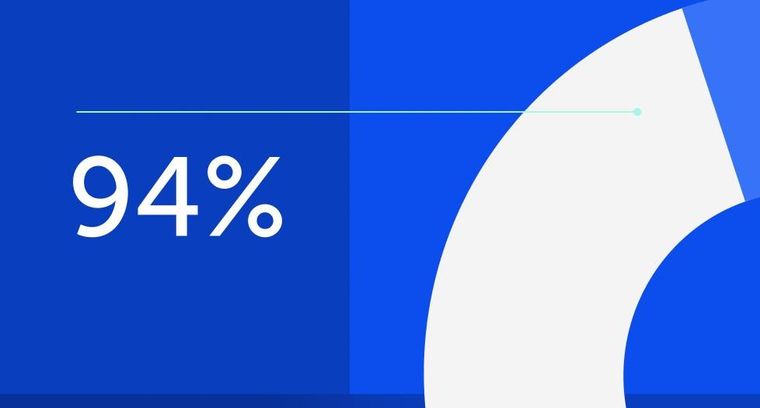
94% of researchers rate our articles as excellent or good
Learn more about the work of our research integrity team to safeguard the quality of each article we publish.
Find out more
ORIGINAL RESEARCH article
Front. Mar. Sci., 28 April 2020
Sec. Global Change and the Future Ocean
Volume 7 - 2020 | https://doi.org/10.3389/fmars.2020.00246
An array of anthropogenic pressures is affecting tropical ecosystems, posing major conservation challenges for scientists, stakeholders and populations. Illegal cyanide fishing is one of the major threats to Indo-Pacific coral reefs, targeting a multitude of colorful species for the marine aquarium trade as well as large-sized groupers and wrasses for the food fish trade. Ultimately, the continued use of this destructive practice as oceans warm may overload tropical ecosystems and result in irreversible ecological damage. Here we show that the impact of cyanide poisoning in an ornamental tropical marine fish is magnified under increased temperatures. A sole pulse exposure of 60 s to 50 mg L–1 of cyanide under current temperature (26°C) caused substantial mortality (50–100%) in eight species of Pomacentridae. The clownfish Amphiprion ocellaris was the most resistant, especially medium-sized fish [average total length and weight of 38 mm and 1.12 g; LC50 (95% CI) = 50.00 (46.76 − 53.24) mg L–1] that showed shorter recovery times and higher survival rates (%) when compared to small-sized ones [average total length and weight of 25 mm and 0.30 g; LC50 (95% CI) = 28.45 (20.17 − 36.72) mg L–1]. However, when the most resistant size-class was concomitantly exposed to a sub-lethal dosage of cyanide (25 mg L–1 instead of 50 mg L–1) and ocean warming scenarios for 2100 (+3°C and heat wave +6°C), survival rates (%) decreased to 60 and 20%, respectively, and recovery times increased in the worst case scenario. Mortality outbreaks, as well as vulnerability to predation, will likely expand in fish inhabiting coral reefs exposed to cyanide fishing unless stronger conservation measures are taken in tropical reefs to limit this destructive practice now and in the oceans of tomorrow.
Cyanide fishing, along with climate change, habitat destruction and pollution, is one of the major threats to Indo-Pacific coral reefs. This highly destructive and illegal fishing practice has been impacting coral reefs in this region since the 1970’s and mainly targets live reef fish to supply premium markets (Burke et al., 2011). While some species illegally collected using cyanide attain top values for human consumption in Asian restaurants (e.g., groupers and napoleon wrasse), others (e.g., clownfish, surgeonfish, and angelfish) supply the multi-million dollar industry of the marine aquarium trade, namely in the EU and the United States (Barber and Pratt, 1998; Dee et al., 2014; Leal et al., 2016). Fish from the family Pomacentridae are highly targeted by fishers and dominate the trade (Green, 2003). Fishers spray ornamental reef species using squirt bottles containing concentrated cyanide solutions (1,400 to 120,000 mg L–1) that often lead to coral and fish mortality (Jones and Steven, 1997; Pet and Djohani, 1999; Cervino et al., 2003). Accurate exposure concentrations are hard to calculate due to the different methods employed, dilution effect in seawater, variability between fishers, and lack of reports on this illegal practice. Laboratory assays to test the effect of cyanide on marine life have used concentrations ranging from a few micrograms to hundreds or thousands of milligrams per liter (Rubec, 1986; Jones and Steven, 1997; Cervino et al., 2003). To date, all efforts to effectively ban cyanide fishing from Indo-Pacific coral reefs have been unsuccessful (National Intelligence Council, 2016).
Cyanide (CN–) strongly affects the performance of marine organisms and is known to cause cytotoxic anoxia via the inhibition of the enzyme cytochrome c oxidase, preventing mitochondria from using oxygen in the electron transport chain and leading to metabolic acidosis due to lactate accumulation (Coppock and Dziwenka, 2015). Tropical reef fish exposed to cyanide show respiratory distress and organ damage (e.g., liver pycnosis and brain necrosis) while reducing swimming capacity, growth, reproductive output and survival (Rubec, 1986; Hanawa et al., 1998). By being easily observed, the effects of toxicants on fish swimming capacity, along with qualitative and quantitative measures of swimming behavior, are commonly monitored in ecotoxicological studies to evaluate sublethal toxicity in fish (Little and Finger, 1990; Ramzy, 2014; Calfee et al., 2016). The combination of such measures with mortality levels provides an overview of the effects of cyanide poisoning on tropical fish. Moreover, inter and intraspecific differences in cyanide tolerance may be anticipated. For example, rates of contaminant uptake and elimination may be determined by species-specific and size-specific metabolic rates (Mason, 2002), leading to different results being recorded according to species and body size (or life-cycle stage). This size-dependent variability has already been documented in other fish exposed to cyanide (Smith et al., 1978; McCraken and Leduc, 1980; Isom, 1993), as well as to other pollutants (Anderson and Weber, 1975; Biswas and Kaviraj, 2002; Capkin et al., 2006), highlighting the importance of addressing size-specific effects of toxicants. Furthermore, coupling destructive fishing practices like cyanide fishing to additional anthropogenic pressures like climate change and pollution most likely exacerbates deleterious physiological effects that lead to population changes, as previously shown for corals (Zaneveld et al., 2016) and algae (Russell et al., 2009).
Climate change is especially relevant in this framework, as tropical sea surface temperature (SST) trends calculated for the past century already show a warming of 0.8–1.0°C in the Indo-Pacific region (Deser et al., 2010). Furthermore, temperature anomalies projected for 2100 range between +0.9 and +3.3°C, depending on RCP (Representative Concentration Pathway) scenarios provided by the Intergovernmental Panel on Climate Change (IPCC, 2013, 2019). Factors such as regional variability, seasonality (dry/wet season) and El Niño Southern Oscillation (ENSO) also contribute to the variation of current and future SST, rainfall patterns and convection in tropical regions. Moreover, ocean isotherms have been shifting significantly in the tropics and ocean areas in the Indo-Pacific between 29 and 30°C have had the largest increase rate (2.29 km × 106 km decade–1) indicating that the warmest ocean areas are expanding (Lin et al., 2011). Recently, the Indo-Pacific region has also experienced severe and extreme marine heatwaves with temperature anomalies of >5°C (Hobday et al., 2018), highlighting the increased thermal load on tropical areas. Such warming is predicted to strongly affect marine ectotherms, as environmental temperature determines metabolic rates and aerobic scope (Pörtner and Farrell, 2008), and defines how organisms respond to other stressors, namely pollutants. In general, the interactive effects of climatic stressors and contaminants can be described in two ways, namely (i) toxicant-induced climate susceptibility or (ii) climate-induced toxicant sensitivity (Hooper et al., 2013). Elevated temperature is predicted to intensify sensitivity to contaminants, as it may increase their uptake and/or elimination (potentially associated with shifting metabolic rates) and modify their bioavailability and toxicity (Sokolova and Lannig, 2008). Physiological models suggest that the impact of elevated temperature will be more severe in tropical organisms than in temperate ones (Tewksbury et al., 2008; Vinagre et al., 2016). Small thermal safety margins and limited acclimation capacity arising from living in a stable environment seem to be the main factors shaping the reported vulnerability of tropical organisms (Tewksbury et al., 2008; Vinagre et al., 2016). Aerobic scope adjustments to meet the higher energetic demands of a warming ocean will ultimately determine the performance of organisms and the persistence of marine populations in future oceans (Pörtner and Knust, 2007). Some tropical reef fish may have the capacity to acclimate over short- and long-term warming (Donelson et al., 2012; Madeira et al., 2016), but performance and fitness trade-offs (locomotion, growth, and reproductive output) are expected (Sunday et al., 2014; Sandersfeld et al., 2015). Despite the array of anthropogenic disturbances and the expected loss of coral reefs and reef associated species (Wilson et al., 2006), to date, the combined effects of cyanide fishing and ocean warming on reef fish, two of the major threats to tropical coral reef communities in the Indo-Pacific region, are still unknown.
The main aim of this study is therefore to evaluate the effects of cyanide exposure not only under present-day temperatures but also under ocean warming (+3°C anomaly predicted for 2100) and heatwave scenarios (+6°C). The first objective (Experiment 1) was to make an interspecific assessment of ornamental pomacentrid reef fish vulnerability to cyanide poisoning. Thus, eight of the most traded species within family Pomacentridae (Amphiprion clarkii, Amphiprion frenatus, Amphiprion ocellaris, Chromis margaritifer, Chromis viridis, Chrysiptera cyanea, Dascyllus melanurus, and Dascyllus trimaculatus) were subjected to a pulse exposure of cyanide (following Hanawa et al., 1998) under current temperature, simulating present-day cyanide poisoning conditions. As grazers and symbionts of sea anemones, these species are ecologically relevant in coral reef communities, exerting bottom-up control of community structure (Smith et al., 2010). Species-dependent resistance to cyanide poisoning was assessed by monitoring swimming behavior (maximum immobilization and recovery times), as well as mortality levels. Maximum times of immobilization and recovery were chosen as proxies for maximum physiological resistance of a species to cyanide poisoning. Afterward, the most resistant species was chosen as a model (the clownfish A. ocellaris) for further resistance testing under a multiple-stressor framework. By selecting the most resistance species, we aimed to address a worst-case scenario (if a significant effect can be detected in the most resistant species, it would be magnified in less resistant ones). Firstly, different sized fish were pulse exposed to different cyanide concentrations to test the hypothesis that body size could determine intraspecific differences in the tolerance to this toxicant (Experiment 2). Subsequently, the most tolerant size-class of A. ocellaris (again framing the study under a worst-case scenario as detailed above) was subjected to a pulse exposure of cyanide under ocean warming scenarios for 2100 (+3 and +6°C) simulating cyanide fishing in future oceans (Experiment 3). We hypothesize that cyanide poisoning and elevated temperature have a negative synergistic effect on the performance and survival of a flag-ship species, the clownfish A. ocellaris (popularly known as “Nemo”).
All experiments were carried out in accordance with the EU legislation for animal experimentation (Directive 2010/63/EU). The protocol was approved by Direção Geral de Alimentação e Veterinária, Portuguese Ministry of Agriculture, Rural Development and Fisheries. All fish were euthanized by cervical transection at the end of the experiments.
Fish were purchased from an international marine aquarium species wholesaler and a local producer (Opérculo Lda. and TMC Iberia, Portugal) assuring that specimens either originated from aquaculture or from geographic locations with no known historic use of cyanide fishing. Fish were stocked at 26°C in the supplier’s facilities, which is considered an average temperature in Indo-Pacific coral reefs. Size (total length, TL) and wet weight (WW) were recorded for all specimens upon arrival to the laboratory using a 0.01 mm precision graduated icthyometer and a 0.01 g precision scale. Fish were housed in either two 270-L glass tanks (1.20 m × 0.50 m × 0.45 m) (fish for experiment 1 and 3, in stocking densities of 39 fish.tank–1 and 30 fish.tank–1, respectively, for each experiment) or nine 90-L glass tanks (0.60 m × 0.60 m × 0.25 m) (fish for experiment 2, approximately 12–14 fish.tank–1) with an internal circulation pump (Turbelle nanostream-6025 Tunze, Germany; flow of 2500 L h–1) and connected to a 125 L sump (1.20 m × 0.35 m × 0.40 m). The sump was equipped with a biological filter (submerged bio-balls), a 50 μm mesh bag for mechanical filtration, a submergible heater (Eheim Jäger 300 W, Germany), a protein skimmer (Deltec, APF600, Germany) and a submerged pump (Eheim 1262, Germany; flow of 3,400 L h–1). Salinity was kept stable by using an automatic water level controller (Reef Set, Portugal) connected to a small water pump that replaced evaporated water with freshwater purified by reverse osmosis to keep salinity at 35 (salinity was checked daily using a hand refractometer). The fish maintenance system used seawater prepared by mixing reverse osmosis water with a synthetic salt mix (Tropic Marine Pro Reef, Wartenberg, Germany). All fish were fed four times a day until satiation with a commercial pelleted feed (Hikari Marine S). Water parameters were maintained within the following optimal ranges for the species: temperature 26.0 ± 0.5°C; no detectable ammonium, nitrite and nitrate; pH 8.0 ± 0.2. Photoperiod was 12 h light: 12 h dark. Nitrogenous compounds were monitored every week using colorimetric tests (Salifert), while pH was monitored using a Pinpoint pH meter (PH 370, American Marine). For further details on life support systems see Rocha (Rocha et al., 2015).
After 1 week of housing, 9–10 fishes from eight different species, namely A. clarkii, A. frenatus, A. ocellaris, C. margaritifer, C. viridis, C. cyanea, D. melanurus, and D. trimaculatus (for details see Table 1) were randomly divided into groups per species for cyanide pulse exposure using a hand net. The duration of the pulse exposure (60 s) was selected according to Hanawa et al. (1998). A preliminary trial revealed that fish handling with a hand-net causes no mortality (Vaz et al., 2012).
All groups were exposed to a solution of 50 mg L–1 of CN– at 26°C (following previous studies namely Hanawa et al., 1998), prepared from a stock solution of 2.060 g L–1 of CN–. The stock solution was prepared by dissolving 2.001 ± 0.001 g of NaCN (97% purity; Sigma-Aldrich, St. Louis, MO, United States) in 500 mL of ultra-pure water, obtained from a Milli-Q Millipore system (Milli-Q plus 185). The pulse exposure was divided in four steps: (1) exposure bath, (2) first cleaning bath, (3) second cleaning bath and (4) third cleaning bath. In the first step, all fishes from one species were collected with hand-nets (maximum of 5 fish net–1, frame 300 mm × 200 mm × 300 mm mesh bag depth) and dipped for 60 s into a 15-L tank filled with synthetic seawater dosed with 50 mg L–1 of CN–. After the pulse exposure all fish from the same species were dipped for 60 s into a 20-L tank filled with synthetic seawater with no cyanide (first cleaning bath). This procedure was repeated two more times (second and third cleaning bath). Overall, this pulse exposure procedure lasted approximately 240 s with 1 s of air exposure between baths. During the exposure procedure, immobilization time was recorded and defined as the time at which the last fish of a species group stopped swimming, lost equilibrium and rested motionless in the bottom of the mesh used for the exposure bath, still showing opercular movements. Following the cleaning baths, all fish were randomly distributed into aerated 1-L glass jars (1 fish jar–1) filled with 1 L of synthetic seawater for the 96 h toxicity trial, which followed the parameters (i) the trial was carried out in a semi-static system, where water was fully replaced every day, (ii) no food was provided to the fish, following OECD (Organization for the Economic Cooperation and Development) guideline 203 (OECD, 1992), (iii) the jars were placed inside a water bath keeping water temperature at 26.0 ± 0.5°C, (iv) photoperiod was 12 h light: 12 h dark provided by full spectra (10,000 K) T5 fluorescent lamps, (v) temperature, salinity, dissolved oxygen, pH, alkalinity, total ammonia–nitrogen, nitrite and nitrate of each jar were measured daily with a YSI 85 Model (Yellow Springs Instruments, Yellow Springs, OH, United States), a pH 100 meter (Yellow Springs Instruments, Yellow Springs, OH, United States) and colorimetric tests, respectively. Temperature was maintained at 26.0 ± 0.1°C, salinity at 35.0 ± 0.1, pH 8.0 ± 0.1, dissolved oxygen concentration at 6.2 mg L–1, nitrite, nitrate and ammonium were not detectable. Following transfer to the glass jars, the behavior of all fish was closely monitored for the first 30 min to record recovery time of normal swimming activity (recovery time was defined as the time at which the last fish of a species group reacquired its normal swimming activity, defined here as swimming in the up-right position, showing self-righting behavior). Survival was evaluated daily. See Figure 1 for details. To test if time to immobilization and survival rate were correlated, a Spearman rank order correlation was carried out in Statistica (StatSoft, v10) (p < 0.05 for significance).
Figure 1. Experimental design. (A) Experiment 1: vulnerability of damselfish to cyanide poisoning. (B) Experiment 2: effects of cyanide on different sized clownfish, Amphiprion ocellaris. (C) Experiment 3: synergistic effect of ocean warming and cyanide poisoning on the physiology of the clownfish, Amphiprion ocellaris. Fish sizes and weights in experiment 1 were (1) Amphiprion ocellaris (n = 10; total length TL ≈ 40.00 ± 0.02 mm, weight 1.57 ± 0.30 g), (2) A. clarkii (N = 9; TL ≈ 35.00 ± 0.01 mm; weight 0.90 ± 0.20 g), (3) A. frenatus (N = 9; TL ≈ 35.00 ± 0.02 mm; weight 1.11 ± 0.15 g), (4) Chrysiptera cyanea (n = 10; TL ≈ 45.00 ± 0.01 mm; weight 1.57 ± 0.17 g), (5) Chromis viridis (n = 10; TL ≈ 30.00 ± 0.01 mm; weight 0.50 ± 0.05 g), (6) C. margaritifer (n = 10; TL ≈ 45.00 ± 0.02 mm; weight 1.58 ± 0.30 g), (7) Dascyllus melanurus (n = 10; TL ≈ 39.00 ± 0.036 mm; weight 1.54 ± 0.34 g), (8) D. trimaculatus (n = 10; TL ≈ 41.70 ± 0.03 mm; weight 2.17 ± 0.40 g).
Fish were acclimated for 2 months prior to experiments (in the conditions described above – see section “Fish Maintenance”). Following the acclimation period, small sized (n = 60, TL 25.00 ± 0.03 mm; weight 0.30 ± 0.09 g) and medium sized (n = 60, TL 38.00 ± 0.02 mm; weight 1.12 ± 0.21 g) A. ocellaris were subjected to cyanide poisoning. The 60 small specimens of A. ocellaris were randomly divided into 6 groups of 10 fish each for cyanide (CN–) pulse exposure at six different concentrations (0, 6.25, 12.5, 25, 50, and 100 mg L–1). The pulse exposure to CN– was divided into four steps as previously described (1) exposure bath, (2) first cleaning bath, (3) second cleaning bath, and (4) third cleaning bath. In the first step, all fish from the control treatment (0 mg L–1) were collected with a hand-net and dipped for 60 s into a 5-L tank filled with synthetic seawater with no cyanide. Afterward, all fish were dipped for 60 s into a 20-L tank filled with synthetic seawater with no cyanide (first cleaning bath). This procedure was repeated two more times (second and third cleaning bath). This protocol was carried out for all fish of the other treatments, increasing gradually the CN– concentrations (6.25; 12.5; 25; 50; and 100 mg L–1). During the exposure procedure, immobilization time was recorded. Following the control test and pulse exposure, all fish were randomly distributed into 1-L glass jars (1 fish per jar) filled with 1 L of synthetic seawater for the 96-h toxicity trial (carried out as described in section “Experiment 1”). The recovery time of normal swimming activity was determined during the first 30 min and mortality was evaluated daily (Figure 1).
To validate the test for cyanide toxicity the following conditions were completely fulfilled: (i) fish were fed 24 h before the beginning of the test; (ii) at the end of the test, the mortality recorded in the control treatment (0 mg L–1 of CN–) did not exceed 10% (or one fish if less than ten were used); (iii) constant conditions of semi-static procedure were maintained; (iv) a 12 h of photoperiod was used; (v) no food was administrated to the fish during the tests; and (vi) a minimum of at least 7 fish was employed per treatment. Lethal concentrations (96-h LC50) and their respective confidence intervals (95%) were calculated using probit analysis tool from Minitab V14 software (Minitab, LLC).
Sixty A. ocellaris (TL ≈36.00 ± 0.05 mm; weight 0.94 ± 0.38 g) were housed for 2 weeks prior to experiments (in the conditions described above – see section “Fish Maintenance”). Following, all fish were divided into three different groups and a thermal ramp (1°C day–1) was applied to reach the following temperatures: control 26°C (stocking temperature at the local supplier facilities, which is within the thermal range that these species experience in the wild) and experimental temperatures of 29 and 32°C. No fish mortality or shifts in water quality parameters were recorded during the thermal ramp performed. The chosen thermal ramp allowed fish to acclimate and prevented sudden heat-shock, falling within values regularly used in other studies (1–2°C day–1) (e.g., Slesinger et al., 2019). The fish were housed at these temperatures (90-L tanks, n = 20 fish tank–1, 1 tank temperature–1) for 1 week, provided by 300-W submergible heater (Eheim Jäger 300 W, Germany). Daily, 50% of the water volume was changed until the beginning of the experiment.
Afterward, fish at each temperature were randomly divided into groups of 10 fish for cyanide (CN–) pulse exposure: (T1) control (no CN– was added) 26°C; (T2) exposed to a 25 mg L–1 of CN– at control temperature 26°C; (T3) exposed to 29°C with no CN–; (T4) exposed to a concentration of 25 mg L–1 of CN– and 29°C; (T5) exposed to 32°C with no CN–; and (T6) exposed to a concentration of 25 mg L–1 of CN– and 32°C. The pulse exposure to CN– was carried out in four steps as previously described (1) exposure bath, (2) first cleaning bath, (3) second cleaning bath and (4) third cleaning bath. In the first step, all fish from the treatments T1, T3, and T5 were collected with a hand-net and dipped for 60 s into a 15-L tank (stocking density of 1 fish per 1.5 L) filled with synthetic seawater with no cyanide (with the respective temperature). Then, all fishes of treatments T2, T4, and T6 were collected with a hand-net and dipped for 60 s into a 15-L tank filled with synthetic seawater dosed with 25 mg L–1 of CN–. After the pulse exposure to cyanide all fish from the same group were dipped for 60 s into a 20-L tank filled with synthetic seawater (with the respective temperature) with no cyanide (first cleaning bath). This procedure was repeated two more times for all fish from all groups (second and third cleaning bath). For treatments with no cyanide, the same procedure of cleaning baths was used. During the exposure procedure, immobilization time was recorded. Following the pulse exposure, all fish were randomly distributed into 1-L glass jars (1 fish per jar) filled with 1 L of synthetic seawater (prepared as described above) and placed in the corresponding water bath (26 ± 0.1, 29 ± 0.1, and 32 ± 0.1°C). Then, recovery time of normal swimming activity was determined. The 96-h toxicity trial was carried out as described in previous sections. The jars were placed inside a water bath keeping water temperatures stable at 26 ± 0.1, 29 ± 0.1, and 32 ± 0.1°C. Mortality was evaluated daily (Figure 1). The conditions to validate this toxicity trial were the same as described in Section “Experiment 2.”
Survival of the different Pomacentridae species enduring a pulse exposure of 50 mg L–1 of CN– under current ocean temperature (26°C) varied between 0 and 50%, with only two out of the eight species tested surviving (50% survival for A. ocellaris and 20% for C. cyanea, Figure 2A). During exposure, fish showed severe gasping followed by loss of equilibrium and respiratory arrest. Species ranking according to decreasing time of immobilization was D. trimaculatus 40 s, C. cyanea 37 s, D. melanurus 32 s, A. ocellaris 28 s, A. frenatus 23 s, Chromis viridis 20 s, and both A. clarkii and C. margaritifer 12 s (Figure 2B). Regarding the two surviving species, immobilization time was shorter for A. ocellaris than C. cyanea, whereas recovery time was longer for A. ocellaris than C. cyanea. Moreover, no relationship was detected between time to immobilization and survival (Spearman rank order correlation, n = 8, r = 0.34, p > 0.05). Raw data is available in Supplementary Table S1.
Figure 2. Effects of cyanide pulse exposure (60 s; 50 mg L– 1 of CN–) on the performance of eight species of family Pomacentridae (n = 9 or 10 individuals per species), the most traded fish family in the marine aquarium industry. (A) Survival (%) 96 h post-exposure; and (B) immobilization (s) and recovery time (min) recorded for each species. Immobilization time is the time at which the last fish of a species stopped swimming and remained motionless while recovery time is the time at which the last fish of a species returned to normal swimming activity. Fish illustrations by DM.
Smaller fish displayed a lower survival than medium-sized fish, especially at concentrations above 6.25 mg L–1, and lower immobilization times (on average, smaller fish immobilized 7 s earlier than medium fish, Figures 3A,B). No mortality was detected in control groups. Moreover, smaller fish took longer to recover from exposure to cyanide when compared to medium fish (+6 min, Figure 3C). The lethal concentration of cyanide (LC50 after 96 h) calculated for smaller fish (average TL 25 mm and weight 0.30 g) was nearly half of that calculated for medium-sized fish (Table 2). Neither size class was able to survive an exposure to a cyanide concentration of 100 mg L–1. In general, for both size classes, immobilization time decreased with increasing concentration of cyanide while recovery time increased with increasing cyanide concentration. Raw data is available in Supplementary Table S2.
Figure 3. Effects of cyanide (CN–) pulse exposure (60 s) on different sized (small 25.00 ± 0.03 mm total length and 0.30 ± 0.09 g weight; medium 38.00 ± 0.02 mm total length and 1.12 ± 0.21 g weight) clownfish Amphiprion ocellaris (A) survival (%) 96-h post-exposure; (B) immobilization time (s); (C) recovery time (min). Ten small and medium-sized fish were used per treatment. Immobilization time is the time at which the last fish of a treatment stopped swimming and remained motionless while recovery time is the time at which the last fish of a treatment returned to normal swimming activity.
Table 2. Lethal concentration for 50% of fish population (LC50, mg L–1) when exposed for 96 h to cyanide (CN–) and respective 95% confidence limits and Pearson goodness-of-fit in small (25.00 ± 0.03 mm total length and 0.30 ± 0.09 g weight) and medium sized (38.00 ± 0.02 mm total length and 1.12 ± 0.21 g weight) Amphiprion ocellaris.
Cyanide exposure at increased temperatures led to higher mortality rates, when compared to the same exposure at control temperature. While there was no mortality in fish subjected to cyanide (25 mg L–1 for 60 s) at control temperature, in the +3 and +6°C above present-day scenarios, the pulse exposure to cyanide led to 40 and 80% mortality, respectively (Figure 4A). Warming alone induced A. ocellaris mortality only in the +6°C above present-day scenario (20% mortality); still, it was lower than when cyanide and warming were combined. Additionally, recovery time from cyanide poisoning was longer in the +3 and +6°C scenarios (3 and 10 min, respectively) when compared to control temperature (2 min, Figure 4B). Raw data is available in Supplementary Table S3.
Figure 4. Effects of cyanide pulse exposure (25 mg L– 1 for 60 s) and elevated temperature (+3 and +6°C above control) on tropical reef clownfish (Amphiprion ocellaris) (A) survival (%) 96-h post-exposure; and (B) immobilization time (s) and recovery time (min). Fish solely exposed to elevated temperature were not tested for these parameters. Ten fish were used per treatment. Immobilization time is the time at which the last fish of a treatment stopped swimming and remained motionless while recovery time is the time at which the last fish of a treatment returned to normal swimming activity.
To date, the marine aquarium trade is still supplied with wild specimens caught in tropical countries using destructive methods, leading to a persistent and unsustainable wildlife trade and coral reef degradation. Here we show that the impact of cyanide poisoning in fish mortality is magnified under elevated temperatures. A sole pulse exposure of cyanide (50 mg L–1 for 60 s) under current temperature caused increased mortality (96-h post-exposure) among fishes of the Pomacentridae family, with only two out of eight species surviving (A. ocellaris and C. cyanea). A previous study reported lower mortality for the same exposure conditions in Dascyllus aruanus, but sampled fish were heavier, with an average weight of 9 g (Hanawa et al., 1998). Mortality rates reported for fish caught with cyanide range between 5 and 75% within a few hours of collection and can reach up to 90% until being offered to hobbyists in retail stores (Rubec et al., 2001; Wabnitz et al., 2003). Physiological condition and species-specific metabolic rates (resting, routine, or maximum metabolic rates, Ikeda, 2016) may be determinant factors explaining interspecific variation in survival/mortality, as these determine uptake and elimination rates of contaminants (Mason, 2002). For instance, juvenile clownfish have a resting metabolic rate of approximately 1 mg O2 h–1 g WW–1, while their maximum metabolic rate can reach over 5 mg O2 h–1 g WW–1 (23–32°C) (Paschke et al., 2018; Velasco-Blanco et al., 2019). However, Chromis species can have resting metabolic rates in the order of 0.2–0.5 mg O2 h–1 g WW–1 and maximum metabolic rates between 1.5 and 2 mg O2 h–1 g WW–1 (Johansen and Jones, 2011; Nadler et al., 2016). Adult specimens of Dascyllus species have been reported to have resting metabolic rates of around 0.1 mg O2 h–1 g WW–1 and maximum metabolic rates of around 1.6–2 mg O2 h–1 g WW–1 (Johansen and Jones, 2011). However, other factors may come into play when analyzing interspecific differences in toxicant tolerance. While lower metabolic rates could promote cyanide tolerance (Doudoroff, 1976), protective mechanisms such as mucus production in clownfish (associated with protection from host anemone’s sting, Mebs, 2009) could reduce toxicant exposure and uptake, accounting for a higher resistance (see Reverter et al., 2018 for a review on fish mucus functions). However, among the three tested Amphiprion species, only A. ocellaris survived. Therefore, the hypothesis that mucus production may protect from this toxicant, needs further and detailed testing (e.g., amount of mucus produced; molecular, microbiome and physicochemical profiling of mucus in the different species).
According to studies in fish, cyanide uptake occurs mainly through the gills, skin and intestinal tract (Eisler, 1991) and the main proposed detoxification pathways include (i) the reaction of cyanide with thiosulfate in the presence of rhodanese to produce thiocyanate, subsequently excreted in urine (ii) conjugation with cystine to form 2-amino-2-thiazoline-4-carboxylic acid and (iii) reaction with hydroxocobalamin to form cyanocobalamin (Eisler, 1991). However, cyanide metabolism in marine fishes is still largely unknown. Thus, studies on cyanide toxicokinetics and excretion physiology in marine fish are urgently needed to clarify detoxification pathways and ascertain inter-specific differences in tolerance and prevalence of cyanide metabolites in marine fish. Ultimately, species specific regulation and activity of enzymes involved in detoxification pathways may explain differences in cyanide tolerance (Madeira and Calado, 2019). In this study, A. ocellaris showed the greatest survival (50%) but was rapidly immobilized and had a longer recovery time than the other surviving species C. cyanea. Thus, immobilization and recovery time seem unrelated to the actual ability of a A. ocellaris to survive a pulse exposure to cyanide. It should be noted, however, that species with longer recovery times (impairment of normal swimming activity for longer timeframes), such as A. ocellaris will likely be more vulnerable to predation when exposed to the poisoning effects of cyanide. Such an effect should be validated in future studies as the presence of predators could trigger other functions that could influence the recovery. Among surviving species, intraspecific variation may be crucial to determine the ecological success of populations in the face of change. Such variation may be attributable to genetic diversity, phenotypic variation, and developmental plasticity (Forsman and Wennersten, 2016). In this study, smaller fish were more vulnerable to cyanide exposure than medium-sized fish supporting the rationale that cyanide toxicity is size-dependent (Smith et al., 1978; McCraken and Leduc, 1980). Similarly, a relationship between body size and resistance to stress has been suggested in other studies using A. ocellaris as a model (Madeira et al., 2016). In general, rates of contaminant uptake have been shown to vary with body size in aquatic organisms including mollusks (Riget et al., 1996) and fish (Merciai et al., 2014). Size-specific respiration and metabolic rates (related to growth processes) may explain body size differences in tolerance as the resistance of fish to cyanide tends to increase with a reduction of metabolic rate (Doudoroff, 1976). This could explain why medium sized fish were more resistant to cyanide, as mass specific metabolic rates tend to decrease as fish grow larger. Interestingly, studies suggest that processes that affect oxygen uptake and use in reef fish larvae may impact development and growth as reef fish larvae have high mass specific metabolic rates (see Hess et al., 2015). Therefore, future studies should also address if cyanide tolerance varies along different life-stages, as cyanide strongly influences cellular respiration (Coppock and Dziwenka, 2015).
The dose-weight ratio in ecotoxicology is based on the assumption that the target sites of the toxicant are proportional to body weight (Anderson and Weber, 1975). Other factors such as surface area to volume ratio (of body and gills, which change throughout development and affect absorption), internal diffusion distances, ratio of brain to body weight (if the toxicant affects neural function, like cyanide, see Pandey, 2014), development of homeostatic and detoxification mechanisms and development of the immune system have been proposed to explain variation in age or size-dependent sensitivity to toxicants (Anderson and Weber, 1975; Mohammed, 2013), possibly also explaining interspecific differences. Nevertheless, this may not be a general trend, as other authors observed no size-dependent differences in tolerance to cyanide [see Doudoroff (1976) for a review]. The 96-h LC50 values in this study ranged between 28,450 and 50,000 μg L–1 of CN– depending on fish size, while for other marine fish the 96-h LC50 reported values ranged from 30 to 285,000 μg L–1 depending on cyanide form (iron-cyanide complexes being the least toxic) and life stage (Pablo et al., 1997; Dung et al., 2005).
The coupling of cyanide fishing with other anthropogenic-related pressures holds the potential to induce further stress in fish populations. Accordingly, cyanide poisoning caused higher mortality rates under ocean warming and heat wave scenarios, when compared to control temperature. Tropical reef fishes from Sulawesi (Indonesia), a well-known source of marine ornamental species, are reported to have Critical Thermal Maxima at temperatures ∼7°C above current average water temperatures in the Indo-Pacific (Eme and Bennett, 2009). Accordingly, acclimation capacity to temperatures between 26 and 30°C has been previously reported for A. ocellaris, even though a cellular stress response onsets at 30°C (Madeira et al., 2016). Beyond this temperature stress levels are too high, as supported by the 20% mortality at 32°C (+6°C simulation) and are clearly exacerbated by cyanide pulse exposure (leading to 80% mortality). Moreover, recovery time from cyanide poisoning was longer in both warming and heatwave scenarios when compared to control temperature leaving A. ocellaris more vulnerable to factors such as predation in the wild, as already documented for other fish (Eisler, 1991). Evidence thus suggests that sub-lethal dosages of cyanide become lethal in warming scenarios, being legitimate to assume that cyanide and warming have negative synergistic effects on fish. Such results may be explained by the stressors’ mode of action, as similar modes of action should result in either synergistic or antagonistic effects. Loss of performance under increasing temperatures is known to be caused by tissue hypoxemia due to a reduction in water dissolved O2, as well as the mismatch between oxygen supply and demand, defining the window of aerobic scope (Pörtner, 2010). Changes in mitochondrial density/efficiency and acclimation of oxygen transport capacity, as well as metabolic adjustments, have been proposed as crucial mechanisms in the thermal acclimation process (Pörtner, 2002). Reduced aerobic performance signs the onset of thermal stress in fish and is followed by the induction of cellular stress responses such as heat shock proteins and antioxidants (Pörtner, 2010; Madeira, 2016) in an attempt to promote survival in thermally challenging conditions. Such mechanisms likely explain the increased mortality levels observed in fish exposed to +6°C and fish concomitantly exposed to cyanide and warming. Moreover, temperature-dependent effects on metabolic rates and consequently contaminant uptake and elimination are also expected (Sokolova and Lannig, 2008). As temperature increases, so does metabolic rate and ventilation, increasing toxicant uptake. Additionally, sensitivity of fish to cyanide poisoning increases at low oxygen concentrations (Doudoroff, 1976), which are prevalent in warmer waters. The decreased O2 supply to tissues due to warming, coupled to decreased heart performance (Sawyer and Heath, 1988) and the inhibition of mitochondrial respiration rates by cyanide, leads to a narrowing of aerobic scope that decreases upper thermal limits and restricts the amount of aerobic ATP available to tissues. Exacerbated tissue hypoxemia under ocean warming and cyanide poisoning could thus increase anaerobic metabolism and cellular damage (e.g., protein denaturation). The need to activate cellular stress response mechanisms and detoxification pathways may also increase energy demand and depletion of energetic reserves under toxicant and elevated temperature exposure, resulting in increased metabolic costs for organisms (Sokolova and Lannig, 2008). Since fish cannot maintain long-term survival based on anaerobic energy production due to deleterious acidosis (Pörtner and Knust, 2007), mortality levels can be magnified. Such hypotheses should be further investigated by measuring oxygen consumption rates, mitochondrial aerobic capacity, and tissue lactate accumulation.
Besides the physiological effects of cyanide and reported population declines associated with destructive fishing in reef fish (Sadovy and Vincent, 2002), exposure of reef structuring organisms like corals to cyanide poisoning leads to outbreaks of bleaching and mortality (Cervino et al., 2003). Moreover, dissociation of the symbiotic relation with zooxanthellae, photosynthesis inhibition, lowered calcification rates via inhibition of carbonic anhydrase and long-term cellular impairments were reported in several coral species after exposure to cyanide (Cervino et al., 2003). Cyanide concentrations used by fisherman in squirt bottles can be as high as 1,400–120,000 mg L–1 (Pet and Djohani, 1999; Cervino et al., 2003), values that are much higher than those needed to induce coral and fish mortality (Cervino et al., 2003). As loss of coral reef habitat and consequent secondary loss of species and biodiversity proceeds (Don McAllister et al., 1999), fishers move to more pristine areas continuing habitat destruction (Cervino et al., 2003). These practices coupled to the targeting of spawning aggregations of reef fish for capture (Sadovy and Vincent, 2002) will undoubtedly lead to loss of reef communities and magnify environmental and societal impacts under global change scenarios, as southeast Asian populations mostly depend on fisheries for food and source of income (FAO, 2014). Despite growing evidence that coral reef fisheries cannot sustain commercial exports without cautious controls (Sadovy and Vincent, 2002), governmental agencies have failed to eradicate destructive fishing from the Indo-Pacific region (National Intelligence Council, 2016). Under this framework, we conclude that if CO2 emissions follow business-as-usual trends, destructive fishing practices such as the use of cyanide poisoning will become even deadlier and depict a major problem in tropical coral reef ecosystems. Efficient conservation measures and collaborative governance are of the upmost importance. The implementation of more sustainable fishing practices (e.g., provide training on the use and access to fine-mesh barrier nets), traceability protocols and certification (Cohen et al., 2013) is urgently needed to promote present-day and future sustainability of tropical fisheries in a changing ocean.
All datasets generated for this study are included in the article/Supplementary Material.
The animal study was reviewed and approved by Direção Geral de Alimentação e Veterinária, Portuguese Ministry of Agriculture, Rural Development and Fisheries.
RC and RR designed and planned the study. JA, VF, and RJR carried out the experiments. DM performed the statistical analyses. DM, ML, and RC interpreted the data. DM wrote the manuscript. All authors revised it critically and gave final approval of the final version submitted.
Thanks are due to FCT/MEC for the financial support to CESAM (UIDP/50017/2020 and UIDB/50017/2020), MARE (UID/MAR/04292/2019), and the individual grants (SFRH/BPD/117491/2016 to DM; SFRH/BPD/115298/2016 to ML; SFRH/BPD/99819/2014 to RJR; and PD/BD/52568/2014 to VF) provided through national funds and to the co-funding by the ERDF, within the PT2020 Partnership Agreement and Compete 2020.
The authors declare that the research was conducted in the absence of any commercial or financial relationships that could be construed as a potential conflict of interest.
The Supplementary Material for this article can be found online at: https://www.frontiersin.org/articles/10.3389/fmars.2020.00246/full#supplementary-material
Anderson, P. D., and Weber, L. J. (1975). Toxic response as a quantitative function of body Size. Toxicol. Appl. Pharmacol. 33, 471–483. doi: 10.1016/0041-008x(75)90073-3
Barber, C. V., and Pratt, V. R. (1998). Poison and profit: cyanide fishing in the Indo-Pacific. Environment 40, 28–34.
Biswas, B., and Kaviraj, A. (2002). Size dependent tolerance of Indian cat fish Heteropneustes fossilis (Bloch) to toxicity of cadmium and composted vegetation. Bull. Environ. Contam. Toxicol. 68, 37–42. doi: 10.1007/s00128-001-0216-6
Burke, L., Reytar, K., Spalding, M., and Perry, A. (2011). Reefs at Risk REVISited. Washington DC: World Resources Institute, doi: 10.1016/0022-0981(79)90136-9
Calfee, R. D., Puglis, H. J., Little, E. E., Brumbaugh, W. G., and Mebane, C. A. (2016). Quantifying fish swimming behavior in response to acute exposure of aqueous copper using computer assisted video and digital image analysis. J. Vis. Exp. 2016, 1–12. doi: 10.3791/53477
Capkin, E., Altinok, I., and Karahan, S. (2006). Water quality and fish size affect toxicity of endosulfan, an organochlorine pesticide, to rainbow trout. Chemosphere 64, 1793–1800. doi: 10.1016/j.chemosphere.2005.12.050
Cervino, J. M., Hayes, R. L., Honovich, M., Goreau, T. J., Jones, S., and Rubec, P. J. (2003). Changes in zooxanthellae density, morphology, and mitotic index in hermatypic corals and anemones exposed to cyanide. Mar. Pollut. Bull. 46, 573–586. doi: 10.1016/S0025-326X(03)00071-7
Cohen, F. P. A., Valenti, W. C., and Calado, R. (2013). Traceability issues in the trade of marine ornamental species. Rev. Fish. Sci. 21, 98–111. doi: 10.1080/10641262.2012.760522
Coppock, R. W., and Dziwenka, M. (2015). “Threats to wildlife by chemical and warfare agents,” in Handbook of Toxicology of Chemical Warfare Agents, ed. R. C. Gupta (Cambridge, MA: Academic Press), 809–814. doi: 10.1016/b978-0-12-800159-2.00055-5
Dee, L. E., Horii, S. S., and Thornhill, D. J. (2014). Conservation and management of ornamental coral reef wildlife: successes, shortcomings, and future directions. Biol. Conserv. 169, 225–237. doi: 10.1016/j.biocon.2013.11.025
Deser, C., Phillips, A. S., and Alexander, M. A. (2010). Twentieth century tropical sea surface temperature trends revisited. Geophys. Res. Lett. 37, 1–6. doi: 10.1029/2010GL043321
Don McAllister, E., Labbish Caho, N., and Shih, C. (1999). Cyanide fisheries: where did they start? SPC Live Reef Fish Info. Bull. 5, 18–21.
Donelson, J. M., Munday, P. L., McCormick, M. I., and Pitcher, C. R. (2012). Rapid transgenerational acclimation of a tropical reef fish to climate change. Nat. Clim. Chang. 2, 30–32. doi: 10.1038/nclimate1323
Doudoroff, P. (1976). Toxicity to fish of Cyanides and Related Compounds: A Review. Minnesota: US Government Publications Division.
Dung, L. Q., Cuong, N. M., Huyen, N. T., and Cu, N. D. (2005). Acute toxicity test to determine the effects of copper, zinc and cyanide on cobia (Rachycentron canadum) resources in North Vietnam. Australas. J. Ecotoxicol. 11, 163–166.
Eisler, R. (1991). Cyanide hazards to fish, wildlife, and invertebrates: a synoptic review- U.S. Fish Wildl. Serv. Biol. Rep. 85, 1–65. doi: 10.5962/bhl.title.11357
Eme, J., and Bennett, W. A. (2009). Critical thermal tolerance polygons of tropical marine fishes from Sulawesi. Indonesia J. Therm. Biol. 34, 220–225. doi: 10.1016/j.jtherbio.2009.02.005
Forsman, A., and Wennersten, L. (2016). Inter-individual variation promotes ecological success of populations and species: evidence from experimental and comparative studies. Ecography (Cop.) 39, 630–648. doi: 10.1111/ecog.01357
Green, E. (2003). “International trade in marine aquarium species: using the global marine aquarium database,” in Marine Ornamental Species: Collection, Culture & Conservation. Part II Progress and Current Trends in Marine Ornamentals A. Trade, Marketing, and Economics, eds J. Cato and C. Brown (Iowa, IA: Iowa State Press), 29–48. doi: 10.1002/9780470752722.ch3
Hanawa, M., Harris, L., Graham, M., Farrell, A. P., and Bendell-Young, L. I. (1998). Effects of cyanide exposure on Dascyllus aruanus, a tropical marine fish species: lethality, anaesthesia and physiological effects. Aquarium Sci. Conserv. 2, 21–34. doi: 10.1023/A:1009672626012
Hess, S., Wenger, A. S., Ainsworth, T. D., and Rummer, J. L. (2015). Exposure of clownfish larvae to suspended sediment levels found on the Great Barrier Reef: impacts on gill structure and microbiome. Sci. Rep. 5:10561. doi: 10.1038/srep10561
Hobday, A., Oliver, E., Sen Gupta, A., Benthuysen, J., Burrows, M., Donat, M., et al. (2018). Categorizing and naming marine heatwaves. Oceanography 31, 162–173. doi: 10.5670/oceanog.2018.205
Hooper, M. J., Ankley, G. T., Cristol, D. A., Maryoung, L. A., Noyes, P. D., and Pinkerton, K. E. (2013). Interactions between chemical and climate stressors: a role for mechanistic toxicology in assessing climate change risks. Environ. Toxicol. Chem. 32, 32–48. doi: 10.1002/etc.2043
Ikeda, T. (2016). Routine metabolic rates of pelagic marine fishes and cephalopods as a function of body mass, habitat temperature and habitat depth. J. Exp. Mar. Bio. Ecol. 480, 74–86. doi: 10.1016/j.jembe.2016.03.012
IPCC (2013). “Summary for Policymakers,” in Proceedings of the Climate Change 2013: The Physical Science Basis. Contribution of Working Group I to the Fifth Assessment Report of the Intergovernmental Panel on Climate Change, eds T. F. Stocker, D. Qin, G.-K. Plattner, M. Tignor, S. K. Allen, J. Doschung, et al. (Cambridge: Cambridge University Press), 33–36. doi: 10.1017/CBO9781107415324
IPCC (2019). Special Report: The Ocean and Cryosphere in a Changing Climate. in preparation. Available online at: https://www.ipcc.ch/report/srocc/ (accessed December 5, 2019).
Isom, G. E. (1993). “Cyanide,” in Handbook of Hazardous Materials, ed. M. Corn (San Diego, CA: Academic Press Inc), 161–172.
Johansen, J. L., and Jones, G. P. (2011). Increasing ocean temperature reduces the metabolic performance and swimming ability of coral reef damselfishes. Glob. Chang. Biol. 17, 2971–2979. doi: 10.1111/j.1365-2486.2011.02436.x
Jones, R. J., and Steven, A. L. (1997). Effects of cyanide on corals in relation to cyanide fishing on reefs. Mar. Freshw. Res. 48, 517–522. doi: 10.1071/MF97048
Leal, M. C., Vaz, M. C. M., Puga, J., Rocha, R. J. M., Brown, C., Rosa, R., et al. (2016). Marine ornamental fish imports in the European Union: an economic perspective. Fish Fish. 17, 459–468. doi: 10.1111/faf.12120
Lin, C. Y., Ho, C. R., Zheng, Q., Kuo, N. J., and Chang, P. (2011). Warm pool variability and heat flux change in the global oceans. Glob. Planet. Change 77, 26–33. doi: 10.1016/j.gloplacha.2011.02.006
Little, E. E., and Finger, S. E. (1990). Swimming behavior as an indicator of sublethal toxicity in fish. Environ. Toxicol. Chem. 9, 13–19. doi: 10.1002/etc.5620090103
Madeira, C., Madeira, D., Diniz, M. S., Cabral, H. N., and Vinagre, C. (2016). Thermal acclimation in clownfish: an integrated biomarker response and multi-tissue experimental approach. Ecol. Indic. 71, 280–292. doi: 10.1016/j.ecolind.2016.07.009
Madeira, D. (2016). Effects of Ocean Warming Throughout the Life Cycle of Sparus aurata?: A Physiological and Proteomic Approach. Ph.D. thesis, Universidade Nova de Lisboa, Lisbon.
Madeira, D., and Calado, R. (2019). Defining research priorities to detect live fi sh illegally collected using cyanide fi shing in Indo-Pacific coral reefs. Ecol. Indic. 103, 659–664. doi: 10.1016/j.ecolind.2019.03.054
Mason, C. (2002). “Aquatic Toxicology,” in Biology of Freshwater Pollution, ed. C. Mason (Upper Saddle River, NJ: Prentice Hall), 21–80.
McCraken, I., and Leduc, G. (1980). “Allometric growth response of exercised rainbow trout to cyanide poisoning,” in Aquatic Toxicology, ASTM STP 707, eds J. Eaton, P. Parrish, and A. Hendricks (West Conshohocken, PA: American Society for Testing and Materials), 303–320.
Mebs, D. (2009). Chemical biology of the mutualistic relationships of sea anemones with fish and crustaceans. Toxicon 54, 1071–1074. doi: 10.1016/j.toxicon.2009.02.027
Merciai, R., Guasch, H., Kumar, A., Sabater, S., and García-Berthou, E. (2014). Trace metal concentration and fish size: variation among fish species in a Mediterranean river. Ecotoxicol. Environ. Saf. 107, 154–161. doi: 10.1016/j.ecoenv.2014.05.006
Mohammed, A. (2013). “Why are early life stages of aquatic organisms more sensitive to toxicants than adults?,” in New Insights into Toxicity and Drug Testing, ed. S. Gowde (London: IntechOpen), doi: 10.5772/55187
Nadler, L. E., Killen, S. S., McClure, E. C., Munday, P. L., and McCormick, M. I. (2016). Shoaling reduces metabolic rate in a gregarious coral reef fish species. J. Exp. Biol. 219, 2802–2805. doi: 10.1242/jeb.139493
National Intelligence Council (2016). Global Implications of Illegal, Unreported, and Unregulated (IUU) Fishing. USA. Available online at: https://www.dni.gov/files/documents/Newsroom/Reports%20and%20Pubs/NIC_White_Paper_on_IUU_Fishing.pdf (accessed September 19, 2016).
OECD (1992). Test Guideline No. 203, Guideline for Testing of Chemicals. Fish, Acute Toxicity Test. Paris: OECD. (accessed July 17, 1992).
Pablo, F., Buckney, R. T., and Lim, R. P. (1997). Toxicity of cyanide and iron-cyanide complexes to Australian bass Macquaria novemaculeata and black bream Acanthopagrus butcheri. Australas. J. Ecotoxicol. 2, 75–84.
Paschke, K., Agüero, J., Gebauer, P., Díaz, F., Mascaró, M., López-Ripoll, E., et al. (2018). Comparison of aerobic scope for metabolic activity in aquatic ectotherms with temperature related metabolic stimulation: a novel approach for aerobic power budget. Front. Physiol. 9:1438. doi: 10.3389/fphys.2018.01438
Pet, J. S., and Djohani, R. H. (1999). Combatting destructive fishing practices in Komondo national park: ban the hookah compressor. Pesisir Lautan 2, 21–34.
Pörtner, H. O. (2002). Climate variations and the physiological basis of temperature dependent biogeography: systemic to molecular hierarchy of thermal tolerance in animals. Comp. Biochem. Physiol. A Mol. Integr. Physiol. 132, 739–761. doi: 10.1016/S1095-6433(02)00045-4
Pörtner, H. O. (2010). Oxygen- and capacity-limitation of thermal tolerance: a matrix for integrating climate-related stressor effects in marine ecosystems. J. Exp. Biol. 213, 881–893. doi: 10.1242/jeb.037523
Pörtner, H. O., and Farrell, A. (2008). Physiology and climate change. Science (80) 322, 690–692. doi: 10.1126/science.1163156
Pörtner, H. O., and Knust, R. (2007). Climate change affects marine fishes through the oxygen limitation of thermal tolerance. Science (80) 315, 95–97. doi: 10.1126/science.1135471
Ramzy, E. M. (2014). Toxicity and stability of sodium cyanide in fresh water fish Nile tilapia. Water Sci. 28, 42–50. doi: 10.1016/j.wsj.2014.09.002
Reverter, M., Tapissier-Bontemps, N., Lecchini, D., Banaigs, B., and Sasal, P. (2018). Biological and ecological roles of external fish mucus: a review. Fishes 3:41. doi: 10.3390/fishes3040041
Riget, F., Johansen, P., and Asmund, G. (1996). Influence of length on element concentrations in blue mussels (Mytilus edulis). Mar. Pollut. Bull. 32, 745–751. doi: 10.1016/0025-326X(96)00067-7
Rocha, R. J. M., Bontas, B., Cartaxana, P., Leal, M. C., Ferreira, J. M., Rosa, R., et al. (2015). Development of a standardized modular system for experimental coral culture. J. World Aquac. Soc. 46, 235–251. doi: 10.1111/jwas.12186
Rubec, P. J. (1986). “The effects of sodium cyanide on coral reefs and marine fish in the Philippines,” in The 1st Asian Fisheries Forum. Asian Fisheries Society, eds J. L. Maclean, L. B. Dizon, and L. V. Hosillos (Manila: Asian Fisheries society), 297–302.
Rubec, P. J., Cruz, F., Pratt, V., Oellers, R., McCullough, B., and Lallo, F. (2001). Cyanide-free net-caught fish for the marine aquarium trade. Aquarium Sci. Conserv. 3, 37–51. doi: 10.1023/A:1011370106291
Russell, B. D., Thompson, J. A. I., Falkenberg, L. J., and Connell, S. D. (2009). Synergistic effects of climate change and local stressors: CO2 and nutrient-driven change in subtidal rocky habitats. Glob. Chang. Biol. 15, 2153–2162. doi: 10.1111/j.1365-2486.2009.01886.x
Sadovy, Y., and Vincent, A. (2002). “Ecological issues and the trades in live reef fishes,” in Coral Reef Fishes: Dynamics and Diversity in a Complex Ecosystem, ed. P. Sale (San Diego, CA: Academic Press), 391–420. doi: 10.1016/b978-012615185-5/50023-2
Sandersfeld, T., Davison, W., Lamare, M. D., Knust, R., and Richter, C. (2015). Elevated temperature causes metabolic trade-offs at the whole-organism level in the Antarctic fish Trematomus bernacchii. J. Exp. Biol. 218, 2373–2381. doi: 10.1242/jeb.122804
Sawyer, P. L., and Heath, A. G. (1988). Cardiac, ventilatory and metabolic responses of two ecologically dissimilar species of fish to waterborne cyanide. Fish Physiol. Biochem. 4, 203–219. doi: 10.1007/BF01871746
Slesinger, E., Andres, A., Young, R., Seibel, B., Saba, V., Phelan, B., et al. (2019). The effect of ocean warming on black sea bass (Centropristis striata) aerobic scope and hypoxia tolerance. PLoS One 14:e0218390. doi: 10.1371/journal.pone.0218390
Smith, J. E., Hunter, C. L., and Smith, C. M. (2010). The effects of top-down versus bottom-up control on benthic coral reef community structure. Oecologia 163, 497–507. doi: 10.1007/s00442-009-1546-z
Smith, L. J., Broderius, S., Oseid, D., Kimball, G., and Koenst, W. (1978). Acute toxicity of hydrogen cyanide to freshwater fishes. Arch. Env. Contam. Toxicol. 7, 325–337. doi: 10.1007/bf02332060
Sokolova, I. M. I. M., and Lannig, G. (2008). Interactive effects of metal pollution and temperature on metabolism in aquatic ectotherms: implications of global climate change. Clim. Res. 37, 181–201. doi: 10.3354/cr00764
Sunday, J. M., Calosi, P., Dupont, S., Munday, P. L., Stillman, J. H., and Reusch, T. B. H. (2014). Evolution in an acidifying ocean. Trends Ecol. Evol. 29, 117–125. doi: 10.1016/j.tree.2013.11.001
Tewksbury, J. J., Huey, R. B., and Deutsch, C. A. (2008). ECOLOGY: putting the heat on tropical animals. Science (80) 320, 1296–1297. doi: 10.1126/science.1159328
Vaz, M. C. M., Rocha-Santos, T. A. P., Rocha, R. J. M., Lopes, I., Pereira, R., Duarte, A. C., et al. (2012). Excreted thiocyanate detects live reef fishes illegally collected using cyanide-A non-invasive and non-destructive testing approach. PLoS One 7:e35355. doi: 10.1371/journal.pone.0035355
Velasco-Blanco, G., Re, A. D., Díaz, F., Ibarra-Castro, L., de la Parra, M. I. A., Rodríguez-Ibarra, L. E., et al. (2019). Thermal preference, tolerance, and thermal aerobic scope in clownfish Amphiprion ocellaris (Cuvier, 1830) predict its aquaculture potential across tropical regions. Int. Aquat. Res 11, 187–197. doi: 10.1007/s40071-019-0228-7
Vinagre, C., Leal, I., Mendonça, V., Madeira, D., Narciso, L., Diniz, M. S., et al. (2016). Vulnerability to climate warming and acclimation capacity of tropical and temperate coastal organisms. Ecol. Indic. 62, 317–327. doi: 10.1016/j.ecolind.2015.11.010
Wabnitz, C., Taylor, M., Green, E., and Razak, T. (2003). From Ocean to Aquarium: The Global Trade in Marine Ornamental Species. Cambridge: UNEP-WCMC.
Wilson, S. K., Graham, N. A. J., Pratchett, M. S., Jones, G. P., and Polunin, N. V. C. (2006). Multiple disturbances and the global degradation of coral reefs: are reef fishes at risk or resilient? Glob. Chang. Biol. 12, 2220–2234. doi: 10.1111/j.1365-2486.2006.01252.x
Keywords: destructive fishing, climate change, Pomacentridae, marine aquarium trade, tropical ecosystems
Citation: Madeira D, Andrade J, Leal MC, Ferreira V, Rocha RJM, Rosa R and Calado R (2020) Synergistic Effects of Ocean Warming and Cyanide Poisoning in an Ornamental Tropical Reef Fish. Front. Mar. Sci. 7:246. doi: 10.3389/fmars.2020.00246
Received: 17 January 2020; Accepted: 30 March 2020;
Published: 28 April 2020.
Edited by:
Christopher Edward Cornwall, Victoria University of Wellington, New ZealandReviewed by:
Bridie J. M. Allan, University of Otago, New ZealandCopyright © 2020 Madeira, Andrade, Leal, Ferreira, Rocha, Rosa and Calado. This is an open-access article distributed under the terms of the Creative Commons Attribution License (CC BY). The use, distribution or reproduction in other forums is permitted, provided the original author(s) and the copyright owner(s) are credited and that the original publication in this journal is cited, in accordance with accepted academic practice. No use, distribution or reproduction is permitted which does not comply with these terms.
*Correspondence: Diana Madeira, ZC5tYWRlaXJhQHVhLnB0; ZGlhbmFibWFyQGdtYWlsLmNvbQ==; Ricardo Calado, cmpjYWxhZG9AdWEucHQ=
Disclaimer: All claims expressed in this article are solely those of the authors and do not necessarily represent those of their affiliated organizations, or those of the publisher, the editors and the reviewers. Any product that may be evaluated in this article or claim that may be made by its manufacturer is not guaranteed or endorsed by the publisher.
Research integrity at Frontiers
Learn more about the work of our research integrity team to safeguard the quality of each article we publish.