- 1Department of Biological Sciences, University of South Florida St. Petersburg, St. Petersburg, FL, United States
- 2NMFS National Systematics Laboratory, National Museum of Natural History, Smithsonian Institution, Washington, DC, United States
Cephalopods are important in midwater ecosystems of the Gulf of Mexico (GOM) as both predator and prey. Vertical distribution and migration patterns (both diel and ontogenic) are not known for the majority of deep-water cephalopods. These varying patterns are of interest as they have the potential to contribute to the movement of large amounts of nutrients and contaminants through the water column during diel migrations. This can be of particular importance if the migration traverses a discrete layer with particular properties, as happened with the deep-water oil plume located between 1000 and 1400 m during the Deepwater Horizon (DWH) oil spill. Two recent studies focusing on the deep-water column of the GOM [2011 Offshore Nekton Sampling and Analysis Program (ONSAP) and 2015–2018 Deep Pelagic Nekton Dynamics of the Gulf of Mexico (DEEPEND)] program, produced a combined dataset of over 12,500 midwater cephalopod records for the northern GOM region. We summarize vertical distribution patterns of cephalopods from the cruises that utilized a 10 m2 Multiple Opening/Closing Net and Environmental Sensing System (MOC10). About 95% of the cephalopods analyzed here either move through or live within 1000–1400 m zone. Species accounts include those with synchronous (e.g., Pterygioteuthis sp.) and asynchronous (e.g., Stigmatoteuthis arcturi) vertical migration. Non-migration patterns of some midwater cephalopods (e.g., Vampyroteuthis infernalis) are also highlighted. Ontogenic shifts are noted for some species examined.
Introduction
The Deepwater Horizon (DWH) oil spill generated one of the largest oil spill responses to date, including millions of dollars allocated to scientific research to examine the impacts of this devastating event (i.e., Gulf of Mexico Research Initiative, NAS Gulf Research Program). It was not only a blowout that affected the seafloor immediately surrounding the wellhead, the surface, and coastline, but also a midwater event. One of the questions post-spill was “Where has all the oil gone”? Passow and Hetland (2016) determined that a deep plume of trapped oil formed between 1000 and 1400 m depth (Socolofsky et al., 2011) due to the petrocarbons that became neutrally buoyant in seawater at that depth. This contaminated layer dispersed from the site via subsurface currents that impinged on the continental slope of the region, leaving extensive oiled sediment (Romero et al., 2015).
Two comprehensive, long-term programs established post-spill have examined the faunal groups found within the water column (0–1500 m) in the northern Gulf of Mexico (GOM). The Offshore Nekton Sampling and Analysis Program (ONSAP) and the Deep Pelagic Nekton Dynamics of the Gulf of Mexico (DEEPEND) consortium have compiled an immense dataset for midwater fauna over a period of 8 years (2011–2018) examining biodiversity and contaminant questions about multiple deep-water pelagic faunal groups (Judkins et al., 2016; Burdett et al., 2017; Richards et al., 2018; Romero et al., 2018).
Historically, the oceanic midwater environment has received little attention (Webb et al., 2010), as it is very difficult to study. However, it is the largest biome on Earth (Robison, 2004; Sutton, 2013). Past deep-sea cephalopod inventories include work conducted using various methods such as closing-net systems as well as large, open trawl nets (Nesis, 1972, 1993; Lu and Clarke, 1975a, b; Young, 1978; Vecchione and Pohle, 2002; Judkins et al., 2016). Recently, remotely operated vehicles have been used to collect precise observations on vertical distribution and other properties (Vecchione et al., 2001; Widder et al., 2005; Robison et al., 2017).
Another, less direct technique that has been utilized to assess cephalopod biodiversity and distributional patterns is the examination of stomach contents from predators. Cephalopods are major prey items for many vertebrates, including seabirds, large tunas and billfishes, and marine mammals (Summers, 1983; Williams, 1995; Lansdell and Young, 2003; Xavier et al., 2018). These studies have examined gut contents and through knowledge of the predators and their migrations and feeding grounds, inferred cephalopod distributions, both spatially as well as vertically.
While faunal inventories and indirect methods to assess deep-sea cephalopod diversity and distribution are important, few vertical distribution patterns have been compiled. Efforts in the 1970s reported vertical distribution of cephalopods in various regions (Clarke and Lu, 1975; Lu and Clarke, 1975a, b; Roper and Young, 1975; Young, 1978). Since that time, vertical distribution patterns have been documented for various zooplankton groups such as fish larvae and cephalopod paralarvae (Hopkins, 1982; Ropke et al., 1993; Salman et al., 2003) but larger specimens have not been included. There are approximately 700 species of cephalopods worldwide (Young et al., 2019) with great morphological and genetic variation among the families, especially those of oceanic habitats. Vertical distribution patterns vary among cephalopod taxa and developmental stages.
The deep oil plume of the DWH oil spill was detected in May, 2010 (Dierks et al., 2010) and persisted for months after that (Camilli et al., 2010; Joye et al., 2011; Reddy et al., 2014) as a horizontal plume that moved toward the southwest with deep-water currents (Melvin et al., 2016). There was also an anomaly of low dissolved oxygen observed at depths of 1100–1200 m below the surface (Kessler et al., 2011). This deep-water oil plume exposed any meso- and/or bathypelagic organisms living in or moving through this plume to potential toxicity.
In light of the need to know which cephalopods may have been exposed to the deep oil plume, as well as a need for comprehensive accounts on deep-sea species, this study reports on 39 cephalopod species from the northern GOM, examining the following questions: (1) How many species move through or are found within the deep oil plume located between 1000 and 1400 m? (2) What are the vertical distribution patterns of deep-sea cephalopods in water column of the northern GOM and how does this relate to past accounts of these species? (3) Are there developmental shifts in vertical distribution of these cephalopods?
Materials and Methods
Two midwater sampling programs provided all material for this analysis. ONSAP (2011) and the DEEPEND (2015–2018) programs sampled in the northern GOM (Figure 1). A 10 m2 Multiple Opening and Closing Net and Environmental Sensing System (MOC10) (Wiebe et al., 1976) with 3 mm mesh was used by both programs (as described in DEEPEND, 2015). The following depths were targeted on each MOC10 tow during both cruise programs: 1200–1500, 1000–1200, 600–1000, 200–600, and 0–200 m at all 169 stations where cephalopods were collected and tows quantified. Sampling depths were chosen based on the following premise: 1200–1500 m was a depth range below where a subsurface hydrocarbon plume was detected during the initial spill; 1000–1200 m fished through the hydrocarbon plume (Reddy et al., 2012); 600–1000 m fished where the vertically migrating species are known to reside during the day; 200–400 m fished where vertical migrators are known to move through during daily migrations; and 0–200 m fished the epipelagic zone where vertical migrators gather at night (Burdett et al., 2017). Sampling was conducted twice at each station per cruise (∼solar noon and ∼midnight).
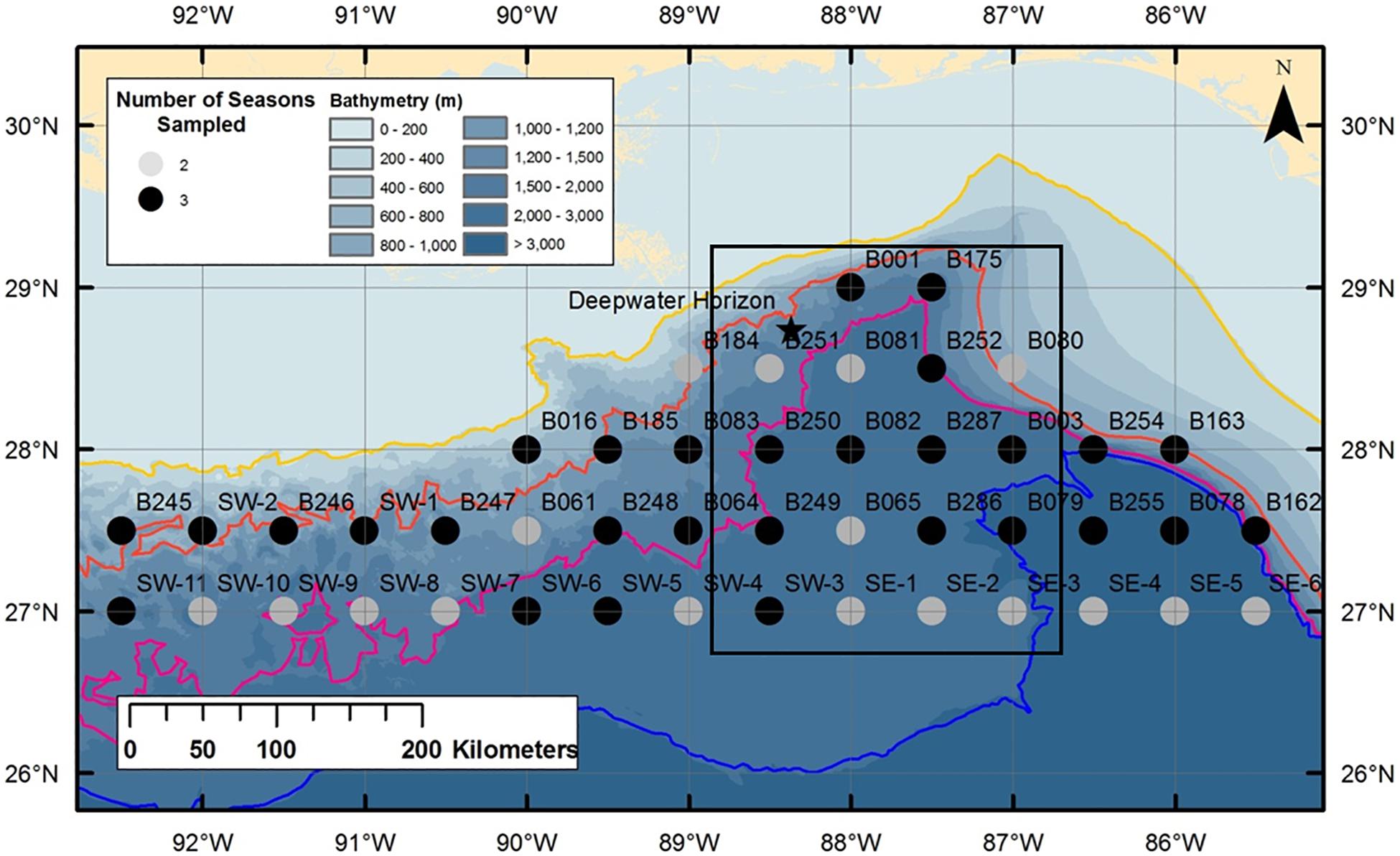
Figure 1. Station sites for ONSAP and DEEPEND MOC10 cruises. Modified from Florida Fish and Wildlife Research Institute SEAMAP cruise stations. Circles, ONSAP stations; black box, DEEPEND stations; star, DWH oil spill site.
Three thousand seven hundred and thirty cephalopods were documented using the MOC10 from both programs. Flow metering on the trawls allowed inference of the amount of water filtered through each net and these were used to standardize abundance per volume of water per tow. The 2150 specimens included in the analyses here were those individuals collected in quantifiable trawl tows. Specimens were measured and identified to species level when possible at sea and either sampled for molecular projects (i.e., Sosnowski, 2017; Timm et al., 2020), frozen, or preserved (10% formalin). Identifications were verified or corrected post-cruise. Non-parametric Kruskal–Wallis analyses were conducted to compare the standardized abundances of the six most abundant species (Table 1) among depth bins, day vs. night.
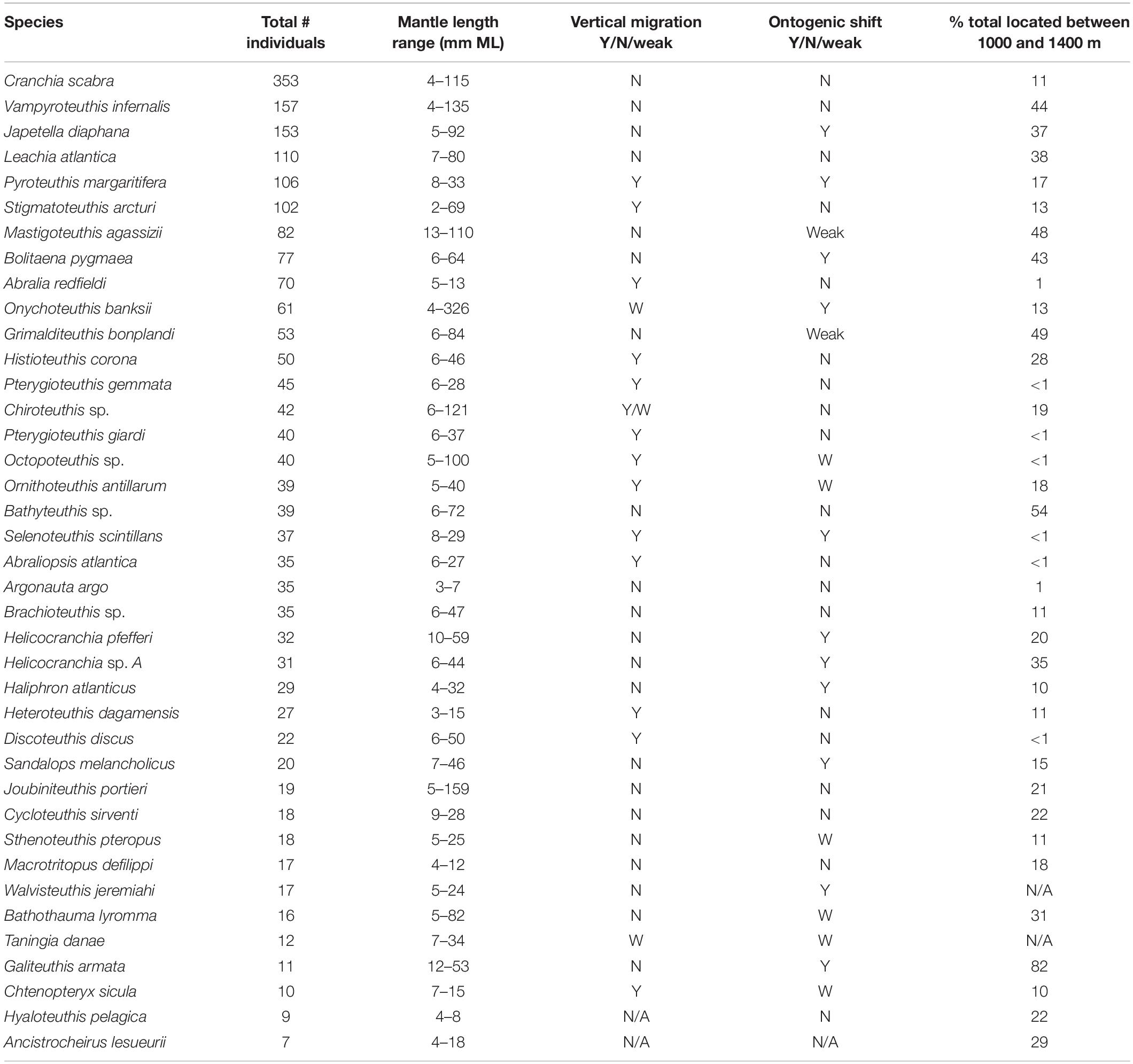
Table 1. Midwater cephalopods ordered by abundance; total individuals examined; mantle length range; evidence of vertical migration; evidence of ontogenic shift; percent of species that was found between 1000 and 1400 m.
The vertical distribution plots (VDP) include 2150 individuals while the ontogenic shift plots (OSP) include 1820 individuals. The numbers vary between the two analyses due to the lack of measurable size (dorsal mantle length) for the ontogenic shift analyses because of collecting damage to some specimens. Plots of vertical migration and ontogenic shifts were created using the R program (Figures 3–10 and Supplementary Materials A, B) (R Core Team, 2013). VDP’s were created for cephalopod species where possible and species were grouped according to their VDP patterns (Figures 3–7 and Supplementary Material A). These groups are based on patterns identified for deep-water fishes of the GOM by T. Sutton (unpublished).
We felt it important to include rare species as Supplementary Material because reports from multiple projects will eventually allow enough published information to accumulate for ecological patterns to be discerned (Vecchione and Pohle, 2002). We arbitrarily chose 20 individuals as an adequate number of individuals to include in VDP and OSP descriptions.
Results
Cephalopods are found throughout the water column with the mesopelagic zone containing more individuals than the epipelagic or the upper bathypelagic zones (Figure 2). Of the 39 species examined for this analysis, 37 species (95%) are found to either live within or move through the 1000–1400 m depth zone (Table 1). Occupancy of this zone varied among species (i.e., 2 of 70 Abralia redfieldi individuals vs. 21 of 39 Bathyteuthis sp. individuals, Table 1).
The DEEPEND team is in the process of analyzing vertical distribution patterns for multiple faunal groups including fishes, crustaceans, gelatinous organisms, and cephalopod, pteropod, and heteropod mollusks1. T. Sutton et al. (unpublished) examined distributional data for oceanic fishes and the results revealed seven major diel vertical patterns for the 151 fish taxa examined. Based on plots of diel vertical distribution, we grouped cephalopods into six of the seven possible vertical-distributional patterns following T. Sutton et al. (unpublished). Although these patterns were visually obvious in the pooled samples presented in the figures, the Kruskal–Wallis analyses of the underlying data failed to reject the null hypothesis of no statistically significant differences in diel patterns in vertical distribution even for the six most abundant species (Table 2). This is likely because of high within-group variance (i.e., variance in abundance among samples within day and night depth bins) not obvious in the pooled figures.
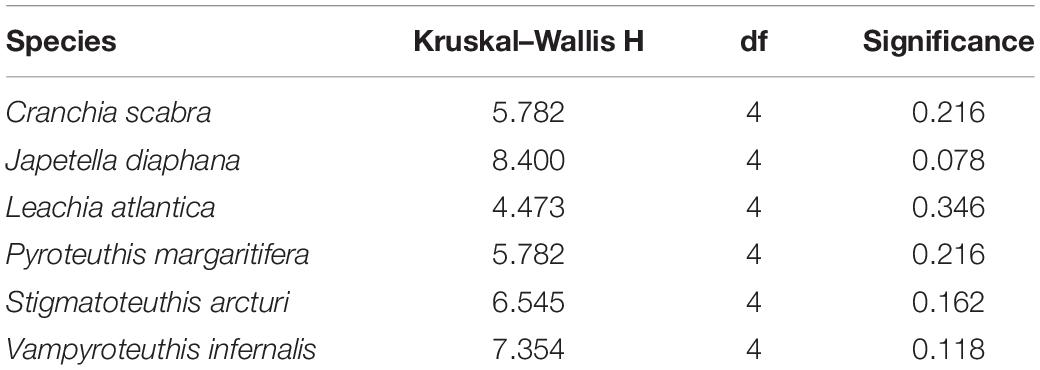
Table 2. Results of Kruskal–Wallis test comparing day/night standardized abundances per species per depth bin.
Based on visual assessment of pooled samples, six species were holoepipelagic non-migrators with centers of abundance the upper 200 m day and night (Figure 3 and Supplementary Material A). The seven nyctoepipelagic synchronous vertically migrating species inhabit the meso- and/or the bathypelagic zones during the day and migrate to the epipelagic zone nightly (Figure 4). The 14 species of mesopelagic asynchronous vertical migrators are found primarily in the mesopelagic during the day and move into or between the epi- or the upper mesopelagic at night (Figures 5, 7 and Supplementary Material A). Chtenopteryx sicula is placed as a mesopelagic non-migrator (Supplementary Material A). There is one species, Joubiniteuthis portieri, that falls into the deep meso-/bathypelagic asynchronous migrator category (Supplementary Material A). The seven deep-meso/bathypelagic non-migrating species are found between 600 and >1000 m depth during the day and night (Figure 6 and Supplementary Material A). Two squid species (Leachia atlantica, Sandalops melancholicus) do not fit into a described pattern and are labeled as “unclassified” here (Figure 7). The nine species with less than 20 individuals are found in the Supplementary Material.
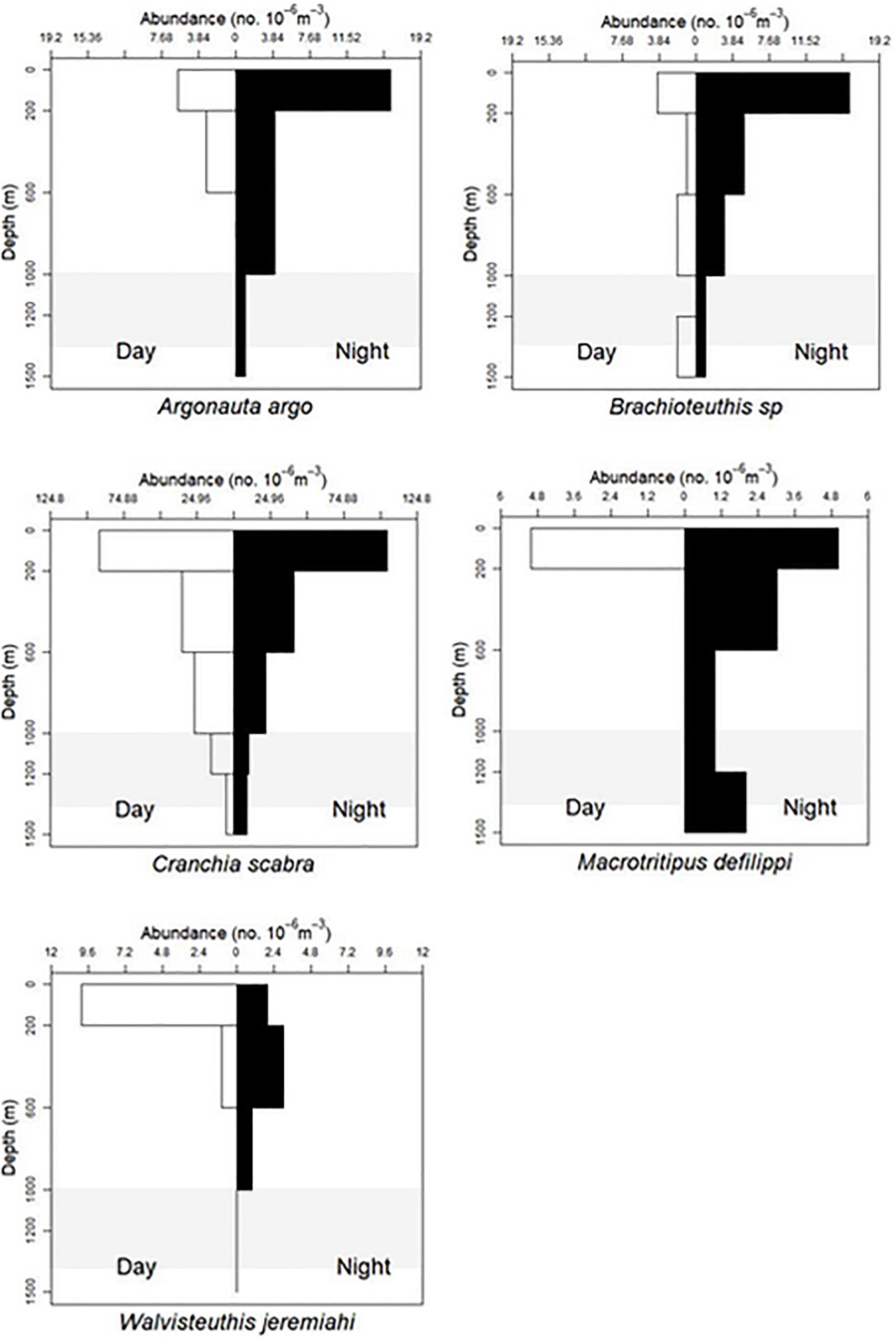
Figure 3. Vertical distribution patterns of five cephalopods. Holoepipelagic non-migration pattern described by T. Sutton et al. (unpublished). A. argo, n = 35; Brachioteuthis sp., n = 35; C. scabra, n = 353; M. defilippi, n = 17; W. jeremiahi, n = 17; shaded region indicates the deep oil plume zone.
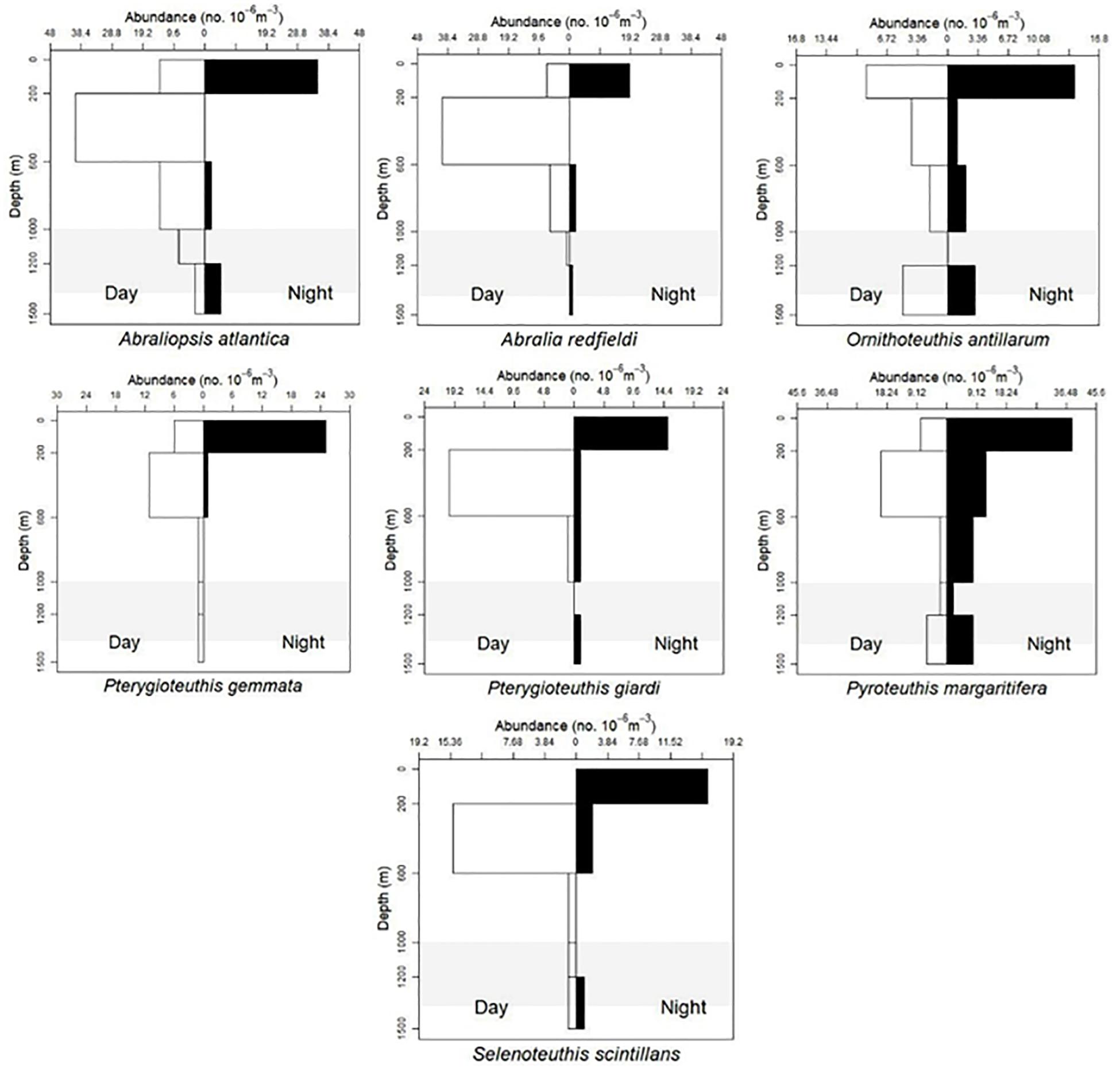
Figure 4. Vertical distribution patterns of seven cephalopods. Nyctoepipelagic synchronous diel migration described by T. Sutton et al. (unpublished). A. atlantica, n = 32; A. redfieldi, n = 70; O. antillarum, n = 39; P. gemmata, n = 45; P. giardi, n = 40; P. margaritifera, n = 106; S. scintillans, n = 37; shaded region indicates the deep oil plume zone.
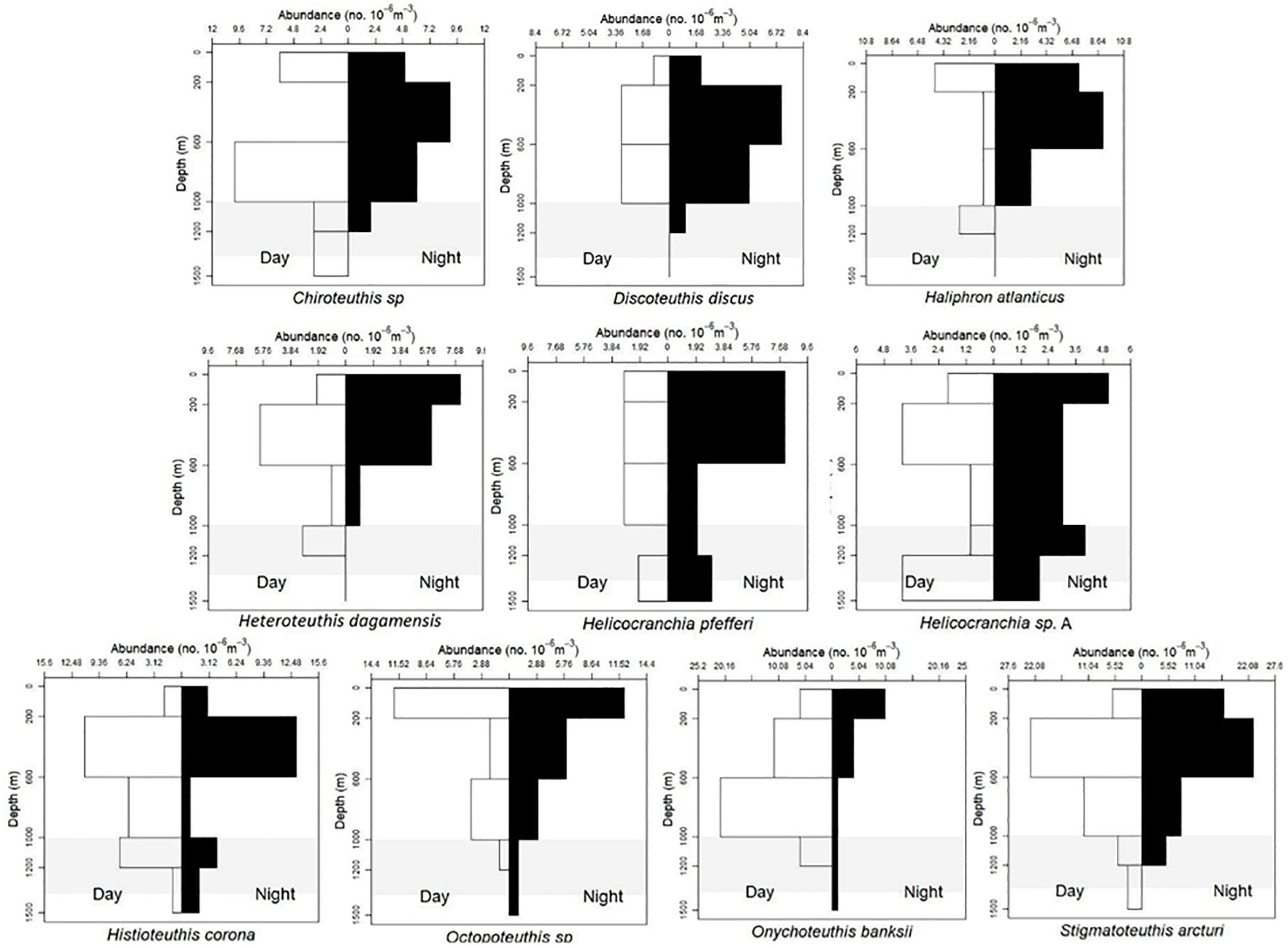
Figure 5. Vertical distribution patterns of 10 cephalopods. Mesopelagic asynchronous migrators as described by T. Sutton et al. (unpublished). Chiroteuthis sp., n = 42; Discoteuthis sp., n = 22; H. atlanticus, n = 29; H. dagamensis, n = 27; Helicocranchia sp. A, n = 31; H. pfefferi, n = 32; H. corona, n = 50; O. banksii, n = 61; S. arcturi, n = 102; S. melancholicus, n = 20; Cycloteuthis sirventi, n = 18; Sthenoteuthis pteropus, n = 18; Octopoteuthis sp., n = 40; shaded region indicates the deep oil plume zone.
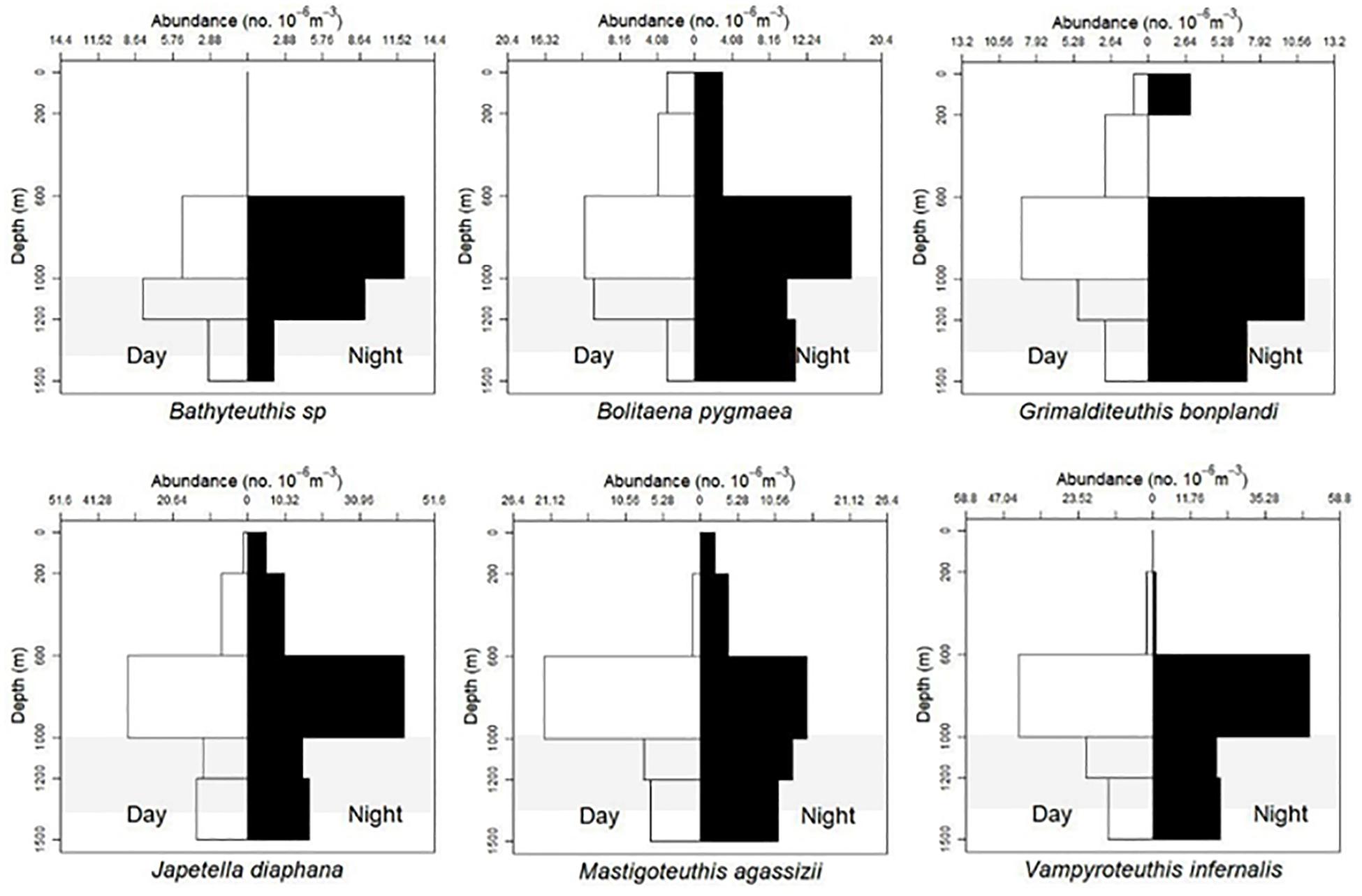
Figure 6. Vertical distribution of six cephalopods. Deep-meso/bathypelagic non-migration as described by T. Sutton et al. (unpublished). Bathyteuthis sp., n = 39; B. pygmaea, n = 77; G. bonplandi, n = 53; J. diaphana, n = 153; M. agassizii, n = 82; V. infernalis, n = 157; shaded region indicates the deep oil plume zone.
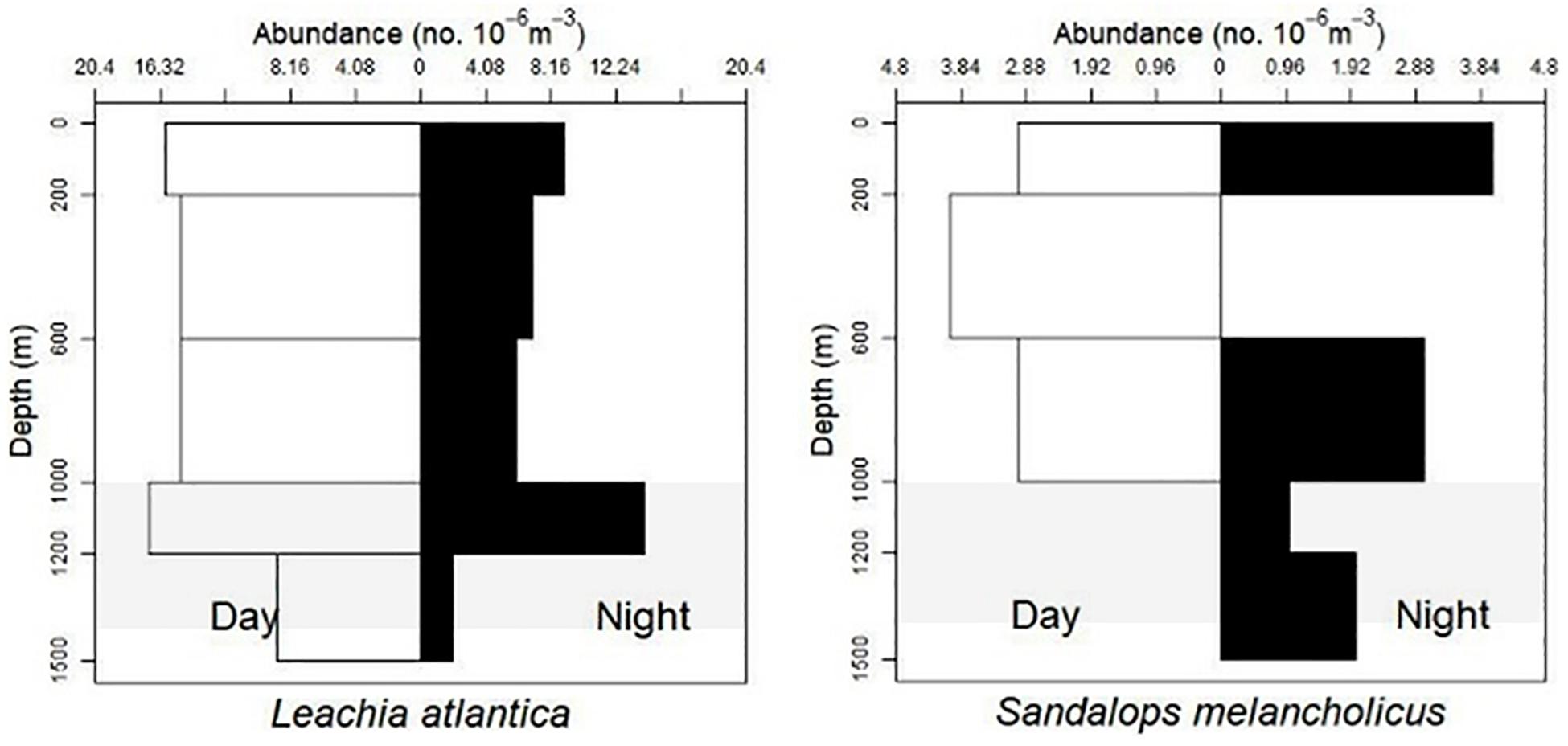
Figure 7. Vertical distribution patterns unclassified. L. atlantica, n = 110; S. melancholicus, n = 20; shaded region indicates the deep oil plume zone.
Ontogenic shift plots’ were created (Figures 8–10 and Supplementary Material B) and patterns reveal that seven cephalopod species descend deeper as they develop (Figure 8). Sixteen species show no evidence of ontogenic shift (Figures 9A,B). There are three species for which it appears that the adults live closer to the surface whereas smaller individuals inhabit a deeper zone (Figure 10). Those OSP’s with less than 20 individuals examined can be found in Supplementary Material B (13 species).
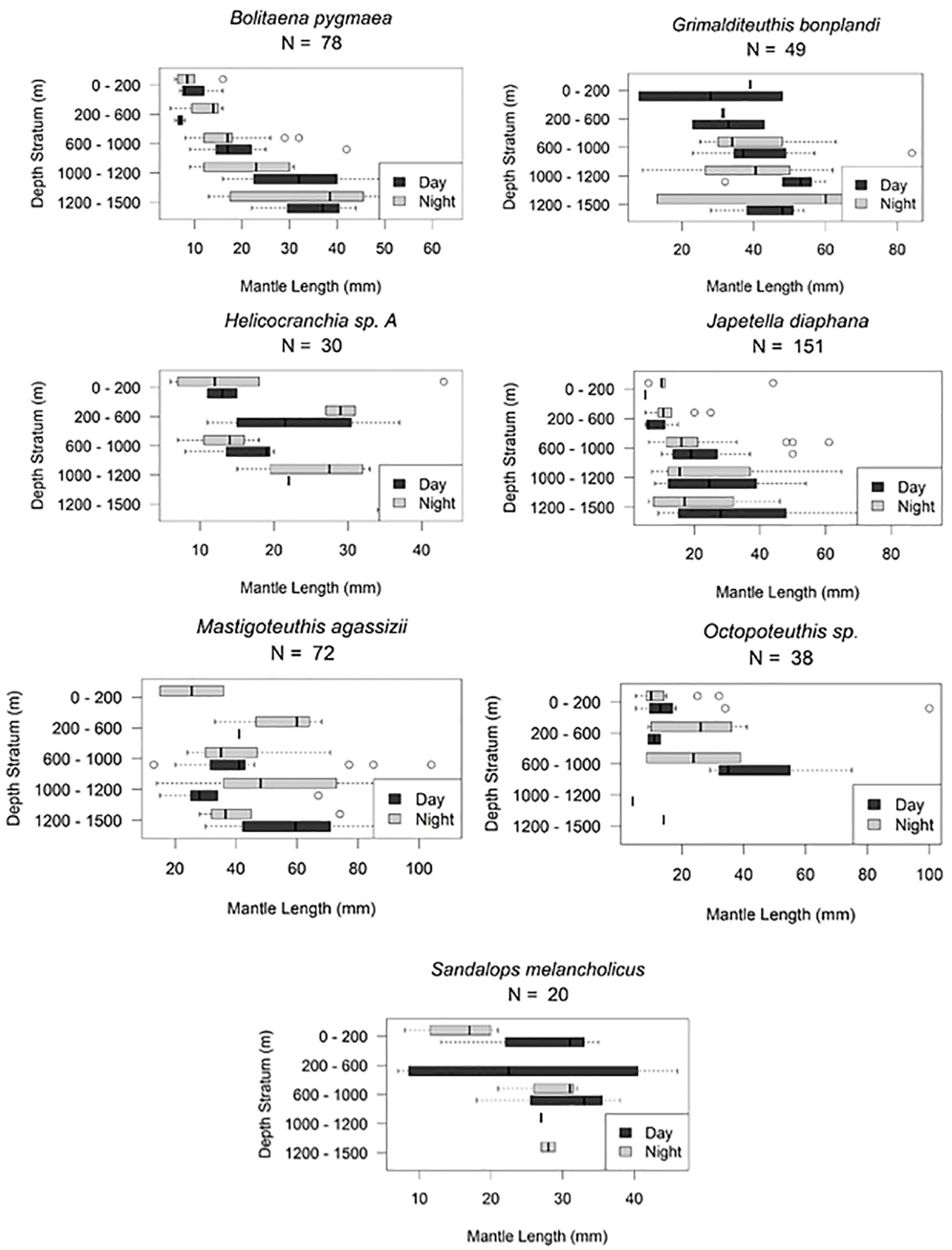
Figure 8. Cephalopod ontogenic shift patterns demonstrating a shift deeper as these species develop. Horizontal line, median; box limits, 1st and 3rd quartiles; circles, outliers.
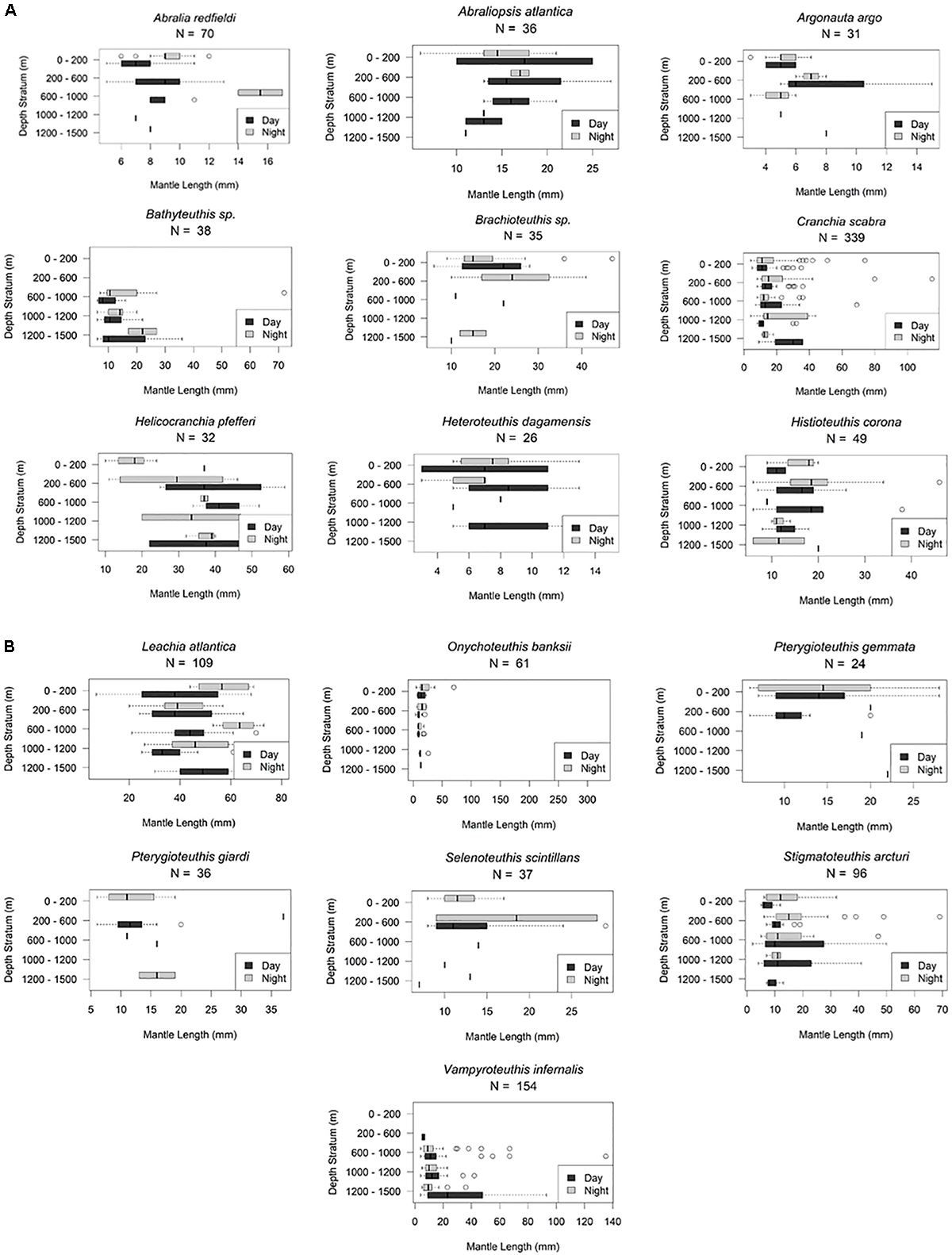
Figure 9. (A,B) Cephalopods showing no evidence of ontogenic shift. Horizontal line, median; box limits, 1st and 3rd quartiles; circles, outliers.
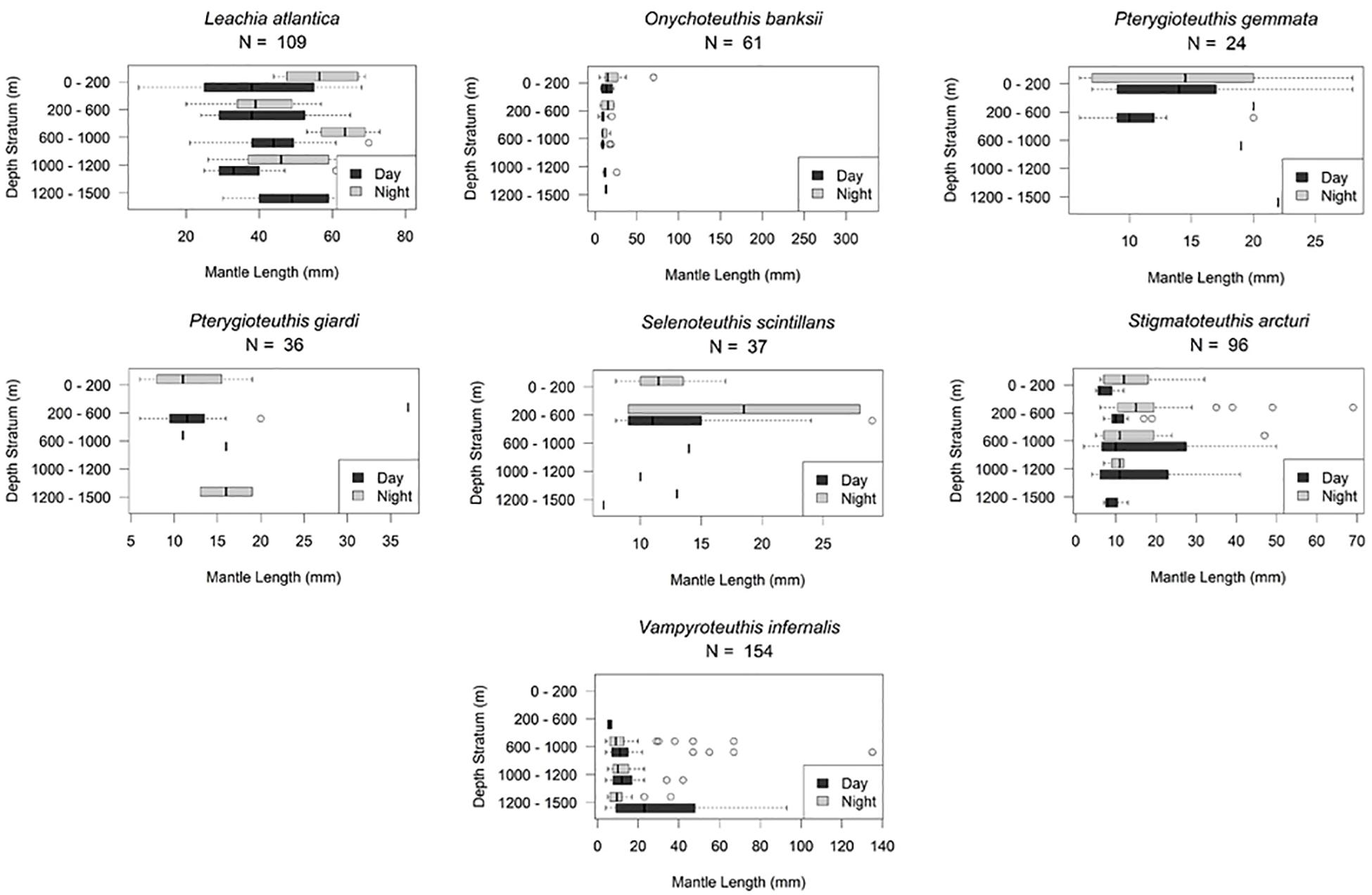
Figure 10. Cephalopods displaying an ontogenic shift from deeper zones to shallow zones as they develop. Horizontal line, median; box limits, 1st and 3rd quartiles; circles, outliers.
Table 1 summarizes the number of individuals included for each VDP, OSP, mantle length (ML) range, whether a species is considered a vertical migrator and the percentage of the species that was found between 1000 and 1400 m. Caution is important when using this analysis because the sample sets in some cases are small. Kruskal–Wallis analyses did not reveal any statistically significant results with regard to distributional differences between day and night even for the six most abundant species (Table 2).
Family Accounts
Vampyroteuthidae
Among 157 Vampyroteuthis infernalis individuals, the majority were below 600 m both day and night (4–135 mm ML). Two individuals (ML = 5 mm, 7 mm) were found between 200 and 600 m. We do not believe that this depth layer is characteristic of juveniles as over 30 individuals of similar MLs found between 600 and 1500 m. Vampires are non-migrators and do not appear to exhibit an ontogenic shift during their life history (Figures 6, 9).
Alloposidae
Twenty-nine Haliphron atlanticus were collected from the surface layer down to 1200 m. Mantle lengths ranged from 4 to 32 mm with no evidence of diel vertical migration. Larger H. atlanticus were found in the upper layers (Figures 5, 10).
Argonautidae
Thirty-five individuals (3–7 mm ML) were examined for the VDP (Figures 3, 9). Nine were collected during day tows and 26 collected at night. Argonauta argo were found in the surface layer both day and night but also down to 1500 m deep. They are classified as holoepipelagic non-migrators here but it should be noted with more sampling, a vertical migration pattern may become apparent. There is no evidence of ontogenic shift.
Octopodidae
Only 5 of 17 Macrotritopus defilippi paralarvae (4–12 mm ML) were collected in day tows at the surface (0–200 m) while during the night tows, 12 specimens were collected from 0 to 1500 m. They appear to be holoepipelagic non-migrators (Figure 3). Additional material needs to be collected to make firm conclusions on vertical migration and any possible ontogenic shift (Supplementary Material B).
Amphitretidae
Seventy-seven Bolitaena pygmaea (6–64 mm ML) are distributed from 0 to 1500 m with no diel migration pattern observed. They do shift to deeper water as they develop with all individuals larger than 20 mm ML caught below 600 m (Figures 6, 8). One hundred fifty-three Japetella diaphana (5–92 mm ML) follow a similar pattern, with no obvious diel migration pattern, and those larger than 26 mm ML living below 600 m, with one exception where a 44 mm ML specimen was collected between 0 and 200 m (Figures 6, 8). For both species, the peak abundance both day and night was in the 600–1000 m depth zone.
Brachioteuthidae
Thirty-five Brachioteuthis sp. (6–47 mm ML) were available for examination of vertical migration and possible ontogenic shifts. Brachioteuthids were found throughout the water column (0–1500 m) during day and night. There is no evidence in this analysis of vertical migration or ontogenic shift (Figures 3, 9).
Chiroteuthidae
Forty-two Chiroteuthis sp. (6–121 mm ML) showed that individuals were caught from 0 to 1500 m during the day and from 0 to 1200 m during the night (Figure 5). There is some evidence of diel vertical migration but plots would need to be broken out by species for further clarification. We found no evidence of ontogenic shift for this genus (Supplementary Material B). Fifty-three Grimalditeuthis bonplandi (6–84 mm ML) were analyzed. They were found from the surface to 1500 m during day and night with the majority of individuals found between 600 and 1500 m. We found no evidence of vertical migration but an ontogenic shift for this species is evident, with larger individuals found in the lower meso- and upper bathypelagic zones (Figures 6, 8).
Joubiniteuthidae
Nineteen J. portieri (5–159 mm ML) documented the species living throughout the water column; six were found in day tows inhabiting the upper and lower mesopelagic zone while thirteen individuals were collected at night from the surface to 1500 m. There is some evidence of vertical migration but no ontogenic shift for this species (Supplementary Materials A, B).
Mastigoteuthidae
The majority of Mastigoteuthis agassizii (13–110 mm ML) were found below 600 m. They are deep meso-/bathypelagic non-migrators. There appears to be a weak ontogenic shift to deeper water as this species becomes larger (Figures 6, 8).
Cranchiidae
Three hundred fifty-three Cranchia scabra (4–115 mm ML) were used for the VDP and the ontogenic shift analysis. They are found distributed between 0 and 1500 m. There is no evidence of vertical migration or ontogenic shift for this species (Figures 3, 9).
Leachia atlantica (7–80 mm ML) demonstrate a non-migration vertical pattern (n = 110) as they are found at all depths (Figure 7). They are currently placed as “unclassified” here. There is no evidence of ontogenic shift (Figures 9A,B).
Thirty-two Helicocranchia pfefferi (10–59 mm ML) were included in the VDP, which showed individuals distributed throughout the water column both day and night with some weak diel vertical migration occurring at the deeper depths (Figure 5). There does not appear to be an ontogenic shift for this species (Figures 9A,B). The VDP and ontogenic shift patterns for a currently undescribed Helicocranchia species, Helicocranchia sp. A (6–44 mm ML) show the same diel vertical distribution pattern but the species does appear to move deeper in the water column as it develops (Figures 5, 8).
The diel vertical distribution of sixteen Bathothauma lyromma (5–82 mm ML) was skewed, as there were 12 collected during day tows but only four individuals captured at night. This species was found throughout the water column during the day and deeper depths (below 600 m) at night. A weak ontogenic shift was noted for this species but more material is required to confirm this pattern (Supplementary Materials A, B).
The distribution of 20 S. melancholicus (7–46 mm ML) encompasses the water column from 0 and 1500 m with no evidence of diel vertical pattern within this small sample set. There appears to be an ontogenic shift with larger animals found at deeper depths (Figures 7, 8).
Eleven Galiteuthis armata (12–53 mm ML) were considered for this analysis. All individuals were found between 600 and 1500 m with no evidence of vertical migration. There appears to be an ontogenic shift by this species (>12 mm ML) but additional material is needed to confirm this (Supplementary Materials A, B).
Cycloteuthidae
Cycloteuthis sirventi (n = 18) are distributed throughout the water column from 0 to 1500 m depth with more collected during night tows than day tows (9–28 mm ML) (Supplementary Materials A, B). Discoteuthis discus (n = 22) was also documented from the surface to 1200 m and is a mesopelagic asynchronous migrator (6–50 mm ML). No ontogenic shift was apparent for either taxon (Figure 5 and Supplementary Material B).
Ancistrocheiridae
Preliminary findings indicate that Ancistrocheirus lesueurii (4–18 mm ML) is found throughout the water column from 0 to 1500 m. Additional records are needed to confirm any pattern of vertical distribution or ontogenic shift as there are only seven specimens available for analysis (Supplementary Materials A, B).
Enoploteuthidae
Thirty-five Abraliopsis atlantica (6–27 mm ML) and 70 A. redfieldi (5–13 mm ML) were plotted and both species are found from 0 to 1500 m. Both are nyctoepipelagic synchronous vertical migrators, living primarily at mesopelagic depths during the day and moving up to the epipelagic zone nightly (Figure 4). However, there is no evidence of ontogenic shift for either species (Figures 9A,B).
Lycoteuthidae
Selenoteuthis scintillans (8–29 mm ML) are nyctoepipelagic synchronous vertical migrators, moving from mainly the upper mesopelagic zone to the epipelagic zone nightly (n = 37). Larger individuals are found in the upper mesopelagic zone (Figures 4, 9).
Pyroteuthidae
One hundred and six Pyroteuthis margaritifera (8–33 mm ML) were distributed throughout the water column (0–1500 m) both day and night but overall they are nyctoepipelagic synchronous migrators, with the majority of the population found in the upper mesopelagic zone during daytime and moving to the epipelagic zone nightly (Figure 4). Smaller individuals were living at depth whereas larger individuals were shallower (Figure 10).
Pterygioteuthis gemmata (6–28 mm ML) were also found mainly in the upper mesopelagic zone in daytime, migrating nightly to the epipelagic zone (Figure 4). Pterygioteuthis giardi (6–37 mm ML) is also a nyctoepipelagic synchronous migrator (Figure 4). Neither of these species appear to undergo an ontogenic shift during development (Figures 9A,B) within the limits of our methods.
Histioteuthidae
Histioteuthis corona (6–46 mm ML) and Stigmatoteuthis arcturi (2–69 mm ML) are found throughout the water column with high concentrations found in the upper mesopelagic zone. They are mesopelagic asynchronous migrators, living primarily from the lower to upper mesopelagic zone at night. We found no evidence of ontogenic shift for either species (Figures 5, 9).
Octopoteuthidae
The two Octopoteuthis species (O. sicula, O. megaptera) found in the northern GOM were combined into one VDP due to the difficulty of identifying specimens confidently to species because many were badly damaged (5–100 mm ML). Forty Octopoteuthis sp. display a mesopelagic asynchronous migration pattern (Figures 5, 8).
Twelve Taningia danae (7–34 mm ML) were included in a VDP (Supplementary Materials A, B) as there is little distribution information in past literature about this species. They were caught from 0 to 1000 m deep with the majority living above 600 m, and indicated a weak vertical migration pattern. Additional material is needed to confirm this assessment (Supplementary Materials A, B).
Ommastrephidae
Ornithoteuthis antillarum (5–40 mm ML) follows the nyctoepipelagic synchronous diel migration pattern with some vertical migration from the meso- to the epipelagic zone. Smaller individuals were found throughout the water column (<15 mm ML) while larger individuals (>23 mm ML) were above 600 m (Figures 4, 10). This could be evidence of a weak ontogenic shift for this species moving upwards as they get larger. Eighteen Sthenoteuthis pteropus (5–25 mm ML) display a uniform distribution from 0–1500 m (Supplementary Material A) with a weak ontogenic shift (Supplementary Material B). We include a VDP for Hyaloteuthis pelagica (4–8 mm ML) in spite of its rarity because of scarce historical records. Nine specimens show a vertical distribution from 0 to 1200 m. No ontogenic pattern is documented at this time (Supplementary Materials A, B).
Onychoteuthidae
Sixty-one Onychoteuthis banksii (4–326 mm ML) showed that the species is found from the surface to 1500 m, with more collected during daytime hours than at night. We found evidence of a mesopelagic asynchronous migration for this species (Figure 5). There is no strong evidence of ontogenic shift as this species grows (Figures 9A,B). Walvisteuthis jeremiahi (5–24 mm ML) were documented from 0 to 1000 m as holoepipelagic non-migrators with a similar ontogenic shift pattern (Supplementary Materials A, B).
Sepiolidae
Heteroteuthis dagamensis (3–15 mm ML) are found from 0 to 1200 m in the northern GOM with evidence of the species being mesopelagic asynchronous vertical migrators. Six larger individuals (>9 mm ML) were found above 600 m while only one was found in the 1000–1200 m depth zone. There is no evidence of ontogenic shift (Figures 5, 9).
Bathyteuthidae
Thirty-nine Bathyteuthis sp. (6–72 mm ML) were used to create a VDP that shows they live solely in the lower meso- and upper bathypelagic zones at all sizes. They are considered deep meso-/bathypelagic non-vertical migrators with no evidence of ontogenic shift (Figures 6, 9).
Chtenopterygidae
Although only 10 specimens of C. sicula (7–15 mm ML) were collected, a VDP is included due to the scarcity of available literature. They occupy depths from the surface to 1200 m with many inhabiting the upper mesopelagic zone. From the plot, they appear to move down the water column at night but this is likely a product of a low sample size (Supplementary Material A). They also exhibit a weak ontogenic shift, as they get larger, they move deeper into the water column (Supplementary Material B). More material is needed for a robust assessment.
Discussion
The DWH spill was an ecological disaster from the surface to the seafloor. The midwater plume lasted for months (Camilli et al., 2010; Melvin et al., 2016) and numerous taxa interacted with it as they either lived within it or moved vertically through it on their nightly migrations (Burdett et al., 2017; Romero et al., 2018). Multiple faunal groups are found within the upper bathypelagic zone including fishes, cephalopods, crustaceans, and gelatinous organisms (Pond et al., 2000; Robison et al., 2010; Sutton et al., 2010; Letessier et al., 2011; Cook et al., 2013; Judkins et al., 2016; Hosia et al., 2017). All of these taxa play a role in the carbon flux from the surface to benthic habitats. This biological carbon pump becomes vulnerable as oil drilling moves farther off the coast into deeper waters. When another spill occurs, knowing the migrating and non-migrating populations that may interact with any contamination will provide a baseline of sorts for species that may be impacted in the upper bathypelagic zone.
We found that 95% of oceanic cephalopod species of the northern GOM spend time in the upper bathypelagic zone for some portion of their lives (1000–1500 m), either migrating through or living within it (Figure 2 and Table 3). Past records support these 37 cephalopod species having been collected from similar depths in various regions around the world (Lu and Clarke, 1975a; Roper and Young, 1975; Young, 1978; Lu and Roper, 1979; Vecchione and Pohle, 2002; Shea et al., 2017). Although the Kruskal–Wallis results reveal no significance for any of the six species examined, this is most likely due to small sample sizes and large within-group variation. Erickson et al. (2017) showed that sampling variability is very high in young pelagic cephalopods. Large sample sizes are needed for a robust statistical analysis.
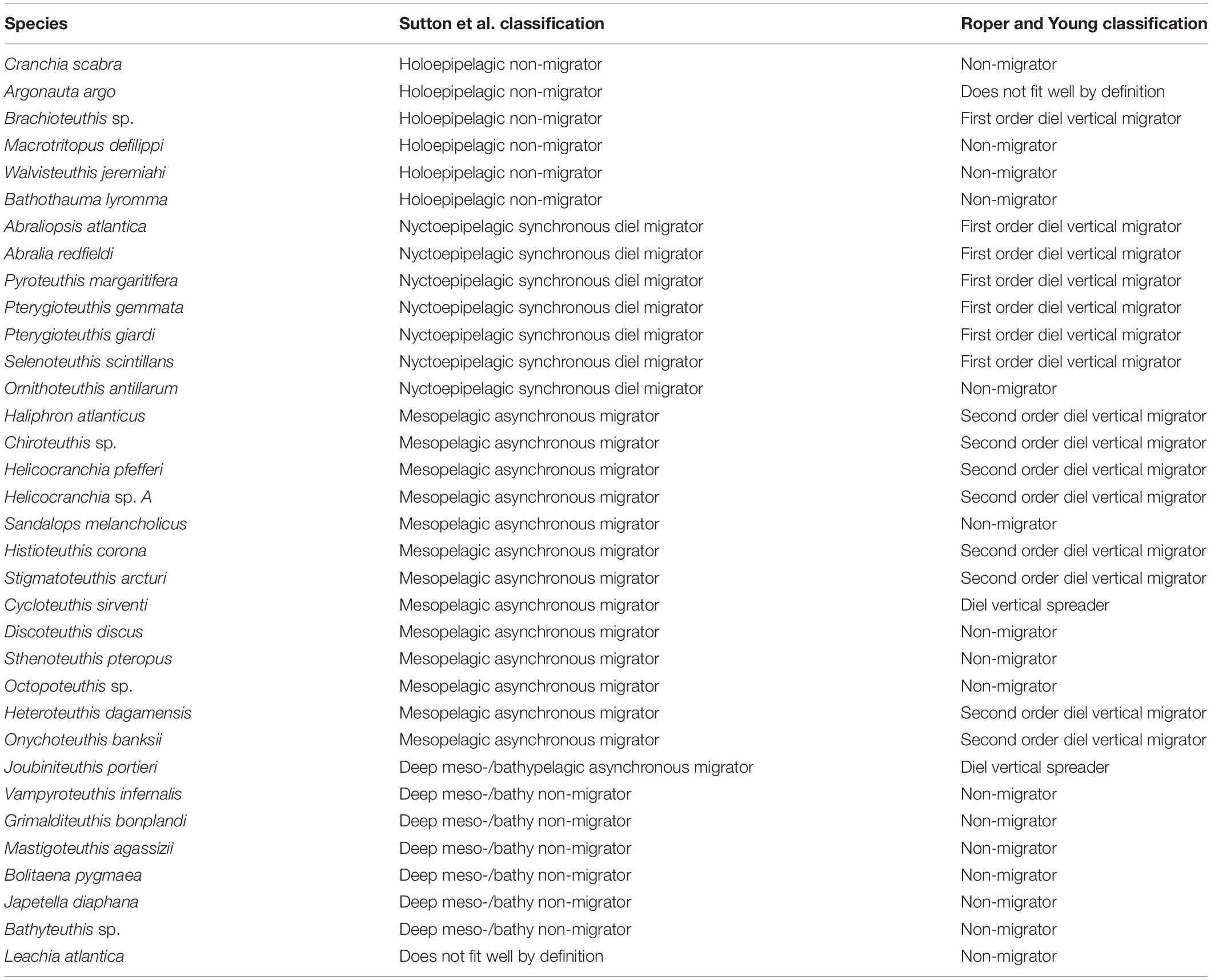
Table 3. Current study species and our placement of cephalopods within the vertical migration pattern categories according to T. Sutton et al. (unpublished) and Roper and Young (1975).
Cephalopods are important links among various components of marine ecosystems. The contribution of nektonic squids to the coupling of energy flows among marine ecosystems during their ontogenic migrations is poorly understood (Arkhipkin, 2013). It is often neglected in ecosystem models despite their capacity to move significant amounts of resources between ecosystems (Arkhipkin, 2013). The vertically migrating squids contribute to this resource flux throughout the water column.
The bathypelagic zone is part of the largest unexplored realm on Earth (Webb et al., 2010). There have been multiple programs to close the gap of deep-sea exploration such as MAR-ECO and CMarZ, both supported by the Census of Marine Life as well as the DEEPEND consortium supported by the Gulf of Mexico Research Initiative (GoMRI). These programs have scratched the surface of the bathypelagic zone which continues to require focused, collaborative sampling efforts. The dataset created here is currently the largest of its kind for midwater cephalopods.
Vertical Migration Patterns
Past studies of cephalopod vertical migration have been conducted using a modified Isaac-Kid midwater trawl (IKMT) or rectangular midwater trawls (RMT 8) (Lu and Clarke, 1975b; Lu and Roper, 1979; Salman et al., 2003; Shea and Vecchione, 2010). One caution using these types of nets, fished either open or with a closing cod-end, is the possibility of organisms being captured during descent or ascent to the desired discreet depth. The RMT8 and MOC10 are opening/closing net systems, which can descend closed to the desired depth and then open, so we have higher confidence in inferred vertical distribution patterns using this type of gear. Roper and Young (1975) examined three programs that documented cephalopod vertical migration patterns using IKMTs. They created categories for the cephalopods based on the vertical migration patterns noting that these are not mutually exclusive; some species may exhibit a combination of patterns and that all patterns are not sharply defined (Roper and Young, 1975).
Many of the plots in the current study reinforce the patterns defined by Roper and Young (1975). Some plots (i.e., P. margaritifera, Octopoteuthis sp., O. banksii, H. pfefferi, A. argo) extend the known depth range for the species. This is likely due to our deeper trawling efforts. The current study found that while some cephalopod species fit within the Roper and Young (1975) categories, others did not because there was not a clear pattern or few individuals included in their original analysis (Table 4). We used the categories created by T. Sutton et al. (unpublished) to compare with those of Roper and Young (1975) as they align better with our observations regarding cephalopods. The categories are more specific by depth zones than those reported by Roper and Young (1975) used due to the capability of the MOC10 to collect discreet depth zone samples in this study (Table 4). Examples of this can be found when looking at certain species (i.e., S. melancholicus, D. discus, S. pteropus) as Roper and Young (1975) classified them as non-migrators but the patterns exhibited with the current plots demonstrate a nightly upward movement into the upper mesopelagic or epipelagic which places these species into the asynchronous migrator category.
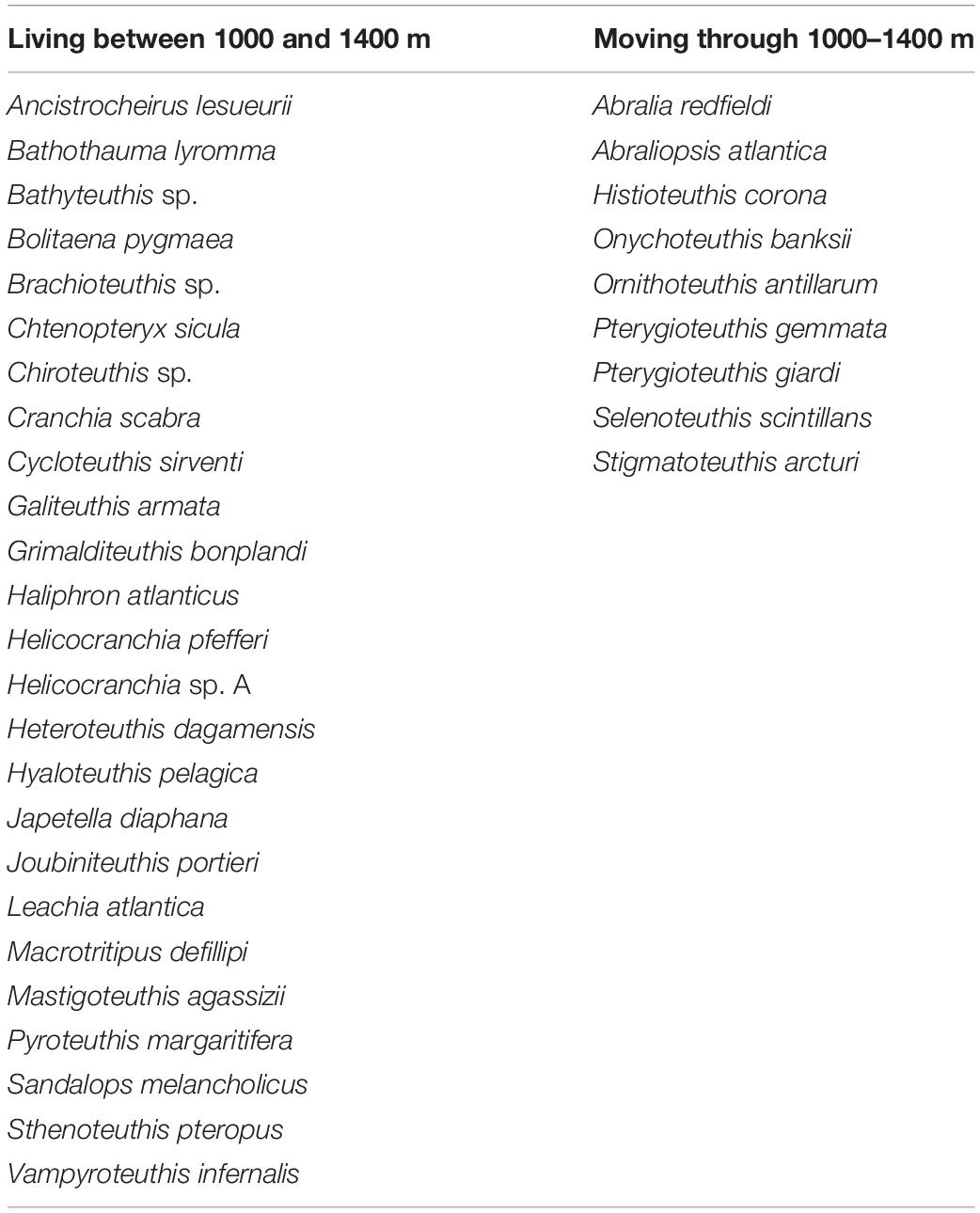
Table 4. Cephalopod species found living between (10% or more of abundance) or moving through 1000–1400 m.
It should be noted that some groups need additional material to strengthen the patterns inferred here (i.e., Chiroteuthis, Brachioteuthis, and Octopoteuthis) because they are grouped by genus and not compared at the species level. As more material becomes available, clearer conclusions can be made.
Ontogenic Shifts
Past studies have focused on ontogenic shifts of various cephalopod groups, notably the ommastrephids (Shigeno et al., 2001; Shea, 2005). Ontogenic examination of deep-water cephalopods is less available; some work has been presented by Villanueva (1992), Quetglas et al. (2010), and Shea and Vecchione (2010). Developmental strategies such as anti-predator behavior changes through ontogeny have been examined as well (York and Bartol, 2016).
Shea and Vecchione (2010) examined paralarvae of three mesopelagic cephalopod species from the North Atlantic Ocean and compared allometric changes with ontogenic changes in their diel vertical migration patterns. They found that the brachioteuthids were caught primarily during the day while we recorded the opposite pattern (Figure 3). We examined 35 brachioteuthids ranging in size from 6 to 47 mm ML and found them in the upper 600 m during the day and from 0 to 1500 m at night. This aligns with a holoepipelagic vertical distribution pattern where the majority of the species spends time in the upper 200 m but can be found deeper as well. There does not appear to be an ontogenic shift within the specimens we examined. These findings do not align closely with what Shea and Vecchione (2010) report but this could be due to differences in sampling depths, specimen sizes, or species considered (Figures 3, 9).
Shea and Vecchione (2010) also documented that C. sicula paralarvae were found between 0 and 300 m when <6.5 mm ML as well as allometric changes that occur once they are below the euphotic zone. We find that C. sicula (7–15 mm ML) are found from 0 to 600 m during the day and from 0 to 1200 m at night. This could align with Shea and Vecchiones’ (2010) thought that there is an ecological change that corresponds with allometric change for this group. However, note that our study has only 10 specimens to examine for this comparison (Supplementary Materials A, B).
The ontogenic migration of Histioteuthis reversa to deeper waters has been observed in the Mediterranean Sea (Quetglas et al., 2010). They concluded that adult females ascend the water column to spawn because spent females have been collected at the surface, as reported elsewhere (Voss et al., 1998). The two histioteuthid species studied here (H. corona and S. arcturi) were found throughout the water column when smaller than 20 mm ML and the larger animals (>20 mm ML) were found at shallower depths both day and night indicating that the pattern inferred for H. reversa may be consistent for the family.
Conclusion
Cephalopods are widely distributed throughout the water column down to 1500 m with the mesopelagic zone containing the largest number of individuals. This study reveals that 95% of the species examined spent all or part of their lives in the upper bathypelagic zone which is where the deep oil plume was located during the DWH oil spill. This study provides new details, as there were no baseline data for midwater cephalopods of the GOM prior to the spill. The VDP and ontogenic shifts analyzed with a reliable collection method contribute additional evidence and include large sample sizes for many species, providing reference data for midwater cephalopods in the GOM.
Data Availability Statement
The datasets generated for this study are available on request to the corresponding author.
Ethics Statement
Ethical review and approval was not required for the animal study because this is not required for cephalopods in the United States at this time.
Author Contributions
HJ and MV were responsible for cephalopod collection and identification as well as writing and reviewing the manuscript.
Conflict of Interest
The authors declare that the research was conducted in the absence of any commercial or financial relationships that could be construed as a potential conflict of interest.
Funding
This research was made possible by a grant from the Gulf of Mexico Research Initiative. GOM data are publicly available through the Gulf of Mexico Research Initiative Information and Data Cooperative (GRIIDC) at https://data.gulfresearchinitiative.org, doi: 10.7266/N70P0X3T and doi: 10.7266/N7XP7385.
Acknowledgments
We would like to thank the crews of the NOAA Ship Pisces and the R/V Point Sur during the sampling process. We thank Tracey Sutton for the use of his vertical migration pattern definitions he is creating for fishes. We would also like to acknowledge April Cook and Rosanna Milligan for their assistance with data and graphs.
Supplementary Material
The Supplementary Material for this article can be found online at: https://www.frontiersin.org/articles/10.3389/fmars.2020.00047/full#supplementary-material
MATERIAL A | Cephalopod vertical distribution plots for species with fewer than 20 individuals for analysis.
MATERIAL B | Ontogenic shift plots of cephalopods with fewer than 20 individuals for analysis.
Footnotes
References
Arkhipkin, A. (2013). Squid as nutrient vectors linking South-west Atlantic marine ecosystems. Deep Sea Res. II 95, 7–20. doi: 10.1016/j.dsr2.2012.07.003
Burdett, E. A., Fine, C. D., Sutton, T. T., Cook, A. C., and Frank, T. M. (2017). Geographic and depth distributions, ontogeny, and reproductive seasonality of decapod shrimps (Caridea: Oplophoridae) from the northeastern Gulf of Mexico. Bull. Mar. Sci. 93, 743–767. doi: 10.5343/bms.2016.1083
Camilli, R., Reddy, C. M., Yoerger, D. R., Van Mooy, B. A. S., Jakuba, M. V., Kinsey, J. C., et al. (2010). Tracking hydrocarbon plume transport and biodegradation at deepwater horizon. Science 330, 201–204. doi: 10.1126/science.1195223
Clarke, M. R., and Lu, C. C. (1975). Vertical distribution of cephalopods at 18°N 25°W in the North Atlantic. J. Mar. Biol. Assoc. U.K. 55, 165–182. doi: 10.1017/s0025315400015812
Cook, A. B., Sutton, T. T., Galbraith, J. K., and Vecchione, M. (2013). Deep-pelagic (0-3000m) fish assemblage structure over the Mid-Atlantic Ridge in the area of the Charlie-Gibbs Fracture Zone. Deep Sea Res. II 98, 279–291. doi: 10.1016/j.dsr2.2012.09.003
DEEPEND, (2015). DEEPEND Cruise Report DP01. Available at: http://www.deependconsortium.org/images/documents/DP01_report.pdf (accessed October 4, 2019).
Dierks, A. R., Highsmith, R. C., Asper, V. L., Joung, D., Zhou, Z., Guo, L., et al. (2010). Characterization of subsurface polycyclic aromatic hydrocarbons at the Deepwater Horizon site. Geophys. Res. Lett. 37:L20602. doi: 10.1029/2010GL045046
Erickson, C. A., Roper, C. F. E., and Vecchione, M. (2017). Variability of paralarval-squid occurrence in meter-net tows from east of Florida, USA. Southeastern Naturalist. 16, 629–642. doi: 10.1656/058.016.0411
Hopkins, T. L. (1982). The vertical distribution of zooplankton in the eastern Gulf of Mexico. Deep Sea Res. I 29, 1069–1083. doi: 10.1016/0198-0149(82)90028-0
Hosia, A., Falkenhaug, T., Baxter, E. J., and Pagès, F. (2017). Abundance, distribution and diversity of gelatinous predators along the northern Mid-Atlantic Ridge: a comparison of different sampling methodologies. PLoS One 12:e0187491. doi: 10.1371/journal.pone.0187491
Joye, S. B., MacDonald, I. R., Leifer, I., and Asper, V. (2011). Magnitude and oxidation potential of hydrocarbon gases released from the BP oil well blowout. Nat. Geosci. 4, 160–164. doi: 10.1038/ngeo1067
Judkins, H., Vecchione, M., Cook, A., and Sutton, T. (2016). Diversity of midwater cephalopods in the northern Gulf of Mexico: comparison of two collecting methods. Mar. Biodivers. 47, 647–657. doi: 10.1007/s12526-016-0597-598
Kessler, J. D., Valentine, D. L., Redmond, M. C., Du, M., Chan, E. W., Mendes, S. D., et al. (2011). A persistent oxygen anomaly reveals the fate of spilled methane in the deep Gulf of Mexico. Science 331, 312–315. doi: 10.1126/science.1199697
Lansdell, M., and Young, H. (2003). Pelagic cephalopods from eastern Australia: species composition, horizontal and vertical distribution determined from the diets of pelagic fishes. Rev. Fish. Biol. Fish. 17, 125–138. doi: 10.1007/s11160-006-9024-8
Letessier, T. B., Falkenhaug, T., Debes, H., Bergstad, O. A., and Brierly, A. S. (2011). Abundance patterns and species assemblages of euphausiids associated with the Mid-Atlantic Ridge. North Atlantic. J. Plank. Res. 33, 1510–1525. doi: 10.1093/plankt/fbr056
Lu, C. C., and Clarke, M. R. (1975a). Vertical distribution of cephalopods at 11°N 20°W in the North Atlantic. J. Mar. Biol. Assoc. U.K. 55, 369–389.
Lu, C. C., and Clarke, M. R. (1975b). Vertical distribution of cephalopods at 40°N 53°W in the North Atlantic. J. Mar. Biol. Assoc. U.K. 55, 143–163. doi: 10.1017/s0025315400015800
Lu, C. C., and Roper, C. F. E. (1979). Cephalopods from Deepwater Dumpsite 106 (Western Atlantic): Vertical Distribution and Seasonal Abundance. Smithsonian Contributions to Zoology; #288. Washington, DC: Smithsonian Institution Press.
Melvin, A. T., Thibodeaux, L. J., Parsons, A. R., Overton, E., Valsaraj, K. T., and Nandakumar, K. (2016). Oil-material fractionation in Gulf deep water horizontal intrusion later: field data analysis with chemodynamic fate model for Macondo 252 oil spill. Mar. Pol. Bull. 105, 110–119. doi: 10.1016/j.marpolbul.2016.02.043
Nesis, K. (1972). Oceanic cephalopods of the Peru Current: horizontal and vertical distribution. Okeanologiya 12, 506–519.
Nesis, K. (1993). Vertical migration of the micronektonic squids Pyroteuthis margaritifera (Ruppell) and Pterygioteuthis gemmata (Chun) from catches with a non-closing net. Okeanologiya 33, 110–115.
Passow, U., and Hetland, R. D. (2016). What happened to all of the oil? Oceanography 29, 88–95. doi: 10.5670/oceanog.2016.73
Pond, D. W., Sargent, J. R., Fallick, A. E., Allen, C., Bell, M. V., and Dixon, D. R. (2000). δ13C values of lipids from phototropic zone microplankton and bathypelagic shrimps at the Azores sector of the Mid-Atlantic Ridge. Deep Sea Res. I 47, 121–136. doi: 10.1016/s0967-0637(99)00050-3
Quetglas, A., de Mesa, A., Ordines, F., and Grau, A. (2010). Life history of the deep-sea cephalopod family Histioteuthidae in the western Mediterranean. Deep sea Res. I 57, 999–1008. doi: 10.1016/j.dsr.2010.04.008
R Core Team (2013). R: A Language and Environment for Statistical Computing. Vienna: R Foundation for Statistical Computing.
Reddy, C. M., Arey, J. S., Seewald, J. S., Sylva, S. P., Lemkau, K. L., Nelson, R. K., et al. (2012). Composition and fate of gas and oil released to the water column during the Deepwater Horizon oil spill. Proc. Matl. Acad. Sci. U.S.A. 109, 20229–20234. doi: 10.1073/pnas.1101242108
Reddy, R. K., Rao, A., Yu, Z., Wu, X., Nandakumar, K., Thibodeaus, L., et al. (2014). Challenges in and Approaches to Modeling the Complexities of Deepwataer Oil and Gas Release. Oil Spill Remediation: Colloid Chemistry-Based Principles and Solutions, 1st Edn, Chap. 4. Hoboken, NJ: John Wiley & Sons, Inc, 89–126.
Richards, T. M., Gipson, E. E., Cook, A., Sutton, T. T., and Wells, R. J. D. (2018). Trophic ecology of meso- and bathypelagic predatory fishes in the Gulf of Mexico. ICES J. Mar. Sci. 76, 662–672. doi: 10.1093/icesjms/fsy074
Robison, B. (2004). Deep pelagic biology. J. Exp. Mar. Bio. Ecol. 300, 253–272. doi: 10.1016/j.jembe.2004.01.012
Robison, B. H., Reisbichler, K. R., and Sherlock, R. E. (2017). The coevolution of midwater research and ROV technology at MBARI. Oceanography 30, 26–37. doi: 10.5670/oceanog.2017.421
Robison, B. H., Sherlock, R. E., and Reisenbichler, K. R. (2010). The bathypelagic community of Monterey Canyon. Deep Sea Res. II 57, 1551–1556. doi: 10.1016/j.dsr2.2010.02.021
Romero, I. C., Schwing, P. T., Brooks, G. R., Larson, R. A., Hastings, D. W., Ellis, G., et al. (2015). Hydrocarbons in deep-sea sediments following the 2010 Deepwater Horizon blowout in the northeast Gulf of Mexico. PLoS One 10:e0128371. doi: 10.137/journal.pone.0128371
Romero, I. C., Sutton, T., Carr, B., Quintana-Rizzo, E., Ross, S. W., Hollander, D. J., et al. (2018). Mesopelagic fishes from the Gulf of Mexico reveals exposure to oil-derived sources. Environ. Sci. Tech. 52, 10985–10996. doi: 10.1021/acs.est.8b02243
Roper, C. F. E., and Young, R. E. (1975). Vertical distribution of pelagic cephalopods; Smithsonian Contributions to Zoology, #209. Washington, DC: Smithsonian Institution Press.
Ropke, A., Nellen, W., and Piatkowski, U. (1993). A comparative study on the influence of the pycnocline on the vertical distribution of fish larvae and cephalopod paralarvae in three ecologically different areas of the Arabian Sea. Deep Sea Res. II 40, 801–819. doi: 10.1016/0967-0645(93)90059-v
Salman, A., Katagan, T., and Benli, H. A. (2003). Vertical distribution and abundance of juvenile cephalopods in the Aegean Sea. Sci. Mar. 67, 167–176. doi: 10.3989/scimar.2003.67n2167
Shea, E., Judkins, H., Staudinger, M. D., Hartigan, V., Lindgren, A., and Vecchione, M. (2017). Cephalopod biodiversity in the vicinity of Bear Seamount, western North Atlantic. Mar. Biodivers. 47:699. doi: 10.1007/s12526-017-0633-633
Shea, E. K. (2005). Ontongeny of the fused tentacles in three species of Ommastrephid squids (Cephalopoda, Ommastrephidae). Invert. Biol. 124, 25–38. doi: 10.1111/j.1744-7410.2005.1241-04.x
Shea, E. K., and Vecchione, M. (2010). Ontogenic changes in diel vertical migration patterns compared with known allometric changes in three mesopelagic squid species suggest an expanded definition of paralarva. ICES J. Mar. Sci. 67, 1436–1443. doi: 10.1093/icesjms/fsq104
Shigeno, S., Kidokoro, H., Goto, T., Tsuchiya, K., and Segawa, S. (2001). Early ontogeny of the Japanese Common squid Todarodes pacificus (Cephalopoda, Ommastrephidae) with special reference to its characteristic morphology and ecological significance. Zool. Sci. 18, 1011–1026. doi: 10.2108/zsj.18.1011
Socolofsky, S. A., Adams, E. E., and Sherwood, C. R. (2011). Formation dynamics of subsurface hydrocarbon intrusions following the Deepwater Horizon blowout. Geophys. Res. Let. 38:L09602. doi: 10.1029/2011GL047174
Sosnowski, A. (2017). Genetic Identification and Population Characteristics of Deep-Sea Cephalopod Species in the Gulf of Mexico and Northwestern Atlantic Ocean. Master’s Thesis, University of South Florida, Florida.
Summers, W. C. (1983). Physiological and trophic ecology of cephalopods. The Mollusca. Ecology 6, 261–279. doi: 10.1016/b978-0-12-751406-2.50013-1
Sutton, T. T. (2013). Vertical ecology of the pelagic ocean: classical patterns and new perspectives. J. Fish. Biol. 83, 1508–1527. doi: 10.1111/jfb.12263
Sutton, T. T., Wiebe, P. H., Madin, L., and Bucklin, A. (2010). Diversity and community structure of pelagic fishes to 5000 m depth in the Sargasso Sea. Deep Sea Res. II 57, 2220–2233. doi: 10.1016/j.dsr2.2010.09.024
Timm, L., Bracken- Grissom, H., Sosnowski, A., Breitbart, M., Vecchione, M., and Judkins, H. (2020). Population genomics of three deep-sea cephalopod species reveals connectivity between the Gulf of Mexico and northwestern Atlantic Ocean. Deep Sea Res. I (in press). doi: 10.1016/j.dsr.2020.103222
Vecchione, M., and Pohle, G. (2002). Midwater cephalopods in the western North Atlantic Ocean off Nova Scotia. Bull. Mar. Sci. 71, 883–892.
Vecchione, M., Young, R. E., Guerra, A., Lindsay, D. J., Clague, D. A., Bernhad, J. M., et al. (2001). Worldwide observations of remarkable deep-sea squids. Science 294:2505. doi: 10.1126/science.294.5551.2505
Villanueva, R. (1992). Deep-sea cephalopods of the northwestern Mediterranean: implications of up-slope ontogenetic migration in two bathybenthic species. J. Zool. Lond. 227, 267–276. doi: 10.1111/j.1469-7998.1992.tb04822.x
Voss, N. A., Nesis, K. N., and Rodhouse, P. G. (1998). “The cephalopod family Histioteuthidae (Oegopsida): systematics, biology, and biogeography,” in Systematics and Biogeography of Cephalopods, eds N. A. Voss, M. Vecchione, R. B. Toll, and M. Sweeney, (Washington D.C: Smithsonian Contributions to Zoology).
Webb, T. J., Vanden Berghe, E., and O’Dor, R. (2010). Biodiversity’s “big wet secret” the global distribution of marine biological records reveals chronic under-exploration of the deep pelagic ocean. PLoS One 5:e10223. doi: 10.1371/journal.pone.0010223
Widder, E., Robison, B. E., Reisenbichler, K., and Haddock, S. (2005). Using red light for in situ observations of deep-sea fishes. Deep Sea Res. I 52, 2077–2085. doi: 10.1016/j.dsr.2005.06.007
Wiebe, P. H., Burt, K. H., Boyd, S. H., and Morton, A. W. (1976). A multiple opening/closing net and environmental sensing system for sapling zooplankton. J. Mar. Res. 34:326.
Xavier, J. C., Cherel, Y., Allcock, L., Rosa, R., Sabirov, R. M., and Blicher, M. E. (2018). A review on the biodiversity, distribution, and trophic roles of cephalopods in the Arctic and Antarctic marine ecosystems under a changing ocean. Mar. Biol. 165:93. doi: 10.1007/s00227-018-3352-3359
York, C. A., and Bartol, I. K. (2016). Anti-predatory behavior of squid throughout ontogeny. J. Exp. Mar. Biol. Ecol. 480, 26–35. doi: 10.1016/j.jembe.2016.03.011
Young, R. E. (1978). Vertical distribution and photosensitive vesicles of pelagic cephalopods from Hawaiian waters. Fish. Bull. 76, 583–614.
Young, R. E., Vecchione, M., and Mangold, K. M. (1922–2003). 2019. Cephalopoda Cuvier 1797. Octopods, Squids, Nautiluses, etc. Available at: http://tolweb.org/Cephalopoda/19386/2019.03.26 (accessed March 26, 2019).
Keywords: squid, octopod, vertical migration, DEEPEND, Deepwater Horizon, deep-sea, development
Citation: Judkins H and Vecchione M (2020) Vertical Distribution Patterns of Cephalopods in the Northern Gulf of Mexico. Front. Mar. Sci. 7:47. doi: 10.3389/fmars.2020.00047
Received: 17 August 2019; Accepted: 24 January 2020;
Published: 21 February 2020.
Edited by:
Jose Angel Alvarez Perez, Universidade do Vale do Itajaí, BrazilReviewed by:
Helena Passeri Lavrado, Federal University of Rio de Janeiro, BrazilRichard Schwarz, GEOMAR Helmholtz Centre for Ocean Research Kiel, Germany
Copyright © 2020 Judkins and Vecchione. This is an open-access article distributed under the terms of the Creative Commons Attribution License (CC BY). The use, distribution or reproduction in other forums is permitted, provided the original author(s) and the copyright owner(s) are credited and that the original publication in this journal is cited, in accordance with accepted academic practice. No use, distribution or reproduction is permitted which does not comply with these terms.
*Correspondence: Heather Judkins, SnVka2luc0BtYWlsLnVzZi5lZHU=