- 1Division of Marine Science and Conservation, Nicholas School of the Environment, Duke University, Beaufort, NC, United States
- 2School of Biology and Environmental Science, College of Science, University College Dublin, Dublin, Ireland
- 3Faculty of Sciences, Ghent University, Ghent, Belgium
- 4Department of Marine, Earth, and Atmospheric Sciences, College of Sciences, North Carolina State University, Raleigh, NC, United States
- 5The King's School, Canterbury, United Kingdom
- 6Oregon Institute of Marine Biology, Department of Biology, University of Oregon, Charleston, OR, United States
Methane seeps provide a variety of ecosystem services, including the provision of complex habitat structures and high levels of primary production, which can act as trophic support to non-seep-endemic species in an otherwise food-limited environment. The discovery of hundreds of seeps on the US Atlantic margin, ranging in depth from ~50 to 1,700 m, provides the opportunity to assess depth-related differences in seep-associated communities. Here, we use photo transects to characterize the megafaunal communities at six seeps along the US Atlantic margin, comparing taxonomic richness and community structure (taxon-abundance patterns) at shallow (~400 m) and deep (~1,500 m) seeps. We use molecular analysis to identify the mussel species present and stable isotope analysis to explore the trophic ecology of bathymodiolin mussels and red crabs (Chaceon quinquedens). Our results suggest a faunal boundary exists between shallow and deep seeps; depth, and the co-varying factor temperature, explained 72% of the variation observed in taxon-abundance patterns. All mussel samples were identified as Bathymodiolus childressi, extending the known dominance of B. childressi at seeps near Baltimore Canyon to seeps off New England. Stable isotope analyses suggest B. childressi relies predominantly, if not entirely, on methane-derived nutrition at both shallow and deep seeps. For red crab, the proportion of methane-derived carbon within muscle tissue is highly variable, contributing ~0% of nutrition for crabs sampled at Shallop East and West but ~30 and 50% of nutrition for two individuals sampled at Chincoteague East. In addition to red crabs using seeps as a food resource, invertebrate larvae samples and observational data suggests Chincoteague East may act as a reproductive hotspot for red crabs. Fifteen mating pairs, three ovigerous females, and numerous zoea larvae (identified as belonging to C. quinquedens) were observed at or above Chincoteague East, providing what we believe is the first evidence that some seeps may act as a reproductive hotspot for a commercially valuable species. This study highlights two ways that seeps may support fishery productivity (i.e., providing trophic support and increasing reproductive success) and encourages future research exploring the connection between deep-sea chemosynthetic ecosystems and commercially valuable species.
Introduction
Methane seeps are highly productive ecosystems where fluids enriched in methane and hydrogen sulfide “seep” out of the surface sediment, supporting chemoautotrophic production by both free-living and symbiotic microbes (Tunnicliffe et al., 2003; Torres and Bohrmann, 2014). Methane seeps are often characterized by symbiont-bearing megafauna (e.g., tubeworms and mussels), which form biogenic habitats that support a variety of additional species (Sibuet and Olu, 1998; Tunnicliffe et al., 2003; Govenar, 2010). Until recently, only three areas of methane seepage were recognized on the US Atlantic margin: a dense mussel community near Baltimore Canyon observed in the early 1980s (B. Hecker, cited in Prouty et al., 2016), and chemosynthetic communities associated with the Blake Ridge (Paull et al., 1995; Van Dover et al., 2003) and Cape Fear salt diapirs (Brothers et al., 2013; Wagner et al., 2013). It was not until 2012 that the US Atlantic Margin was recognized for widespread methane seepage following the discovery of ~570 gas plumes between Cape Hatteras and Georges Bank (Skarke et al., 2014). The seeps north of Cape Hatteras range in depth from 50 to 1,700 m along ~950 km of the continental shelf (Skarke et al., 2014), providing the opportunity to assess differences in biodiversity across a range of shallow and deep seeps.
Depth has been recognized as an important factor in structuring biological communities throughout the deep sea (Gage and Tyler, 1991; Carney, 2005). For example, macro-infaunal communities at seeps follow depth-related patterns in biodiversity throughout the world's oceans, with different macro-infaunal communities occupying upper-bathyal (200–1,500 m) and lower-bathyal/abyssal (>1,500 m) depths (Bernardino et al., 2012). On the US Atlantic margin, different macro-infaunal communities have been found at seeps near Baltimore (~400 m) and Norfolk Canyon (~1,500 m; Bourque et al., 2017), further supporting this depth-related faunal separation. A faunal boundary around 1,300–1,500 m has been well-documented for non-seep endemic echinoderms, decapod crustaceans, and demersal fish across the western North Atlantic (Musick, 1979; Haedrich et al., 1980; Snelgrove and Haedrich, 1985; Baker et al., 2012; Quattrini et al., 2015; Ross et al., 2015), but the presence of depth-related separation has not been investigated for benthic megafaunal seep communities.
In addition to depth, environmental variables such as food availability, temperature, latitude, and substrate type are known to influence biodiversity patterns and community composition (Rex et al., 2000; Levin et al., 2001; Yasuhara and Danovaro, 2016). Species diversity tends to vary unimodally with food availability (measured as particulate organic carbon [POC] flux), peaking at moderate levels of productivity (Levin et al., 2001; Leduc et al., 2012; McClain et al., 2012). Species diversity may also vary unimodally with temperature, peaking at moderate temperatures in the range of ~5–10°C (Yasuhara and Danovaro, 2016). Temperature may only play an important role in structuring communities at relatively high (>10°C) or low (<5°C) levels (Yasuhara and Danovaro, 2016), consistent with the 4°C isotherm that often defines a faunal boundary within the deep sea (Gage and Tyler, 1991) and may partially explain the faunal break in demersal fish populations observed at Baltimore and Norfolk Canyon (Ross et al., 2015). At intermediate temperatures, factors such as POC flux may play a more important role in determining diversity patterns (Yasuhara and Danovaro, 2016). However, understanding the impact of food availability on community structure is made more complex by latitudinal differences in productivity (Lambshead et al., 2002), and a general decrease in POC flux as depth (Suess, 1980; Martin et al., 1987) and distance from shore increases (Cosson et al., 1997; Levin et al., 2001). At seep ecosystems specifically, the presence of authigenic carbonate and the type of biogenic habitat present (e.g., tubeworm bushes, mussel beds) are also known to influence community composition (Cordes et al., 2009, 2010). Community composition can also vary within a biogenic habitat due to variation in fluid flux and chemical composition, which determine the distribution of symbiont-bearing megafauna (MacDonald et al., 1989; Cordes et al., 2010). For example, the proportion of live and dead mussels has been shown to influence the community structure of demersal fish at seeps near Baltimore Canyon on the US Atlantic margin (Ross et al., 2015).
Seeps north of Cape Hatteras are dominated by bathymodiolin mussels (Skarke et al., 2014; Quattrini et al., 2015; Ross et al., 2015; Prouty et al., 2016; Bourque et al., 2017; McVeigh et al., 2018; Coykendall et al., 2019), with Bathymodiolus childressi prevalent at Norfolk, Chincoteague, and Baltimore seeps (Coykendall et al., 2019). In addition to harboring methanotrophic endosymbionts, B. childressi is capable of filter feeding (Page et al., 1990; Pile and Young, 1999) and thus has the potential to use both photosynthetically and chemosynthetically-derived food resources (Tyler et al., 2007). Since the quantity of photosynthetically-derived carbon (POC flux) available to benthic communities decreases with depth (e.g., Suess, 1980; Martin et al., 1987), the importance of photosynthetically-derived food resources may be greater at shallow seeps than at deep seeps. To our knowledge, the importance of photosynthetically-derived carbon to the nutrition of symbiont-bearing megafauna has not been tested as a function of depth for seep ecosystems. This pattern has been observed at hydrothermal vent systems, where a species of Rimicaris shrimp uses photosynthetically-derived food resources at the Von Damm vent system (2,300 m) but not at the deeper Piccard vent system (4,980 m; Bennett et al., 2015).
Bathymodiolin mussels often dominate biomass at seeps and act as habitat engineers, increasing habitat complexity, and modifying the physical and chemical environment to allow other fauna to colonize (Govenar, 2010). Seep-associated fauna are described as either a resident or vagrant species, with vagrant species roaming between the seep and surrounding seabed, foraging on a combination of photosynthetically and chemosynthetically-derived food resources (MacAvoy et al., 2002). In a recent study of food-web dynamics at Baltimore and Norfolk seeps, the fishes Dysommina rugosa and Symphurus nebulosus, the asteroid Odontaster robustus and the sea urchins Echinus wallisi and Gracilechinus affinis have been shown to utilize chemosynthetically-derived food resources (Demopoulos et al., 2019). Similarly, in the Gulf of Mexico, the eel Synaphobranchus sp., the hake Urophycis cirratus, and the commercially important deep-sea red crab (Chaceon quinquedens; referred to herein as “red crab”) are known to be seep vagrants, with some dependence on chemosynthetic food resources (MacAvoy et al., 2002). Vagrant species can transfer chemosynthetically-derived production to the surrounding environment (Levin et al., 2016a) and, when the species is commercially exploited, potentially transfer seep-derived carbon to humans.
Methane seeps are recognized for a multitude of ecosystem services, including the provision of habitat and food resources for a variety of deep-sea species (Armstrong et al., 2012; Niemann et al., 2013; Thurber et al., 2014; Levin et al., 2016a). Supporting services (e.g., primary and secondary productivity, breeding and nursery sites) are necessary to maintain the ecosystem services that contribute directly to human well-being, including all provisioning services (e.g., fish catch), regulating services (e.g., climate regulation) and cultural services (e.g., inspiration for the arts; MEA, 2005; Le et al., 2017). For example, intense levels of primary and secondary productivity present at seeps have long been hypothesized to contribute to fishery productivity on the continental margins of Europe (Hovland et al., 1985; Judd and Hovland, 1989; Berndt, 2005) and Canada (Levy and Lee, 1988). Recently, molecular gut content analysis and compound specific stable isotope analysis has shown that seeps off the west coast of Canada provide some nutritional support to the commercially important tanner crab (Chionoecetes tanneri), which formed dense aggregations around the Barkley Canyon seep in 2014 (Seabrook et al., 2019). Seeps are also recognized as reproductive hotspots for octopus (Graneledone sp.), sculpin (Psychrolutes phrictus; Drazen et al., 2003) and skates (Treude et al., 2011), and may have served as nursery sites for catsharks over millions of years (Treude et al., 2011). Breeding and nursery sites are important areas to conserve for maintaining species populations and ensuring reproductive success (Beck et al., 2001; Roberts et al., 2005). When the species is commercially fished, degradation of breeding sites by human activities may result in decreased fisheries landings, i.e., the provisioning service upon which humans derive benefit (Le et al., 2017).
On the US Atlantic margin, the commercially important red crab occurs at seeps near Baltimore and Norfolk Canyon (Ross et al., 2015), as well as Shallop Canyon, Veatch Canyon and at New England Seep 2 off the coast of New England (Quattrini et al., 2015; McVeigh et al., 2018). Red crab is caught by a small (4–5 vessel) fishing cooperative that targets male crabs at a depth of 400–800 m along the continental shelf, from the US-Canadian border to Cape Hatteras, North Carolina (Wahle et al., 2008). The fishery catches ~2,000 mt (~4.4 million pounds) of crab each year (NEFSC, 2006), with an average revenue of ~$3.1 million USD per annum (NEFMC, 2013). Red crab occur in high densities at some seeps (Quattrini et al., 2015; McVeigh et al., 2018), reaching an abundance of ~1.3 individuals 10 m−2 at New England Seep 2 (Quattrini et al., 2015). Adult male and female crabs generally occupy different depth zones, with females occupying shallower depths (Wigley et al., 1975). Female crabs undergo a downslope migration, either individually or by being carried by males that have migrated upslope, with reproduction occurring at mid-slope depths (Hastie, 1995). The high density of red crab at some US Atlantic margin seeps leads us to ask whether seeps could act as a reproductive hotspot, as well as a food resource, for red crabs.
In this study, using bathymodiolin mussel samples collected at each seep, we ask: (1) what mussel species is present at seeps north of Cape Hatteras? (2) Do seep mussels use photosynthetically as well as methane-derived food resources? We expected that (1) mussel beds will be dominated by Bathymodiolus childressi, which is already known from Norfolk, Chincoteague and Baltimore seeps (Coykendall et al., 2019), and (2) that mussels sampled from shallow seeps may use a combination of photosynthetically and methane-derived food resources, whereas mussels at deep seeps will rely on methane-derived nutrition because the availability of photosynthetically-derived food resources decreases with depth (e.g., Suess, 1980; Martin et al., 1987).
Using photo transect data we set out to answer the following three basic questions regarding seep-associated megafauna on the US Atlantic margin: (1) what megafauna, including both benthic invertebrates and demersal fishes, are present? (2) Do megafaunal communities differ between shallow (~400 m) and deep (~1,500 m) seeps? (3) What abiotic factors correlate with patterns of taxon-abundance? Based on previous studies and our current understanding of seep ecology and biogeography, we expected that shallow and deep seeps support different megafaunal communities, correlated with factors (e.g., temperature and food availability) that co-vary with depth.
Lastly, following the observation of the commercially exploited red crab (Chaceon quinquedens) at a number of seep sites, we explore whether seeps may act as a food resource and (or) reproductive hotspot for red crab using a combination of carbon stable isotope analysis, larvae samples, and observational data.
Materials and Methods
HOV Alvin dives were conducted at nine seeps along the US Atlantic margin in 2015 (R/V Atlantis cruise AT29-04, CLVD, Chief Scientist; 28 July−7 August; Figure 1). Four seeps were located at ~400 m depth (shallow): Pick-Up Sticks, Baltimore, Shallop East and Shallop West (Table 1). One seep was located at ~1,000 m (intermediate depth): Chincoteague East (Table 1). Four seeps were located at ~1,500 m depth (deep): Norfolk East, Norfolk West, Veatch, and New England Seep 2 (Table 1).
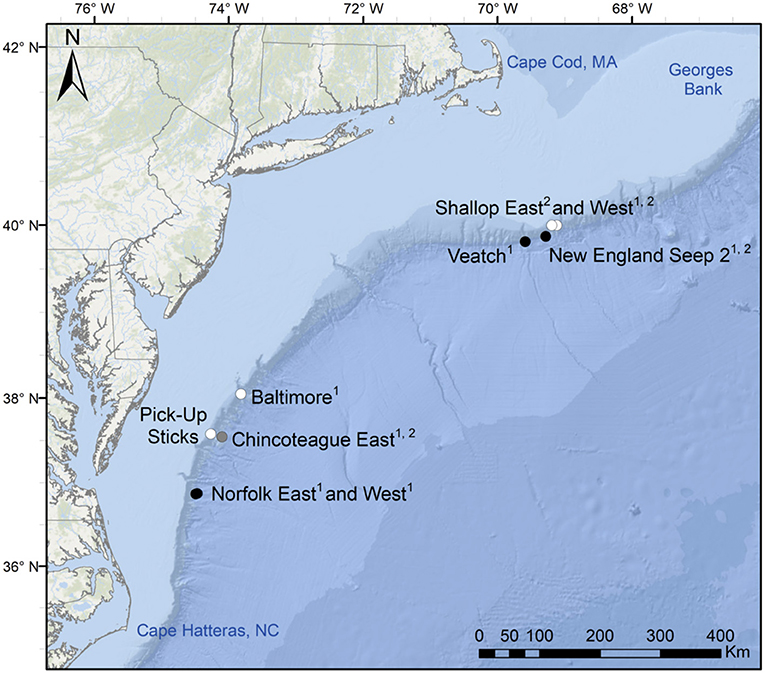
Figure 1. US Atlantic margin seep study sites. White symbols: shallow sites (~400 m), gray symbol: intermediate depth (~1,000 m), black symbols: deep sites (~1,500 m). Locations of samples for stable isotope analyses: 1bathymodiolin mussels, 2red crabs. Service layer credits: Esri, Garmin, GEBCO, NOAA NGDC, and other contributors.
Bathymodiolin Mussel Distribution and Trophic Ecology
Molecular Taxonomy of Bathymodiolin Mussels
To identify the Bathymodiolus species present at each site a 544 base-pair region of the mitochondrial cytochrome oxidase I gene was sequenced for 44 individuals. Sites include: Baltimore (n = 15), Shallop West (n = 3), Chincoteague East (n = 8), Norfolk East (n = 1), Norfolk West (n = 5), Veatch (n = 5), and New England Seep 2 (n = 7). Mitochondrial DNA was extracted from ethanol-fixed muscle tissue (foot) samples using a CTAB (cetyltrimethylammonium bromide) protocol (Möller et al., 1992) and amplified using the primers and PCR conditions in Olu-Le Roy et al. (2007). The resulting PCR products were purified (Meyer et al., 2016) and sequenced on a ABI 3730xl DNA analyzer (Applied Biosystems International). Sequences were compared to those within the GenBank database using BLASTn. Generated sequences were deposited in GenBank (Supplementary Table 1).
Stable Isotope Analysis of Bathymodiolin Mussels
To investigate the trophic ecology of bathymodiolin mussels, muscle tissue (foot) samples were collected from 44 individuals for stable isotope analysis. Sites include: Baltimore (n = 5), Shallop West (n = 6), Chincoteague East (n = 5), Norfolk East (n = 9), Norfolk West (n = 5), Veatch (n = 9), and New England Seep 2 (n = 5).
Samples were dried at 35–40°C overnight and stored in pre-combusted (500°C) glass vials following the protocol of Levin and Currin (2012). Samples were sealed, frozen in liquid nitrogen, and then ground to a homogenized powder. Samples were weighed, packaged in tin capsules, and shipped for nitrogen (δ15N) and carbon (δ13C) stable isotope analysis. Precision within runs (i.e., sample repeats) was within 0.27‰ ± 0.19 (mean ± standard deviation) for δ15N, and 0.28‰ ± 0.20 for δ13C.
To investigate differences in trophic ecology between shallow and deep seeps the relationship between δ13C and δ15N values with depth was tested using linear regression.
Community Structure Analysis
During dives, photo transects were conducted to characterize the megafaunal community. A photo transect was not conducted at Shallop East and only short transects were conducted at Norfolk East (n = 33 frames across two transects) and New England Seep 2 (n = 9 frames); therefore, these sites were not included in the community structure analysis. Sufficient coverage was obtained for six seeps (i.e., Pick-Up Sticks, Baltimore, Shallop West, Chincoteague East, Norfolk West, and Veatch) but at Pick-Up Sticks and Shallop West, extensive mussel beds were not found and only areas of active methane seepage (i.e., bubbling) were observed (Table 1).
Video records from each dive, in its entirety, were used to estimate mussel bed area, the abundance of red crabs outside of the photo transects, and to document red crab feeding and mating behavior. Screenshots (taken at a 10 s interval) from the Alvin Frame-Grabber System were analyzed for the number of red crabs and features of interest (e.g., methane hydrate, active bubbling, edges of mussel beds). Screenshots that contained live and/or dead mussels were extracted from the Frame-Grabber image analysis and their locations plotted in ArcGIS (version 10.5) alongside the HOV Alvin dive tracks; data were displayed using the WGS-1984 geographic coordinate system. The longest axis of the mussel bed and the perpendicular axis were measured in ArcGIS (using Geodesic measurements) to estimate mussel bed area as a rectangle; this was the most consistent method to estimate area and gives an idea of relative size, but should not be taken as true or used for comparisons outside of this study.
Photo Transects of Seep Communities
Megafaunal abundances, including both benthic invertebrates and demersal fishes, were estimated from photo transects of the seeps. At each seep, photo transects were conducted across the mussel bed or, in the case of Pick-Up Sticks and Shallop West, where mussels were absent, across the area of active methane seepage. For each transect, the camera (SubC Imaging 1Cam MK5) was perpendicular to the seabed and set to take pictures at intervals of 5–7 s. The speed and altitude of HOV Alvin remained relatively constant during a photo transect but ranged from 0.10 to 0.17 ms−1 and from 1.2 to 4.8 meters above bottom (mab) between transects. For each photo transect overlapping images were removed manually. Megafauna (>4 cm) present within the remaining frames were counted and identified to the lowest possible taxonomic level.
For photo transects at a mussel bed, percent coverage of live and dead mussels was calculated for each image to test whether faunal composition varies with substratum type. A 10 × 10 grid was placed over the image using Adobe® Photoshop® software (version 19.1.4) and the dominant substratum type (i.e., live mussels, dead mussels, sediment/rock) within each cell was identified. Only images with dense mussel coverage [i.e., ≥75% coverage of live, dead or mixed (live and dead) mussels] were used for the community structure analysis.
To calculate the total area surveyed per seep and for each habitat type (i.e., live, dead, and mixed mussel coverage), the area of each image within the photo transect was calculated according to Jones et al. (2009). In Equation 1, a is camera altitude in meters, the horizontal field of view (θ) is 54° and the vertical field of view (ω) is 34° (SubC Imaging, pers. comm.); we applied an altitude correction of 1.09 m to account for the altimeter of HOV Alvin being positioned higher than the camera. For the number of frames and area analyzed for each seep and habitat combination, see Supplementary Table 2.
Taxonomic Richness and Multivariate Analyses
To compare the faunal communities at each seep, taxonomic richness (i.e., the number of different species or morphotypes observed) was estimated using the iNEXT package (Hsieh et al., 2016) in R Core Team (2017). Asymptotic estimates were calculated by combining data for images with live, dead and mixed mussel coverage, with extrapolation limited to double the sample size (as recommended by Chao et al., 2014; Hsieh et al., 2016).
For the community structure analysis, multivariate analyses were performed using PRIMER v7 (Clarke and Gorley, 2015) with habitat type (i.e., live, dead, and mixed mussel coverage) treated as a factor. To reduce the effect of varying sample size between seeps, taxon-abundance data were standardized to the total number of individuals at each seep. To reduce the effect of numerically dominant species (e.g., red crabs Chaceon quinquedens, and the onuphid quill worm Hyalinoecia artifex; Table 2), taxon-abundance data were square-root transformed. A Bray-Curtis similarity index was calculated for each seep-habitat combination. The resulting similarity matrix was used to create a non-metric multidimensional scaling (MDS) ordination plot and to conduct a hierarchical cluster analysis with group-averaged linking. A similarity profile routine (SIMPROF test) was used to identify clustered data but we did not have the replication needed to conduct an ANOSIM test and identify significant differences among clusters. Similarity percentage (SIMPER) analysis determined which taxa contributed to the differentiation of clusters.
Community Variation With Environmental Variables
To determine whether seep communities differed with varying environmental conditions, we gathered environmental data thought to be important in structuring seep communities (Table 3). Latitude, depth, temperature, and salinity were obtained from the cruise data and HOV Alvin sensors, with temperature and salinity representing conditions at a single point in time for each site. POC flux to the seafloor, dissolved oxygen and pH were obtained from global seabed models for present-day environmental conditions (Sweetman et al., 2017), representing annual means at a coarse spatial resolution of 0.5 degrees. Distance from shore was calculated using the near tool in ArcGIS; data were displayed using the North American 1927 geographic coordinate system, the distance between seeps and the United States coastline was calculated using Geodesic measurements. For the multivariate analysis we included only latitude, depth, POC flux, and distance from shore. We eliminated temperature due to its co-correlation with depth (Pearson's correlation coefficient: −0.94). We eliminated pH, dissolved O2 and salinity due to their lack of variability across seeps.
To identify the subset of environmental variables that optimizes agreement between community structure and environmental data, we used the BEST selection procedure (Clarke and Gorley, 2015). The environmental data were normalized and a similarity matrix constructed using Euclidean distance in PRIMER v7; no transformations were applied because draftsman plots created in PRIMER did not indicate skewness within the environmental data. Because we have a limited number of environmental variables (n = 4), we used the BIOENV procedure to search all possible combinations and identify the subset of environmental variables that best matched taxon-abundance patterns. The degree to which patterns in both the environmental data and taxon-abundance data matched was measured using Spearman's rank correlation. To calculate the statistical significance of the best matching combination of environmental variables, the global BEST test was performed with 999 permutations.
Red Crab Autecology
Stable Isotope Analysis of Red Crabs
To investigate the trophic ecology of red crabs, muscle tissue (chela) samples were collected for stable isotope analysis. Red crab samples from Chincoteague East were collected during the 2015 R/V Atlantis SeepC cruise (AT29-04, Chief Scientist Cindy L Van Dover). Red crab samples from seeps off New England (i.e., New England Seep 2, Shallop East and West) were collected during the 2016 R/V Atlantis EAGER cruise (AT36-01, Chief Scientist Adam Skarke).
Red crab samples were processed following the same methodology as outlined in the stable isotope analysis of bathymodiolin mussels.
The proportion of methane-derived carbon within the diet of red crab was calculated using a two-source mixing model (Equation 2):
where fseep is the proportion of diet originating from methane-derived carbon, is the isotopic signature of the bathymodiolin mussels, is the isotopic signature of photosynthetic material, and is the isotopic signature of the individual red crab tissue sample. For this analysis, was −20 ± 2‰ according to values recorded for organic matter in surface sediments within the mid-Atlantic bight between 50 and 2,250 m (Walsh et al., 1985) and values south of Cape Cod between 200 and 2,000 m (Hunt, 1970). was taken to be the δ13C value of the mussel tissue analyzed at each seep. Due to a lack of mussels at Shallop East, we used the δ13C value of the mussel tissue analyzed at Shallop West to assess the diet of red crab sampled at both Shallop East and West.
Sampling for Crab Larvae
To test for the presence of red crab larvae above US Atlantic margin seeps, larval samples were collected 5–10 mab using the Sentry Precision Impeller Driven (SyPRID) Sampler (Billings et al., 2017) on the AUV Sentry. Samples were rinsed into collecting bins with filtered seawater, stored at 4°C (<12 h) and sorted under dissection microscopes. Crab larvae were counted and a representative larva was photographed.
Molecular Taxonomy of Crab Larvae
To determine whether crab larvae collected above seeps belonged to Chaceon quinquedens, a 658 base-pair region of the mitochondrial cytochrome oxidase I gene was sequenced from 4 individuals. Mitochondrial DNA was extracted from ethanol-fixed larvae using Instagene Matrix (Biorad) following manufacturer's protocol, and amplified using primers jgLCO1490 (Geller et al., 2013) and HCO2198 (Folmer et al., 1994). Amplification was carried out with the following parameters: incubation at 95°C for 2 min, followed by 35 cycles of 95°C for 40 s, 45°C for 40 s and 72°C for 1 min, followed by incubation at 72°C for 2 min. PCR was conducted in 20 μL volume of nuclease-free water containing 0.2 μL of DNA extract, 500 nM of each primer, 1U of Go Taq Polymerase (Promega), 1X GoTaq Buffer, and 200 μM dNTPs (NE Biolabs). PCR products containing a single bright band of the expected size were purified using SV Wizard Gel and PCR clean up Kit (Promega), quantified using gel electrophoresis with Low Mass Ladder (NE Biolabs) and sequenced in both directions using the original PCR primers by Sequetech, Inc. (Moutain View, CA). Sequences were trimmed, assembled into contigs and consensus sequences created for each sample (trimming any bases with a cumulative Phred quality score of <20) using Geneious v. 11.0.4 (Biomatters, Ltd.). Sequences were compared to those within the BOLD database. Sites include: Norfolk East (Larva No. 74), Chincoteague East (Larvae No. 147 and 148) and Veatch (Larva No. 443). Generated sequences were deposited in GenBank (accession numbers: MN265823, MN265821, MN265820, MN265822).
Results
Bathymodiolin Mussel Distribution and Trophic Ecology
All mussels sampled for molecular analysis were identified as Bathymodiolus childressi, sites included: Baltimore, Shallop West, Chincoteague East, Norfolk East, Norfolk West, Veatch, and New England Seep 2. Bathymodiolus heckerae may also occur at Norfolk West, where numerous shells and a few live individuals were observed alongside B. childressi (Figures 2A,H), but samples of B. heckerae were not collected for molecular analysis.
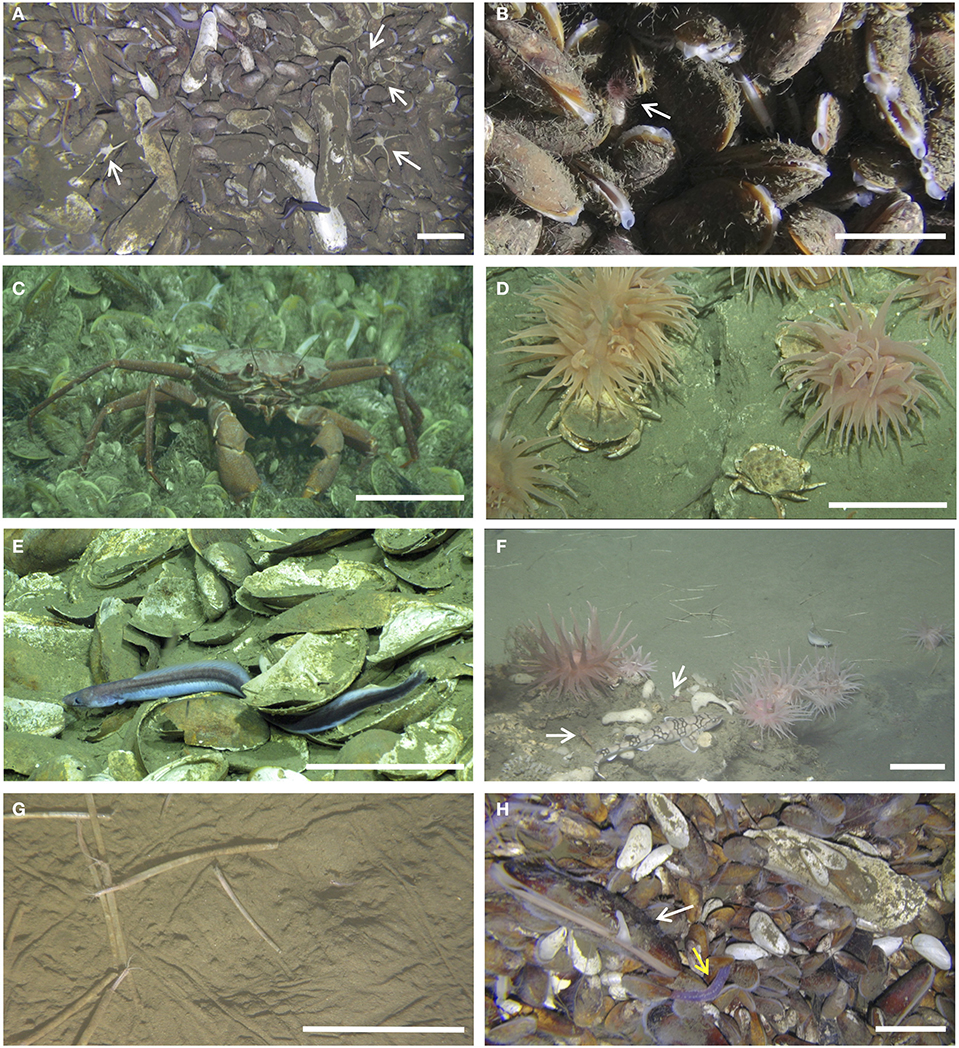
Figure 2. Fauna important in distinguishing seep communities. (A) Ophiuroids (arrows) at Norfolk West, along with dead mussel shells cf. Bathymodiolus heckerae (Dive 4803). (B) Small, red anemone Actiniaria sp. 4 (arrow) at Norfolk East (Dive 4802). (C) Red crab (Chaceon quinquedens) at Norfolk East (Dive 4802). (D) Jonah crab (Cancer borealis) and the anemone Urticina sp. at Shallop West (Dive 4812). (E) A cutthroat eel (Dysommina rugosa) at Baltimore (image courtesy of S W Ross; Ross et al., 2015). (F) The anemone Urticina sp. at Pick-Up Sticks, along with sponges, a chain catshark (Scyliorhinus retifer), caridean shrimp (arrows) and, on the background sediment, onuphid quill worms (Hyalinoecia artifex) and a short-beard codling (Laemonema barbatulum; Dive 4805). (G) H. artifex at Pick-Up Sticks (Dive 4805). (H) A live mussel cf. B. heckerae (white arrow) and the purple holothurian Chiridota heheva (yellow arrow) at Norfolk West (Dive 4803). Scale bars: ~10 cm.
In terms of trophic ecology, there was no relationship between depth and the δ13C or δ15N values of mussel tissue (Figures 3B,C) but there were site-specific isotope signatures. Across seep sites δ15N values ranged from −0.6‰ at Baltimore to 2.2‰ at Shallop West and δ13C values ranged from −60‰ at New England Seep 2 to −70‰ at Chincoteague East (Table 4, Figure 3A). In addition, δ13C values differed among mussels sampled at Shallop West, ranging from −56.2‰ to −69.6‰ within the seep (Figure 3A).
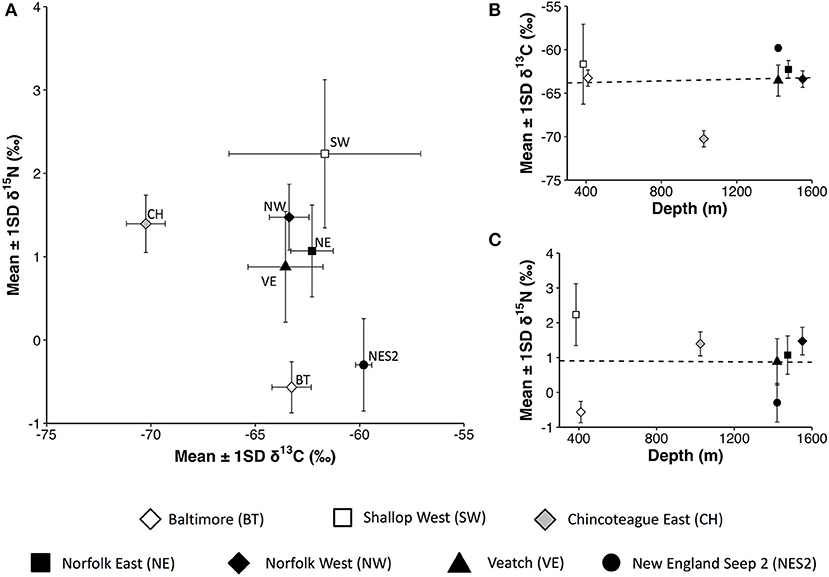
Figure 3. Bathymodiolus childressi stable isotope biplot and relationships with depth. (A) δ15N vs. δ13C (‰). (B) δ13C (‰) vs. Depth (m). (C) δ15N (‰) vs. Depth (m). White symbols: shallow sites (~400 m), gray symbol: intermediate depth (~1,000 m); black symbols: deep sites (~1,500 m). Dashed lines: linear regression for δ13C [F(1, 5) = 0.026, p = 0.88, r2 = 0.005, slope = 4.6 × 10−4] and δ15N [F(1, 5) = 0.0013, p = 0.97, r2 = 2.3 × 10−4, slope = −3.2 × 10−5].
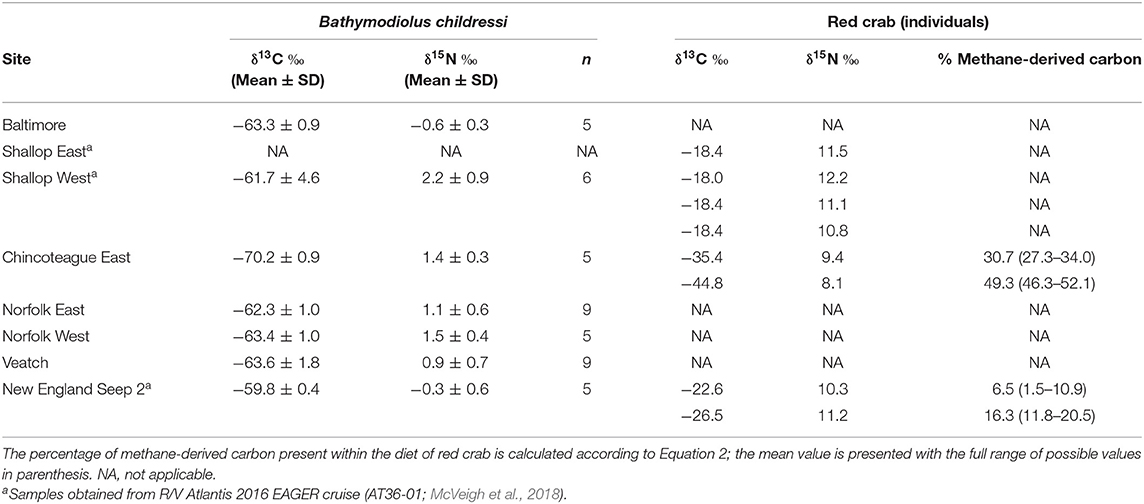
Table 4. Stable isotope (δ13C and δ15N) data for samples of bathymodiolin mussel (Bathymodiolus childressi) and red crab (Chaceon quinquedens).
Community Structure Analysis
From 1,873 individuals, a total of 35 taxa were identified within the photo transects (Table 2). The total number of taxa observed at each seep ranged from six taxa at Veatch to 16 taxa at Baltimore (Table 2). Demersal fish formed the most diverse taxonomic group (n = 14 taxa), with seven taxa observed only at shallow (~400 m) seeps (Table 2). Thirty-one of the 35 taxa identified were only observed within a single depth category: 19 taxa were only observed at shallow seeps, four at Chincoteague East (~1,000 m), and eight at deep (~1,500 m) seeps. Only a single species within the photo transects, a small orange anemone (Actiniaria sp. 3; Supplementary Figure 1B), was observed at both shallow and deep seeps (Table 2).
Taxonomic Richness
For seeps where taxonomic richness could be estimated from photo transects, two of the shallow seeps (Baltimore, Pick-Up Sticks) had approximately twice the taxonomic richness of the intermediate-depth seep (Chincoteague East) and one of the deep seeps (Norfolk West; Figure 4). Insufficient sampling precluded the estimation of taxonomic richness at both Veatch and Shallop West, illustrated by the lack of a horizontal asymptote within Figure 4.
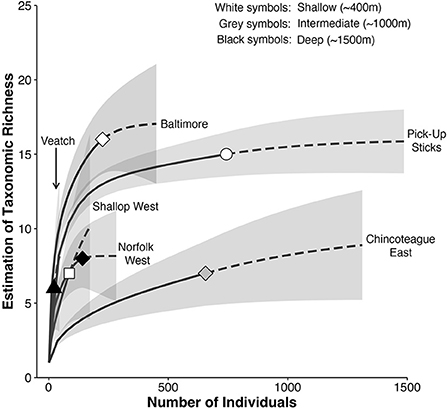
Figure 4. Taxonomic richness (rarefaction and extrapolation) estimates from photo transect data. Solid lines: interpolated results, dotted lines: extrapolated results, each with 95% confidence intervals. Symbols: observed values.
Multivariate Analyses
Seep community structure (based on taxon-abundance data from photo transects) clustered by depth (Figure 5). The deep seep communities (Norfolk West and Veatch) formed a distinct cluster with <1% similarity to the other seep communities. Community structure at Chincoteague East, the intermediate depth seep, was <1% similar to the deep seeps and ~12% similar to the shallow seep communities (Baltimore, Pick-Up Sticks and Shallop West). The three shallow seeps had ~34% similarity and comprised of two clusters; the two non-mussel-dominated seep communities (i.e., Pick-Up Sticks and Shallop West) had ~40% similarity and differed from the mussel bed community (i.e., Baltimore) by 66%.
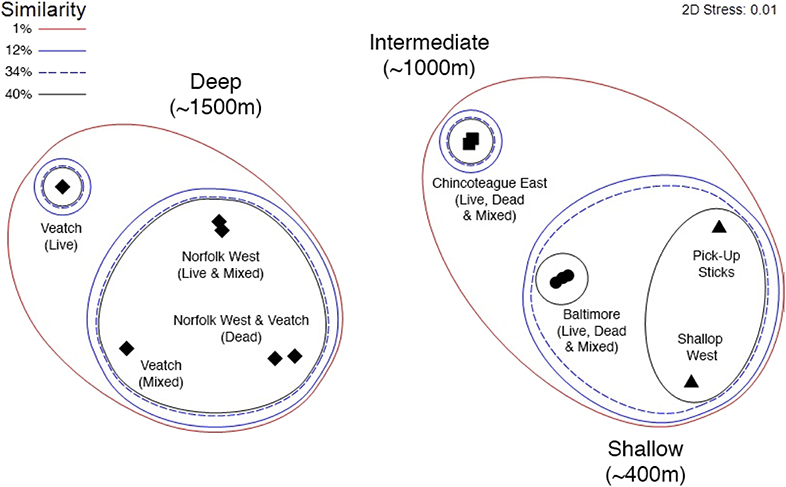
Figure 5. Non-metric multidimensional scaling (MDS) ordination from photo transect data. Symbols represent the four clusters identified by the similarity profile routine (SIMPROF test): (i) Norfolk West and Veatch (diamonds), (ii) Chincoteague East (squares), (iii) Baltimore (circles), (iv) Pick-Up Sticks and Shallop West (triangles). Parentheses: habitat type [i.e., live, dead, and mixed (live and dead) mussel coverage].
The overall clustering of seep communities was driven by the distribution of six taxa (Figure 2): ophiuroids, the small red anemone Actiniaria sp. 4, red crabs (Chaceon quinquedens), Jonah crabs (Cancer borealis), cutthroat eels (Dysommina rugosa), and the large colorful anemone Urticina sp. Norfolk West and Veatch (deep seeps) were allied due to the abundance of ophiuroids (56% contribution to similarity) and the small red anemone Actiniaria sp. 4 (22% contribution to similarity). These taxa were not observed at the intermediate and shallow seeps. Chincoteague East (intermediate depth) was distinctive in its abundance of red crabs (n = 612; Table 2). Baltimore (shallow) was characterized by three abundant taxa: red crabs, Jonah crabs, and cutthroat eels (D. rugosa; Table 2). Pick-Up Sticks and Shallop West (shallow seeps) were allied due to the high abundance of the anemone Urticina sp. (45% contribution to similarity) but shared a low level of similarity overall (~40%). The separation between Pick-Up Sticks and Shallop West was likely related to the abundance of sponges (n = 119) and onuphid quill worms (Hyalinoecia artifex; n = 326) at Pick-Up Sticks, and their absence at Shallop West (Table 2, Figure 2).
Megafaunal community structure at Baltimore (shallow) and Chincoteague East (intermediate depth) was similar regardless of the proportion of live and dead mussel coverage (i.e., substratum type). There were differences in the community associated with different substrata at Norfolk West and Veatch (deep seeps; Figure 5). At Norfolk West and Veatch, taxa observed on dead mussel patches had ~80% similarity but differed from taxa at mixed (live and dead) or live mussel patches within the same mussel bed (Figure 5). The similarity between dead mussel patches at Norfolk West and Veatch was attributed to the presence of ophiuroids and the small red anemone Actiniaria sp. 4. These were the only two taxa shared across dead mussel patches at the two seeps (Table 2). The community observed on live mussel patches at Veatch was distinct, with only ~10% similarity to the community observed on mixed (live and dead) or dead mussel patches. This result may reflect insufficient sampling, with only a single urchin (Echinoidea sp. 1) being observed on the live mussel patches at Veatch (Table 2).
Variation in Community Structure With Environmental Variables
Within the multivariate analysis, there was a significant correlation (Rho = 0.78, p < 0.001) between the taxon abundance data and the environmental variables of latitude, depth, POC flux, and distance from shore. Depth explained the greatest amount of variation (72%), followed by POC flux (58%), distance from shore (42%) and latitude (30%). Depth and POC flux together explained the greatest amount of variation (78%) for any combination of variables.
Red Crab Autecology
Within the video records from each dive, red crabs were observed at shallow, intermediate, and deep seeps, with especially dense aggregations observed at Chincoteague East (intermediate depth; Table 5, Figure 6A). Within the photo transects, red crabs were only observed at two seeps: Baltimore (shallow; ~0.5 individuals 10 m−2) and Chincoteague East (~15 individuals per 10 m−2). At Chincoteague East most individuals (~76%) were observed in areas of dense live-mussel coverage (Table 2).
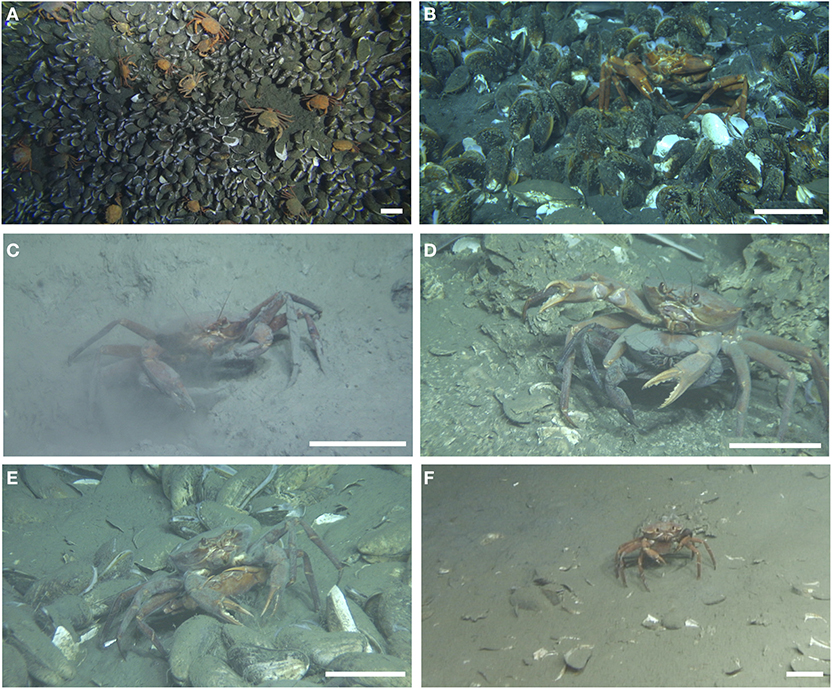
Figure 6. Red crabs, Chaceon quinquedens. (A) Dense aggregations at Chincoteague East (Dive 4804). (B) Feeding on a bathymodiolin mussel at Baltimore (Dive 4808). (C) Sifting through sediment in an area of active methane bubbling at New England Seep 2 (Dive 4815). (D) Mating pair in precopulatory embrace at Chincoteague East (Dive 4806). (E) Mating pair in copulatory embrace at Chincoteague East (Dive 4806). (F) Ovigerous female at Chincoteague East (Dive 4806). Scale bars: ~10 cm.
Red crabs were observed feeding in areas of active methane bubbling. One individual was observed feeding on a bathymodiolin mussel at Baltimore seep (shallow; Figure 6B), seven individuals were observed sifting through sediment in an area of active methane bubbling at New England Seep 2 (deep; Figure 6C, Supplementary Video 1).
The proportion of methane-derived carbon within the diet of red crabs varied among individuals and seeps (Table 4). For red crabs collected from Shallop East and West, the proportion of methane-derived nutrition could not be calculated using the mixing model because the red crab δ13C values exceed the end-member used for photosynthetically-derived nutrition (−20 ± 2‰). The higher δ13C values of the red crab tissue suggest that methane-derived carbon does not feature in the diet of red crabs collected from Shallop East and West (shallow seeps). Two red crabs collected from New England Seep 2 (deep) were estimated to derive ~7 and 16% of their diet from methane-derived food resources; two individuals from Chincoteague East (intermediate depth) derived as much as ~31 and 49% of their carbon from methane-derived food resources (Table 4).
Fifteen mating pairs of red crab were observed at the intermediate depth site Chincoteague East. Eight pairs were observed in a precopulatory embrace, where the male forms a protective cage around the female as she molts (Figure 6D). Seven pairs were observed in a copulatory embrace, where the female is inverted under the male (Figure 6E). No mating pairs were observed at any other seep, in any of the HOV Alvin data sets (i.e., photo transects and video records). Three ovigerous females were also observed at Chincoteague East; their abdomens were flexed open, suggesting they were ventilating their egg masses (Figure 6F).
Decapod larvae (zoea) accounted for ~70% of the total number of invertebrate larvae collected above Chincoteague East (Figure 7A), but accounted for <3% of individuals at Veatch and Norfolk East and were absent from samples above Baltimore and New England Seep 2. The zoea larvae from Chincoteague East were inferred to belong to that of red crab based on morphological comparisons (Figures 7B,C). Four zoea larvae (two from Chincoteague East, one from Norfolk East, one from Veatch) were confirmed to belong to Chaceon quinquedens by DNA-barcoding; all four CO1 sequences were identical to each other and the top match in the BOLD database was a sequence of C. quinquedens from Nova Scotia, Canada (99.69% identical using K2P distance calculation).
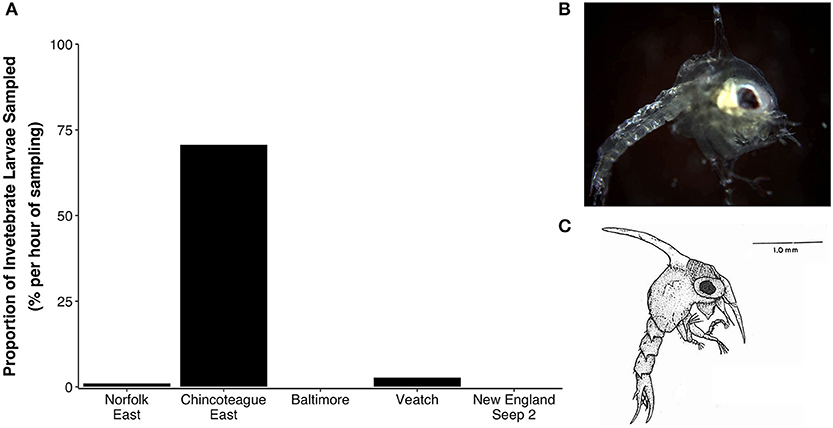
Figure 7. Zoea larvae. (A) Zoea larvae sampled per hour at 5–10 m above bottom (mab), presented as a percentage of all invertebrate larval forms. (B) Image of a zoea larva (one of 37 individuals) collected 5 mab at Chincoteague East. (C) Illustration of a first stage red crab (Chaceon quinquedens) zoea larva from Perkins (1972).
Discussion
Biogeography of Symbiont-Bearing Mega Fauna
This study extends the range of seeps where Bathymodiolus childressi dominates from Norfolk, Chincoteague and Baltimore seeps (Coykendall et al., 2019) to seeps off New England (i.e., Veatch, Shallop West, and New England Seep 2). In terms of the dominant mussel species present, the seeps north of Cape Hatteras are more closely aligned to seeps within the Gulf of Mexico (e.g., Faure et al., 2015) and off Trinidad and Tobago (Amon et al., 2017) than to seeps at the Blake Ridge diapir and West Florida Escarpment, which are dominated by B. heckerae (Gustafson et al., 1998; Van Dover et al., 2003). Bathymodiolus heckerae was observed at Norfolk West, where recent genetic analysis has confirmed their presence (Coykendall et al., 2019). The closest known population of B. heckerae is at the Blake Ridge diapir (~2,200 m; Van Dover et al., 2003), which is geographically closer than B. childressi populations within the Gulf of Mexico and the Caribbean Sea.
There are at least three non-exclusive explanations for the abundance of Bathymodiolus childressi at seeps north of Cape Hatteras and their absence at the Blake Ridge diapir. Firstly, the chemical environment (e.g., methane flux, as well as methane and sulfide concentrations) within the study area may favor B. childressi, which then outcompetes B. heckerae for space and resources. Bathymodiolus childressi is thought to rely predominantly on methanotrophic endosymbionts for nutrition but can also possess thiotrophic epibionts (Assié et al., 2016; Coykendall et al., 2019). Bathymodiolus heckerae is known to possess four co-occurring endosymbionts: one methanotrophic, one methylotrophic, and two thiotrophic (Duperron et al., 2007). The different compositions of symbiotic bacteria may cause the two mussel species to have different sulfide tolerances, with B. childressi being better adapted to lower sulfide concentrations (Cordes et al., 2010). Differences in sulfide physiology may subsequently impact growth rates and reproductive output (Barry and Kochevar, 1998), causing B. childressi to outcompete B. heckerae wherever the chemical environment is more favorable. At the Blake Ridge diapir seep, porewater sulfide concentrations were found to be high (up to 12 mmol l−1) wherever beds of dead vesicomyid clams were observed, suggesting sulfide toxicity is important in determining clam bed distributions (Heyl et al., 2007); high sulfide concentrations may be toxic for B. childressi, preventing colonization at Blake Ridge diapir and resulting in monospecific mussel beds of B. heckerae.
Secondly, differences in larval behavior and duration may enable Bathymodiolus childressi larvae to consistently colonize seeps north of Cape Hatteras, whilst colonization of B. heckerae is determined by more episodic events. Bathymodiolus childressi larvae are long-lived (with an estimated larval duration of more than a year; Young et al., 2012) and have been collected from near-surface waters (i.e., in the top 100 m) where faster currents may facilitate dispersal (Arellano et al., 2014). Although it has yet to be demonstrated that larvae in surface waters recruit successfully to seabed habitats, B. childressi populations in the Gulf of Mexico may be connected to populations north of Cape Hatteras via the Gulf Stream (Cordes et al., 2007; Young et al., 2012). Bathymodiolus heckerae connectivity may rely on meanders in the Gulf Stream (Zeng and He, 2016) to transport larvae from the Blake Ridge diapir to seeps north of Cape Hatteras. In the absence of large Gulf Stream meanders, if B. heckerae larvae remain in deeper waters, larvae may be transported south along the deep western boundary current and away from the study area (Cutler, 1975; Bower and Hunt, 2000; Cordes et al., 2007).
Thirdly, as discussed by Coykendall et al. (2019), Norfolk seeps may lie within a depth-related transition zone between shallower Bathymodiolus childressi mussel beds, and deeper B. heckerae mussel beds. Within the Gulf of Mexico, B. childressi occupies shallower depths (1,000–2,300 m; Cordes et al., 2010) and B. heckerae occupies deeper depths (2,200–3,300 m; Cordes et al., 2007, 2010). The discovery of B. childressi at Baltimore seep extends the upper depth margin to 400 m, resulting in a distribution of 400–3,300 m for both species. Norfolk West (1,500 m) is in the middle of this depth distribution, offering a potential explanation for the co-occurrence of B. childressi and B. heckerae at Norfolk seeps.
Bathymodiolin mussels were the only symbiont-bearing megafauna observed during this study and there is an apparent lack of vesicomyid clams at US Atlantic margin seeps north of Cape Hatteras (Quattrini et al., 2015; McVeigh et al., 2018; this study). Vesicomyid clams are known to occur at seeps to the north and south of the study area; for example, Vesicomya cf. venusta is known from the Blake Ridge and Cape Fear diapirs (Van Dover et al., 2003; Brothers et al., 2013) and dense communities of vesicomyid clams are known from the Laurentian Fan seep communities (~3,800 m; Mayer et al., 1988). It is possible that hydrographic barriers prevent sufficient larval supply to the study area. If vesicomyid larvae remain in deeper waters, the deep western boundary current (Cutler, 1975; Bower and Hunt, 2000; Cordes et al., 2007) may prevent sufficient larval supply from south of Cape Hatteras, and the complex vertical structure of North Atlantic Deep Water (which consists of three different water masses: Upper Labrador Sea Water at 700–1,500 m, Labrador Sea Water at 1,500–2,000 m, and the Iceland-Scotland and Denmark Strait Overflow Water at 2,500–4,000m; Bower and Hunt, 2000) may prevent sufficient larval supply from north of Georges Bank. Alternatively, it is possible that populations of vesicoymid clams are present but have not yet been discovered on the US Atlantic margin. Previous studies had also suggested that there was a lack of siboglinid polychaetes at seeps north of Cape Hatteras (Quattrini et al., 2015; McVeigh et al., 2018; this study); however, in April 2019 the DEEP SEARCH (DEEP Sea Exploration to Advance Research on Coral/Canyon/Cold seep Habitats) program observed tubeworms at seeps off North Carolina (~125 km south of Norfolk East and West; NOAA, 2019) showing that new discoveries continue to be made at seeps along the US Atlantic margin.
Nutrition of Bathymodiolus childressi
In addition to harboring methanotrophic endosymbionts and thiotrophic epibionts (Assié et al., 2016; Coykendall et al., 2019), Bathymodiolus childressi is capable of filter feeding (Page et al., 1990; Pile and Young, 1999) and has the potential to also use photosynthetically-derived food resources (Tyler et al., 2007). In this study, the lack of relationship between depth and carbon isotope values suggests that B. childressi may rely predominantly, if not entirely, on methane-derived carbon for nutrition at both shallow and deep seeps. A dependence on methane-derived nutrition is supported by nitrogen isotope values (−0.6‰−2.2‰) lower than those in animals reliant on photosynthetically-derived food resources (>5‰; Demopoulos et al., 2017), and is consistent with previous morphological observations (i.e., reduced digestive tracts; Gustafson et al., 1998) and carbon isotope values within the Gulf of Mexico, which ranged from −40 to −70‰ (Childress et al., 1986; Brooks et al., 1987; Dattagupta et al., 2004). A dependence on chemosynthetically-derived nutrition has also been documented for mussels sampled at Baltimore (400 m) and Norfolk (1,500 m) seeps by Demopoulos et al. (2019). Methanotrophs were the main dietary contributor with thiotrophic microbes contributing between 0.1 and 16% of nutrition (Demopoulos et al., 2019). Stable isotope mixing models suggest that mixotrophy does exist but the importance of phytodetritus may depend on resource (methane) availability, seasonal variations in POC flux and (or) ontogenetic shifts in resource use (Demopoulos et al., 2019). Our results represent the trophic ecology of B. childressi at one point in time; before the importance of photosynthetically-derived food resources can be discounted, additional sampling is needed throughout the year to investigate the importance of seasonality and whether photosynthetically-derived nutrition becomes a more important dietary component during periods of high POC flux.
Taxonomic Richness and Seep Community Structure
The seep community was typically dominated by mussel (Bathymodiolus childressi) beds, but the associated megafauna varied between shallow (~400 m) and deep (~1,500 m) seeps. At the shallow Baltimore seep, mussels typically occurred with non-seep-endemic decapod crabs (e.g., Chaceon quinquedens and Cancer borealis) and fish (e.g., Dysommina rugosa). At the deep seeps (Norfolk West and Veatch), mussels typically occurred with ophiuroids and the small, red anemone Actiniaria sp. 4. Only one species, a small orange anemone, was shared between shallow and deep seeps within the photo transects (Actiniaria sp. 3; Supplementary Figure 1B). Other non-seep-endemic taxa, especially the large anemone Urticina sp., were found to take advantage of the hard substrate habitats (authigenic carbonates) associated with methane seepage at Pick-Up Sticks and Shallop West.
Taxonomic richness estimates suggest a greater diversity at the shallow seeps of Baltimore and Pick-Up Sticks, compared to the deep seeps of Norfolk West and Veatch. Although our results suggest some shallow seeps have greater diversity (richness) than some deep seeps, insufficient sampling at Veatch and the inclusion of only one shallow seep with a mussel bed (Baltimore) means we do not have enough evidence to draw conclusions on depth-related patterns in diversity. In fact, throughout the Atlantic Equatorial Belt, species richness is often highest at seeps of intermediate depth between 1,000 and 2,000 m, where species from both shallow and deep communities overlap (Olu et al., 2010). Chincoteague East included a combination of taxa observed at shallow (i.e., red crab) and deep (i.e., Alvinocaris sp., Coryphaenoides rupestris) seeps but was dominated by red crabs. This study is limited by the inclusion of only one intermediate-depth site and to test whether seep-associated megafauna conform to a mid-slope diversity maximum would require additional sampling at a more continuous range of depths.
The little-to-no overlap in megafauna between shallow (~400 m) and deep (~1,500 m) seeps suggests that a depth-related faunal boundary may exist for US Atlantic margin seep communities. Similar faunal differentiation with depth has been observed at seeps across the Gulf of Mexico continental slope (Cordes et al., 2007), with a transition between shallow and deep communities occurring at ~1,000 m (Cordes et al., 2010). For example, first-level consumers at shallow seeps (~600 m) are dominated by gastropods (Bathynerita naticoidea and Provanna sculpta) and bresilid shrimp (Alvinocaris stactophila; Cordes et al., 2007), whereas deeper communities (>1,200 m) are characterized by an increasing abundance of ophiuroids (Ophioctenella acies; Cordes et al., 2010). Gulf of Mexico seeps also demonstrate depth-related differences in foundation species, with Bathymodiolus childressi occupying shallower depths and B. heckerae deeper depths (Cordes et al., 2007, 2010). Differences in the mussel species present is thought to reflect differences in habitat chemistry, which could also result in community differences between shallow (B. childressi) and deep (B. heckerae) mussel beds in the Gulf of Mexico (Cordes et al., 2010). In this study, B. childressi dominated every mussel bed; therefore, the differences in community structure between shallow (i.e., Baltimore) and deep (i.e., Norfolk West and Veatch) mussel beds suggest that depth related differences can exist for seep-associated megafauna, regardless of the underlying foundation species.
Depth zonation on continental margins has been attributed to a suite of co-varying factors, including temperature and food availability (Carney, 2005; Olu et al., 2010; Wei et al., 2010). Of the environmental variables included in this study, depth and the co-varying factor temperature were important drivers of seep-community structure, explaining ~72% of the variation observed. Food availability (POC flux) explained very little variation, contributing an additional 6% to the variation explained by depth and temperature. For taxa that rely predominantly on chemosynthetically-derived nutrition, food availability would not be expected to influence community structure (Olu et al., 2010). However, for non-seep endemic taxa, seep colonists and vagrant species would be expected to follow depth-zonation patterns driven by food availability (Olu et al., 2010). In this study, the small amount of additional variation explained by POC flux may be caused by the large temperature difference observed between shallow (average temperature of 9.0°C) and deep (average temperature of ~4.2°C) seeps. Temperature may only play an important role in deep-sea diversity patterns at relatively high (>10°C) and low (<5°C) levels, whereas POC flux may play a more prominent role in structuring deep-sea communities at more intermediate temperatures (Yasuhara and Danovaro, 2016). Alternatively, the importance of POC flux as an explanatory variable may have been masked by the use of low-resolution global models, which do not reflect temporal variation or small-scale spatial variation in food availability.
Factors contributing to depth-related zonation on the US Atlantic Margin are further complicated by taxon-specific habitat preferences that varied with depth. In this study, substratum type (i.e., the proportion of live vs. dead mussel coverage) influenced taxon-abundance patterns at deep seeps (Norfolk West and Veatch), with ophiuroids and the small, red anemone Actiniaria sp. 4 being more abundant in the presence of dead mussels. At shallow seeps, substratum type was not found to influence community structure. The increased importance of substratum type with depth is in contrast to the pattern observed for demersal fish at Baltimore and Norfolk seeps, which showed a reduced habitat specificity as depth increased (Ross et al., 2015). The contrasting results between this study and that of Ross et al. (2015) suggest that taxon-specific habitat preferences may vary with depth.
Overall, depth and factors that co-vary with depth were found to be more significant variables in structuring megafaunal seep communities than geographic distance between seeps. Within the multivariate analysis, seeps from opposite ends of the study area grouped together by depth category (Figure 5); Norfolk West and Veatch grouped together as deep seeps, Pick-Up Sticks and Shallop West grouped together as shallow non-mussel-dominated seeps (Figure 5). The similarity of taxon-abundance patterns within a depth category mirrors results across the Atlantic Equatorial Belt, where seeps in the eastern Atlantic shared ~30% of megafauna with seeps from similar depths in the western Atlantic, despite a geographic distance of more than 7,000 km (Olu et al., 2010).
Red Crab Autecology
Of the megafauna observed, one of the most conspicuous was red crab (Chaceon quinquedens). Observations from Baltimore and New England Seep 2, where red crabs were observed feeding on a crushed mussel shell and sifting through sediment in an area of active methane bubbling (Figures 6B,C), suggest that red crabs may use at least two potential sources of chemosynthetically-derived nutrition—i.e., bathymodiolin mussel tissue and free-living chemoautotrophic bacteria. The proportion of methane-derived carbon within an individual red crabs diet ranged from ~0% at Shallop East and West to ~50% for an individual sampled at Chincoteague East (Table 4). These results mirror those for other vagrant predators and scavengers caught at seeps within the Gulf of Mexico, where chemosynthetic productivity contributed between 0 and 50% of nutrition for spider crabs (Rochina crassa), giant isopods (Bathynomus giganteus) and hagfish (Eptatretus sp.; MacAvoy et al., 2002).
Recently, a comparison of stable isotope methodologies has suggested bulk stable isotope analysis (as used here) may underestimate the role of chemosynthetically-derived nutrition in seep-associated fauna (Seabrook et al., 2019). At methane seeps off British Columbia (Canada), seep-associated bacteria were identified as dietary sources for tanner crabs (Chionoecetes tanneri) based on fatty acid analysis and molecular analysis of stomach contents, while carbon isotope values of the leg muscle suggested the species relied solely on photosynthetically-derived carbon (Seabrook et al., 2019). Differing results between compound specific fatty acid analysis and bulk stable isotope analysis may be explained by the amount of time needed for muscle tissue to reflect the isotopic composition of the food ingested (Seabrook et al., 2019). Fatty acids have a turnover time of a few days to a few weeks (Ruess and Chamberlain, 2010), while muscle tissue provides an integrated signal of the individual's diet over a period of months (Lorrain et al., 2002; Freire et al., 2009). Consequently, the values presented in this study are likely to underestimate the contribution of chemosynthetic productivity to the diet of red crab. For the two crabs sampled at Chincoteague East, bulk stable isotope analysis attributed ~30 and ~50% of nutrition to methane-derived food resources, suggesting that the individuals spent prolonged periods of time at the seep and that Chincoteague seep communities may serve as an important food resource for red crabs. This study is limited by sample size (i.e., eight crabs across four seeps), which prevents a clear understanding of how the utilization of methane-derived food resources varies within and across seeps; however, the combination of observational data and stable isotope results show that seeps can provide trophic support to red crab populations.
Prior to this study, two papers discuss trophic interaction between commercial species and seep ecosystems (see Supplementary Table 3 for literature review). At a seep off Svalbard, a single snow crab (Chionoecetes opilio) was observed grazing within a bacterial mat (Sen et al., 2018). At seeps off the coast of British Columbia (Canada), tanner crabs (Chionoecetes tanneri) have been observed sifting through areas of active methane bubbling and feeding on bacterial mats, with chemosynthetically-derived carbon providing some trophic support (Seabrook et al., 2019). Within the broader literature, commercially important species are known to occur in high abundance at seeps around the world. Atlantic cod and northern shrimp are abundant at seeps off Svalbard (Sen et al., 2018); Norway lobster, rose shrimp and European hake are targeted by commercial trawlers near mud volcanoes in the Gulf of Cádiz (Rueda et al., 2012; Sitjà et al., 2019); Patagonian toothfish is abundant at the Concepción methane seep area in Chile (Sellanes et al., 2008, 2012); rockfishes are present at seeps off the coast of Alaska (Jones et al., 2012) and in Grays Canyon off Washington State, which is also an important fishing ground for the spot-prawn industry (Powell et al., 2018); sablefish, Pacific dover sole, and thornyheads are abundant at seeps off the coast of California (Grupe et al., 2015; Levin et al., 2016b), with the Del Mar seep supporting densities of longspine thornyheads (Sebastolobus altivelis) twice that of the surrounding seafloor (Grupe et al., 2015). Despite the known occurrence of commercially important species at seeps around the world, there is a general lack of information on the interactions between commercial species and seep ecosystems.
Two previous studies discuss a possible connection between seeps and reproduction of commercially important species. Berndt (2005) hypothesized that seeps on the continental margins of Europe may represent breeding grounds for some fish populations. De Juan and Lleonart (2010) noted that the Gulf of Lyon (Mediterranean Sea) includes numerous submarine canyons with seeps and hypothesized that these sites might serve as a refuge for European hake, monkfish, and shrimp that spawn in the area. In this study, red crab reproductive behaviors and an abundance of zoea larvae in benthopelagic larvae samples at Chincoteague East, provide what we believe is the first evidence that some seeps may act as a reproductive hotspot for commercially valuable species. Male and female red crabs generally occupy different depth zones, with most females occupying depths <500 m (Wigley et al., 1975). Reproduction is thought to occur at mid-slope depths, with females undergoing a downslope migration either individually or by being carried by males that have migrated upslope (Hastie, 1995). The intermediate depth of Chincoteague East (1,000 m) offers a potential explanation for why high densities of red crab, reproductive behaviors, and an abundance of zoea larvae were observed at Chincoteague and not at other seeps. Chincoteague East was also the largest mussel bed area surveyed (~41,000 m2, Table 1), the extensive nature of the habitat and a high abundance of food offers an additional non-exclusive explanation for the abundance of red crab and the high proportion of methane-derived carbon present in the diet of the individuals sampled. The dense aggregation of red crabs at Chincoteague East (n = 612, ~14 individuals 10 m−2) was ~10 times the abundance previously recorded at New England Seep 2 (Quattrini et al., 2015) and 30 times the abundance observed at Baltimore seep (this study). Dense aggregations of red crab were also observed at Chincoteague East during the IMMeRSS (Interagency Mission on Methane Research at Seafloor Seeps) cruise in 2017 (Toran et al., 2017), suggesting that such aggregations may either be long-lived or recur at the same location. Regular sampling of red crab and repeated larval surveys are needed to determine if Chincoteague seep consistently acts as a food resource and reproductive hotspot, with aggregations perhaps coinciding with a seasonal reproductive pattern. Furthermore, it is only with continued study of the hundreds of unexplored methane seeps on the US Atlantic margin (Skarke et al., 2014) that we will understand whether the Chincoteague seep is an exception, or just an example of the importance of seep ecosystems to red crab life history.
Conclusion
In summary, Bathymodiolus childressi dominates US Atlantic margin seeps north of Cape Hatteras and there is evidence for depth-related zonation in the seep-associated megafauna observed at shallow (~400 m) and deep (~1,500 m) seeps. Depth, as well as environmental variables that co-vary with depth (e.g., temperature), were found to be more important in structuring seep communities than geographic distance. In addition, US Atlantic margin seeps can provide trophic support to commercially important red crab populations and some seeps may act as a reproductive hotspot for red crabs. Numerous studies have found commercially important species to be present at seep ecosystems but few studies discuss trophic (Seabrook et al., 2019; this study) or reproductive (this study) connections between seeps and commercial species, suggesting that this is a fruitful area for future research. Given the hundreds of unexplored methane seeps on the US Atlantic margin, continued exploration will build a more complete picture of the ecosystem services provided by methane seeps.
Data Availability Statement
The datasets generated for this study can be found in GenBank: MN265823, MN265821, MN265820, MN265822, KX159910, KX159911, KX159912, KX159913, KX159914, KX159915, KX159916, KX159917, KX159918, KX159919, KX159920, KX159921, KX159922, KX159923, KX159924, KX159884, KX159891, KX159892, KX159889, KX159903, KX159904, KX159905, KX159906, KX159907, KX159908, KX159909, KX159898, KX159866, KX159899, KX159900, KX159901, KX159902, KX159883, KX159885, KX159886, KX159887, KX159888, KX159882, KX159890, KX159893, KX159894, KX159895, KX159896, and KX159897.
Author Contributions
PT conducted the majority of photo transects during data collection, analyzed the data, and wrote the manuscript. BB conducted molecular analysis of the mussel samples and interpreted the results. SM and MP conducted molecular analysis of the crab larvae and interpreted the results. ZD assisted with assessing red crab abundance at Chincoteague East. AF-B and LV assisted with the collection and analysis of environmental data. IG and DM assisted with the frame-grabber photo analysis. CY and SM were co-principle investigators on the SEEPC grant and CY led the plankton sampling effort on board the R/V Atlantis. CV was principle investigator on the SEEPC grant, provided supervision during data analysis, and critical revisions of the manuscript. All authors provided edits for the manuscript and approved the manuscript for publication.
Funding
This research was supported by NSF (Biological Oceanography) awards OCE-1031050 to CV and OCE-1030453 to CY and SM. PT was also supported by the Graduate School of Duke University.
Conflict of Interest
The authors declare that the research was conducted in the absence of any commercial or financial relationships that could be construed as a potential conflict of interest.
Acknowledgments
We thank the crew of the R/V Atlantis, the ship and shore science teams, the DSV Alvin and AUV Sentry teams, as well as the Advanced Imaging and Visualization Laboratory at Woods Hole Oceanographic Institute. We thank cruise participants from the Oregon Institute of Marine Biology and North Carolina State University for their help in data collection and processing samples, as well as Adam Skarke, Jill Bourque, and Carolyn Ruppel for valuable discussions concerning seeps on the US Atlantic margin.
Supplementary Material
The Supplementary Material for this article can be found online at: https://www.frontiersin.org/articles/10.3389/fmars.2020.00075/full#supplementary-material
Supplementary Video 1. Deep-sea red crab (Chaceon quinquedens) observed sifting through sediment and feeding in an area of active methane seepage at New England Seep 2 (Alvin dive 4815, depth ~1,400 m).
References
Amon, D. J., Gobin, J., Van Dover, C. L., Levin, L. A., Marsh, L., and Raineault, N. A. (2017). Characterization of methane-seep communities in a deep-sea area designated for oil and natural gas exploitation off Trinidad and Tobago. Front. Mar. Sci. 4:342. doi: 10.3389/fmars.2017.00342
Arellano, S. M., Van Gaest, A. L., Johnson, S. B., Vrijenhoek, R. C., and Young, C. M. (2014). Larvae from deep-sea methane seeps disperse in surface waters. Proc. R. Soc. B Biol. Sci. 281:20133276. doi: 10.1098/rspb.2013.3276
Armstrong, C. W., Foley, N. S., Tinch, R., and van den Hove, S. (2012). Services from the deep: steps towards valuation of deep sea goods and services. Ecosyst. Serv. 2, 2–13. doi: 10.1016/j.ecoser.2012.07.001
Assié, A., Borowski, C., van der Heijden, K., Raggi, L., Geier, B., Leisch, N., et al. (2016). A specific and widespread association between deep-sea Bathymodiolus mussels and a novel family of Epsilonproteobacteria. Environ. Microbiol. Rep. 8, 805–813. doi: 10.1111/1758-2229.12442
Baker, K. D., Haedrich, R. L., Snelgrove, P. V. R., Wareham, V. E., Edinger, E. N., and Gilkinson, K. D. (2012). Small-scale patterns of deep-sea fish distributions and assemblages of the Grand Banks, Newfoundland continental slope. Deep Sea Res. Part I Oceanogr. Res. Pap. 65, 171–188. doi: 10.1016/j.dsr.2012.03.012
Barry, J. P., and Kochevar, R. E. (1998). A tale of two clams: differing chemosynthetic life styles among vesicomyds in Monterey Bay cold seeps. Cah. Biol. Mar. 39, 329–331.
Beck, M. W., Heck, K. L., Able, K. W., Childers, D. L., Eggleston, D. B., Gillanders, B. M., et al. (2001). The identification, conservation, and management of estuarine and marine nurseries for fish and invertebrates: a better understanding of the habitats that serve as nurseries for marine species and the factors that create site-specific variability in nurse. Bioscience 51, 633–641. doi: 10.1641/0006-3568(2001)051[0633:TICAMO]2.0.CO;2
Bennett, S. A., Van Dover, C. L., Breier, J. A., and Coleman, M. (2015). Effect of depth and vent fluid composition on the carbon sources at two neighboring deep-sea hydrothermal vent fields (Mid-Cayman Rise). Deep Sea Res. Part I Oceanogr. Res. Pap. 104, 122–133. doi: 10.1016/j.dsr.2015.06.005
Bernardino, A. F., Levin, L. A., Thurber, A. R., and Smith, C. R. (2012). Comparative composition, diversity and trophic ecology of sediment macrofauna at vents, seeps and organic falls. PLoS ONE 7:e33515. doi: 10.1371/journal.pone.0033515
Berndt, C. (2005). Focused fluid flow in passive continental margins. Philos. Trans. R. Soc. A 363, 2855–2871. doi: 10.1098/rsta.2005.1666
Billings, A., Kaiser, C., Young, C. M., Hiebert, L. S., Cole, E., Wagner, J. K. S., et al. (2017). SyPRID sampler: a large-volume, high-resolution, autonomous, deep-ocean precision plankton sampling system. Deep Sea Res. Part II Top. Stud. Oceanogr. 137, 297–306. doi: 10.1016/j.dsr2.2016.05.007
Bourque, J. R., Robertson, C. M., Brooke, S., and Demopoulos, A. W. J. (2017). Macrofaunal communities associated with chemosynthetic habitats from the U.S. Atlantic margin: a comparison among depth and habitat types. Deep Sea Res. Part II Top. Stud. Oceanogr. 137, 42–55. doi: 10.1016/j.dsr2.2016.04.012
Bower, A. S., and Hunt, H. D. (2000). Lagrangian observations of the deep western boundary current in the North Atlantic Ocean. J. Phys. Oceanogr. 30, 764–783. doi: 10.1175/1520-0485(2000)030<0764:LOOTDW>2.0.CO;2
Brooks, J. M., Kennicutt, M. C., Fisher, C. R., Macko, S. A., Cole, K., Childress, J. J., et al. (1987). Deep-sea hydrocarbon seep communities: evidence for energy and nutritional carbon sources. Science 238, 1138–1142. doi: 10.1126/science.238.4830.1138
Brothers, L. L., Van Dover, C. L., German, C. R., Kaiser, C. L., Yoerger, D. R., Ruppel, C. D., et al. (2013). Evidence for extensive methane venting on the southeastern U.S. Atlantic margin. Geology 41, 807–810. doi: 10.1130/G34217.1
Carney, R. S. (2005). Zonation of deep biota on continental margins. Oceanogr. Mar. Biol. 43, 211–278. doi: 10.1201/9781420037449.ch6
Chao, A., Gotelli, N. J., Hsieh, T. C., Sander, E. L., Ma, K. H., Colwell, R. K., et al. (2014). Rarefaction and extrapolation with Hill numbers: a framework for sampling and estimation in species diversity studies. Ecol. Monogr. 84, 45–67. doi: 10.1890/13-0133.1
Childress, J. J., Fisher, C. R., Brooks, J. M., Kennicutt, M. C., Bidigare, R., and Anderson, A. E. (1986). A methanotrophic marine molluscan (Bivalvia, Mytilidae) symbiosis: mussels fueled by gas. Science 233, 1306–1308. doi: 10.1126/science.233.4770.1306
Clarke, K. R., and Gorley, R. N. (2015). PRIMER v7: User Manual/Tutorial. Lutton; Ivybridge: PRIMER-E Ltd.
Cordes, E. E., Becker, E. L., Hourdez, S., and Fisher, C. R. (2010). Influence of foundation species, depth, and location on diversity and community composition at Gulf of Mexico lower-slope cold seeps. Deep Sea Res. Part II Top. Stud. Oceanogr. 57, 1870–1881. doi: 10.1016/j.dsr2.2010.05.010
Cordes, E. E., Bergquist, D. C., and Fisher, C. R. (2009). Macro-ecology of gulf of mexico cold seeps. Ann. Rev. Mar. Sci. 1, 143–168. doi: 10.1146/annurev.marine.010908.163912
Cordes, E. E., Carney, S. L., Hourdez, S., Carney, R. S., Brooks, J. M., and Fisher, C. R. (2007). Cold seeps of the deep Gulf of Mexico: community structure and biogeographic comparisons to Atlantic equatorial belt seep communities. Deep Sea Res. Part I Oceanogr. Res. Pap. 54, 637–653. doi: 10.1016/j.dsr.2007.01.001
Cosson, N., Sibuet, M., and Galeron, J. (1997). Community structure and spatial heterogeneity of the deep-sea macrofauna at three contrasting stations in the tropical northeast Atlantic. Deep Sea Res. Part I Oceanogr. Res. Pap. 44, 247–269. doi: 10.1016/S0967-0637(96)00110-0
Coykendall, D. K., Cornman, R. S., Prouty, N. G., Brooke, S., Demopoulos, A. W. J., and Morrison, C. L. (2019). Molecular characterization of Bathymodiolus mussels and gill symbionts associated with chemosynthetic habitats from the U.S. Atlantic margin. PLoS ONE 14:e0211616. doi: 10.1371/journal.pone.0211616
Cutler, E. B. (1975). Zoogeographical barrier on the continental slope off cape lookout, Nort Carolina. Deep Sea Res. Oceanogr. Abstr. 22, 893–901. doi: 10.1016/0011-7471(75)90091-1
Dattagupta, S., Bergquist, D. C., Szalai, E. B., Macko, S. A., and Fisher, C. R. (2004). Tissue carbon, nitrogen, and sulfur stable isotope turnover in transplanted Bathymodiolus childressi mussels: relation to growth and physiological condition. Limnol. Oceanogr. 49, 1144–1151. doi: 10.4319/lo.2004.49.4.1144
De Juan, S., and Lleonart, J. (2010). A conceptual framework for the protection of vulnerable habitats impacted by fishing activities in the Mediterranean high seas. Ocean Coast. Manag. 53, 717–723. doi: 10.1016/j.ocecoaman.2010.10.005
Demopoulos, A., McClain-Counts, J., Ross, S., Brooke, S., and Mienis, F. (2017). Food-web dynamics and isotopic niches in deep-sea communities residing in a submarine canyon and on the adjacent open slopes. Mar. Ecol. Prog. Ser. 578, 19–33. doi: 10.3354/meps12231
Demopoulos, A. W. J., McClain-Counts, J. P., Bourque, J. R., Prouty, N. G., Smith, B. J., Brooke, S., et al. (2019). Examination of Bathymodiolus childressi nutritional sources, isotopic niches, and food-web linkages at two seeps in the US Atlantic margin using stable isotope analysis and mixing models. Deep Sea Res. Part I Oceanogr. Res. Pap. 148, 53–66. doi: 10.1016/j.dsr.2019.04.002
Drazen, J. C., Goffredi, S. K., Schlining, B., and Stakes, D. S. (2003). Aggregations of egg-brooding deep-sea fish and cephalopods on the gorda escarpment: a reproductive hot spot. Biol. Bull. 205, 1–7. doi: 10.2307/1543439
Duperron, S., Sibuet, M., MacGregor, B. J., Kuypers, M. M. M., Fisher, C. R., and Dubilier, N. (2007). Diversity, relative abundance and metabolic potential of bacterial endosymbionts in three Bathymodiolus mussel species from cold seeps in the Gulf of Mexico. Environ. Microbiol. 9, 1423–1438. doi: 10.1111/j.1462-2920.2007.01259.x
Faure, B., Schaeffer, S. W., and Fisher, C. R. (2015). Species distribution and population connectivity of deep-sea mussels at hydrocarbon seeps in the Gulf of Mexico. PLoS ONE 10:e0118460. doi: 10.1371/journal.pone.0118460
Folmer, O., Black, M., Hoeh, W., Lutz, R., and Vrijenhoek, R. (1994). DNA primers for amplification of mitochondrial cytochrome c oxidase subunit I from diverse metazoan invertebrates. Mol. Mar. Biol. Biotechnol. 3, 294–299.
Freire, J., Carabel, S., Verísimo, P., Bernárdez, C., and Fernández, L. (2009). Patterns of juvenile habitat use by the spider crab Maja brachydactyla as revealed by stable isotope analyses. Sci. Mar. 73, 39–49. doi: 10.3989/scimar.2009.73n1039
Gage, J. D., and Tyler, P. A. (1991). Deep-Sea Biology: A Natural History of Organisms at the Deep-Sea Floor. Cambridge: Cambridge University Press. doi: 10.1017/CBO9781139163637
Geller, J., Meyer, C., Parker, M., and Hawk, H. (2013). Redesign of PCR primers for mitochondrial cytochrome c oxidase subunit I for marine invertebrates and application in all-taxa biotic surveys. Mol. Ecol. Resour. 13, 851–861. doi: 10.1111/1755-0998.12138
Govenar, B. (2010). “Shaping vent and seep communities: habitat provision and modification by foundation species,” in The Vent and Seep Biota: Aspect from Microbes to Ecosystems, ed S. Kiel (Dordrecht: Springer), 403–432. doi: 10.1007/978-90-481-9572-5_13
Grupe, B. M., Krach, M. L., Pasulka, A. L., Maloney, J. M., Levin, L. A., and Frieder, C. A. (2015). Methane seep ecosystem functions and services from a recently discovered southern California seep. Mar. Ecol. 36, 91–108. doi: 10.1111/maec.12243
Gustafson, R. G., Turner, R. D., Lutz, R. A., and Vrijenhoek, R. C. (1998). A new genus and five new species of mussels (Bivalvia: Mytilidae) from deep-sea sulfide/hydrocarbon seeps in the Gulf of Mexico. Malacologia 40, 63–112.
Haedrich, R. L., Rowe, G. T., and Polloni, P. T. (1980). The megabenthic fauna in the deep sea south of New England, USA. Mar. Biol. 57, 165–179. doi: 10.1007/BF00390735
Hastie, L. C. (1995). Deep-water geryonid crabs: a continental slope resource. Oceanogr. Mar. Biol. An Annu. Rev. 33, 561–584.
Heyl, T. P., Gilhooly, W. P., Chambers, R. M., Gilchrist, G. W., Macko, S. A., Ruppel, C. D., et al. (2007). Characteristics of vesicomyid clams and their environment at the Blake Ridge cold seep, South Carolina, USA. Mar. Ecol. Prog. Ser. 339, 169–184. doi: 10.3354/meps339169
Hovland, M., Judd, A., and Maisey, G. (1985). North Sea gas feed the North Sea fisheries. New Sci. 1468, 26.
Hsieh, T. C., Ma, K. H., and Chao, A. (2016). iNEXT: an R package for rarefaction and extrapolation of species diversity (Hill numbers). Methods Ecol. Evol. 7, 1451–1456. doi: 10.1111/2041-210X.12613
Hunt, J. M. (1970). “The significance of carbon isotope variations in marine sediments,” in Advances in Organic Geochemistry, eds G. Hobson and G. Speers (Oxford: Pergamon Press Ltd.), 27–35. doi: 10.1016/B978-0-08-012758-3.50005-0
Jones, D. O. B., Bett, B. J., Wynn, R. B., and Masson, D. G. (2009). The use of towed camera platforms in deep-water science. Underw. Technol. 28, 41–50. doi: 10.3723/ut.28.041
Jones, D. T., Wilson, C. D., De Robertis, A., Rooper, C. N., Weber, T. C., and Butler, J. L. (2012). Evaluation of rockfish abundance in untrawlable habitat: combining acoustic and complementary sampling tools. Fish. Bull. 110, 332–343. Available online at: https://pdfs.semanticscholar.org/f079/7523560e8391515379527a3cc771829006ac.pdf
Judd, A. G., and Hovland, M. (1989). The role of chemosynthesis in supporting fish stocks in the North Sea. J. Fish Biol. 35, 329–330. doi: 10.1111/j.1095-8649.1989.tb03077.x
Lambshead, P. J. D., Brown, C. J., Ferrero, T. J., Mitchell, N. J., Smith, C. R., Hawkins, L. E., et al. (2002). Latitudinal diversity patterns of deep-sea marine nematodes and organic fluxes: a test from the central equatorial Pacific. Mar. Ecol. Prog. Ser. 236, 129–135. doi: 10.3354/meps236129
Le, J. T., Levin, L. A., and Carson, R. T. (2017). Incorporating ecosystem services into environmental management of deep-seabed mining. Deep Sea Res. Part II Top. Stud. Oceanogr. 137, 486–503. doi: 10.1016/j.dsr2.2016.08.007
Leduc, D., Rowden, A. A., Bowden, D. A., Probert, P. K., Pilditch, C. A., and Nodder, S. D. (2012). Unimodal relationship between biomass and species richness of deep-sea nematodes: implications for the link between productivity and diversity. Mar. Ecol. Prog. Ser. 454, 53–64. doi: 10.3354/meps09609
Levin, L. A., Baco, A. R., Bowden, D. A., Colaco, A., Cordes, E. E., Cunha, M. R., et al. (2016a). Hydrothermal vents and methane seeps: rethinking the sphere of influence. Front. Mar. Sci. 3:72. doi: 10.3389/fmars.2016.00072
Levin, L. A., and Currin, C. (2012). Stable Isotope Protocols: Sampling and Sample Processing. La Jolla. Available online at: https://escholarship.org/uc/item/3jw2v1hh (accessed April 23, 2019).
Levin, L. A., Etter, R. J., Rex, M. A., Gooday, A. J., Smith, C. R., Pineda, J., et al. (2001). Environmental Influences on Regional Deep-Sea species diversity. Annu. Rev. Ecol. Syst. 32, 51–93. doi: 10.1146/annurev.ecolsys.32.081501.114002
Levin, L. A., Girguis, P. R., German, C. R., Brennan, M. L., Tüzün, S., Wagner, J., et al. (2016b). “Exploration and discovery of methane seeps and associated communities in the California Borderland,” in New Frontiers in Ocean Exploration: The E/V Nautilus and NOAA Ship Okeanos Explorer 2015 Field Season, eds K. Bell, M. Brennan, J. Flanders, N. Raineault, and K. Wagner (Rockville, MD: The Oceanography Society), 40–43.
Levy, E. M., and Lee, K. (1988). Potential contribution of natural hydrocarbon seepage to benthic productivity and the fisheries of Atlantic Canada. Can. J. Fish. Aquat. Sci. 45, 349–352. doi: 10.1139/f88-041
Lorrain, A., Paulet, Y. M., Chauvaud, L., Savoye, N., Donval, A., and Saout, C. (2002). Differential δ13C and δ15N signatures among scallop tissues: implications for ecology and physiology. J. Exp. Mar. Bio. Ecol. 275, 47–61. doi: 10.1016/S0022-0981(02)00220-4
MacAvoy, S. E., Carney, R. S., Fisher, C. R., and Macko, S. A. (2002). Use of chemosynthetic biomass by large, mobile, benthic predators in the Gulf of Mexico. Mar. Ecol. Prog. Ser. 225, 65–78. doi: 10.3354/meps225065
MacDonald, I. R., Boland, G. S., Baker, J. S., Brooks, J. M., Kennicutt, II M. C., and Bidigare, R. R. (1989). Gulf of Mexico hydrocarbon seep communities. Mar. Biol. 101, 235–247. doi: 10.1007/BF00391463
Martin, J. H., Knauer, G. A., Karl, D. M., and Broenkow, W. W. (1987). VERTEX: carbon cycling in the northeast Pacific. Deep Sea Res. Part A. Oceanogr. Res. Pap. 34, 267–285. doi: 10.1016/0198-0149(87)90086-0
Mayer, L. A., Shor, A. N., Hughes Clarke, J., and Piper, D. J. W. (1988). Dense biological communities at 3850 m on the Laurentian Fan and their relationship to the deposits of the 1929 grand banks earthquake. Deep Sea Res. Part A. Oceanogr. Res. Pap. 35, 1235–1246. doi: 10.1016/0198-0149(88)90079-9
McClain, C. R., Allen, A. P., Tittensor, D. P., and Rex, M. A. (2012). Energetics of life on the deep seafloor. Proc Natl Acad Sci U.S.A. 109:15366–15371. doi: 10.1073/pnas.1208976109
McVeigh, D., Skarke, A., Dekas, A. E., Borrelli, C., Hong, W. L., Marlow, J. J., et al. (2018). Characterization of benthic biogeochemistry and ecology at three methane seep sites on the Northern U.S. Atlantic margin. Deep Sea Res. Part II Top. Stud. Oceanogr. 150, 41–56. doi: 10.1016/j.dsr2.2018.03.001
Meyer, K. S., Wagner, J. K. S., Ball, B., Turner, P. J., Young, C. M., and Van Dover, C. L. (2016). Hyalinoecia artifex: Field notes on a charismatic and abundant epifaunal polychaete on the US Atlantic continental margin. Invertebr. Biol. 135, 211–224. doi: 10.1111/ivb.12132
Möller, E. M., Bahnweg, G., Sandermann, H., and Geiger, H. H. (1992). A simple and efficient protocol for isolation of high molecular weight DNA from filamentous fungi, fruit bodies, and infected plant tissues. Nucleic Acids Res. 20, 6115–6116. doi: 10.1093/nar/20.22.6115
Musick, J. A. (1979). Community structure of fishes on the continental slope and rise off the middle Atlantic coast of the United States. Spec. Sci. Rep. Virginia Inst. Mar. Sci. 96:126. doi: 10.21220/m2-96a5-sn27
NEFMC (2013). Atlantic Deep-Sea Red Crab Fishing Years 2014-2016 Specifications, Including a Regulatory Flexibility Analysis. 33. Available online at: http://archive.nefmc.org/crab/annual_specs/Submitted121313_RedCrabSpecificationPackageSubmission.pdf (accessed June 28, 2019).
NEFSC (2006). “Stock assessment of deep sea red crab,” in 43rd Northeast Regional Stock Assessment Workshop: 43rd SAW Assessment Report (Woods Hole, MA: Northeast Fisheries Science Center), 400. Available online at: https://www.nefsc.noaa.gov/publications/crd/crd0614/
Niemann, H., Linke, P., Knittel, K., MacPherson, E., Boetius, A., Brückmann, W., et al. (2013). Methane-carbon flow into the benthic food web at cold seeps – a case study from the Costa Rica Subduction Zone. PLoS ONE 8:e74894. doi: 10.1371/journal.pone.0074894
NOAA (2019). Scientists Discover Deep-sea Tubeworms Living at Seeps 36 Miles Offshore North Carolina. NOAA Ocean Explorer Research 1. Available online at: https://oceanexplorer.noaa.gov/explorations/19deepsearch/logs/may8/welcome.html (accessed June 28, 2019).
Olu, K., Cordes, E. E., Fisher, C. R., Brooks, J. M., Sibuet, M., and Desbruyères, D. (2010). Biogeography and potential exchanges among the atlantic equatorial belt cold-seep faunas. PLoS ONE 5:e11967. doi: 10.1371/journal.pone.0011967
Olu-Le Roy, K., von Cosel, R., Hourdez, S., Carney, S. L., and Jollivet, D. (2007). Amphi-Atlantic cold-seep Bathymodiolus species complexes across the equatorial belt. Deep Sea Res. Part I Oceanogr. Res. Pap. 54, 1890–1911. doi: 10.1016/j.dsr.2007.07.004
Page, H. M., Fisher, C. R., and Childress, J. J. (1990). Role of filter-feeding in the nutritional biology of a deep-sea mussel with methanotrophic symbionts. Mar. Biol. 104, 251–257. doi: 10.1007/BF01313266
Paull, C. K., Ussler, W., Borowski, W. S., and Spiess, F. N. (1995). Methane-rich plumes on the Carolina continental rise: associations with gas hydrates. Geology 23, 89–92. doi: 10.1130/0091-7613(1995)023<0089:MRPOTC>2.3.CO;2
Perkins, H. C. (1972). The larval stages of the deep sea red crab, Geryon quinquedens Smith, reared under labratory conditions (Decapoda: Brachyrhyncha). Fish. Bull. 71, 69–82.
Pile, A. J., and Young, C. M. (1999). Plankton availability and retention efficiencies of cold-seep symbiotic mussels. Limnol. Oceanogr. 44, 1833–1839. doi: 10.4319/lo.1999.44.7.1833
Powell, A., Clarke, M. E., Fruh, E., Chaytor, J. D., Reiswig, H. M., and Whitmire, C. E. (2018). Characterizing the sponge grounds of Grays Canyon, Washington, USA. Deep Sea Res. Part II Top. Stud. Oceanogr. 150, 146–155. doi: 10.1016/j.dsr2.2018.01.004
Prouty, N. G., Sahy, D., Ruppel, C. D., Roark, E. B., Condon, D., Brooke, S., et al. (2016). Insights into methane dynamics from analysis of authigenic carbonates and chemosynthetic mussels at newly-discovered Atlantic Margin seeps. Earth Planet. Sci. Lett. 449, 332–344. doi: 10.1016/j.epsl.2016.05.023
Quattrini, A. M., Nizinski, M. S., Chaytor, J. D., Demopoulos, A. W. J., Roark, E. B., France, S. C., et al. (2015). Exploration of the canyon-incised continental margin of the Northeastern United States reveals dynamic habitats and diverse communities. PLoS ONE 10:e0139904. doi: 10.1371/journal.pone.0139904
R Core Team (2017). R: A Language and Environment for Statistical Computing. Vienna: R Foundation for Statistical Computing. Available online at: https://www.R-project.org/
Rex, M. A., Stuart, C. T., and Coyne, G. (2000). Latitudinal gradients of species richness in the deep-sea benthos of the North Atlantic. Proc. Natl. Acad. Sci. 97, 4082–4085. doi: 10.1073/pnas.050589497
Roberts, C. M., Hawkins, J. P., and Gell, F. R. (2005). The role of marine reserves in achieving sustainable fisheries. Philos. Trans. R. Soc. London B Biol. Sci. 360, 123–132. doi: 10.1098/rstb.2004.1578
Ross, S. W., Rhode, M., and Quattrini, A. M. (2015). Demersal fish distribution and habitat use within and near Baltimore and Norfolk Canyons, U.S. middle Atlantic slope. Deep Sea Res. Part I Oceanogr. Res. Pap. 103, 137–154. doi: 10.1016/j.dsr.2015.06.004
Rueda, J. L., Díaz-Del-Río, V., Sayago-Gil, M., López-González, N., Fernández-Salas, L. M., and Vázquez, J. T. (2012). “Fluid venting through the seabed in the Gulf of Cadiz (SE Atlantic Ocean, Western Iberian Peninsula): geomorphic features, habitats, and associated Fauna,” in Seafloor Geomorphology as Benthic Habitat: GeoHAB Atlas of Seafloor Geomorphic Features and Benthic Habitats, eds P. Harris and E. Baker (Elsevier), 831–842. doi: 10.1016/B978-0-12-385140-6.00061-X
Ruess, L., and Chamberlain, P. M. (2010). The fat that matters: Soil food web analysis using fatty acids and their carbon stable isotope signature. Soil Biol. Biochem. 42, 1898–1910. doi: 10.1016/j.soilbio.2010.07.020
Seabrook, S., De Leo, F. C., and Thurber, A. R. (2019). Flipping for food: the use of a methane seep by tanner crabs (Chionoecetes tanneri). Front. Mar. Sci. 6:43. doi: 10.3389/fmars.2019.00043
Sellanes, J., Pedraza, M. J., and Zapata Hernandez, G. (2012). Las areas de filtracion de metano constituyen zonas de agregación del bacalao de profundidad (Dissostichus eleginoides) frente a Chile central. Lat. Am. J. Aquat. Res. 40, 980–991. doi: 10.3856/vol40-issue4-fulltext-14
Sellanes, J., Quiroga, E., and Neira, C. (2008). Megafauna community structure and trophic relationships at the recently discovered Concepcion Methane Seep Area, Chile, 36 S. ICES J. Mar. Sci. 65, 1102–1111. doi: 10.1093/icesjms/fsn099
Sen, A., Åström, E. K. L., Hong, W. L., Portnov, A., Waage, M., Serov, P., et al. (2018). Geophysical and geochemical controls on the megafaunal community of a high Arctic cold seep. Biogeosciences 15, 4533–4559. doi: 10.5194/bg-15-4533-2018
Sibuet, M., and Olu, K. (1998). Biogeography, biodiversity and fluid dependence of deep-sea cold-seep communities at active and passive margins. Deep Sea Res. Part II Top. Stud. Oceanogr. 45, 517–567. doi: 10.1016/S0967-0645(97)00074-X
Sitjà, C., Maldonado, M., Farias, C., and Rueda, J. L. (2019). Deep-water sponge fauna from the mud volcanoes of the Gulf of Cadiz (North Atlantic, Spain). J. Mar. Biol. Assoc. United Kingdom 99, 807–831. doi: 10.1017/S0025315418000589
Skarke, A., Ruppel, C., Kodis, M., Brothers, D., and Lobecker, E. (2014). Widespread methane leakage from the sea floor on the northern US Atlantic margin. Nat. Geosci. 7, 657–661. doi: 10.1038/ngeo2232
Snelgrove, P. V. R., and Haedrich, R. L. (1985). Structure of the deep demersal fish fauna off Newfoundland. Mar. Ecol. Prog. Ser. 27, 99–107. doi: 10.3354/meps027099
Suess, E. (1980). Particulate organic carbon flux in the oceans—surface productivity and oxygen utilization. Nature 288, 260–263. doi: 10.1038/288260a0
Sweetman, A. K., Thurber, A. R., Smith, C. R., Levin, L. A., Mora, C., Wei, C. L., et al. (2017). Major impacts of climate change on deep-sea benthic ecosystems. Elem Sci Anth. 5:4. doi: 10.1525/elementa.203
Thurber, A. R., Sweetman, A. K., Narayanaswamy, B. E., Jones, B., Ingels, J., and Hansman, R. L. (2014). Ecosystem function and services provided by the deep sea. Biogeosciences 11, 3941–3963. doi: 10.5194/bg-11-3941-2014
Toran, A., Ruppel, C., Demopoulos, A., and Prouty, N. (2017). Real-Time Public Engagement in Deep-Water Remotely Operated Vehicle Dives at Methane Seeps. USGS Sound Waves. Available online at: https://archive.usgs.gov/archive/sites/soundwaves.usgs.gov/2017/05/outreach.html (accessed August 13, 2019).
Torres, M. E., and Bohrmann, G. (2014). “Cold seeps,” in Encyclopedia of Marine Geosciences, eds J. Harff, M. Meschede, S. Petersen, and J. Thiede (Dordrecht: Springer). doi: 10.1007/978-94-007-6644-0_153-1
Treude, T., Kiel, S., Linke, P., Peckmann, J., and Goedert, J. L. (2011). Elasmobranch egg capsules associated with modern and ancient cold seeps: a nursery for marine deep-water predators. Mar. Ecol. Prog. Ser. 437, 175–181. doi: 10.3354/meps09305
Tunnicliffe, V., Juniper, S. K., and Sibuet, M. (2003). “Reducing environments of the deep-sea floor,” in Ecosystems of the Deep Oceans, ed P. A. Tyler (Amsterdam: Elsevier Science B.V.), 81–110.
Tyler, P., Young, C. M., Dolan, E., Arellano, S. M., Brooke, S. D., and Baker, M. (2007). Gametogenic periodicity in the chemosynthetic cold-seep mussel “Bathymodiolus” childressi. Mar. Biol. 150, 829–840. doi: 10.1007/s00227-006-0362-9
Van Dover, C. L., Aharon, P., Bernhard, J. M., Caylor, E., Doerries, M., Flickinger, W., et al. (2003). Blake Ridge methane seeps: characterization of a soft-sediment, chemosynthetically based ecosystem. Deep Sea Res. Part I Oceanogr. Res. Pap. 50, 281–300. doi: 10.1016/S0967-0637(02)00162-0
Wagner, J. K. S., McEntee, M. H., Brothers, L. L., German, C. R., Kaiser, C. L., Yoerger, D. R., et al. (2013). Cold-seep habitat mapping: High-resolution spatial characterization of the Blake Ridge Diapir seep field. Deep Sea Res. Part II 92, 183–188. doi: 10.1016/j.dsr2.2013.02.008
Wahle, R. A., Bergeron, C. E., Chute, A. S., Jacobson, L. D., and Chen, Y. (2008). The Northwest Atlantic deep-sea red crab (Chaceon quinquedens) population before and after the onset of harvesting. ICES J. Mar. Sci. 65, 862–872. doi: 10.1093/icesjms/fsn058
Walsh, J. J., Premuzic, E. T., Gaffney, J. S., Rowe, G. T., Harbottle, G., Stoenner, R. W., et al. (1985). Organic storage of CO2 on the continental slope off the mid-Atlantic bight, the southeastern Bering Sea, and the Peru coast. Deep Sea Res. 32, 853–883. doi: 10.1016/0198-0149(85)90120-7
Wei, C.-L., Rowe, G. T., Hubbard, G. F., Scheltema, A. H., Wilson, G. D. F., Petrescu, I., et al. (2010). Bathymetric zonation of deep-sea macrofauna in relation to export of surface phytoplankton production. Mar. Ecol. Prog. Ser. 399, 1–14. doi: 10.3354/meps08388
Wigley, R. L., Theroux, R. B., and Murray, H. E. (1975). Deep-Sea red crab, Geryon quinquedens, survey off Northeastern United States. Mar. Fish. Rev. 37, 1–21.
Yasuhara, M., and Danovaro, R. (2016). Temperature impacts on deep-sea biodiversity. Biol. Rev. 91, 275–287. doi: 10.1111/brv.12169
Young, C. M., He, R., Emlet, R. B., Li, Y., Qian, H., Arellano, S. M., et al. (2012). Dispersal of Deep-Sea Larvae from the Intra-American Seas: simulations of trajectories using ocean models. Integr. Comp. Biol. 52, 483–496. doi: 10.1093/icb/ics090
Keywords: cold seeps, Western Atlantic, ecosystem services, fishery, fisheries, Bathymodiolus, biogeography
Citation: Turner PJ, Ball B, Diana Z, Fariñas-Bermejo A, Grace I, McVeigh D, Powers MM, Van Audenhaege L, Maslakova S, Young CM and Van Dover CL (2020) Methane Seeps on the US Atlantic Margin and Their Potential Importance to Populations of the Commercially Valuable Deep-Sea Red Crab, Chaceon quinquedens. Front. Mar. Sci. 7:75. doi: 10.3389/fmars.2020.00075
Received: 09 September 2019; Accepted: 30 January 2020;
Published: 27 February 2020.
Edited by:
Erik Cordes, Temple University, United StatesReviewed by:
David A. Bowden, National Institute of Water & Atmospheric Research, Auckland, New ZealandAmanda Demopoulos, United States Geological Survey (USGS), United States
Andrew R. Thurber, Oregon State University, United States
Copyright © 2020 Turner, Ball, Diana, Fariñas-Bermejo, Grace, McVeigh, Powers, Van Audenhaege, Maslakova, Young and Van Dover. This is an open-access article distributed under the terms of the Creative Commons Attribution License (CC BY). The use, distribution or reproduction in other forums is permitted, provided the original author(s) and the copyright owner(s) are credited and that the original publication in this journal is cited, in accordance with accepted academic practice. No use, distribution or reproduction is permitted which does not comply with these terms.
*Correspondence: Phillip J. Turner, cGhpbGxpcC50dXJuZXJAYWx1bW5pLmR1a2UuZWR1