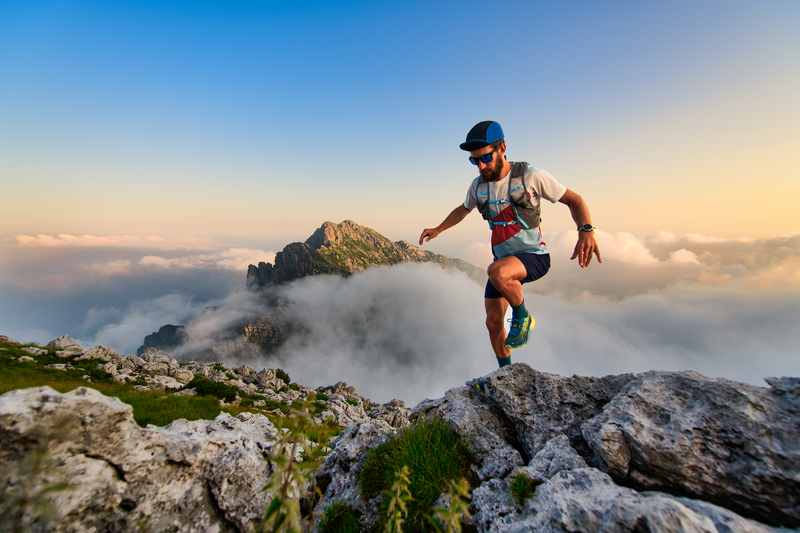
95% of researchers rate our articles as excellent or good
Learn more about the work of our research integrity team to safeguard the quality of each article we publish.
Find out more
ORIGINAL RESEARCH article
Front. Mar. Sci. , 18 March 2020
Sec. Global Change and the Future Ocean
Volume 7 - 2020 | https://doi.org/10.3389/fmars.2020.00143
A carbon budget for the northwest European continental shelf seas (NWES) was synthesized using available estimates for coastal, pelagic and benthic carbon stocks and flows. Key uncertainties were identified and the effect of future impacts on the carbon budget were assessed. The water of the shelf seas contains between 210 and 230 Tmol of carbon and absorbs between 1.3 and 3.3 Tmol from the atmosphere annually. Off-shelf transport and burial in the sediments account for 60–100 and 0–40% of carbon outputs from the NWES, respectively. Both of these fluxes remain poorly constrained by observations and resolving their magnitudes and relative importance is a key research priority. Pelagic and benthic carbon stocks are dominated by inorganic carbon. Shelf sediments contain the largest stock of carbon, with between 520 and 1600 Tmol stored in the top 0.1 m of the sea bed. Coastal habitats such as salt marshes and mud flats contain large amounts of carbon per unit area but their total carbon stocks are small compared to pelagic and benthic stocks due to their smaller spatial extent. The large pelagic stock of carbon will continue to increase due to the rising concentration of atmospheric CO2, with associated pH decrease. Pelagic carbon stocks and flows are also likely to be significantly affected by increasing acidity and temperature, and circulation changes but the net impact is uncertain. Benthic carbon stocks will be affected by increasing temperature and acidity, and decreasing oxygen concentrations, although the net impact of these interrelated changes on carbon stocks is uncertain and a major knowledge gap. The impact of bottom trawling on benthic carbon stocks is unique amongst the impacts we consider in that it is widespread and also directly manageable, although its net effect on the carbon budget is uncertain. Coastal habitats are vulnerable to sea level rise and are strongly impacted by management decisions. Local, national and regional actions have the potential to protect or enhance carbon storage, but ultimately global governance, via controls on emissions, has the greatest potential to influence the long-term fate of carbon stocks in the northwestern European continental shelf.
Continental shelf seas play an important role in the global cycling of carbon. Although they account for less than 10% of the global ocean area (Harris et al., 2014), shelf seas contribute 80% of the organic carbon burial in sediments and 50% of the organic carbon delivery to the deep ocean (Bauer et al., 2013). They are responsible for 8–19% (Bauer et al., 2013; Laruelle et al., 2014) of the ocean carbon sink of 2.4 Pg C year–1 (Le Quéré et al., 2018).
The northwest European shelf (NWES; Figure 1) is a large area of shallow temperate seas which occupies between 3 and 4% of global continental shelf area (Wakelin et al., 2012; Harris et al., 2014) and is globally significant in terms of the uptake of atmospheric CO2 (Frankignoulle and Borges, 2001; Laruelle et al., 2014). As one of the most studied and impacted shelf sea regions it is also a key region for developing understanding of shelf sea biogeochemistry applicable to other, less intensively studied or impacted shelf systems. The mechanism of CO2 uptake by seasonally stratifying shelf seas has been termed the continental shelf pump (Tsunogai et al., 1999; Thomas et al., 2004) and relies on both the biological productivity of shelf seas and their physical oceanography. This mechanism has been observed in the North Sea (Thomas et al., 2004; Bozec et al., 2005), with the export of carbon into the North Atlantic Ocean (Thomas et al., 2005) driven by the downwelling circulation on the shelf (Holt et al., 2009).
Figure 1. A map of the northwest European continental shelf. Numbers indicate the following regions, referred to in this study. 1: Southern North Sea, 2: Central North Sea, 3: Northern North Sea, 4: English Channel, 5: Skagerrak and Kattegat, 6: Norwegian Trench, 7: Shetland Shelf, 8: Irish Shelf, 9: Irish Sea, 10: Celtic Sea, 11: Armorican Shelf.
Globally, the uptake of CO2 by shelf seas is thought to have increased over the last two decades as the difference between air and shelf water pCO2 has increased (Laruelle et al., 2018). This trend may be driven by faster shelf-edge exchange and increased primary production due to anthropogenic nutrient inputs (Laruelle et al., 2018). Over longer timescales, coastal oceans may have shifted from pre-industrial CO2 sources to modern CO2 sinks due to increasing atmospheric CO2 and increased nutrient inputs (Bauer et al., 2013).
There is increasing interest in the role of the ocean in mitigating atmospheric CO2 increases, both in terms of the response to increasing emissions and the opportunities for managing and even enhancing carbon storage as “negative emissions” (Gallo et al., 2017; Gattuso et al., 2018; Smale et al., 2018). Carbon sequestration has clear societal benefit and society may wish to recognize this benefit in terms of economic value, in order to protect vulnerable ecosystems, aid policy decisions or widen the market for carbon management and trading (e.g., Nellemann et al., 2009; Lau, 2013; Luisetti et al., 2019). The NWES also provides a range of resources and services to society such as seafood, natural products, aggregates for construction, marine renewable energy and recreation (Turner et al., 2014). It is therefore particularly important for scientists and policy makers to understand the role of shelf seas in the global carbon cycle, how ongoing human actions are affecting the carbon budget and the health of these waters, and how the system is likely to respond to future changes.
In spite of much recent progress in understanding shelf carbon cycling on the NWES (e.g., Stahl et al., 2004; Thomas et al., 2005; Johnson et al., 2013; Hale et al., 2017; Hicks et al., 2017; Carr et al., 2018; Humphreys et al., 2018; Chaichana et al., 2019; Davis et al., 2019; García-Martín et al., 2019), there is still a great deal of uncertainty in both the mechanisms governing carbon dynamics and the magnitudes of contemporary carbon stocks and flows, as well as their responses to future change. Identifying key research priorities and knowledge gaps at this juncture is important for directing future research effort. Recent large-scale syntheses of shelf carbon budgets have demonstrated new insights into carbon flows on the North American shelves (Najjar et al., 2018; Fennel et al., 2019).
Here we present the first large-scale synthesis of the carbon budget for the NWES, including both carbon stocks and flows, with the aim of providing a reference point for policymakers and non-specialists as well as a baseline for future scientific study of carbon in the region.
This work presents temporally averaged estimates of individual stocks and flows of carbon on the northwest European continental shelf, using empirical data and model predictions, and is explicit about the associated uncertainties and unknowns. Given the disparity of methodologies and scales applied to estimate the various stocks and flows, a balanced budget was not expected. However, imbalances between inputs and outputs or large uncertainties on elements of the budget highlight key knowledge gaps and research priorities. The future impacts of various global and regional changes on the carbon budget were considered.
Marine and coastal carbon sinks cover a wider area than the vegetated coastal and intertidal habitats (salt marsh, seagrasses, and mangroves) considered by many authors as “Blue Carbon” (Nellemann et al., 2009; Mcleod et al., 2011; Duarte, 2017). Sand and mud flats, macroalgae, shelf sea sediments and the flow of water into the deep ocean are all potential net sinks for carbon and so provide value to society (Nellemann et al., 2009; Gattuso et al., 2018). This study synthesizes a budget for all organic and inorganic carbon on the NWES, from intertidal habitats to the shelf edge, here defined as the 200 m isobath. We also included the deeper Norwegian Trench, Skagerrak and Kattegat (Figure 1). The inshore limit was defined as the top of the intertidal zone and estuarine environments. Riverine input includes rivers draining into the study area shown in Figure 1, including the low-salinity Baltic Sea outflow.
Carbon stocks on the NWES were divided into coastal, pelagic and benthic components. The coastal component of our budget includes intertidal habitats such as salt marshes, mud flats and sand flats, as well as seagrass beds and kelp forests. The benthic component includes the shelf sediments in regions 1–11 of Figure 1, including fjords. Slope sediments deeper than 200m, including submarine canyons, are known to store significant amounts of carbon exported from the shelf (Walsh, 1991; Buscail and Germain, 1997; Masson et al., 2010). However, these fall outside of our study area and are therefore not quantified.
The pelagic component includes particulate and dissolved carbon in the water column. The following fluxes (flows) of carbon into, out of and within the NWES were quantified: air-sea CO2 flux; riverine input; cross shelf exchange; pelagic-benthic flux; CO2 flux between coastal habitats and the atmosphere; exchange between coastal habitats and the water column; carbon burial within coastal habitats; burial of kelp carbon in sediments. These stocks and flows were subdivided into various types, based on biogeochemical and geographic separations as described below. Where possible, organic and inorganic, and particulate and dissolved carbon were quantified separately. Present-day estimates of the stocks and flows of carbon, by type, were quantified in per-area or per-volume units and scaled to the whole NWES. This scaling approach allows the consideration of habitats and ecosystem components that are too patchy to represent in regional or global models and allows the relative magnitude of various stocks and flows to be assessed.
The best method of dividing a complex budget for this type of observation-based scaling approach cannot be determined a priori. Instead, the division of the budget into types was determined by the resolution and variability of the available data for each stock or flow. Thus, each part of the budget was divided to ensure that the available data were representative of the stock or flow in question, whilst attempting to minimize the aliasing of temporal and spatial variability. Details of the full budget synthesis, including stocks and flows for each type, are given in the Supplementary Material Part A.
The water column of the NWES is strongly spatially and seasonally variable with respect to carbon stocks and flows. Strong interannual variability has also been observed in both dissolved inorganic carbon (DIC) and dissolved organic carbon (DOC) concentrations and air-sea CO2 fluxes (Clargo et al., 2015; Hartman et al., 2018; Carr et al., 2018; Humphreys et al., 2018; Chaichana et al., 2019; Kitidis et al., 2019). Because of this variability and the patchy coverage of data (both in time and space), the water column was treated as a single box in the budget. Similarly, cross-shelf exchange was considered as a single estimate for each carbon type in the budget.
Sediment carbon stocks and benthic-pelagic fluxes were calculated using different spatial divisions to each other, due to differences in data availability. Observations and modeling (Diesing et al., 2017; Wilson et al., 2018) allowed carbon stocks to be resolved at a regional level so the seabed of the NWES was divided into 13 areas (the 11 regions in Figure 1, plus sediment in Scottish lochs and Norwegian fjords). Benthic-pelagic fluxes were estimated over the whole shelf area due to data paucity, with the exception of lochs, fjords, the Norwegian Trench and the Skagerrak and Kattegat, which were quantified separately.
Only carbon in the top 0.1 m of sediment was considered when quantifying benthic and coastal carbon stocks, following the protocol used in previous studies (Burrows et al., 2017; Diesing et al., 2017; Luisetti et al., 2019; Queirós et al., 2019). This depth does not reflect the vertical limit of carbon storage, which is likely to vary across coastal and benthic habitats with sediment type, degree of bioturbation, and sedimentation rate. However, it provides a useful benchmark for comparison of different sediment types and with previous studies. We acknowledge that any fixed depth boundary is somewhat arbitrary and in reality surface sediment will vary in carbon content, age, depth and sedimentation rate [e.g., outside river mouths and fjords have higher sediment accumulation rates than continental shelf sediments (Smith et al., 2015)].
Benthic and pelagic measurements of POC and PIC inevitably include some but not all living biomass. For example, phytoplankton and small zooplankton are typically included in pelagic POC measurements, but larger organisms are not captured in oceanographic sampling and are thus omitted. Similarly, large benthic epifauna and infauna are not normally captured in sediment stock estimates. Higher trophic levels are not usually included in biogeochemical budget studies but do make a contribution to the standing stock of carbon in benthic and pelagic habitats so we estimated the size of these living carbon stocks, based on the available data for the region (de Wilde et al., 1986; Sparholt, 1990; Mackinson and Daskalov, 2007).
The coastal component of the budget was divided into four habitats considered to contain the largest carbon stocks: salt marshes, seagrass beds, kelp forests and mud/sand flats. Due to differences in the structure and function of these habitats, their stocks and flows were considered separately. The sediment in salt marshes, seagrass beds and mud/sand flats is an integral part of these habitats and also contains the vast majority of their carbon. The carbon stocks quantified for these habitats therefore includes both sediment and living material. For consistency with the benthic component, only the top 0.1 m of sediment in salt marshes, seagrass beds and mud/sand flats were quantified.
Recent work has highlighted the potential role of macroalgae in contributing to carbon sequestration (Krause-Jensen and Duarte, 2016; Krause-Jensen et al., 2018; Queirós et al., 2019). The dominant group of macroalgae in Europe is kelp (Araujo et al., 2016), which is thought to contribute more to carbon sequestration than smaller, shorter-lived groups of macroalgae (Krause-Jensen et al., 2018). As kelp attaches primarily to hard substrata, any organic matter exported from kelp forests that is subsequently buried is quantified here as a flux of carbon from kelp to sediments. Kelp forest carbon stock presented here represents only the living kelp fronds.
Intertidal habitats exchange carbon directly with the atmosphere when exposed and the majority of primary production measurements in these habitats are taken during tidal emersion (Underwood and Kromkamp, 1999; van Colen et al., 2014; Nedwell et al., 2016). We know little about the carbon exchanges in salt marshes and mud/sand flats when submerged (Hanlon et al., 2006; McKew et al., 2013), so the exchange of carbon between the water column and these intertidal habitats was quantified as the residual of atmospheric CO2 uptake and burial. The uptake of CO2 from the atmosphere in salt marshes and mud/sand flats was quantified whereas seagrass beds and kelp forests exchange carbon only with the water column and/or sediment.
The stocks and flows of marine and coastal carbon on the NWES are summarized in Figure 2 and are expressed in units of Tmol (1012 moles of carbon) and Tmol year–1, respectively. For each stock and flow a range of possible values is determined based on the data synthesis and calculations presented in full in the Supplementary Material. In this section, we consider individual components of the budget synthesized in Figure 2. The benthic and coastal components of the budget are summarised in Figures 3, 4, respectively. Knowledge gaps and research priorities are identified and these are summarized in Figure 5 at the end of this section.
Figure 2. A summary of the carbon budget of the north west European shelf. Stocks (blue) are given in units of Tmol (1012 moles of carbon) and flows (black arrows) are given in units of Tmol year– 1. Ranges are based on a synthesis of literature values (Supplementary Material Part A).
Figure 3. The benthic concentration of particulate inorganic carbon (left) and particulate organic carbon (right), modeled using PIC and POC data and a suite of predictor variables (Supplementary Material Part A).
Figure 4. A breakdown of the carbon budget in coastal habitats of the north west European shelf. Ranges are based on a synthesis of literature values. Saltmarshes: Boorman (2003), Wood et al. (2015), Dijkema (1991), Davidson et al. (1991), Skov et al. (2016), Thornton et al. (2002), Barillé-Boyer et al. (2003), Beaumont et al. (2014), Burrows et al. (2014), Luisetti et al. (2019), Mcowen et al. (2017), Artigas et al. (2015), and Houghton and Woodwell (1980). Mud and sand flats: Maddock (2008), Dijkema (1991), Barillé-Boyer et al. (2003), Thornton et al. (2002), Adams et al. (2012), Nedwell et al. (2016), Underwood et al. (2016), and Wood et al. (2015). Seagrass beds: Maddock (2008), Essink et al. (2005), Fourqurean et al. (2012), Burrows et al. (2017), and Duarte (2017). Kelp forests: Burrows et al. (2014), Krause-Jensen and Duarte (2016), and Krause-Jensen et al. (2018). Full synthesis details given in Supplementary Material Part A.
Figure 5. The key knowledge gaps and research priorities in the contemporary carbon budget for the north west European shelf.
Large-scale modeling and observational studies of air-sea CO2 flux in the seas around northwest Europe find these waters to be a net sink of atmospheric CO2 of between 1.3 and 3.3 Tmol year–1 (Wakelin et al., 2012; Shutler et al., 2016; Kitidis et al., 2019) (Figure 2). Recent NEMO-ERSEM (European Regional Seas Ecosystem Model) (Butenschön et al., 2016) output for the period 1990–2015 estimates an air to sea CO2 flux of 2.9 Tmol year–1 which is consistent with this range. While there is consensus that the NWES as a whole is a net CO2 sink, regional studies within the NWES have a wide range of per-unit area air-sea CO2 flux estimates, with specific areas ranging from a weak source to a strong sink of CO2 (Thomas et al., 2004; Borges et al., 2006; Laruelle et al., 2014; Hartman et al., 2018). Understanding the shelf uptake of CO2 and the processes driving it are a key part of the shelf carbon budget and thus it is essential that surface ocean carbonate system measurements continue to be made and synthesized across the shelf (Bakker et al., 2016), along with synoptic scale atmospheric and sea state observations. Monitoring of this sink is important as it is likely to change with time, particularly as atmospheric CO2 concentrations and sea temperatures continue to increase.
The magnitude of the shelf-wide air–sea CO2 flux estimate is strongly affected by the choice of the boundary between the continental shelf and the open ocean. Upwelling at the shelf break brings cold, nutrient rich water driving primary productivity and high CO2 uptake near the shelf break (Shutler et al., 2016). A relatively small change in the horizontal location of the shelf sea boundary definition can therefore have a significant impact on the estimated net exchange. Changing the shelf edge definition from the 200 m isobath to the 1000 m isobath increases the shelf area by roughly 18% but increases net air–sea flux by up to 42% (Shutler et al., 2016; Kitidis et al., 2019). To ensure that our estimates are comparable to the majority of the literature we used the 200 m isobath and therefore the lower range of possible CO2 uptakes.
The largest unknown on shelf-wide air-sea CO2 flux is the contribution of near shore waters. The intertidal habitats (salt marshes, mud flats and sand flats) are a net sink of atmospheric CO2 when exposed (Figure 4). However, subtracting burial rates from this uptake of atmospheric CO2 suggests that these habitats are, overall, net sources of carbon to the water column (Figure 4). This coastal input of carbon to the water, combined with riverine inputs, makes near-shore waters a likely net source of CO2 to the atmosphere (Borges et al., 2005, 2006; Chen and Borges, 2009; Laruelle et al., 2010). As the majority of CO2 measurements used to quantify air-sea flux on the NWES are taken outside of these coastal and estuarine areas, observation-based estimates of shelf-wide CO2 uptake are likely to be overestimated. The complex tapestry of habitats makes coastal carbon fluxes difficult to model and we suggest that more observations of air-sea CO2 flux in near-shore waters are needed to quantify the size and variability of this flux (Kuwae et al., 2016) and thus better constrain the shelf-wide air-sea CO2 flux.
Rivers are estimated to deliver between 2.3 and 5.0 Tmol year–1 of carbon to the seas of the NWES (Figure 2), with between 0.2 and 2.4 Tmol year–1 as organic matter. These values are based on discharge data, measured carbon concentrations and modeling studies (Abril et al., 2002; Thomas et al., 2005; Lenhart et al., 2010; Artioli et al., 2012; Wakelin et al., 2012; Jarvie et al., 2017). Riverine carbon input was calculated using climatological river discharge data and therefore does not account for interannual variability of river flow. Measurements show that the discharge of individual rivers and the Baltic Sea outflow can be highly variable between years due to variations in weather and human interventions (Omstedt et al., 2004; Kwadijk et al., 2016), and that carbon loadings vary with flow (Mehring et al., 2013). Interannual variability in the riverine flux of carbon and nutrients to shelf seas is very likely to cause variability in other components of the NWES carbon budget.
Estimation of riverine carbon flux is hindered by a lack of observational data as most nations do not routinely measure sufficient parameters to quantitatively assess carbon fluxes with well constrained uncertainties. For example, rather than being measured directly, riverine inorganic carbon flux is commonly calculated from empirical relationships of DIC and total alkalinity (TA) derived from salinity data, which relies on a series of underlying assumptions, and the results can therefore be subject to increased uncertainties (Cai et al., 2010; Artioli et al., 2012; Land et al., 2015). More frequent and coordinated observations of carbon concentrations or the parameters required to derive these (e.g., carbonate system variables) within major river systems would help to refine these estimates. The values for riverine organic carbon inputs to the NWES used in model simulations are particularly important to better constrain as these will have direct impacts on the predictions of DOC concentrations and ecosystem responses, with knock-on effects for air-sea CO2 flux estimates.
Pelagic stocks represent a significant component in the carbon budget (210–230 Tmol) with DIC dominating the pelagic budget (93–97% of total pelagic carbon). DOC occupies a further 2–5% with the remainder comprising a minor contribution from POC, PIC and macrofauna (Supplementary Material). Ranges of DIC, DOC, PIC and POC concentrations were based on measurements and model results (Buitenhuis et al., 1996; Weston et al., 2004; Thomas et al., 2005; Suratman et al., 2009; Salt et al., 2013; Poulton et al., 2014; Clargo et al., 2015; Hartman et al., 2018; Painter et al., 2018; Chaichana et al., 2019; Davis et al., 2019) and were multiplied by the volume of water on the shelf (9.91 × 1013 m3, Wakelin et al., 2012) to give total carbon stocks. The non-DIC component of the pelagic carbon budget (approximately 6–16 Tmol) is large relative to coastal habitats (see section “Coastal habitats”) and potentially sensitive to anthropogenic influence (see section “Responses of Carbon Stocks and Flows on the NWES to Future Changes”).
The pelagic system is important not only because of large stocks but also because of the processes which lead to carbon uptake, processing and export (recently termed the “engine of productivity,” Kröger et al., 2018), which ultimately control the magnitude of the continental shelf carbon pump. The majority of pelagic DIC originates from and returns to the open ocean via cross-shelf exchange and could be considered a “spectator” in the shelf carbon cycle. However the marginal changes in DIC concentration ultimately drive the uptake of atmospheric CO2 by shelf waters and are therefore key to the active carbon fluxes through the system.
Organic carbon is produced in situ from CO2 in the water column by primary production. This transformation from inorganic to organic pools is usually limited by nutrient (mostly nitrogen) availability. Most studies conclude that the majority of pelagic primary production on the NWES is supported by nutrient inputs from the open ocean (e.g., Vermaat et al., 2008; Holt et al., 2012; Ruiz-Castillo et al., 2018) and therefore, it is key to the functioning of the shelf carbon pump that whilst there is net cross-shelf export of carbon, there is net import of nutrients. Furthermore, the role of transport and circulation in the dynamics of carbon uptake has recently been highlighted, both in terms of transport of riverine material to the shelf break and exchange of carbon between the shelf and open ocean (Sharples et al., 2016; Humphreys et al., 2018; Chaichana et al., 2019). Ultimately, the production and processing of organic matter is likely a key factor in the ability of shelf seas to take carbon up from the atmosphere, in spite of heavy riverine carbon loadings (e.g., Humphreys et al., 2018).
Organic matter may be flocculated and buried, photolyzed, outgassed, or exported into the open ocean. The production of refractory DOC in coastal waters may reduce the amount of carbon which is remineralized on the shelf, thereby reducing the amount of CO2 released to the atmosphere and strengthening the shelf carbon pump (Kuwae et al., 2016). However, the amount of this coastal DOC which crosses the shelf break is largely unknown (Painter et al., 2018). Labile terrigenous organic matter is likely respired in estuaries and coastal waters, contributing to the net efflux of CO2 from these near-shore waters to the atmosphere (Cai, 2011; Bauer et al., 2013; Regnier et al., 2013). CO2 emissions from European estuaries alone represent 5–10% of Western Europe’s anthropogenic CO2 emissions (Frankignoulle et al., 1998). Some terrigenous DOC (tDOC) is flocculated and buried within shelf and ocean sediments, contributing up to 30% to ocean sedimentary burial (Burdige, 2005; Kandasamy and Nagender Nath, 2016). More refractory tDOC may be transported far from the coast and even off-shelf. In the Celtic Sea, up to 30% of DOC at the shelf edge is of terrestrial origin (Carr et al., 2018), whereas a study in the North Sea suggests that little tDOC reaches the open ocean (Painter et al., 2018). These contrasting results suggest that regional dynamics play an important role in the transport of terrigenous material across the shelf. It has long been recognized that the fate of terrestrial organic matter in the ocean is a key knowledge gap in the global carbon cycle (e.g., Hedges et al., 1997) and this remains an intriguing gap in our understanding of the shelf sea carbon cycle. The Natural Environment Research Council (NERC) funded LOCATE (Land Ocean Carbon Transfer) program is currently tackling this key unknown through combined observations and modeling effort1.
Modeling studies predict small net carbon flux to the sediments and suggest that carbon inputs to the NWES are largely balanced by net off-shelf transport (Wakelin et al., 2012; Butenschön et al., 2016). The estimated net off-shelf transport of between 3.5 and 8.2 Tmol year–1 is the residual of much larger exchanges across the shelf break which are net on-shelf in the upper water column and net off-shelf below the permanent pycnocline (Wakelin et al., 2012). Our estimates of cross-shelf carbon transport rely on modeling results due to the limited spatial coverage of observations. Quantification of cross-shelf carbon transport requires knowledge of both the physical exchange of water masses and the carbon content of these water masses. Recent work has improved our understanding of the physical processes at the shelf edge (Porter et al., 2016; Spingys, 2017) but the total exchange of water and particularly carbon across the shelf edge remains poorly constrained by observations. In situ biogeochemical and physical measurements in key locations across the shelf break using moorings and gliders in combination with synoptic-scale monitoring from satellite data, could help to resolve this key unknown in the carbon budget of the shelf.
A widespread feature of continental shelf slopes which requires particular research effort in terms of impact on the carbon budget is submarine canyons. The heads of many canyons incise the edge of the NWES (Harris and Whiteway, 2011) and they are known to enhance shelf-edge exchange through advection and mixing, and can act as major conduits of dissolved and particulate matter from shallow to deep water (Allen and Durrieu De Madron, 2009; Puig et al., 2014). A recent study found that reducing the grid spacing in the AMM (Atlantic Margin Model) from 7 km to 1.5 km allowed smaller scale features such as canyons to be resolved, which contributed to stronger downwelling circulation in the higher resolution model (Graham et al., 2018). The models used here to estimate cross-shelf exchange do not resolve submarine canyons and this is clearly a priority for future work.
The wide range of net benthic burial estimates (−2.2 to 6.0 Tmol year−1; Supplementary Material Part A) reflects our limited knowledge of pelagic-benthic carbon fluxes, including their spatial distribution (Stahl et al., 2004; Burrows et al., 2017; Diesing et al., 2017; Queirós et al., 2019). Most POC delivered to the sea bed is thought to be oxidized through aerobic respiration and sulfate reduction (Krumins et al., 2013) but observational measurements of POC deposition and especially DIC release are scarce. Recent data suggests that these fluxes can be highly variable seasonally and that POC burial may be greater than expected from our current modeling, depending on processes varying temporally and spatially (Snelgrove et al., 2018; Queirós et al., 2019). Furthermore, a substantial proportion of the empirical benthic carbon data is derived from cohesive sediment studies, with the exception of a few (e.g., Boudreau et al., 2001; Precht and Huettel, 2003; Rao et al., 2008; Burt et al., 2014; Aldridge et al., 2017), yet the wide spatial coverage of permeable sediments on the shelf means there is likely to be an underestimation of the influence of advective flow on the carbon dynamics within benthic stocks (Boudreau et al., 2001; de Beer et al., 2005). More data, from a range of sediment types across the shelf and a better coverage of seasonality (measurements of carbon fluxes as well as physical and biological process rates) are needed to better quantify the balance of POC deposition and DIC release throughout the year. This would markedly improve the ability of modeling studies to constrain the net flux of carbon between the water column and the sea bed.
Given the paucity of existing observations and the range of estimates for both pelagic-benthic exchange and cross-shelf transport, the relative importance of these fluxes in driving the uptake of atmospheric CO2 remains uncertain. Clarifying the balance between burial and off-shelf transport of carbon is an important piece in the puzzle of how the NWES sink of atmospheric carbon will respond to future changes. A key challenge in addressing this subject is to develop and integrate numerical models that are simultaneously capable of (i) generating estimates of terrigenous carbon entering aquatic ecosystems, (ii) transporting this material to and within the ocean, and (iii) representing the various removal and modification processes that act upon it as it traverses the land ocean continuum.
Figure 3 shows the particulate organic and inorganic carbon stocks in the top 0.1 m of sediment of the NWES. Stocks of POC were calculated using observed relationships between POC data and various predictor variables (Diesing et al., 2017; Wilson et al., 2018), substantially extending the analysis in Diesing et al. (2017) to the whole of the NWES. Particulate inorganic carbon (PIC) stock was estimated using the same approach (Supplementary Material) but was done for the first time as a part of this study. We find that PIC stocks dominate the benthic budget, accounting for roughly 95% of the 520–1600 Tmol of carbon stored in the sediment. Shelf sediments are dominated by permeable (sandy) sediment (Boudreau et al., 2001; Hall, 2002; Glud, 2008; Rao et al., 2008; Hicks et al., 2017) which typically have lower organic and higher inorganic carbon concentrations compared to cohesive (muddy) sediments. The distribution of PIC in shelf sediments aligns with the coverage of permeable sediments (Wilson et al., 2018), particularly in areas of high carbonate sediments such as the Shetland and Orkney Islands (see Figure 3).
Particulate inorganic carbon is deposited in sediments via authigenic precipitation (within the sediment) of calcium carbonate, and from sedimentation of calcium carbonate rich biogenic material (such as coccolithophores), and may be linked to decomposition of organic carbon (Yu et al., 2018). Burial of inorganic carbon linked to the formation of calcium carbonate can account for up to 80% of carbon removed from the carbon cycle (Sun and Turchyn, 2014). Organic carbon that sinks to the seabed is processed by macrofauna, meiofauna, and microbes (Hicks et al., 2017; Middelburg, 2018), and eventually microbial oxidation converts the organic carbon into inorganic carbon, which is usually in dissolved form (Sun and Turchyn, 2014). Most of this will diffuse back into the water column, or form authigenic carbonate (Sun and Turchyn, 2014). Although inorganic carbon constitutes a significant amount within marine sediments, the relative contribution of authigenic carbonate formation is relatively unknown (Schrag et al., 2013; Sun and Turchyn, 2014).
The highest POC concentrations are generally associated with muddy sediments (de Haas et al., 1997). However, the greater spatial extent and greater dry bulk density of coarse grained sediments means that much of the POC stored on the shelf is in coarse-grained sediments (Diesing et al., 2017). The largest stock of POC per unit area is the Norwegian Trench, which stores between 54 and 120 mol m–2 of POC, while estimates of POC stock for other regions on the shelf range from approximately 12–60 mol m–2. The relatively deep Norwegian Trench forms a major sink for North Sea sediments because the lower current velocities and wave energy allow fine sediments to settle out of suspension (de Haas et al., 1997).
Estimates of benthic carbon stocks have a large associated uncertainty compared to the carbon stored in the overlying water column. This is largely due to the high spatial variability combined with a very large range in per-unit area carbon storage in the sediments (Figure 3) compared to the water column, where DIC is dominant and has a relatively constant and well constrained concentration. Future research should aim to characterize the sediment depth profile of organic and inorganic carbon in sediments across the shelf, to allow better quantification of carbon stocks and to better define the zone of active organic carbon degradation, below which carbon can be considered as removed from the active surficial carbon cycle (Keil, 2017). Considering the multiple influences affecting carbon cycling on the NWES (see section “Responses of Carbon Stocks and Flows on the NWES to Future Changes”), it is possible that benthic carbon stocks are changing spatially and temporally in response to anthropogenic influence. Carbon stocks and flows on the NWES may also be changing over much longer timescales; these shelf seas are the product of post-glacial sea level rise and relict terrestrial carbon in shelf sediments may be being slowly remineralized over millennial timescales (de Haas et al., 2002).
Both the pelagic and benthic carbon stocks are dominated by inorganic carbon, with organic compounds only accounting for roughly 5% of carbon stocks overall. A key difference between the pelagic and benthic stocks is the likely residence time of inorganic carbon. In the water column, much of the carbon is available for exchange with the atmosphere (i.e., in inorganic form) and shelf waters, and thus the carbon they contain, exchanges with the open ocean on the timescale of a few years. In the sediment however, the residence time of inorganic carbon is much longer. Most of the carbon exchange between water and sediment involves the deposition of POC, followed by its oxidation and release to the water as dissolved compounds (Krumins et al., 2013). By contrast, the flux of PIC to the seafloor is much smaller (Krumins et al., 2013; Burrows et al., 2017) but can remain in the sediment for centuries or millennia. It is important to note that over long timescales this PIC stock represents a large carbon store (subduction being the long-term sink for atmospheric CO2 in the global carbon cycle). However, the production of PIC indirectly results in release of CO2 to the atmosphere on short timescales (Frankignoulle et al., 1994), due to the consumption of alkalinity, and so PIC production also modulates the oceanic sink of CO2 (Shutler et al., 2013).
Previous research has focused on salt marshes and seagrass beds as these are considered particularly intense carbon sinks in temperate regions (Duarte et al., 2005; Nellemann et al., 2009; Luisetti et al., 2019). Awareness of the importance of these vegetated coastal habitats as carbon sources and sinks is growing, although they continue to be under-represented in large scale carbon budgets (Duarte, 2017).
Total coastal carbon stocks and flows in these habitats (Figure 4) are small compared to pelagic and benthic components (Figure 2). The vegetation and top 0.1 m of sediment in these habitats represent a stock of carbon of 0.7–3.4 Tmol. Salt marshes store more carbon per unit area than other coastal habitats and also bury the most carbon per unit area on an annual basis. Over the whole NWES, however, mud and sand flats contain more carbon due to their areal extent of between 1 × 1010 and 1.8 × 1010 m2. Seagrass beds store 35–64 mol C m–2 and take up between 0.7 and 7 mol m–2 from the water column annually. Their small total area means that seagrass beds make a small contribution to the coastal component of the NWES carbon budget.
Carbon stocks in kelp forests are small per unit area compared to other coastal habitats because kelp attach to hard substrata and sediments do not accumulate. Although their standing stock is relatively small, kelp species are highly productive, converting 10–100 moles of inorganic carbon into organic matter per square meter per year (Burrows et al., 2014). However, the proportion of this organic matter which is remineralized and released back into the water column compared to the proportion which is buried in the sediment is largely unknown. Organic matter from kelp forests is also exported to other coastal habitats where it can enter benthic carbon stocks though this process is still largely unconstrained given the limited availability of existing data (Krause-Jensen et al., 2018; Smale et al., 2018; Queirós et al., 2019). Without a better understanding of the fate of this organic matter, it is difficult to accurately quantify its contribution to the net coastal to pelagic carbon flux and the net burial of kelp in the sediment. Considering the area of kelp forests on the NWES of between 9.6 × 109 and 1.5 × 1010 m2 (based on Burrows et al., 2014), this represents a significant knowledge gap in the coastal carbon budget. The fate of primary production in coastal habitats as a whole is a key unknown – measurements of primary production alone do not provide information about how much of this fixed carbon is remineralized and how much is stored long-term in sediments.
The percentage uncertainties associated with the coastal carbon stocks are large compared to those associated with the pelagic and benthic components (Supplementary Material Part B). This is caused partly by uncertainty on the per unit area stock estimates in coastal habitats but is also due to uncertainty on the areal extent of these habitats across the NWES. More comprehensive mapping of these coastal habitats across the NWES would help to constrain the coastal carbon budget. However, the spatial heterogeneity of these habitats, and high levels of temporal variability across seasons in process rates (Thornton et al., 2002; Bohórquez et al., 2017) adds to the challenges of accurately quantifying shelf-wide carbon stocks and flows.
Although the total stocks and flows of carbon in coastal habitats may be small compared to the pelagic and benthic components, these coastal habitats play an important role in the biogeochemical and ecological functioning of the shelf sea system as a whole. Coastal habitats acts as nutrient sinks (Jickells et al., 2000; Nedwell et al., 2016), thereby reducing eutrophication in coastal seas as well as storing carbon. Coastal areas also provide important marine nurseries (Green et al., 2009) and directly benefit the adjacent human population through natural sea defense, recreation opportunities and fisheries (Turner et al., 2014).
Here we consider a range of potential influences on future carbon stocks and flows on the NWES. The likely effect of a range of future changes on coastal, benthic and pelagic carbon stocks and flows is summarized in Figure 6 and discussed in the following sections. Note that we do not discuss every potential influence, rather we focus on the 6 future changes likely to be most significant for each of coastal, benthic and pelagic systems.
Figure 6. The expected impact of key future changes on coastal, pelagic and benthic carbon stocks, considered in isolation to each other. Arrow direction indicates whether the stock in question is expected to increase or decrease given the influence on the left, and the color of the arrow represents the confidence we have in this expected change, with white, gray and black representing low, medium and high confidence, respectively. Question marks indicate that the stock is expected to change but the overall direction of the change is unknown. Influences which are directly manageable within the study region are shaded in blue. Only the 6 most important or potentially important influences (in the view of these authors) are considered for each system (benthic, pelagic and coastal).
Sea levels have risen on the NWES by an average of 0.1–0.2 cm year–1 over the course of the 20th Century (United Kingdom data, Horsburgh and Lowe, 2013) and rates of sea level rise are likely to increase over coming decades. Under a medium emissions scenario sea level is expected to rise by between 20 and 105 cm in many parts of the NWES coasts by 2100 (Lowe et al., 2009; Katsman et al., 2011).
Sea level rise can cause the erosion, drowning and loss of intertidal habitats, especially where these habitats are prevented from migrating laterally and vertically by, for example, hard sea defenses. Nearly all tidal salt marshes in Great Britain are expected to be in retreat by 2100 (Horton et al., 2018). Soft management options, which have been used increasingly over the past couple of decades, can slightly reduce the loss of area (Luisetti et al., 2013). A reduction in the area of salt marshes and mud flats will reduce the amount of carbon they sequester, and the erosion of sediment in these habitats may reduce their carbon stocks, although the preservation of these sediments depends on the rate of sea level rise (Andrews et al., 2012).
Given the estimates of habitat area losses due to sea level rise (Nottage and Robertson, 2005; Jones et al., 2011; Luisetti et al., 2019) we calculate a decrease in the combined burial of carbon by salt marshes, mud and sand flats, and seagrass beds of between 0.3 × 108 and 13 × 108 moles of carbon per year (Supplementary Material Part C). This estimate does not include a loss of carbon stock in these habitats. The loss of stocks is hard to predict but may be significant if sediments are eroded and remineralized as a result of sea level rise.
Globally, there has been an increase in riverine nutrient inputs to coastal oceans due to agricultural fertilizers and other anthropogenic sources since the onset of industrial agriculture (Jickells, 1998; Seitzinger et al., 2010; Bauer et al., 2013). In Europe however, riverine phosphate concentrations have decreased steadily since 1992, largely due to improvements in wastewater treatment, and nitrate inputs have stabilized and are likely to decrease in the future (Grimvall et al., 2000; European Environment Agency [EEA], 2015a). While the concentration of nutrients in rivers discharging into European shelf seas is directly manageable, the amount of runoff is determined by precipitation which is not manageable on a regional scale (McMellor and Underwood, 2014; Wakelin et al., 2015). Winter precipitation and river flows are expected to increase across northern Europe (European Environment Agency [EEA], 2015b), leading to increased discharge into the North Sea from rivers and the Baltic. The net effect of increasing runoff and decreasing nutrient concentrations on the total amount of nutrient input to the shelf is uncertain.
Changes in nutrient input are likely to cause changes in the uptake and storage of carbon through the impact on primary production and the resulting change in uptake of DIC, production of DOC and delivery of POC to the sea bed. This impact will be especially strong in near-shore waters and coastal habitats, near to the terrestrial point sources of nutrients. Outside of the highly productive coastal zone, most primary production on the NWES is thought to be driven by oceanic nutrient input (Holt et al., 2012; Große et al., 2017; Ruiz-Castillo et al., 2018). While most estuarine and coastal waters are net sources of CO2 to the atmosphere (Borges et al., 2005), high inorganic nutrient inputs can lead to partial inhibition of these emissions or even to coastal waters being a net sink of atmospheric CO2 (Kuwae et al., 2016). Studies of Dutch and Belgian coastal waters suggest that decreasing nutrient inputs from rivers, due to increased regulation of discharges since the 1980s, has led to reduced primary production and decreasing pH (Borges and Gypens, 2010; Provoost et al., 2010). A reduction in nutrient inputs is likely therefore to weaken the net uptake of atmospheric CO2 by the NWES, or conversely an increase in nutrient inputs may enhance carbon uptake, but the magnitude of these changes are highly uncertain.
Increasing nutrient inputs to the coastal seas is not a valid management technique because large amounts of greenhouse gases are released during the manufacture of fertilizers (e.g., Hasler et al., 2015), acting against any increase in the marine CO2 sink that might be caused by agricultural runoff. Intense near-shore productivity, associated with riverine nutrient inputs, is also associated with methane and N2O release (Upstill-Goddard, 2011; Bakker et al., 2013) so the net effect on climate of increased productivity related to riverine nutrients is unclear. Furthermore, eutrophication in coastal areas has several other negative impacts including reduced biodiversity and damage to recreation and coastal fisheries (Aertebjerg et al., 2001). Vegetated coastal habitats are especially vulnerable to elevated nutrients; seagrass beds and salt marshes can be damaged by algal growth caused by eutrophication, potentially reducing the ability of these habitats to store carbon long-term (Orth et al., 2006; Airoldi and Beck, 2007). Conversely, where eutrophication is reduced through management options, then productivity may decline, and species may change (Underwood and Kromkamp, 1999; McMellor and Underwood, 2014).
Changing riverine inputs may affect the ability of marine and coastal systems to sequester carbon, although the mechanisms involved are poorly understood and there are a lack of time series observations. Mechanisms include the effect of changing nutrient stoichiometry on the phytoplankton community (and how this in turn impacts higher trophic levels and carbon export); the impact of terrestrial organic matter C:N stoichiometry and the role of inorganic nitrogen and phosphorous in making terrestrial and marine refractory DOM bioavailable for breakdown (Bauer et al., 2013; Jiao et al., 2014; Chaichana et al., 2019); and the role of anoxic remineralization in eutrophied systems in adding alkalinity to the system and therefore resulting in a net uptake of atmospheric CO2 (Thomas et al., 2009).
Overall, it is likely that elevated nutrient inputs would lead to increased primary production and increased carbon burial on the shelf, and possibly affect off-shelf carbon transport. However, this simplistic view neglects the wider deleterious impacts of increased nutrient inputs to shelf seas.
The degradation of coastal habitats or conversion to other uses, such as agriculture or industry, potentially exposes stored organic carbon to oxygen, allowing microbial activity to release carbon. Habitat destruction therefore normally results in a decrease in carbon sequestration and decreased carbon stocks. Between 25 and 100% of carbon stock is lost when coastal habitats are destroyed or converted to other uses (Pendleton et al., 2012). Given this range and that of carbon stocks in coastal habitats (Figure 4), we calculate that the destruction of 1 ha of mud/sand flat, salt marsh or seagrass bed would reduce the carbon stocks by 1 × 105–1.4 × 106, 5 × 105–3.6 × 106, and 1 × 105–6 × 105 mol, respectively. Given that we only account for the top 0.1 m of sediment in this budget, these loss estimates are likely to be highly conservative.
Protection of coastal habitats therefore protects these carbon stocks and also maintains the flows of carbon in these habitats. A reduction in disturbance may allow greater biomass, potentially increasing both the standing stock of carbon and the storage of buried organic matter. Protection of coastal habitats also maintains the numerous co-benefits that they provide, such as natural sea defense, leading to reduced flood risk (Jones et al., 2011); social and recreation benefits; the provision of habitats which are important for many coastal species and the uptake of nutrients, especially nitrogen (Jickells et al., 2000; Nedwell et al., 2016). Coastal land use management can also include positive interventions around habitat (re)creation and restoration (Cousins et al., 2017). The riverine input of carbon and nutrients to coastal habitats is strongly influenced by land use management throughout the river catchment, especially urban development, agricultural practices, forestry and upstream dams (Pendleton et al., 2012).
The protection of areas further offshore is also likely to impact the carbon budget of the shelf and may have added value in conserving sediment carbon stocks (Avelar et al., 2017; Howard et al., 2017). While Marine Protected Areas (MPAs) are primarily designated to protect biodiversity through regulation of human activities, the reduction in benthic disturbance may also increase the carbon stock (see section “Trawling”). We have medium confidence that the existence of MPAs increases and protects carbon stocks, particularly inorganic carbon sediments relating to coral structures (Burrows et al., 2017). MPAs are manageable and of increasing spatial importance on the NWES. MPAs and Special Areas of Conservation (SACs) also provide a valuable monitoring opportunity because routine observations of carbon stocks and flows in protected and unprotected areas could help us to understand the impact of various direct influences, such as trawling, on the shelf sea carbon budget.
Atmospheric CO2 concentration is increasing at a rate of approximately 2 ppm year–1 and will likely continue to increase (Ciais et al., 2013). This influence is ubiquitous across the NWES and will affect pelagic, coastal and benthic carbon stocks and flows. This influence is not directly manageable at a local level; abatement of the rising CO2 trend requires international action.
Increasing atmospheric CO2 will increase pelagic carbon stocks, particularly in shelf seas which mix and ventilate annually. This is demonstrated by observations which show the concentration of DIC (the majority of the pelagic carbon stock) increasing in the North Sea over the last decade (Figure 7). Scaling the current rate of increase observed in the North Sea to the whole shelf gives an increase in the pelagic carbon stock of 0.3 Tmol year–1, which represents an approximate 0.15% increase in the pelagic stock. This long-term absorption of atmospheric CO2, and resultant increase in DIC is causing ocean acidification on the NWES. Over the last 30 years this has decreased pH by 0.0035 pH units per year in the North Sea (Ostle et al., 2016). Increasing DIC also increases the DIC:TA ratio, lowering the buffering capacity of seawater and reduces the potential for future CO2 uptake on the shelf (Thomas et al., 2007; Clargo et al., 2015).
Figure 7. Observations of increasing dissolved inorganic carbon concentration in summer in waters of the northern North Sea (orange) and southern North Sea (blue). Data 2001–2011 from Clargo et al. (2015) and from 2015 from Hartman et al. (2018). Vertical bars represent ± 1 standard deviation of the range of observed values across the survey areas. Much of the intra-annual variability represents spatial variability, and increases in the mean values through time is representative of the real increase in the DIC inventory in the region (Clargo et al., 2015).
The processes which transform DIC to organic carbon or transport it off the continental shelf, into the deep ocean, act to both ameliorate the effects of ocean acidification and control CO2 removal from the atmosphere. The magnitude of air-sea CO2 flux (Figure 2) is determined by the CO2 concentration difference and the rate of exchange at the air-water interface, so the future strength of this important CO2 sink depends not only on atmospheric CO2 but also on the biological and physical processes controlling and modulating CO2 in the water.
Increasing atmospheric CO2 could also have a range of large scale impacts on the benthic carbon budget, through changes in temperature, pH and oxygen. It is likely that the largest impact will be caused by changes in the amount of organic matter delivery to the bed from the water column. Not only would this impact benthic POC stocks but could also cause a release of alkalinity from the sea bed to the water column as an increase in POC deposition would increase PIC dissolution (Krumins et al., 2013).
Acidification of the overlying water is also likely to result in decreasing benthic calcium carbonate saturation states, leading to carbonate dissolution and TA release (Doney et al., 2009; Krumins et al., 2013). An increase in the benthic release of TA, relative to DIC, would increase the buffering capacity of seawater, increasing the potential of the water to take up atmospheric CO2 (Brenner et al., 2016) which could act as a short-term negative feedback on increasing atmospheric CO2. However, this TA-driven CO2 uptake would come at the expense of carbonate sediments which are slow to accumulate and currently represent the majority of benthic carbon stocks. Dissolution of carbonates and a reduction in the flux of inorganic carbon from the water column to the benthos (Burrows et al., 2017) may mean that benthic PIC stocks will decrease as atmospheric CO2 increases. Release of this carbon from long-term, secure stock into the DIC pool available for exchange with the atmosphere will likely contribute to global warming and ocean acidification in the long term by making more DIC available to drive higher stabilization concentrations in the atmosphere as CO2 emissions decrease, for instance.
The continued increase in atmospheric CO2 may lead to an enhancement of primary production in coastal habitats, which is likely to increase coastal carbon stocks. However, this impact is likely to be small and it is hard to quantify as our knowledge of the amount of organic matter which is stored long-term is poor. Ocean acidification may benefit some algae and other coastal plants (Artioli et al., 2014; Brodie et al., 2014; Celis-Plá et al., 2015), whilst having a generally negative effect on invertebrates and fish (Hennige et al., 2014). However, there is high variability in responses, with complex interactions (e.g., Duarte et al., 2016), and long-term impacts on carbon cycling and storage remain uncertain.
The impact of increasing atmospheric CO2 will be ubiquitous across the NWES but there remains large uncertainty on the overall direction of change in benthic carbon stocks. Changes to both POC and PIC deposition remain uncertain, as do the direct effects on the sea bed such as the response of benthic fauna to increasing CO2 and the dissolution of calcium carbonate sediments.
Storms have a range of effects which can affect the carbon budget of the NWES. These include increased turbulent mixing, rain inputs, storm surge inundation and biomass destruction associated with increased wave energy. Anthropogenic climate change is considered to be affecting the strength and location of the storms (Lowe et al., 2009; Shepherd, 2014) and current model simulations generally predict an increase in storm activity over north-west Europe (Harvey et al., 2012), although not all studies agree (Lowe et al., 2009; Tinker et al., 2016). Model results also suggest that precipitation will increase across NE Europe, especially Scandinavia (Alfieri et al., 2015), leading to increased discharge into the North Sea from rivers and the Baltic. The riverine input of carbon and nutrients to the marine environment is therefore likely to increase, especially DOC and POC (Bauer et al., 2013). Changes to large scale weather patterns are likely to affect the whole NWES and are not directly manageable at a local level.
Increased storminess would very likely result in the destruction of coastal biomass and reduced primary productivity (Nedwell et al., 2016), and may increase the erosion of sediment in coastal habitats (Jones et al., 2011), leading to a decrease in coastal carbon stocks. Remobilization of sediment may also reduce the effectiveness of some coastal habitats to act as sea defenses.
The location and strength of storms across NW Europe may impact the pelagic carbon budget in the coming century through their impact on mixing and air-sea gas exchange. Enhanced mixing of the water column may affect primary production by altering nutrient availability and light conditions, although the net effect remains unknown. During periods of low seawater DIC, increased surface turbulence may enhance the uptake of atmospheric CO2, increasing DIC stocks. However, increased mixing may also alter circulation and may inhibit the net sink of atmospheric CO2 by bringing high DIC water to the surface. Overall, the net impact of changing storminess on the pelagic carbon budget remains uncertain.
Between 1984 and 2014 water temperature in the seas around the United Kingdom increased at an average of 0.28°C decade–1, with considerable spatial and interannual variability within this trend (Hughes et al., 2017). By the end of the century (2069–2098), the NWES sea surface temperatures are projected to have warmed by 2.9°C (± 2σ = 0.82°C) compared to the recent past (1960–1989) under the A1B scenario (Tinker et al., 2016).
Increasing temperature is likely to influence coastal habitats in several ways. Primary productivity of coastal systems will increase with temperature up to an optimum (around 25°C for salt marshes and intertidal biofilms, e.g., Simas et al., 2001; van Colen et al., 2014). Higher temperature coupled with desiccation and salt stress, probably reduce both intertidal microalgal and salt marsh vascular productivity (Nedwell et al., 2016). Increasing temperature may also result in species shifts and increased rates of microbial degradation of organic matter. Macrophyte-based ecosystems such as seagrass meadows and kelp forests are particularly vulnerable to ocean warming (Ehlers et al., 2008; Raybaud et al., 2013; Arias-Ortiz et al., 2018). Overall, increasing temperature may decrease United Kingdom/European coastal carbon stocks (Nedwell et al., 2016), although the impacts on the various carbon stocks and flows are poorly understood.
There are several impacts of increasing temperature on the pelagic carbon budget. The dominant effect is expected to be the reduction in CO2 solubility, leading to a decrease in CO2 uptake and a decrease in DIC stock. An increase in temperature is also expected to increase the intensity of water column stratification across the shelf (Holt et al., 2016). Increasing stratification has competing effects on productivity but, overall, is expected to decrease primary production on the NWES due to decreasing nutrient availability, including input across the shelf break (Gröger et al., 2013). A decrease in primary production is likely to decrease the pelagic-benthic flux of POC and also decrease oceanic uptake of atmospheric CO2. Overall, increasing temperature is expected to decrease pelagic carbon stocks on the NWES but with significant uncertainty and regional variability (Holt et al., 2016).
A decrease in primary productivity and an increase in pelagic remineralization would result in a decreased delivery of POC to the seabed and decreased benthic carbon stocks. Some studies demonstrate a decrease in POC burial in sediments with warming (e.g., Holmer, 1996; Ståhlberg et al., 2006; Malinverno and Martinez, 2015), and this relationship is supported by modeling approaches (Boudreau, 2000; van der Molen et al., 2013). However, warming is also linked to deoxygenation, particularly in coastal areas (Diaz and Rosenberg, 2008), potentially enhancing carbon sequestration (see section “Decreasing Oxygen”). Overall, we expect that increasing temperature will decrease benthic carbon stocks.
Increasing sea temperatures in the region will also affect biodiversity and the depth and latitudinal distribution of marine species (e.g., Kovats et al., 2014), although the impact on the carbon budget of future changes to ecosystem structure and function as species distribution changes is unknown. Warming-induced changes to both pelagic and benthic plankton species composition, combined with ocean acidification, could change the amount of carbonate precipitation thereby affecting PIC stocks and flows. Our knowledge of benthic PIC stocks and flows is very limited (Figure 2), making it hard to predict future changes to this important part of the carbon budget.
Observations suggest that global oceanic oxygen concentrations have been decreasing over the past several decades (Helm et al., 2011; Schmidtko et al., 2017; Oschlies et al., 2018) and that this trend may be stronger in coastal regions (Gilbert et al., 2010). Models predict a continued decrease in seawater oxygen concentrations in the coming century due to increased stratification and decreasing solubility, caused by warming (Bopp et al., 2013).
In the seasonally stratified North Sea, oxygen depletion is known to vary seasonally and spatially and future oxygen trends are likely to be connected to increasing temperature, changes in primary production and increasing stratification duration (Greenwood et al., 2010; Queste et al., 2013, 2016; van der Molen et al., 2013; Ciavatta et al., 2016; Große et al., 2016). Due to the complex interactions between key physical and biological processes, trends in oxygen concentration in the waters of the NWES are uncertain but the expected increase in the duration of stratification (Lowe et al., 2009; Sharples et al., 2013) may cause a decrease in bottom water oxygen concentrations in some areas during summer although severe oxygen depletion events are likely to be transient and localized (Greenwood et al., 2010; Queste et al., 2013; Große et al., 2016).
It is probable that benthic carbon stocks will be affected by decreasing oxygen concentrations, directly though changes to sediment redox zonations (Sun et al., 1993; Dauwe et al., 2001; Neubacher, 2009), and indirectly by altering macrofaunal communities and their associated bioturbation (Sun and Dai, 2005). Even relatively small oxygen depletion in the overlying water can have a measurable effect on benthic biogeochemistry (Neubacher, 2009). Factors other than oxygenation also influence benthic carbon storage, including, amongst other things, the rate of supply from the overlying water column (Henrichs and Reeburgh, 1987), the biochemical composition (quality) of the organic matter (Eyre et al., 2013; Cavan et al., 2017), its absolute concentration (Mayor et al., 2012) and the characteristics of the receiving sediment (Hicks et al., 2017). Thus, the relationship between sediment oxygenation, carbon stocks and storage is complex (Canfield, 1994; Burdige, 2007; Arndt et al., 2013), and the impact not directly manageable at a local scale.
Bottom trawling is ubiquitous across the NWES and there are very few areas of the sea bed that can be considered as unaffected (Eigaard et al., 2017). The disturbance of the seabed by trawling has several opposing mechanisms of impact on the benthic carbon budget.
Resuspension of sediment by trawling decreases carbon storage (Oberle et al., 2016a), particularly in muddy sediments, causing an increase in benthic to pelagic carbon flux and water column remineralization (Jennings et al., 2001; Durrieu De Madron et al., 2005). Studies in the deep sea and NW Iberian shelf have demonstrated lower organic carbon content and lower 210Pb concentrations in surface sediments post trawling (Martín et al., 2014a; Oberle et al., 2016b). However, resuspension and remineralization of sediment also releases nutrients back to the water column, which may stimulate primary production and increase deposition of new POC (e.g., Duplisea et al., 2001). Repeated trawling will prolong sediment resuspension and may disrupt existing carbon storage (Martín et al., 2014a, b; Oberle et al., 2016b), as well as increase the likelihood of long-lasting change (Mayer et al., 1991; Jones, 1992) in carbon concentration and spatial distribution.
Loss of fauna may increase carbon storage through the reduction of carbon consumption by benthic biomass (Duplisea et al., 2001; van der Molen et al., 2013). However, an increase in bacterial remineralization following trawling may decrease benthic POC. Reduced infaunal densities would lead to a decrease in the mixing of sediments, and exchange of pore-water fluids across the sediment water interface due to decreased bioturbation and bioirrigation (Snelgrove et al., 2018). This, in turn, reduces the availability of dissolved oxygen within the sediments, consequently reducing organic carbon re-mineralization and release of DIC across the sediment water interface (Middelburg and Meysman, 2007; Snelgrove et al., 2018; Queirós et al., 2019).
The net effect of these processes is highly uncertain, and likely depends on several local as well as broader scale conditions, such as sediment type, gear type, currents, and seasonal timing of the events. While it is certain trawling has a significant impact on the benthic system, its net effect on carbon stocks and fluxes at shelf scale is highly uncertain due to the complex interacting processes as detailed above.
The extent and intensity of trawling is directly manageable and a reduction in trawling would have co-benefits such as the protection of habitats and species diversity. Importantly, the impact of trawling on benthic habitats is highly non-linear with trawling effort. Most damage to the biological organisms (from species level to community composition) is carried out by the first trawl, which may have implications for the regulation of the extent and frequency of trawling effort (Jennings et al., 2001).
The sea surface salinity of the NWES seas is predicted to decrease during this century (Holt et al., 2010; Tinker et al., 2016) and this freshening may impact the pelagic carbon budget. Temperature and salinity changes are predicted to cause an increase in stratification strength (Tinker et al., 2016) and duration (Holt et al., 2009) on the shelf which may impact primary production and therefore potentially alter air-sea CO2 flux and the POC flux to the benthos. A decrease in salinity may also be associated with a decrease in TA which would reduce the buffering capacity of seawater and reduce its capacity to take up atmospheric CO2. Overall the impact of future salinity changes on the carbon budget is unknown.
The large transport of carbon from the NW European shelf, into the deep North Atlantic (Figure 2) allows the shelf seas to be a net sink of atmospheric CO2. The strength of this off-shelf carbon transport is largely determined by cross-shelf water mass exchange so changes to circulation in the region could lead to major changes in the carbon budget. Models predict a weakening of the shelf edge current, especially in the eastward circulation in the northern North Sea (Holt et al., 2010; Tinker et al., 2016) and it is possible that this weakened circulation may reduce the strength of off-shelf carbon transport. However, recent work has highlighted that models with spatial resolutions of the order of 1 km are needed to resolve key processes controlling cross-shelf exchange (Graham et al., 2018), and there is a lack of suitable synoptic scale observations for verifying such models (Bôas et al., 2019).
It is thought that inter-annual variability caused by the North Atlantic Oscillation (NAO) leads to variability in the North Atlantic carbon sink (e.g., Gruber et al., 2002; Schuster and Watson, 2007) and circulation changes caused by the NAO are also likely to impact the carbon budget on the NWES. Salt et al. (2013) found that under more positive NAO conditions, the anti-clockwise circulation of the North Sea is stronger, with reduced mixing between the southern and northern regions. These circulation changes cause the shelf sea carbon pump to be stronger during positive NAO conditions. NAO conditions are difficult to predict and can currently only be reliably modeled a year or two in advance (Dunstone et al., 2016), making the future influence of the NAO on the carbon budget uncertain. Overall, we are confident that future changes in circulation will lead to major changes in the carbon budget, but we cannot predict with confidence what these changes will be.
We have constructed a carbon budget for the northwest European continental shelf seas, synthesizing available estimates for all carbon stocks and flows. Key knowledge gaps in the carbon budget were identified (Figure 5) and future impacts on the pelagic, benthic and coastal components of the carbon budget were assessed. The main points arising from this study are:
• Carbon Stocks and Flows on the NWES Are Globally Significant
The NWES stores between 730 – 1800 Tmol of carbon, of which 71–87% reside in the shelf sediments, 13–29% in the water and 0.1–0.2% in coastal habitats. Benthic and pelagic macrofauna account for only 0.1–0.6% of total carbon stocks, although they may play important roles in carbon cycling and storage processes (e.g., Lutz and Martin, 2014; Atwood et al., 2015). The budget presented here identifies that the NWES riverine input, air-sea exchange and off shelf transport respectively account for 3.2–7.1, 3.5–8.8, and 4.9–12% of the global shelf carbon fluxes given by Bauer et al. (2013). Given that the NWES occupies about 3.5% of total global continental shelf area, this suggests that it may be above average in its role in carbon cycling and storage.
According to this budget, approximately 60% (between 56 and 63%) of the carbon entering the NWES is delivered by rivers and approximately 40% (between 37 and 44%) is from the atmosphere. These riverine and atmospheric proportions are very similar to those of recent carbon budgets of the east coast of North America (Najjar et al., 2018) and the whole North American shelf (Fennel et al., 2019), despite very different geographies and latitudinal ranges of the three study areas.
• NWES Carbon Flows Are Significant in Comparison With Regional Anthropogenic Emissions
To appreciate the scale of the flows of carbon, we can compare them with a direct impact of humans on the carbon system – our CO2 emissions. The total EU carbon dioxide emission in 2016 (including international aviation) is of the order of 100 Tmol year–1 (Eurostat, 2018). The uptake of atmospheric CO2 by coastal and marine systems of the NWES is thus between 1.3 and 3.5% of the magnitude of EU emissions, and the output to the North Atlantic is between 2.3 and 8.2% of the magnitude of EU emissions. The lower end of the range for shelf sea CO2 uptake is comparable to CO2 emissions from domestic combustion in the United Kingdom of 1.4 Tmol year–1 (National Atmospheric Emissions Inventory [NAEI], 2018).
• Carbon in Shelf Sediments Is the Largest and Most Uncertain Stock, and Is Potentially Manageable, Making It a Priority for Research
The largest carbon stock on the shelf is in the sediment (Figure 2) but this component of the budget also has the largest uncertainties. Carbon stock observations are scarce and estimates are limited by assumptions made about the depth of sediment considered, which does not always reflect the different sediment accumulation rates, and there is very little empirical data on this across the NWES. Furthermore, these large but poorly constrained benthic carbon stocks may be significantly impacted in the future by factors which are manageable on a local scale, such as trawling and the designation of protected areas (Figure 6). This contrasts with the pelagic component, where the large DIC stocks will be primarily influenced by large-scale changes such as CO2 increase (and associated acidification), warming and circulation changes, which are not locally manageable. Given their size, uncertainty and potential manageability, benthic carbon stocks stand out as a key area for future research.
• Understanding the Balance Between Off-Shelf Carbon Flux and Burial in Shelf Sediments Is Critical
Off-shelf carbon transport accounts for between 60 and 100% of carbon outputs from the NWES and burial in sediment accounts for between 0 and 40%. These values are consistent with the percentage contributions of 80 and 20% for off-shelf transport and sediment burial, respectively, presented by Najjar et al. (2018) for eastern North America. Fennel et al. (2019) find a roughly 70%/30% split between their inferred off-shelf transport and burial of carbon in sediments across the whole North American shelf.
More work is needed to understand the mechanisms behind both cross-shelf carbon transport and the burial of carbon in the sea bed, and to resolve their relative importance in driving the uptake of atmospheric CO2. Both of these fluxes are likely to be impacted by future influences to the NWES, but whereas cross-shelf exchange will be mostly affected by large-scale climatic changes, pelagic-benthic exchange will also be influenced by regional change and national management decisions. Better quantification of these two fluxes may therefore inform land and ocean management decisions.
• The Pelagic System Drives Carbon Uptake, Burial and Export but the Benthic System Is the Major Carbon Store
The two largest stocks of carbon in the NWES are inorganic carbon in the pelagic (DIC) and benthic (PIC). These stocks differ in their role in the shelf carbon cycle and their lifetimes and origin. The lifetime of pelagic DIC is of the order of a few years and is controlled by the flushing time of the shelf (Holt et al., 2012). DIC concentrations are dominated by the input from the open ocean and fluctuations around this “baseline” DIC concentration of ∼2050 μmol kg–1 due to in situ processes drive uptake of atmospheric CO2. The resulting enrichment in DIC of a few percent contributes to the export of carbon off the shelf. DOC stocks are much smaller but show greater variability and may play an important role in off-shelf export (Carr et al., 2018; Humphreys et al., 2018; Chaichana et al., 2019). The pelagic system is therefore important in the shelf carbon cycle due to the processes which fix carbon from the atmosphere and physical circulation which removes this carbon to the open ocean, where it can be stored for centuries or longer.
Compared to DIC in the water, PIC in the sediment has a very long residence time. This very large, long-lived store of carbon will become more vulnerable to dissolution as ocean acidification and temperature- and stratification-driven oxygen depletion increase while benthic DOC stocks will probably become more stable. Dissolution of carbonates, and a reduction in their production and deposition may initially act to increase atmospheric CO2 uptake and ameliorate ocean acidification but at the expense of slow-to-accumulate benthic PIC stocks. Therefore, being able to predict the nature and rate of the response of benthic carbon storage to ocean acidification is a future research priority.
• Inorganic Carbon Dominates Stocks but the Role of Biology Is Critical to Carbon Sequestration
PIC and DIC dominate the carbon stocks in the benthic and pelagic budgets, respectively. The major carbon-sequestering fluxes in the budget are mediated by biological activity. Organic carbon is directly sequestered as POC in sediments where a proportion remains for the long term. Biological activity also enriches the pelagic system in DOC or DIC (the latter through remineralization of organic carbon) which is then transported off the shelf. Although small relative to inorganic carbon, pelagic and benthic organic carbon stocks are still large relative to coastal stocks.
• Carbon Storage Is Sensitive to Natural Variability as Well as Human Activities
Whilst this budget aims to capture annual average flows, there is strong and asymmetrical seasonality in many of the flows (for instance atmospheric CO2 uptake), and changes in seasonality may have significant effects on overall carbon storage. Many fluxes also exhibit significant interannual variability, for example river discharge, atmospheric uptake and cross-shelf exchange are known to be linked to climate indices such as the North Atlantic Oscillation. Furthermore, the stocks quantified here may not be at steady state on centennial to millennial timescales and in the case of sedimentary carbon stores, in both shelf and coastal environments, the direction of the long-term natural trend is unclear. For instance, shelf sediments in the North Sea may be slowly accumulating carbon produced in the pelagic, while estuaries may be slowly releasing Holocene (or older) terrestrial organic carbon deposits (Andrews et al., 2000). It is particularly difficult to resolve this given that our data on carbon stocks and storage rates are made in a system heavily perturbed by trawling and climate pressure.
• The Future of the NWES Carbon Sink Remains Uncertain
Despite the importance of the NWES as a sink of atmospheric CO2 and despite considerable research effort, there remain several key gaps in our knowledge of the carbon budget (Figure 5). It remains uncertain whether the strength of the shelf sea carbon sink will continue to increase over the coming decades as this complex biogeochemical system is being significantly perturbed by multiple, interacting influences. We suggest that there is no large-scale biogeochemical basis on which we can say that the sink strength will increase or decrease.
• Marine Carbon Science and Management Are International Issues
The carbon stocks and flows quantified in this budget cross geopolitical boundaries and therefore the material and processes which are present in a nation state’s territory cannot be considered in isolation. International scientific collaboration is needed to tackle these challenges. Furthermore we expect most perturbations to the carbon budget of the NWES in the future to be caused by global-scale changes which are only manageable at an international level (Figure 6). Therefore, global governance and international cooperation potentially have more leverage on climate regulation by shelf sea systems than local management (e.g., Turner et al., 2014).
• Care Must be Taken to Consider Marine Ecosystems Holistically
As part of the natural world, humans are inextricably connected to, and supported by, natural ecosystems. While the discretization and quantification of nature may be used as a decision making tool, the breadth of value provided by natural ecosystems to humanity cannot be comprehensively measured in units of carbon or of money.
All datasets generated for this study are included in the article/Supplementary Material.
OL and MJ conceived the study and led the manuscript preparation. NH, TJ, MJ, and OL led the data compilation and synthesis effort. MD undertook the analysis of benthic carbon stocks. All authors contributed to the data synthesis, manuscript preparation, and editing.
This work was supported by the jointly funded NERC-Defra Shelf Sea Biogeochemistry research program, through grant awards (NE/K00168X/1, NE/K001698/1, NE/K001701/1, NE/K001833/2, NE/K001833/1, NE/K001914/1, NE/K001922/1, NE/K001957/1, NE/K001973/1, NE/K002007/1, NE/K002015/1, NE/K002015/2, and NE/K002058/1) to several of the authors and other NERC-funded activities (NE/N007999/1 and NE/N018087/1). MB was supported by the NERC-Defra funded NE/L003058/1, “MERP: Integrated Macroecology and Modeling to Elucidate Regulation of Services from Ecosystems.” JT was supported by the Met Office Hadley Centre Climate Programme funded by BEIS and Defra. Data from L4 were acquired under the NERC-DEFRA Marine Ecosystems Research Program (NE/L003279/1) and the NERC National Capability Western Channel Observatory.
The authors declare that the research was conducted in the absence of any commercial or financial relationships that could be construed as a potential conflict of interest.
We recognize and thank the many hundreds of researchers that have contributed data to the many large datasets that have fed, directly or indirectly via other publications, into this work. In particular this work would not have been possible without the many researchers and funding agencies responsible for the collection of data and quality control of the Surface Ocean CO2 Atlas (SOCAT). We are grateful to Sonja van Leeuwen for her advice on riverine inputs to the NWES. Figures 2, 4, 5 were illustrated by Simon Riviere – many thanks for his skill and patience in presenting the data in a fun and accessible way.
The Supplementary Material for this article can be found online at: https://www.frontiersin.org/articles/10.3389/fmars.2020.00143/full#supplementary-material
Abril, G., Noguiera, M., Etcheber, H., Cabecades, G., Lemaire, E., and Brogueira, M. J. (2002). Behaviour of organic carbon in nine contrasting estuaries. Estuar. Coast. Shelf Sci. 54, 241–262. doi: 10.1006/ecss.2001.0844
Adams, C. A., Andrews, J. E., and Jickells, T. (2012). Nitrous oxide and methane fluxes vs. carbon, nitrogen and phosphorous burial in new intertidal and saltmarsh sediments. Sci. Total Environ. 434, 240–251. doi: 10.1016/j.scitotenv.2011.11.058
Aertebjerg, G., Carstensen, J., Dahl, K., Hansen, J., Nygaard, K., Rygg, B., et al. (2001). Eutrophication in Europe’s Coastal Waters. Copenhagen: European Environment Agency (EEA).
Airoldi, L., and Beck, M. (2007). Loss, status and trends for coastal marine habitats of Europe. Oceanogr. Mar. Biol. Annu. Rev. 45, 345–405. doi: 10.1201/9781420050943.ch7
Aldridge, J. N., Lessin, G. L., Amoudtry, L. O., Hicks, N., Hull, T., Klar, J. K., et al. (2017). Comparing benthic biogeochemistry at a sandy and a muddy site in the Celtic Sea using a model and observations. Biogeochemistry 135, 155–182. doi: 10.1007/s10533-017-0367-0
Alfieri, L., Burek, P., Feyen, L., and Forzieri, G. (2015). Global warming increases the frequency of river floods in Europe. Hydrol. Earth Syst. Sci. 19, 2247–2260. doi: 10.5194/hess-19-2247-2015
Allen, S. E., and Durrieu De Madron, X. (2009). A review of the role of submarine canyons in deep-ocean exchange with the shelf. Ocean Sci. 5, 607–620. doi: 10.5194/os-5-607-2009
Andrews, J. E., Jickells, T. D., Adams, C. A., Parkes, D. J., and Kelly, S. D. (2012). “Sediment record and storage of organic carbon and the nutrient elements (N, P, Si) in estuaries and near coastal seas. Chapter 4.2,” in Treatise on Estuarine and Coastal Science, Vol. 4, eds E. Wolanski McLusky (Waltham: Academic Press), 9–38. doi: 10.1016/B978-0-12-374711-2.00402-2
Andrews, J. E., Samways, G., Dennis, P. F., and Maher, B. A. (2000). “Origin, abundance and storage of organic carbon and sulphur in the Holocene Humber estuary: emphasizing human impact on storage changes,” in Holocene Land–Ocean Interaction and Environmental Change Around the North Sea, eds I. Shennan and J. E. Andrews (London: Geological Society), 145–170. doi: 10.1144/gsl.sp.2000.166.01.09
Araujo, R. M., Assis, J., Aguillar, R., Airoldi, L., Barbara, I., Bartsch, I., et al. (2016). Status, trends and drivers of kelp forests in Europe: an expert assessment. Biodivers. Conserv. 25, 1319–1348. doi: 10.1007/s10531-016-1141-7
Arias-Ortiz, A., Serrano, O., Masqué, P., Lavery, P. S., Mueller, U., Kendrick, G. A., et al. (2018). A marine heatwave drives massive losses from the world’s largest seagrass carbon stocks. Nat. Clim. Chang. 8, 338–344. doi: 10.1038/s41558-018-0096-y
Arndt, S., Jørgensen, B. B., La Rowe, D. E., Middelburg, J. J., Pancost, R. D., and Regnier, P. (2013). Quantifying the degradation of organic matter in marine sediments: a review and synthesis. Earth Sci. Rev. 123, 53–86. doi: 10.1016/j.earscirev.2013.02.008
Artigas, F., Young, J., Hobble, C., Marti-donati, A., Schäfer, K. V. R., and Pechmann, I. (2015). Long term carbon storage potential and CO2 sink strength of a restored salt marsh in New Jersey. Agric. For. Meteorol. 200, 313–321. doi: 10.1016/j.agrformet.2014.09.012
Artioli, Y., Blackford, J. C., Butenschön, M., Holt, J. T., Wakelin, S. L., Thomas, H., et al. (2012). The carbonate system in the North Sea: sensitivity and model validation. J. Mar. Syst. 10, 1–13. doi: 10.1016/j.jmarsys.2012.04.006
Artioli, Y., Blackford, J. C., Nondal, G., Bellerby, R. G. J., Wakelin, S. L., Holt, J. T., et al. (2014). Heterogeneity of impacts of high CO2 on the North Western European Shelf. Biogeosciences 11, 601–612. doi: 10.5194/bg-11-601-2014
Atwood, T. B., Connolly, R. M., Ritchie, E. G., Lovelock, C. E., Heithaus, M. R., Hays, G. C., et al. (2015). Predators help protect carbon stocks in blue carbon ecosystems. Nat. Clim. Chang. 5, 1038–1045. doi: 10.1038/nclimate2763
Avelar, S., Voort, T. S., and Eglinton, T. I. (2017). Relevance of carbon stocks of marine sediments for national greenhouse gas inventories of maritime nations. Carbon Balance Manag. 12:10. doi: 10.1186/s13021-017-0077-x
Bakker, D. C. E., Bange, H. W., Gruber, N., Johannessen, T., Upstill-Goddard, R. C., Borges, A. V., et al. (2013). “Air-sea interactions of natural long-lived greenhouse gases (CO2, N2O, CH4) in a changing climate,” in Ocean-Atmosphere Interactions of Gases and Particles, eds P. Liss and M. Johnson (Berlin: Springer), doi: 10.1007/978-3-642-25643-1_3
Bakker, D. C. E., Pfeil, B., Landa, C. S., Metzl, N., O’Brien, K. M., Olsen, A., et al. (2016). A multi-decade record of high quality fCO2 data in version 3 of the Surface Ocean CO2 Atlas (SOCAT). Earth Syst. Sci. Data 8, 383–413.
Barillé-Boyer, A. L., Barillé, L., Massé, H., Razet, D., and Héral, M. (2003). Correction for particulate organic matter as estimated by loss on ignition in estuarine ecosystems. Estuar. Coast. Shelf Sci. 58, 147–153. doi: 10.1016/S0272-7714(03)00069-6
Bauer, J. E., Cai, W. J., Raymond, P. A., Bianchi, T. S., Hopkinson, C. S., and Regnier, P. A. (2013). The changing carbon cycle of the coastal ocean. Nature 504, 61–70. doi: 10.1038/nature12857
Beaumont, N. J., Jones, L., Garbutt, A., Hansom, J. D., and Tobermann, M. (2014). The value of carbon sequestration and storage in coastal habitats. Estuar. Coast. Shelf Sci. 137, 32–40. doi: 10.1016/j.ecss.2013.11.022
Bôas, A. B. V., Ardhuin, F., Ayet, A., Bourassa, M. A., Brandt, P., Chapron, B., et al. (2019). Integrated observations of global surface winds, currents, and waves: requirements and challenges for the next decade. Front. Mar. Sci. 6:425. doi: 10.3389/fmars.2019.00425
Bohórquez, J., McGenity, T. J., Papaspyrou, S., García-Robledo, E., Corzo, A., and Underwood, G. J. (2017). Different types of diatom-derived extracellular polymeric substances drive changes in heterotrophic bacterial communities from intertidal sediments. Front. Microbiol. 8:245. doi: 10.3389/fmicb.2017.00245
Boorman, L. (2003). Salt Marsh Review: An Overview of Coastal Salt Marshes, their Dynamic and Sensitivity Characteristics for Conservation and Management. JNCC Report No. 334. Peterborough: JNCC Peterborough.
Bopp, L., Resplandy, L., Orr, J. C., Doney, S. C., Dunne, J. P., Gehlen, M., et al. (2013). Multiple stressors of ocean ecosystems in the 21st century: projections with CMIP5 models. Biogeosciences 10, 6225–6245. doi: 10.5194/bg-10-6225-2013
Borges, A. V., Delille, B., and Frankignoulle, M. (2005). Budgeting sinks and sources of CO2 in the coastal ocean: diversity of ecosystems counts. Geophys. Res. Lett. 32:L14601. doi: 10.1029/2005GL023053
Borges, A. V., and Gypens, N. (2010). Carbonate chemistry in the coastal zone responds more strongly to eutrophication than to ocean acidification. Limnol. Oceanogr. 55, 346–353. doi: 10.4319/lo.2010.55.1.0346
Borges, A. V., Schiettecatte, L.-S., Abril, G., Delille, B., and Gazeau, F. (2006). Carbon dioxide in European coastal waters. Estuar. Coast. Shelf Sci. 70, 375–387. doi: 10.1016/j.ecss.2006.05.046
Boudreau, B. P. (2000). The mathematics of early diagenesis: from worms to waves. Rev. Geophys. 38, 389–416. doi: 10.1029/2000rg000081
Boudreau, B. P., Huettel, M., Forster, S., Jahnke, R. A., McLachlan, A., Middelburg, J. J., et al. (2001). Permeable marine sediments: overturning an old paradigm. EOS 82, 133–140.
Bozec, Y., Thomas, H., Elkalay, K., and de Baar, H. J. W. (2005). The continental shelf pump for CO2 in the North Sea—evidence from summer observation. Mar. Chem. 93, 131–147. doi: 10.1016/j.marchem.2004.07.006
Brenner, H., Braeckman, U., Le Guitton, M., and Meysman, F. J. R. (2016). The impact of sedimentary alkalinity release on the water column CO2 system in the North Sea. Biogeosciences 13, 841–863. doi: 10.5194/bg-13-841-2016
Brodie, J., Williamson, C. J., Smale, D. A., Kamenos, N. A., Mieszkowska, N., Santos, R., et al. (2014). The future of the northeast Atlantic benthic flora in a high CO2 world. Ecol. Evol. 4, 2787–2798. doi: 10.1002/ece3.1105
Buitenhuis, E., Van Bleijswijk, J., Bakker, D., and Veldhuis, M. (1996). Trends in inorganic and organic carbon in a bloom of Emiliania huxleyi in the North Sea. Mar. Ecol. Prog. Ser. 143, 271–282. doi: 10.3354/meps143271
Burdige, D. J. (2005). Burial of terrestrial organic matter in marine sediments: a re-assessment. Glob. Biogeochem. Cycles 19:GB4011. doi: 10.1029/2004GB002368
Burdige, D. J. (2007). Preservation of organic matter in marine sediments: Controls, mechanisms, and an imbalance in sediment in sediment organic carbon budgets? Chem. Rev. 107, 467–485. doi: 10.1021/cr050347q
Burrows, M. T., Hughes, D. J., Austin, W. E. N., Smeaton, C., Hicks, N., Howe, J. A., et al. (2017). Assessment of Blue Carbon Resources in Scotland’s Inshore Marine Protected Area Network. Scottish Natural Heritage Commissioned Report No. 957. St Andrews: University of St Andrews.
Burrows, M. T., Kamenos, N. A., Hughes, D. J., Stahl, H., Howe, J. A., et al. (2014). Assessment of Carbon Budgets and Potential Blue Carbon Stores in Scotland’s Coastal and Marine Environment. Scottish Natural Heritage Commissioned Report No 761. St Andrews: University of St Andrews.
Burt, W. J., Thomas, H., Pätsch, J., Omar, A. M., Schrum, C., Daewel, U., et al. (2014). Radium isotopes as a tracer of sediment-water column exchange in the North Sea. Glob. Biogeochem. Cycles 28, 786–804. doi: 10.1002/2014GB004825
Buscail, R., and Germain, C. (1997). Present-day organic matter sedimentation on the NW Mediterranean margin: Importance of off-shelf export. Limnol. Oceanogr. 42, 217–229. doi: 10.4319/lo.1997.42.2.0217
Butenschön, M., Clark, J., Aldridge, J. N., Allen, J. I., Artioli, Y., Blackford, J., et al. (2016). ERSEM 15.06: a generic model for marine biogeochemistry and the ecosystem dynamics of the lower trophic levels. Geosci. Model Dev. 9, 1293–1339. doi: 10.5194/gmd-9-1293-2016
Cai, W.-J. (2011). Estuarine and coastal ocean carbon paradox: CO2 sinks or sites of terrestrial carbon incineration? Annu. Rev. Mar. Sci. 3, 123–145. doi: 10.1146/annurev-marine-120709-142723
Cai, W.-J., Hu, X., Huang, W. J., Jiang, L. Q., Wang, Y., Peng, T.-H., et al. (2010). Alkalinity distribution in the western North Atlantic Ocean margins. J. Geophys. Res. 115, 1–15. doi: 10.1029/2009JC005482
Canfield, D. (1994). Factors influencing organic carbon preservation in marine sediments. Chem. Geol. 114, 315–329. doi: 10.1016/0009-2541(94)90061-2
Carr, N., Davis, C. E., Blackbird, S., Daniels, L. R., Preece, C., Woodward, M., et al. (2018). Seasonal and spatial variability in the optical characteristics of DOM in a temperate shelf sea. Prog. Oceanogr. 177:101929. doi: 10.1016/j.pocean.2018.02.025
Cavan, E. L., Trimmer, M., Shelley, F., and Sanders, R. (2017). Remineralization of particulate organic carbon in an ocean oxygen minimum zone. Nat. Commun. 8:14847. doi: 10.1038/ncomms14847
Celis-Plá, P. S., Hall-Spencer, J. M., Horta, P. A., Milazzo, M., Korbee, N., Cornwall, C. E., et al. (2015). Macroalgal responses to ocean acidification depend on nutrient and light levels. Front. Mar. Sci. 2:26. doi: 10.3389/fmars.2015.00026
Chaichana, S., Jickells, T., and Johnson, M. (2019). Interannual variability in the summer dissolved organic matter inventory of the North Sea: implications for the continental shelf pump, 2019. Biogeosciences 16, 1073–1096. doi: 10.5194/bg-16-1073-2019
Chen, C. A., and Borges, A. V. (2009). Reconciling opposing views on carbon cycling in the coastal ocean: continental shelves as sinks and near-shore ecosystems as sources of atmospheric CO2. Deep. Sea Res. 56, 578–590. doi: 10.1016/j.dsr2.2008.12.009
Ciais, P., Sabine, C., Bala, G., Bopp, L., Brovkin, V., Canadell, J., et al. (2013). “Carbon and other biogeochemical cycles,” in Climate Change 2013: The Physical Science Basis. Contribution of Working Group I to the Fifth Assessment Report of the Intergovernmental Panel on Climate Change, eds T. F. Stocker, D. Qin, G.-K. Plattner, M. Tignor, S. K. Allen, and J. Boschung et al. (Cambridge: Cambridge University Press), 465–570.
Ciavatta, S., Kay, S., Saux-Picart, S., Butenschon, M., and Allen, J. I. (2016). Decadal reanalysis of biogeochemical indicators and fluxes in the North West European shelf-sea ecosystem. J. Geophys. Res. Oceans 121, 1824–1845. doi: 10.1002/2015jc011496
Clargo, N. M., Salt, L. A., Thomas, H., and de Baar, H. J. W. (2015). Rapid increase of observed DIC and pCO2 in the surface waters of the North Sea in the 2001-2011 decade ascribed to climate change superimposed by biological processes. Mar. Chem. 177, 566–581. doi: 10.1016/j.marchem.2015.08.010
Cousins, L. J., Cousins, M. S., Gardiner, T., and Underwood, G. J. C. (2017). Factors influencing the initial establishment of salt marsh vegetation on engineered sea wall terraces in south east England. Ocean Coast. Manag. 143, 96–104. doi: 10.1016/j.ocecoaman.2016.11.010
Dauwe, B., Middelburg, J. J., and Herman, P. M. J. (2001). Effect of oxygen on the degradability of organic matter in subtidal and intertidal sediments of the North Sea area. Mar. Ecol. Prog. Ser. 215, 13–22. doi: 10.3354/meps215013
Davidson, N. C., Laffoley, D., Doody, J. P., Way, L. S., Gordon, J., Key, R., et al. (1991). Nature conservation and estuaries in Great Britain. Peterborough: Nature Conservancy Council.
Davis, C. E., Blackbird, S., Wolff, G., Woodward, M., and Mahaffey, C. (2019). Seasonal organic matter dynamics in a temperate shelf sea. Prog. Oceanogr. 177:101925. doi: 10.1016/j.pocean.2018.02.021
de Beer, D., Wenzhöfer, F., Ferdelman, T. G., Boehme, S. E., Huettel, M., van Beusekom, J. E. E., et al. (2005). Transport and mineralization rates in North Sea sandy intertidal sediments, Sylt-Rømø Basin, Wadden Sea. Limnol. Oceanogr. 50, 113–127. doi: 10.4319/lo.2005.50.1.0113
de Haas, H., Boer, W., and van Weering, T. C. E. (1997). Recent sedimentation and organic carbon burial in a shelf sea: the North Sea. Mar. Geol. 144, 131–146. doi: 10.1016/s0025-3227(97)00082-0
de Haas, H., van Weering, T. C. E., and de Stigter, H. (2002). Organic carbon in shelf seas: sinks or sources, processes and products. Cont. Shelf Res. 22, 691–717. doi: 10.1016/s0278-4343(01)00093-0
de Wilde, P. A. W. J., Berghuis, E. M., and Kok, A. (1986). Biomass and activity of benthic fauna on the fladen ground (northern North Sea). Netherlands J. Sea Res. 20, 313–323. doi: 10.1016/0077-7579(86)90053-0
Diaz, R. J., and Rosenberg, R. (2008). Spreading dead zones and consequences for marine ecosystems. Science 321, 926–929. doi: 10.1126/science.1156401
Diesing, M., Kröger, S., Parker, R., Jenkins, C., Mason, C., and Weston, K. (2017). Predicting the standing stock of organic carbon in surface sediments of the North–West European continental shelf. Biogeochemistry 135, 183–200. doi: 10.1007/s10533-017-0310-4
Dijkema, K. S. (1991). Towards a Habitat Map of The Netherlands, German and Danish Wadden Sea. Ocean Shorel. Manag. 16, 1–21. doi: 10.1016/0951-8312(91)90036-2
Doney, S. C., Fabry, V. J., Feely, R. A., and Kleypas, J. A. (2009). Ocean acidification: the other CO2 problem. Ann. Rev. Mar. Sci. 1, 169–192. doi: 10.1146/annurev.marine.010908.163834
Duarte, C., López, J., Benítez, S., Manríquez, P. H., Navarro, J. M., Bonta, C. C., et al. (2016). Ocean acidification induces changes in algal palatability and herbivore feeding behavior and performance. Oecologia 180, 453–462. doi: 10.1007/s00442-015-3459-3
Duarte, C. M. (2017). Reviews and syntheses: hidden forests, the role of vegetated coastal habitats in the ocean carbon budget. Biogeosciences 14, 301–310. doi: 10.5194/bg-14-301-2017
Duarte, C. M., Middelburg, J. J., and Caraco, N. (2005). Major role of marine vegetation on the oceanic carbon cycle. Biogeosciences 2, 1–8. doi: 10.1371/journal.pone.0052932
Dunstone, N., Smith, D., Scaife, A., Hermanson, L., Eade, R., Robinson, N., et al. (2016). Skilful predictions of the winter North Atlantic Oscillation one year ahead. Nat. Geosci. 9, 809–814. doi: 10.1038/ngeo2824
Duplisea, D. E., Jennings, S., Malcolm, S. J., Parker, R., and Sivyer, D. B. (2001). Modelling potential impacts of bottom trawl fisheries on soft sediment biogeochemistry in the North Sea. Geochem. Trans. 2:112. doi: 10.1186/1467-4866-2-112
Durrieu De Madron, X., Ferré, B., Le Corre, G., Grenz, C., Conan, P., Buscail, R., et al. (2005). Trawling-induced resuspension and dispersal of muddy sediments and dissolved elements in the Gulf of Lion (NW Mediterranean). Cont. Shelf Res. 25, 2387–2409. doi: 10.1016/j.csr.2005.08.002
Ehlers, A., Worm, B., and Reusch, T. B. H. (2008). Importance of genetic diversity in eelgrass Zostera marina for its resilience to global warming. Mar. Ecol. Prog. Ser. 355, 1–7. doi: 10.3354/meps07369
Eigaard, O. R., Bastardie, F., Hintzen, N. T., Buhl-Mortensen, L., Buhl-Mortensen, P., Catarino, R., et al. (2017). The footprint of bottom trawling in European waters: distribution, intensity, and seabed integrity. ICES J. Mar. Sci. 74, 847–865. doi: 10.1093/icesjms/fsw194
Essink, K., Dettmann, C., Farke, H., Laursen, K., Lüerßen, G., Marencic, H., et al. (2005). Wadden Sea Ecosystem No. 19. Wilhelmshaven: Common Wadden Sea Secretariat.
European Environment Agency [EEA] (2015a). State and Outlook 2015 the European Environment, Cross-Country Comparisons, Freshwater Quality - Nutrients in Rivers. Copenhagen: European Environment Agency.
European Environment Agency [EEA] (2015b). The European Environment - State and Outlook 2015: Synthesis Report. Copenhagen: European Environment Agency, 212.
Eurostat (2018). Air Emissions by NACE Rev. 2 Activity [dataset]. Available at: http://appsso.eurostat.ec.europa.eu/nui/show.do?dataset=env_ac_ainah_r2&lang=en (accessed September, 2018).
Eyre, B. D., Maher, D. T., and Squire, P. (2013). Quantity and quality of organic matter (detritus) drives N2 effluxes (net denitrification) across seasons, benthic habitats, and estuaries. Global Biogeochem. Cycles 27, 1083–1095. doi: 10.1002/2013GB004631
Fennel, K., Alin, S., Barbero, L., Evans, W., Bourgeois, T., Cooley, S., et al. (2019). Carbon cycling in the North American coastal ocean: a synthesis. Biogeosciences 16, 1281–1304.
Fourqurean, J. W., Duarte, C. M., Kennedy, H., Marbà, N., Holmer, M., Mateo, M. A., et al. (2012). Seagrass ecosystems as a globally significant carbon stock. Nat. Geosci. 5, 505–509. doi: 10.1038/ngeo1477
Frankignoulle, M., Abril, G., Borges, A., Bourge, I., Canon, C., Delille, B., et al. (1998). Carbon dioxide emission from European estuaries. Science 282, 434–436. doi: 10.1126/science.282.5388.434
Frankignoulle, M., and Borges, A. V. (2001). European continental shelf as a significant sink for atmospheric carbon dioxide. Glob. Biogeochem. Cycles 15, 569–576. doi: 10.1029/2000gb001307
Frankignoulle, M., Canon, C., and Gatusso, J.-P. (1994). Marine calcification as a source of carbon dioxide: positive feedback on increasing atmospheric CO2. Limnol. Oceanogr. 39, 458–462. doi: 10.4319/lo.1994.39.2.0458
Gallo, N. D., Victor, D. G., and Levin, L. A. (2017). Ocean commitments under the Paris Agreement. Nat. Clim. Chang. 7, 833–838. doi: 10.1038/nclimate3422
García-Martín, E. E., Daniels, C. J., Davidson, K., Davis, C. E., Mahaffey, C., Mayers, K. M., et al. (2019). Seasonal changes in plankton respiration and bacterial metabolism in a temperate shelf sea. Prog. Oceanogr. 177:101884. doi: 10.1016/j.pocean.2017.12.002
Gattuso, J.-P., Magnan, A. K., Bopp, L., Cheung, W. W. L., Duarte, C. M., Hinkel, J., et al. (2018). Ocean solutions to address climate change and its effects on marine ecosystems. Front. Mar. Sci. 5:337. doi: 10.3389/FMARS.2018.00337
Gilbert, D., Rabalais, N. N., Díaz, R. J., and Zhang, J. (2010). Evidence for greater oxygen decline rates in the coastal ocean than in the open ocean. Biogeosciences 7, 2283–2296. doi: 10.5194/bg-7-2283-2010
Glud, R. (2008). Oxygen dynamics of marine sediments. Mar. Biol. Res. 4, 243–289. doi: 10.1080/17451000801888726
Graham, J. A., Rosser, J. P., Dea, E. O., and Hewitt, H. T. (2018). Resolving shelf break exchange around the European Northwest shelf. Geophys. Res. Lett. 45, 12,386-12,395. doi: 10.1029/2018GL079399
Green, B. C., Smith, D. J., Earley, S. E., Hepburn, L. J., and Underwood, G. J. C. (2009). Seasonal changes in community composition and trophic structure of fish populations of five salt marshes along the Essex coastline, United Kingdom. Estuar. Coast. Shelf Sci. 85, 247–256. doi: 10.1016/j.ecss.2009.08.008
Greenwood, N., Parker, E. R., Fernand, L., Sivyer, D. B., Weston, K., Painting, S. J., et al. (2010). Detection of low bottom water oxygen concentrations in the North Sea; implications for monitoring and assessment of ecosystem health. Biogeosciences 7, 1357–1373. doi: 10.5194/bg-7-1357-2010
Grimvall, A., Stålnacke, P., and Tonderski, A. (2000). Time scales of nutrient losses from land to sea - a European perspective. Ecol. Eng. 14, 363–371. doi: 10.1016/s0925-8574(99)00061-0
Gröger, M., Maier-Reimer, E., Mikolajewicz, U., Moll, A., and Sein, D. (2013). NW European shelf under climate warming: implications for open ocean – shelf exchange, primary production, and carbon absorption. Biogeosciences 10, 3767–3792. doi: 10.5194/bg-10-3767-2013
Große, F., Greenwood, N., Kreus, M., Lenhart, H. J., Machoczek, D., Pätsch, J., et al. (2016). Looking beyond stratification: a model-based analysis of the biological drivers of oxygen deficiency in the North Sea. Biogeosciences 13, 2511–2535. doi: 10.5194/bg-13-2511-2016
Große, F., Kreus, M., Lenhart, H.-J., Pätsch, J., and Pohlmann, T. (2017). A novel modeling approach to quantify the influence of nitrogen inputs on the oxygen dynamics of the North Sea. Front. Mar. Sci. 4:383. doi: 10.3389/fmars.2017.00383
Gruber, N., Keeling, C. D., and Bates, N. R. (2002). Interannual variability in the North Atlantic Ocean Carbon Sink. Science 298, 2374–2378. doi: 10.1126/science.1077077
Hale, R., Godbold, J. A., Sciberras, M., Dwight, J., Wood, C., Hiddink, J. G., et al. (2017). Mediation of macronutrients and carbon by post- disturbance shelf sea sediment communities. Biogeochemistry 135, 121–133. doi: 10.1007/s10533-017-0350-9
Hall, S. J. (2002). The continental shelf benthic ecosystem: current status, agents for change and future prospects. Environ. Conserv. 29, 350–374. doi: 10.1017/s0376892902000243
Hanlon, A. R. M., Bellinger, B., Haynes, K., Xiao, G., Hofmann, T. A., Gretz, M. R., et al. (2006). Dynamics of EPS production and loss in an estuarine, diatom-dominated, microalgal biofilm over a tidal emersion-immersion period. Limnol. Oceanogr. 51, 79–93. doi: 10.4319/lo.2006.51.1.0079
Harris, P. T., Macmillan-Lawler, M., Rupp, J., and Baker, E. K. (2014). Geomorphology of the oceans. Mar. Geol. 352, 4–24. doi: 10.1016/j.margeo.2014.01.011
Harris, P. T., and Whiteway, T. (2011). Global distribution of large submarine canyons: geomorphic differences between active and passive continental margins. Mar. Geol. 285, 69–86. doi: 10.1016/j.margeo.2011.05.008
Hartman, S. E., Humphreys, M. P., Kivimäe, C., Woodward, E. M. S., Kitidis, V., McGrath, T., et al. (2018). Seasonality and spatial heterogeneity of the surface ocean carbonate system in the northwest European continental shelf. Prog. Oceanogr. 177:101909. doi: 10.1016/j.pocean.2018.02.005
Harvey, B. J., Shaffrey, L. C., Woollings, T. J., Zappa, G., and Hodges, K. I. (2012). How large are projected 21st century storm track changes. Geophys. Res. Lett. 39:L18707. doi: 10.1029/2012GL052873
Hasler, K., Bröring, S., Omta, S. W. F., and Olfs, H. (2015). Life cycle assessment (LCA) of different fertilizer product types. Eur. J. Agron. 69, 41–51. doi: 10.1016/j.eja.2015.06.00
Hedges, J. K., Keil, R. G., and Benner, R. (1997). What happens to terrestrial organic material in the ocean? Org. Geochem. 27, 195–212. doi: 10.1016/s0146-6380(97)00066-1
Helm, K. P., Bindoff, N. L., and Church, J. A. (2011). Observed decreases in oxygen content of the global ocean. Geophys. Res. Lett. 38:L23602. doi: 10.1029/2011GL049513
Hennige, S. J., Roberts, J. M., and Williamson, P. (2014). An Updated Synthesis of the Impacts of Ocean Acidification on Marine Biodiversity. Montreal: CBD, 99.
Henrichs, S. M., and Reeburgh, W. S. (1987). Anaerobic mineralization of marine sediment organic matter: rates and the role of anaerobic processes in the oceanic carbon economy. Geomicrobiol. J. 5, 191–237. doi: 10.1080/01490458709385971
Hicks, N., Ubbara, G. R., Silburn, B., Smith, H. E. K., Kröger, S., Parker, E. R., et al. (2017). Oxygen dynamics in shelf seas sediments incorporating seasonal variability. Biogeochemistry 135, 35–47. doi: 10.1007/s10533-017-0326-9
Holmer, M. (1996). Composition and fate of dissolved organic carbon derived from phytoplankton detritus in coastal marine sediments. Mar. Ecol. Prog. Ser. 41, 217–228. doi: 10.3354/meps141217
Holt, J., Butenschön, M., Wakelin, S. L., Artioli, Y., and Allen, J. I. (2012). Oceanic controls on the primary production of the northwest European continental shelf: model experiments under recent past conditions and a potential future scenario. Biogeosciences 9, 97–117. doi: 10.5194/bg-9-97-2012
Holt, J., Schrum, C., Cannaby, H., Daewel, U., Allen, I., Artioli, Y., et al. (2016). Potential impacts of climate change on the primary production of regional seas: a comparative analysis of five European seas. Prog. Oceanogr. 140, 91–115. doi: 10.1016/j.pocean.2015.11.004
Holt, J., Wakelin, S., and Huthnance, J. (2009). Down-welling circulation of the northwest European continental shelf: a driving mechanism for the continental shelf carbon pump. Geophys. Res. Lett. 36:L14602. doi: 10.1029/2009GL038997
Holt, J., Wakelin, S., Lowe, J., and Tinker, J. (2010). The potential impacts of climate change on the hydrography of the northwest European continental shelf. Prog. Oceanogr. 86, 361–379. doi: 10.1016/j.pocean.2010.05.003
Horsburgh, K., and Lowe, J. (2013). Impacts of climate change on sea level. MCCIP Sci. Rev. 27–33. doi: 10.14465/2013.arc04.027-033
Horton, B. P., Shennan, I., Bradley, S. L., Cahill, N., Kirwan, M., Kopp, R. E., et al. (2018). Predicting marsh vulnerability to sea-level rise using Holocene relative sea-level data. Nat. Commun. 9:2687. doi: 10.1038/s41467-018-05080-0
Houghton, R. A., and Woodwell, G. M. (1980). The flax pond ecosystem study: exchanges of CO2 between a salt marsh and the atmosphere. Ecology 61, 1434–1445. doi: 10.2307/1939052
Howard, J., McLeod, E., Thomas, S., Eastwood, E., Fox, M., Wenzl, L., et al. (2017). The potential to integrate blue carbon into MPA design and management. Aquat. Conserv. Mar. Freshw. Ecosyst. 27, 100–115. doi: 10.1002/aqc.2809
Hughes, S. L., Tinker, J., Dye, S., Andres, O., Berry, D. I., Hermanson, L., et al. (2017). Temperature MCCIP Science Review 2017. Lowestoft: Marine Climate Change Impacts Partnership. doi: 10.14465/2017.arc10.003.tem
Humphreys, M. P., Achterberg, E. P., Hopkins, J. E., Chowdhury, M. Z. H., Griffiths, A. M., Hartman, S. E., et al. (2018). Mechanisms for a nutrient-conserving carbon pump in a seasonally stratified, temperate continental shelf sea. Prog. Oceanogr. 177:101961. doi: 10.1016/j.pocean.2018.05.001
Jarvie, H. P., King, S. M., and Neal, C. (2017). Inorganic carbon dominates total dissolved carbon concentrations and fluxes in British rivers: application of the THINCARB model- Thermodynamic modelling of inorganic carbon in freshwaters. Sci. Total Environ. 575, 496–512. doi: 10.1016/j.scitotenv.2016.08.201
Jennings, S., Dinmore, T. A., Duplisea, D. E., Warr, K. J., and Lancaster, J. E. (2001). Trawling disturbance can modify benthic production processes. J. Anim. Ecol. 70, 459–475. doi: 10.1046/j.1365-2656.2001.00504
Jiao, N., Robinson, C., Azam, F., Thomas, H., Baltar, F., Dang, H., et al. (2014). Mechanisms of microbial carbon sequestration in the ocean; future research directions. Biogeosciences 11, 5285–5306. doi: 10.5194/bg-11-5285-2014
Jickells, T. (1998). Nutrient biogeochemistry of the coastal zone. Science 281, 217–222. doi: 10.1126/science.281.5374.217
Jickells, T., Andrews, J., Samways, G., Sanders, R., Malcolm, S., Sivyer, D., et al. (2000). Nutrient fluxes through the humber estuary: past, present and future. Ambio 29, 130–135. doi: 10.1579/0044-7447-29.3.130
Johnson, M. T., Greenwood, N., Sivyer, D. B., Thomson, M., Reeve, A., Weston, K., et al. (2013). Characterising the seasonal cycle of dissolved organic nitrogen using Cefas SmartBuoy high-resolution time-series samples from the southern North Sea. Biogeochemistry 113, 23–36. doi: 10.1007/s10533-012-9738-8
Jones, J. B. (1992). Environmental impact of trawling on the seabed: a review. N. Z. J. Mar. Freshw. Res. 26, 59–67. doi: 10.1080/00288330.1992.9516500
Jones, L., Angus, S., Cooper, A., Doody, P., Everard, M., Garbutt, A., et al. (2011). Coastal Margins, UK National Ecosystem Assessment, Technical Report Broad Habitats. Cambridge: UNEP-WCMC.
Kandasamy, S., and Nagender Nath, B. (2016). Perspectives on the terrestrial organic matter transport and burial along the land-deep sea continuum: caveats in our understanding of biogeochemical processes and future needs. Front. Mar. Sci. 3:259. doi: 10.3389/fmars.2016.00259
Katsman, C. A., Sterl, A., Beersma, J. J., van den Brink, H. W., Church, J. A., Hazeleger, W., et al. (2011). Exploring high-end scenarios for local sea level rise to develop flood protection strategies for a low-lying delta-the Netherlands as an example. Clim. Change 109, 617–645. doi: 10.1007/s10584-011-0037-5
Keil, R. (2017). Anthropogenic forcing of carbonate and organic carbon preservation in marine sediments. Annu. Rev. Mar. Sci. 9, 151–172. doi: 10.1146/annurev-marine-010816-060724
Kitidis, V., Shutler, J. D., Ashton, I., Warren, M., Brown, I., Findlay, H., et al. (2019). Winter weather controls net influx of atmospheric CO2 on the north-west European shelf. Sci. Rep. 9:20153. doi: 10.1038/s41598-019-56363-5
Kovats, R. S., Valentini, R., Bouwer, L. M., Georgopoulou, E., Jacob, D., Martin, E., et al. (2014). “Europe,” in Climate Change 2014: Impacts, Adaptation, and Vulnerability. Part B: Regional Aspects. Contribution of Working Group II to the Fifth Assessment Report of the Intergovernmental Panel on Climate Change, eds V. R. Barros, C. B. Field, D. J. Dokken, M. D. Mastrandrea, K. J. Mach, T. E. Bilir, et al. (Cambridge: Cambridge University Press), 1267–1326. doi: 10.1017/CBO9781107415386.003
Krause-Jensen, D., and Duarte, C. M. (2016). Substantial role of macroalgae in marine carbon sequestration. Nat. Geosci. 9, 737–742. doi: 10.1038/ngeo2790
Krause-Jensen, D., Lavery, P., Serrano, O., Marbà, N., Masque, P., and Duarte, C. M. (2018). Sequestration of macroalgal carbon: the elephant in the Blue Carbon room. Biol. Lett. 14:20180236. doi: 10.1098/rsbl.2018.0236
Kröger, S., Parker, R., Cripps, G., and Williamson, P. (eds) (2018). Shelf Seas: The Engine of Productivity, Policy Report on NERC-Defra Shelf Sea Biogeochemistry Programme. Lowestoft: Cefas. doi: 10.14465/2018.ssb18.pbd
Krumins, V., Gehlen, M., Arndt, S., Van Cappellen, P., and Regnier, P. (2013). Dissolved inorganic carbon and alkalinity fluxes from coastal marine sediments: model estimates for different shelf environments and sensitivity to global change. Biogeosciences 10, 371–398. doi: 10.5194/bg-10-371-2013
Kuwae, T., Kanda, J., Kubo, A., Nakajima, F., Ogawa, H., Sohma, A., et al. (2016). Blue carbon in human-dominated estuarine and shallow coastal systems. Ambio 45, 290–301. doi: 10.1007/s13280-015-0725-x
Kwadijk, J., Arnell, N. W., Mudersbach, C., and De Weerd, M. (2016). “Recent change - river flow,” in North Sea Region Climate Change Assessment, eds M. Quante and F. Colijn (Berlin: Springer), 137–146. doi: 10.1007/978-3-319-39745-0
Land, P. E., Shutler, J. D., Findlay, H. S., Girard-ardhuin, F., Sabia, R., Reul, N., et al. (2015). Salinity from space unlocks satellite-based assessment of ocean acidification. Environ. Sci. Technol. 49, 1987–1994. doi: 10.1021/es504849s
Laruelle, G. G., Cai, W. J., Hu, X., Gruber, N., Mackenzie, F. T., and Regnier, P. (2018). Continental shelves as a variable but increasing global sink for atmospheric carbon dioxide. Nat. Commun. 9:454. doi: 10.1038/s41467-017-02738-z
Laruelle, G. G., Dürr, H. H., Slomp, C. P., and Borges, A. V. (2010). Evaluation of sinks and sources of CO2 in the global coastal ocean using a spatially-explicit typology of estuaries and continental shelves. Geophys. Res. Lett. 37:L15607. doi: 10.1029/2010GL043691
Laruelle, G. G., Lauerwald, R., Pfeil, B., and Regnier, P. (2014). Regionalized global budget of the CO2 exchange at the air-water interface in continental shelf seas. Glob. Biogeochem. Cycles 28, 1199–1214. doi: 10.1002/2014GB004832.Received
Lau, W. W. Y. (2013). Beyond carbon: conceptualizing payments for ecosystem services in blue forests on carbon and other marine and coastal ecosystem services. Ocean Coast. Manag. 83, 5–14. doi: 10.1016/j.ocecoaman.2012.03.011
Le Quéré, C., Andrew, R. M., Friedlingstein, P., Sitch, S., Pongratz, J., Manning, A. C., et al. (2018). Global Carbon Budget 2017. Earth Syst. Sci. Data 10, 405-448.
Lenhart, H. J., Mills, D. K., Baretta-Bekker, H., van Leeuwen, S. M., van der Molen, J., Baretta, J. W., et al. (2010). Predicting the consequences of nutrient reduction on the eutrophication status of the North Sea. J. Mar. Syst. 81, 148–170. doi: 10.1016/j.jmarsys.2009.12.014
Lowe, J., Howard, T., Pardaens, A., Tinker, J., Holt, J., Wakelin, S., et al. (2009). UK Climate Projections Science Report: Marine & Coastal Projections. Exeter: Met Office Hadley Centre.
Luisetti, T., Jackson, E. L., and Turner, R. K. (2013). Valuing the European “coastal blue carbon” storage benefit. Mar. Pollut. Bull. 71, 101–106. doi: 10.1016/j.marpolbul.2013.03.029
Luisetti, T., Turner, R. K., Andrews, J. E., Jickells, T. D., Kröger, S., Diesing, M., et al. (2019). Quantifying and valuing carbon flows and stores in coastal and shelf ecosystems in the UK. Ecosyst. Serv. 35, 67–76. doi: 10.1016/j.ecoser.2018.10.013
Lutz, S. J., and Martin, A. H. (2014). Fish Carbon: Marine Vertebrate Carbon Services. Arendal: GRID-Arendal.
Mackinson, S., and Daskalov, G. (2007). An Ecosystem Model of the North Sea to Support an Ecosystem Approach to Fisheries Management: Description and Parameterisation. Cefas Science Series Technical Report. Lowestoft: Cefas.
Maddock A. (ed.) (2008). UK Biodiversity Action Plan: Priority Habitat Descriptions. Peterborough: Joint Nature Conservation Committee.
Malinverno, A., and Martinez, E. A. (2015). The effect of temperature on organic carbon degradation in marine sediments. Sci. Rep. 5:17861. doi: 10.1038/srep17861
Martín, J., Puig, P., Palanques, A., and Giamportone, A. (2014a). Commercial bottom trawling as a driver of sediment dynamics and deep seascape evolution in the Anthropocene. Anthropocene 7, 1–15. doi: 10.1016/j.ancene.2015.01.002
Martín, J., Puig, P., Masqué, P., Palanques, A., and Sánchez-Gómez, A. (2014b). Impact of bottom trawling on deep-sea sediment properties along the flanks of a submarine canyon. PLoS One 9:e104536. doi: 10.1371/journal.pone.0104536
Masson, D. G., Huvenne, V. A. I., De Stigter, H. C., Wolff, G. A., Kiriakoulakis, K., Arzola, R. G., et al. (2010). Efficient burial of carbon in a submarine canyon. Geology 38, 831–834. doi: 10.1130/G30895.1
Mayer, L. M., Schick, D. F., Findlay, R. H., and Rice, D. L. (1991). Effects of commercial dragging on sedimentary organic matter. Mar. Environ. Res. 31, 249–261. doi: 10.1016/0141-1136(91)90015-z
Mayor, D. J., Thornton, B., and Zuur, A. F. (2012). Resource quantity affects benthic microbial community structure and growth efficiency in a temperate intertidal mudflat. PLoS One 7:e38582. doi: 10.1371/journal.pone.0038582
McKew, B. A., Dumbrell, A., Taylor, J. D., McGenity, T. J., and Underwood, G. J. C. (2013). Differences between aerobic and anaerobic degradation of microphytobenthic biofilm-derived organic matter within intertidal sediments. FEMS Microbiol. Ecol. 84, 495–509. doi: 10.1111/1574-6941.12077
Mcleod, E., Chmura, G. L., Bouillon, S., Salm, R., Björk, M., Duarte, C. M., et al. (2011). A blueprint for blue carbon: toward an improved understanding of the role of vegetated coastal habitats in sequestering CO2. Front. Ecol. Environ. 9, 552–560. doi: 10.1890/110004
McMellor, S., and Underwood, G. J. C. (2014). Water policy effectiveness: 30 Years of change in the hypernutrified Colne estuary, England. Mar. Pollut. Bull. 81, 200–209. doi: 10.1016/j.marpolbul.2014.01.018
Mcowen, C., Weatherdon, L., Bochove, J.-W., Sullivan, E., Blyth, S., Zockler, C., et al. (2017). A global map of salt marshes. Biodivers. Data J. 5:e11764. doi: 10.3897/BDJ.5.e11764
Mehring, A. S., Lowrance, R. R., Helton, A. M., Pringle, C. M., Thompson, A., Bosch, D. D., et al. (2013). Interannual drought length governs dissolved organic carbon dynamics in blackwater rivers of the western upper Suwannee River basin. J. Geophys. Res. Biogeosci. 118, 1636–1645. doi: 10.1002/2013jg002415
Middelburg, J. J. (2018). Review and syntheses: to the bottom of carbon processing at the seafloor. Biogeosciences 15, 413–427. doi: 10.5194/bg-15-413-2018
Najjar, R. G., Herrmann, M., Alexander, R., Boyer, E. W., Burdige, D. J., Butman, D., et al. (2018). Carbon budget of tidal wetlands, estuaries, and shelf waters of Eastern North America. Glob. Biogeochem. Cycles 32, 389–416. doi: 10.1002/2017gb005790
National Atmospheric Emissions Inventory [NAEI] (2018). UK NAEI - National Atmospheric Emissions Inventory. Available at: naei.beis.gov.uk [accessed January, 2018].
Nedwell, D. B., Underwood, G. J. C., McGenity, T. J., Whitby, C., and Dumbrell, A. J. (2016). The Colne estuary: a long-term microbial ecology observatory. Adv. Ecol. Res. 55, 227–281. doi: 10.1016/bs.aecr.2016.08.004
Nellemann, C., Corcoran, E., Duarte, C. M., Valdés, L., De Young, C., Fonseca, L., et al. (2009). Blue Carbon: A Rapid Response Assessment, United Nations Environment Programme. Arendal: GRID-Arendal.
Neubacher, E. (2009). Oxygen and Nitrogen Cycling in Sediments of the Southern North Sea, Ph.D. thesis, Queen Mary University, London.
Nottage, A. S., and Robertson, P. A. (2005). The Salt Marsh Creation Handbook: A Project Managers Guide to the Creation of Salt Marsh and Intertidal Mud Flat. London: The RSPB, Sandy & CIWEM.
Oberle, F. K., Storlazzi, C. D., and Hanebuth, T. J. (2016a). What a drag: quantifying the global impact of chronic bottom trawling on continental shelf sediment. J. Mar. Syst. 159, 109–119. doi: 10.1016/j.jmarsys.2015.12.007
Oberle, F. K., Swarzenski, P. W., Reddy, C. M., Nelson, R. K., Baasch, B., and Hanebuth, T. J. (2016b). Deciphering the lithological consequences of bottom trawling to sedimentary habitats on the shelf. J. Mar. Syst. 159, 120–131. doi: 10.1016/j.jmarsys.2015.12.008
Omstedt, A., Elken, J., Lehmann, A., and Piechura, J. (2004). Knowledge of the Baltic Sea physics gained during the BALTEX and related programmes. Prog. Oceanogr. 63, 1–28. doi: 10.1016/j.pocean.2004.09.001
Orth, R. J., Carruthers, T. J. B., Dennison, W. C., Carlos, M., Fourqurean, J. W. Jr., Heck, K. L., et al. (2006). A global crisis for seagrass ecosystems. Bioscience 56, 987–996. doi: 10.1016/j.tree.2019.04.004
Oschlies, A., Brandt, P., Stramma, L., and Schmidtko, S. (2018). Drivers and mechanisms of ocean deoxygenation. Nat. Geosci. 11, 467–473. doi: 10.1038/s41561-018-0152-2
Ostle, C., Williamson, P., Artioli, Y., Bakker, D. C. E., Birchenough, S., Davis, C. E., et al. (2016). Carbon Dioxide and Ocean Acidification Observations in UK Waters Synthesis Report with a Focus on 2010 –2015. Norwich: University of East Anglia.
Painter, S. C., Lapworth, D. J., Woodward, E. M. S., Kroeger, S., Evans, C. D., Mayor, D. J., et al. (2018). Terrestrial dissolved organic matter distribution in the North Sea. Sci. Total Environ. 630, 630–647. doi: 10.1016/j.scitotenv.2018.02.237
Pendleton, L., Donato, D. C., Murray, B. C., Crooks, S., Jenkins, W. A., Sifleet, S., et al. (2012). Estimating global “Blue Carbon” emissions from conversion and degradation of vegetated coastal ecosystems. PLoS One 7:e43542. doi: 10.1371/journal.pone.0043542
Porter, M., Inall, M. E., Green, J. A. M., Simpson, J. H., Dale, A. C., and Miller, P. I. (2016). Drifter observations in the summer time Bay of Biscay slope current. J. Mar. Syst. 157, 65–74. doi: 10.1016/j.jmarsys.2016.01.002
Poulton, A. J., Stinchcombe, M. C., Achterberg, E. P., Bakker, D. C. E., Dumousseaud, C., Lawson, H. E., et al. (2014). Coccolithophores on the north-west European shelf: calcification rates and environmental controls. Biogeosciences 11, 3919–3940. doi: 10.5194/bg-11-3919-2014
Precht, E., and Huettel, M. (2003). Advective pore-water exchange driven by surface gravity waves and its ecological implications. Limnol. Oceanogr. 48, 1674–1684. doi: 10.4319/lo.2003.48.4.1674
Provoost, P., Van Heuven, S., Soetaert, K., Laane, R. W. P. M., and Middelburg, J. J. (2010). Seasonal and long-term changes in pH in the Dutch coastal zone. Biogeosciences 7, 3869–3878. doi: 10.5194/bg-7-3869-2010
Puig, P., Palanques, A., and Mart, J. (2014). Contemporary sediment-transport processes in submarine canyons. Ann. Rev. Mar. Sci. 6, 53–77. doi: 10.1146/annurev-marine-010213-135037
Queirós, A. M., Stephens, N., Widdicombe, S., Tait, K., McCoy, S., Ingels, J., et al. (2019). Connected macroalgal-sediment systems: blue carbon and foodwebs in the deep coastal ocean. Ecol. Monogr. 10:e01366.
Queste, B. Y., Fernand, L., Jickells, T. D., and Heywood, K. J. (2013). Spatial extent and historical context of North Sea oxygen depletion in August 2010. Biogeochemistry 113, 53–68. doi: 10.1007/s10533-012-9729-9
Queste, B. Y., Fernand, L., Jickells, T. D., Heywood, K. J., and Hind, A. J. (2016). Drivers of summer oxygen depletion in the central North Sea. Biogeosciences 13, 1209–1222. doi: 10.5194/bg-13-1209-2016
Rao, A. M. F., McCarthy, M. J., Gardner, W. S., and Jahnke, R. A. (2008). Respiration and denitrification in permeable continental shelf deposits on the South Atlantic Bight: N2:Ar and isotope pairing measurements in sediment column experiments. Cont. Shelf Res. 28, 602–613. doi: 10.1016/j.csr.2007.11.007
Raybaud, V., Beaugrand, G., Goberville, E., Delebecq, G., Destombe, C., Valero, M., et al. (2013). Decline in kelp in West Europe and climate. PLoS One 8:e66044. doi: 10.1371/journal.pone.0066044
Regnier, P. A. G., Friedlingstein, P., Ciais, P., Mackenzie, F. T., Gruber, N., Janssens, I. A., et al. (2013). Anthropogenic perturbation of the carbon fluxes from land to ocean. Nat. Geosci. 6, 597–607. doi: 10.1038/ngeo1830
Ruiz-Castillo, E., Sharples, J., Hopkins, J., and Woodward, M. (2018). Seasonality in the cross-shelf physical structure of a temperate shelf sea and the implications for nitrate supply. Prog. Oceanogr. 177:101985. doi: 10.1016/j.pocean.2018.07.006
Salt, L. A., Thomas, H., Prowe, A. E. F., Borges, A. V., Bozec, Y., and De Baar, H. J. W. (2013). Variability of North Sea pH and CO2 in response to North Atlantic Oscillation forcing. J. Geophys. Res. Biogeosci. 118, 1584–1592. doi: 10.1002/2013JG002306
Schmidtko, S., Stramma, L., and Visbeck, M. (2017). Decline in global oceanic oxygen content during the past five decades. Nature 542, 335–339. doi: 10.1038/nature21399
Schrag, D. P., Higgins, J. A., Macdonald, F. A., and Johnston, D. T. (2013). “Authigenic carbonate and the history of the global carbon cycle”. Science 339, 540–543. doi: 10.1126/science.1229578
Schuster, U., and Watson, A. J. (2007). A variable and decreasing sink for atmospheric CO2 in the North Atlantic. J. Geophys. Res. 112:C11006. doi: 10.1029/2006JC003941
Seitzinger, S. P., Mayorga, E., Bouwman, A. F., Kroeze, C., Beusen, A. H. W., Billen, G., et al. (2010). Global river nutrient export: a scenario analysis of past and future trends. Glob. Biogeochem. Cycles 24:GB0A08. doi: 10.1016/j.scitotenv.2009.12.015
Sharples, J., Holt, J., and Dye, S. (2013). Impacts of climate change on shelf sea stratification. MCCIP Sci. Rev. 67–70. doi: 10.14465/2013.arc08.067-070
Sharples, J., Middelburg, J. J., Fennel, K., and Jickells, T. D. (2016). What proportion of riverine nutrients reaches the open ocean? Glob. Biogeochem. Cycles 31, 39–58. doi: 10.1002/2016gb005483
Shepherd, T. G. (2014). Atmospheric circulation as a source of uncertainty in climate change projections. Nat. Geosci. 7, 703–708. doi: 10.1038/NGEO2253
Shutler, J. D., Land, P. E., Brown, C. W., Findlay, H. S., Donlon, C. J., Medland, M., et al. (2013). Coccolithophore surface distributions in the North Atlantic and their modulation of the air-sea flux of CO2 from 10 years of satellite Earth observation data. Biogeosciences 10, 2699–2709. doi: 10.5194/bg-10-2699-2013
Shutler, J. D., Land, P. E., Piolle, J. F., Woolf, D. K., Goddijn-Murphy, L., Paul, F., et al. (2016). FluxEngine: a flexible processing system for calculating atmosphere – ocean carbon dioxide gas fluxes and climatologies. J. Atmos. Ocean. Technol. 33, 741–756. doi: 10.1175/JTECH-D-14-00204.1
Simas, T., Nunes, J. P., and Ferreira, J. G. (2001). Effects of global climate change on coastal salt marshes. Ecol. Model. 139, 1–15. doi: 10.1016/s0304-3800(01)00226-5
Skov, M. W., Ford, H., Webb, J., Kayoueche-Reeve, M., Hockley, N., Paterson, D., et al. (2016). The Saltmarsh Carbon Stock Predictor - A Tool for Predicting Carbon Stocks of Welsh and English Salt Marshes. CBESS, Biodiversity and Ecosystem Service Sustainability Programme (NERC NE/J015350/1). Bangor: Bangor University.
Smale, D. A., Moore, P. J., Queiros, A. M., Higgs, N. D., and Burrows, M. T. (2018). Appreciating interconnectivity between habitats is key to Blue Carbon management. Front. Ecol. Environ. 16, 71–73. doi: 10.1002/fee.1765
Smith, R. W., Allison, M. A., Savage, C., Bianchi, T. S., and Galy, V. (2015). High rates of organic carbon burial in fjord sediments globally. Nat. Geosci. 8, 450–454. doi: 10.1038/ngeo2421
Snelgrove, P. V., Soetaert, K., Solan, M., Thrush, S., Wei, C. L., Danovaro, R., et al. (2018). Global carbon cycling on a heterogeneous seafloor. Trends Ecol. Evol. 33, 96–105. doi: 10.1016/j.tree.2017.11.004
Sparholt, H. (1990). An estimate of the total biomass of fish in the North-Sea. J. Cons. Int. Explor. Mer. 46, 200–210. doi: 10.1093/icesjms/46.2.200
Spingys, C. P. (2017). Volume Exchange across the Shelf Edge: The Role of the Internal Tide and Other Physical Processes. Ph.D. thesis, University of Liverpool, Liverpool.
Stahl, H., Tengberg, A., Brunnegård, J., Bjørnbom, E., Forbes, T. L., Josefson, A. B., et al. (2004). Factors influencing organic carbon recycling and burial in Skagerrak sediments. J. Mar. Res. 62, 867–907. doi: 10.1357/0022240042880873
Ståhlberg, C., Bastviken, D., Svensson, B. H., and Rahm, L. (2006). Mineralisation of organic matter in coastal sediments at different frequency and duration of resuspension. Estuar. Coast. Shelf Sci. 70, 317–325. doi: 10.1016/j.ecss.2006.06.022
Sun, M.-Y., and Dai, J. (2005). Relative influences of bioturbation and physical mixing on degradation of bloom-derived particulate organic matter: clue from microcosm experiments. Mar. Chem. 96, 201–218. doi: 10.1016/j.marchem.2004.11.003
Sun, M.-Y., Lee, C., and Aller, R. C. (1993). Laboratory studies of oxic and anoxic degradation of chlorophyll-a in Long Island Sound sediments. Geochim. Cosmochim. Acta 57, 147–157. doi: 10.1016/0016-7037(93)90475-c
Sun, X., and Turchyn, A. B. (2014). Significant contribution of authigenic carbonate to marine carbon burial. Nat. Geosci. 7, 201–204. doi: 10.1038/ngeo2070
Suratman, S., Weston, K., Jickells, T., and Fernand, L. (2009). Spatial and seasonal changes of dissolved and particulate organic C in the North Sea. Hydrobiologia 628, 13–25. doi: 10.1007/s10750-009-9730-z
Thomas, H., Bozec, Y., De Baar, H. J. W., Elkalay, K., Frankignoulle, M., Schiettecatte, L. S., et al. (2005). The carbon budget of the North Sea. Biogeosciences 2, 87–96. doi: 10.5194/bg-2-87-2005
Thomas, H., Bozec, Y., Elkalay, K., and de Baar, H. J. W. (2004). Enhanced open ocean storage of CO2 from Shelf Sea pumping. Science 304, 1005–1008. doi: 10.1126/science.1095491
Thomas, H., Friederike Prowe, A. E., van Heuven, S., Bozec, Y., de Baar, H. J. W., Schiettecatte, L.-S., et al. (2007). Rapid decline of the CO2 buffering capacity in the North Sea and implications for the North Atlantic Ocean. Glob. Biogeochem. Cycles 21:GB4001. doi: 10.1029/2006GB002825
Thomas, H., Schiettecatte, L.-S., Suykens, K., Koné, Y. J. M., Shadwick, E. H., Prowe, A. E. F., et al. (2009). Enhanced ocean carbon storage from anaerobic alkalinity generation in coastal sediments. Biogeosciences 2, 267–274. doi: 10.5194/bg-6-267-2009
Thornton, D. C. O., Dong, L. F., Underwood, G. J. C., and Nedwell, D. B. (2002). Factors affecting microphytobenthic biomass, species composition and production in the Colne Estuary (UK). Aquat. Microb. Ecol. 27, 285–300. doi: 10.3354/ame027285
Tinker, J., Lowe, J., Pardaens, A., Holt, J., and Barciela, R. (2016). Uncertainty in climate projections for the 21st century northwest European shelf seas. Prog. Oceanogr. 148, 56–73. doi: 10.1016/j.pocean.2016.09.003
Tsunogai, S., Watanabe, S., and Sato, T. (1999). Is there a “continental shelf pump” for the absorption of atmospheric CO2? Tellus B Chem. Phys. Meteorol. 51, 701–712. doi: 10.3402/tellusb.v51i3.16468
Turner, K., Schaafsma, M., Elliott, M., Burdon, D., Atkins, J., Jickells, T., et al. (2014). UK National Ecosystem Assessment Follow-on. Work Package 4: Coastal/Marine Ecosystem Services: Principles and Practice. Cambridge: UNEP-WCMC.
Underwood, G. J. C., Cotton, A., McGenity, T. J., and Dumbrell, A. J. (2016). Coastal Biodiversity and Ecosystem Service Sustainability (CBESS) Nutrient Exchange Fluxes Between Sediment Cores and Overlying Site Water. Bailrigg: NERC Environmental Information Data Centre.
Underwood, G. J. C., and Kromkamp, J. (1999). Primary production by phytoplankton and microphytobenthos in estuaries. Adv. Ecol. Res. 29, 93–153. doi: 10.1016/s0065-2504(08)60192-0
Upstill-Goddard, R. C. (2011). “The production of trace gases in the estuarine and coastal environment,” in Treatise on estuarine and coastal science, Geochemistry of estuaries and coasts, Vol. 2, eds E. Wolanski and D. S. McLusky (Amsterdam: Elsevier), 271–309. doi: 10.1016/b978-0-12-374711-2.00412-5
van Colen, C., Underwood, G. J. C., Serôdio, J., and Paterson, D. M. (2014). Ecology of intertidal microbial biofilms: mechanisms, patterns and future research needs. J. Sea Res. 92, 2–5. doi: 10.1016/j.seares.2014.07.003
van der Molen, J., Aldridge, J. N., Coughlan, C., Parker, E. R., Stephens, D., and Ruardij, P. (2013). Modelling marine ecosystem response to climate change and trawling in the North Sea. Biogeochemistry 113, 213–236. doi: 10.1007/s10533-012-9763-7
Vermaat, J. E., McQuatters-Gollop, A., Eleveld, M. A., and Gilbert, A. J. (2008). Past, present and future nutrient loads of the North Sea: causes and consequences. Estuar. Coast. Shelf Sci. 80, 53–59. doi: 10.1016/j.ecss.2008.07.005
Wakelin, S. L., Artioli, Y., Butenschön, M., Allen, J. I., and Holt, J. T. (2015). Modelling the combined impacts of climate change and direct anthropogenic drivers on the ecosystem of the northwest European continental shelf. J. Mar. Syst. 152, 51–63. doi: 10.1016/j.jmarsys.2015.07.006
Wakelin, S. L., Holt, J. T., Blackford, J. C., Allen, J. I., Butenschön, M., and Artioli, Y. (2012). Modeling the carbon fluxes of the northwest European continental shelf: validation and budgets. J. Geophys. Res. 117, 1–17. doi: 10.1029/2011JC007402
Walsh, J. J. (1991). Importance of continental margins in the marine biogeochemical cycling of carbon and nitrogen. Nature 350, 53–55. doi: 10.1038/350053a0
Weston, K., Jickells, T. D., Fernand, L., and Parker, E. R. (2004). Nitrogen cycling in the southern North Sea: consequences for total nitrogen transport. Estuar. Coast. Shelf Sci. 59, 559–573. doi: 10.1016/j.ecss.2003.11.002
Wilson, R. J., Speirs, D. C., Sabatino, A., and Heath, M. R. (2018). A synthetic map of the north-west European Shelf sedimentary environment for applications in marine science. Earth Syst. Dyn. 10, 109–130. doi: 10.5194/essd-10-109-2018
Wood, C. L., Hawkins, S. J., Godbold, J. A., and Solan, M. (2015). Coastal Biodiversity and Ecosystem Service Sustainability (CBESS) Total Organic Carbon in Mudflat and Saltmarsh Habitats. Bailrigg: NERC Environmental Information Data Centre.
Keywords: carbon, marine, coastal, shelf, biogeochemistry, budget, impacts
Citation: Legge O, Johnson M, Hicks N, Jickells T, Diesing M, Aldridge J, Andrews J, Artioli Y, Bakker DCE, Burrows MT, Carr N, Cripps G, Felgate SL, Fernand L, Greenwood N, Hartman S, Kröger S, Lessin G, Mahaffey C, Mayor DJ, Parker R, Queirós AM, Shutler JD, Silva T, Stahl H, Tinker J, Underwood GJC, Van Der Molen J, Wakelin S, Weston K and Williamson P (2020) Carbon on the Northwest European Shelf: Contemporary Budget and Future Influences. Front. Mar. Sci. 7:143. doi: 10.3389/fmars.2020.00143
Received: 15 May 2019; Accepted: 24 February 2020;
Published: 18 March 2020.
Edited by:
Robinson W. (Wally) Fulweiler, Boston University, United StatesReviewed by:
Isaac R. Santos, Southern Cross University, AustraliaCopyright © 2020 Legge, Johnson, Hicks, Jickells, Diesing, Aldridge, Andrews, Artioli, Bakker, Burrows, Carr, Cripps, Felgate, Fernand, Greenwood, Hartman, Kröger, Lessin, Mahaffey, Mayor, Parker, Queirós, Shutler, Silva, Stahl, Tinker, Underwood, Van Der Molen, Wakelin, Weston and Williamson. This is an open-access article distributed under the terms of the Creative Commons Attribution License (CC BY). The use, distribution or reproduction in other forums is permitted, provided the original author(s) and the copyright owner(s) are credited and that the original publication in this journal is cited, in accordance with accepted academic practice. No use, distribution or reproduction is permitted which does not comply with these terms.
*Correspondence: Oliver Legge, by5sZWdnZUB1ZWEuYWMudWs=; Martin Johnson, bWFydGluLmpvaG5zb25AdWVhLmFjLnVr
Disclaimer: All claims expressed in this article are solely those of the authors and do not necessarily represent those of their affiliated organizations, or those of the publisher, the editors and the reviewers. Any product that may be evaluated in this article or claim that may be made by its manufacturer is not guaranteed or endorsed by the publisher.
Research integrity at Frontiers
Learn more about the work of our research integrity team to safeguard the quality of each article we publish.