650. doi: 10.1371/journal.pone.0194650
Richards, C. L., Alonso, C., Becker, C., Bossdorf, O., Bucher, E., Colomé-Tatché, M., et al. (2017). Ecological plant epigenetics: evidence from model and non-model species, and the way forward. Ecol. Lett. 20, 1576–1590. doi: 10.1111/ele.12858
Rilov, G. (2016). Multi-species collapses at the warm edge of a warming sea. Sci. Rep. 6:36897. doi: 10.1038/srep36897
Rilov, G., and Galil, B. (2009). “Marine bioinvasions in the Mediterranean sea–history, distribution and ecology,” in Biological Invasions in Marine Ecosystems. Ecological Studies (Analysis and Synthesis), eds G. Rilov and J. Crooks (Berlin; Hiedelberg: Springer), 549–575. doi: 10.1007/978-3-540-79236-9_31
Rindi, F., Maltagliati, F., Rossi, F., Acunto, S., and Cinelli, F. (1999). Algal flora associated with a Halophila stipulacea (forsskål and niebuhr) Ascherson (Hydrocharitaceae, Helobiae) stand in the western Mediterranean. Oceanol. Acta 22, 421–429. doi: 10.1016/S0399-1784(00)88725-3
Robinson, M. K. (1973). Atlas of monthly mean sea surface and subsurface temperatures in the Gulf of California, Mexico. San Diego Soc. Nat. Hist., Mem. 5, 1–97.
Roca, G., Alcoverro, T., Krause-Jensen, D., Balsby, T. J. S., van Katwijk, M. M., Marbà, N., et al. (2016). Response of seagrass indicators to shifts in environmental stressors: a global review and management synthesis. Ecol. Indic. 63, 310–323. doi: 10.1016/j.ecolind.2015.12.007
Rosenberg, E., Koren, O., Reshef, L., Efrony, R., and Zilber-Rosenberg, I. (2007). The role of microorganisms in coral health, disease and evolution. Nat. Rev. Microbiol. 5, 355–362. doi: 10.1038/nrmicro1635
Rotini, A., Mejia, A. Y., Costa, R., Migliore, L., and Winters, G. (2017). Ecophysiological plasticity and bacteriome shift in the seagrass Halophila stipulacea along a depth gradient in the northern Red sea. Front. Plant Sci. 7:2015. doi: 10.3389/fpls.2016.02015
Rout, M. E., Chrzanowski, T. H., Westlie, T. K., DeLuca, T. H., Callaway, R. M., and Holben, W. E. (2013). Bacterial endophytes enhance competition by invasive plants. Am. J. Bot. 100, 1726–1647. doi: 10.3732/ajb.1200577
Rudnick, D. T., Ortner, P. B., Browder, J. A., and Davis, S. M. (2005). A conceptual ecological model of Florida Bay. Wetlands 25, 870–883. doi: 10.1672/0277-5212(2005)025[0870:ACEMOF]2.0.CO;2
Ruggiero, M. V., and Procaccini, G. (2004). The rDNA ITS region in the Lessepsian marine angiosperm Halophila stipulacea (Forssk.) aschers. (Hydrocharitaceae): intragenomic variability and putative pseudogenic sequences. J. Mol. Evol. 58, 115–121. doi: 10.1007/s00239-003-2536-0
Ruiz, H., Ballantine, D., and Sabater, J. (2017). Continued spread of the seagrass Halophila stipulacea in the Caribbean: documentation in Puerto Rico and the British Virgin islands. Gulf Caribb. Res. 28, SC5–SC7. doi: 10.18785/gcr.2801.05
Ruiz, H., and Ballantine, D. L. (2004). Occurrence of the seagrass Halophila stipulacea in the tropical west Atlantic. Bull. Mar. Sci. 75, 131–135.
Ruocco, M., Marín-Guirao, L., and Procaccini, G. (2019). Within- and among-leaf variations in photo-physiological functions, gene expression and DNA methylation patterns in the large-sized seagrass Posidonia oceanica. Mar. Biol. 166:24. doi: 10.1007/s00227-019-3482-8
Russo, A., Carniel, S., Sclavo, M., and KrŽelj, M. (2012). “Climatology of the northern-central Adriatic sea,” in Modern Climatology, eds S-Y. Wang and R. Gillies. (London, UK: InTech), 177–212. doi: 10.5772/34693
Sakai, A. K., Allendorf, F. W., Holt, J. S., Lodge, D. M., Molofsky, J., With, K. A., et al. (2001). The population biology of invasive species. Ann. Rev. Ecol. Syst. 32, 305–332. doi: 10.1146/annurev.ecolsys.32.081501.114037
Salo, T., Pedersen, M. F., and Boström, C. (2014). Population specific salinity tolerance in eelgrass (Zostera marina). J. Exp. Mar. Biol. Ecol. 461, 425–429. doi: 10.1016/j.jembe.2014.09.010
Savva, I., Bennett, S., Roca, G., Jord,à, G., and Marbà, N. (2018). Thermal tolerance of Mediterranean marine macrophytes: vulnerability to global warming. Ecol. Evol. 8, 12032–12043. doi: 10.1002/ece3.4663
Scheibling, R. E., Patriquin, D. G., and Filbee-Dexter, K. (2018). Distribution and abundance of the invasive seagrass Halophila stipulacea and associated benthic macrofauna in Carriacou, Grenadines, Eastern Caribbean. Aquat. Bot. 144, 1–8. doi: 10.1016/j.aquabot.2017.10.003
Schrey, A. W., Richards, C. L., Meller, V., Sollars, V., and Ruden, D. M. (2012). The role of epigenetics in evolution: the extended synthesis. Genetics Res. Int. 2012:286164. doi: 10.1155/2012/286164
Schwarz, A-M., and Hellblom, F. (2002). The photosynthetic light response of Halophila stipulacea growing along a depth gradient in the Gulf of Aqaba, the Red sea. Aquat. Bot. 74, 263–272. doi: 10.1016/S0304-3770(02)00080-3
Sghaier, Y. R., Zakhama-Sraieb, R., Benamer, I., and Charfi-Cheikhrouha, F. (2011). Occurrence of the seagrass Halophila stipulacea (Hydrocharitaceae) in the southern Mediterranean sea. Bot. Mar. 54, 575–582. doi: 10.1515/BOT.2011.061
Sghaier, Y. R., Zakhama-Sraieb, R., and Charfi-Cheikhrouha, F. (2014). “Effects of the invasive seagrass Halophila stipulacea on the native seagrass Cymodocea nodosa,” in Proceedings of the Fifth Mediterranean Symposium on Marine Vegetation. PortoroŽ (Slovenia), 167–171.
Shaltout, M., and Omstedt, A. (2014). Recent sea surface temperature trends and future scenarios for the Mediterranean sea. Oceanologia 56, 411–443. doi: 10.5697/oc.56-3.411
Sharon, Y., and Beer, S. (2008). Diurnal movements of chloroplasts in Halophila stipulacea and their effect on PAM fluorometric measurements of photosynthetic rates. Aquat. Bot. 88, 273–276. doi: 10.1016/j.aquabot.2007.11.006
Sharon, Y., Dishon, G., and Beer, S. (2011a). The effects of UV radiation on chloroplast clumping and photosynthesis in the seagrass Halophila stipulacea grown under high-PAR conditions. J. Mar. Biol. 2011:483428. doi: 10.1155/2011/483428
Sharon, Y., Levitan, O., Spungin, D., Berman-Frank, I., and Beer, S. (2011b). Photoacclimation of the seagrass Halophila stipulacea to the dim irradiance at its 48-meter depth limit. Limnol. Oceanogr. 56, 357–362. doi: 10.4319/lo.2011.56.1.0357
Sharon, Y., Silva, J., Santos, R., Runcie, J. W., Chernihovsky, M., and Beer, S. (2009). Photosynthetic responses of Halophila stipulacea to a light gradient. II. acclimations following transplantation. Aquat. Biol. 7, 153–157. doi: 10.3354/ab00148
Short, F. T., and Duarte, C. M. (2001). “Methods for the measurement of seagrass growth and production,” in Global Seagrass Research Methods, eds F. Short, R. Coles, and C. Short (Amsterdam: Elsevier Science BV), 155–198. doi: 10.1016/B978-044450891-1/50009-8
Simpson, D. (1989). “Hydrocharitaceae” in Flora of Tropical East Africa, ed R. Polhill (Rotterdam: Balkema), 1–29.
Singh, R. P., and Reddy, C. (2014). Seaweed–microbial interactions: key functions of seaweed-associated bacteria. FEMS Microbiol. Rev. 88, 213–230. doi: 10.1111/1574-6941.12297
Smulders, F. O. H., Vonk, J. A., Engel, M. S., and Christianen, M. J. A. (2017). Expansion and fragment settlement of the non-native seagrass Halophila stipulacea in a Caribbean bay. Mar. Biol. Res. 13, 967–974. doi: 10.1080/17451000.2017.1333620
Sol, D., Maspons, J., Vall-Llosera, M., Bartomeus, I., García-Peña, G. E., Piñol, J., et al. (2012). Unraveling the life history of successful invaders. Science 337, 580–583. doi: 10.1126/science.1221523
Spalding, M., Taylor, M., Ravilious, C., Short, F., and Green, E. (2003). The Distribution and Status of Seagrasses. Berkeley, California: World atlas of seagrasses. University of California Press, 5–26.
Stachowicz, J. J., Terwin, J. R., Whitlatch, R. B., and Osman, R. W. (2002). Linking climate change and biological invasions: ocean warming facilitates nonindigenous species invasions. Proc. Nat. Acad. Sci. U.S.A. 99, 15497–15500. doi: 10.1073/pnas.242437499
Steiner, S., Macfarlane, K., Price, L., and Willette, D. (2010). The distribution of seagrasses in Dominica, Lesser Antilles. Rev. Biol. Trop. 58, 89–98.
Steiner, S., and Willette, D. (2015a). The expansion of Halophila stipulacea (Hydrocharitaceae, Angiospermae) is changing the seagrass landscape in the commonwealth of Dominica, Lesser Antilles. Caribb. Nat 22, 1–19.
Steiner, S., and Willette, D. A. (2015b). Dimming sand halos in Dominica and the expansion of the invasive seagrass Halophila stipulacea. Reef Encount 30, 43–45.
Steiner, S., and Willette, D. A. (2010). Distribution and size of benthic marine habitats in Dominica, Lesser Antilles. Rev. Biol. Trop. 58, 589–602. doi: 10.15517/rbt.v58i2.5231
Streftaris, N., and Zenetos, A. (2006). Alien marine species in the Mediterranean - the 100 'Worst Invasives' and their impact. Mediterr. Mar. Sci. 7, 87–118. doi: 10.12681/mms.180
Stuij, T. (2018). distinct microbiomes in three Tropical seagrasses around the Island of Curaçao: Halophila stipulacea, Halodule wrightii and Thalassia testudinum (Master's Thesis). Universidade do Algarve, Faro, Portugal.
Talluto, M. V., Boulangeat, I., Ameztegui, A., Aubin, I., Berteaux, D., Butler, A., et al. (2016). Cross-scale integration of knowledge for predicting species ranges: a metamodelling framework. Glob. Ecol. Biogeogr. 25, 238–249. doi: 10.1111/geb.12395
Taylor, M. W., Radax, R., Steger, D., and Wagner, M. (2007). Sponge-associated microorganisms: evolution, ecology, and biotechnological potential. Microbiol. Mol. Biol. Rev. 71, 295–347. doi: 10.1128/MMBR.00040-06
Tsiamis, K., Montesanto, B., Panayotidis, P., katsaros, C., and Verlaque, M. (2010). Updated records and range expansion of alien marine macrophytes in Greece 2009. Mediterr. Mar. Sci. 11, 61–80. doi: 10.12681/mms.91
Tsirika, A., and Haritonidis, S. (2005). A survey of the benthic flora in the National marine park of zakynthos (Greece). Bot. Mar.48, 38–45. doi: 10.1515/BOT.2005.002
Valentine, J. F., and Heck, K. L. (2005). Perspective review of the impacts of overfishing on coral reef food web linkages. Coral Reefs 24, 209–213. doi: 10.1007/s00338-004-0468-9
Van der Velde, G., and Den Hartog, C. (1992). Continuing range extension of Halophila stipulacea (Forssk.) aschers (Hydrocharitaceae) in the Mediterranean - now found at Kefallinia and Ithaki (Ionian sea). Acta bot. Neerl. 41, 345–348. doi: 10.1111/j.1438-8677.1992.tb01341.x
van Tussenbroek, B. I., van Katwijk, M., Bouma, T., van der Heide, T., Govers, L., and Leuven, R. (2016). Non-native seagrass Halophila stipulacea forms dense mats under eutrophic conditions in the Caribbean. J. Sea Res. 115, 1–5. doi: 10.1016/j.seares.2016.05.005
Varela-Álvarez, E., Rindi, F., Cavas, L., Serrão, E., Duarte, C. M., and Marrá, N. (2011). Molecular identification of the tropical seagrass Halophila stipulacea from Turkey. Cah. Biol. Mar. 52, 227–232.
Vera, B., Collado-Vides, L., Moreno, C., and Tussenbroek, B. I.. (2014). Halophila stipulacea (hydrocharitaceae): a recent introduction to the continental waters of Venezuela. Caribb. J. Sci.48, 66–70. doi: 10.18475/cjos.v48i1.a11
Viana, I. G., Siriwardane-de Zoysa, R., Willette, D. A., and Gillis, L. G. (2019). Exploring how non-native seagrass species could provide essential ecosystems services: a perspective on the highly invasive seagrass Halophila stipulacea in the Caribbean sea. Biol. Invasions 21, 1461–1472. doi: 10.1007/s10530-019-01924-y
Viso, A-C., Pesando, D., Bernard, P., and Marty, J-C. (1993). Lipid components of the Mediterranean seagrass Posidonia oceanica. Phytochemistry 34, 381–387. doi: 10.1016/0031-9422(93)80012-H
Wahbeh, M. I. (1984). The growth and production of the leaves of the seagrass Halophila stipulacea (Forsk.) Aschers. from Aqaba, Jordan. Aqua. Bot. 20, 33–41. doi: 10.1016/0304-3770(84)90025-1
Waycott, M., Duarte, C. M., Carruthers, T. J., Orth, R. J., Dennison, W. C., Olyarnik, S., et al. (2009). Accelerating loss of seagrasses across the globe threatens coastal ecosystems. Proc. Nat. Acad. Sci. U.S.A. 106, 12377–12381. doi: 10.1073/pnas.0905620106
Willette, D. A., and Ambrose, R. F. (2009). The distribution and expansion of the invasive seagrass Halophila stipulacea in Dominica, West Indies, with a preliminary report from St. Lucia. Aquat. Bot. 91, 137–142. doi: 10.1016/j.aquabot.2009.04.001
Willette, D. A., and Ambrose, R. F. (2012). Effects of the invasive seagrass Halophila stipulacea on the native seagrass, Syringodium filiforme, and associated fish and epibiota communities in the Eastern Caribbean. Aquat. Bot. 103, 74–82. doi: 10.1016/j.aquabot.2012.06.007
Willette, D. A., Chalifour, J., Debrot, A. D., Engel, M. S., Miller, J., Oxenford, H. A., et al. (2014). Continued expansion of the trans-Atlantic invasive marine angiosperm Halophila stipulacea in the Eastern Caribbean. Aquat. Bot. 112, 98–102. doi: 10.1016/j.aquabot.2013.10.001
Willette, D. A., Chiquillo, K. L., Cross, C., Fong, P., Kelley, T., Toline, C. A., et al. (2020). Growth and recovery after small-scale disturbance of a rapidly-expanding invasive seagrass in St. John, U.S. Virgin islands. J. Exp. Mar. Biol. Ecol. 523:151265. doi: 10.1016/j.jembe.2019.151265
Williams, S. (2007). Introduced species in seagrass ecosystems: status and concerns. J. Exp. Mar. Biol. Ecol. 350, 89–110. doi: 10.1016/j.jembe.2007.05.032
Williamson, M. H., and Fitter, A. (1996). The characters of successful invaders. Biol. Conserv. 78, 163–170. doi: 10.1016/0006-3207(96)00025-0
Winters, G., Edelist, D., Shem-Tov, R., Beer, S., and Rilov, G. (2017). A low cost field-survey method for mapping seagrasses and their potential threats: an example from the northern Gulf of Aqaba, Red sea. Aquat. Conserv. 27, 324–339. doi: 10.1002/aqc.2688
Wisz, M. S., Pottier, J., Kissling, W. D., Pellissier, L., Lenoir, J., Damgaard, C. F., et al. (2013). The role of biotic interactions in shaping distributions and realised assemblages of species: implications for species distribution modelling. Biol. Rev. 88, 15–30. doi: 10.1111/j.1469-185X.2012.00235.x
Woodin, S. A., Hilbish, T. J., Helmuth, B., Jones, S. J., and Wethey, D. S. (2013). Climate change, species distribution models, and physiological performance metrics: predicting when biogeographic models are likely to fail. Ecol. Evol. 3, 3334–3346. doi: 10.1002/ece3.680
Yang, Y., Sage, T. L., Liu, Y., Ahmad, T. R., Marshall, W. F., Shiu, S-H., et al. (2011). CLUMPED CHLOROPLASTS 1 is required for plastid separation in Arabidopsis. Proc. Nat. Acad. Sci. U.S.A. 108, 18530–18535. doi: 10.1073/pnas.1106706108
Zarnetske, P. L., Skelly, D. K., and Urban, M. C. (2012). Biotic multipliers of climate change. Science 336, 1516–1518. doi: 10.1126/science.1222732
Zenetos, A., Gofas, S., Morri, C., Rosso, A., Violanti, D., Raso, J. G., et al. (2012). Alien species in the Mediterranean sea by 2012. A contribution to the application of European Union's Marine Strategy Framework Directive (MSFD). part 2. introduction trends and pathways. Mediterr. Mar. Sci.13, 328–352. doi: 10.12681/mms.327
Zhang, Y. Y., Fischer, M., Colot, V., and Bossdorf, O. (2013). Epigenetic variation creates potential for evolution of plant phenotypic plasticity. New Phytol. 197, 314–322. doi: 10.1111/nph.12010
Zieman, J. C., Fourqurean, J. W., and Frankovich, T. A. (1999). Seagrass die-off in Florida Bay: long-term trends in abundance and growth of turtle grass, Thalassia testudinum. Estuaries 22, 460–470. doi: 10.2307/1353211
Keywords: Halophila stipulacea, alien species, invasiveness, Red Sea, Mediterranean Sea, Caribbean Sea, climate change, predictions
Citation: Winters G, Beer S, Willette DA, Viana IG, Chiquillo KL, Beca-Carretero P, Villamayor B, Azcárate-García T, Shem-Tov R, Mwabvu B, Migliore L, Rotini A, Oscar MA, Belmaker J, Gamliel I, Alexandre A, Engelen AH, Procaccini G and Rilov G (2020) The Tropical Seagrass Halophila stipulacea: Reviewing What We Know From Its Native and Invasive Habitats, Alongside Identifying Knowledge Gaps. Front. Mar. Sci. 7:300. doi: 10.3389/fmars.2020.00300
Received: 06 January 2020; Accepted: 15 April 2020;
Published: 26 May 2020.
Edited by:
Heliana Teixeira, University of Aveiro, PortugalReviewed by:
Jillian Lean-Sim Ooi, University of Malaya, MalaysiaCopyright © 2020 Winters, Beer, Willette, Viana, Chiquillo, Beca-Carretero, Villamayor, Azcárate-García, Shem-Tov, Mwabvu, Migliore, Rotini, Oscar, Belmaker, Gamliel, Alexandre, Engelen, Procaccini and Rilov. This is an open-access article distributed under the terms of the Creative Commons Attribution License (CC BY). The use, distribution or reproduction in other forums is permitted, provided the original author(s) and the copyright owner(s) are credited and that the original publication in this journal is cited, in accordance with accepted academic practice. No use, distribution or reproduction is permitted which does not comply with these terms.
*Correspondence: Gidon Winters, d2ludGVyc2dAYWRzc2Mub3Jn
Disclaimer: All claims expressed in this article are solely those of the authors and do not necessarily represent those of their affiliated organizations, or those of the publisher, the editors and the reviewers. Any product that may be evaluated in this article or claim that may be made by its manufacturer is not guaranteed or endorsed by the publisher.
Research integrity at Frontiers
Learn more about the work of our research integrity team to safeguard the quality of each article we publish.