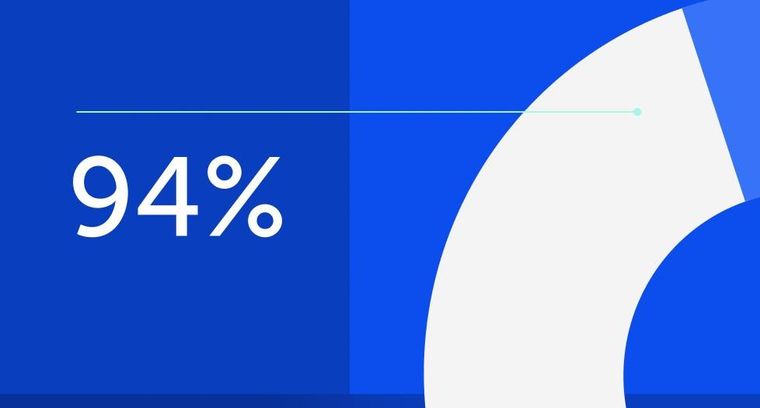
94% of researchers rate our articles as excellent or good
Learn more about the work of our research integrity team to safeguard the quality of each article we publish.
Find out more
REVIEW article
Front. Mar. Sci., 21 April 2020
Sec. Deep-Sea Environments and Ecology
Volume 7 - 2020 | https://doi.org/10.3389/fmars.2020.00239
This article is part of the Research TopicManaging Deep-sea Ecosystems at Ocean Basin Scale, Volume 1View all 29 articles
Circulation patterns in the North Atlantic Ocean have changed and re-organized multiple times over millions of years, influencing the biodiversity, distribution, and connectivity patterns of deep-sea species and ecosystems. In this study, we review the effects of the water mass properties (temperature, salinity, food supply, carbonate chemistry, and oxygen) on deep-sea benthic megafauna (from species to community level) and discussed in future scenarios of climate change. We focus on the key oceanic controls on deep-sea megafauna biodiversity and biogeography patterns. We place particular attention on cold-water corals and sponges, as these are ecosystem-engineering organisms that constitute vulnerable marine ecosystems (VME) with high associated biodiversity. Besides documenting the current state of the knowledge on this topic, a future scenario for water mass properties in the deep North Atlantic basin was predicted. The pace and severity of climate change in the deep-sea will vary across regions. However, predicted water mass properties showed that all regions in the North Atlantic will be exposed to multiple stressors by 2100, experiencing at least one critical change in water temperature (+2°C), organic carbon fluxes (reduced up to 50%), ocean acidification (pH reduced up to 0.3), aragonite saturation horizon (shoaling above 1000 m) and/or reduction in dissolved oxygen (>5%). The northernmost regions of the North Atlantic will suffer the greatest impacts. Warmer and more acidic oceans will drastically reduce the suitable habitat for ecosystem-engineers, with severe consequences such as declines in population densities, even compromising their long-term survival, loss of biodiversity and reduced biogeographic distribution that might compromise connectivity at large scales. These effects can be aggravated by reductions in carbon fluxes, particularly in areas where food availability is already limited. Declines in benthic biomass and biodiversity will diminish ecosystem services such as habitat provision, nutrient cycling, etc. This study shows that the deep-sea VME affected by contemporary anthropogenic impacts and with the ongoing climate change impacts are unlikely to withstand additional pressures from more intrusive human activities. This study serves also as a warning to protect these ecosystems through regulations and by tempering the ongoing socio-political drivers for increasing exploitation of marine resources.
Deep-sea ecosystems (i.e., >200 m water depth; Levin et al., 2019) have been traditionally considered vast habitats characterized by a stable and homogeneous environment (i.e., darkness and constant low temperatures). Such conditions were thought to indicate a lack of barriers for dispersal, resulting in large biogeographic areas with broad distributions of deep-sea species (Smith et al., 2006; McClain and Hardy, 2010). In the last few decades, new technologies have enabled sampling of some remote ecosystems, revealing new descriptions of species, and ecological paradigms (Danovaro et al., 2014; Cunha et al., 2017). These include the discovery of various geomorphological features and the diversity of their associated ecosystems, such as those in submarine canyons (Fernandez-Arcaya et al., 2017), seamounts (Morato et al., 2013), hydrothermal vents (Ramirez-Llodra et al., 2007b), cold-water coral reefs (Roberts et al., 2006) or sponge grounds (Hogg et al., 2010). Biodiversity has been also proved to be enhanced by numerous factors, including high habitat complexity (Buhl-Mortensen et al., 2010; Henry et al., 2010; Beazley et al., 2013), carbon cycling (Duineveld et al., 2012; White et al., 2012; Cathalot et al., 2015; Rix et al., 2016; Soetaert et al., 2016), recently discovered ecological interactions (Buhl-Mortensen and Mortensen, 2004; Carreiro-Silva et al., 2017; Henry and Roberts, 2017), or chemosynthetic production in the deep-sea (Ramirez-Llodra et al., 2007a; Rodrigues et al., 2013; Levin et al., 2016). Thus, the old view of low diversity, food-poor, and low metabolic activity has been reconsidered in many deep-sea areas (Ramirez-Llodra et al., 2010; Danovaro et al., 2014; McIntyre et al., 2016; Cunha et al., 2017).
Besides seafloor topography and sedimentary features (Levin and Sibuet, 2012; Tong et al., 2012; Collart et al., 2018), many other factors can drive biodiversity and biogeography in the deep-sea (Henry et al., 2014; Radice et al., 2016). Most of them are inherent to, or related to, the water mass characteristics, including temperature (Yasuhara and Danovaro, 2014), flow regime (Mienis et al., 2007; van Haren et al., 2014), oxygen concentration (Levin, 2003; Woulds and Cowie, 2007), organic matter supply (White et al., 2012; Cathalot et al., 2015), and circulation patterns (Henry et al., 2014; Somoza et al., 2014) among others. In the North Atlantic Ocean, the Atlantic Meridional Overturning Circulation (AMOC) re-distributes warm, saline water masses of the upper water column northwards, compensated by a returning flow of cooler fresher waters at depth. In addition, the properties and spatial extent of water masses in the North Atlantic vary on inter-annual to decadal timescales creating a fluctuating environment at local, regional and basin scales. To preserve deep-sea biodiversity and ensure sustainable socio-economic development in the North Atlantic Ocean, ecosystem-based management is needed on the same spatial and temporal scales as the key ocean circulation features and atmospheric drivers (Johnson et al., 2018; Armstrong et al., 2019). Re-organizations in the North Atlantic water mass structure have been occurring since the Atlantic’s genesis 200 mya, influencing the biodiversity, distribution and connectivity of deep-sea communities and species. For instance, the re-organizations in water mass structure related to AMOC and Sub-Polar Gyre dynamics during the early Holocene triggered an unprecedented post-glacial re-expansion of the cold-water coral (CWC) Lophelia pertusa over 7,500 km in ∼400 years (Frank et al., 2011; Henry et al., 2014; Wienberg and Witschack, 2017; Hebbeln et al., 2019). Thus, changes in the North Atlantic deep-water circulation are of particular interest in order to understand deep-sea species distribution, community composition, ecosystem functioning and dynamics. Moreover, this can shed light on future climate changes and how they affect deep-sea organisms.
This review does not intend to provide an exhaustive summary of all previous studies on oceanographic factors influencing deep-sea megafauna, but instead focuses on studies that clearly discuss the implications of water mass properties on biodiversity and biogeography. Thus, we address the importance of the North Atlantic circulation, from 20° to 70° N, and the role of the key water masses and their properties to derive a basin-scale synoptic view of key ocean controls on the deep-sea biodiversity and biogeography patterns. We particularly focus on sessile megafaunal species, such as CWCs and sponges, compiling results obtained from experimental work, field observations and modeling on these organisms from physiological to community level. These organisms form Vulnerable Marine Ecosystems (VME), which occur from the continental shelves to the bathyal regions of the deep-sea, connected in many cases across the entire North Atlantic through the basin-scale circulation. The vulnerability of these ecosystems is defined as the likelihood to experience substantial alteration from short-term or chronic disturbance, and the likelihood to recover in a given time frame (FAO, 2009). This, in turn, is related to the ecosystem characteristics such as rarity, fragility, structural and functional significance, longevity, slow growth rates or similar traits that limit the probability of recovery (FAO, 2009). Cold-water corals and sponges present many of these life-history traits and are also ecosystem-engineering organisms (Jones et al., 1994) which are able to form complex three-dimensional habitats such as CWC reefs, coral gardens or sponge grounds that promote life and enhance biodiversity in the deep-sea.
The North Atlantic circulation patterns, as well as the physical and chemical properties of the deep ocean, are already experiencing changes due to climate change (Purkey and Johnson, 2010; Helm et al., 2011; Levin and Le Bris, 2015; Perez et al., 2018). A clear understanding of the patterns and drivers of deep-sea benthic biodiversity is needed to inform and develop future projections under climate change conditions. In this sense, different projections for future water mass properties in the deep North Atlantic basin have been simulated and are presented in this work. The possible consequences for deep-sea benthic communities in these future scenarios are discussed considering the effects of the current and future water mass properties. This review aims to facilitate the integration of current knowledge on biodiversity related to water masses properties as well as the likelihood of future changes and consequences on the ecological and evolutionary trajectories of deep-sea ecosystems. Such information is needed to identify the assessment, management and regulation actions to support proper management and preservation of the deep-sea VMEs.
The North Atlantic is globally important due to the conversion of upper waters to denser intermediate and deep waters. In this review, we mainly considered and discussed the circulation patterns and water masses in the North Atlantic ranging from 20° to 70° N latitude and 78° W to 10° E longitude, but occasionally address some aspects of the tropical and more northern regions when necessary. The AMOC consists of a northward flowing upper limb balanced by a southward flow at depth. The warm and salty waters transported northwards within the Gulf Stream, both recirculate within the subtropical gyre and flow, via the North Atlantic Current (NAC), into more northern areas (Figure 1). Around 40% of the water entering subpolar latitudes continues northward over the Greenland-Scotland Ridge into the Nordic Seas (Sarafanov et al., 2012) where it is subject to further heat loss and mixing. The resulting, denser intermediate and bottom waters are trapped to the north of the Greenland-Scotland Ridge; however, some overflows into the North Atlantic (Hansen and Østerhus, 2000). The remaining 60% of the water transported northwards in the NAC recirculates cyclonically within the subpolar gyre (Sarafanov et al., 2012). As the waters circulate, they become cooler and fresher, and are subject to winter storms, particularly in the Labrador and Irminger Seas (Yashayaev, 2007; Jong and Steur, 2016). The resulting intermediate water mass flows southward, along with the overflow waters, in the Deep Western Boundary Current forming the return limb of the AMOC (Figure 1). Recent work suggests that the AMOC is dominated by dynamics to the east of Greenland, rather than convection in the Labrador Sea (Lozier et al., 2019).
Figure 1. Schematic of the main circulation in the North Atlantic Ocean color coded by water mass type. Main currents are labeled as: the North Atlantic Current (NAC), Azores Current (AC), Irminger Current (IC), Labrador Current (LC), and Deep Western Boundary Current (DWBC).
AMOC strength has varied through time. During the last glacial maximum, 19–21 kya, a moderate to strong AMOC probably persisted in the intermediate layers (Lynch-Stieglitz et al., 2007; Lippold et al., 2009; Ritz et al., 2013). This was followed by a slowdown of the AMOC between 12 and 19 kya, coinciding with the abrupt Younger Dryas event (Hughen et al., 1998). Fossil records indicate that the currently wide-spread CWC Lophelia pertusa remained in a few refugia during the last glacial maximum and Younger Dryas (Henry et al., 2014). The rapid spin-up of the AMOC after the Younger Dryas (Rogerson et al., 2006; Xie et al., 2012) coincided with a rapid and wide-spread return of L. pertusa to northern Europe after a hiatus of over 65 kyr (Frank et al., 2011). Today, the AMOC is still a crucial part of the Earth’s climate system. However, models indicate that it is likely to weaken by 2100 (Gregory et al., 2005), whilst recent studies suggest that the AMOC may have weakened in the past 100–200 years (Caesar et al., 2018; Thornalley et al., 2018).
As the properties and depth distribution of water masses varies spatially across the North Atlantic (Liu and Tanhua, 2019), we describe the key water masses in general terms in four depth bands: upper bathyal (200–1000 m), intermediate bathyal (1000–2000 m), lower bathyal (2000–3000 m) and abyssal (>3000 m). Mean properties and associated variability ranges for each depth band are shown in Table 1.
Table 1. Present-day (year 2000) and predicted future (year 2100) average values [range] of the main water mass properties, temperature (T), particulate organica carbon (POC), pH, aragonite and calcite saturation states (Ω) and dissolved oxygen (DO), influencing deep-sea biodiversity in the North Atlantic in the upper (200–1000 m), intermediate (1000–2000 m), lower (2000–3000 m) bathyal, and abyssal (>3000 m) regions.
In the upper bathyal depth range (200–1000 m), the predominant upper water mass is North Atlantic Central Water (NACW), which is found in the topmost 500–800 m. However, several denominations of this water mass exist. For example, “subtropical” NACW (found south of around 42°N) is warmer and more saline, whilst “subpolar” NACW found to the north is cooler and fresher (Ríos et al., 1992). NACW properties also vary zonally (Ríos et al., 1992; Pollard et al., 2004). In eastern areas, the NACW (termed eastern NACW, ENACW) is warmer and more saline, whilst western NACW (WNACW) is cooler and fresher. However, the freshest, coldest and densest water mass found in the upper 800 m is seen in the western subpolar basins and is termed SubArctic Intermediate Water (SAIW; García-Ibáñez et al., 2015). This water mass is also found further east and south, but in these areas, it is found below the NACW layer at around 500 m (Pollard et al., 1996; Wade et al., 1997). The final key upper bathyal water mass is Antarctic Intermediate Water (AAIW) which is again found below the NACW layer. AAIW, which has been observed as far north as 60°N (Tsuchiya, 1989), has a slightly elevated salinity but is most easily identified by a mid-depth silicate maximum (Read, 2000).
At intermediate bathyal depths (1000–2000 m), two main water masses are observed: Mediterranean Outflow Water (MOW) and Labrador Sea Water (LSW). The MOW overflows the Straits of Gibraltar and spreads out at around 1200 m in the Atlantic (García-Ibáñez et al., 2015). It is characterized by a mid-depth salinity maximum with low dissolved oxygen (DO) concentrations and has been traced to at least 60° W and 52° N (Needler and Heath, 1975; Bower et al., 2002). The LSW is a denser water mass characterized by a broad salinity minimum and high DO concentrations (Read, 2000). It is centered upon approximately 1500 m, although its exact density varies inter-annually with changes in convection depth in the Labrador Sea (Yashayaev, 2007).
In the lower bathyal range (2000–3000 m), the main water masses are those that have overflowed the Greenland-Scotland Ridge. The main locations for dense overflow are south of the Faroes and between Greenland and Iceland (Hansen et al., 2016; Jochumsen et al., 2017). These overflow waters are cool, dense and well oxygenated, and together with the less dense LSW exit the subpolar gyre as North Atlantic Deep Water (NADW).
The densest water mass in the North Atlantic, found at abyssal depths (>3000 m), is Antarctic Bottom water (AABW). This water mass is easily identified by its high silicate concentration (McCartney, 1992). Whilst it is more prevalent in southern areas, a diluted form is also seen in the eastern subpolar basins (Van Aken and De Boer, 1995; New and Smythe-Wright, 2001).
Marine biodiversity at the global-scale, shows decreasing biodiversity with both increasing depth (Rex et al., 2006; Roberts et al., 2009b; Costello and Chaudhary, 2017) and increasing latitude (Rex et al., 1993; Lambshead et al., 2000; Gage, 2004). These patterns have been usually related to the decreasing organic carbon flux to the seafloor (Willig et al., 2003; Rex et al., 2005) and to decreasing water temperature at depth (Lambshead et al., 2000). These different trends in biodiversity and distribution are commonly driven by the physical, chemical, and biological properties of the water masses (e.g., Levin et al., 2001; Mohn et al., 2014; Yasuhara and Danovaro, 2014; Buhl-Mortensen et al., 2015; Eigaard et al., 2016). However, the strong collinearity among water mass properties and depth, make it very difficult to discern the roles of hydrostatic pressure and other depth-correlated parameters (Mortensen et al., 2001; Lundsten et al., 2010). For instance, L. pertusa reefs off Norway occur in waters as shallow as 50 m; with it being impossible to determine whether temperature (up to 10°C) or salinity (down to 32) limit the upper bathymetrical distribution (Mortensen et al., 2001). Therefore, assertions on the potential controls of deep-sea species distributions should be taken with caution (Huff et al., 2013). In addition, the relative importance of these factors varies between taxa and regions, but also with the spatial and temporal window of observation (Buhl-Mortensen et al., 2015; Radice et al., 2016). Temperature is usually one of the leading factors at the global scale and over longer time scales (Watling et al., 2013; Yasuhara and Danovaro, 2014; Buhl-Mortensen et al., 2015), whereas at medium spatial scales (10 s of km), the presence of large geomorphological features and biological structures is significant (Henry and Roberts, 2007; Buhl-Mortensen et al., 2010). Substrate typology and food supply appear more important at finer spatial scales (m to km; Vetter and Dayton, 1998; McClain and Barry, 2010; Collart et al., 2018) and over shorter time scales (Yasuhara and Danovaro, 2014).
In fact, observations of deep-sea biodiversity actually showed a wide variability of trends with depth or latitude, from increases (e.g., polychetes, bivalves, and foraminifera; Sanders, 1968; Allen and Sanders, 1996; Olabarria et al., 2005), decreases or irregular trends (e.g., gastropods Stuart and Rex, 2009), to no relationship with depth or latitude (e.g., asteroids, Price et al., 1999) depending on the taxa considered (Gage, 2004; Stuart and Rex, 2009; Costello and Chaudhary, 2017). These trends can also result from other factors, such as large-scale environmental heterogeneity imposed by topography, bottom currents, and strong variations in nutrient input (Willig et al., 2003; Kendall and Haedrich, 2006), or oxygen minimum zone impingement (Levin and Gage, 1998; Rogers, 2000).
This variability in controls of deep-sea species distribution illustrates the complexity of large-scale patterns in deep-sea biodiversity and the difficulty of interpreting them as the product of a single mechanism (Lambshead et al., 2000; Danovaro et al., 2009; Costello and Chaudhary, 2017). Chronic under-sampling of the deep-sea (Ramirez-Llodra et al., 2010) frustrate efforts to obtain a comprehensive broad scale snapshot of the spatial distribution of biodiversity and prevents the full characterization of species distributional ranges. This renders the extraction of biogeographic patterns difficult (Costello and Chaudhary, 2017), where species richness, isolation and endemicity are also likely to play important roles (McClain and Hardy, 2010; Moalic et al., 2012; Watling et al., 2013; Costello and Chaudhary, 2017). For instance, broad faunal distributions across ocean-basin scales have been described for some soft-bottom taxa inhabiting the vast abyssal plains (Smith et al., 2006). However, large scale distributions (Van Dover et al., 2002; McClain, 2007; Clark et al., 2010) and genetic homogeneity (McClain and Hardy, 2010; Teixeira et al., 2013) have also been observed for several endemic species from highly patchy and specialized environments, such as hydrothermal vents and seamounts. In addition, the published information on the effect of water mass properties in the deep-sea megabenthic communities heavily relies on the responses of the most studied organism, the reef-forming CWC L. pertusa. Due to the imbalance level of information available among the different taxa, caution should be exercise since the responses of L. pertusa might not be necessarily representative of other less- or unstudied taxa.
The relative spatio-temporal stability of water temperature below ∼1000 m depth, has historically led to this factor receiving less attention as a possible control of deep-sea biodiversity (Yasuhara and Danovaro, 2014). However, it is likely that stability makes deep-sea organisms highly sensitive to small temperature changes, as evidenced by significant temperature–diversity relationships detected in several studies (Danovaro et al., 2004; O’Hara and Tittensor, 2010; Yasuhara and Danovaro, 2014). Temperature might be the most important factor shaping recent large-scale biodiversity patterns (at least at the alpha diversity level) due to different physiological tolerances of deep-sea taxa (Tittensor et al., 2010; Yasuhara and Danovaro, 2014). Over a broad range of temperatures, the response of deep-sea diversity is unimodal with a peak at around 5–10°C (Yasuhara and Danovaro, 2014). Nevertheless, the responses to temperature at species level are widely variable and usually related to the life-history traits. For instance, the response might be size dependent as observed for the mussel Bathymodiolus azoricus. The thermal niche in this species is broader for intermediate sizes than for small or large mussels (Husson et al., 2017). Overall, small species with very short generation times from days to months, such as nematodes (Danovaro et al., 2004), react strongly and rapidly to temperature changes but also may adapt to such changes through generations. In contrast, large taxa with longer generation times of years, such as mollusks, corals or echinoderms (Rowe et al., 1992), might in general be more resilient to the effects of rapid changes, but then may be unable to adapt over short time scales (Yasuhara and Danovaro, 2014).
Thermal boundaries and optimal temperature seem to be mainly species-specific in deep-sea taxa. The distribution of the reef-forming CWCs L. pertusa and Madrepora oculata, 4–12°C (Freiwald, 2002; Davies and Guinotte, 2011; Naumann et al., 2014), match the temperature range of cold intermediate North Atlantic waters below the summer thermocline. These conditions are generally found in relatively shallow waters (50–1000 m) at high latitudes, and at great depths (up to 4000 m) at low latitudes (Roberts et al., 2006, 2009b). Some deep-sea octocorals, like Radicipes gracilis, Acanella arbuscula and Acanthogorgia armata, can be found at lower temperatures, 1.5–6.1°C (Buhl-Mortensen et al., 2015). However, thermal ranges also vary intra-specifically between populations inhabiting different geographic regions. For example, L. pertusa is commonly found between 12.5 and 14°C in the Mediterranean Sea (Tursi et al., 2004; Freiwald et al., 2009). Likewise, M. oculata can temporarily tolerate temperatures up to 20°C in the Indian Ocean (Keller and Os’kina, 2008).
Species-specific tolerance, together with the magnitude and time of exposure to increased temperatures, are determinant for the response of the deep-sea organisms as observed in their natural environment (Guihen et al., 2012; Mienis et al., 2014), as well as in experimental conditions (Dodds et al., 2007; Naumann et al., 2014). For instance, a natural increase of 4°C in 24 h (up to 12°C) was observed at Tisler reef (Norway) followed by a mass mortality event of the sponge Geodia baretti, although it did not affect the L. pertusa reef despite exceeding its typical physiological temperature limit (Guihen et al., 2012). However, a subsequent ex situ experiment exposing the same sponge to acute thermal conditions (up to 5°C above ambient temperature for 14 days) did not induce any mortality (Strand et al., 2017). These results suggest that other processes (e.g., low oxygen concentrations, elevated nutrients levels, reduced salinity and disease) in combination with the heat wave could be responsible for the mortality event in those sponges. The upper temperature limit for L. pertusa is around 15°C but, it can survive short term (24 h) temperature spikes up to 20°C as demonstrated in aquaria experiments (Brooke et al., 2013). Temperature changes have a strong effect on CWC metabolism, with a specific acclimation among taxa. Experimental research proved that L. pertusa has a greater capacity to change its respiratory metabolism and acclimatize to decreased temperature (from 12 to 6°C) than M. oculata (Naumann et al., 2014). The different thermal tolerances have been also used to explain the dominance of M. oculata in warmer Mediterranean waters and L. pertusa in colder regions of the NE Atlantic. However, L. pertusa also forms important reefs off SE USA and the Gulf of Mexico (Reed et al., 2006; Brooke and Schroeder, 2007; Mienis et al., 2012a; Georgian et al., 2014), highlighting also the importance of different geographic populations (Brooke and Schroeder, 2007; Georgian et al., 2014; Brooke et al., 2017). The scleractinian CWC Dendrophyllia cornigera also reduced respiration and calcification rates when temperature was experimentally lowered to 8°C (Gori et al., 2014). The physiological performance of this species increased when elevating temperatures from 12 to 17.5°C, while that temperature change negatively impacted other CWC species such as Desmophyllum dianthus (Naumann et al., 2013). Indeed, D. cornigera seems to be absent from most of the NE Atlantic, where temperatures range from 5 to 10°C (Purser et al., 2013; Dullo et al., 2008; Roberts et al., 2009a).
In addition, temperature might trigger more important effects on deep-sea biota in combination with other factors or stressors (synergies), such as ocean acidification, low food availability, or reduced oxygen (e.g., Lunden et al., 2014; Gori et al., 2016; Büscher et al., 2017).
Overall, salinity only varies up to one unit worldwide at deep depths, but some biogeographic areas of distinct salinity can be identified in the main basins (Watling et al., 2013). In the North Atlantic, the most saline waters are those carried in the upper column, the Gulf Stream, and in the MOW northward spreading into the eastern Atlantic (Figure 1). In contrast, salinity becomes more uniform in the deepest waters below 3500 m, with some influence of upper water layers down to 2000 m depth (Watling et al., 2013). Salinities up to 800 m depth in the northernmost areas of the NW Atlantic show much lower values due to the influence of ice cover (Watling et al., 2013). For instance, glacial meltwater has had a large impact on freshwater inputs to the NW Atlantic, where increasingly large seasonal fluxes have been observed (Belkin, 2004).
The occurrence of CWCs in the NE Atlantic has been correlated in some areas with water density, a parameter defined by salinity and temperature (Dullo et al., 2008; Davies et al., 2010). Living L. pertusa reefs were firstly delimited to a narrow water density envelope (potential density; σθ = 27.35–27.65 kg m–3), which further studies in other North Atlantic regions proved to be larger than initially suggested (σθ = 27.1–27.84 kg m–3; Davies et al., 2010; Huvenne et al., 2011; Kenchington et al., 2017). This highlights the importance of physical conditions for CWCs growth and distribution, and the role of intermediate water masses limiting these boundaries (Mortensen et al., 2001; Bryan and Metaxas, 2006). Another example is the presence of a peak in the number of deep-sea taxa in the depth range (800–1300 m) where MOW is often found (Schönfeld and Zahn, 2000), with variations in near-bottom MOW current strength linked to abundance variability (Zenk and Armi, 1990).
Salinity changes during larval stages of benthic organisms are likely to significantly affect their survival rates (Pechenik, 1999). Although this topic is scarcely investigated, experimental work conducted with L. pertusa demonstrated that larvae can survive at salinities as low as 25 for long periods of time (up to 10 months) and cope with changes in salinity of up to five units (Strömberg and Larsson, 2017). Vertical migration of Lophelia larvae were also observed in aquaria (Strömberg and Larsson, 2017), suggesting that in nature, Lophelia larvae may migrate to the surface, where they would likely be exposed to a wide range of salinities and water densities and may cross water mass boundaries (Strömberg and Larsson, 2017). In contrast, other experiments with shallow water invertebrates (e.g., mussels, starfish, and sea urchins) revealed that the presence of haloclines inhibit larvae vertical migration (Sameoto and Metaxas, 2008). Thus, this high plasticity to salinity gradients and the great longevity of L. pertusa larvae could facilitate the wide geographical dispersal range and distribution for this species. Whether this versatility is shared by other deep-sea species due to selective pressure for broad scale dispersal remains to be investigated and could shed light on the role of currents and water masses on connectivity patterns.
Food availability is one of the main drivers related to the occurrence, distribution abundance and diversity of deep-sea taxa (Tittensor et al., 2011; Pape et al., 2013; Pusceddu et al., 2016). The vertical flux of organic matter from surface waters to the sea floor (∼1–10 g C org⋅m–2⋅yr–1; Glover and Smith, 2003; Watling et al., 2013) is the principal food source for most of the deep-sea organisms below 200 m (Davies et al., 2009; Wagner et al., 2011). For instance, the carbon of CWCs skeletons is mainly derived from this surface organic carbon (Griffin and Druffel, 1989; Sherwood et al., 2005; Roark et al., 2009).
Food particles are also transported laterally by advective fluxes, facilitating the expansion in the distribution of deep-sea taxa to areas not located beneath regions with high primary production (Davies et al., 2009; Mienis et al., 2009; Hebbeln and Wienberg, 2016). Thus, the occurrence of deep-sea benthic suspension feeders, such as CWCs and sponges, is frequently associated with highly hydrodynamic environments (Mohn et al., 2014). These areas are characterized by strong current velocities, with ∼15 cm⋅s–1 on average (Duineveld et al., 2007; Davies et al., 2009; Mienis et al., 2012b; Khripounoff et al., 2014) but peaking up to 80 cm⋅s–1 (Davies et al., 2009), that enhance particle suspension (Mienis et al., 2007; Roberts et al., 2009a; White and Dorschel, 2010; Khripounoff et al., 2014; van Haren et al., 2014) and prevent burial by sediments (White et al., 2005; Roberts et al., 2006; Mienis et al., 2007). Cold-water corals in particular seem to prefer areas with periodically varying flow speeds (Ruggeberg et al., 2007; Davies et al., 2009; Mienis et al., 2012a; van Haren et al., 2014), which allow high food supply during the peak current period and high capture rates under low flow (Purser et al., 2010; Orejas et al., 2016). To maximize encounter rates with food particles, CWCs can adjust their colony morphologies to face into the prevailing bottom current (Mortensen and Buhl-Mortensen, 2004; Roberts et al., 2009a). Intensified hydrodynamics are also associated with unique current patterns, such as recirculation gyres, or topographically steered currents like water cascading in submarine canyons (Canals et al., 2006; Palanques et al., 2006; Mulder et al., 2012; Khripounoff et al., 2014), and collision of water masses against seamounts (Genin and Dower, 2007; White et al., 2007a; Lavelle and Mohn, 2010). These areas are usually hot-spots for CWCs and sponges (Thiem et al., 2006; Rowden et al., 2010; Huvenne et al., 2011; Wagner et al., 2011; Howell et al., 2016; Serrano et al., 2017a).
The boundaries between water masses can also help to redistribute and pump the suspended food particles to deeper ecosystems (White et al., 2005, 2007b). Internal waves can occur at the interface of two water masses with different densities as well as along the pycnocline (Dorschel et al., 2007; Mienis et al., 2007; Wienberg et al., 2009; Pomar et al., 2012). For example, coral mounds in the Campeche Bank (Gulf of Mexico) were formed only during interglacial periods when an interface between the AAIW and the Tropical Atlantic Central Water existed; since during glacial ones this boundary was replaced by a vertically homogeneous water column (Matos et al., 2017). Similarly, fossil records of coral mounds at the Porcupine Seabight (NE Atlantic) indicate that mound aggradation was active when the boundary between MOW and ENACW was at a similar depth level as the mounds (Raddatz et al., 2014). While current coral mounds are located in the permanent thermocline between these two water masses (De Mol et al., 2005; White and Dorschel, 2010). Occurrence of conspicuous concentrations of deep-sea sponge grounds (Rice et al., 1990; Klitgaard et al., 1997) and L. pertusa reefs (Frederiksen et al., 1992) have been found on the upper slope all around NW Europe, where the combination of a steep slope with the hydrographic conditions cause internal waves that accelerate local currents (Mienis et al., 2007).
Recent studies showed reciprocal feedback between the occurrence of suspension feeders and the enhancement of food supply in their inhabiting areas. It has been suggested that cold-water sponges transform dissolved organic matter to particulate forms, making it available to higher trophic levels (De Goeij et al., 2013; Cathalot et al., 2015; McIntyre et al., 2016; Rix et al., 2016). In addition, CWC framework and mounds areas have more suspended particles than the surrounding coral rubble or no-reefs areas (Guihen et al., 2013; Cathalot et al., 2015; Soetaert et al., 2016; Van Oevelen et al., 2018).
Carbonate chemistry influences marine organisms through its critical role in mediating physiological reactions and determining the availability of carbonate ions required for biocalcification (Pörtner and Farrell, 2008; Doney et al., 2012). Deep waters are characterized by low pH, resulting from microbial degradation of organic matter, and low concentrations of carbonate ions (Wenzhöfer et al., 2001; Perez et al., 2018). Therefore, deep-sea calcifying organisms, such as, corals, mollusks, echinoderms and bryozoans, may be particularly sensitive to changes in carbonate chemistry (Guinotte et al., 2006; Doney, 2009; Wicks and Roberts, 2012). Calcium carbonate minerals in the oceans occur mainly in the form of aragonite and calcite. Aragonite presents a more resistant structure for high energy environments and it is formed by scleractinian corals, some mollusks and bryozoans. In contrast, calcite is less prone to dissolution and forms the shells and skeletons of foraminifera, echinoderms, crustaceans, calcareous sponges and most gorgonian corals (Ries et al., 2009; Ries, 2010). However, both minerals dissolve at low carbonate ion concentrations. Thus, the distributions of deep-sea ecosystem-engineering organisms with carbonate skeletons, are largely restricted to waters above the carbonate saturation horizon (SH; Guinotte et al., 2006; Davies and Guinotte, 2011; Yesson et al., 2012), i.e., those oversaturated for calcium carbonate minerals. Other calcifying taxa, such as echinoderms, are found throughout the world’s oceans, but are far less abundant or even absent in undersaturated waters (Lebrato et al., 2010). The carbonate SH in the North Atlantic is very deep, >2000 m (Guinotte et al., 2006), but still constrains the distribution of multiple deep-sea calcifying organisms.
Ocean absorption of anthropogenic CO2 has already reduced natural pH values (∼0.12) since the preindustrial time (Hennige et al., 2014) and the calcium carbonate saturation state (Ω; Orr et al., 2005; Perez et al., 2018); affecting particularly the intermediate and deep waters masses (Sweetman et al., 2017). Therefore, multiple studies have already focused on the impacts of ocean acidification (OA) in deep-sea organisms in the last decade.
Experimental OA studies on corals, mollusks and echinoderms, have predominantly showed reduced growth/calcification in response to reduced pH in adults and juveniles (Wicks and Roberts, 2012; Gazeau et al., 2013; Wall et al., 2015). However, responses can be species-specific or even region specific for a given taxa (Ries et al., 2009; Gazeau et al., 2013; Maier et al., 2019). For instance, low pH values (∼7.9–7.7) caused the deformation of skeletons in the CWC L. pertusa (Hennige et al., 2015; Wall et al., 2015). Nevertheless, experimental studies using the CWCs L. pertusa and M. oculata from the NE Atlantic and the Mediterranean Sea showed that the two species were able to calcify and grow in acidic and undersaturated conditions (Form and Riebesell, 2012; Maier et al., 2013, 2019; Hennige et al., 2014; Büscher et al., 2017). Also, Norwegian populations of L. pertusa could maintain growth at low pH with elevating feeding rates (Georgian et al., 2016). In contrast, L. pertusa inhabiting low carbonate or undersaturated conditions in the Gulf of Mexico and California showed great reductions in calcification rates and increased skeletal dissolution (Lunden et al., 2014; Georgian et al., 2016; Kurman et al., 2017; Gómez et al., 2018). Thus, resilience to OA effects might be related to adaptation in CWC, either genetic or due to acclimation in early stages (Kurman et al., 2017). The capacity of CWCs to cope with OA has been related to their ability to increase internal pH at the site of calcification (McCulloch et al., 2012a; Raybaud et al., 2017). However, it remains unclear whether calcification can be sustained indefinitely, as this is an energy demanding process (20–30% of CWC energy budget; Cohen and Holcomb, 2009) and OA also affects the coral metabolism (Hennige et al., 2014) and, the loss of the tissue surrounding and connecting polyps (Gammon et al., 2018).
Another important reported consequence of OA is the dissolution of shells and skeletons exposed to corrosive low pH seawater (Rodolfo-Metalpa et al., 2011; Doney et al., 2012). The dissolution effect is more pronounced in dead carbonate skeletons which lack the organic protective tissue, as observed in different mollusk gastropods, CWCs (Tunnicliffe et al., 2009; Rodolfo-Metalpa et al., 2011) and tissue-free portions of skeletons of L. pertusa (Hennige et al., 2015; Wall et al., 2015). Therefore, one of the most important predicted consequences of OA is the dissolution and weakening of the reef framework that may result in their structural collapse (Hennige et al., 2015; Büscher et al., 2019).
The impact of OA on other ecosystem-engineering organisms, such as octocorals or sponges, is still poorly known. Experimental studies showed a reduction in calcification and growth in Corallium rubrum (Cerrano et al., 2013), suggesting that gorgonians may also be negatively affected by OA. However, a study from the Southern Ocean found that, despite some evidences of dissolution in gorgonians, many species thrive in undersaturated conditions to at least −30% without major impacts in skeletal structure or growth rates (Thresher et al., 2011). The long-term acclimation to these conditions and unlimiting food supply are thought to facilitate tolerance of low-carbonate environments (Thresher et al., 2011). Calcareous sponges are expected to suffer similar impacts than other calcifying organisms under acidified conditions. However, most sponges have siliceous spicules and therefore, are generally more tolerant of OA and might even benefit from future acidified conditions (Bell et al., 2018). The silica cycle in the deep ocean is still not well understood (Ragueneau et al., 2000) but the silica flux from surface to deep waters could be strongly affected by OA (Petrou et al., 2019). However, the dissolved silica from the spicule mats formed by the sponges might be enough to build their new skeletal material (Chu et al., 2011; Maldonado et al., 2017, 2019). The very few studies on the impacts of OA in calcareous sponges, mainly included shallow water species and short-term responses (Goodwin et al., 2014; Morrow et al., 2015; Bates and Bell, 2018; Bell et al., 2018). Despite the high tolerance to OA reported (Goodwin et al., 2014; Morrow et al., 2015), species- specific differences were found (Bell et al., 2018) resulting in negative impacts on some sponges such as, mortality, tissue degradation (Bates and Bell, 2018) or changes in the associated microbial community (Bell et al., 2018). Bioeroding sponges have been the major focus of the OA studies in this group since they are potentially becoming more efficient under acidified conditions, thereby, accelerating the weakening of CWC reef frameworks (Wisshak et al., 2012, 2014). In addition to its important role in biomineralization/dissolution processes, OA might also lead to mortality, compromised reproduction (Verkaik et al., 2017) and/or the development of early life stages of benthic organisms (Kurihara, 2008; Dupont and Thorndyke, 2009; Byrne, 2011). Although synergic effects of OA and other factors have been seldom studied for deep-sea taxa, the few published studies suggest a great susceptibility of CWCs species to OA in combination with warming and/or deoxygenation, with decreased metabolic and calcification rates, increased skeletal dissolution, and induced mortality (Lunden et al., 2014; Georgian et al., 2016; Gori et al., 2016).
Most of the global ocean has DO values near saturation, ranging from 2.5 ml⋅l–1 off SW Africa to 6 ml⋅l–1 in the NW Atlantic (Watling et al., 2013). The highest DO values are generally associated with the colder, deeper, and more recently formed waters since DO solubility decreases with increasing temperatures and is consumed during degradation of organic matter. For instance, a drastic drop in DO, from 6.85 to 5.40 ml⋅l–1was observed in the Galicia Bank at MOW depths between 800 and 1200 m, which is warmer than the surrounding ENACW and NADW (Wienberg et al., 2009; Serrano et al., 2017a). Indeed, the L. pertusa reefs in this area inhabit the MOW-ENACW boundary (Somoza et al., 2014; Serrano et al., 2017a, b). The majority of L. pertusa colonies found in the North Atlantic coincide with a range of relatively low to moderate DO values (∼1.5–5 ml⋅l–1), which occurs between 300 and 1600 m water depth (Davies et al., 2010; Somoza et al., 2014; Brooke et al., 2017).
Most organisms are not very sensitive to variations in DO until it drops below a certain threshold, when they suffer from a variety of stresses related to reduced metabolic rates, leading ultimately to death, if the low oxygen concentrations remain for too long (Keeling et al., 2010). Thresholds for hypoxia vary greatly between marine taxa (Vaquer-Sunyer and Duarte, 2008), but when concentrations are above 1 ml⋅l–1, effects on mega- and macrobenthic diversity are probably minor (Levin and Gage, 1998).
Hypoxic and anoxic conditions are common in all oceans (Kamykowski and Zentara, 1990), varying from permanent zones to gradients over geologic time (Reichart et al., 1998; Ramirez-Llodra et al., 2010), seasonally varying areas (Guihen et al., 2018) or episodic events (Rogers, 2000; Levin, 2018). These different temporal scales drive different adaptations and tolerance levels in benthic taxa. Extensive permanent hypoxic zones, where oxygen is limiting for living organisms (Levin et al., 2001; Stramma et al., 2010), occur at midwater regions (100–1200 m depth), being present in the Atlantic only in the south basin, off Namibia and Angola (Hebbeln et al., 2017; Hanz et al., 2019). These oxygen minimum zones (OMZs; 0.2–0.5 ml⋅l–1) are formed by microbial degradation of organic matter beneath highly productive waters (Levin et al., 2001). For the bathyal fauna in the Atlantic, hypoxia might have more influence on species richness, while organic matter regulates species distribution and community evenness (Levin and Gage, 1998). Thus, within OMZs the mega- and macrofauna exhibit low species richness and very high dominance, but dense aggregations at the edges, where high organic matter fluxes and still suitable DO levels may occur (Levin, 2003). While annelid species are prevalent in the OMZ cores (Rogers, 2000; Soltwedel, 2000; Levin et al., 2001; Ramirez-Llodra et al., 2010), taxa with calcified shells or exoskeletons, such as echinoderms, crustaceans and mollusks, tend to be absent (Rogers, 2000). Benthic organisms inhabiting OMZs maximize the oxygen uptake through morphological and physiological adaptations, such as: raised ventilation rates, increased respiratory surfaces, reduced diffusion distances, development of respiratory pigments, vertical migrations, or enzymatic adaptation to anaerobic metabolism (Levin, 2003). OMZs may also present a barrier, disrupting species distribution or isolating populations across the inhospitable waters (Rogers, 2000). Thus, oxygen exerts tremendous control on marine biodiversity mostly through thresholds effects on evolution, physiology, reproduction, behavior, and species interactions (Levin and Le Bris, 2015).
However, living colonies of calcified organisms such as the CWCs L. pertusa and M. oculata have been found in South Atlantic OMZs at DO levels of 0.6 and 1.1 ml⋅l–1, respectively (Hebbeln et al., 2017; Hanz et al., 2019), drastically reducing the previous limiting DO levels obtained by modeling and experimentation (2.37 ml⋅l–1; Dodds et al., 2007; Davies et al., 2008). The effect of hypoxia on the occurrence of L. pertusa when food supply is high enough is difficult to be determined (Levin and Gage, 1998; Freiwald, 2002; White et al., 2005; Roberts et al., 2006). Nevertheless, recent studies in these OMZs suggest that CWCs and the associated fauna may compensate unfavorable conditions induced by low DO and high temperatures with an enhanced food availability (Hanz et al., 2019). Experimental research revealed that L. pertusa maintains constant rates of oxygen consumption over a range of DO concentrations, and colonies are able to survive short-time periods of anoxia (1 h) and hypoxia (up to 96 h; Dodds et al., 2007). However, both oxygen consumption and survivorship are sensitive to temperatures (6.5–9°C; Dodds et al., 2007). Indeed, oxygen consumption of L. pertusa at 11°C was 50% higher than that recorded at 9°C (Dodds et al., 2007). Complete mortality of L. pertusa nubbins has been observed at DO levels of 1.5 ml⋅l–1 and 14°C (Lunden et al., 2014).
The biodiversity of VMEs and the biogeographical affinities in deep-sea benthic communities can vary over space and time due to regional and basin-scale changes in the oceanographic conditions. On one hand, this section discusses local scale effects through examples of how key water masses and oceanographic processes affect VMEs at particular locations of the North Atlantic. On the other hand, we also provide a larger scale overview of the distribution of the main VMEs at a basin-scale and the critical roles of water masses in the species distribution, connectivity, endemicity and biodiversity patterns. We mainly focused on ecosystem-engineering organisms such as CWC and sponges, but more information on VME records across the North Atlantic can be found in the open access databases of multiple international organizations related to scientific, management and conservation purposes (e.g., ICES, NOAA).
Cold-water corals have a global distribution (Tittensor et al., 2009; Yesson et al., 2012), being particularly well described throughout the North Atlantic in both eastern (e.g, Roberts et al., 2009a; Foubert et al., 2011; Buhl-Mortensen and Buhl-Mortensen, 2014; Serrano et al., 2017b) and western basins (e.g., Reed et al., 2006; Brooke and Schroeder, 2007; Buhl-Mortensen et al., 2015; Hourigan et al., 2017). Aggregations of CWCs have previously been described as either “reefs” or “coral gardens” (OSPAR, 2008). Coral gardens can consist of very different species and groups of corals inhabiting the seafloor in close spatial proximity (Rossi et al., 2017), and are very often found to be dominated by gorgonians, bamboo corals (Octocoral, Alcyonacea) and/or black corals (Hexacorallia, Antipatharia; ICES, 2007; OSPAR, 2008). Their flexible skeletons and the arborescent vertical development of many of these species give them the appearance of “animal forests” (Rossi et al., 2017). In contrast, Scleractinians (also known as stony corals) often occur at relative high densities or develop reefs with multigenerational growth that might produce sizable calcium carbonate structures over time (Rossi et al., 2017). There are few scleractinian CWCs that build reefs and L. pertusa, being one of the main reef-building organisms in the deep-sea (Figure 2), is the most ubiquitous and studied species in the North Atlantic. Overall, the distribution of CWCs have been mainly correlated with water temperature and highly hydrodynamic areas (e.g., Frederiksen et al., 1992; Davies et al., 2009; Buhl-Mortensen et al., 2015). In particular, L. pertusa reefs are present in waters with salinities higher than 34 and temperatures between 4 and 12°C, corresponding to depths as shallow as 100 m at high latitudes (Mortensen et al., 2001; Dullo et al., 2008; Buhl-Mortensen et al., 2015) but down to 1200 m in other continental margins (De Mol et al., 2002) and canyons (De Mol et al., 2011; Hourigan et al., 2017; van den Beld et al., 2017).
Figure 2. Images showing the diversity of Vulnerable Marine Ecosystems, including cold-water coral reefs, coral gardens and sponge grounds, at different locations across the North Atlantic Ocean. (A) Astrophorid sponge ground typical of the Davis Strait area (2500 m), credit: Department of Fisheries and Oceans, Canada. (B) Cold-water coral reef dominated by Madrepora oculata, with scarce colonies of Lophelia pertusa and black corals (Antipathes dichotoma) at Gazul mud volcano (380 m, northern Gulf of Cadiz), credit: Instituto Español de Oceanografía, INDEMARES project. (C) Lophelia pertusa reef from Logachev Mounds (600 m, Rockall Bank), credit: J.M. Roberts, University of Edinburgh (Changing Oceans Expedition 2012, RRS James Cook cruise 073). (D) Coral garden formed mainly by Narella versluysi and N. bellisima with some Hexactinellid sponges (1000 m) in the Formigas seamount (Azores), credit: MEDWAVES, ATLAS project. (E) Cup corals (Desmophyllum dianthus), Solenosmilia variabilis, bivalves (Acesta cryptadelphe), a brisingid seastar (Novodinia sp.), and an octopod in a consolidated mud wall (1300 m) at Norfolk Canyon, credit: Steve Ross. (F) Coral garden of white and red Paragorgia arborea in Baltimore Canyon (430 m), credit: Steve Ross.
The MOW seems to represent one of the main water masses driving the distribution of CWCs across the NE Atlantic (Dullo et al., 2008; Somoza et al., 2014), promoting connectivity by the natural export of Mediterranean species and populations (Henry et al., 2014; Arnaud-Haond et al., 2017; Boavida et al., 2019). Several studies noted the presence of deep-sea suspension feeding species previously known only from the Mediterranean (e.g., the sponges Geodia anceps, Coelosphaera cryosi, and Petrosia raphida) at NE Atlantic locations, such as the Gulf of Cadiz (Spain; Palomino et al., 2016; Sitjá et al., 2018). This area is dominated by aggregations of scleractinians and gardens of large gorgonians and black corals (e.g., M. oculata, Acanthogorgia spp., Antipathes dichotoma, Leiopathes glaberrima; Figure 2) with particular locations, such as Gazul mud volcano, representing a biodiversity hotspot with more than 400 associated species (Rueda et al., 2016; Ramalho et al., 2018; Sitjá et al., 2018). The current distribution of living L. pertusa and M. oculata in the canyons of the Bay of Biscay coincides with the lower limit of the boundary between the ENACW and the MOW (De Mol et al., 2011), as does the distribution of carbonate mounds and CWC reefs in the Porcupine Seabight (De Mol et al., 2002, 2005; White and Dorschel, 2010) and other contourite systems in the NE Atlantic (Van Rooij et al., 2007a, b; Hernández-Molina et al., 2009). Indeed, the intensification of currents and the increased flow of MOW into the NE Atlantic after the last glacial period, mentioned in the previous section, is thought to have allowed Mediterranean CWCs to recolonize the Porcupine Seabight (De Mol et al., 2005; Henry and Roberts, 2008; Henry et al., 2014). This hypothesis was also supported by genetic data for L. pertusa (Henry et al., 2014; Boavida et al., 2019). It may also hold true for M. oculata, although this species shows a more complex pattern of genetic differentiation. This suggests multiple refugia at the origin of the post-glacial recolonization of the NE Atlantic, as well as stronger present day barriers to connectivity (Boavida et al., 2019).
Biogeographic regions or affinities are also related to water mass properties (McClain and Hardy, 2010; Watling et al., 2013). Faunistic communities in the Galicia Bank (NW Spain) encompass Macaronesian, Mediterranean, NE and NW Atlantic species, due to the confluence of different intermediate and deep water masses in the area (Ruiz-Villarreal et al., 2006; Cartes et al., 2013). The afore mentioned turbulent ENACW-MOW boundary might allow the transport of coral larvae along the NE Atlantic margins (Somoza et al., 2014). At the Mid Atlantic Ridge, the Azores region hosts an exceptional biodiversity of CWCs (Figure 2), mostly gorgonians (75% of the octocoral species of European waters), with a greater affinity to the Lusitanian-Mediterranean biogeographic region (71% shared species) and to a lesser extent to the NW Atlantic (Braga-Henriques et al., 2013; Sampaio et al., 2019). The presence of multiple intermediate and deep water masses (NACW, subpolar origin waters, MOW, AAIW, NADW; Johnson and Stevens, 2000; Amorim et al., 2017) along the slopes of many seamounts are hypothesized to shape composition and bathymetrical distribution of coral gardens in the Azores (Braga-Henriques et al., 2013). Braga-Henriques et al., 2013 reported maximum Antipatharian diversity at 400–500 m coinciding with the cold and less dense NACW; whilst the maximum diversity of Alcyonacea (e.g., Viminella flagellum, D. meteor, Callogorgia verticillata, and A. armata) occurs at 500–600 m coinciding with the less saline subpolar-origin waters. The maximum diversity of Scleractinians (e.g., Caryophyllia cyathus, M. oculata, and L. pertusa) occurs at 500–800 m and for Stylasteridae (e.g., Errina dabneyi) at 800–1000 m, which appear to be more diverse and abundant where the saltier and warmer MOW waters occur. It should be noticed, however, that this pattern was based mostly on corals collected as bycatch from longline fishing.
In the NW Atlantic, a L. pertusa reef was recently discovered in the Davis Strait area (Kenchington et al., 2017) on a steep slope with relatively stable bottom temperature, the highest salinities at intermediate depths (800–900 m) and exceptional persistently high currents of >15 cm s–1 at 1000 m. Signals of consistent vertical and horizontal transport over the reef area likely arose through a combination of local convection from the surface and advection of cooled and freshened waters at depth from the Irminger Sea to the east (Kenchington et al., 2017). Further to the south, in the Flemish Cap, biodiversity patterns of trawl caught invertebrates, including CWCs, were associated with the intensity of long-term trawling effort (NAFO, 2018). But also, the presence of the warmer NAC supports higher diversity in Flemish Cap areas under the influence of this water mass (NAFO, 2018). Whether the NAC supports higher diversity only by warming the bottom water or also through the northward transport of larvae from richer biogeographic regions in the south remains unclear.
Finally, the Gulf Stream mainly influences the oceanography of much of the southern part of the NW Atlantic, delimiting a biogeographic latitudinal boundary between warm-temperate and cool-temperate regions, particularly in the vicinity of Cape Hatteras (offshore North Carolina, United States). This is a transition region for many faunal groups, particularly fish and corals, which reach their southern and northern limits in this area (Ross and Quattrini, 2007; Obelcz et al., 2014; Csa Ocean Sciences et al., 2017). Off Nova Scotia (Canada) and the Mid-Atlantic Bight (United States), CWCs occur (Buhl-Mortensen et al., 2015; Csa Ocean Sciences et al., 2017) mainly in areas influenced by warm southwestward meanders of the Gulf Stream (Mienis et al., 2014). Similarly to the NE Atlantic, L. pertusa re-established at ∼7 kya in the NW Atlantic, in the Cape Lookout area (offshore North Carolina, United States), after a glacial period (Hebbeln et al., 2019). Ocean surface productivity and deep-sea temperatures reached interglacial levels several thousand years earlier, but did not facilitate L. pertusa to return to this area. Only the dramatic increase of the hydrodynamics ∼7 kya was likely to have enabled the return of this CWC (Matos et al., 2015). The intrusion of the Gulf Stream into the upper slope and the consequent increase of food supply is assumed to have promoted the re-establishment of L. pertusa (Matos et al., 2015; Hebbeln et al., 2019).
An intriguing feature of invertebrate communities in the deep-sea of the North Atlantic is the occurrences of large-sized (massive) sponges which form distinct habitats referred to as sponge grounds. One of the first reports of these habitats was by Rice et al. (1990), who observed dense aggregations (density values ∼1.5 ind⋅m–2) of the hexactinellid Pheronema carpenteri (Figure 2) in the Porcupine Seabight (NE Atlantic). Subsequently, records of sponge grounds came both from the eastern (e.g., Klitgaard and Tendal, 2004; Howell et al., 2016; Roberts et al., 2018; Kazanidis et al., 2019) and western deep North Atlantic (e.g., Murillo et al., 2012, 2016a, 2018; Knudby et al., 2013; Kenchington et al., 2014; Beazley et al., 2018). These included records not only of hexactinellids, but also of demosponges and especially those from the family Geodidae (Order Astrophorida, commonly known as ostur; Figure 2). Specifically, extensive studies in the NE Atlantic by Klitgaard and Tendal (2004) revealed two types of ostur: firstly, a boreal ostur dominated by G. barretti, G. macandrewi, G. atlantica, Isopsphle graei, Stryphnus ponderosus, and Stelletta normani found at the Faroe Islands, Norway, Sweden, Barents Sea, and Iceland. Secondly, a cold water ostur characterized by the same genera but represented by different species, i.e., G. mesotriaena, I. graei pyriformis, and S. rhaphidiophora found north of Iceland, Denmark Strait, East Greenland, and north of Spitzbergen. Cárdenas et al. (2013) supported the observations of boreal and arctic species of geodids put forward by Klitgaard and Tendal (2004) and extended the description to the full North Atlantic.
The species composition of deep-sea sponge grounds in the NW Atlantic, from Davis Strait to Flemish Cap (Murillo et al., 2012, 2016b, 2018), is similar to the boreal sponge grounds described by Klitgaard and Tendal (2004) for the NE Atlantic, while arctic astrophorids are found in southern Baffin Bay (Murillo et al., 2018). Dense aggregations of a globally unique population of the glass sponge Vazella pourtalesii is found on the Scotian Shelf over large spatial scales (Beazley et al., 2018). Glass sponges are also common on the walls of submarine canyons in the Mid-Atlantic Bight (Csa Ocean Sciences et al., 2017). In contrast, glass sponge grounds formed by P. carpenteri form extensive aggregations in the NE Atlantic. Further south in the NE Atlantic, the Gorringe Bank (SW off Portugal coast) is a hotspot for demosponge fauna (Xavier and Van Soest, 2007). The influence of the Azores current and the MOW in this area and the presence of several seamounts, allows the presence of demosponge assemblages with a wide Atlanto – Mediterranean distribution (61%) together with highly endemic (28%) species (Xavier and Van Soest, 2007).
Hydrography plays an important role in shaping the distribution of these dense sponge grounds, and several authors have noted the association of sponges with particular water masses due to their temperature and salinity characteristics or the hydrodynamic conditions, such as tides and internal waves, which enhance the food supply (Bett, 2001; Klitgaard and Tendal, 2004; Murillo et al., 2012; Beazley et al., 2015, 2018; Roberts et al., 2018; Davison et al., 2019; Kazanidis et al., 2019). For example, in the continental margin offshore of Newfoundland (eastern Canada), there is evidence for a relationship between dense sponge aggregations with a warm (∼3.0–3.5oC), and salty (∼34.85–34.90), water mass that occurs between ∼1300 and 1723 m depth and is thought to be a remnant of the Irminger Current (Beazley et al., 2015). Modeling work to predict the presence of deep-sea sponge grounds concluded that depth and bottom minimum salinity were major predictors of the distribution of sponge grounds in these areas (Knudby et al., 2013). As in the Flemish Cap area, boreal geodiid species in Davis Strait and Baffin Bay (NW Atlantic) were associated with the presence of water transported in the Irminger Current (Figure 1), while the Arctic geodiids were associated with Baffin Bay Deep Water between 1200 and 1800 m depth, and Baffin Bay Bottom Water > 1800 m. This association of boreal sponge grounds with Irminger Water in the areas mentioned above, points to it being a major determinant of distribution, with the NAC and its branch, the Irminger Current, providing a connectivity pathway between the NE and NW Atlantic (Bersch et al., 1999), potentially explaining the basin-scale distribution of some of these species. As the NAC and Irminger Currents (Figure 1) are integral to the AMOC (Cuny et al., 2002), any changes in this circulation may impact the distribution of deep-water sponges. Interestingly, geodiid sponge grounds off the Flemish Cap and Grand Banks have persisted for the last 130 kya (Murillo et al., 2016a), despite large shifts in water mass structure alternating between the warmer Atlantic waters and the cooler Labrador Current. Murillo et al., 2018 further noted that the structure-forming Geodia species were found in areas with high primary production and fast currents that would provide the high food supply needed to reach their large biomasses. The importance of current regime and interactions between water masses has also been shown for the Faroe-Shetland Channel deep-sea sponge aggregations (Kazanidis et al., 2019), the Arctic sponge ground on the Schultz Seamount (Arctic Mid-Ocean Ridge; Roberts et al., 2018) and deep-sea aggregations of hexactinellids in the NE Atlantic (Rice et al., 1990; Barthel et al., 1996).
The occurrence of CWCs reefs and gardens (Mortensen et al., 2001) and sponge grounds (Murillo et al., 2012; Beazley et al., 2015; Howell et al., 2016) have been tightly related to water mass properties and circulation (e.g., Davies et al., 2009; Mohn et al., 2014; Kenchington et al., 2017; Lacharité and Metaxas, 2018) as well as the forcing of climate conditions such as glacial periods, the presence and strength of fronts, etc. (e.g., Frank et al., 2011; Montero-Serrano et al., 2011; Hebbeln et al., 2019). However, climate models forecast that anthropogenic climate change will modify circulation patterns and fluxes, energy flow and water mass properties of the Atlantic Ocean by 2100 (Mora et al., 2013; Rahmstorf et al., 2015; Sweetman et al., 2017; Osman et al., 2019). A 25% weakening of the AMOC is predicted, and although a collapse of the AMOC is thought unlikely, it has not been excluded (Schmittner, 2005; Liu et al., 2018; Thornalley et al., 2018). The deep ocean is already experiencing changes in physical and chemical properties due to warming, OA or deoxygenation, which are predicted to impact deep-sea ecosystems (Levin and Le Bris, 2015; Johnson et al., 2018).
Based on the projections of Mora et al. (2013); Sweetman et al. (2017), and Morato et al. (2020), which used all available data generated by Earth System Models (Earth System Grid Federation, 2019), we reviewed the forecasted changes in the water mass properties at the seafloor in the deep North Atlantic (20°–70° N and 78° W–10° E). Namely, we evaluated how potential temperature at the seafloor (°C), particulate organic carbon (POC) flux at 100 m depth (mg C⋅m–2⋅d–1), DO concentration at the seafloor (ml⋅l–1) and pH at the seafloor, and were forecasted to change from 1951 to 2000 (historical simulation) to 2081–2100 (RCP8.5 or business-as-usual scenario). The POC flux at the seafloor was calculated was calculated based on CIMP5 ocean biogeochemical nutrient–phytoplankton–zooplankton– detritus models (Fu et al., 2016) and the Martin curve (Martin et al., 1987) with an export depth set to 100 m. Forecasted changes in aragonite and calcite Ω at the seafloor, as described in Morato et al. (2020), were also evaluated here. The multimodel averages of the projections of water mass properties based on all available Earth System Models ensure the highest possible precision and accuracy of the predictions (Mora et al., 2013). Further details of projections and model performance can be found in Mora et al. (2013). It is important to note that the business-as-usual scenario is the IPCC worst-case scenario where greenhouse gas emissions, world population growth and technology development (Riahi et al., 2011) continuously increase. Consequently, the projected future water mass properties are the most extreme forecasts and present greater uncertainty than other intermediate scenarios. Nevertheless, these forecasts and their possible consequences for VMEs in the North Atlantic are critical for decision-makers for the development of long-term sustainable management plans (Rheuban et al., 2018).
Global warming due to the influx of CO2 to the atmosphere increased ocean temperatures both in surface and deep waters (Purkey and Johnson, 2010; Levitus et al., 2012). The average global temperature of the upper ocean (0–2000 m) has already warmed by ∼0.09°C between 1955 and 2010 (Levitus et al., 2012). Recent climate models forecast an average 0.76°C temperature increase at bathyal depths but with no major changes in the abyss by 2100 (Table 1). However, some northern areas of the North Atlantic will experience an increase in temperature >1°C or >2°C (Figure 3), with the largest increases in temperature in the upper and intermediate bathyal layers (Table 1). In contrast, a large bathyal area between the Azores and Iceland has been forecasted to experience a decrease in seafloor temperatures of ∼0.7°C (Figure 3). This is one of the few areas in the world’s oceans where seafloor temperatures are predicted to decrease instead of increase.
Figure 3. Present and future scenarios predicted for temperature, particulate organic carbon (POC) flux and dissolved oxygen at the seafloor in the North Atlantic Ocean. Changes, as increment (Δ) between periods, were measured as the difference between present-day and the modeled conditions by 2100. Areas with critical changes in the values of a given water mass property are highlighted.
Enhanced warming of the upper ocean, stratification and OA is expected to reduce the export flux and quality of POC that reaches the seafloor (Smith et al., 2008; Jones et al., 2014; Sweetman et al., 2017). On average, most of North Atlantic bathyal seafloor is not currently food limited (POC flux > 10 mg C⋅m–2⋅day–1; Table 1 and Figure 3). However, the POC flux is predicted to decrease by 2100 up to 40% in the continental margins and critically to reduce by >50% in the central-eastern Atlantic (Figure 3).
The deep North Atlantic is the most alkaline of the world oceans (Sweetman et al., 2017) with present-day pH values ranging on average from 7.91 to 8.14 (Figure 4 and Table 1). Model predictions indicate that pH at the seafloor will further decrease by ∼0.2 in the bathyal zone, with smaller changes in the abyss (Figure 4 and Table 1). The greatest reduction in pH (>0.3) is expected at higher latitudes (Figure 4) in waters shallower than 1000 m, as a result of the subduction of high-CO2 waters via the AMOC (Gehlen et al., 2014; Sweetman et al., 2017; Perez et al., 2018).
Figure 4. Present and future scenarios predicted for pH and aragonite and calcite saturation states at the seafloor in the North Atlantic Ocean. Changes, as increment (Δ) between periods, were measured as the difference between present-day and the modeled conditions by 2100. Areas with critical changes in the values of a given water mass property are highlighted.
Large decreases in the carbonate Ω have been predicted worldwide (Orr et al., 2005), with severe changes in the North Atlantic (Perez et al., 2018). Present-day aragonite Ω in the North Atlantic varies greatly with depth (Figure 4). In general, bathyal depths are supersaturated, while abyssal areas are undersaturated (Table 1). However, aragonite Ω is expected to decrease by ∼32% above 3000 m depth and by ∼8% at the bathyal seafloor (Table 1). Thus, most of the deep North Atlantic will become undersaturated in aragonite by 2100 (Figure 4). On average, the aragonite SH will shoal by ∼800 m to depths of around 3000 m in the western North Atlantic, and by ∼1500 m to depths of 2000 m in the central and eastern North Atlantic (Figure 4). Shoaling will be greater in the northernmost areas moving aragonite SH to depths as shallow as 200 m. Areas deeper than 3000 m will experience smaller changes (Figure 4). Most of the North Atlantic seafloor is also currently supersaturated in calcite, except for areas below 5000 m (Table 1 and Figure 4). Similar reductions are expected for calcite Ω in the bathyal and abyssal areas, but with most of the seafloor remaining supersaturated. The calcite SH will shoal to 4500 m in the southernmost parts of the North Atlantic (Figure 4).
Ocean warming also causes the loss of DO in ocean waters, through reduced solubility, intensified biological respiration, and increased stratification of water masses (Keeling et al., 2010; Levin, 2018). The open ocean has already lost on average 2% of DO since the preindustrial era, particularly in intermediate waters between 100 and 1000 m depth (Stramma et al., 2010; Bopp et al., 2013; Levin, 2018). Model simulations forecast that most of the seafloor below 200 m is currently well oxygenated (>5 ml⋅l–1; Figure 3 and Table 1). A slight decrease of ∼5% in both abyssal and bathyal zones is expected by 2100 (Figure 3 and Table 1). A maximum decrease of 10% in DO will occur in northernmost areas (Figure 3), but no moderately (∼1.5 ml⋅l–1) or severely (< 0.5 ml⋅l–1) hypoxic conditions are expected in the North Atlantic in the future.
The assessment of ecologically critical climate conditions requires an understanding of how climate (i.e., environmental conditions) drives biological responses (see section Water Mass Properties as Drivers of Deep-Sea Biodiversity and Biogeography), forecasts of how climate will change (see section Future Water Mass Properties in the North Atlantic), and an identification of where those critical climate changes will occur (Diffenbaugh and Field, 2013). To contribute to this discussion, we built maps of cumulative critical changes. Cumulative critical changes were assumed to be (1) temperature increases >1°C (Figure 5A) or >2°C (Figure 5B; IPCC, 2014), (2) POC flux reductions >40% (Figure 5A) or >50% (Figure 5B; Mora et al., 2013; Sweetman et al., 2017), (4) pH reductions >0.3 (IPCC, 2013), and (4) new areas where aragonite and (5) calcite saturation was reduced to levels <1 (Caldeira and Wickett, 2003; Orr et al., 2005). Two values of critical changes were adopted for temperature and POC flux reduction because of the uncertainty about the degree of change that is critical for the survival of deep-sea benthic communities. Maps of cumulative critical changes were produced by identifying how many of the five water mass properties will change to the afore mentioned critical water mass properties at a certain cell. Most abyssal areas of the North Atlantic are forecasted to experience critical environmental changes affecting deep-sea benthic biodiversity (Figure 5). Northern areas, such as the Labrador Sea, Davis Strait and Western Greenland, will experience critical changes of pH, aragonite saturation and temperature (Figure 5). The NE Atlantic region close to the Bay of Biscay will experience changes in aragonite, pH, and POC flux. Several regions will also experience critical changes of at least two parameters. A large area of the Azores is expected to present critical changes in aragonite saturation and POC flux, while the NW Atlantic will face critical changes in aragonite saturation or temperature (Figure 5).
Figure 5. Predicted cumulative critical changes in seafloor conditions in the North Atlantic Ocean by 2100. Critical conditions include temperature increase of (A) >1°C or (B) >2°; particulate organic carbon (POC) flux reduction of (A) >40% or (B) >50%; pH reduction >0.3 and aragonite and calcite saturation levels <1. Dissolved oxygen will not likely achieve hypoxic conditions values <1.5 ml⋅l– 1 in 2100 so it was not included.
Climate models forecast that the deep seafloor at high latitudes of the North Atlantic is likely to experience warming of 2°C. Despite many CWC reefs occurring in these regions, temperature is not expected to exceed the range (4–12°C) where L. pertusa is generally found in the North Atlantic (Gass and Roberts, 2006; Roberts et al., 2009b), nor exceed the mortality temperatures reported, >14°C (Brooke et al., 2013; Lunden et al., 2014). Warming is more likely to impact CWCs living close to their upper temperature limit of physiological tolerance (e.g., Gulf of Mexico; Brooke et al., 2013; Lunden et al., 2014, and the Mediterranean Sea; Gori et al., 2016). In addition to increased average temperatures, marine heat waves are already becoming more frequent under climate change conditions (Frölicher et al., 2018), causing devastating impacts on marine ecosystems and/or even mass mortality events (Guihen et al., 2012; Cavole et al., 2016; Frölicher and Laufkötter, 2018). Despite the tendency of marine heatwaves to mainly propagate in surface waters, they might impact deep-sea ecosystems as well, particularly in the northernmost areas of the North Atlantic, where CWCs and sponges inhabit shallower waters due to the colder conditions.
Some scleractinian CWC species are able to calcify and grow under warmer conditions (Δ 3–4°C), even when combined with acidified waters (Hennige et al., 2014; Büscher et al., 2017; Maier et al., 2019). However, the energetic cost of these process in such conditions may diminish other biological and physiological processes, or prevent sustained growth over long-term periods (Dodds et al., 2007; Hennige et al., 2015). This is particularly true when combined with aragonite undersaturation and low food conditions. Thus, the resilience of CWCs to warming and acidification may be reduced in areas with limited food (McCulloch et al., 2012b), compromising coral metabolism and even the survival of corals (Maier et al., 2016).
The predicted shoaling of the aragonite SH is expected to lead to a loss of suitable habitat for slow-growing reef-forming CWC species (Davies and Guinotte, 2011; Perez et al., 2018), which are commonly found at aragonite SH depth at present-day (Guinotte et al., 2006; Davies and Guinotte, 2011). According to model simulations, during the pre-industrial period, 87% of CWC reefs were surrounded by aragonite oversaturated waters, while projections for 2100 suggest that around 73% of these reefs will suffer from undersaturated waters (Guinotte et al., 2006; Zheng and Cao, 2014). Scleractinian CWCs in the NE Atlantic are predicted to be particularly vulnerable to OA since they are mainly formed by aragonite, with 75% of CWCs below 1000 m being exposed to aragonite undersaturated waters by 2060 under the “business as usual” RCP 8.5 scenario (Perez et al., 2018). According to climate models forecasts, northern areas within the Reykjanes Ridge below 1000 m and Davis Strait and western Greenland as shallow as 200 m may be exposed to corrosive (i.e., aragonite understaturated) waters in the future. Despite the apparent physiological resilience of CWCs to OA, how OA or the high energetic cost for adaptation will affect other important physiological processes, such as reproduction, is still unknown. The long-term survival of reefs may be impaired by the chemical dissolution and biological erosion of the unprotected tissue of the reef framework exposed to corrosive waters (Hennige et al., 2015; Schönberg et al., 2017; see also section Water Mass Properties as Drivers of Deep-Sea Biodiversity and Biogeography). The balance between construction and erosion processes ultimately determines if the reef will grow or recede (Schönberg et al., 2017). Shifts toward net negative balances may lead to loss of reef growth potential, reef structural collapse, and diminished ecosystem service provisioning such as nutrient cycling, carbon storage and habitat provision, with severe consequences such as the loss of biodiversity associated with these ecosystems (Rossi et al., 2019).
This shoaling of aragonite undersaturated waters and the resultant reduction of habitat suitability for L. pertusa in the NW Atlantic due to OA can also have important consequences on the connectivity pathway between the NW and NE Atlantic populations (Kenchington et al., 2014). However, most of the large living CWCs reefs and banks on the Atlantic coast of USA occur in waters shallower than 1000 m depth and some in the NE Atlantic above 200 m (e.g., Mingulay reef complex, Rockall Bank on the UK continental shelf, CWC reefs on the Norwegian shelf), which will not be subjected to corrosive waters. Thus, those might provide a refuge for important habitat-building species such as L. pertusa in the North Atlantic. The conservation of these VMEs within Marine Protected Areas (Johnson and Kenchington, 2019) where for example trawling is banned, provides additional support to ensure the long-term survival of these habitats under regional predictions of 85% CWC loss due to aragonite SH shoaling for this area of the NE Atlantic (Jackson et al., 2014). Deep-sea ecosystems in more southerly locations of the North Atlantic (e.g., Cape Cod, the Azores or the Bay of Biscay) are located above the aragonite SH shoaling depths predicted for 2100 and will thus be protected from corrosive waters. However, as suggested from several experimental studies, reduction of pH even continuing within supersaturated water, might also negatively impact CWCs and gorgonians growth (Maier et al., 2009; Bramanti et al., 2013; Gómez et al., 2018).
The model forecasting suggests large reductions in POC fluxes in areas where shoaling of the aragonite SH and warming is also expected, including Iceland, Greenland and Eastern Canada, where the northernmost populations of L. pertusa are found. This will likely reduce the biogeographic distribution of this reef-forming species. The reductions in POC can have extreme effects in areas such as the Azores, where food availability is already limited (Jones et al., 2014). In particular, seamount features, such as the many seamounts in the northern Mid-Atlantic Ridge, are projected to experience large declines in POC fluxes and benthic biomass (Jones et al., 2014). Global projections of changes in POC export associated with other climate change effects suggest CWC ecosystems will suffer the greatest declines in benthic biomass and biodiversity and as a consequence, in ecological functioning and services provided (Jones et al., 2014; Armstrong et al., 2019).
With the potentially significant future changes in ocean water masses and circulation, deep-sea VMEs, such as the CWC reefs, gardens and sponge grounds found in the North Atlantic, are very likely to experience a dramatic loss of species diversity and population densities. However, many knowledge gaps remain in the biological processes and oceanographic dynamics in the deep-sea. Critical information for predicting tipping points, adaptation or resilience are still unknown for most of the deep-sea benthic organisms at species, population, and community-level processes (Hebbeln et al., 2019). An improved understanding of these drivers would help enable us to more clearly identify and describe the various impacts of climate change. It will also help us to understand the risks and how to mitigate them better, e.g., where we need to be more precautionary in terms of economic development of marine resources, where we may need to step up investment in monitoring and management, or how to establish efficient conservation strategies to preserve these fragile and precious deep-sea ecosystems.
The loss in biodiversity will potentially reduce severely the ecosystem resilience to both climate change adaptation and mitigation. Despite of the socio-political pressures for increasing the exploitation of marine resources, the deep-sea VMEs which are more likely to be impacted by multiple stressors are unlikely to withstand these additional pressures from human activities. Multiple interactive stressors imposed by global climate change and anthropogenic activities will impair the biological impacts and responses beyond the influence of any single variable (Hofmann et al., 2010; Büscher et al., 2017). Therefore, the backdrop of climate change will narrow the margins for safe ecological and thus operational limits of existing and emerging ocean industries over the next century. Further, most of the areas currently closed to protect VMEs in the North Atlantic will be impacted by climate change (Johnson et al., 2018) and will require monitoring and reconsideration of boundaries in future. Beyond marine spatial planning, regulatory frameworks and conservation actions, the blue economy should adopt precautionary approaches so as not to further push living marine resources beyond their tipping points and to ensure sustainable development.
PP, CO, L-AH, and MC-S designed the structure of the manuscript. PP coordinated, supervised, and wrote the manuscript. C-LW developed the projections of future environmental conditions for the deep North Atlantic. TM, JG-I, and MC-S downscaled future projections and designed critical changes. All authors were substantially involved in the literature search and writing of the different sections of the manuscript, contributed to the final product in significant ways, and provided approval for publication.
This study was supported by the European Union’s Horizon 2020 Research and Innovation Program, under the ATLAS project (Grant Agreement No. 678760). This output reflects only the author’s view, and the European Union cannot be held responsible for any use that may be made of the information contained therein. TM was supported by Program Investigador FCT (IF/01194/2013), IFCT Exploratory Project (IF/01194/2013/CP1199/CT0002) from the Fundação para a Ciência e Tecnologia (POPH and QREN), PO2020 MapGes (Acores-01-0145-FEDER-000056). TM and MC-S also acknowledge the Fundação para a Ciência e a Tecnologia (FCT) through the strategic project (UID/05634/2020) granted to OKEANOS.
The authors declare that the research was conducted in the absence of any commercial or financial relationships that could be construed as a potential conflict of interest.
We thank the reviewers for the valuable comments provided.
Allen, J. A., and Sanders, H. L. (1996). The zoogeography, diversity and origin of the deep-sea protobranch bivalves of the Atlantic: the epilogue. Prog. Oceanogr. 38, 95–153. doi: 10.1016/s0079-6611(96)00011-0
Amorim, P., Perán, A. D., Pham, C. K., Juliano, M., Cardigos, F., Tempera, F., et al. (2017). Overview of the ocean climatology and its variability in the Azores region of the North Atlantic including environmental characteristics at the seabed. Front. Mar. Sci. 4:56. doi: 10.3389/fmars.2017.00056
Armstrong, C. W., Vondolia, G. K., Foley, N. S., Henry, L.-A., Needham, K., and Ressurreição, A. (2019). Expert assessment of risks posed by climate change and anthropogenic activities to ecosystem services in the deep North Atlantic. Front. Mar. Sci. 6:158. doi: 10.3389/fmars.2019.00158
Arnaud-Haond, S., Van den Beld, I. M. J., Becheler, R., Orejas, C., Menot, L., Frank, N., et al. (2017). Two “pillars” of cold-water coral reefs along Atlantic European margins: prevalent association of Madrepora oculata with Lophelia pertusa, from reef to colony scale. DeepRes. Part II Top. Stud. Oceanogr. 145, 110–119. doi: 10.1016/j.dsr2.2015.07.013
Barthel, D., Tendal, O. S., and Thiel, H. (1996). A wandering population of the HexactinelIid sponge Pheronema carpenteri on the continental slope off Morocco, Northwest Africa. Mar. Ecol. 17, 603–616. doi: 10.1111/j.1439-0485.1996.tb00420.x
Bates, T. E. M., and Bell, J. J. (2018). Responses of two temperate sponge species to ocean acidification. New Zeal. J. Mar. Freshw. Res. 52, 247–263. doi: 10.1080/00288330.2017.1369132
Beazley, L., Kenchington, E., Yashayaev, I., and Murillo, F. J. (2015). Drivers of epibenthic megafaunal composition in the sponge grounds of the Sackville Spur, northwest Atlantic. DeepRes. Part I Oceanogr. Res. 98, 102–114. doi: 10.1016/j.dsr.2014.11.016
Beazley, L., Wang, Z., Kenchington, E., Yashayaev, I., Rapp, H. T., Xavier, J. R., et al. (2018). Predicted distribution of the glass sponge Vazella pourtalesii on the Scotian Shelf and its persistence in the face of climatic variability. PLoS One 13:e0205505. doi: 10.1371/journal.pone.0205505
Beazley, L. I., Kenchington, E. L., Murillo, F. J., and Sacau, M. (2013). Deep-sea sponge grounds enhance diversity and abundance of epibenthic megafauna in the Northwest Atlantic. ICES J. Mar. Sci. 70, 1471–1490. doi: 10.1093/icesjms/fst124
Belkin, I. M. (2004). Propagation of the “Great Salinity Anomaly” of the 1990s around the northern North Atlantic. Geophys. Res. Lett. 31:8306. doi: 10.1029/2003GL019334
Bell, J. J., Bennett, H. M., Rovellini, A., and Webster, N. S. (2018). Sponges to be winners under near-future climate scenarios. Bioscience 68, 955–968. doi: 10.1093/biosci/biy142
Bersch, M., Meincke, J., and Sy, A. (1999). Interannual thermohaline changes in the northern North Atlantic 1991–1996. Deep Sea Res. Part II Top. Stud. Oceanogr. 46, 55–75. doi: 10.1016/S0967-0645(98)00114-3
Bett, B. J. (2001). UK atlantic margin environmental survey: introduction and overview of bathyal benthic ecology. Cont. Shelf Res. 21, 917–956. doi: 10.1016/S0278-4343(00)00119-9
Boavida, J. R. H., Becheler, R., Choquet, M., Frank, N., Taviani, M., Bourillet, J.-F. F., et al. (2019). Out of the Mediterranean? Post-glacial colonization pathways varied among cold-water coral species. J. Biogeogr. 46, 915–931. doi: 10.1111/jbi.13570
Bopp, L., Resplandy, L., Orr, J. C., Doney, S. C., Dunne, J. P., Gehlen, M., et al. (2013). Multiple stressors of ocean ecosystems in the 21st century: projections with CMIP5 models. Biogeosciences 10, 6225–6245. doi: 10.5194/bg-10-6225-2013
Bower, A. S., Le Cann, B., Rossby, T., Zenk, W., Gould, J., Speer, K., et al. (2002). Directly measured mid-depth circulation in the northeastern North Atlantic Ocean. Nature 419, 603–607. doi: 10.1038/nature01078
Braga-Henriques, A., Porteiro, F. M., Ribeiro, P. A., De Matos, V., Sampaio, Í, Ocaña, O., et al. (2013). Diversity, distribution and spatial structure of the cold-water coral fauna of the Azores (NE Atlantic). Biogeosciences 10, 4009–4036. doi: 10.5194/bg-10-4009-2013
Bramanti, L., Movilla, J., Guron, M., Calvo, E., Gori, A., Dominguez-Carrió, C., et al. (2013). Detrimental effects of ocean acidification on the economically important Mediterranean red coral (Corallium rubrum). Glob. Change Biol. 19, 1897–1908. doi: 10.1111/gcb.12171
Brooke, S., Ross, S. W., Bane, J. M., Seim, H. E., and Young, C. M. (2013). Temperature tolerance of the deep-sea coral Lophelia pertusa from the southeastern United States. Deep Res. Part II Top. Stud. Oceanogr. 92, 240–248. doi: 10.1016/j.dsr2.2012.12.001
Brooke, S., and Schroeder, W. W. (2007). “State of deep coral ecosystems in the Gulf of Mexico region: Texas to the florida straits,” in The State of Deep Coral Ecosystems of the United States, eds S. E. Lumsden, T. F. Hourigan, A. W. Bruckner, and G. Dorr (Silver Spring, MD: NOAA), 271–306.
Brooke, S. D., Watts, M. W., Heil, A. D., Rhode, M., Mienis, F., Duineveld, G. C. A., et al. (2017). Distributions and habitat associations of deep-water corals in Norfolk and Baltimore Canyons, Mid-Atlantic Bight, USA. Deep Sea Res. Part II Top. Stud. Oceanogr. 137, 131–147. doi: 10.1016/j.dsr2.2016.05.008
Bryan, T. L., and Metaxas, A. (2006). Distribution of deep-water corals along the North American continental margins: relationships with environmental factors. Deep Res. Part I Oceanogr. Res. 53, 1865–1879. doi: 10.1016/j.dsr.2006.09.006
Buhl-Mortensen, L., and Mortensen, P. B. (2004). Symbiosis in deep-water corals. Symbiosis 37, 33–61.
Buhl-Mortensen, L., Olafsdottir, S. H., Buhl-Mortensen, P., Burgos, J. M., and Ragnarsson, S. A. (2015). Distribution of nine cold-water coral species (Scleractinia and Gorgonacea) in the cold temperate North Atlantic: effects of bathymetry and hydrography. Hydrobiologia 759, 39–61. doi: 10.1007/s10750-014-2116-x
Buhl-Mortensen, L., Vanreusel, A., Gooday, A. J., Levin, L. A., Priede, I. G., Buhl-Mortensen, P., et al. (2010). Biological structures as a source of habitat heterogeneity and biodiversity on the deep ocean margins. Mar. Ecol. 31, 21–50. doi: 10.1111/j.1439-0485.2010.00359.x
Buhl-Mortensen, P., and Buhl-Mortensen, L. (2014). Diverse and vulnerable deep-water biotopes in the hardangerfjord. Mar. Biol. Res. 10, 253–267. doi: 10.1080/17451000.2013.810759
Büscher, J. V., Form, A. U., and Riebesell, U. (2017). Interactive effects of ocean acidification and warming on growth, fitness and survival of the cold-water coral Lophelia pertusa under different food availabilities. Front. Mar. Sci. 4:101.
Büscher, J. V., Wisshak, M., Form, A. U., Titschack, J., Nachtigall, K., and Riebesell, U. (2019). In situ growth and bioerosion rates of Lophelia pertusa in a Norwegian fjord and open shelf cold-water coral habitat. PeerJ 7:e7586. doi: 10.7717/peerj.7586
Byrne, M. (2011). Impact of ocean warming and ocean acidification on marine in vertebrate life history stages?: vulnerabilities and potential for persistence in a changing ocean. Oceanogr. Mar. Biol. An Annu. Rev. 49, 1–42.
Caesar, L., Rahmstorf, S., Robinson, A., Feulner, G., and Saba, V. (2018). Observed fingerprint of a weakening Atlantic Ocean overturning circulation. Nature 556, 191–196. doi: 10.1038/s41586-018-0006-5
Caldeira, K., and Wickett, M. E. (2003). Anthropogenic carbon and ocean pH. Nature 425:365. doi: 10.1038/425365a
Canals, M., Puig, P., de Madron, X. D., Heussner, S., Palanques, A., and Fabres, J. (2006). Flushing submarine canyons. Nature 444, 354–357. doi: 10.1038/nature05271
Cárdenas, P., Rapp, H. T., Klitgaard, A. B., Best, M., Thollesson, M., and Tendal, O. S. (2013). Taxonomy, biogeography and DNA barcodes of Geodia species (Porifera, Demospongiae, Tetractinellida) in the Atlantic boreo-arctic region. Zool. J. Linn. Soc. 169, 251–311. doi: 10.1111/zoj.12056
Carreiro-Silva, M., Ocaña, O., Stankoviæ, D., Sampaio, Í, Porteiro, F. M., Fabri, M.-C., et al. (2017). Zoantharians (Hexacorallia: Zoantharia) associated with cold-water corals in the Azores region: new species and associations in the deep sea. Front. Mar. Sci. 4:88. doi: 10.3389/fmars.2017.00088
Cartes, J. E., Papiol, V., Frutos, I., Macpherson, E., González-Pola, C., Punzón, A., et al. (2013). Distribution and biogeographic trends of decapod assemblages from Galicia Bank (NE Atlantic) at depths between 700 to 1800 m, with connexions to regional water masses. Deep Res. Part II Top. Stud. Oceanogr. 106, 165–178. doi: 10.1016/j.dsr2.2013.09.034
Cathalot, C., Van Oevelen, D., Cox, T. J. S., Kutti, T., Lavaleye, M., Duineveld, G., et al. (2015). Cold-water coral reefs and adjacent sponge grounds: hotspots of benthic respiration and organic carbon cycling in the deep sea. Front. Mar. Sci. 2:37. doi: 10.3389/fmars.2015.00037
Cavole, L. M., Demko, A. M., Diner, R. E., Giddings, A., Koester, I., Pagniello, C. M. L. S., et al. (2016). Biological impacts of the 2013–2015 warm-water anomaly in the northeast Pacific: winners, losers, and the future. Oceanography 29, 273–285. doi: 10.5670/oceanog.2016.32
Cerrano, C., Cardini, U., Bianchelli, S., Corinaldesi, C., Pusceddu, A., and Danovaro, R. (2013). Red coral extinction risk enhanced by ocean acidification. Sci. Rep. 3:1457. doi: 10.1038/srep01457
Chu, J., Maldonado, M., Yahel, G., and Leys, S. (2011). Glass sponge reefs as a silicon sink. Mar. Ecol. Prog. Ser. 441, 1–14. doi: 10.3354/meps09381
Clark, M. R., Rowden, A. A., Schlacher, T., Williams, A., Consalvey, M., Stocks, K. I., et al. (2010). The ecology of seamounts: structure, function, and human impacts. Ann. Rev. Mar. Sci. 2, 253–278. doi: 10.1146/annurev-marine-120308-181109
Cohen, A. L., and Holcomb, M. (2009). Why corals care about ocean acidification: uncovering the mechanism. Oceanography 22, 118–127. doi: 10.5670/oceanog.2009.102
Collart, T., Verreydt, W., Hernández-Molina, F. J., Llave, E., León, R., Gómez-Ballesteros, M., et al. (2018). Sedimentary processes and cold-water coral mini-mounds at the Ferrol canyon head, NW Iberian margin. Prog. Oceanogr. 169, 48–65. doi: 10.1016/j.pocean.2018.02.027
Costello, M. J., and Chaudhary, C. (2017). Marine biodiversity, biogeography, deep-sea gradients, and conservation. Curr. Biol. 27, R511–R527. doi: 10.1016/j.cub.2017.04.060
Csa Ocean Sciences International, Ross, S., Brooke, S., Baird, E., Coykendall, E., Davies, A., et al. (2017). Atlantic Deepwater Hard Bottom Habitats and Shipwrecks with Emphasis on Canyons and Coral Communities: Atlantic Deepwater Canyons Study. Washington, DC: U.S. Dept. of the Interior.
Cunha, M. R., Hilário, A., Santos, R. S., Hilario, A., and Santos, R. S. (2017). Advances in deep-sea biology: biodiversity, ecosystem functioning and conservation. An introduction and overview. Deep Res. Part II Top. Stud. Oceanogr. 137, 1–5. doi: 10.1016/j.dsr2.2017.02.003
Cuny, J., Rhines, P. B., Niiler, P. P., and Bacon, S. (2002). Labrador Sea boundary currents and the fate of the Irminger Sea Water. J. Phys. Oceanogr. 32, 627–647. doi: 10.1175/1520-04852002032<0627:LSBCAT>2.0.CO;2
Danovaro, R., Canals, M., Gambi, C., Heussner, S., Lampadariou, N., and Vanreusel, A. (2009). Exploring benthic biodiversity patterns and hot spots on European margin slopes. Oceanography 22, 16–25. doi: 10.5670/oceanog.2009.02
Danovaro, R., Dell’Anno, A., and Pusceddu, A. (2004). Biodiversity response to climate change in a warm deep sea. Ecol. Lett. 7, 821–828. doi: 10.1111/j.1461-0248.2004.00634.x
Danovaro, R., Snelgrove, P. V., and Tyler, P. (2014). Challenging the paradigms of deep-sea ecology. Trends Ecol. Evol. 29, 465–475. doi: 10.1016/j.tree.2014.06.002
Davies, A. J., Duineveld, G. C. A., van Weering, T. C. E., Mienis, F., Quattrini, A. M., Seim, H. E., et al. (2010). Short-term environmental variability in cold-water coral habitat at Viosca Knoll, Gulf of Mexico. Deep Res. Part I Oceanogr. Res. Pap. 57, 199–212. doi: 10.1016/j.dsr.2009.10.012
Davies, A. J., Duineveld, G. C. A. A., Lavaleye, M. S. S. S., Bergman, M. J. N. N., Van Haren, H., and Roberts, J. M. (2009). Downwelling and deep-water bottom currents as food supply mechanisms to the cold-water coral Lophelia pertusa (Scleractinia) at the Mingulay Reef complex. Limnol. Oceanogr. 54, 620–629. doi: 10.4319/lo.2009.54.2.0620
Davies, A. J., and Guinotte, J. M. (2011). Global habitat suitability for framework-forming Cold-Water Corals. PLoS One 6:e18483. doi: 10.1371/journal.pone.0018483
Davies, A. J., Wisshak, M., Orr, J. C., and Roberts, J. M. (2008). Predicting suitable habitat for the cold-water coral Lophelia pertusa (Scleractinia). Deep Res. Part I Oceanogr. Res. 55, 1048–1062. doi: 10.1016/j.dsr.2008.04.010
Davison, J. J., van Haren, H., Hosegood, P., Piechaud, N., and Howell, K. L. (2019). The distribution of deep-sea sponge aggregations (Porifera) in relation to oceanographic processes in the faroe-shetland channel. Deep Res. Part I Oceanogr. Res. Pap. 146, 55–61. doi: 10.1016/j.dsr.2019.03.005
De Goeij, J. M., van Oevelen, D., Vermeij, M. J. A., Osinga, R., Middelburg, J. J., de Goeij, A. F. P. M., et al. (2013). Surviving in a marine desert: the sponge loop retains resources within coral reefs. Science 342, 108–110. doi: 10.1126/science.1241981
De Mol, B., Henriet, J.-P., and Canals, M. (2005). “Development of coral banks in porcupine seabight: do they have Mediterranean ancestors?,” in Cold-Water Corals and Ecosystems, eds A. Freiwald and J. M. Roberts (Berlin: Springer), 515–533. doi: 10.1007/3-540-27673-4_26
De Mol, B., Van Rensbergen, P., Pillen, S., Van Herreweghe, K., Van Rooij, D., McDonnell, A., et al. (2002). Large deep-water coral banks in the Porcupine Basin, southwest of Ireland. Mar. Geol. 188, 193–231. doi: 10.1016/S0025-3227(02)00281-5
De Mol, L., Van Rooij, D., Pirlet, H., Greinert, J., Frank, N., Quemmerais, F., et al. (2011). Cold-water coral habitats in the Penmarc’h and guilvinec canyons (Bay of Biscay): deep-water versus shallow-water settings. Mar. Geol. 282, 40–52. doi: 10.1016/j.margeo.2010.04.011
Diffenbaugh, N. S., and Field, C. B. (2013). Changes in ecologically critical terrestrial climate conditions. Science 341, 486–492. doi: 10.1126/science.1237123
Dodds, L. A., Roberts, J. M., Taylor, A. C., and Marubini, F. (2007). Metabolic tolerance of the cold-water coral Lophelia pertusa (Scleractinia) to temperature and dissolved oxygen change. J. Exp. Mar. Bio. Ecol. 349, 205–214. doi: 10.1016/j.jembe.2007.05.013
Doney, S. (2009). The consequences of human-driven ocean acidification for marine life. Biol. Rep. 1, 1–36. doi: 10.3410/B1-36
Doney, S. C., Ruckelshaus, M., Emmett Duffy, J., Barry, J. P., Chan, F., English, C. A., et al. (2012). Climate change impacts on marine ecosystems. Ann. Rev. Mar. Sci. 4, 11–37. doi: 10.1146/annurev-marine-041911-111611
Dorschel, B., Hebbeln, D., Foubert, A., White, M., and Wheeler, A. J. (2007). Hydrodynamics and cold-water coral facies distribution related to recent sedimentary processes at Galway Mound west of Ireland. Mar. Geol. 244, 184–195. doi: 10.1016/j.margeo.2007.06.010
Duineveld, G. C. A., Jeffreys, R. M., Lavaleye, M. S. S., Davies, A. J., Bergman, M. J. N., Watmough, T., et al. (2012). Spatial and tidal variation in food supply to shallow cold-water coral reefs of the mingulay reef complex (Outer Hebrides, Scotland). Mar. Ecol. Prog. Ser. 444, 97–115. doi: 10.3354/meps09430
Duineveld, G. C. A., Lavaleye, M. S. S., Bergman, M. J. N., De Stigter, H., and Mienis, F. (2007). Trophic structure of a cold-water coral mound community (Rockall Bank, NE Atlantic) in relation to the near-bottom particle supply and current regime. Bull. Mar. Sci. 81, 449–467.
Dullo, W. C., Flogel, S., Ruggeberg, A., Flögel, S., and Rüggeberg, A. (2008). Cold-water coral growth in relation to the hydrography of the Celtic and Nordic European continental margin. Mar. Ecol. Prog. Ser. 371, 165–176. doi: 10.3354/meps07623
Dupont, S., and Thorndyke, M. C. (2009). Impact of CO2-driven ocean acidification on invertebrates early life-history – What we know, what we need to know and what we can do. Biogeosci. Discuss 6, 3109–3131. doi: 10.5194/bgd-6-3109-2009
Earth System Grid Federation (2019). Available online at: https://esgf-node.llnl.gov/projects/esgf-llnl/ (accessed April 2, 2019). doi: 10.5194/bgd-6-3109-2009
Eigaard, O. R., Bastardie, F., Hintzen, N. T., Buhl-Mortensen, L., Buhl-Mortensen, P., Catarino, R., et al. (2016). The footprint of bottom trawling in European waters: distribution, intensity, and seabed integrity. ICES J. Mar. Sci. 74:fsw194. doi: 10.1093/icesjms/fsw194
FAO (2009). International Guidelines for the Management of Deep-Sea Fisheries in the High Seas. Available online at: http://www.fao.org/3/i0816t/i0816t00.htm (accessed January 15, 2020).
Fernandez-Arcaya, U., Ramirez-Llodra, E., Aguzzi, J., Allcock, A. L., Davies, J. S., Dissanayake, A., et al. (2017). Ecological role of submarine canyons and need for canyon conservation: a review. Front. Mar. Sci. 4:5. doi: 10.3389/fmars.2017.00005
Form, A. U., and Riebesell, U. (2012). Acclimation to ocean acidification during long-term CO2 exposure in the cold-water coral Lophelia pertusa. Glob. Chang. Biol. 18, 843–853. doi: 10.1111/j.1365-2486.2011.02583.x
Foubert, A., Huvenne, V. A. I., Wheeler, A., Kozachenko, M., Opderbecke, J., and Henriet, J.-P. (2011). The Moira Mounds, small cold-water coral mounds in the Porcupine Seabight, NE Atlantic: part B - Evaluating the impact of sediment dynamics through high-resolution ROV-borne bathymetric mapping. Mar. Geol. 282, 65–78. doi: 10.1016/j.margeo.2011.02.008
Frank, N., Freiwald, A., López Correa, M., Wienberg, C., Eisele, M., Hebbeln, D., et al. (2011). Northeastern Atlantic cold-water coral reefs and climate. Geology 39, 743–746. doi: 10.1130/g31825.1
Frederiksen, R., Jensen, A., and Westerberg, H. (1992). The distribution of the Scleractinian coral Lophelia pertusa around the Faroe islands and the relation to internal tidal mixing. Sarsia 77, 157–171. doi: 10.1080/00364827.1992.10413502
Freiwald, A. (2002). “Reef-forming cold-water corals,” in Ocean Margin Systems, eds G. Wefer, D. Billett, D. Hebbeln, B. B. Jørgensen, M. Schlüter, and T. C. E. van Weering (Berlin: Springer), 365–385. doi: 10.1007/978-3-662-05127-6_23
Freiwald, A., Beuck, L., Rueggeberg, A., Taviani, M., and Hebbeln, D. (2009). The white coral community in the central Mediterranean sea revealed by ROV surveys. Oceanography 22, 58–74. doi: 10.5670/oceanog.2009.06
Frölicher, T. L., Fischer, E. M., and Gruber, N. (2018). Marine heatwaves under global warming. Nature 560, 360–364. doi: 10.1038/s41586-018-0383-389
Frölicher, T. L., and Laufkötter, C. (2018). Emerging risks from marine heat waves. Nat. Commun. 9, 2015–2018. doi: 10.1038/s41467-018-03163-6
Fu, W., Randerson, J. T., and Moore, J. K. (2016). Climate change impacts on net primary production (NPP) and export production (EP) regulated by increasing stratification and phytoplankton community structure in the CMIP5 models. Biogeosciences 13, 5151–5170. doi: 10.5194/bg-13-5151-2016
Gage, J. D. (2004). Diversity in deep-sea benthic macrofauna: the importance of local ecology, the larger scale, history and the Antarctic. Deep Res. Part II Top. Stud. Oceanogr. 51, 1689–1708. doi: 10.1016/j.dsr2.2004.07.013
Gammon, M. J., Tracey, D. M., Marriott, P. M., Cummings, V. J., and Davy, S. K. (2018). The physiological response of the deep-sea coral Solenosmilia variabilis to ocean acidification. PeerJ 6:e5236. doi: 10.7717/peerj.5236
García-Ibáñez, M. I., Pardo, P. C., Carracedo, L. I., Mercier, H., Lherminier, P., Ríos, A. F., et al. (2015). Structure, transports and transformations of the water masses in the Atlantic Subpolar Gyre. Prog. Oceanogr. 135, 18–36. doi: 10.1016/j.pocean.2015.03.009
Gass, S. E., and Roberts, J. M. (2006). The occurrence of the cold-water coral Lophelia pertusa (Scleractinia) on oil and gas platforms in the North Sea: colony growth, recruitment and environmental controls on distribution. Mar. Pollut. Bull. 52, 549–559. doi: 10.1016/j.marpolbul.2005.10.002
Gazeau, F., Parker, L. M., Comeau, S., Gattuso, J.-P., O’Connor, W. A., Martin, S., et al. (2013). Impacts of ocean acidification on marine shelled molluscs. Mar. Biol. 160, 2207–2245. doi: 10.1007/s00227-013-2219-3
Gehlen, M., Séférian, R., Jones, D. O. B., Roy, T., Roth, R., Barry, J., et al. (2014). Projected pH reductions by 2100 might put deep North Atlantic biodiversity at risk. Biogeosciences 11, 6955–6967. doi: 10.5194/bg-11-6955-2014
Genin, A., and Dower, J. F. (2007). “Seamount Plankton Dynamics,” in Seamounts: Ecology, Fisheries & Conservation, eds T. J. Pitcher, T. Morato, P. J. B. Hart, M. R. Clark, N. Haggan, and R. S. Santos (Hoboken: Wiley), 87–100. doi: 10.1002/9780470691953.ch5
Georgian, S. E., Dupont, S., Kurman, M., Butler, A., Strömberg, S. M., Larsson, A. I., et al. (2016). Biogeographic variability in the physiological response of the cold-water coral Lophelia pertusa to ocean acidification. Mar. Ecol. 37, 1345–1359.
Georgian, S. E., Shedd, W., and Cordes, E. E. (2014). High-resolution ecological niche modelling of the cold-water coral Lophelia pertusa in the Gulf of Mexico. Mar. Ecol. Prog. Ser. 506, 145–161. doi: 10.3354/meps10816
Glover, A. G., and Smith, C. R. (2003). The deep-sea floor ecosystem: current status and prospects of anthropogenic change by the year 2025. Environ. Conserv. 30, 219–241. doi: 10.1017/S0376892903000225
Gómez, C. E., Wickes, L., Deegan, D., Etnoyer, P. J., and Cordes, E. E. (2018). Growth and feeding of deep-sea coral Lophelia pertusa from the California margin under simulated ocean acidification conditions. PeerJ 6:e5671. doi: 10.7717/peerj.5671
Goodwin, C., Rodolfo-Metalpa, R., Picton, B., and Hall-Spencer, J. M. (2014). Effects of ocean acidification on sponge communities. Mar. Ecol. 35, 41–49. doi: 10.1111/maec.12093
Gori, A., Ferrier-Pages, C., Hennige, S. J., Murray, F., Rottier, C., Wicks, L. C., et al. (2016). Physiological response of the cold-water coral Desmophyllum dianthus to thermal stress and ocean acidification. PeerJ 4:16. doi: 10.7717/peerj.1606
Gori, A., Reynaud, S., Orejas, C., Gili, J. M., and Ferrier-Pages, C. (2014). Physiological performance of the cold-water coral Dendrophyllia cornigera reveals its preference for temperate environments. Coral Reefs 33, 665–674. doi: 10.1007/s00338-014-1167-1169
Gregory, J. M., Dixon, K. W., Stouffer, R. J., Weaver, A. J., Driesschaert, E., Eby, M., et al. (2005). A model intercomparison of changes in the Atlantic thermohaline circulation in response to increasing atmospheric CO2 concentration. Geophys. Res. Lett. 32:L12703. doi: 10.1029/2005GL023209
Griffin, S., and Druffel, E. R. M. (1989). Sources of carbon to deep-sea corals. Radiocarbon 31, 533–543. doi: 10.1017/S0033822200012121
Guihen, D., White, M., and Lundälv, T. (2012). Temperature shocks and ecological implications at a cold-water coral reef. Mar. Biodivers. Rec. 5:e68. doi: 10.1017/S1755267212000413
Guihen, D., White, M., and Lundälv, T. (2013). Boundary layer flow dynamics at a cold-water coral reef. J. Sea Res. 78, 36–44. doi: 10.1016/j.seares.2012.12.007
Guihen, D., White, M., and Lundälv, T. (2018). Zooplankton drive diurnal changes in oxygen concentration at Tisler cold-water coral reef. Coral Reefs 37, 1013–1025. doi: 10.1007/s00338-018-1711-1710
Guinotte, J. M., Orr, J., Cairns, S., Freiwald, A., Morgan, L., and George, R. (2006). Will human-induced changes in seawater chemistry alter the distribution of deep-sea Scleractinian corals? Front. Ecol. Environ. 4, 141–146. doi: 10.1890/1540-92952006004[0141:WHCISC]2.0.CO;2
Hansen, B., Husgaro, K. M., Hátún, H., and Østerhus, S. (2016). A stable Faroe Bank Channel overflow 1995-2015. Ocean Sci. 12, 1205–1220. doi: 10.5194/os-12-1205-2016
Hansen, B., and Østerhus, S. (2000). North Atlantic–Nordic Seas exchanges. Prog. Oceanogr. 45, 109–208. doi: 10.1016/S0079-6611(99)00052-X
Hanz, U., Wienberg, C., Hebbeln, D., Duineveld, G., Lavaleye, M., Juva, K., et al. (2019). Environmental factors influencing cold-water coral ecosystems in the oxygen minimum zones on the Angolan and Namibian margins. Biogeosci. Discuss 16, 4337–4356. doi: 10.5194/bg-2019-2052
Hebbeln, D., Portilho-Ramos, R., da, C., Wienberg, C., and Titschack, J. (2019). The fate of cold-water corals in a changing world: a geological perspective. Front. Mar. Sci. 6:119. doi: 10.3389/fmars.2019.00119
Hebbeln, D., and Wienberg, C. (2016). Good neighbours shaped by vigorous currents: cold-water coral mounds and contourites in the North Atlantic. Mar. Geol. 378, 171–185. doi: 10.1016/J.MARGEO.2016.01.014
Hebbeln, D., Wienberg, C., Bender, M., Bergmann, F., Dehning, K., Dullo, W.-C., et al. (2017). ANNA - Cold-water coral ecosystems off Angola and Namibia (Cruise No. M122). Walvis Bay: Technische Informations (TIB).
Helm, K. P., Bindoff, N. L., and Church, J. A. (2011). Observed decreases in oxygen content of the global ocean. Geophys. Res. Lett. 38:L23602. doi: 10.1029/2011GL049513
Hennige, S. J., Wicks, L. C., Kamenos, N. A., Bakker, D. C. E. E., Findlay, H. S., Dumousseaud, C., et al. (2014). Short-term metabolic and growth responses of the cold-water coral Lophelia pertusa to ocean acidification. Deep Res. Part II Top. Stud. Oceanogr. 99, 27–35. doi: 10.1016/j.dsr2.2013.07.005
Hennige, S. J., Wicks, L. C., Kamenos, N. A., Perna, G., Findlay, H. S., and Roberts, J. M. (2015). Hidden impacts of ocean acidification to live and dead coral framework. Proc. R. Soc. London B Biol. Sci. 282:20150990. doi: 10.1098/rspb.2015.0990
Henry, L.-A., Davies, A. J., and Roberts, J. M. (2010). Beta diversity of cold-water coral reef communities off western Scotland. Coral Reefs 29, 427–436. doi: 10.1007/s00338-009-0577-576
Henry, L.-A., Frank, N., Hebbeln, D., Wienberg, C., Robinson, L., de Flierdt, T., et al. (2014). Global ocean conveyor lowers extinction risk in the deep sea. Deep Res. Part I Oceanogr. Res. 88, 8–16. doi: 10.1016/j.dsr.2014.03.004
Henry, L.-A., and Roberts, J. M. (2007). Biodiversity and ecological composition of macrobenthos on cold-water coral mounds and adjacent off-mound habitat in the bathyal Porcupine Seabight, NE Atlantic. Deep Res. Part I Oceanogr. Res. 54, 654–672. doi: 10.1016/j.dsr.2007.01.005
Henry, L.-A., and Roberts, J. M. (2008). First record of Bedotella armata (Cnidaria: Hydrozoa) from the Porcupine Seabight: do north-east Atlantic carbonate mound fauna have Mediterranean ancestors? Mar. Biodivers. Rec. 1:e24. doi: 10.1017/S1755267206002302
Henry, L.-A., and Roberts, J. M. (2017). “Global biodiversity in cold-water coral reef ecosystems,” in Marine Animal Forests. The Ecology of Benthic Biodiversity Hotspots, eds S. Rossi, L. Bramanti, A. Gori, and C. Orejas (Switzerland: Springer International Publishing).
Hernández-Molina, F. J., Nombela, M. A., Van Rooij, D., Roson, G., Ercilla, G., Urgorri, V., et al. (2009). “The Ortegal spur contourite depositional dystem (Bay of Biscay): the implications of the Mediterranean Outflow Waters in sedimentary processes and cold-water coral ecosystems,” in Proceedings of the 6th Simposio sobre el Margen Ibérico Atlántico, Madrid, 281–284.
Hofmann, G. E., Barry, J. P., Edmunds, P. J., Gates, R. D., Hutchins, D. A., Klinger, T., et al. (2010). The effect of ocean acidification on calcifying organisms in marine ecosystems: an organism-to-ecosystem perspective. Annu. Rev. Ecol. Evol. Syst. 41, 127–147. doi: 10.1146/annurev.ecolsys.110308.120227
Hogg, M. M., Tendal, O. S., Conway, K. W., Pomponi, S. A., van Soest, R. W. M., Gutt, J., et al. (2010). Deep-Sea Sponge Grounds: Reservoirs of Biodiversity. Cambridge, MA: UNEP-WCMC.
Hourigan, T. F., Etnoyer, P. J., and Cairns, S. D. (2017). The State of Deep-Sea Coral and Sponge Ecosystems of the United States. Silver Spring, MD: NOAA.
Howell, K. L., Piechaud, N., Downie, A. L., and Kenny, A. (2016). The distribution of deep-sea sponge aggregations in the North Atlantic and implications for their effective spatial management. Deep Res. Part I Oceanogr. Res. 115, 203–220. doi: 10.1016/j.dsr.2016.07.005
Huff, D. D., Yoklavich, M. M., Love, M. S., Watters, D. L., Chai, F., and Lindley, S. T. (2013). Environmental factors that influence the distribution, size, and biotic relationships of the Christmas tree coral Antipathes dendrochristos in the Southern California Bight. Mar. Ecol. Prog. Ser. 494, 159–177. doi: 10.3354/meps10591
Hughen, K. A., Overpeck, J. T., Lehman, S. J., Kashgarian, M., Southon, J., Peterson, L. C., et al. (1998). Deglacial changes in ocean circulation from an extended radiocarbon calibration. Nature 391, 65–68. doi: 10.1038/34150
Husson, B., Sarradin, P. M., Zeppilli, D., and Sarrazin, J. (2017). Picturing thermal niches and biomass of hydrothermal vent species. Deep Res. Part II Top. Stud. Oceanogr. 137, 6–25. doi: 10.1016/j.dsr2.2016.05.028
Huvenne, V. A. I., Tyler, P. A., Masson, D. G., Fisher, E. H., Hauton, C., Huhnerbach, V., et al. (2011). A picture on the wall: innovative mapping reveals cold-water coral refuge in submarine canyon. PLoS One 6:e28755. doi: 10.1371/journal.pone.0028755
IPCC (2013). Climate Change 2013: The Physical Science Basis. Contribution of Working Group I to the Fifth Assessment Report of the Intergovernmental Panel on Climate Change. Geneva: IPCC.
IPCC (2014). Climate Change 2014: Synthesis Report. Contribution of Working Groups, I., II and III to the Fifth Assessment Report of the Intergovernmental Panel on Climate Change. Geneva: IPCC.
Jackson, E. L., Davies, A. J., Howell, K. L., Kershaw, P. J., and Hall-Spencer, J. M. (2014). Future-proofing marine protected area networks for cold water coral reefs. ICES J. Mar. Sci. 9, 2621–2629. doi: 10.1093/icesjms/fsu099
Jochumsen, K., Moritz, M., Nunes, N., Quadfasel, D., Larsen, K. M. H., Hansen, B., et al. (2017). Revised transport estimates of the Denmark Strait overflow. J. Geophys. Res. Ocean. 122, 3434–3450. doi: 10.1002/2017JC012803
Johnson, D., Adelaide Ferreira, M., and Kenchington, E. (2018). Climate change is likely to severely limit the effectiveness of deep-sea ABMTs in the North Atlantic. Mar. Policy 87, 111–122. doi: 10.1016/j.marpol.2017.09.034
Johnson, D. E., and Kenchington, E. L. (2019). Should potential for climate change refugia be mainstreamed into the criteria for describing EBSAs? Conserv. Lett. 12:e12634. doi: 10.1111/conl.12634
Johnson, J., and Stevens, I. (2000). A fine resolution model of the eastern North Atlantic between the Azores, the Canary Islands and the Gibraltar Strait. Deep Res. Part I Oceanogr. Res. 47, 875–899. doi: 10.1016/S0967-0637(99)00073-4
Jones, C. G., Lawton, J. H., and Shachak, M. (1994). Organisms as ecosystem engineers. Oikos 69, 373–386. doi: 10.2307/3545850
Jones, D. O. B., Yool, A., Wei, C.-L., Henson, S. A., Ruhl, H. A., Watson, R. A., et al. (2014). Global reductions in seafloor biomass in response to climate change. Glob. Chang. Biol. 20, 1861–1872. doi: 10.1111/gcb.12480
Jong, M. F., and Steur, L. (2016). Strong winter cooling over the Irminger Sea in winter 2014–2015, exceptional deep convection, and the emergence of anomalously low SST. Geophys. Res. Lett. 43, 7106–7113. doi: 10.1002/2016GL069596
Kamykowski, D., and Zentara, S.-J. (1990). Hypoxia in the world ocean as recorded in the historical data set. Deep Sea Res. Part A. Oceanogr. Res. Pap. 37, 1861–1874. doi: 10.1016/0198-0149(90)90082-7
Kazanidis, G., Vad, J., Henry, L.-A., Neat, F., Berx, B., Georgoulas, K., et al. (2019). Distribution of deep-sea sponge aggregations in an area of multisectoral activities and changing oceanic conditions. Front. Mar. Sci. 6:163. doi: 10.3389/fmars.2019.00163
Keeling, R. F., Körtzinger, A., and Gruber, N. (2010). Ocean Deoxygenation in a Warming World. Ann. Rev. Mar. Sci. 2, 199–229. doi: 10.1146/annurev.marine.010908.163855
Keller, N. B., and Os’kina, N. S. (2008). Habitat temperature ranges of azooxantellate scleractinian corals in the World Ocean. Oceanology 48, 77–84. doi: 10.1007/s11491-008-1009-3
Kenchington, E., Murillo, F. J., Lirette, C., Sacau, M., Koen-Alonso, M., Kenny, A., et al. (2014). Kernel density surface modelling as a means to identify significant concentrations of vulnerable marine ecosystem indicators. PLoS One 9:e109365. doi: 10.1371/journal.pone.0109365
Kenchington, E., Yashayaev, I., Tendal, O. S., and Jørgensbye, H. (2017). Water mass characteristics and associated fauna of a recently discovered Lophelia pertusa (Scleractinia: Anthozoa) reef in Greenlandic waters. Polar Biol. 40, 321–337. doi: 10.1007/s00300-016-1957-3
Kendall, V. J., and Haedrich, R. L. (2006). Species richness in Atlantic deep-sea fishes assessed in terms of the mid-domain effect and Rapoport’s rule. Deep Sea Res. Part I Oceanogr. Res. Pap. 53, 506–515. doi: 10.1016/j.dsr.2005.12.005
Khripounoff, A., Caprais, J. C., Le Bruchec, J., Rodier, P., Noel, P., and Cathalot, C. (2014). Deep cold-water coral ecosystems in the Brittany submarine canyons (Northeast Atlantic): hydrodynamics, particle supply, respiration, and carbon cycling. Limnol. Oceanogr. 59, 87–98. doi: 10.4319/lo.2014.59.1.0087
Klitgaard, A. B., and Tendal, O. S. (2004). Distribution and species composition of mass occurrences of large-sized sponges in the northeast Atlantic. Prog. Oceanogr. 61, 57–98. doi: 10.1016/j.physc.2004.06.002
Klitgaard, A. B., Tendal, O. S., and Westerberg, H. (1997). “Mass occurrences of large sponges (Porifera) in Faroe Island (NE Atlantic) shelf and slope areas: characteristics, distribution and possible causes,” in Proceedings of the 30th European Marine Biology Symposium, ed. L. E. Hawkins (Southampton: Southampton Ocenography Centre), 129–142.
Knudby, A., Kenchington, E., and Murillo, F. J. (2013). Modeling the distribution of Geodia sponges and sponge grounds in the Northwest Atlantic. PLoS One 8:e82306. doi: 10.1371/journal.pone.0082306
Kurihara, H. (2008). Effects of CO2-driven ocean acidification on the early developmental stages of invertebrates. Mar. Ecol. Prog. Ser. 373, 275–284. doi: 10.3354/meps07802
Kurman, M. D., Gómez, C. E., Georgian, S. E., Lunden, J. J., and Cordes, E. E. (2017). Intra-specific variation reveals potential for adaptation to ocean acidification in a cold-water coral from the Gulf of Mexico. Front. Mar. Sci. 4:111. doi: 10.3389/fmars.2017.00111
Lacharité, M., and Metaxas, A. (2018). Environmental drivers of epibenthic megafauna on a deep temperate continental shelf: a multiscale approach. Prog. Oceanogr. 162, 171–186. doi: 10.1016/j.pocean.2018.03.002
Lambshead, P. J., Tietjen, J., Ferrero, T., and Jensenf, P. (2000). Latitudinal gradients in the deep sea with special reference to North Atlantic nematodes. Mar. Ecol. Prog. Ser. 194, 159–167. doi: 10.3354/meps194159
Lavelle, J. W., and Mohn, C. (2010). Motion, commotion, and biophysical connections at deep ocean seamounts. Oceanography 23, 90–103. doi: 10.5670/oceanog.2010.64
Lebrato, M., Iglesias-Rodríguez, D., Feely, R. A., Greeley, D., Jones, D. O. B., Suarez-Bosche, N., et al. (2010). Global contribution of echinoderms to the marine carbon cycle: CaCO3 budget and benthic compartments. Ecol. Monogr. 80, 441–467. doi: 10.1890/09-0553.1
Levin, L. A. (2003). Oxygen minimum zone benthos: adaptation and community response to hypoxia. Oceanogr. Mar. Biol. an Annu. Rev. 41, 1–45.
Levin, L. A. (2018). Manifestation, drivers, and emergence of open ocean deoxygenation. Ann. Rev. Mar. Sci. 10, 229–260. doi: 10.1146/annurev-marine-121916-163359
Levin, L. A., Baco, A. R., Bowden, D. A., Colaco, A., Cordes, E. E., Cunha, M. R., et al. (2016). Hydrothermal vents and methane seeps: rethinking the sphere of influence. Front. Mar. Sci. 3:72. doi: 10.3389/fmars.2016.00072
Levin, L. A., Bett, B. J., Gates, A. R., Heimbach, P., Howe, B. M., Janssen, F., et al. (2019). Global observing needs in the Deep Ocean. Front. Mar. Sci. 6:241. doi: 10.3389/fmars.2019.00241
Levin, L. A., Etter, R. J., Rex, M. A., Gooday, A. J., Smith, C. R., Pineda, C., et al. (2001). Environmental influence on regional deep-sea species diversity. Annu. Rev. Ecol. Syst. 32, 51–93.
Levin, L. A., and Gage, J. D. (1998). Relationships between oxygen, organic matter and the diversity of bathyal macrofauna. Deep Res. Part II Top. Stud. Oceanogr. 45, 129–163. doi: 10.1016/S0967-0645(97)00085-4
Levin, L. A., and Le Bris, N. L. (2015). The deep ocean under climate change. Science 350, 766–768. doi: 10.1126/science.aad0126
Levin, L. A., and Sibuet, M. (2012). Understanding continental margin biodiversity: a new imperative. Ann. Rev. Mar. Sci. 4, 79–112. doi: 10.1146/annurev-marine-120709-142714
Levitus, S., Antonov, J. I., Boyer, T. P., Baranova, O. K., Garcia, H. E., Locarnini, R. A., et al. (2012). World ocean heat content and thermosteric sea level change (0-2000 m), 1955-2010. Geophys. Res. Lett. 39, 1–5. doi: 10.1029/2012GL051106
Lippold, J., Grützner, J., Winter, D., Lahaye, Y., Mangini, A., and Christi, M. (2009). Does sedimentary 231Pa/230Th from the bermuda rise monitor past atlantic meridional overturning circulation? Geophys. Res. Lett. 36, 1–6. doi: 10.1029/2009GL038068
Liu, M., and Tanhua, T. (2019). Characteristics of water masses in the Atlantic Ocean based on GLODAPv2 data. Ocean Sci. Discuss doi: 10.5194/os-2018-139
Liu, W., Fedorov, A., and Sévellec, F. (2018). The mechanisms of the Atlantic meridional overturning circulation slowdown induced by Arctic Sea ice decline. J. Clim. 32, 977–996. doi: 10.1175/JCLI-D-18-0231.1
Lozier, M. S., Li, F., Bacon, S., Bahr, F., Bower, A. S., Cunningham, S. A., et al. (2019). A sea change in our view of overturning in the subpolar North Atlantic. Science 363, 516–521. doi: 10.1126/science.aau6592
Lunden, J. J., McNicholl, C. G., Sears, C. R., Morrison, C. L., and Cordes, E. E. (2014). Acute survivorship of the deep-sea coral Lophelia pertusa from the Gulf of Mexico under acidification, warming, and deoxygenation. Front. Mar. Sci. 1:78. doi: 10.3389/fmars.2014.00078
Lundsten, L., Schlining, K. L., Frasier, K., Johnson, S. B., Kuhnz, L. A., Harvey, J. B. J., et al. (2010). Time-series analysis of six whale-fall communities in Monterey Canyon, California, USA. Deep Res. Part I Oceanogr. Res. 57, 1573–1584. doi: 10.1016/j.dsr.2010.09.003
Lynch-Stieglitz, J., Adkins, J. F., Curry, W. B., Dokken, T., Hall, I. R., Herguera, J. C., et al. (2007). Atlantic meridional overturning circulation during the last glacial maximum. Science 316, 66–70. doi: 10.1126/science.1137127
Maier, C., Hegeman, J., Weinbauer, M. G., and Gattuso, J. P. (2009). Calcification of the cold-water coral Lophelia pertusa under ambient and reduced pH. Biogeosciences 6, 1671–1680. doi: 10.1098/rspb.2011.1763
Maier, C., Popp, P., Sollfrank, N., Weinbauer, M. G., Wild, C., and Gattuso, J. P. (2016). Effects of elevated pCO2 and feeding on net calcification and energy budget of the Mediterranean cold-water coral Madrepora oculata. J. Exp. Biol. 219, 3208–3217. doi: 10.1242/jeb.127159
Maier, C., Schubert, A., Sanchez, M. M. B., Weinbauer, M. G., Watremez, P., and Gattuso, J.-P. (2013). End of the century pCO2 levels do not impact calcification in Mediterranean cold-water corals. PLoS One 8:e62655. doi: 10.1371/journal.pone.0062655
Maier, C., Weinbauer, M. G., and Gattuso, J.-P. (2019). “Fate of Mediterranean Scleractinian cold-water corals as a result of global climate change. a synthesis,” in Mediterranean cold-water corals: Past, present and future - Unerstanding the deep-sea realm of corals, eds C. Orejas and C. Jiménez (Switzerland: Springer International Publishing), 517–530.
Maldonado, M., Aguilar, R., Bannister, R. J., Bell, J. J., Conway, K. W., Dayton, P. K., et al. (2017). “Sponge grounds as key marine habitats: a synthetic review of types, structure, functional roles and conservation concerns,” in Marine Animal Forests. The Ecology of Benthic Biodiversity Hotspots, eds S. Rossi, L. Bramanti, A. Gori, and C. Orejas (Switzerland: Springer International Publishing), 1–39. doi: 10.1007/978-3-319-17001-5_24-1
Maldonado, M., López-Acosta, M., Sitjà, C., García-Puig, M., Galobart, C., Ercilla, G., et al. (2019). Sponge skeletons as an important sink of silicon in the global oceans. Nat. Geosci. 12, 815–822. doi: 10.1038/s41561-019-0430-7
Martin, J. H., Knauer, G. A., Karl, D. M., and Broenkow, W. W. (1987). VERTEX: carbon cycling in the northeast Pacific. Deep Sea Res. Part A. Oceanogr. Res. Pap. 34, 267–285. doi: 10.1016/0198-0149(87)90086-0
Matos, L., Mienis, F., Wienberg, C., Frank, N., Kwiatkowski, C., Groeneveld, J., et al. (2015). Interglacial occurrence of cold-water corals off cape lookout (NW Atlantic): first evidence of the gulf stream influence. Deep Sea Res. Part I Oceanogr. Res. Pap. 105, 158–170. doi: 10.1016/j.dsr.2015.09.003
Matos, L., Wienberg, C., Titschack, J., Schmiedl, G., Frank, N., Abrantes, F., et al. (2017). Coral mound development at the Campeche cold-water coral province, southern Gulf of Mexico: implications of Antarctic Intermediate Water increased influence during interglacials. Mar. Geol. 392, 53–65. doi: 10.1016/j.margeo.2017.08.012
McCartney, M. (1992). Recirculating components to the deep boundary current of the North Atlantic. Prog. Oceanogr. 29, 283–383. doi: 10.1016/0079-6611(92)90006-l
McClain, C. R. (2007). Seamounts: identity crisis or split personality? J. Biogeogr. 34, 2001–2008. doi: 10.1111/j.1365-2699.2007.01783.x
McClain, C. R., and Barry, J. P. (2010). Habitat heterogeneity, disturbance, and productivity work in concert to regulate biodiversity in deep submarine canyons. Ecology 91, 964–976. doi: 10.1890/09-0087.1
McClain, C. R., and Hardy, S. M. (2010). The dynamics of biogeographic ranges in the deep sea. Proc. R. Soc. London B Biol. Sci. 277, 3533–3546. doi: 10.1098/rspb.2010.1057
McCulloch, M., Falter, J., Trotter, J., and Montagna, P. (2012a). Coral resilience to ocean acidification and global warming through pH up-regulation. Nat. Clim. Chang. 2, 623–627. doi: 10.1038/srep42405
McCulloch, M., Trotter, J., Montagna, P., Falter, J., Dunbar, R., Freiwald, A. A., et al. (2012b). Resilience of cold-water scleractinian corals to ocean acidification: boron isotopic systematics of pH and saturation state up-regulation. Geochim. Cosmochim. Acta 87, 21–34. doi: 10.1016/j.gca.2012.03.027
McIntyre, F. D., Drewery, J., Eerkes-Medrano, D., and Neat, F. C. (2016). Distribution and diversity of deep-sea sponge grounds on the Rosemary Bank Seamount, NE Atlantic. Mar. Biol. 163, 1–11. doi: 10.1007/s00227-016-2913-z
Mienis, F., de Stigter, H. C., de Haas, H., and van Weering, T. C. E. (2009). Near-bed particle deposition and resuspension in a cold-water coral mound area at the Southwest Rockall Trough margin, NE Atlantic. Deep Res. Part I Oceanogr. Res. 56, 1026–1038. doi: 10.1016/j.dsr.2009.01.006
Mienis, F., de Stigter, H. C., White, M., Dulneveldc, G., de Haas, H., and van Weering, T. C. E. (2007). Hydrodynamic controls on cold-water coral growth and carbonate-mound development at the SW and SE Rockall Trough margin, NE Atlantic ocean. Deep Res. Part I Oceanogr. Res. 54, 1655–1674. doi: 10.1016/j.dsr.2007.05.013
Mienis, F., Duineveld, G. C. A., Davies, A. J., Lavaleye, M. M. S., Ross, S. W., Seim, H., et al. (2014). Cold-water coral growth under extreme environmental conditions, the Cape Lookout area, NW Atlantic. Biogeosciences 11, 2543–2560. doi: 10.5194/bg-11-2543-2014
Mienis, F., Duineveld, G. C. A., Davies, A. J., Ross, S. W., Seim, H., Bane, J., et al. (2012a). Cold-water coral growth under extreme environmental conditions, the Cape Lookout area, NW Atlantic. Deep Res. Part I Oceanogr. Res. 60, 32–45. doi: 10.1016/j.dsr.2011.10.007
Mienis, F., Duineveld, G. C. A., Davies, A. J., Ross, S. W., Seim, H., Bane, J., et al. (2012b). The influence of near-bed hydrodynamic conditions on cold-water corals in the Viosca Knoll area, Gulf of Mexico. Deep Res. Part I Oceanogr. Res. 60, 32–45. doi: 10.1016/j.dsr.2011.10.007
Moalic, Y., Desbruyères, D., Duarte, C. M., Rozenfeld, A. F., Bachraty, C., and Arnaud-Haond, S. (2012). Biogeography revisited with network theory: retracing the history of hydrothermal vent communities. Syst. Biol. 61, 127–137. doi: 10.1093/sysbio/syr088
Mohn, C., Rengstorf, A., White, M., Duineveld, G., Mienis, F., Soetaert, K., et al. (2014). Linking benthic hydrodynamics and cold-water coral occurrences: a high-resolution model study at three cold-water coral provinces in the NE Atlantic. Prog. Oceanogr. 122, 92–104. doi: 10.1016/j.pocean.2013.12.003
Montero-Serrano, J.-C., Frank, N., Colin, C., Wienberg, C., and Eisele, M. (2011). The climate influence on the mid-depth Northeast Atlantic gyres viewed by cold-water corals. Geophys. Res. Lett. 38:L19604. doi: 10.1029/2011gl048733
Mora, C., Wei, C.-L., Rollo, A., Amaro, T., Baco, A. R., Billett, D., et al. (2013). Biotic and human vulnerability to projected changes in ocean biogeochemistry over the 21st Century. PLoS Biol. 11:e1001682. doi: 10.1371/journal.pbio.1001682
Morato, T., Gonzaìlez-Irusta, J. M., Dominguez-Carrioì, C., Wei, C.-L., Davies, A. A., Sweetman, A. K., et al. (2020). Climate-induced changes in the habitat suitability of cold-water corals and commercially important deep-sea fish in the North Atlantic. Glob. Chang. Biol. 26, 2181–2202. doi: 10.1111/gcb.14996
Morato, T., Kvile, K., Taranto, G. H., Tempera, F., Narayanaswamy, B. E., Hebbeln, D., et al. (2013). Seamount physiography and biology in the north-east Atlantic and Mediterranean Sea. Biogeosciences 10, 3039–3054. doi: 10.5194/bg-10-3039-2013
Morrow, K. M., Bourne, D. G., Humphrey, C., Botté, E. S., Laffy, P., Zaneveld, J., et al. (2015). Natural volcanic CO2 seeps reveal future trajectories for host–microbial associations in corals and sponges. ISME J. 9, 894–908. doi: 10.1038/ismej.2014.188
Mortensen, P. B., and Buhl-Mortensen, L. (2004). Distribution of deep-water gorgonian corals in relation to benthic habitat features in the Northeast Channel (Atlantic Canada). Mar. Biol. 144, 1223–1238. doi: 10.1007/s00227-003-1280-8
Mortensen, P. B., Hovland, T., Fossa, J. H., Furevik, D. M., Fosså, J. H., and Furevik, D. M. (2001). Distribution, abundance and size of Lophelia pertusa coral reefs in mid-Norway in relation to seabed characteristics. J. Mar. Biol. Assoc. United Kingdom 81, 581–597. doi: 10.1017/S002531540100426X
Mulder, T., Zaragosi, S., Garlan, T., Mavel, J., Cremer, M., Sottolichio, A., et al. (2012). Present deep-submarine canyons activity in the Bay of Biscay (NE Atlantic). Mar. Geol. 295–298, 113–127. doi: 10.1016/j.margeo.2011.12.005
Murillo, F., Kenchington, E., Tompkins, G., Beazley, L., Baker, E., Knudby, A., et al. (2018). Sponge assemblages and predicted archetypes in the eastern Canadian Arctic. Mar. Ecol. Prog. Ser. 597, 115–135. doi: 10.3354/meps12589
Murillo, F. J., Kenchington, E., Lawson, J. M., Li, G., and Piper, D. J. W. (2016a). Ancient deep-sea sponge grounds on the Flemish Cap and Grand Bank, northwest Atlantic. Mar. Biol. 163, 1–11. doi: 10.1007/s00227-016-2839-5
Murillo, F. J., Muñoz, P. D., Cristobo, J., Ríos, P., González, C., Kenchington, E., et al. (2012). Deep-sea sponge grounds of the flemish cap, flemish pass and the Grand Banks of Newfoundland (Northwest Atlantic Ocean): distribution and species composition. Mar. Biol. Res. 8, 842–854. doi: 10.1080/17451000.2012.682583
Murillo, F. J., Serrano, A., Kenchington, E., and Mora, J. (2016b). Epibenthic assemblages of the Tail of the Grand Bank and flemish cap (northwest Atlantic) in relation to environmental parameters and trawling intensity. Deep Res. Part I Oceanogr. Res. 109, 99–122. doi: 10.1016/j.dsr.2015.08.006
NAFO (2018). Report of the 11th Meeting of the NAFO Scientific Council Working Group on Ecosystem Science and Assessment (WG-ESA, NAFO SCS Doc. 18/23 Serial No. N6900). Dartmouth: NAFO.
Naumann, M. S., Orejas, C., and Ferrier-Pagès, C. (2013). High thermal tolerance of two Mediterranean cold-water coral species maintained in aquaria. Coral Reefs 32, 749–754. doi: 10.1007/s00338-013-1011-7
Naumann, M. S., Orejas, C., and Ferrier-Pagès, C. (2014). Species-specific physiological response by the cold-water corals Lophelia pertusa and Madrepora oculata to variations within their natural temperature range. Deep Res. Part II Top. Stud. Oceanogr. 99, 36–41. doi: 10.1016/j.dsr2.2013.05.025
Needler, G. T., and Heath, R. A. (1975). Diffusion coefficients calculated from the Mediterranean salinity anomaly in the North Atlantic Ocean. J. Phys. Oceanogr. 5, 173–182. doi: 10.1175/1520-0485(1975)005<0173:dccftm>2.0.co;2
New, A. L., and Smythe-Wright, D. (2001). Aspects of the circulation in the Rockall Trough. Cont. Shelf Res. 21, 777–810. doi: 10.1016/S0278-4343(00)00113-8
Obelcz, J., Brothers, D., Chaytor, J., Brink, U., ten Ross, S. W., and Brooke, S. (2014). Geomorphic characterization of four shelf-sourced submarine canyons along the U.S. Mid-Atlantic continental margin. Deep Res. Part II Top. Stud. Oceanogr. 104, 106–119. doi: 10.1016/j.dsr2.2013.09.013
O’Hara, T. D., and Tittensor, D. P. (2010). Environmental drivers of ophiuroid species richness on seamounts. Mar. Ecol. 31, 26–38. doi: 10.1111/j.1439-0485.2010.00373.x
Olabarria, C., Sanders, H. L., Allen, J. A., and Sanders, H. L. (2005). Patterns of bathymetric zonation of bivalves in the Porcupine Seabight and adjacent abyssal plain, NE Atlantic. Deep Res. Part I Oceanogr. Res. 52, 15–31. doi: 10.1016/j.dsr.2004.09.005
Orejas, C., Gori, A., Rad-menéndez, C., Last, K. S., Davies, A. J., Beveridge, C. M., et al. (2016). The effect of flow speed and food size on the capture efficiency and feeding behaviour of the cold-water coral Lophelia pertusa. J. Exp. Mar. Bio. Ecol. 481, 34–40. doi: 10.1016/j.jembe.2016.04.002
Orr, J. C., Fabry, V. J., Aumont, O., Bopp, L., Doney, S. C., Feely, R. A., et al. (2005). Anthropogenic ocean acidification over the twenty-first century and its impact on calcifying organisms. Nature 437, 681–686. doi: 10.1038/nature04095
Osman, M. B., Das, S. B., Trusel, L. D., Evans, M. J., Fischer, H., Grieman, M. M., et al. (2019). Industrial-era decline in subarctic Atlantic productivity. Nature 569, 551–555. doi: 10.1038/s41586-019-1181-8
OSPAR (2008). Descriptions of Habitats on the OSPAR List of Threatened and/or Declining Species and Habitats. London: OSPAR.
Palanques, A., Durrieu de Madron, X., Puig, P., Fabres, J., Guillén, J., Calafat, A., et al. (2006). Suspended sediment fluxes and transport processes in the Gulf of Lions submarine canyons. The role of storms and dense water cascading. Mar. Geol. 234, 43–61. doi: 10.1016/j.margeo.2006.09.002
Palomino, D., López-González, N., Vázquez, J.-T., Fernández-Salas, L. M., Rueda, J. L., Sánchez-Leal, R., et al. (2016). Multidisciplinary study of mud volcanoes and diapirs and their relationship to seepages and bottom currents in the Gulf of Cádiz continental slope (northeastern sector). Mar. Geol. 378, 196–212. doi: 10.1016/j.margeo.2015.10.001
Pape, E., Bezerra, T. N., Jones, D. O. B. B., and Vanreusel, A. (2013). Unravelling the environmental drivers of deep-sea nematode biodiversity and its relation with carbon mineralisation along a longitudinal primary productivity gradient. Biogeosciences 10, 3127–3143. doi: 10.5194/bg-10-3127-2013
Pechenik, J. A. (1999). On the advantages and disadvantages of larval stages in benthic marine invertebrate life cycles. Mar. Ecol. Prog. Ser. 177, 269–297. doi: 10.3354/meps177269
Perez, F. F., Fontela, M., García-Ibáñez, M. I., Mercier, H., Velo, A., Lherminier, P., et al. (2018). Meridional overturning circulation conveys fast acidification to the deep Atlantic Ocean. Nature 554, 515–518. doi: 10.1038/nature25493
Petrou, K., Baker, K. G., Nielsen, D. A., Hancock, A. M., Schulz, K. G., and Davidson, A. T. (2019). Acidification diminishes diatom silica production in the Southern Ocean. Nat. Clim. Chang. 9, 781–786. doi: 10.1038/s41558-019-0557-y
Pollard, R. T., Grifftths, M. J., Cunningham, S. A., Read, J. F., Pérez, F. F., and Ríos, A. F. (1996). Vivaldi 1991 - A study of the formation, circulation and ventilation of Eastern North Atlantic Central Water. Prog. Oceanogr. 37, 167–192. doi: 10.1016/S0079-6611(96)00008-0
Pollard, R. T., Read, J. F., Holliday, N. P., and Leach, H. (2004). Water masses and circulation pathways through the Iceland Basin during Vivaldi 1996. J. Geophys. Res. Ocean. 109:C04004. doi: 10.1029/2003JC002067
Pomar, L., Morsilli, M., Hallock, P., and Bádenas, B. (2012). Internal waves, an under-explored source of turbulence events in the sedimentary record. Earth Sci. Rev. 111, 56–81. doi: 10.1016/j.earscirev.2011.12.005
Pörtner, H. O., and Farrell, A. P. (2008). Physiology and climate change. Science 322, 690–692. doi: 10.1126/science.1163156
Price, A. R. G., Keeling, M. J., and O’Callghan, C. J. (1999). Ocean-scale patterns of ‘biodiversity’ of Atlantic asteroids determined from taxonomic distinctness and other measures. Biol. J. Linn. Soc. 66, 187–203. doi: 10.1111/j.1095-8312.1999.tb01883.x
Purkey, S. G., and Johnson, G. C. (2010). Warming of global abyssal and deep Southern Ocean waters between the 1990s and 2000s: contributions to global heat and sea level rise budgets. J. Clim. 23, 6336–6351. doi: 10.1175/2010JCLI3682.1
Purser, A., Larsson, A. I., Thomsen, L., and van Oevelen, D. (2010). The influence of flow velocity and food concentration on Lophelia pertusa (Scleractinia) zooplankton capture rates. J. Exp. Mar. Bio. Ecol. 395, 55–62. doi: 10.1016/j.jembe.2010.08.013
Purser, A., Orejas, C., Gori, A., Unnithan, V., and Thomsen, L. (2013). Local variation in the distribution of benthic megafauna species associated with cold-water coral reefs on the Norwegian margin. Cont. Shelf Res. 54, 37–51. doi: 10.1016/j.csr.2012.12.013
Pusceddu, A., Carugati, L., Gambi, C., Mienert, J., Petani, B., Sanchez-Vidal, A., et al. (2016). Organic matter pools, C turnover and meiofaunal biodiversity in the sediments of the western Spitsbergen deep continental margin, Svalbard Archipelago. Deep Res. Part I Oceanogr. Res. 107, 48–58. doi: 10.1016/j.dsr.2015.11.004
Raddatz, J., Rüggeberg, A., Liebetrau, V., Foubert, A., Hathorne, E. C., Fietzke, J., et al. (2014). Environmental boundary conditions of cold-water coral mound growth over the last 3 million years in the Porcupine Seabight, Northeast Atlantic. Deep Sea Res. Part II Top. Stud. Oceanogr. 99, 227–236. doi: 10.1016/j.dsr2.2013.06.009
Radice, V. Z., Quattrini, A. M., Wareham, V. E., Edinger, E. N., and Cordes, E. E. (2016). Vertical water mass structure in the North Atlantic influences the bathymetric distribution of species in the deep-sea coral genus Paramuricea. Deep Res. Part I Oceanogr. Res. 116, 253–263. doi: 10.1016/j.dsr.2016.08.014
Ragueneau, O., Tréguer, P., Leynaert, A., Anderson, R. F., Brzezinski, M. A., DeMaster, D. J., et al. (2000). A review of the Si cycle in the modern ocean: recent progress and missing gaps in the application of biogenic opal as a paleoproductivity proxy. Glob. Planet. Change 26, 317–365. doi: 10.1594/PANGAEA.787476
Rahmstorf, S., Box, J. E., Feulner, G., Mann, M. E., Robinson, A., Rutherford, S., et al. (2015). Exceptional twentieth-century slowdown in Atlantic Ocean overturning circulation. Nat. Clim. Chang. 5:475. doi: 10.1038/nclimate2554
Ramalho, L. V., López-Fé, C. M., and Rueda, J. L. (2018). Three species of Reteporella (Bryozoa: Cheilostomata) in a diapiric and mud volcano field of the Gulf of Cádiz, with the description of Reteporella victori n. sp. Zootaxa 4375, 90–104. doi: 10.11646/zootaxa.4375.1.4
Ramirez-Llodra, E., Brandt, A., Danovaro, R., De Mol, B., Escobar, E., German, C. R., et al. (2010). Deep, diverse and definitely different: unique attributes of the world’s largest ecosystem. Biogeosciences 7, 2851–2899. doi: 10.5194/bg-7-2851-2010
Ramirez-Llodra, E., Ballesteros, M., Company, J. B., Dantart, L., and Sarda, F. (2007a). Spatio-temporal variations of biomass and abundance in bathyal non-crustacean megafauna in the Catalan Sea (North-western Mediterranean). Mar. Biol. 153, 297–309. doi: 10.1007/s00227-007-0805-y
Ramirez-Llodra, E., Shank, T., and German, C. (2007b). Biodiversity and biogeography of hydrothermal vent species: thirty years of discovery and investigations. Oceanography 20, 30–41. doi: 10.5670/oceanog.2007.78
Raybaud, V., Tambutté, S., Ferrier-Pagès, C., Reynaud, S., Venn, A. A., and Tambutté, É, et al. (2017). Computing the carbonate chemistry of the coral calcifying medium and its response to ocean acidification. J. Theor. Biol. 424, 26–36. doi: 10.1016/j.jtbi.2017.04.028
Read, J. F. (2000). CONVEX-91: water masses and circulation of the Northeast Atlantic subpolar gyre. Prog. Oceanogr. 48, 461–510. doi: 10.1016/S0079-6611(01)00011-8
Reed, J. K., Weaver, D. C., and Pomponi, S. A. (2006). Habitat and fauna of deep-water Lophelia pertusa coral reefs off the southeastern US: blake Plateau, Straits of Florida, and Gulf of Mexico. Bull. Mar. Sci. 78, 343–375.
Reichart, G. J., Lourens, L. J., and Zachariasse, W. J. (1998). Temporal variability in the nort hern Arabian Sea oxygen minimum zone (OMZ) during the last 225,000 years. Paleoceanography 13, 607–621. doi: 10.1029/98PA02203
Rex, M. A., Crame, J. A., Stuart, C. T., and Clarke, A. (2005). Large-scale biogeographic patterns in marine mollusks: a confluence of history and productivity? Ecology 86, 2288–2297. doi: 10.1890/04-1056
Rex, M. A., Etter, R. J., Morris, J. S., Crouse, J., McClain, C. R., Johnson, N. A., et al. (2006). Global bathymetric patterns of standing stock and body size in the deep-sea benthos. Mar. Ecol. Prog. Ser. 317, 1–8. doi: 10.3354/meps317001
Rex, M. A., Stuart, C. T., Hessler, R. R., Allen, J. A., Sanders, H. L., and Wilson, G. D. F. (1993). Global-scale latitudinal patterns of species diversity in the deep-sea benthos. Nature 365, 636–639. doi: 10.1038/365636a0
Rheuban, J. E., Doney, S. C., Cooley, S. R., and Hart, D. R. (2018). Projected impacts of future climate change, ocean acidification, and management on the US Atlantic sea scallop (Placopecten magellanicus) fishery. PLoS One 13:e0203536. doi: 10.1371/journal.pone.0203536
Riahi, K., Rao, S., Krey, V., Cho, C., Chirkov, V., Fischer, G., et al. (2011). RCP 8.5—A scenario of comparatively high greenhouse gas emissions. Clim. Change 109:33. doi: 10.1007/s10584-011-0149-y
Rice, A. L., Thurston, M. H., and New, A. L. (1990). Dense aggregations of a hexactinellid sponge, Pheronema carpenteri, in the porcupine seabight (northeast Atlantic Ocean), and possible causes. Prog. Oceanogr. 24, 179–196. doi: 10.1016/0079-6611(90)90029-2
Ries, J. B. (2010). Review: Geological and experimental evidence for secular variation in seawater Mg/Ca (calcite-aragonite seas) and its effects on marine biological calcification. Biogeosciences 7, 2795–2849. doi: 10.5194/bg-7-2795-2010
Ries, J. B., Cohen, A. L., and McCorkle, D. C. (2009). Marine calcifiers exhibit mixed responses to CO2-induced ocean acidification. Geology 37, 1131–1134. doi: 10.1130/g30210a.1
Ríos, A. F., Pérez, F. F., and Fraga, F. (1992). Water masses in the upper and middle North Atlantic Ocean east of the Azores. Deep Res. Part I Oceanogr. Res. 39, 645–658. doi: 10.1016/0198-0149(92)90093-9
Ritz, S. P., Stocker, T. F., Grimalt, J. O., Menviel, L., and Timmermann, A. (2013). Estimated strength of the Atlantic overturning circulation during the last deglaciation. Nat. Geosci. 6:208. doi: 10.1038/ngeo1723
Rix, L., De Goeij, J. M., Mueller, C. E., Struck, U., Middelburg, J. J., Van Duyl, F. C., et al. (2016). Coral mucus fuels the sponge loop in warm-and cold-water coral reef ecosystems. Sci. Rep. 6, 1–11. doi: 10.1038/srep18715
Roark, E. B., Guilderson, T. P., Dunbar, R. B., Fallon, S. J., and Mucciarone, D. A. (2009). Extreme longevity in proteinaceous deep-sea corals. Proc. Natl. Acad. Sci. U.S.A. 106, 5204–5208. doi: 10.1073/pnas.0810875106
Roberts, E. M., Mienis, F., Rapp, H. T., Hanz, U., Meyer, H. K., and Davies, A. J. (2018). Oceanographic setting and short-timescale environmental variability at an Arctic seamount sponge ground. Deep Sea Res. Part I Oceanogr. Res. Pap. 138, 98–113. doi: 10.1016/j.dsr.2018.06.007
Roberts, J. M., Roberts, J. M., Wheeler, A. J., and Freiwald, A. (2006). Reefs of the deep: The biology and geology of cold-water coral ecosystems. Science 312, 543–548. doi: 10.1126/science.1119861
Roberts, J. M., Davies, A. J., Henry, L. A., Dodds, L. A., Duineveld, G. C. A. A., Lavaleye, M. S. S. S., et al. (2009a). Mingulay reef complex: an interdisciplinary study of cold-water coral habitat, hydrography and biodiversity. Mar. Ecol. Prog. Ser. 397, 139–151. doi: 10.3354/meps08112
Roberts, J. M., Wheeler, A., Freiwald, A., and Cairns, S. (2009b). Cold-Water Corals: The Biology and Geology of Deep-Sea Coral Habitats. Cambridge, MA: Cambridge University Press.
Rodolfo-Metalpa, R., Houlbrèque, F., Tambutté, É, Boisson, F., Baggini, C., Patti, F. P., et al. (2011). Coral and mollusc resistance to ocean acidification adversely affected by warming. Nat. Clim. Chang. 1, 308–312. doi: 10.1038/nclimate1200
Rodrigues, C. F., Hilário, A., and Cunha, M. R. (2013). Chemosymbiotic species from the Gulf of Cadiz (NE Atlantic): distribution, life styles and nutritional patterns. Biogeosciences 10, 2569–2581. doi: 10.5194/bg-10-2569-2013
Rogers, A. D. (2000). The role of the oceanic oxygen minima in generating biodiversity in the deep sea. Deep Res. Part II Top. Stud. Oceanogr. 47, 119–148. doi: 10.1016/S0967-0645(99)00107-1
Rogerson, M., Rohling, E. J., Weaver, P., and Rogerson, C. (2006). Promotion of meridional overturning by Mediterranean-derived salt during the last deglaciation. Paleoceanography 21:A4101. doi: 10.1029/2006PA001306
Ross, S. W., and Quattrini, A. M. (2007). The fish fauna associated with deep coral banks off the southeastern United States. Deep Res. Part I Oceanogr. Res. 54, 975–1007. doi: 10.1016/j.dsr.2007.03.010
Rossi, S., Bramanti, L., Gori, A., and Orejas, C. (eds) (2017). Marine Animal Forest: The Ecology of Benthic Biodiversity Hotspots. Cham: Springer International Publishing.
Rossi, S., Isla, E., Bosch-Belmar, M., Galli, G., Gori, A., Gristina, M., et al. (2019). Changes of energy fluxes in marine animal forests of the Anthropocene: factors shaping the future seascape. ICES J. Mar. Sci. 76, 2008–2019. doi: 10.1093/icesjms/fsz147
Rowden, A. A., Dower, J. F., Schlacher, T. A., Consalvey, M., and Clark, M. R. (2010). Paradigms in seamount ecology: fact, fiction and future. Mar. Ecol. 31, 226–241. doi: 10.1111/j.1439-0485.2010.00400.x
Rowe, G. T., Gage, J. D., Tyler, P. A., and Rowe, G. T. (1992). Deep-Sea Biology: A Natural History of Organisms at the Deep-Sea Floor. Chicago, IL: University of Chicago Press.
Rueda, J. L., Gonzalez-Garcia, E., Krutzky, C., Lopez-Rodriguez, F. J., Bruque, G., Lopez-Gonzalez, N., et al. (2016). From chemosynthesis-based communities to cold-water corals: vulnerable deep-sea habitats of the Gulf of Cadiz. Mar. Biodivers. 46, 473–482. doi: 10.1007/s12526-015-0366-0
Ruggeberg, A., Dullo, C., Dorschel, B., and Hebbeln, D. (2007). Environmental changes and growth history of a cold-water carbonate mound (Propeller Mound, Porcupine Seabight). Int. J. Earth Sci. 96, 57–72. doi: 10.1007/s00531-005-0504-1
Ruiz-Villarreal, M., González-Pola, C., Diaz del Rio, G., Lavin, A., Otero, P., Piedracoba, S., et al. (2006). Oceanographic conditions in North and Northwest Iberia and their influence on the Prestige oil spill. Mar. Pollut. Bull. 53, 220–238. doi: 10.1016/j.marpolbul.2006.03.011
Sameoto, J. A., and Metaxas, A. (2008). Interactive effects of haloclines and food patches on the vertical distribution of 3 species of temperate invertebrate larvae. J. Exp. Mar. Bio. Ecol. 367, 131–141. doi: 10.1016/j.jembe.2008.09.003
Sampaio, Í, Freiwald, A., Porteiro, F. M., Menezes, G., and Carreiro-Silva, M. (2019). Census of octocorallia (cnidaria: Anthozoa) of the azores (NE atlantic) with a nomenclature update. Zootaxa 4550, 451–498. doi: 10.11646/zootaxa.4550.4.1
Sanders, H. L. (1968). Marine benthic diversity - A comparative study. Am. Nat. 102, 243–282. doi: 10.1086/282541
Sarafanov, A., Falina, A., Mercier, H., Sokov, A., Lherminier, P., Gourcuff, C., et al. (2012). Mean full-depth summer circulation and transports at the northern periphery of the Atlantic Ocean in the 2000s. J. Geophys. Res. Ocean. 117:C01014. doi: 10.1029/2011JC007572
Schmittner, A. (2005). Marine ecosystem decline caused by a reduction of the Atlantic Meridional Overturning (AMO) circulation. Lett. Nat. 434, 628–633. doi: 10.1038/nature03429.1
Schönberg, C. H. L., Fang, J. K. H., Carreiro-Silva, M., Tribollet, A., and Wisshak, M. (2017). Bioerosion: the other ocean acidification problem. ICES J. Mar. Sci. 74, 895–925. doi: 10.1093/icesjms/fsw254
Schönfeld, J., and Zahn, R. (2000). Late Glacial to Holocene history of the Mediterranean outflow. Evidence from benthic foraminiferal assemblages and stable isotopes at the Portuguese margin. Palaeogeogr. Palaeoclimatol. Palaeoecol. 159, 85–111. doi: 10.1016/S0031-0182(00)00035-3
Serrano, A., Cartes, J. E., Papiol, V., Punzón, A., García-Alegre, A., Arronte, J. C., et al. (2017a). Epibenthic communities of sedimentary habitats in a NE Atlantic deep seamount (Galicia Bank). J. Sea Res. 130, 154–165. doi: 10.1016/j.seares.2017.03.004
Serrano, A., González-Irusta, J. M., Punzón, A., García-Alegre, A., Lourido, A., Ríos, P., et al. (2017b). Deep-sea benthic habitats modeling and mapping in a NE Atlantic seamount (Galicia Bank). Deep Res. Part I Oceanogr. Res. 126, 115–127. doi: 10.1016/j.dsr.2017.06.003
Sherwood, O., Heikoop, J. M., Scott, D. B., Risk, M., Guilderson, T. P., and McKinney, R. A. (2005). Stable isotopic composition of deep-sea gorgonian corals Primnoa spp.: a new archive of surface processes. Mar. Ecol. Prog. Ser. 301, 135–148. doi: 10.3354/meps301135
Sitjá, C., Maldonado, M., Farias, C., and Rueda, J. L. (2018). Deep-water sponge fauna from the mud volcanoes of the Gulf of Cadiz (North Atlantic, Spain). J. Mar. Biol. Assoc. United Kingdom 99, 807–831. doi: 10.1017/S0025315418000589
Smith, C. R., De Leo, F. C., Bernardino, A. F., Sweetman, A. K., and Arbizu, P. M. (2008). Abyssal food limitation, ecosystem structure and climate change. Trends Ecol. Evol. 23, 518–528. doi: 10.1016/j.tree.2008.05.002
Smith, C. R., Drazen, J., and Mincks, S. L. (2006). “Deep-sea Biodiversity and Biogeography: perspectives from the Abyss,” in Proceedings of the International Seabed Authority Seamount Biodiversity Symposium, Honolulu, 1–13.
Soetaert, K., Mohn, C., Rengstorf, A., Grehan, A., and Van Oevelen, D. (2016). Ecosystem engineering creates a direct nutritional link between 600 m deep cold-water coral mounds and surface productivity. Sci. Rep. 6, 1–9. doi: 10.1038/srep35057
Soltwedel, T. (2000). Metazoan meiobenthos along continental margins: a review. Prog. Oceanogr. 46, 59–84. doi: 10.1016/s0079-6611(00)00030-6
Somoza, L., Ercilla, G., Urgorri, V., León, R., Medialdea, T., Paredes, M., et al. (2014). Detection and mapping of cold-water coral mounds and living Lophelia reefs in the Galicia Bank, Atlantic NW Iberia margin. Mar. Geol. 349, 73–90. doi: 10.1016/j.margeo.2013.12.017
Stramma, L., Schmidtko, S., Levin, L. A., and Johnson, G. C. (2010). Ocean oxygen minima expansions and their biological impacts. Deep Res. Part I Oceanogr. Res. 57, 587–595. doi: 10.1016/j.dsr.2010.01.005
Strand, R., Whalan, S., Webster, N. S., Kutti, T., Fang, J. K. H., Luter, H. M., et al. (2017). The response of a boreal deep-sea sponge holobiont to acute thermal stress. Sci. Rep. 7:1660. doi: 10.1038/s41598-017-01091-x
Strömberg, S. M., and Larsson, A. I. (2017). Larval behavior and longevity in the cold-water coral Lophelia pertusa indicate potential for long distance dispersal. Front. Mar. Sci. 4:411. doi: 10.3389/fmars.2017.00411
Stuart, C. T., and Rex, M. A. (2009). Bathymetric patterns of deep-sea gastropod species diversity in 10 basins of the Atlantic Ocean and Norwegian Sea. Mar. Ecol. Evol. Perspect. 30, 164–180. doi: 10.1111/j.1439-0485.2008.00269.x
Sweetman, A. K., Thurber, A. R., Smith, C. R., Levin, L. A., Mora, C., Wei, C.-L., et al. (2017). Major impacts of climate change on deep-sea benthic ecosystems. Elem. Sci. Anth. 5, 2–23. doi: 10.1525/elementa.203
Teixeira, S., Olu, K., Decker, C., Cunha, R. L., Fuchs, S., Hourdez, S., et al. (2013). High connectivity across the fragmented chemosynthetic ecosystems of the deep Atlantic Equatorial Belt: efficient dispersal mechanisms or questionable endemism? Mol. Ecol. 22, 4663–4680. doi: 10.1111/mec.12419
Thiem, O., Ravagnan, E., Fosså, J. H., and Berntsen, J. (2006). Food supply mechanisms for cold-water corals along a continental shelf edge. J. Mar. Syst. 60, 207–219. doi: 10.1016/j.jmarsys.2005.12.004
Thornalley, D. J. R., Oppo, D. W., Ortega, P., Robson, J. I., Brierley, C. M., Davis, R., et al. (2018). Anomalously weak Labrador Sea convection and Atlantic overturning during the past 150 years. Nature 556, 227–230. doi: 10.1038/s41586-018-0007-4
Thresher, R. E., Tilbrook, B., Fallon, S., Wilson, N. C., and Adkins, J. (2011). Effects of chronic low carbonate saturation levels on the distribution, growth and skeletal chemistry of deep-sea corals and other seamount megabenthos. Mar. Ecol. Prog. Ser. 442, 87–99. doi: 10.3354/meps09400
Tittensor, D. P., Baco, A. R., Brewin, P. E., Clark, M. R., Consalvey, M., Hall-Spencer, J., et al. (2009). Predicting global habitat suitability for stony corals on seamounts. J. Biogeogr. 36, 1111–1128. doi: 10.1111/j.1365-2699.2008.02062.x
Tittensor, D. P., Baco, A. R., Hall-Spencer, J. M., Orr, J. C., and Rogers, A. D. (2010). Seamounts as refugia from ocean acidification for cold-water stony corals. Mar. Ecol. 31, 212–225. doi: 10.1111/j.1439-0485.2010.00393.x
Tittensor, D. P., Rex, M. A., Stuart, C. T., McClain, C. R., and Smith, C. R. (2011). Species-energy relationships in deep-sea molluscs. Biol. Lett. 7, 718–722. doi: 10.1098/rsbl.2010.1174
Tong, R., Purser, A., Unnithan, V., and Guinan, J. (2012). Multivariate statistical analysis of distribution of deep-water gorgonian corals in relation to seabed topography on the Norwegian Margin. PLoS One 7:e43534. doi: 10.1371/journal.pone.0043534
Tsuchiya, M. (1989). Circulation of the antarctic intermediate water in the North Atlantic Ocean. J. Mar. Res. 47, 747–755. doi: 10.1357/002224089785076136
Tunnicliffe, V., Davies, K. T. A., Butterfield, D. A., Embley, R. W., Rose, J. M., and Chadwick, W. W. (2009). Survival of mussels in extremely acidic waters on a submarine volcano. Nat. Geosci. 2, 344–348. doi: 10.1038/ngeo500
Tursi, A., Mastrototaro, F., Matarrese, A., Maiorano, P., and D’onghia, G. (2004). Biodiversity of the white coral reefs in the ionian sea (Central Mediterranean). Chem. Ecol. 20, 107–116. doi: 10.1080/02757540310001629170
Van Aken, H. M., and De Boer, C. J. (1995). On the synoptic hydrography of intermediate and deep water masses in the Iceland Basin. Deep Res. Part I Oceanogr. Res. 42, 165–189. doi: 10.1016/0967-0637(94)00042-Q
van den Beld, I. M. J., Bourillet, J.-F., Arnaud-Haond, S., de Chambure, L., Davies, J. S., Guillaumont, B., et al. (2017). Cold-water coral habitats in submarine canyons of the Bay of Biscay. Front. Mar. Sci. 4:118. doi: 10.3389/fmars.2017.00118
Van Dover, C. L., German, C. R., Speer, K. G., Parson, L. M., and Vrijenhoek, R. C. (2002). Evolution and biogeography of deep-sea vent and seep invertebrates. Science 295, 1253–1257. doi: 10.1126/science.1067361
van Haren, H., Mienis, F., Duineveld, G. C. A., and Lavaleye, M. S. S. (2014). High-resolution temperature observations of a trapped nonlinear diurnal tide influencing cold-water corals on the Logachev mounds. Prog. Oceanogr. 125, 16–25. doi: 10.1016/j.pocean.2014.04.021
Van Oevelen, D., Mueller, C. E., Lundälv, T., Van Duyl, F. C., De Goeij, J. M., and Middelburg, J. J. (2018). Niche overlap between a cold-water coral and an associated sponge for isotopically enriched particulate food sources. PLoS One 13:e0194659. doi: 10.1371/journal.pone.0194659
Van Rooij, D., Blamart, D., Kozachenko, M., and Henriet, J.-P. (2007a). Small mounded contourite drifts associated with deep-water coral banks, Porcupine Seabight, NE Atlantic Ocean. Geol. Soc. Lond. Spec. Publ. 276, 225–244. doi: 10.1144/gsl.sp.2007.276.01.11
Van Rooij, D., Blamart, D., Richter, T., Wheeler, A., Kozachenko, M., and Henriet, J. P. (2007b). Quaternary sediment dynamics in the Belgica mound province, Porcupine Seabight: ice-rafting events and contour current processes. Int. J. Earth Sci. 96, 121–140. doi: 10.1007/s00531-006-0086-6
Vaquer-Sunyer, R., and Duarte, C. M. (2008). Thresholds of hypoxia for marine biodiversity. Proc. Natl. Acad. Sci. U.S.A. 105, 15452–15457. doi: 10.1073/pnas.0803833105
Verkaik, K., Hamel, J. F., and Mercier, A. (2017). Impact of ocean acidification on reproductive output in the deep-sea annelid Ophryotrocha sp. (Polychaeta: Dorvilleidae). Deep Res. Part II Top. Stud. Oceanogr. 137, 368–376. doi: 10.1016/j.dsr2.2016.05.022
Vetter, E. W., and Dayton, P. K. (1998). Macrofaunal communities within and adjacent to a detritus-rich submarine canyon system. Deep Res. Part II Top. Stud. Oceanogr. 45, 25–54. doi: 10.1016/s0967-0645(97)00048-9
Wade, I. P., Ellett, D. J., and Heywood, K. J. (1997). The influence of intermediate waters on the stability of the eastern North Atlantic. Deep Res. Part I Oceanogr. Res. 44, 1405–1426. doi: 10.1016/S0967-0637(97)00023-X
Wagner, H., Purser, A., Thomsen, L., Jesus, C. C., Lundälv, T., and Lundalv, T. (2011). Particulate organic matter fluxes and hydrodynamics at the Tisler cold-water coral reef. J. Mar. Syst. 85, 19–29. doi: 10.1016/j.jmarsys.2010.11.003
Wall, M., Ragazzola, F., Foster, L. C., Form, A., and Schmidt, D. N. (2015). PH up-regulation as a potential mechanism for the cold-water coral Lophelia pertusa to sustain growth in aragonite undersaturated conditions. Biogeosciences 12, 6869–6880. doi: 10.5194/bg-12-6869-2015
Watling, L., Guinotte, J., Clark, M. R., and Smith, C. R. (2013). A proposed biogeography of the deep ocean floor. Prog. Oceanogr. 111, 91–112. doi: 10.1016/j.pocean.2012.11.003
Wenzhöfer, F., Adler, M., Kohls, O., Hensen, C., Strotmann, B., Boehme, S., et al. (2001). Calcite dissolution driven by benthic mineralization in the deep-sea: in situ measurements of Ca2 +, pH, pCO2 and O2. Geochim. Cosmochim. 65, 2677–2690. doi: 10.1016/S0016-7037(01)00620-2
White, M., Bashmachnikov, I., Arístegui, J., and Martins, A. (2007a). “Physical Processes and Seamount Productivity,” in Seamounts: Ecology, Fisheries & Conservation Wiley Online Books, eds T. J. Pitcher, T. Morato, P. J. B. Hart, M. R. Clark, N. Haggan, and R. S. Santos (Oxford: Blackwell Publishing), 65–84.
White, M., and Dorschel, B. (2010). The importance of the permanent thermocline to the cold water coral carbonate mound distribution in the NE Atlantic. Earth Planet. Sci. Lett. 296, 395–402. doi: 10.1016/j.epsl.2010.05.025
White, M., Mohn, C., de Stigter, H., and Mottram, G. (2005). “Deep-water coral development as a function of hydrodynamics and surface productivity around the submarine banks of the Rockall Trough, NE Atlantic,” in Cold-Water Corals and Ecosystems, eds A. Freiwald and J. M. Roberts (Berlin: Springer-Verlag), 503–514. doi: 10.1007/3-540-27673-4_25
White, M., Roberts, J. M., and van Weering, T. (2007b). Do bottom-intensified diurnal tidal currents shape the alignment of carbonate mounds in the NE Atlantic? Geo Mar. Lett. 27, 391–397. doi: 10.1007/s00367-007-0060-8
White, M., Wolff, G. A., Lundälv, T., Guihen, D., Kiriakoulakis, K., Lavaleye, M., et al. (2012). Cold-water coral ecosystem (Tisler Reef, Norwegian shelf) may be a hotspot for carbon cycling. Mar. Ecol. Prog. Ser. 465, 11–23. doi: 10.3354/meps09888
Wicks, L., and Roberts, J. (2012). “Benthic invertebrates in a high CO2 world,” in Oceanography and Marine Biology: An Annual Review, eds R. N. Gibson, R. J. A. Atkinson, J. D. M. Gordon, and R. N. Hughes (Boca Raton, FL: CRC Press), 127–188. doi: 10.1201/b12157-4
Wienberg, C., Hebbeln, D., Fink, H. G., Mienis, F., Dorschel, B., Vertino, A., et al. (2009). Scleractinian cold-water corals in the Gulf of Cádiz-First clues about their spatial and temporal distribution. Deep Res. Part I Oceanogr. Res. 56, 1873–1893. doi: 10.1016/j.dsr.2009.05.016
Wienberg, C., and Witschack, J. (2017). “Framework-forming scleractinian cold-water corals through space and time: a late Quaternary North Atlantic perspective,” in Marine Animal Forests: The Ecology of Benthic Biodiversity Hotspots, eds S. Rossi, L. Bramanti, A. Gori, and C. Orejas (Switzerland: Springer International Publishing), 1–34. doi: 10.1007/978-3-319-17001-5_16-1
Willig, M. R., Kaufman, D. M., and Stevens, R. D. (2003). Latitudinal gradients of biodiversity: pattern, process, scale, and synthesis. Annu. Rev. Ecol. Evol. Syst. 34, 273–309. doi: 10.1146/annurev.ecolsys.34.012103.144032
Wisshak, M., Schönberg, C. H. L., Form, A., and Freiwald, A. (2012). Ocean acidification accelerates reef bioerosion. PLoS One 7:e45124. doi: 10.1371/journal.pone.0045124
Wisshak, M., Schönberg, C. H. L., Form, A., and Freiwald, A. (2014). Sponge bioerosion accelerated by ocean acidification across species and latitudes? Helgol. Mar. Res. 68, 253–262. doi: 10.1007/s10152-014-0385-4
Woulds, C., and Cowie, G. (2007). Oxygen as a control on sea floor biological communities and their roles in sedimentary carbon cycling. Limnol. Oceanogr. 52, 1698–1709. doi: 10.2307/4502324
Xavier, J., and Van Soest, R. (2007). Demosponge fauna of Ormonde and Gettysburg seamounts (Gorringe Bank, north-east Atlantic): diversity and zoogeographical affinities. J. Mar. Biol. Assoc. United Kingdom 87, 1643–1653. doi: 10.1017/S0025315407058584
Xie, R. C., Marcantonio, F., and Schmidt, M. W. (2012). Deglacial variability of Antarctic Intermediate Water penetration into the North Atlantic from authigenic neodymium isotope ratios. Paleoceanography 27, 1–12. doi: 10.1029/2012PA002337
Yashayaev, I. (2007). Hydrographic changes in the Labrador Sea, 1960–2005. Prog. Oceanogr. 73, 242–276. doi: 10.1016/j.pocean.2007.04.015
Yasuhara, M., and Danovaro, R. (2014). Temperature impacts on deep-sea biodiversity. Biol. Rev. 91, 275–287. doi: 10.1111/brv.12169
Yesson, C., Taylor, M. L., Tittensor, D. P., Davies, A. J., Guinotte, J., Baco, A., et al. (2012). Global habitat suitability of cold-water octocorals. J. Biogeogr. 39, 1278–1292. doi: 10.1111/j.1365-2699.2011.02681.x
Zenk, W., and Armi, L. (1990). The complex spreading pattern of Mediterranean Water off the Portuguese continental slope. Deep Sea Res. Part A. Oceanogr. Res. Pap. 37, 1805–1823. doi: 10.1016/0198-0149(90)90079-B
Keywords: North Atlantic, deep-sea, biodiversity, biogeography, water masses, vulnerable marine ecosystems
Citation: Puerta P, Johnson C, Carreiro-Silva M, Henry L-A, Kenchington E, Morato T, Kazanidis G, Rueda JL, Urra J, Ross S, Wei C-L, González-Irusta JM, Arnaud-Haond S and Orejas C (2020) Influence of Water Masses on the Biodiversity and Biogeography of Deep-Sea Benthic Ecosystems in the North Atlantic. Front. Mar. Sci. 7:239. doi: 10.3389/fmars.2020.00239
Received: 11 November 2019; Accepted: 27 March 2020;
Published: 21 April 2020.
Edited by:
Frank Edgar Muller-Karger, College of Marine Science, University of South Florida, United StatesReviewed by:
Emil Petruncio, Ocean Exploration Trust, United StatesCopyright © 2020 Puerta, Johnson, Carreiro-Silva, Henry, Kenchington, Morato, Kazanidis, Rueda, Urra, Ross, Wei, González-Irusta, Arnaud-Haond and Orejas. This is an open-access article distributed under the terms of the Creative Commons Attribution License (CC BY). The use, distribution or reproduction in other forums is permitted, provided the original author(s) and the copyright owner(s) are credited and that the original publication in this journal is cited, in accordance with accepted academic practice. No use, distribution or reproduction is permitted which does not comply with these terms.
*Correspondence: Patricia Puerta, cGF0cmljaWEucHVlcnRhQGllby5lcw==
Disclaimer: All claims expressed in this article are solely those of the authors and do not necessarily represent those of their affiliated organizations, or those of the publisher, the editors and the reviewers. Any product that may be evaluated in this article or claim that may be made by its manufacturer is not guaranteed or endorsed by the publisher.
Research integrity at Frontiers
Learn more about the work of our research integrity team to safeguard the quality of each article we publish.