- 1Key Laboratory of Aquatic Nutrition and Feed Science of Jiangsu Province, College of Animal Science and Technology, Nanjing Agricultural University, Nanjing, China
- 2National Laboratory of Animal Science, College of Animal Science and Technology, Nanjing Agricultural University, Nanjing, China
A previous study showed that diets with high levels of docosahexaenoic acid (DHA) cause growth retardation in Megalobrama amblycephala. In order to explore the mechanisms involved, a feeding trial and a primary hepatocyte culture experiment were designed. In vivo, fish (average weight 26.40 ± 0.11 g) were randomly divided into groups and were fed three levels of DHA (0, 0.2, and 1.6% diets, respectively.) for 8 weeks. The results showed that the final body weight and weight gain of fish fed 1.6% DHA were significantly lower than those of fish fed 0.2% DHA (P < 0.05). P53 gene and protein expression levels were significantly increased (P < 0.05), while expression of downstream Cyclin D1 and Cyclin E1 was significantly inhibited in the 1.6% DHA group when compared with the 0 and 0.2% DHA groups (P < 0.05). In vitro, primary hepatocytes isolated from Megalobrama amblycephala incubated with 500 μM DHA showed significantly increased cell cycle arrest and apoptosis (P < 0.05), significantly increased P53 gene and protein levels (P < 0.05), and significantly decreased Cyclin D1 and Cyclin E1 levels (P < 0.05) when compared with other groups. When primary hepatocytes were incubated with DHA and a P53 inhibitor (pifithrin-α), P53 expression and P53-mediated signaling were inhibited, cell cycle progression recovered, and apoptosis was reduced. In summary, high levels of DHA activated the P53/Cyclin pathway to induce cell cycle arrest. Inhibition of P53 activity may be a potential way of reducing the side effects of DHA on the growth of Megalobrama amblycephala.
Introduction
Docosahexaenoic acid (C22:6n-3; DHA) is an essential n-3 polyunsaturated fatty acid (n-3 PUFA) that is commonly used as a feeding supplement for aquatic animals (Hossain et al., 2012; Hong et al., 2017; Wang et al., 2019), pigs (Gabler et al., 2007; Li et al., 2009), chickens (Allen and Danforth, 1998; Howe et al., 2002), and other species. DHA is also an important health product for human use (Holub, 2001). Conversion of α-linolenic acid (C18:3n-3, ALA) into DHA is almost negligible in the human body, so DHA must be obtained directly from foods (Plourde and Cunnane, 2007). DHA supplementation at appropriate levels is beneficial to animals and humans, but an excess may produce adverse effects. DHA, a highly unsaturated fatty acid, is susceptible to attack by active free radicals, resulting in lipid peroxidation, cell membrane damage, and various physiological abnormalities and disease (Tappel, 1973). Some studies have focused on the damage caused by the peroxidation of DHA. A previous study reported that high DHA levels led to increased plasma urea concentration and fish redness (Glencross and Rutherford, 2011). MedihaYildirim-Aksoy et al. (2007) showed that fish fed excessive amounts of fish oil had abnormally high red and white blood cell counts and suffered from infection or disease. However, there have been very few studies focusing on the damage caused by unoxidized DHA. Our previous study found that a high level of DHA reduced growth performance, indicating that excessive DHA, not oxidized, may be harmful to fish growth (Wang et al., 2019). Investigating whether there is possible damage from high levels of unoxidized DHA is important for human and animal nutrition.
P53, a tumor suppressor, is a key transcriptional regulator that affects various physiological functions, such as apoptosis (Yonish-Rouach et al., 1991; Chipuk and Green, 2004), cell cycle progression (Livingstone et al., 1992; Agarwal et al., 1995) and antiviral immunity (Takaoka et al., 2003; Munoz-Fontela et al., 2008), by regulating relevant genes (Lane, 1992; Francis et al., 2007). When cells are stimulated by the external environment, P53 can become activated to maintain a stable internal environment by regulating a series of related genes (Lane, 1992). P53 has been widely studied in mammals, where it plays an indispensable role (Levine, 1997; El-Deiry, 1998), but there have been few studies on the physiological functions of P53 in lower vertebrates (such as fish). Mai et al. (2012) reported that P53 is cooperatively activated via the ataxia telangiectasia mutated (ATM)-P53 pathway in response to stress and DNA damage signals in Tilapia. On the other hand, Guo et al. (2016) showed that P53 plays a critical role in the immune and antiviral responses of mandarin fish (Siniperca chuatsi).
The blunt snout bream (Megalobrama amblycephala) is an herbivorous freshwater fish and teleost species (Wang et al., 2019) that is vulnerable to oxidative stress and hepatocyte apoptosis when it is fed high-fat diets (Lu et al., 2017). This is because it has an herbivorous instinct and a relatively small hepatosomatic index (HSI) (Zhang et al., 2014; Cao et al., 2019a). Hence, this species is a suitable model to study fatty acid toxicity. To investigate why high levels of DHA reduced the growth of M. amblycephala (Wang et al., 2019) and to explore the causes of problems caused by excessive fish oil in fish farming, in this study we exposed fish and hepatocytes to high levels of DHA and investigated its effects in vivo and in vitro, which will be beneficial for elucidating the DHA nutrition of other animals and humans.
Materials and Methods
All experimental procedures involving animals were conducted following the Guidance for the Care and Use of Laboratory Animals in China. This study was approved by the Animal Care and Use Committee of Nanjing Agricultural University (Nanjing, China) [Permit number: SYXK (Su) 2011–0036].
Diets, Feeding Trial, and Sample Collection
Three diets containing different DHA levels were prepared. The dietary DHA levels were 0, 0.2, and 1.6%, respectively. The 0% DHA diet was given to the control group. The three diets contained 31% crude protein and 6% crude lipids. Proteins were supplied by defatted fishmeal (3.5% lipid), soybean meal, rapeseed meal, and cottonseed meal. Lipids were supplied by soybean and DHA oils (DHA content, 50% of oil). Ethoxyquin was used as an antioxidant. Freshly prepared diets were kept out of direct sunlight, dried by ventilation, and stored at –20°C. The fatty acid composition of soybean oil and DHA oil and the formulation of six diets is referenced in our previous study (Supplementary Table S1, S2; Wang et al., 2019).
M. amblycephala was obtained from the Fish Hatchery of Yangzhou (Jiangsu, China). The fish were fed commercial diets for 2 weeks to allow them to adapt to the environment. A total of 240 fish (average initial body weight 26.40 ± 0.11 g) were randomly distributed into 12 floating net cages (2 × 1 × 1 m, L: W: H), with four cages per group and with 20 fish in each cage. All fish were hand-fed to satiation thrice a day (8:00, 12:00, and 16:00) for 8 weeks. The presence of dead fish, water temperature (28–34°C), dissolved oxygen (5.0 mg L–1), and pH (7.4–7.6) were recorded daily.
After 8 weeks, fish were anesthetized with MS-222 (100 mg L–1, tricaine methanesulfonate, Sigma, United States). The quantity and weight of the fish in each cage were recorded. Three fish were randomly sampled per cage (total per group, 12 fish). Livers were collected, frozen in liquid nitrogen, and stored at –80°C for molecular analyses.
Primary Hepatocyte Culture
The primary hepatocyte culture was performed according to Cao et al. (2019a). Briefly, the liver was carefully removed under sterile conditions and rinsed with sterile phosphate buffer saline (PBS) supplemented with streptomycin (100 μg mL–1) and penicillin (100 IU mL–1). The liver was cut into pieces (1 mM3) with sterilized tweezers and scissors, and the liver pieces were digested with 0.25% trypsin in a thermostatic water bath at 28°C for 40 min; the liquid was shaken every 5 min. The digested liver solution was filtered with a nylon cell strainer (200 mesh) and collected into a sterilized 50-mL centrifugal tube. Next, the filtrate was centrifuged (300 × g, 10 min) to obtain the cell pellet. The pelleted hepatocytes were resuspended in DMEM/F12 medium containing glutamine (1 mmol L–1), 10% FBS, streptomycin (100 μg mL–1), and penicillin (100 IU mL–1). The hepatocytes were cultured at a density of 106 cells mL–1 in a 5% CO2 incubator at 28°C for 48 h. After 24 h of DHA treatment, the cells were collected for subsequent analysis. The reagents were prepared as follows: DHA (Sigma, D2534, United States) and the P53 inhibitor, pifithrin-α (Apexbio, A4206, United States), were dissolved in dimethyl sulfoxide (DMSO) and mixed thoroughly with cell media containing FBS, following the procedure described by Ferguson et al. (2018). The final concentrations of DHA were 100, 500, and 1,000 μM, and the final concentration of pifithrin-α was 10 μM. Media and vehicle alone were added to the controls. All the solutions were stored in the dark at –20°C.
We designed a small experiment to test whether DHA was oxidized. Cells were collected and lysed 0 h and 24 h after being exposed to 500 μM DHA. Media and vehicle were the control groups. The DHA content was measured with the GC/MS (Trace ISQ, United States) method. The results are shown in Supplementary Figure S1.
Quantitative Real-Time PCR
Total RNA was extracted from liver and hepatocytes with Trizol reagent (Invitrogen, United) and treated with RQ1 RNase-free DNase (Promega, United States) to eliminate genomic DNA contamination. The concentration of the extracted RNA was determined by spectrophotometric analysis, and the purity was assessed based on the OD260/OD280 ratio (1.8–2.0). cDNA was generated from 500 ng of DNase-treated RNA using the ExScriptTM RT-PCR kit (Takara, Japan). The PCR protocol is described in Cao et al. (2019b), and the target cDNA was diluted and amplified using the SYBR Green II Fluorescence Kit (Takara, Japan). Following Livak and Schmittgen (2001), relative gene expression was calculated using the 2–Δ Δ CT method.
The normalized gene expression of the control groups (0% DHA in vivo; media alone in vitro) was set to 1. Normalized gene expression of the control group (0% DHA in vivo; Media group in vitro) was set to 1. The ATM, Checkpoint kinase 2 (Chk-2), P53, Cyclin D1, Cyclin E1, Cyclin A2, Cyclin B1, and Elongation factor 1α (EF1α) primers used are shown in Table 1. EF1α was used as the reference for relative quantification.
Western Blot Analysis
RIPA lysis buffer (#ab156034, Abcam, United Kingdom) was added to the liver and hepatocytes, and total proteins were extracted on ice with a glass tissue grinder (Kimble Chase, United States). Protein concentration was quantified using a BCA protein assay kit (Tiangen, China). Heat-denatured protein lysates (20 μg of protein) were separated by reducing SDS-PAGE electrophoresis and transferred to a PVDF membrane. The primary antibodies used had the following specificities: β-Actin (ABclonal, AC004, China), ATM (ABclonal, A18257, China), Chk-2 (ABclonal, A2145, China), P53 (ABclonal, A0263, China), Cyclin D1 (ABclonal, A11310, China), Cyclin E1 (ABclonal, A14225, China), Cyclin A2 (ABclonal, A2891, China), and Cyclin B1 (ABclonal, A2056, China). The secondary antibodies used were the following: goat anti-rabbit (IgG H + L: ABclonal, AS003, China) and goat anti-mouse (IgG H + L: ABclonal, AS014, China). The bands were visualized by adding electrochemiluminescence (ECL) reagents (GoodHere, China) and with a luminescent image analyzer (Fujifilm LAS-3000, Japan). Protein levels were quantified using Image J software (National Institutes of Health, United States).
Cell Cycle Assay
Five treatments (Media, Vehicle, Pifithrin-α, 500 μM DHA, and 500 μM DHA + Pifithrin-α) were added to hepatocytes for 24 h, then the hepatocytes were collected and rinse twice with cold PBS. The hepatocytes were resuspended in 400 μL PBS, after which 300 μL cold absolute 75% ethanol was added to the cell suspensions, which were then fixed overnight at 4°C. Precipitated cells were collected by centrifugation the next day. Next, 400 μL propidium iodide (50 μg mL–1 PI) solution was added to incubate for 30 min (4°C, light avoided). Cells were immediately detected with an Accuri C6 II flow cytometer (Beckman, United States). Hepatocytes were exposed to five different conditions (media alone, vehicle, pifithrin-α, 500 μM DHA, and 500 μM DHA + Pifithrin-α) for 24 h. After 24 h, hepatocytes were collected, rinsed twice with cold PBS, and resuspended in 400 μL PBS. Next, 300 μL of ice-cold 75% ethanol was added to the cell suspensions, for overnight fixation at 4°C. The precipitated cells were collected by centrifugation the next day, 400 μL of propidium iodide (PI) solution was added, and the cell suspensions were incubated for another 30 min at 4°C in the dark. Cells were analyzed with an Accuri C6 II flow cytometer (Beckman, United States).
Apoptosis Assay
Cellular apoptosis was measured by annexin V-FITC/PI staining and flow cytometry (FCM) analysis. Hepatocytes were exposed in triplicate to five different conditions (media alone, vehicle, pifithrin-α, 500μM DHA, and 500μM DHA + pifithrin-α) for 24 h. After removing the medium, the hepatocytes were rinsed twice with cold PBS and collected in a flow tube, and 400 μL of 1X annexin V binding buffer was added to resuspend the cells (106 cells mL–1). Next, 5 μL of annexin V-FITC solution was added, and the cells were incubated for 15 min at 4°C in the dark, followed by 10 μL of PI and an additional 5 min incubation at 4°C in the dark. Cells were immediately analyzed with the Accuri C6 II flow cytometer (Beckman, United States). After fluorescence compensation, the annexin V-FITC (FL1) fluorescence was set as the abscissa, and the PI (FL2) fluorescence was set as the ordinate. Results are shown as quadrant dot plots and percentage of stained cells.
Statistical Analysis
The normality and homogeneity of the data were evaluated by the Kolmogorov-Smirnov and Levene tests. All data were analyzed by one-way ANOVA using SPSS 16.0 (SPSS, United States) for Windows. Significant differences between groups were determined by the Tukey multiple range test. The level of significance was set at P < 0.05. All results are expressed as the mean ± S.E.M. (standard error of the mean) of four replicates (n = 4) in vivo experiments and three replicates (n = 3) f in vitro.
Results
Results in vivo
Growth Performance
As shown in Figure 1, the final body weight and weight gain of fish fed 1.6% DHA was significantly lower than that of fish fed 0.2% DHA (P < 0.05).
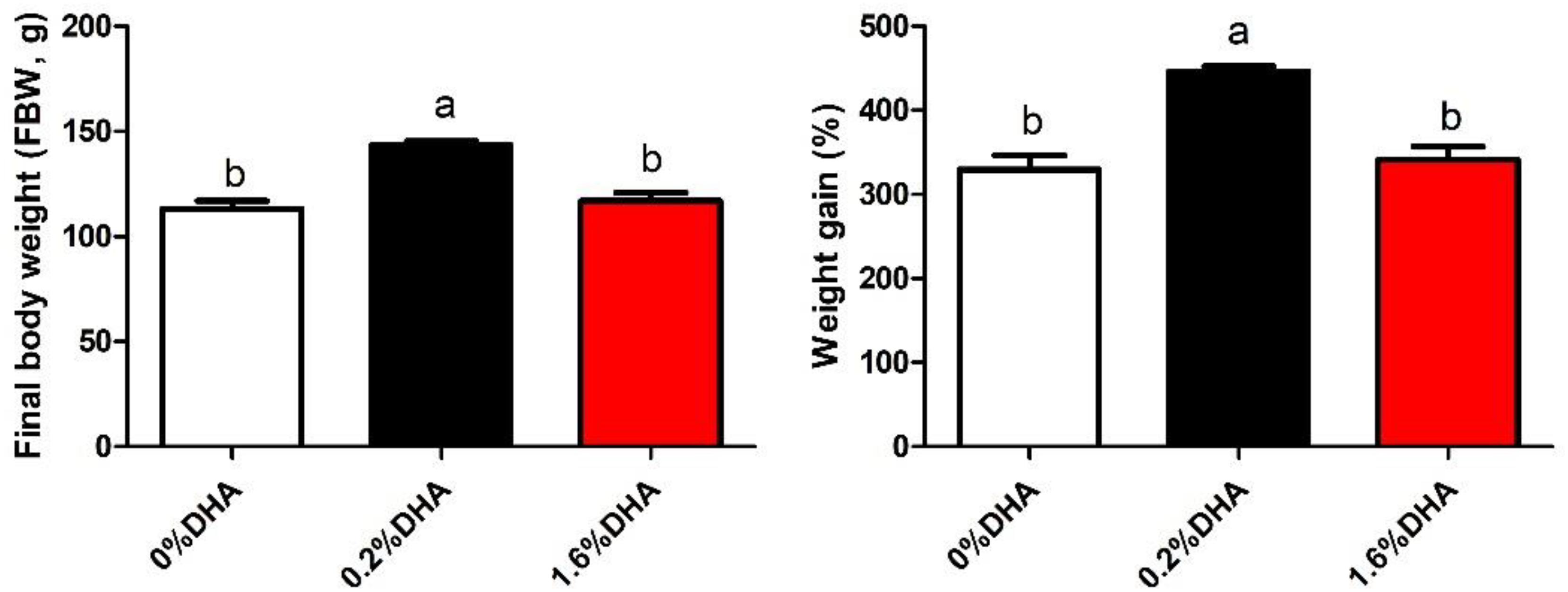
Figure 1. Growth of M. amblycephala fed the experimental diets. Values are means ± SEM of four replicates (n = 4) represented by vertical bars. Different superscript letters indicate significantly different values (P < 0.05). Final weight (g) = total final weight/total number. Weight gain rate (WGR,%) = (Final body weight - initial body weight) × 100/initial body weight.
Gene Expression in Liver
As shown in Figure 2, ATM, Chk-2, and P53 gene expression levels were significantly higher (Figures 2A–C), while Cyclin D1 and Cyclin E1 gene expression levels were significantly lower, in the 1.6% DHA group when compared with the control and 0.2% DHA groups (P < 0.05; Figures 2D,E). There were no significant differences in Cyclin A2 and Cyclin B1 gene expression between treatments (P > 0.05; Figures 2F,G).
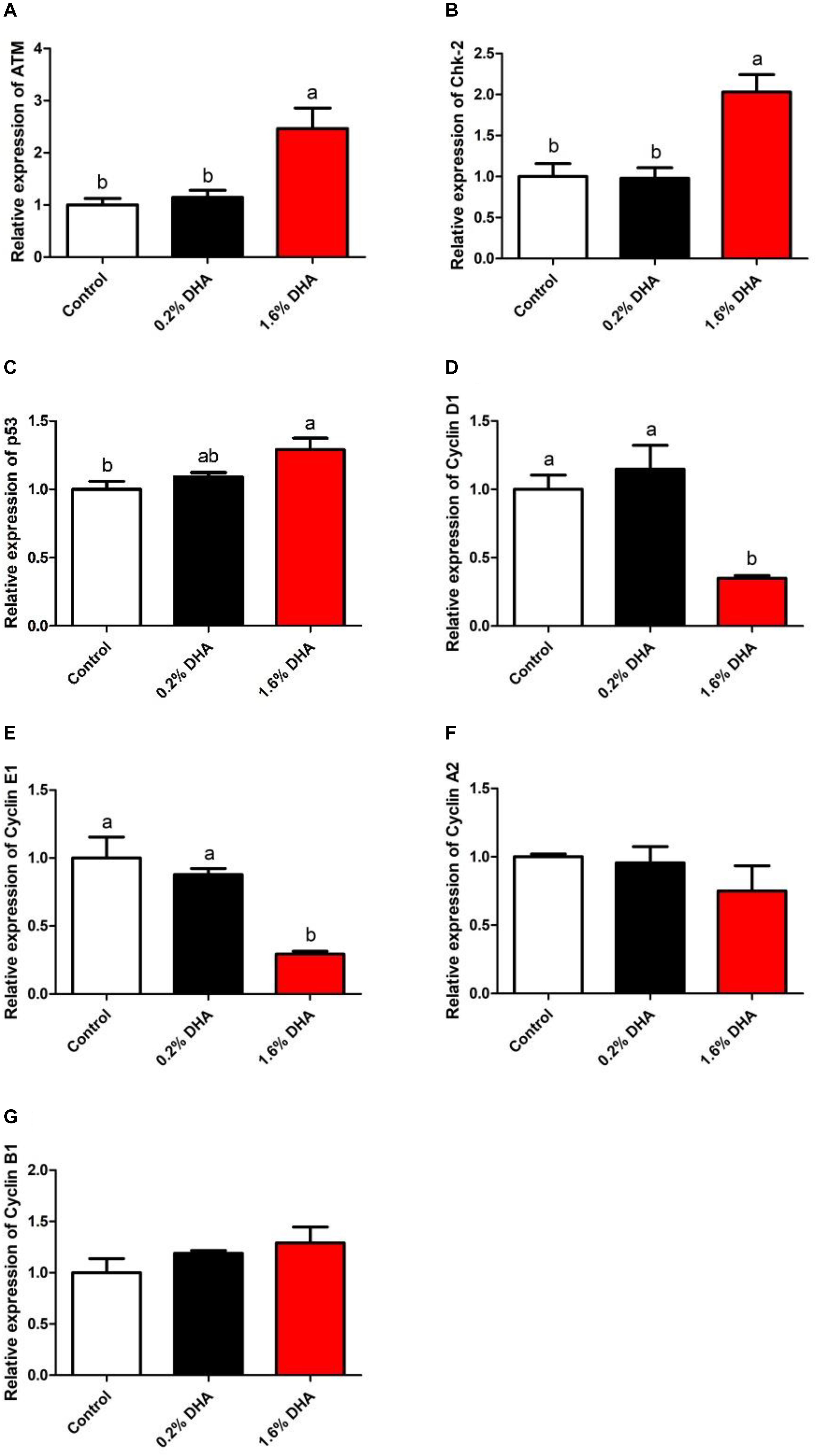
Figure 2. Relative gene expression of ATM, Chk-2, P53, CyclinD1, CyclinE1, CyclinA2, and CyclinB1 in the liver of M. amblycephala fed the experimental diets (A–G). Values are means ± SEM of four replicates (n = 4) represented by vertical bars. Different superscript letters indicate significantly different values (P < 0.05).
Protein Expression in Liver
Compared with the control group, fish fed 1.6% DHA showed significantly higher hepatic ATM and P53 protein levels (Figures 3A,C), whereas Cyclin D1, Cyclin E1, and Cyclin A2 protein levels were significantly lower (P < 0.05; Figures 3D,E,G). No significant differences were observed in Chk-2 and Cyclin B1 protein levels between the three groups (P > 0.05; Figures 3B,F).
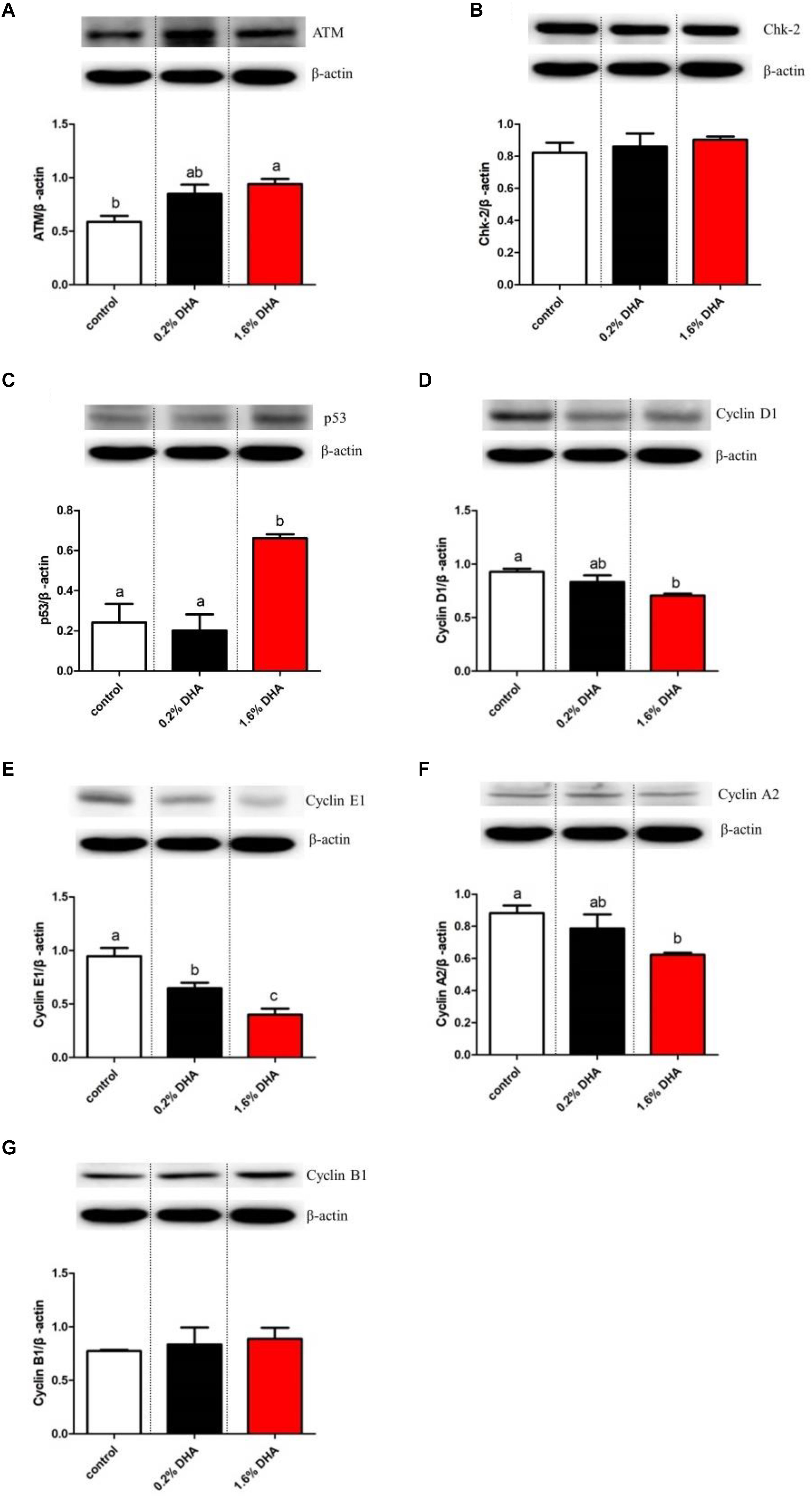
Figure 3. Western blot analysis of ATM, Chk-2, P53, CyclinD1, CyclinE1, CyclinA2, and CyclinB1 in the liver of M. amblycephala fed the experimental diets (A–G). Values are means ± SEM of four replicates (n = 4) represented by vertical bars. Different superscript letters indicate significantly different values (P < 0.05).
Results in vitro
Three concentrations of DHA (100, 500, and 1,000 μM) were tested for toxic effects in hepatocytes (Supplementary Figure S2). DHA significantly influenced the ATM and P53 gene expression levels, which peaked at 500 μM DHA and decreased when the DHA concentration was increased to 1,000 μM.
Cell Cycle Arrest
Treatment with 500 μM DHA significantly increased the ratio of hepatocytes in the G1 phase and decreased the ratio of hepatocytes in the S phase (P < 0.05; Figure 4). On the other hand, treatment with both 500 μM DHA and pifithrin-α significantly decreased the ratio of hepatocytes in the G1 phase when compared with the 500 μM DHA group (P < 0.05; Figure 4).
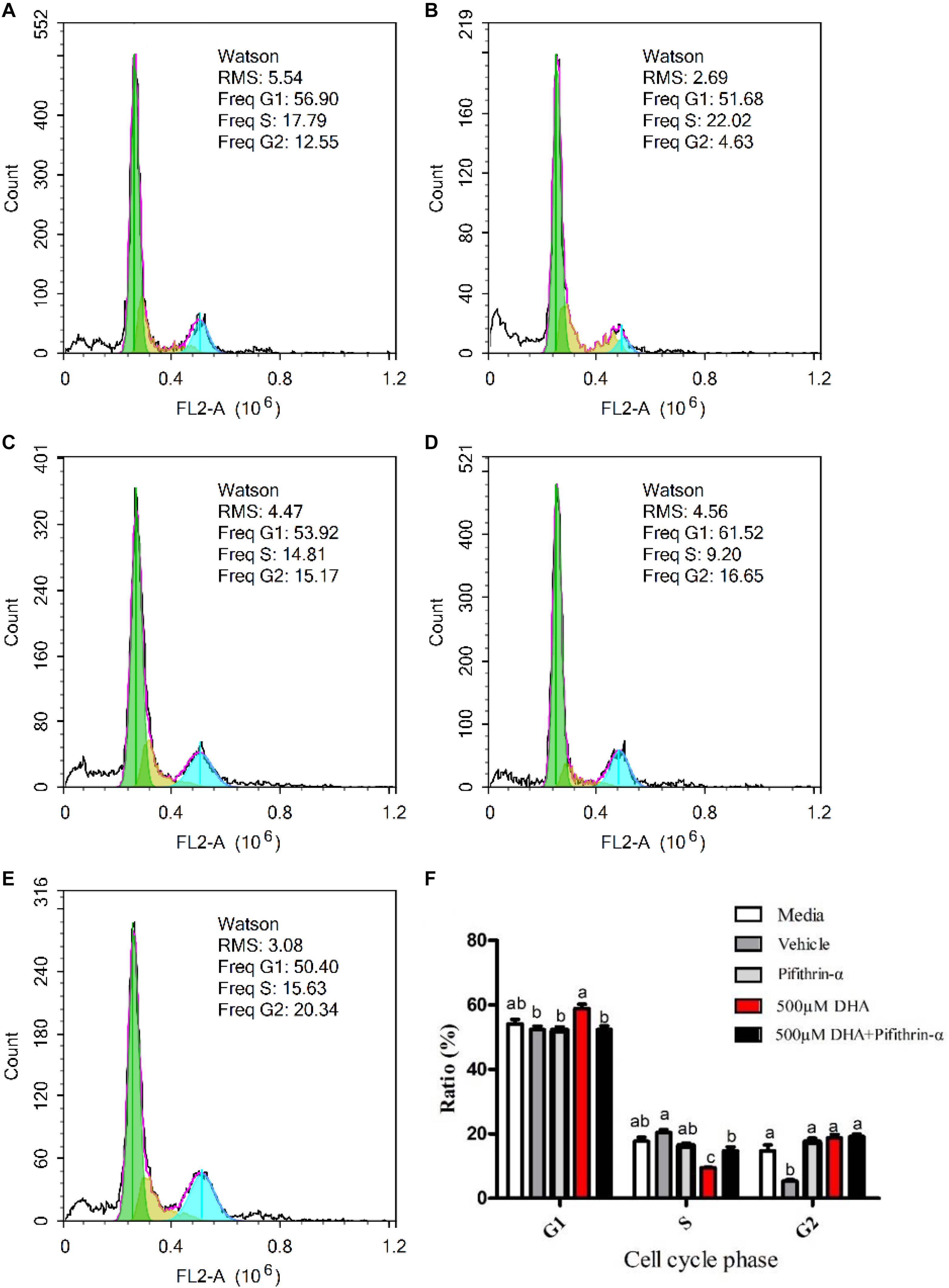
Figure 4. Effects of DHA on hepatocytes cell cycle. The cell cycles of the hepatocytes exposed to Media, Vehicle, Pifithrin-α, 500 μM DHA, and 500 μM DHA + Pifithrin-α, respectively (A–E). The ratio of cells at the G1, S, and G2 phases were measured by flow cytometry (F). Values are means ± SEM of three replicates (n = 3) represented by vertical bars. RMS: root mean square. Different superscript letters indicate significantly different values (P < 0.05).
Apoptosis in Primary Hepatocytes
Figure 5 shows apoptosis in DHA-treated hepatocytes. The apoptosis rate (early and late) was significantly increased in hepatocytes treated with 500 μM DHA when compared with other groups (P < 0.05; Figures 5-1D). Treatment of cells with pifithrin-α significantly decreased the DHA-induced apoptosis rate (P < 0.05; Figures 5-1E, 5-2).
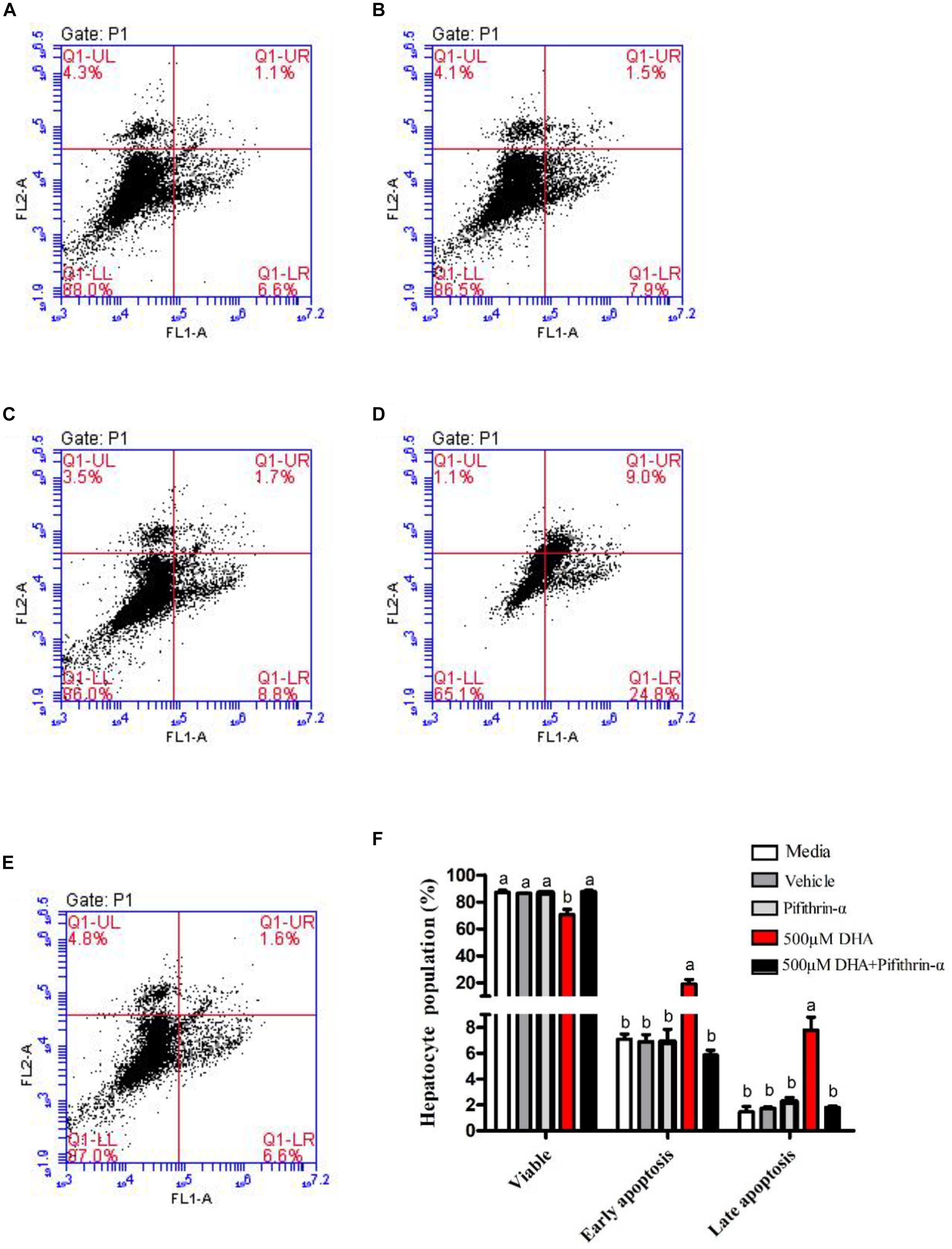
Figure 5. Effects of DHA on hepatocyte apoptosis. The apoptosis of hepatocytes exposed to Media, Vehicle, Pifithrin-α, 500 μM DHA, and 500 μM DHA + Pifithrin-α, respectively (A–E). The percentages of apoptotic cells were measured by flow cytometry (F). Values are means ± SEM of three replicates (n = 3) represented by vertical bars. Different superscript letters indicate significantly different values (P < 0.05).
Gene Expression in Hepatocytes
ATM and P53 gene expression levels were significantly increased (Figures 6A,C), while downstream Cyclin D1 and Cyclin E1 gene expression levels were significantly reduced, in hepatocytes treated with 500 μM DHA when compared with the control and co-treated (500 μM DHA + pifithrin-α) groups (P < 0.05; Figures 6A,C–E). There were no significant differences in Chk-2 and Cyclin B1 gene expression levels between treatments (P > 0.05; Figures 6B,F).
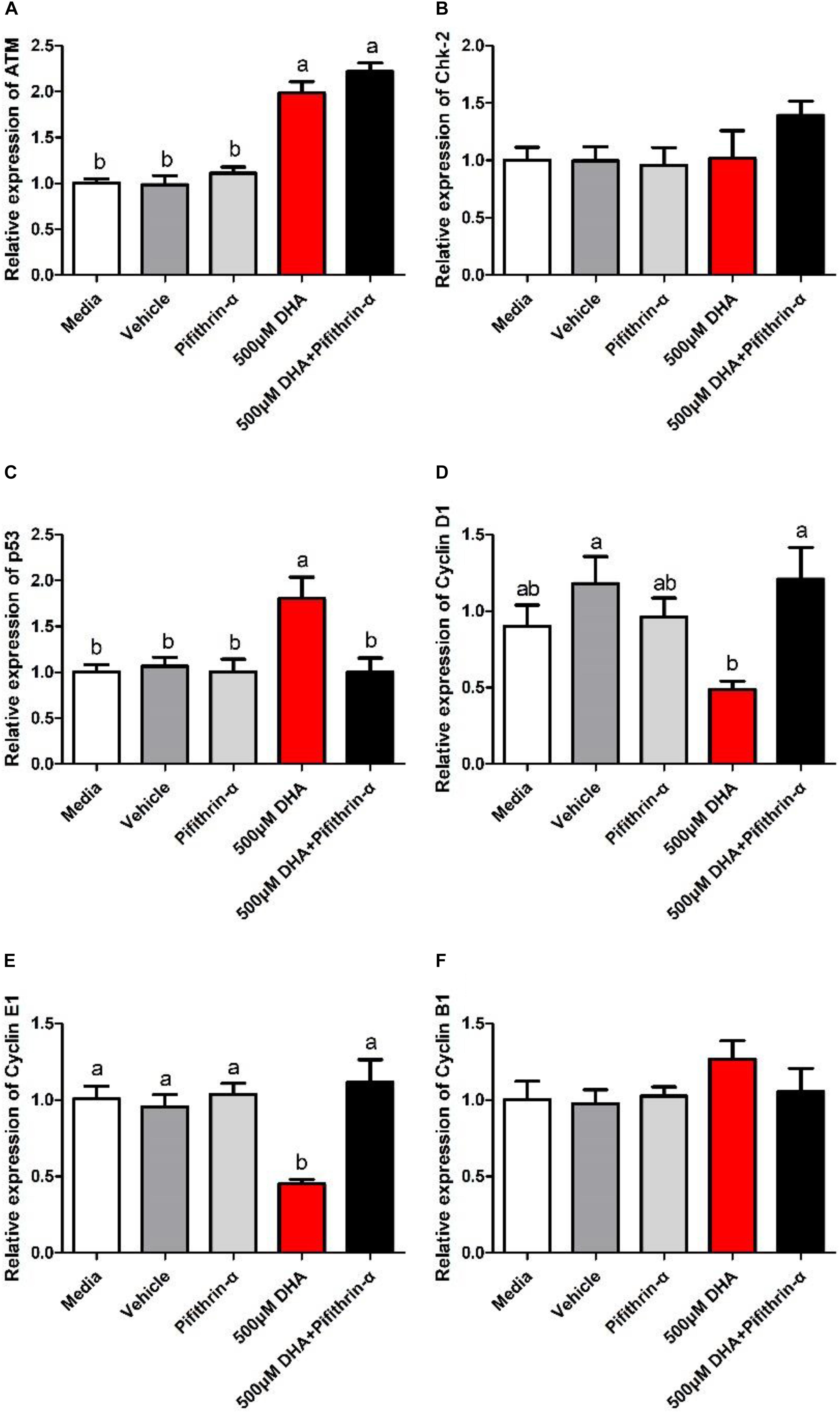
Figure 6. Relative gene expression of ATM, Chk-2, P53, CyclinD1, CyclinE1, and CyclinB1 in the hepatocytes exposed to Media, Vehicle, Pifithrin-α, 500 μM DHA, and 500 μM DHA + Pifithrin-α, respectively (A–F). Values are means ± SEM of three replicates (n = 3) represented by vertical bars. Different superscript letters indicate significantly different values (P < 0.05).
Protein Expression in Hepatocytes
P53 protein levels were significantly upregulated (P < 0.05; Figure 7B), while Cyclin D1 and Cyclin E1 protein levels were significantly downregulated, in hepatocytes treated with 500 μM DHA when compared with other groups (P < 0.05; Figures 7C,D). On the other hand, P53 protein levels were significantly reduced (Figure 7B), whereas Cyclin D1 and Cyclin E1 protein levels were significantly increased, in hepatocytes treated with both 500 μM DHA and pifithrin-α when compared with the 500 μM DHA group (P < 0.05; Figures 7C,D). There were no significant differences in Chk-2 and cyclin B1 protein levels (P > 0.05; Figures 7A,E).
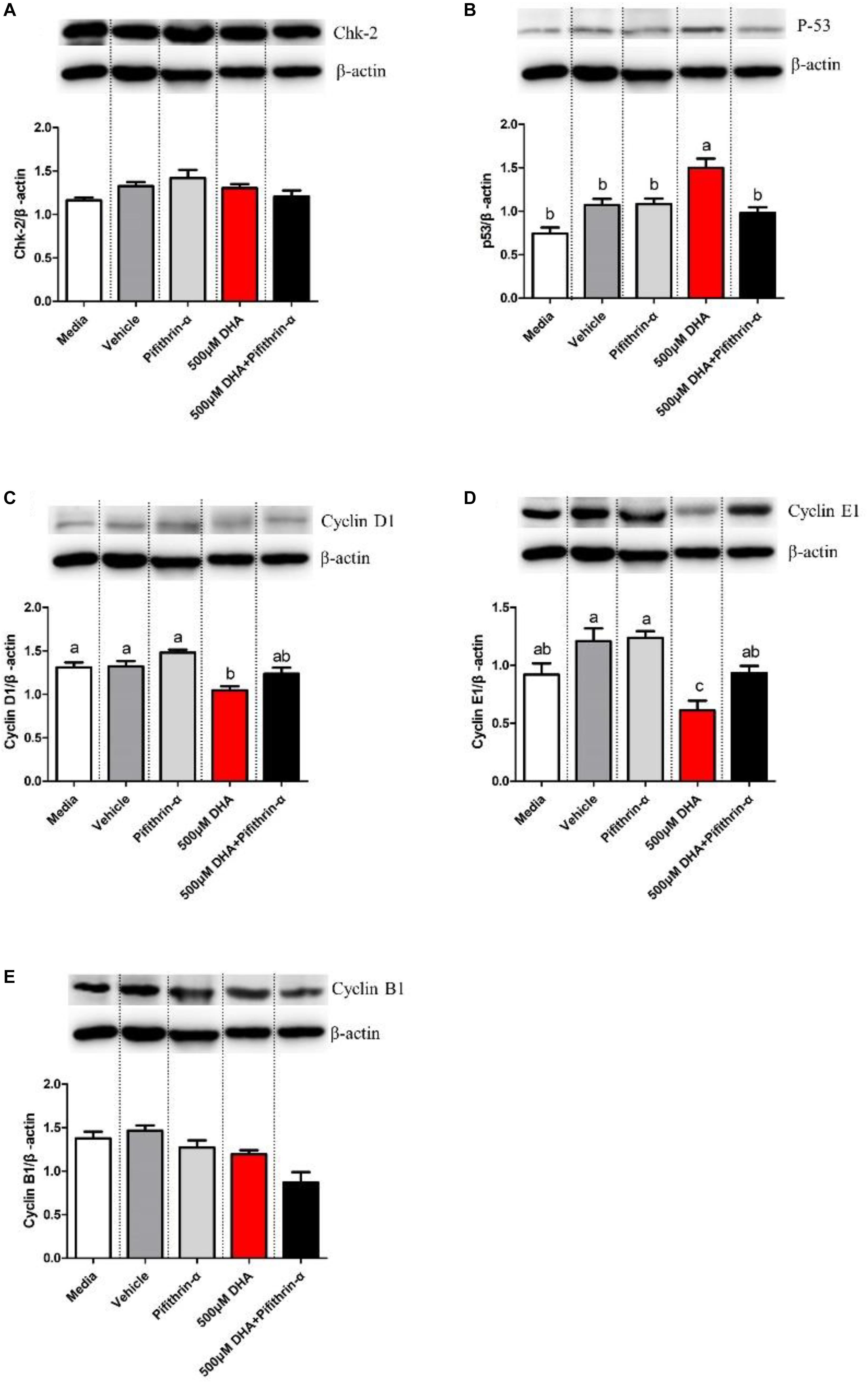
Figure 7. Western blot analysis of Chk-2, P53, CyclinD1, CyclinE1, and CyclinB1 in the hepatocytes exposed to Media, Vehicle, Pifithrin-α, 500 μM DHA, and 500 μM DHA + Pifithrin-α, respectively (A–E). Values are means ± SEM of three replicates (n = 3) represented by vertical bars. Different superscript letters indicate significantly different values (P < 0.05).
Discussion
DHA plays an important role not only regulating the growth (Villalta et al., 2005; Moran et al., 2018), dietary oil source selection (Haak et al., 2008), and nutritional qualities (Raes et al., 2004; Moreno-Indias et al., 2012) of commercially important species but also in human health (Conquer and Holub, 1998; Holub, 2001). This study and our previous study found that excess DHA caused growth retardation in blunt snout bream (Wang et al., 2019). Glencross and Rutherford (2011) also found that high doses of DHA decreased growth and led to an increase in plasma urea concentration and fish redness in juvenile barramundi (Lates calcarifer). DHA has been well investigated as a health supplement, but why would fish fed high levels of DHA show such effects? This is an interesting result that needs to be explored.
The cell cycle is divided into cellular interphase and mitosis. In turn, the cellular interphase is divided mainly into gap1 (G1), DNA synthesis (S), and gap2/mitosis (G2/M) phases (Ishikawa et al., 2006; Chen et al., 2017; Dai et al., 2019). The cyclin family plays a pivotal role in regulating the timing and progression of the cell cycle (Ohki et al., 2000). P53 is a potent cyclin-dependent kinase inhibitor that regulates the expression of genes associated with cell growth and the cell cycle (Ohki et al., 2000; Francis et al., 2007). Previous studies reported that multiple inflammatory factors could activate the ATM-Chk2-P53 signaling pathway to induce cell cycle arrest (Chen et al., 2017; Dai et al., 2019). Also, Mai et al. (2012) reported that P53 was cooperatively activated by the ATM-P53 pathway in response to stress and DNA-damaging signals in Oreochromis niloticus. In this study, the effects of DHA on cell cycle regulation in Megalobrama amblycephala were analyzed, including the P53 pathway (P53, Chk2, and ATM), G1 phase (Cyclin D1, Cyclin E1), S phase (Cyclin A2), and G2/M phase (Cyclin B1) (Huang et al., 2010). The feeding trial showed that ATM and P53 were activated in response to 1.6% DHA, indicating the possibility of DNA damage and apoptosis. On the other hand, downstream Cyclin D1 and Cyclin E1, which promote G1/S phase progression (Cunningham et al., 2009), were inhibited. These results indicate that high levels of DHA affect the cell cycle, resulting in decreased growth of M. amblycephala. To better understand the negative effects of high levels of DHA, we conducted in vitro experiments with primary hepatocytes.
When primary hepatocytes were incubated with DHA, 1,000 μM DHA has a lower effect on the gene expression of ATM and P53 than 500 μM, but they were all significantly higher than the control group. This result may indicate that 500 μM caused cell damage. The reason for the downward trend at 1,000 μM is still worthy of further study. When primary hepatocytes were incubated with 500 μM DHA, the ratio of cells in the G1 phase was significantly increased, a result that was consistent with the observed reduction of cyclin D1 and cyclin E1gene and protein expression levels. These results indicate that high levels of DHA induce cell cycle arrest in the G1 phase. Other compounds that can influence the cell cycle have been reported. For example, Chen et al. (2017) reported that cyclin E1 and cyclin A1 mRNA levels decreased in male zebrafish treated with 229.05 μg/L TDCPP, suggesting cell cycle arrest at the G1 to S phase. Cell cycle arrest is usually accompanied by increased apoptosis (Slotkin and Seidler, 2012). For example, a previous study found that tris (1,3-dichloro-2-propyl) phosphate (TDCPP) induced cell cycle arrest and caused cell apoptosis in zebrafish (Huang et al., 2010). Saquib et al. (2012) showed that it induced cell cycle arrest and apoptosis, adversely affecting normal cellular function in rats. In this study, 500 μM DHA induced cell cycle arrest and apoptosis, indicating that excess DHA could affect normal cellular function in Megalobrama amblycephala. Similar results have been reported in previous studies using tumor cell lines, demonstrating that apoptosis is a common response to DHA (Fasano et al., 2012; Sam et al., 2016; Song and Kim, 2016). However, very few studies have investigated the negative effects of high DHA levels on normal organisms or cells. Although DHA is a common health supplement and has excellent anti-cancer effects (Conquer and Holub, 1998), our results indicate that excessive DHA is likely to have adverse effects on normal cellular function. This is worthy of attention and should be verified in other animal species.
P53 and downstream (cyclin D1 and cyclin E1) gene and protein expression levels were significantly affected by 500 μM DHA in our in vitro experiments, which was consistent with the results of the in vivo trial. When primary hepatocytes were incubated with a P53 inhibitor, P53-mediated signaling was inhibited, cell cycle progression recovered, and apoptosis decreased, suggesting that DHA-induced cytotoxicity is mediated by P53. Hence, high levels of DHA caused negative effects in Megalobrama amblycephala, which may be attributed to the activated P53/cyclin pathway. Similarly, Zand et al. (2007) documented how DHA induced apoptosis through P53. Another study with zebrafish also showed that cell cycle arrest and apoptosis were induced through the activation of P53 (Zhu et al., 2015). Thus, inhibition of P53 activity could be a potential approach to reduce cell damage in fish fed DHA.
Conclusion
In summary, the feeding trial showed that 1.6% DHA in the diet has no “growth-promoting” effect in Megalobrama amblycephala when compared with 0% DHA. In primary hepatocytes, 500 μM DHA treatment significantly induced cell cycle arrest in the G0/G1 phase and, consequently, apoptosis. In vivo and in vitro, high levels of DHA significantly upregulated P53 gene and protein expression and decreased downstream cyclin D1 and cyclin E1 expression. Thus, DHA can activate the P53/cyclin pathway to induce cell cycle arrest. Inhibiting P53 activity could be a potential way of reducing the side effects of DHA on the growth of Megalobrama amblycephala.
Data Availability Statement
All datasets generated for this study are included in article/Supplementary Material.
Ethics Statement
This animal study was approved by the Animal Care and Use. Committee of Nanjing Agricultural University (Nanjing, China) (permit number: SYXK (Su) 2011–0036).
Author Contributions
CW was responsible for doing experiments, analyzing data, and writing the article. GJ provided substantial contributions to the conception and design of experiments. WL provided valuable advice. YH, XW, and KX assisted in experiments. XC, XL, and DZ provided writing assistance.
Funding
This research was supported by the Youth Talent Project for the College of Animal Science and Technology of Nanjing Agricultural University (DKQB201902) and the China Agriculture Research System (CARS-45-14).
Conflict of Interest
The authors declare that the research was conducted in the absence of any commercial or financial relationships that could be construed as a potential conflict of interest.
Supplementary Material
The Supplementary Material for this article can be found online at: https://www.frontiersin.org/articles/10.3389/fmars.2020.00286/full#supplementary-material
References
Agarwal, M. L., Agarwal, A., and Stark, T. G. R. (1995). P53 controls both the g2/m and the g1cell cycle checkpoints and mediates reversible growth arrest in human fibroblasts. Proc. Natl. Acad. Sci. U.S.A. 92, 8493–8497. doi: 10.2307/2368084
Allen, P. C., and Danforth, H. D. (1998). Effects of dietary supplementation with n-3 fatty acid ethyl esters on coccidiosis in chickens. Poult. Sci. 77, 1631–1635. doi: 10.1093/ps/77.11.1631
Cao, X. F., Dai, Y. J., Liu, M. Y., Yuan, X. Y., Wang, C. C., Huang, Y. Y., et al. (2019a). High-fat diet induces aberrant hepatic lipid secretion in blunt snout bream by activating endoplasmic reticulum stress-associated IRE1/XBP1 pathway. Biochim. Biophys. Acta 1864, 213–223. doi: 10.1016/j.bbalip.2018.12.005
Cao, X. F., Liu, W. B., Zheng, X. C., Yuan, X. Y., Wang, C. C., and Jiang, G. Z. (2019b). Effects of high-fat diets on growth performance, endoplasmic reticulum stress and mitochondrial damage in blunt snout bream Megalobrama amblycephala. Aquacult. Nutr. 25, 97–109. doi: 10.1111/anu.12834
Chen, H., Wang, P., Du, Z., Wang, G., and Gao, S. (2017). Oxidative stress, cell cycle arrest, DNA damage and apoptosis in adult zebrafish (Danio rerio) induced by tris(1,3-dichloro-2-propyl) phosphate. Aquat. Toxicol. 194:37. doi: 10.1016/j.aquatox.2017.11.001
Chipuk, J. E., and Green, D. R. (2004). Direct activation of Bax by p53 mediates mitochondrial membrane permeabilization and Apoptosis. Science 303, 1010–1014. doi: 10.1126/science.1092734
Conquer, J. A., and Holub, B. J. (1998). Effect of supplementation with different doses of dha on the levels of circulating dha as non-esterified fatty acid in subjects of asian indian background. J. Lipid Res. 39:286. doi: 10.1089/jir.1998.18.125
Cunningham, J. M., Vierkant, R. A., Sellers, T. A., Phelan, C., Rider, D. N., Liebow, M., et al. (2009). Cell cycle genes and ovarian cancer susceptibility: a tagSNP analysis. Br. J. Cancer 101, 1461–1468. doi: 10.1038/sj.bjc.6605284
Dai, Y. J., Cao, X. F., Zhang, D. D., Li, X. F., Liu, W. B., and Jiang, G. Z. (2019). Chronic inflammation is a key to inducing liver injury in blunt snout bream (Megalobrama amblycephala) fed with high-fat diet. Dev. Compar. Immunol. 97, 28–37. doi: 10.1016/j.dci.2019.03.009
Fasano, E., Serini, S., Piccioni, E., Toesca, A., Monego, G., Cittadini, A. R., et al. (2012). Dha induces apoptosis by altering the expression and cellular location of grp78 in colon cancer cell lines. Biochim. Biophys. Acta 1822, 1762–1772. doi: 10.1016/j.bbadis.2012.08.003
Ferguson, J. F., Roberts, K., Borcea, C., Smith, H. M., Midgette, Y., and Shah, R. (2018). Omega-3 polyunsaturated fatty acids attenuate inflammatory activation and alter differentiation in human adipocytes. J. Nutr. Biochem. 64, 45–49. doi: 10.1016/j.jnutbio.2018.09.027
Francis, R., Judith, C., and Dipa, B. (2007). Two faces of p53: aging and tumor suppression. Nucleic Acids Res. 35, 7475–7484. doi: 10.1093/nar/gkm744
Gabler, N. K., Spencer, J. D., Webel, D. M., and Spurlock, M. E. (2007). In utero and postnatal exposure to long chain (n-3) pufa enhances intestinal glucose absorption and energy stores in weanling pigs. J. Nutr. 137, 2351–2358. doi: 10.1093/jn/137.11.2351
Glencross, B., and Rutherford, N. (2011). A determination of the quantitative requirements for docosahexaenoic acid for juvenile barramundi (Lates calcarifer). Aquacult. Nutr. 17, e536–e548. doi: 10.1111/j.1365-2095.2010.00795.x
Guo, H., Fu, X., Li, N., Lin, Q., Liu, L., and Wu, S. (2016). Molecular characterization and expression pattern of tumor suppressor protein p53 in mandarin fish, siniperca chuatsi following virus challenge. Fish Shellfish Immunol. 51, 392–400. doi: 10.1016/j.fsi.2016.03.003
Haak, L., De Smet, S., Fremaut, D., Van Walleghem, K., and Raes, K. (2008). Fatty acid profile and oxidative stability of pork as influenced by duration and time of dietary linseed or fish oil supplementation. J. Anim. Sci. 86, 1418–1425. doi: 10.2527/jas.2007-0032
Holub, B. J. (2001). Docosahexaenoic acid in human health. ACS Symp. 788, 54–65. doi: 10.1021/bk-2001-0788.ch005
Hong, J., Lee, S., Yun, H., Moniruzzaman, M., Park, Y., Shahkar, E., et al. (2017). The optimum dietary docosahexaenoic acid level based on growth and non-specific immune responses in juvenile rock bream. Oplegnathus fasciatus. Aquacult. Res. 48, 3401–3412. doi: 10.1111/are.13167
Hossain, M. A., Almatar, S. M., and James, C. M. (2012). Effects of varying dietary docosahexaenoic acid levels on growth, proximate composition and tissue fatty acid profile of juvenile silver pomfrets, pampus argenteus (euphrasen, 1788). Aquacult. Res. 43, 1599–1610. doi: 10.1111/j.1365-2109.2011.02964.x
Howe, P. R. C., Downing, J. A., Grenyer, B. F. S., Grigonis-Deane, E. M., and Bryden, W. L. (2002). Tuna fishmeal as a source of dha for n?3 pufa enrichment of pork, chicken, and eggs. Lipids 37, 1067–1076. doi: 10.1007/s11745-002-1002-3
Huang, C., Gu, S., Yu, P., Yu, F., Feng, C., Gao, N., et al. (2010). Deficiency of smarcal1 causes cell cycle arrest and developmental abnormalities in zebrafish. Dev. Biol. 339, 0–100. doi: 10.1016/j.ydbio.2009.12.018
Ishikawa, K., Ishii, H., and Saito, T. (2006). DNA damage-dependent cell cycle checkpoints and genomic stability. DNA Cell Biol. 25, 406–411. doi: 10.1089/dna.2006.25.406
Levine, A. J. (1997). p53, the cellular gatekeeper for growth and division. Cell 87, 323–331. doi: 10.1016/S0092-8674(00)81871-1
Li, P., Kim, S. W., Li, X., Datta, S., Pond, W. G., and Wu, G. (2009). Dietary supplementation with cholesterol and docosahexaenoic acid affects concentrations of amino acids in tissues of young pigs. Amino Acids 37, 709–716. doi: 10.1007/s00726-008-0196-5
Livak, K. J., and Schmittgen, T. D. (2001). Analysis of relative gene expression data using realtime quantitative PCR and the 2-ΔΔCt method. Methods 25, 402–408. doi: 10.1006/meth.2001.1262
Livingstone, L. R., White, A., Sprouse, J., Livanos, E., Jacks, T., and Tlsty, T. D. (1992). Altered cell cycle arrest and gene amplification potential accompany loss of wild-type p53. Cell 70, 923–935. doi: 10.1016/0092-8674(92)90243-6
Lu, K. L., Wang, L. N., Zhang, D. D., Liu, W. B., and Xu, W. N. (2017). Berberine attenuates oxidative stress and hepatocytes apoptosis via protecting mitochondria in blunt snout bream megalobrama amblycephala fed high-fat diets. Fish Physiol. Biochem. 43, 65–76. doi: 10.1007/s10695-016-0268-5
Mai, W., Peng, L., and Wang, W. (2012). Characterization of the tilapia p53 gene and its role in chemical-induced apoptosis. Biotechnol. Lett. 34, 1797–1805. doi: 10.1007/s10529-012-0980-x
MedihaYildirim-Aksoy, C. L., Allendavis, D., Richard, S., and Klesius, P. (2007). Influence of dietary lipid sources on the growth performance, immune response and resistance of Nile tilapia, oreochromis niloticus, to streptococcus iniae challenge. J. Appl. Aquacult. 19, 29–49. doi: 10.1300/J028v19n02_02
Moran, C. A., Morlacchini, M., Keegan, J. D., Delles, R., and Fusconi, G. (2018). Effects of a dha-rich unextracted microalgae as a dietary supplement on performance, carcass traits and meat fatty acid profile in growing-finishing pigs. J. Anim. Physiol. Anim. Nutr. 102, 1026–1038. doi: 10.1111/jpn.12911
Moreno-Indias, A., Sánchez-Macías, A., Martínez-de la Puente, A., Morales-dela Nuez, N. Y., and Argüello, A. (2012). The effect of diet and dha addition on the sensory quality of goat kid meat. Meat Sci. 90:397. doi: 10.1016/j.meatsci.2011.08.004
Munoz-Fontela, C., Macip, S., Martinez-Sobrido, L., Brown, L., Ashour, J., Garcia-Sastre, A., et al. (2008). Transcriptional role of p53 in interferon-mediated antiviral immunity. J. Exp. Med. 205, 1929–1938. doi: 10.1084/jem.20080383
Ohki, R., Nemoto, J., Murasawa, H., Oda, E., Inazawa, J., Tanaka, N., et al. (2000). Reprimo, a new candidate mediator of the p53-mediated cell cycle arrest at the G2 phase. J. Biol. Chem. 275, 22627–22630. doi: 10.1074/jbc.c000235200
Plourde, M., and Cunnane, S. C. (2007). Extremely limited synthesis of long chain polyunsaturates in adults: implications for their dietary essentiality and use as supplements. Appl. Physiol. Nutr. Metab. 32, 619–634. doi: 10.1139/H07-034
Raes, K., DeSmet, S., and Demeyer, D. (2004). Effect of dietary fatty acids on incorporation of long chain polyunsaturated fatty acids and conjugated linoleic acid in lamb, beef and pork meat: a review. Anim. Feed Sci. Technol. 113, 199–221. doi: 10.1016/j.anifeedsci.2003.09.001
Sam, M. R., Esmaeillou, M., and Shokrgozar, M. A. (2016). Fish-oil-derived dha-mediated enhancement of apoptosis in acute lymphoblastic leukemia cells is associated with accumulation of p53, downregulation of survivin, and caspase-3 activation. Nutr. Cancer 69, 64–73. doi: 10.1080/01635581.2017.1247884
Saquib, Q., Attia, S. M., Siddiqui, M. A., Aboul-Soud, M. A., Al-Khedhairy, A. A., Giesy, J. P., et al. (2012). Phorate-induced oxidative stress, DNA damage and transcrip tional activation of p53 and caspase genes in male Wistar rats. Toxicol. Appl. Pharmacol. 259, 54–65. doi: 10.1016/j.taap.2011.12.006
Slotkin, T. A., and Seidler, F. J. (2012). Developmental neurotoxicity of organophosphates targets cell cycle and apoptosis: revealed by transcriptional profiles in vivo and in vitro. Neurotoxicol. Teratol. 34, 232–241. doi: 10.1016/j.ntt.2011.12.001
Song, E., and Kim, H. (2016). Docosahexaenoic acid induces oxidative dna damage and apoptosis, and enhances the chemosensitivity of cancer cells. Int. J. Mol. Sci. 17:1257. doi: 10.3390/ijms17081257
Takaoka, A., Hayakawa, S., Yanai, H., Stoiber, D., Negishi, H., Kikuchi, H., et al. (2003). Integration of interferon-α/β signalling to p53 responses in tumour suppression and antiviral defence. Nature 424, 516–523. doi: 10.1038/nature01850
Tappel, A. L. (1973). Lipid peroxidation damage to cell components. Clin. Pathol. 32, 1870–1874. doi: 10.1016/j.palaeo.2015.08.026
Villalta, M., Estévez, A., Bransden, M. P., and Bell, J. G. (2005). The effect of graded concentrations of dietary dha on growth, survival and tissue fatty acid profile of senegal sole (Solea senegalensis) larvae during the artemia feeding period. Aquaculture 249, 0–365. doi: 10.1016/j.aquaculture.2005.03.037
Wang, C. C., Jiang, G. Z., Cao, X. F., Dai, Y. J., Huang, Y. Y., Li, X. F., et al. (2019). Effects of dietary docosahexaenoic acid on growth performance, fatty acid profile and lipogenesis of blunt snout bream (Megalobrama amblycephala). Aquaclt. Nutr. 00, 0–14. doi: 10.1111/anu.13012
Yonish-Rouach, E., Resnftzky, D., Lotem, J., Sachs, L., Kimchi, A., and Oren, M. (1991). Wild-type p53 induces apoptosis of myeloid leukaemic cells that is inhibited by interleukin-6. Nature 352, 345–347. doi: 10.1038/352345a0
Zand, H., Rhimipour, A., Bakhshayesh, M., Shafiee, M., Mohammadi, I. N., and Salimi, S. (2007). Involvement of ppar-γ and p53 in dha-induced apoptosis in reh cells. Mol. Cell. Biochem. 304, 71–77. doi: 10.1007/s11010-007-9487-5
Zhang, D., Lu, K., Dong, Z., Jiang, G., Xu, W., and Liu, W. (2014). The effect of exposure to a high-fat diet on microRNA expression in the liver of blunt snout bream (Megalobrama amblycephala). PLoS One 9:e96132. doi: 10.1371/journal.pone.0096132
Keywords: docosahexaenoic acid, cell cycle arrest, apoptosis, P53, cycling family, Megalobrama amblycephala
Citation: Wang C, Liu W, Cao X, Huang Y, Wang X, Xiao K, Li X, Zhang D and Jiang G (2020) Excess DHA Induces Cell Cycle Arrest by Activating the P53/Cycling Pathway in Blunt Snout Bream (Megalobrama amblycephala). Front. Mar. Sci. 7:286. doi: 10.3389/fmars.2020.00286
Received: 07 February 2020; Accepted: 07 April 2020;
Published: 15 May 2020.
Edited by:
Kang-le Lu, Jimei University, ChinaReviewed by:
Shengming Sun, Shanghai Ocean University, ChinaChengdong Liu, Ocean University of China, China
Jian Gao, Huazhong Agricultural University, China
Copyright © 2020 Wang, Liu, Cao, Huang, Wang, Xiao, Li, Zhang and Jiang. This is an open-access article distributed under the terms of the Creative Commons Attribution License (CC BY). The use, distribution or reproduction in other forums is permitted, provided the original author(s) and the copyright owner(s) are credited and that the original publication in this journal is cited, in accordance with accepted academic practice. No use, distribution or reproduction is permitted which does not comply with these terms.
*Correspondence: Guang-zhen Jiang, amlhbmdnekBuamF1LmVkdS5jbg==