- 1Department of Coastal Systems, Royal Netherlands Institute for Sea Research and Utrecht University, Den Burg, Netherlands
- 2Marine Evolution and Conservation, Groningen Institute of Life Sciences, University of Groningen, Groningen, Netherlands
- 3Department of Ocean Systems, Royal Netherlands Institute for Sea Research and Utrecht University, Den Burg, Netherlands
- 4Department of Marine Microbiology and Biogeochemistry, Royal Netherlands Institute for Sea Research and Utrecht University, Den Burg, Netherlands
- 5Department of Earth Sciences, Faculty of Geosciences, Utrecht University, Utrecht, Netherlands
Seafloor massive sulfide (SMS) deposits are commonly found at hydrothermal vents and recently gained the special interest of mining industries. These deposits contain valuable metals and methods are currently developed to mine deep sea SMS deposits. However, excavation of SMS deposits potentially pose a threat to benthic life at the mining site itself, and also in the surrounding environment with plumes of suspended sediment and fine-grained SMS debris created during deep sea mining activities being highlighted as one of the major threats to deep-sea benthic fauna. The benthic communities surrounding the vents are, however, poorly known. As they are often exposed to natural plumes studying such communities could provide valuable information on their resilience toward mining related plumes. The Rainbow hydrothermal vent site at the Mid-Atlantic Ridge is a site characterized by one of the largest continuous natural plumes, which is found persisting over an extensive area. Sediment and water samples were taken both upstream and downstream of the Rainbow hydrothermal vent. Approximately 25 km away from the vent reference sites were samples as well. In addition to detecting the plume itself, concentrations of major and trace-metals in the sediments were used as tracers for long time sustained plume influence. At all sites, we assessed benthic species composition and detected larvae. Metabarcoding methods were used to determine species composition. Benthic species composition in the sediment was shown to differ between all locations and was highly influenced by the plume’s fall out. Arthropoda were more dominant closer to the vent whereas Annelida and Nematoda were more dominant at the reference locations. Conservation and restoration of all these communities after a deep sea mining event will be difficult due to the spatial variation of these benthic communities.
Introduction
Seafloor massive sulfides (SMS) are commonly deposited at and near hydrothermal vent sites through precipitation of metal sulfides as acidic hydrothermal fluids exit the seafloor in these volcanic active regions (Collins et al., 2013). SMS deposits contain high concentrations of metals, particularly copper, zinc, and cobalt. Over 300 vent sites have been listed by the International Seabed Authority (ISA) ocean wide, of which a third is supposed to have SMS deposits containing valuable metals (Baker and German, 2004). Because of worldwide shortages of these valuable metals in the context of the current energy revolution, these areas have recently gained the special interest of mining industries. Suspended sediment plumes created during mining activities are highlighted as one of the major potential threats to deep-sea benthic fauna (Nakajima et al., 2015). Whereas the excavation process itself will wipe out the benthic communities on the vents’ edifices, the mining induced plumes might impact a much larger area, possibly affecting areas further away from the mining site. Background communities nearby natural vents tend to host higher biomass densities compared to the surrounding deep sea, likely due to their high primary productivity input from the central vent area (Galkin, 1997; Sen et al., 2014). However, background communities have received considerably less research effort than the vent-communities themselves (Boschen et al., 2013).
The soft-sediment background fauna is spatially fragmented, mainly due to the large geomorphic variability around the vents. The main process connecting the sessile benthic populations of meio, macro, and megafauna is the dispersal of larvae (Cowen and Sponaugle, 2009; Boschen et al., 2013). Hence, in the case of a disruption of the background community by a natural or anthropogenic event, larval supply is a crucial step in recolonization. Larval dispersal, connectivity and recolonization of typical hydrothermal vent species has been fairly well studied in the past decades (e.g., Metaxas, 2004; Mullineaux et al., 2010; Beaulieu et al., 2015; Gollner et al., 2015; Vic et al., 2018; Xu et al., 2018). In contrast, larval supply is practically unknown for background communities. Filling this gap is essential for credible valuation of impacts of SMS mining on species and community level, also further away from the mining site.
With the first commercial SMS mining in a preparatory phase (Solware I, Collins et al., 2013) the effects of artificial mining plumes are still unknown. Importantly, also the effects of natural plumes on the underlying benthic habitats have been rarely studied, which could hinder the assessment of potential impacts. The Rainbow hydrothermal vent site at the Mid-Atlantic Ridge (MAR) (Figure 1) is known for emitting one of the strongest plumes at MAR (German and Parson, 1996; Fouquet et al., 1997; Khripounoff et al., 2001). The Rainbow hydrothermal vent plume originates at ∼2,300 m depth and rises ∼200 m until becoming neutrally buoyant after which it disperses horizontally (German et al., 1998). This neutrally buoyant plume is subsequently transported by tidal currents over the background community on the Rainbow ridge up to 50 km from its source (Edmonds and German, 2004). Sediments underlying the plume’s track have been found to contain particularly high concentrations of copper during the first part of its track (up to 5 km from the vent) while iron and manganese show high concentrations further away from the vent (up to 25 km) due to chemical fractionation associated with the hydrothermal plume fallout (Cave et al., 2002). Although fluxes of these metals are considerable, the amount of plume fallout and effect on the underlying benthic communities is unknown. Knowledge about these plume-effected habitats by analogy provides a first order base line for predicting the scale of impact by artificial plumes. Firstly because this may show how chemical laden plumes affect and alter a benthic community. Secondly, it may indicate the distance from the source to which this impact is still affecting the benthic community in the chemically enriched environments downstream of the vent.
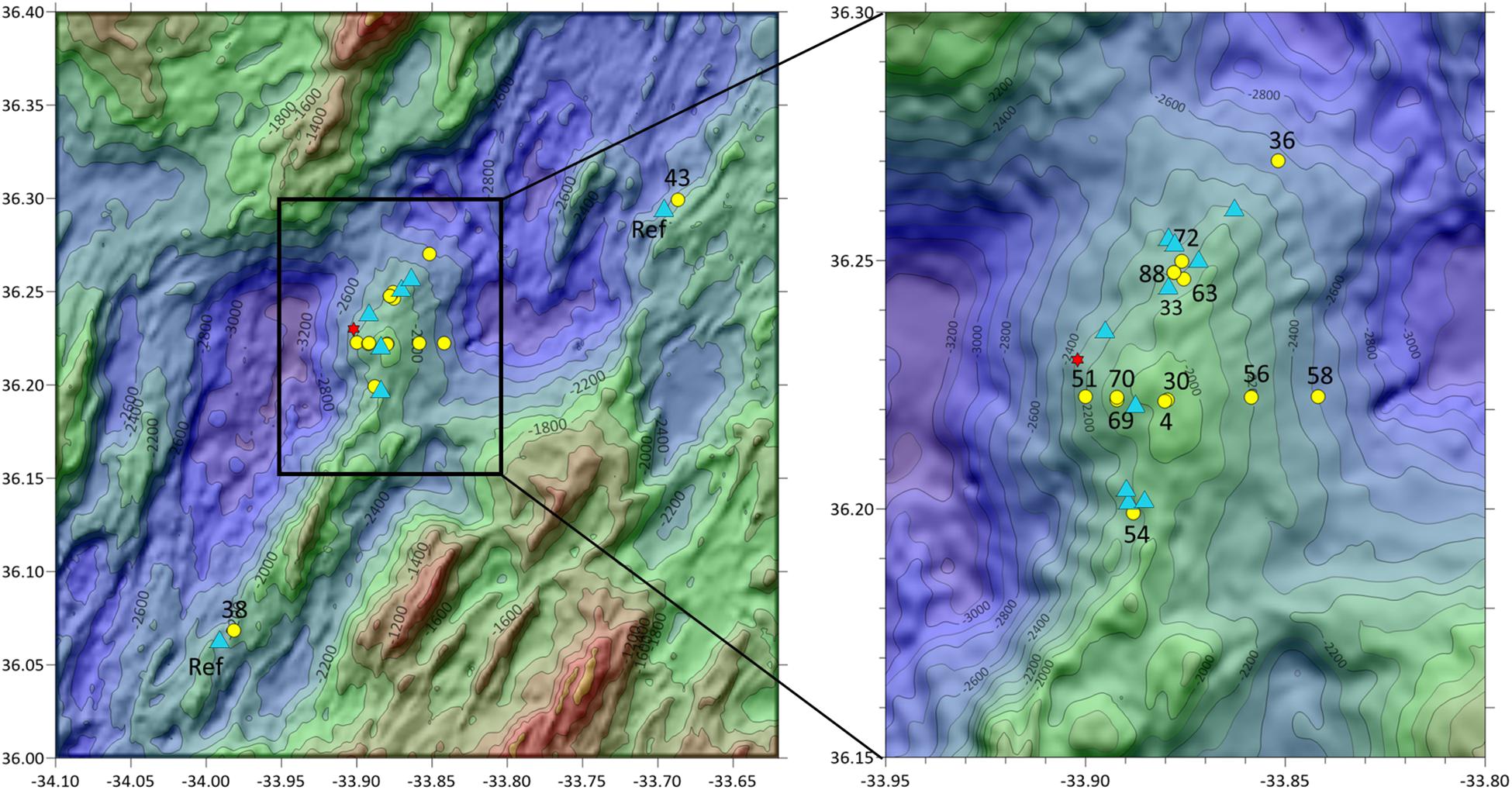
Figure 1. Sampling map. Sampling stations of box core (yellow circle) and water/larvae (blue triangle) samples around the Rainbow hydrothermal vent (red star). Stations numbers depict the box core sample stations. The analysis of the water samples was clustered per area: NE and SE of the vent site and Reference.
Conventional studies of benthic communities around vent sites at the MAR are based on taxonomic identification of species using morphological characteristics (e.g., Desbruyères and Seqonzac, 1997; Sarrazin et al., 2015; Sen et al., 2016). This inherently requires detailed and extensive taxonomic knowledge, especially for the rare and extremely diverse deep sea communities (Danovaro et al., 2014; Guardiola et al., 2016). Hence, these studies are often restricted to macrofauna species (Cardoso et al., 2011; Cowart et al., 2015) even though meiofauna is an equally useful estimator for the status of the environment around deep-sea hydrothermal vents (Zeppilli and Danovaro, 2009; Vanreusel et al., 2010; Sarrazin et al., 2015). Recent developments in the form of genetic barcodes (Hebert et al., 2003) derived from DNA extracted from environmental samples combined with next generation sequencing technologies may offer a solution. These so-called metabarcoding techniques (Taberlet et al., 2012a) have been applied to a wide range of marine benthic communities including those in the deep sea (e.g., Chariton et al., 2015; Dell’Anno et al., 2015; Guardiola et al., 2016; Lanzén et al., 2016; Sinniger et al., 2016). The same technique could in principle be applied to samples from the water column in order to detect and identify pelagic larvae of benthic organisms, which is otherwise a challenge given their low numbers and undiscriminating habitus (Adams et al., 2012).
The aim of the current study is to apply a metabarcoding approach to: (1) detect patterns in the marine benthic communities in- and outside the path of the Rainbow hydrothermal plume whereby trace metal concentrations are used as proxy for influence of the plume; (2) test if larvae of benthic animals can be detected in discrete water samples with a limited volume, and if so, explore patterns in their distribution around the Rainbow vent.
Materials and Methods
Study Site and Sampling Design
Samples and measurements were collected during three cruises with RV Pelagia (May 2014, April 2015, and June/July 2016) around the Rainbow hydrothermal vent field (Figure 1). This vent field, located at the Mid Atlantic Ridge (MAR) at 36°14′N, 33°54′W and at 2,300 m depth was chosen as study site due to its extensive buoyant plume (German and Parson, 1996; Severmann et al., 2004). The vent field is known to produce plumes relatively rich in transition metals resulting in SMS deposits enriched in Cu, Zn, Co, and Ni (Charlou et al., 2002; Douville et al., 2002; Findlay et al., 2015). This plume could still be detected up to 50 km away from the vent site, moving predominantly to the north and east, following the contours of the Rainbow Ridge at ∼2,100 m depth (Severmann et al., 2004). Moreover, the plume could be consistently traced up to 25 km away from the vent source based on turbidity measurements and the chemical analysis of water samples (Haalboom et al., 2019). The study of Haalboom et al. (2019), showed an enrichment of metals compared to the overlying clear water in the neutrally buoyant plume with a high proportion of chalcophile elements closest to the vent site.
Sediment samples were collected using the NIOZ box corer with a 25 cm radius and 55 cm sampling depth (equivalent to a maximum of roughly 100 L of sediment). The box corer is equipped with a tightly closing lid to prevent the sediment from being washed out during retrieval. A total of 15 stations were sampled, of which 13 sites within a 5 km radius of the Rainbow vent site and 2 reference sites at approximately 25 km distance from the vent site. From each box core two samples were collected for molecular identifications. A smaller sediment sample was collected using a sub corer with a 1.25 cm radius and 10 cm sampling depth (equivalent to ±50 mL of sediment) and immediately stored at −80°C. And a larger sample (approximately 500 cm2 surface) was collected from the top 15 cm of the remaining sediment, sieved over a 200 μm mesh sieve on board and this was stored at −80°C as well, immediately upon sieving. Next to the fauna samples, a sub core (5 cm radius) was retrieved for sedimentological analysis and stored at 4°C. Collection of sedimentological sample was not possible at sample location 51.
Water samples were collected from the water column 5 m above the seafloor either using 24 Niskin bottles of each 12 L, which were mounted on the CTD-frame (total volume 288l), or using the NIOZ Large Volume Sampler (LVS), with a volume of 1,000 L (Duinveld and Shipboard Scientific Party, 2016). The LVS consists of a cylindrical polyester tank with 0.5 m diameter openings at the top and bottom which can be remotely closed with a lid through the ship’s CTD operation unit. On board the water contained in the tank and Niskin bottles was drained off through a tap at the bottom of the tank and filtered over a 30 μm nylon mesh sieve. The sieve residues were stored at −80°C. In this way, a total of 17 water samples were taken of which 15 within a 5 km radius of the vent site and 2 reference sites (Figure 1).
Chemical Analysis
Major and trace elements were determined for the top 1 cm of all box-cores by Inductively Coupled Plasma Mass Spectrometry (ICP-MS) analyses. A 100 mg of cryodesiccated surface sediments were completely fully digested in 3.5 mL of HF:HNO3 mixture (2.5:1), to which 1 mL 1M HCl and 1 mL perchloric acid were added. The sample-acid mixtures were heated in closed Teflon bombs for 48 h at 125°C in a hot block, after which the bombs were opened and left to evaporate for 24 h at 125°C. The dried residue was subsequently transferred to in 1 M HNO3 and an internal standard was added. Before analyses the samples were diluted by a factor ten thousand with milli-Q water. The obtained solution was measured with the Thermo Scientific Element-2 ICP-MS, including two blanks and three standards (PACS-2, JDo-1, and MESS-3). These standards were chosen for their expected element resemblance with the TREASURE cores. ELEMENT 2/XR software was used to convert data into concentrations in ppm. Standard deviations were based on six replications per sample. Detection limit was calculated as three times the standard deviation of six-replicate measurements of a blank, averaged for two blanks.
Molecular Identification
Environmental DNA (eDNA) is considered to comprise DNA shed from living species and DNA leaking from fragments and dead organisms (Corinaldesi et al., 2011; Taberlet et al., 2012b; Guardiola et al., 2015, 2016). Because of this inclusiveness, for benthic fauna often only small amounts of sediment (up to 10 g) are used for the analysis of the metazoan community (Sinniger et al., 2016; López-Escardó et al., 2018). However, the detection of macrofauna species might be biased as the release and fate of eDNA differs per species (Barnes and Turner, 2016). In this study, DNA was isolated from a small amount of unsieved sediment, to include all smaller specimen and an additional DNA extraction on the sieved sediment fraction was used, to improve the detection of macrofauna species (Brannock and Halanych, 2015; Klunder et al., 2019). Therefore, the sieve residues from the sieved samples were cryodesiccated and ground in an agate mortar using liquid nitrogen. Subsequently, a subsample (10 g) was taken from each sample for further molecular analysis. For the unsieved samples, a subsample (10 g) was taken from each sub core at the following depth intervals: 0–2 cm, 2–3 cm, and 5–6 cm. All subsamples of the sieved (n = 15) and unsieved (n = 3 × 15) fractions served as starting material for further molecular analysis of the benthic fauna. The sieve residue of the water samples served as starting material for further molecular analysis of the pelagic larvae samples. The molecular analysis and subsequent bioinformatics analysis followed the protocols as described in Klunder et al. (2018) using the F04 (5′-GCTTGTCTCAAAGATTAAGCC-3′) and R22mod (5′-CCTGCTGCCTTCCTTRGA-3′) primer pair (Sinniger et al., 2016) except for the DNA extraction of the larvae samples. Here, the DNEASY PowerSoilTM kit (MoBio Inc.) was used instead of the Powermax SoilTM kit. Within the bioinformatics analysis, only clusters with at least two reads after de-replication were retained and further clustering of operational taxonomic units (OTUs) was at a 98% similarity cut-off. Taxonomic assignments were performed with a 0.8 minimum confidence against the SILVA 18S rRNA database (release 128, Pruesse et al., 2007) using the RDP Classifier (Wang et al., 2007). The molecular analysis was completed for all samples except for box core location 36 as DNA-extraction failed.
Reproducibility of PCR and Illumina Sequencing
The reproducibility of sequencing output was evaluated using duplicate samples. Each duplicate sample was run in a separate PCR and Illumina sequencing run. The taxonomic composition of metazoan orders was comparable (Supplementary Figure S1). Also, the relative abundance of reads for all metazoan OTUs were highly similar between the two duplicates based on linear regression (R2 of 0.88, slope of 0.93; P < 0.001) (Supplementary Figure S2). This similarity means the most abundant OTUs were recovered consistently for each duplicate sample. Therefore, it is assumed potential biases introduced by separate PCR and sequencing runs are small, and reliable estimates about the metazoan diversity can be drawn for all samples within this project.
Data Analysis
All data visualizations, calculations and statistical analyses were performed in RStudio. Trace-metal concentrations were calculated in mg/kg (ppm) of the sediment and were normalized to Al, to correct for differences in the aluminosilicate fraction of the sediment. The trace-metal data were prior to ordination with a PCA, normalized using the decostand- function within the R-package ‘vegan’ to distribute the weight of each metal evenly (Oksanen et al., 2018). Bray–Curtis dissimilarities were calculated and used for the PCA. Simper analysis was used to discriminate the effect of each trace-metal.
The read numbers per OTU for all molecular samples were transformed into a relative abundance of reads per OTU per sample. This approach was preferred over rarefaction as it does not omit valid read abundance data (McMurdie and Holmes, 2014; Lanzén et al., 2016). All subsequent analyses were based on these relative data. A threshold for false-positives was calculated from the mock community and was set at a relative read abundance of 0.0005%. The reads were Hellinger transformed (Legendre and Gallagher, 2001) using the vegan function ‘decostand’ and dissimilarity distances were calculated using the Bray–Curtis equation. These dissimilarities were further used for analysis of variance between the locations (permanova) using the adonis2 function and for the simper analysis to discriminate between the effect of each individual OTU.
Results
Trace Elements
The concentration of 23 different trace elements in the sediment was measured for all sampling stations (Supplementary Table S1). PCA and cluster analysis based on the element/Al ratios showed a division into three main groups (Figure 2). The reference locations (38 and 43), which were supposed to be the least affected by the vent plume because of their large distance from the vent are clustered together with sample locations 58, 56, 54, and 36 which are also situated further away from the vent site, either upstream or downstream of the plume. The two other sample groups that could be distinguished based on their trace elemental composition are also spatially clustered: either north-east (72, 63, 33, and 88) or south-east (70, 30, 69, and 4) of the vent-site. SIMPER analysis showed that the difference between the reference sites and the NE sites was mainly due to relatively higher element/Al ratios of Zn, Cu, and Co in the latter, elements that are typically present as sulfides near hydrothermal vents. The ratios of these elements were also high at the SE sites compared to the reference sites, however, not as high as at the NE sites (Table 1). The relative concentrations of Al, Ca, and Sr were on average higher at the reference locations. All three elements are associated with background sedimentation of pelagic carbonate settling from overlying water masses and/or aluminosilicates transported from large distances as clay particles. Although the sample locations 58, 56, 54, and 36 clustered together with the reference sites in the ordinations, their levels of trace-metals typical for hydrothermal vents such as Zn, Cu, and Co are still higher compared to the reference locations (Table 1, ‘Other’). Therefore, these samples will be labeled as ‘Other’ for the rest of the analysis and will be treated as plume-affected.
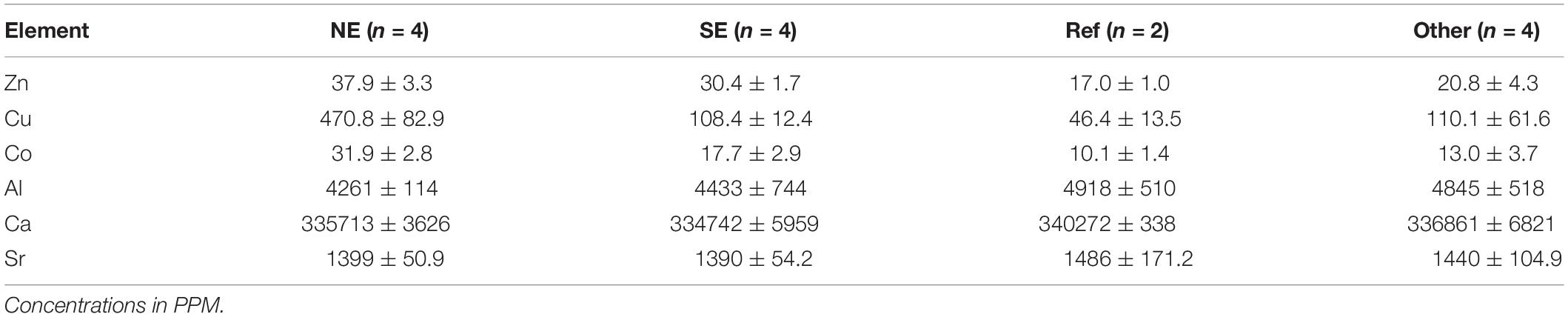
Table 1. Mean values and their standard deviations of sediment trace-element concentrations for groups of stations south-east (SE), north-east (NE), Reference (Ref), and Other stations.
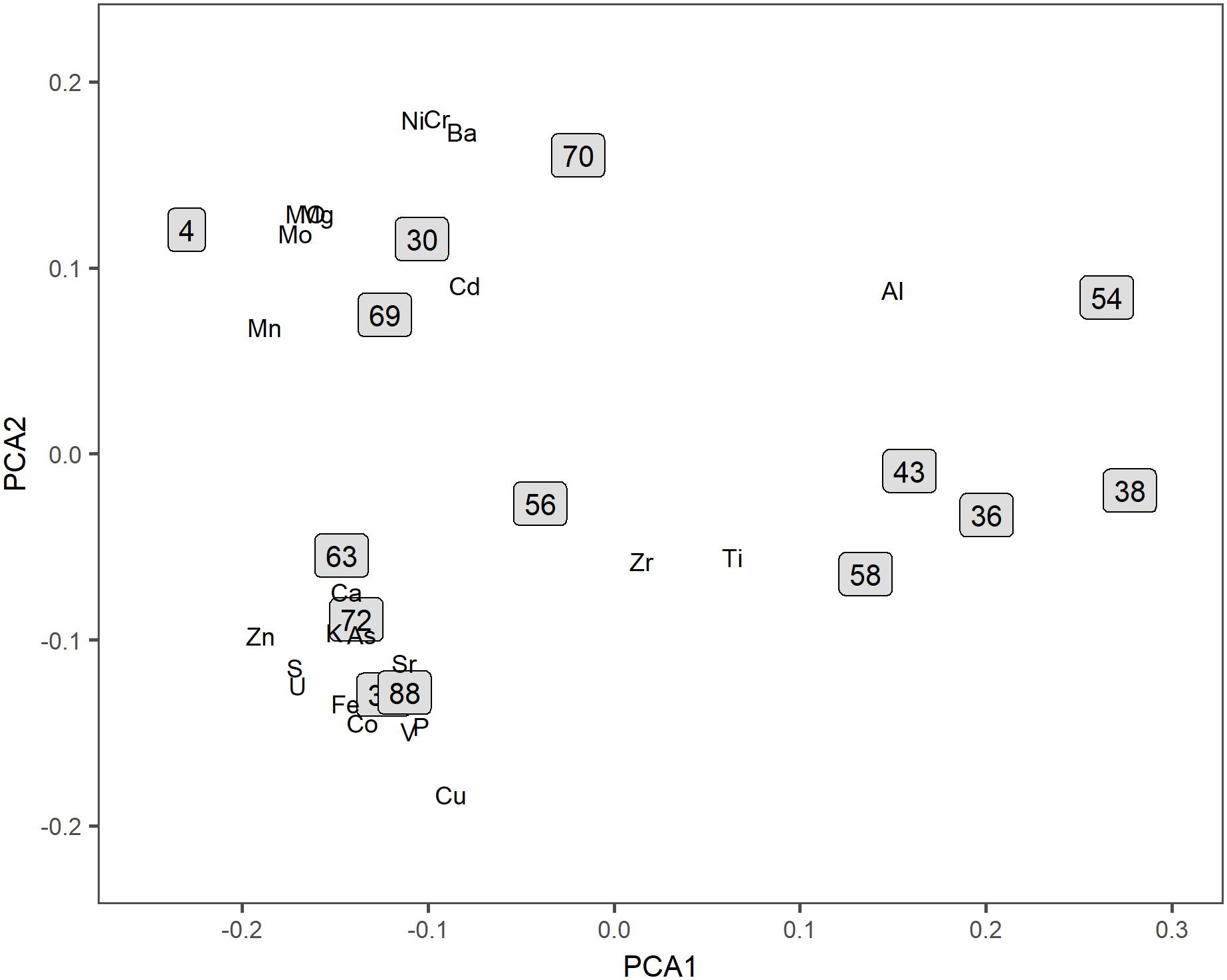
Figure 2. Ordination based on trace-elements. Biplot of the PCA calculated for the concentration of trace-elements and all sampling stations.
Benthic Community Composition
A total of 12.3 million reads passed quality filtering, of which 4.8 million could be confidently assigned to metazoans. Clustering at a threshold at >98% and the removal of singletons resulted in 5,367 metazoan OTUs. MDS ordinations of stations were made based on the relative abundance of reads for all metazoan OTUs separately (Figure 3). We subsequently explored whether distances found in Figure 3 were linked to the chemical composition (Figure 2) by grouping fauna accordingly. The ordination showed a statistically significant difference in composition between the plume-affected sites as compared to the reference sites (Permanova; OTU: F1,13 = 1.285, p = 0.046). Only few of the separate OTUs could be taxonomically assigned beyond the order level. Therefore, Simper analysis of the contribution by OTUs to the dissimilarity between the plume-affected and reference sites mostly gave information at the order level. Simper analysis showed that the difference between the plume-affected sites and the reference sites was mainly due to a lower abundance of an OTU taxonomically assigned to Enoplida (Nematoda) and a higher abundance of Harpacticoida (Arthropoda) at the plume-affected sites. OTUs which could be identified down to genus level and which were important for the dissimilarity between groups were the annelid Myriochele (Sabellida) and Anobothrus (Terebellida). The relative abundance of Myriochele was highest at the distant reference sites. Also at the Other sites Myriochele abundances made up a comparatively large proportion of the OTUs. The relative abundance of Anobothrus was highest at the plume-affected sites, especially at the sites close to the vent (groups NE and SE).
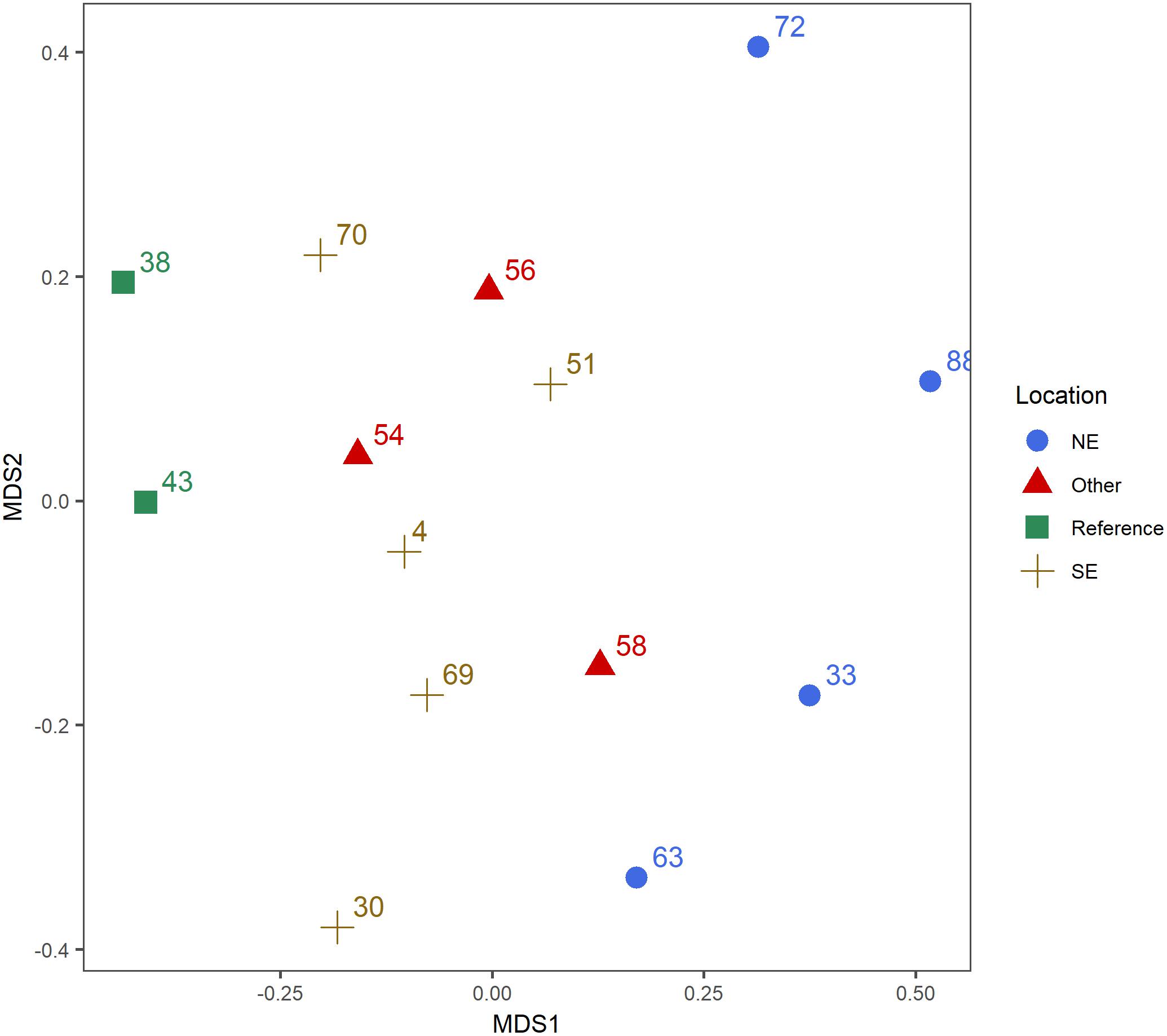
Figure 3. Non-metric multidimensional scaling (nMDS) plot for community composition. The nMDS is based on Bray–Curtis dissimilarities after square root transformation of the relative abundance of reads for all metazoan OTUs.
In total, 18 metazoan phyla were identified (Figure 4). The phyla Annelida, Arthropoda, Nematoda, Platyhelminthes, and Kinorhyncha were recovered from all sampling stations. The arthropods were most proportionally abundant at the two groups of plume-affected sites (i.e., 41% of the metazoan reads for NE and 32% for SE) whereas the nematodes (40%) and annelids (36%) were most abundant at the reference sites. Although there are small differences in taxonomic composition between the stations within a clustered group (i.e., NE, SE, Reference, or Other), the patterns found within each group are distinctly similar (Figure 4). The Shannon-Wiener index was calculated for the relative read abundance of all OTUs for each location. Diversity was on average lowest in the SE locations (H = 2.77) whereas the other locations were comparable (NE: H = 3.29, Other: H = 3.20, Ref: H = 3.29).
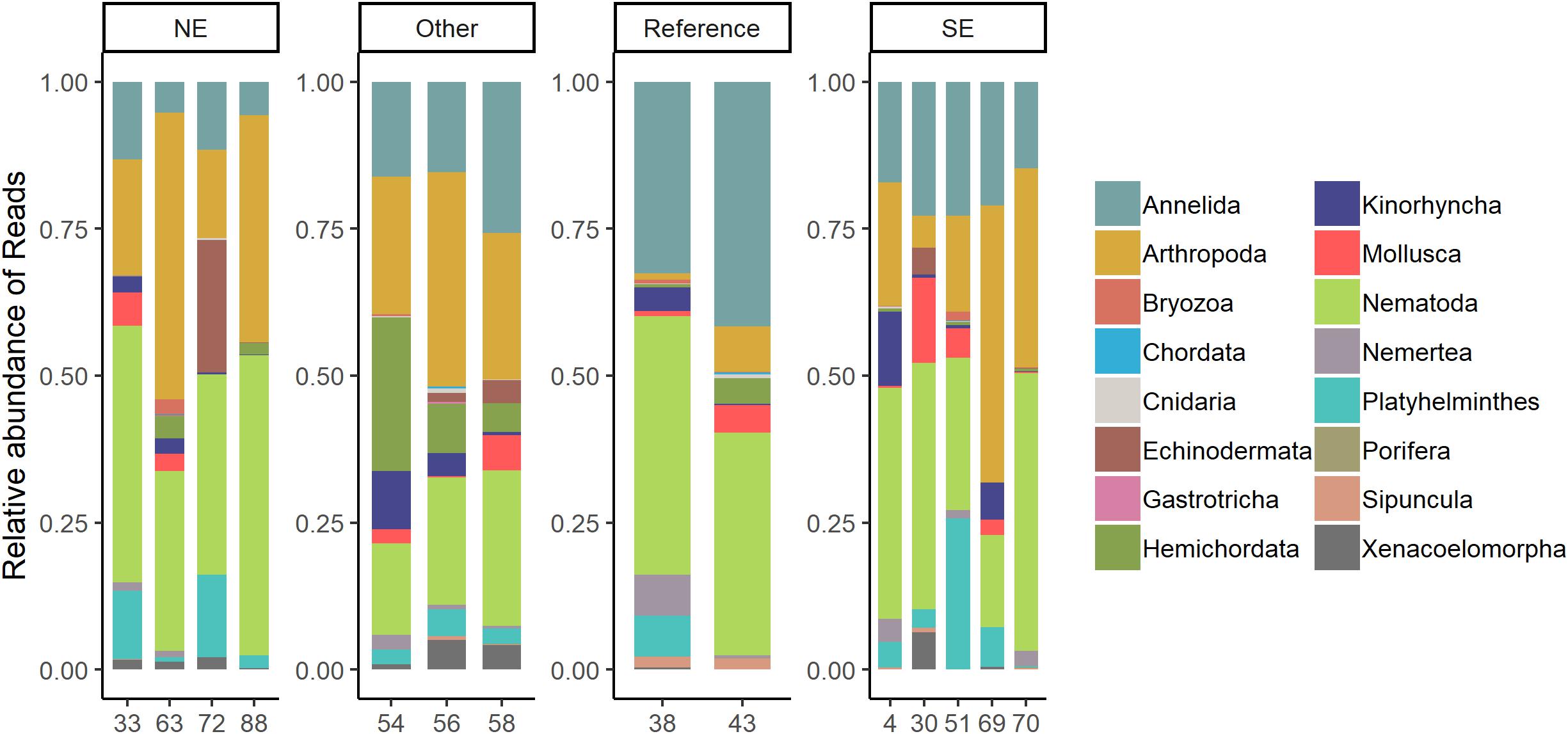
Figure 4. Phyletic composition of the benthic samples per station. For each station the relative abundance of reads per phylum is shown. Stations were clustered into four sites: plume-affected (NE, SE, and Other) and reference based on the clusters found for the trace-elements PCA (Figure 2).
Relative abundance of reads at the order level for the three most abundant phyla (Annelida, Arthropoda, and Nematoda) in each cluster are shown in Figure 5. The most pronounced difference within the annelids, is the high abundance of the order Terebellida at the SE sites whereas the order Spionida was most abundant at the other three groups of sites. The arthropod and nematode orders did show a clear distinction between the plume-affected sites (NE and SE) and the reference sites. The arthropod order Harpacticoida was the dominant order at the plume-affected sites whereas the Malacostraca was the most abundant arthropod group at the reference sites. Similarly, the nematode order Monhysterida was most abundant at the plume-affected sites whereas the Enoplida was more dominant at the reference sites.
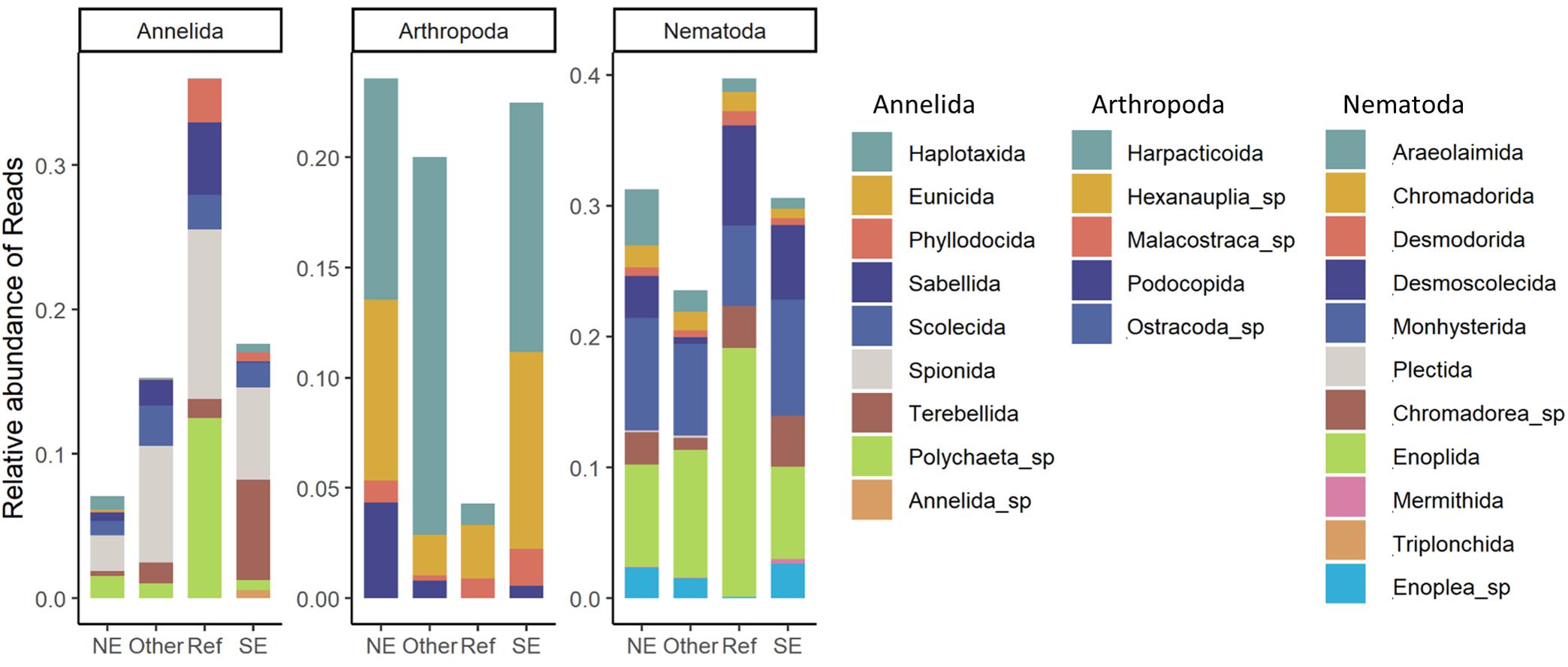
Figure 5. Taxonomic composition for the three dominant phyla. For each site (NE, SE, Other, or Reference) the relative abundance of reads for the taxonomic orders is shown.
Larvae
A total of 2.3 million metazoan reads were obtained for the pelagic larvae samples. The majority of the reads (99.6%) were assigned to pelagic groups such as the planktonic copepod taxon Calanoida; the free swimming tunicate taxon Doliolida and the krill taxon Euphausia. The remaining reads (0.4% ≈ 9,000 reads) were assigned to genuinely benthic taxa. As the number of reads for the benthic taxa were low, only the presence-absence of these taxa were scored (Table 2). Five benthic annelid taxa were present at the NE sites whereas no annelid taxa were found at the SE sites. The only benthic order found at the SE sites was the Actiniaria. Benthic taxa within the Mollusca phylum were only found at the reference sites.
Discussion
The present study applied a metabarcoding approach to analyze the composition of soft-sediment benthic assemblages from various locations a differing influence of fall out of the hydrothermal plume emitted by the Rainbow vent. The pattern observed for major- and trace metals was used to indicate the influence of hydrothermal plume fall out at the sampled locations. Also, an attempt was made to detect pelagic larvae of benthic species in the surrounding of the vent site. This study represents one of the few attempts to study the soft-sediment background communities of hydrothermal vents. Communities which are often neglected, but possibly at great risk with the emerging deep sea mining industry.
Metabarcoding for Taxonomic Identification
The combination of high-throughput sequencing and metabarcoding has been shown before as a useful methodology for community analysis (i.e., Chariton et al., 2015; Guardiola et al., 2016; Lanzén et al., 2017) and this study further exemplifies its applicability for exploring benthic deep-sea communities which are otherwise hard to analyze. Still, the method is not without caveats. A major shortcoming of metabarcoding are the considerable gaps in the reference sequence databases (Wangensteen et al., 2018). Despite growing barcoding efforts in the past decades, reference sequences for deep sea benthic species are rare (Dell’Anno et al., 2015). Very low numbers of OTUs could be identified down to the family or genus level, especially for OTUs within groups such as Bryozoans, Platyhelminthes, and Nemerteans. Although more specific taxonomic identifications were possible for the Annelida, Arthropoda, and Nematoda, most OTUs within these phyla could still not be identified beyond the family level.
The 18S rRNA gene was used to both infer the benthic community as well as the larval community. The V1-V2 region of the 18S rRNA locus targeted in this study allows identification of species across a wide taxonomic range for benthic communities (Fonseca et al., 2010; Sinniger et al., 2016). The cytochrome c oxidase subunit I gene (COI) has been used in barcoding studies for single larvae within a sanger-sequencing approach (i.e., Brandão et al., 2016) or for the analysis of larvae from secluded groups (i.e., Gollner et al., 2016). However, the COI gene has less-conserved primer binding sites as compared to the 18S rRNA gene, which might lead to false-negatives in a metabarcoding study due to primer mismatches (Deagle et al., 2014). As we were interested in larvae from all benthic species present, we chose the more conserved 18S rRNA gene as our marker. Also, the use same marker for both samples, sediment and larvae, facilitated the direct comparison of OTUs between the samples.
Within this project, we have chosen the SILVA reference database to perform the taxonomic assignment instead of GenBank. The SILVA database is a curated database and few mis-annotations exist in this database as opposed to the GenBank database (Pruesse et al., 2007; Bik et al., 2012). Although the SILVA database is argued as being too strict (Balvočiūtė and Huson, 2017; López-Escardó et al., 2018), we favored a small loss of diversity over the taxonomic annotations of false positives as these false reads might imply the incorrect presence of rare taxa.
Within this study, the relative abundance of reads for all metazoan OTUs was used as proxy for abundance. The use of amplicon data for abundancy measurements has been disputed (Porazinska et al., 2010; Elbrecht and Leese, 2015; Barnes and Turner, 2016; Pawlowski et al., 2018). However, several studies have found positive correlations between the relative abundance of reads and biomass measurements for metazoan groups including marine invertebrates (Lejzerowicz et al., 2015; Aylagas and Rodríguez-Ezpeleta, 2016). The match between actual (relative) biomass and the relative abundance of reads will not be perfect, but we believe that the relative abundance does reflect abundancy differences between major taxonomic groups. Also, the reproducibility of the PCR and Illumina sequencing as shown in this paper support our approach for abundancy measurements.
Influence of Hydrothermal Plume on Sediment and Benthic Fauna
The analysis of major- and trace metals from the sediments around the Rainbow hydrothermal vent field showed a spatial zonation separating the sample locations, which is related to a spatial pattern in plume fall out. The pattern found is likely caused by a combination of residual currents and distance from the vent site. The plume emitted by the Rainbow hydrothermal vent field has been shown to move predominantly in the NE direction, following the Rainbow Ridge (Severmann et al., 2004). Sample locations in the direct path of the plume (33, 62, 72, and 88; approximately 3 km NE of the vent site) were found to have highest element/Al molar ratios for the chalcophile elements (Zn, Cu, and Co) compared to the other sample locations. These elements precipitate rapidly from vent fluids as sulfides (Edmonds and German, 2004) and higher concentrations of these metals in the proximity of the Rainbow hydrothermal vent have been found before (Cave et al., 2002). The sample locations SE of the vent site (4, 33, 69, 70; approximately 1 km distance) were not in the trajectory of the plume depicted by Severmann et al. (2004). However, the element/Al ratios of the chalcophile elements were still higher than the ratios measured for the reference locations at 25 km from the vent sites. Plume dispersion in the SE direction was already shown (German et al., 2010; Haalboom et al., 2019), which could explain the enrichment by chalcophiles of the sediments at the SE locations. The element/Al ratios for the sediments collected at locations further away from the vent site (36, 54, 56, and 58) were more similar to the ratios found at the reference locations rather than the NE and SE locations. Only sample locations 56 showed slightly elevated levels of both the chalcophiles as well as Ba, Ni, and Mo compared to the reference locations. This location is probably both influenced by the dispersion of the plume in the NE as well as the SE direction.
The spatial patterns found in the distribution of benthic communities match the patterns found for the major- and trace metals analysis. The difference between the benthic communities at the plume-affected sites and the reference sites show an impact of the plume on the benthic communities surrounding the vent area (up to several kilometers). Spatial patterns within benthic communities surrounding hydrothermal vent areas have been shown before (Podowski et al., 2010; Boschen et al., 2016; Sen et al., 2016) and are thought to be mainly defined by chemistry rather than topography (Podowski et al., 2010; Bowden et al., 2016). The fall out of the plume toward the underlying sediments is partly due to the interaction between the chemistry of the vent fluids and the microbial communities in the plume. The vent fluids emitted differ for each vent location and are influenced by the underlying host rock (Wetzel and Shock, 2000; Cave et al., 2002). Subsequently, microbial activity alters the metal composition in the plume by scavenging and oxidation (Cowen et al., 1990; Dick et al., 2009) whereas the microbial community itself also show a shifting composition and diversity with distance from the vent site (Haalboom et al., 2019). The fall out of major elements and trace metals as well as organic matter synthesized by the chemosynthetic microbial communities could both affect the benthic communities (Sarrazin et al., 2015; Sen et al., 2016).
Distinction Between Communities
A clear difference was found between the benthic communities at the locations influenced by the plume compared to the reference sites. The relative abundances of Arthropoda were higher at the plume affected sites, whereas the Annelida and Nematoda were more dominant in the reference areas. Also, the plume-affected sites NE and SE of the vent differed from each other. Especially at the sample locations NE of the vent site (i.e., the locations most influenced by the plume), the relative abundances of Annelida were low whereas the relative abundance of Annelida in the SE were slightly higher due to a higher relative abundance of order Terebellida (Annelida). Species within this order are predominantly deposit-feeders (Zhadan and Tzetlin, 2002; Jumars et al., 2015) and rely on organic matter deposited on the seafloor. As the SE sample locations were the nearest locations to the vent site, the influx of nutrients could possibly be higher compared to the other locations (Sarrazin et al., 2015; Boschen et al., 2016). Another striking difference seen between the plume-affected sites and the reference site is the relative read abundance of Harpacticoida. This high abundance of copepods relative to other species has been shown before at nutrient rich environments such as hydrothermal vents and cold seeps (Gollner et al., 2010; Plum et al., 2015). The faunal assemblages at the other sites within this cluster (54, 56, and 58) did significantly differ from the reference location as well as from the sample locations in the direct path of the plume which implies a spatial zonation away from the vent site. Peripheral benthic communities of an active vent have been shown to be distinct up to 200 m from the vent, forming a so called ‘transition-zone’ between the vent-communities and the regular deep-sea benthic communities (Sen et al., 2014; Boschen et al., 2016). Here, we show that this pattern might be extended up to several kilometers from the vent site. As hydrothermal vents are rare and often spatially isolated within the entire deep sea, the plume-affected communities could be a potentially unique community and thereby valuable in terms of overall benthic diversity.
Detection of Larvae
A small group of genuinely benthic taxa could be detected from the water samples taken at several locations surrounding the hydrothermal vent area. Most invertebrate groups have both continuous and seasonal reproduction. Whereas groups like isopods, amphipods, cumacea, and tanaids are direct developers (brooding eggs), pelagic larvae are still common in the deep-sea, however, mainly lecithotrophic (non-feeding) (Gage and Tyler, 1991). Sea anemones (Actiniaria, Cnidaria) were detected both upstream and downstream of the vent area. The majority of species within this taxonomic group require hard substrata for their adult stages; a substrate found aplenty in the MAR area. Moreover, several species of sea anemones have been documented living on hydrothermal vent edifices (López-González et al., 2003). Actiniaria were not detected in the reference area although hard substrata were abundant in these areas as well. Their absence here was confirmed in video footage as acquired during the research cruises, and might be explained to a relatively lower abundance of Actiniaria in the reference areas, below the detection threshold, compared to the vent areas.
Most of the pelagic taxonomic groups detected are known to contain species living in the deep-sea. However, also a reasonable part of the OTUs were assigned to groups living in the epipelagic zone such as the Siphonophorae. The Siphonophorae consist of mainly pelagic species living in the upper few hundred meters of the water column, while fewer species within this group live in the deeper parts of the ocean (Mapstone, 2014). The relative abundance of reads for Siphonophorae in the water samples obtained with the LVS was close to zero, whereas the relative abundance for this taxonomic group was considerably higher for the water samples obtained with Nisking bottles, mounted on a CTD-carrousel. Many specimens of a pelagic member of this group, the Physalia physalis (Portuguese man o’war), a species bearing long sticking tentacles, were detected at the water surface during the sampling cruises and one was even found attached to the CTD frame as the sampling gear was recovered on deck. We assume the higher abundance of Siphonophorae in the Niskin-water samples was due to a difference in volume/surface ratio between the two sampling devices as a lower volume/surface ratio is prone to sampling these Physalia physalis as they might stick to the surface during the downcast and probably release vast amounts of eDNA within the sampled water. Therefore, the detection of these free swimming and floating species seems to be mainly a sampling artifact.
Possible Effect of Mining Plumes
This study shows the spatial distinction of benthic communities surrounding the Rainbow hydrothermal vent. It highlights that distinctive and potentially valuable communities for the overall deep sea benthic fauna exist on a small geographic scale. Deep sea mining is postulated to create mining plumes due to resuspension and discharge (Boschen et al., 2013; Van Dover, 2014; Miller et al., 2018). These plumes can affect the soft-sediment background fauna in two ways: (1) via increased sedimentation (Boschen et al., 2013) or, (2) via toxification by (heavy) metals becoming bioavailable upon oxidation (Van Dover, 2011; Levin et al., 2016; Weaver et al., 2018). Biological diversity was lowest at locations closest to the vent site, possibly as a result of the toxicity of the vent effluent. Mining plumes will possibly disperse by ocean currents, and the area of impact on the communities might therefore be similar to the area affected by a natural continuous vent plume, depending on the settling rates of the particles. Due to the spatial variation of benthic communities that we found in the plume’s trajectory, conservation and restoration of all these communities in the event of deep-sea mining will be difficult. The actual effects of these plumes on the soft-sediment communities are yet unknown, but our results show that these communities are different under the plume than at reference sites, a strong indication that they are affected by the plume. Therefore, it cannot be excluded that the mining of SMS deposits and the thereby created plumes will affect these unique benthic communities.
Data Availability Statement
Sequencing data have been deposited in the European Nucleotide Archive (ENA) under accession number: PRJEB36829.
Author Contributions
LK, HS, ML, G-JR, and GD conceived and designed the experiments. LK, HS, ML, and GD collected the data. LK performed the laboratory work, and LK analyzed the data, LK wrote the manuscript. HS, ML, JB, HV, G-JR, and GD reviewed the drafts of the manuscript.
Funding
This study was carried out in the framework of the TREASURE (Towards Responsible ExtrAction of SUbmarine REsources) project, funded (Grant Number 13273) by the Applied and Engineering Sciences (AES) domain of the Netherlands Organization for Scientific Research (NWO) and by partners from the Dutch maritime industry. Topsector Water, a collaborative effort of Dutch industry, academia and government, funded ship time.
Conflict of Interest
The authors declare that the research was conducted in the absence of any commercial or financial relationships that could be construed as a potential conflict of interest.
Acknowledgments
We thank Patrick Laan and Wim Boer for assistance in the chemical analyses of the collected samples and the students who helped processing all molecular samples. We also thank the crew and captain of the RV Pelagia, as well as NIOZ technicians for their essential assistance during all cruises.
Supplementary Material
The Supplementary Material for this article can be found online at: https://www.frontiersin.org/articles/10.3389/fmars.2020.00134/full#supplementary-material
References
Adams, D. K., Arellano, S. M., and Govenar, B. (2012). Larval dispersal: vent life in the water column. Oceanography 25, 256–268. doi: 10.2307/24861164
Aylagas, E., and Rodríguez-Ezpeleta, N. (2016). Analysis of Illumina MiSeq Metabarcoding Data: Application to Benthic Indices For Environmental Monitoring. New York, NY: Humana Press, 237–249. doi: 10.1007/978-1-4939-3774-5-16
Baker, E. T. E., and German, C. C. R. (2004). On the global distribution of hydrothermal vent fields. Geophys. Monogr. Ser. 148, 245–266. doi: 10.1029/148GM10
Balvočiūtė, M., and Huson, D. H. (2017). SILVA, RDP, greengenes, NCBI and OTT – how do these taxonomies compare? BMC Genomics 18:114. doi: 10.1186/s12864-017-3501-4
Barnes, M. A., and Turner, C. R. (2016). The ecology of environmental DNA and implications for conservation genetics. Conserv. Genet. 17, 1–17. doi: 10.1007/s10592-015-0775-4
Beaulieu, S. E., Sayre-Mccord, R. T., Mills, S. W., Pradillon, F., and Watanabe, H. (2015). Swimming speeds of polychaete larvae collected near deep-sea hydrothermal vents. Mar. Ecol. 36, 133–143. doi: 10.1111/maec.12207
Bik, H. M., Porazinska, D. L., Creer, S., Caporaso, J. G., Knight, R., and Thomas, W. K. (2012). Sequencing our way towards understanding global eukaryotic biodiversity. Trends Ecol. Evol. 27, 233–243. doi: 10.1016/j.tree.2011.11.010
Boschen, R. E., Rowden, A. A., Clark, M. R., and Gardner, J. P. A. (2013). Mining of deep-sea seafloor massive sulfides: a review of the deposits, their benthic communities, impacts from mining, regulatory frameworks and management strategies. Ocean Coast. Manag. 84, 54–67. doi: 10.1016/j.ocecoaman.2013.07.005
Boschen, R. E., Rowden, A. A., Clark, M. R., Pallentin, A., and Gardner, J. P. A. (2016). Seafloor massive sulfide deposits support unique megafaunal assemblages: implications for seabed mining and conservation. Mar. Environ. Res. 115, 78–88. doi: 10.1016/j.marenvres.2016.02.005
Bowden, D. A., Rowden, A. A., Leduc, D., Beaumont, J., and Clark, M. R. (2016). Deep-sea seabed habitats: do they support distinct mega-epifaunal communities that have different vulnerabilities to anthropogenic disturbance? Deep. Res. Part I Oceanogr. Res. Pap. 107, 31–47. doi: 10.1016/j.dsr.2015.10.011
Brandão, M. C., Freire, A. S., and Burton, R. S. (2016). Estimating diversity of crabs (Decapoda: Brachyura) in a no-take marine protected area of the SW Atlantic coast through DNA barcoding of larvae. Syst. Biodivers. 3, 288–302. doi: 10.1080/14772000.2016.1140245
Brannock, P. M., and Halanych, K. M. (2015). Meiofaunal community analysis by high-throughput sequencing: comparison of extraction, quality filtering, and clustering methods. Mar. Genomics 23, 67–75. doi: 10.1016/j.margen.2015.05.007
Cardoso, P., Erwin, T. L., Borges, P. A. V., and New, T. R. (2011). The seven impediments in invertebrate conservation and how to overcome them. Biol. Conserv. 144, 2647–2655. doi: 10.1016/j.biocon.2011.07.024
Cave, R. R., German, C. R., Thomson, J., and Nesbitt, R. W. (2002). Fluxes to sediments underlying the rainbow hydrothermal plume at 36°14’N on the Mid-Atlantic Ridge. Geochim. Cosmochim. Acta 66, 1905–1923. doi: 10.1016/S0016-7037(02)00823-2
Chariton, A. A., Stephenson, S., Morgan, M. J., Steven, A. D. L., Colloff, M. J., Court, L. N., et al. (2015). Metabarcoding of benthic eukaryote communities predicts the ecological condition of estuaries. Environ. Pollut. 203, 165–174. doi: 10.1016/j.envpol.2015.03.047
Charlou, J., Donval, J., Fouquet, Y., Jean-Baptiste, P., and Holm, N. (2002). Geochemistry of high H2 and CH4 vent fluids issuing from ultramafic rocks at the Rainbow hydrothermal field (36°14′N. MAR). Chem. Geol. 191, 345–359. doi: 10.1016/S0009-2541(02)00134-1
Collins, P. C., Croot, P., Carlsson, J., Colaço, A., Grehan, A., Hyeong, K., et al. (2013). A primer for the environmental impact assessment of mining at seafloor massive sulfide deposits. Mar. Policy 42, 198–209. doi: 10.1016/J.MARPOL.2013.01.020
Corinaldesi, C., Barucca, M., Luna, G. M., and Dell’Anno, A. (2011). Preservation, origin and genetic imprint of extracellular DNA in permanently anoxic deep-sea sediments. Mol. Ecol. 20, 642–654. doi: 10.1111/j.1365-294X.2010.04958.x
Cowart, D. A., Pinheiro, M., Mouchel, O., Maguer, M., Grall, J., Miné, J., et al. (2015). Metabarcoding is powerful yet still blind: a comparative analysis of morphological and molecular surveys of seagrass communities. PLoS One 10:e0117562. doi: 10.1371/journal.pone.0117562
Cowen, J. P., Massoth, G. J., and Feely, R. A. (1990). Scavenging rates of dissolved manganese in a hydrothermal vent plume. Deep Sea Res. Part A Oceanogr. Res. Pap. 37, 1619–1637. doi: 10.1016/0198-0149(90)90065-4
Cowen, R. K., and Sponaugle, S. (2009). Larval dispersal and marine population connectivity. Ann. Rev. Mar. Sci. 1, 443–466. doi: 10.1146/annurev.marine.010908.163757
Danovaro, R., Snelgrove, P. V. R., and Tyler, P. (2014). Challenging the paradigms of deep-sea ecology. Trends Ecol. Evol. 29, 465–475. doi: 10.1016/J.TREE.2014.06.002
Deagle, B. E., Jarman, S. N., Coissac, E., Pompanon, F., and Taberlet, P. (2014). DNA metabarcoding and the cytochrome c oxidase subunit I marker: not a perfect match. Biol. Lett. 10(20140562). doi: 10.1098/rsbl.2014.0562
Dell’Anno, A., Carugati, L., Corinaldesi, C., Riccioni, G., and Danovaro, R. (2015). Unveiling the biodiversity of deep-sea nematodes through metabarcoding: are we ready to bypass the classical taxonomy? PLoS One 10:e0144928. doi: 10.1371/journal.pone.0144928
Desbruyères, D., and Seqonzac, M. (1997). Handbook of Deep-Sea Hydrothermal Vent Fauna. Brest: IFREMER.
Dick, G. J., Clement, B. G., Webb, S. M., Fodrie, F. J., Bargar, J. R., and Tebo, B. M. (2009). Enzymatic microbial Mn(II) oxidation and Mn biooxide production in the Guaymas Basin deep-sea hydrothermal plume. Geochim. Cosmochim. Acta 73, 6517–6530. doi: 10.1016/J.GCA.2009.07.039
Douville, E., Charlou, J., Oelkers, E., Bienvenu, P., Jove Colon, C., Donval, J., et al. (2002). The rainbow vent fluids (36°14′N, MAR): the influence of ultramafic rocks and phase separation on trace metal content in Mid-Atlantic Ridge hydrothermal fluids. Chem. Geol. 184, 37–48. doi: 10.1016/S0009-2541(01)00351-5
Duinveld, G. Shipboard Scientific Party (2016). Report Pelagia cruise 64pe412 TREASURE (Towards Responsible ExtrAction of SUbmarine Resources). Horta – Horta 25 June – 13 July 2016. NIOZ-report, 41. Genève: Zenodo.
Edmonds, H. N., and German, C. R. (2004). Particle geochemistry in the rainbow hydrothermal plume, mid-atlantic ridge. Geochim. Cosmochim. Acta 68, 759–772. doi: 10.1016/S0016-7037(03)00498-8
Elbrecht, V., and Leese, F. (2015). Can DNA-based ecosystem assessments quantify species abundance? Testing primer bias and biomass-sequence relationships with an innovative metabarcoding protocol. PLoS One 10:e0130324. doi: 10.1371/journal.pone.0130324
Findlay, A. J., Gartman, A., Shaw, T. J., and Luther, G. W. (2015). Trace metal concentration and partitioning in the first 1.5 m of hydrothermal vent plumes along the Mid-Atlantic Ridge: TAG, Snakepit, and Rainbow. Chem. Geol. 412, 117–131. doi: 10.1016/J.CHEMGEO.2015.07.021
Fonseca, V. G., Carvalho, G. R., Sung, W., Johnson, H. F., Power, D. M., Neill, S. P., et al. (2010). Second-generation environmental sequencing unmasks marine metazoan biodiversity. Nat. Commun. 1:98. doi: 10.1038/ncomms1095
Fouquet, Y., Charlou, J. L., and Ondreas, H. (1997). Discovery and first submersible investigations on the Rainbow hydrothermal field on the MAR. EOS Trans. AGU 78(46).
Gage, J. D., and Tyler, P. A. (1991). Deep-Sea Biology. A Natural History of Organisms at The Deep-Sea Floor. Cambridge: Cambridge University Press, 504.
Galkin, S. V. (1997). Megafauna associated with hydrothermal vents in the Manus Back-Arc Basin (Bismarck Sea). Mar. Geol. 142, 197–206. doi: 10.1016/S0025-3227(97)00051-0
German, C. R., and Parson, L. M. (1996). Hydrothermal exploration near the azores triple junction: tectonic control of venting at slow-spreading ridges? Earth Planet. Sci. Lett. 138, 93–104. doi: 10.1016/0012-821X(95)00224-Z
German, C. R., Richards, K. J., Rudnicki, M. D., Lam, M. M., and Charlou, J. L. (1998). Topographic control of a dispersing hydrothermal plume. Earth Planet. Sci. Lett. 156, 267–273. doi: 10.1016/S0012-821X(98)00020-X
German, C. R., Thurnherr, A. M., Knoery, J., Charlou, J. L., Jean-Baptiste, P., and Edmonds, H. N. (2010). Heat, volume and chemical fluxes from submarine venting: a synthesis of results from the Rainbow hydrothermal field, 36°N MAR. Deep. Res. Part I Oceanogr. Res. Pap. 57, 518–527. doi: 10.1016/j.dsr.2009.12.011
Gollner, S., Govenar, B., Arbizu, P. M., Mills, S., Le Bris, N., Weinbauer, M., et al. (2015). Differences in recovery between deep-sea hydrothermal vent and vent-proximate communities after a volcanic eruption. Deep. Res. Part I Oceanogr. Res. Pap. 106, 167–182. doi: 10.1016/j.dsr.2015.10.008
Gollner, S., Riemer, B., Arbizu, P. M., Le Bris, N., and Bright, M. (2010). Diversity of meiofauna from the 9 50’n East Pacific Rise across a gradient of hydrothermal fluid emission. PLoS One 5:e12321. doi: 10.1371/journal.pone.0012321
Gollner, S., Stuckas, H., Kihara, T. C., Laurent, S., Kodami, S., and Martinez Arbizu, P. (2016). Mitochondrial DNA analyses indicate high diversity, expansive population growth and high genetic connectivity of vent copepods (Dirivultidae) across different oceans. PLoS One 11:e0163776. doi: 10.1371/journal.pone.0163776
Guardiola, M., Uriz, M. J., Taberlet, P., Coissac, E., Wangensteen, O. S., and Turon, X. (2015). Deep-sea, deep-sequencing: metabarcoding extracellular DNA from sediments of marine canyons. PLoS One 10:e0139633. doi: 10.1371/journal.pone.0139633
Guardiola, M., Wangensteen, O. S., Taberlet, P., Coissac, E., Uriz, M. J., and Turon, X. (2016). Spatio-temporal monitoring of deep-sea communities using metabarcoding of sediment DNA and RNA. PeerJ 4:e2807. doi: 10.7717/peerj.2807
Haalboom, S., Price, D. M., Mienis, F., van Bleijswijk, J. D. L., de Stigter, H. C., Witte, H. J., et al. (2019). Successional patterns of (trace) metals and microorganisms in the rainbow hydrothermal vent plume at the Mid-Atlantic ridge. Biogeosci. Discuss. doi: 10.5194/bg-2019-189
Hebert, P. D. N., Cywinska, A., Ball, S. L., and deWaard, J. R. (2003). Biological identifications through DNA barcodes. Proc. R. Soc. B Biol. Sci. 270, 313–321. doi: 10.1098/rspb.2002.2218
Jumars, P. A., Dorgan, K. M., and Lindsay, S. M. (2015). Diet of worms emended: an update of polychaete feeding guilds. Ann. Rev. Mar. Sci. 7, 497–520. doi: 10.1146/annurev-marine-010814-020007
Khripounoff, A., Vangriesheim, A., Crassous, P., Segonzac, M., Colaço, A., Desbruyères, D., et al. (2001). Particle flux in the rainbow hydrothermal vent field (Mid-Atlantic Ridge): dynamics, mineral and biological composition. J. Mar. Res. 59, 633–656. doi: 10.1357/002224001762842217
Klunder, L., Duineveld, G. C. A., Lavaleye, M. S. S., van der Veer, H. W., Palsbøll, P. J., and van Bleijswijk, J. D. L. (2019). Diversity of Wadden Sea macrofauna and meiofauna communities highest in DNA from extractions preceded by cell lysis. J. Sea Res. 152, 101764. doi: 10.1016/J.SEARES.2019.101764
Klunder, L., Lavaleye, M. S. S., Filippidi, A., van Bleijswijk, J. D. L., Reichart, G.-J., van der Veer, H. W., et al. (2018). Impact of an artificial structure on the benthic community composition in the southern North Sea: assessed by a morphological and molecular approach. ICES J. Mar. Sci. fsz252 [Preprint]. doi: 10.1093/icesjms/fsy114
Lanzén, A., Lekang, K., Jonassen, I., Thompson, E. M., and Troedsson, C. (2016). High-throughput metabarcoding of eukaryotic diversity for environmental monitoring of offshore oil-drilling activities. Mol. Ecol. 25, 4392–4406. doi: 10.1111/mec.13761
Lanzén, A., Lekang, K., Jonassen, I., Thompson, E. M., and Troedsson, C. (2017). DNA extraction replicates improve diversity and compositional dissimilarity in metabarcoding of eukaryotes in marine sediments. PLoS One 12:e0179443. doi: 10.1371/journal.pone.0179443
Legendre, P., and Gallagher, E. D. (2001). Ecologically meaningful transformations for ordination of species data. Oecologia 129, 271–280. doi: 10.1007/s004420100716
Lejzerowicz, F., Esling, P., Pillet, L., Wilding, T. A., Black, K. D., and Pawlowski, J. (2015). High-throughput sequencing and morphology perform equally well for benthic monitoring of marine ecosystems. Sci. Rep. 5:13932. doi: 10.1038/srep13932
Levin, L. A., Baco, A. R., Bowden, D. A., Colaco, A., Cordes, E. E., Cunha, M. R., et al. (2016). Hydrothermal vents and methane seeps: rethinking the sphere of influence. Front. Mar. Sci. 3:72. doi: 10.3389/fmars.2016.00072
López-Escardó, D., Paps, J., de Vargas, C., Massana, R., Ruiz-Trillo, I., and del Campo, J. (2018). Metabarcoding analysis on European coastal samples reveals new molecular metazoan diversity. Sci. Rep. 8:9106. doi: 10.1038/s41598-018-27509-8
López-González, P. J., Rodríguez, E., Gili, J.-M., and Segonzac, M. (2003). New records on sea anemones (Anthozoa: Actiniaria) from hydrothermal vents and cold seeps. Zool. Verh. 345, 215–243.
Mapstone, G. M. (2014). Global diversity and review of Siphonophorae (Cnidaria: Hydrozoa). PLoS One 9:e87737. doi: 10.1371/journal.pone.0087737
McMurdie, P. J., and Holmes, S. (2014). Waste not, want not: Why rarefying microbiome data is inadmissible. PLoS Comput. Biol. 10:e1003531. doi: 10.1371/journal.pcbi.1003531
Metaxas, A. (2004). Spatial and temporal patterns in larval supply at hydrothermal vents in the northeast Pacific Ocean. Limnol. Oceanogr. 49, 1949–1956. doi: 10.4319/lo.2004.49.6.1949
Miller, K. A., Thompson, K. F., Johnston, P., and Santillo, D. (2018). An overview of seabed mining including the current state of development, environmental impacts, and knowledge gaps. Front. Mar. Sci. 4:418. doi: 10.3389/fmars.2017.00418
Mullineaux, L. S., Adams, D. K., Mills, S. W., and Beaulieu, S. E. (2010). Larvae from afar colonize deep-sea hydrothermal vents after a catastrophic eruption. Proc. Natl. Acad. Sci. U.S.A. 107, 7829–7834. doi: 10.1073/pnas.0913187107
Nakajima, R., Yamamoto, H., Kawagucci, S., Takaya, Y., Nozaki, T., Chen, C., et al. (2015). Post-drilling changes in seabed landscape and megabenthos in a deep-sea hydrothermal system, the Iheya North field, Okinawa Trough. PLoS One 10:e0123095. doi: 10.1371/journal.pone.0123095
Oksanen, J., Blanchet, F. G., Friendly, M., Kindt, R., Legendre, P., McGlinn, D., et al. (2018). VEGAN: Community Ecology Package. R Package Version 2.5-2. Available online at: https://CRAN.R-project.org/package=vegan
Pawlowski, J., Kelly-Quinn, M., Altermatt, F., Apothéloz-Perret-Gentil, L., Beja, P., Boggero, A., et al. (2018). The future of biotic indices in the ecogenomic era: integrating (e)DNA metabarcoding in biological assessment of aquatic ecosystems. Sci. Total Environ. 63, 1295–1310. doi: 10.1016/j.scitotenv.2018.05.002
Plum, C., Gollner, S., Martinez-Arbizu, P., and Bright, M. (2015). Diversity and composition of the copepod communities associated with megafauna around a cold seep in the Gulf of Mexico with remarks on species biogeography. Mar. Biodivers. 4, 419–432. doi: 10.1007/s12526-014-0310-8
Podowski, E., Ma, S., Luther, G., Wardrop, D., and Fisher, C. (2010). Biotic and abiotic factors affecting distributions of megafauna in diffuse flow on andesite and basalt along the eastern lau spreading center, Tonga. Mar. Ecol. Prog. Ser. 418, 25–45. doi: 10.3354/meps08797
Porazinska, D. L., Sung, W., Giblin-Davis, R. M., and Thomas, W. K. (2010). Reproducibility of read numbers in high-throughput sequencing analysis of nematode community composition and structure. Mol. Ecol. Resour. 10, 666–676. doi: 10.1111/j.1755-0998.2009.02819.x
Pruesse, E., Quast, C., Knittel, K., Fuchs, B. M., Ludwig, W., Peplies, J., et al. (2007). SILVA: a comprehensive online resource for quality checked and aligned ribosomal RNA sequence data compatible with ARB. Nucleic Acids Res. 35, 7188–7196. doi: 10.1093/nar/gkm864
Sarrazin, J., Legendre, P., de Busserolles, F., Fabri, M. C., Guilini, K., Ivanenko, V. N., et al. (2015). Biodiversity patterns, environmental drivers and indicator species on a high-temperature hydrothermal edifice, Mid-Atlantic Ridge. Deep. Res. Part II Top. Stud. Oceanogr. 121, 177–192. doi: 10.1016/j.dsr2.2015.04.013
Sen, A., Kim, S., Miller, A. J., Hovey, K. J., Hourdez, S., Luther, G. W., et al. (2016). Peripheral communities of the Eastern lau spreading center and Valu Fa ridge: community composition, temporal change and comparison to near-vent communities. Mar. Ecol. 37, 599–617. doi: 10.1111/maec.12313
Sen, A., Podowski, E. L., Becker, E. L., Shearer, E. A., Gartman, A., Yücel, M., et al. (2014). Community succession in hydrothermal vent habitats of the Eastern Lau Spreading Center and Valu Fa Ridge. Tonga. Limnol. Oceanogr. 59, 1510–1528. doi: 10.4319/lo.2014.59.5.1510
Severmann, S., Johnson, C. M., Beard, B. L., German, C. R., Edmonds, H. N., Chiba, H., et al. (2004). The effect of plume processes on the Fe isotope composition of hydrothermally derived Fe in the deep ocean as inferred from the Rainbow vent site, Mid-Atlantic Ridge, 36°14′N. Earth Planet. Sci. Lett. 225, 63–76. doi: 10.1016/j.epsl.2004.06.001
Sinniger, F., Pawlowski, J., Harii, S., Gooday, A. J., Yamamoto, H., Chevaldonné, P., et al. (2016). Worldwide analysis of sedimentary DNA reveals major gaps in taxonomic knowledge of deep-sea benthos. Front. Mar. Sci. 3:92. doi: 10.3389/fmars.2016.00092
Taberlet, P., Coissac, E., Pompanon, F., Brochmann, C., and Willerslev, E. (2012a). Towards next-generation biodiversity assessment using DNA metabarcoding. Mol. Ecol. 21, 2045–2050. doi: 10.1111/j.1365-294X.2012.05470.x
Taberlet, P., Prud’Homme, S. M., Campione, E., Roy, J., Miquel, C., Shehzad, W., et al. (2012b). Soil sampling and isolation of extracellular DNA from large amount of starting material suitable for metabarcoding studies. Mol. Ecol. 21, 1816–1820. doi: 10.1111/j.1365-294X.2011.05317.x
Van Dover, C. L. (2011). Mining seafloor massive sulphides and biodiversity: what is at risk? ICES J. Mar. Sci. 68, 341–348. doi: 10.1093/icesjms/fsq086
Van Dover, C. L. (2014). Impacts of anthropogenic disturbances at deep-sea hydrothermal vent ecosystems: a review. Mar. Environ. Res. 102, 59–72. doi: 10.1016/j.marenvres.2014.03.008
Vanreusel, A., Fonseca, G., Danovaro, R., Da Silva, M. C., Esteves, A. M., Ferrero, T., et al. (2010). The contribution of deep-sea macrohabitat heterogeneity to global nematode diversity. Mar. Ecol. 31, 6–20. doi: 10.1111/j.1439-0485.2009.00352.x
Vic, C., Gula, J., Roullet, G., and Pradillon, F. (2018). Dispersion of deep-sea hydrothermal vent effluents and larvae by submesoscale and tidal currents. Deep Sea Res. Part I Oceanogr. Res. Pap. 133, 1–18. doi: 10.1016/J.DSR.2018.01.001
Wang, Q., Garrity, G. M., Tiedje, J. M., and Cole, J. R. (2007). Naïve Bayesian classifier for rapid assignment of rRNA sequences into the new bacterial taxonomy. Appl. Environ. Microbiol. 73, 5261–5267. doi: 10.1128/AEM.00062-07
Wangensteen, O. S., Palacín, C., Guardiola, M., and Turon, X. (2018). DNA metabarcoding of littoral hard-bottom communities: high diversity and database gaps revealed by two molecular markers. PeerJ 6:e4705. doi: 10.7717/peerj.4705
Weaver, P. P. E., Billett, D. S. M., and Van Dover, C. L. (2018). Environmental Risks Of Deep-Sea Mining, In: Handbook On Marine Environment Protection. Cham: Springer International Publishing.
Wetzel, L. R., and Shock, E. L. (2000). Distinguishing ultramafic-from basalt-hosted submarine hydrothermal systems by comparing calculated vent fluid compositions. J. Geophys. Res. Solid Earth 105, 8319–8340. doi: 10.1029/1999JB900382
Xu, G., McGillicuddy, D. J., Mills, S. W., and Mullineaux, L. S. (2018). Dispersal of hydrothermal vent larvae at East-Pacific Rise 9-10°N Segment. J. Geophys. Res. Ocean. 123, 7877–7895. doi: 10.1029/2018JC014290
Zeppilli, D., and Danovaro, R. (2009). Meiofaunal diversity and assemblage structure in a shallow-water hydrothermal vent in the Pacific Ocean. Aquat. Biol. 5, 75–84. doi: 10.3354/ab00140
Keywords: metabarcoding, deep sea mining, background fauna, hydrothermal vents, plume, larvae
Citation: Klunder L, de Stigter H, Lavaleye MSS, van Bleijswijk JDL, van der Veer HW, Reichart G-J and Duineveld GCA (2020) A Molecular Approach to Explore the Background Benthic Fauna Around a Hydrothermal Vent and Their Larvae: Implications for Future Mining of Deep-Sea SMS Deposits. Front. Mar. Sci. 7:134. doi: 10.3389/fmars.2020.00134
Received: 08 October 2019; Accepted: 19 February 2020;
Published: 06 March 2020.
Edited by:
Iliana B. Baums, Pennsylvania State University (PSU), United StatesReviewed by:
Jian-Wen Qiu, Hong Kong Baptist University, Hong KongLeocadio Blanco Bercial, Bermuda Institute of Ocean Sciences (BIOS), Bermuda
Copyright © 2020 Klunder, de Stigter, Lavaleye, van Bleijswijk, van der Veer, Reichart and Duineveld. This is an open-access article distributed under the terms of the Creative Commons Attribution License (CC BY). The use, distribution or reproduction in other forums is permitted, provided the original author(s) and the copyright owner(s) are credited and that the original publication in this journal is cited, in accordance with accepted academic practice. No use, distribution or reproduction is permitted which does not comply with these terms.
*Correspondence: Lise Klunder, bGlzZS5rbHVuZGVyQG5pb3oubmw=