- 1The UWA Oceans Institute, The University of Western Australia, Crawley, WA, Australia
- 2Instituto de Biociências de Botucatu, Universidade Estadual Paulista, Botucatu, Brazil
- 3School of Science and Centre for Marine Ecosystems Research, Edith Cowan University, Joondalup, WA, Australia
- 4Ocean Vision Environmental Research, Fremantle, WA, Australia
- 5Red Sea Research Center, King Abdullah University of Science and Technology, Thuwal, Saudi Arabia
Estimates of organic carbon (Corg) storage by seagrass meadows which consider inter-habitat variability are essential to understand their potential to sequester carbon dioxide (CO2) and derive robust global and regional estimates of blue carbon storage. In this study, we provide baseline estimates of seagrass extent, and soil Corg stocks and accumulation rates from different seagrass habitats at Rottnest Island (in Amphibolis spp., Posidonia spp., Halophila ovalis, and mixed Posidonia/Amphibolis spp. meadows). The Corg stocks in 0.5 m thick seagrass soil deposits, derived from 24 cores, were 5.1 ± 0.7 kg Corg m–2 (mean ± SE, ranging from 0.05 to 12.9 kg Corg m–2), accumulating at 23.2 ± 3.2 g Corg m–2 year–1 (ranging from 0.22 to 58.9 g Corg m–2 year–1) over the last decades. There were significant differences in Corg content (%) and stocks (mg Corg cm–3), stable carbon isotope composition of the soil organic matter (δ13C), and soil grain size among the seagrass meadows studied, highlighting that biotic and abiotic factors influence seagrass soil Corg storage. Mixed meadows of Posidonia/Amphibolis spp. and monospecific meadows of Posidonia spp. and Amphibolis spp. had the highest Corg stocks (ranging from 6.2 to 6.4 kg Corg m–2), while Halophila spp. meadows had the lowest Corg stocks (1.2 ± 0.6 kg Corg m–2). We estimated a total soil Corg stock of 48.1 ± 8.5 Gg Corg beneath the 755 ha of Rottnest Island’s seagrasses, and a Corg sequestration capacity of 0.81 ± 0.06 Gg Corg year–1, which is equivalent to the sequestration of ∼22% of the island’s current annual CO2 emissions. Our results contribute to the existing global dataset on seagrass soil Corg storage and show a significant potential of seagrass to sequester CO2, which are particularly relevant in the context of achieving carbon neutrality through conservation actions in environmentally-marketed, tourist destinations such as Rottnest Island.
Introduction
The carbon storage capacity of seagrass has been recognized since the early 1980s (e.g., Smith, 1981) but interest has recently intensified with the recognition of blue carbon ecosystems and their potential to contribute to climate change mitigation (Duarte et al., 2005, 2013; Nellemann and Corcoran, 2009; Mcleod et al., 2011; Fourqurean et al., 2012a). While occupying only 0.1% of the ocean surface, seagrass ecosystems have been estimated to bury 27–44 Tg organic carbon (Corg) year–1 globally, accounting for 10–18% of the total carbon burial in the oceans, and have soil Corg stocks comparable to those of temperate and tropical forests, mangroves, and tidal marshes (Duarte et al., 2005; Fourqurean et al., 2012a). However, there has been a trend to estimate seagrass Corg storage potential from a limited dataset, based largely on the Corg content of superficial soils and a limited range of seagrass habitats (Fourqurean et al., 2012a; Lavery et al., 2013).
Seagrasses comprise a wide variety of species across a range of depositional environments (Carruthers et al., 2007), and the variability in the sedimentary Corg stocks among seagrass habitats had been found to be high (up to 18-fold; Lavery et al., 2013). The seagrass itself may exert a primary control on Corg storage through its biomass, productivity, and nutrient content (Mateo et al., 1997; Lavery et al., 2013; Miyajima et al., 2015). In addition, both autochthonous (e.g., plant detritus and epiphytes) and allochthonous (e.g., seston and terrestrial matter) sources contribute to the Corg pool in seagrass soils (Hendriks et al., 2008; Kennedy et al., 2010). Moreover, once Corg is buried in the soil, biotic and abiotic factors are likely to control the degree of Corg accumulation and preservation (Mateo et al., 2006). These factors include the rates of sediment accumulation, sediment grain-size, and biochemical composition of the organic matter (Keil and Hedges, 1993; Torbatinejad et al., 2007; Serrano et al., 2016a), and also vary among seagrass meadows (De Falco et al., 2000; Kennedy et al., 2010). As such, considerable variation exists in the estimates of Corg storage in seagrass soils worldwide (ranging from 4.2 to 8.4 Pg Corg; Fourqurean et al., 2012a) and for any given location (Lavery et al., 2013; Campbell et al., 2015; Röhr et al., 2016). In order to improve existing estimates of Corg storage in seagrass ecosystems, further studies that expand the current knowledge on geomorphological and biological factors driving Corg storage are needed (Serrano et al., 2016a; Gullström et al., 2018; Mazarrasa et al., 2018).
The recent focus on carbon trading provides the opportunity to avoid or mitigate CO2 emissions through the conservation and restoration of seagrass meadows, which rank among the most endangered habitats in terms of global loss rates. Despite recent studies showed that seagrass extent remained stable or increased since 2000s (Carmen et al., 2019), seagrass losses have been estimated at 29% of their global extent since 1880 (Waycott et al., 2009), largely resulting from coastal eutrophication and mechanical disturbance (Short and Wyllie-Echeverria, 1996; Orth et al., 2006). Australia has one of the largest areas of seagrass worldwide (Carruthers et al., 2007) but over the last decades has experienced severe seagrass loss (e.g., Cambridge and McComb, 1984; Arias-Ortiz et al., 2018), even in relatively pristine environments such as in Rottnest Island, Western Australia, a tourism destination but where the deployment of permanent moorings led to fragmented meadows (Walker et al., 1989; Hastings et al., 1995) and CO2 emissions from seagrass soil Corg stocks (Serrano et al., 2016d).
While there is considerable interest in bringing seagrasses and other blue carbon ecosystems into national accounting and mitigation frameworks, there is also significant interest at a more local scale, with local or regional governments, or even private companies, often exploring the potential to become carbon neutral or offset their carbon emissions (e.g., Gössling, 2009). Therefore, Rottnest Island represents an important target area due to its large seagrass meadows and management strategies to mitigate climate change (RIA, 2018). Rottnest Island Authority (RIA) aims to implement actions to reduce greenhouse gas emissions and to investigate offset plans through revegetation programs (RIA, 2018); hence, the results from this study would be useful to guide potential carbon sequestration strategies in Rottnest Island. As with larger scale assessments, the known variability in seagrass carbon stocks requires that the area and the stocks of the different habitats are accounted for when making assessments.
Here we present the results of an extensive survey of seagrass meadows along Rottnest Island (Perth, Western Australia), which included habitat mapping, to produce regional estimates of soil Corg stocks and sequestration rates, and also to provide insights into the contribution of seagrass conservation to achieve a carbon neutral policy in the Island. While this study focuses on one region, the results obtained contribute to understand the differences in Corg storage between seagrass species, highlighting the importance of accounting for habitat variability when scaling up estimates. Finally, to place our results within a broader context, we compare these data to estimates from global datasets, emphasizing recognized variation across seagrass habitats.
Materials and Methods
Study Site, Sampling, and Laboratory Procedures
Rottnest Island is a marine reserve located 19 km off the coast of Perth (Western Australia, 32°00′07″ S, 115°31′01″ E), surrounded by extensive sub-tidal seagrass ecosystems generally found in sheltered bays and areas protected by reefs (Wells et al., 1993; RIA, 2015; Figure 1). Nine species of seagrass have been recorded at Rottnest Island, the dominant species belonging to the genera Posidonia and Amphibolis (Wells et al., 1993). Posidonia sinuosa, Posidonia australis, Amphibolis antarctica and Amphibolis griffithii all form mono-specific meadows and are considered to be “climax” species (Lavery and Vanderklift, 2002), but can also be found in mixed meadows (Kendrick et al., 2000; Carruthers et al., 2007).
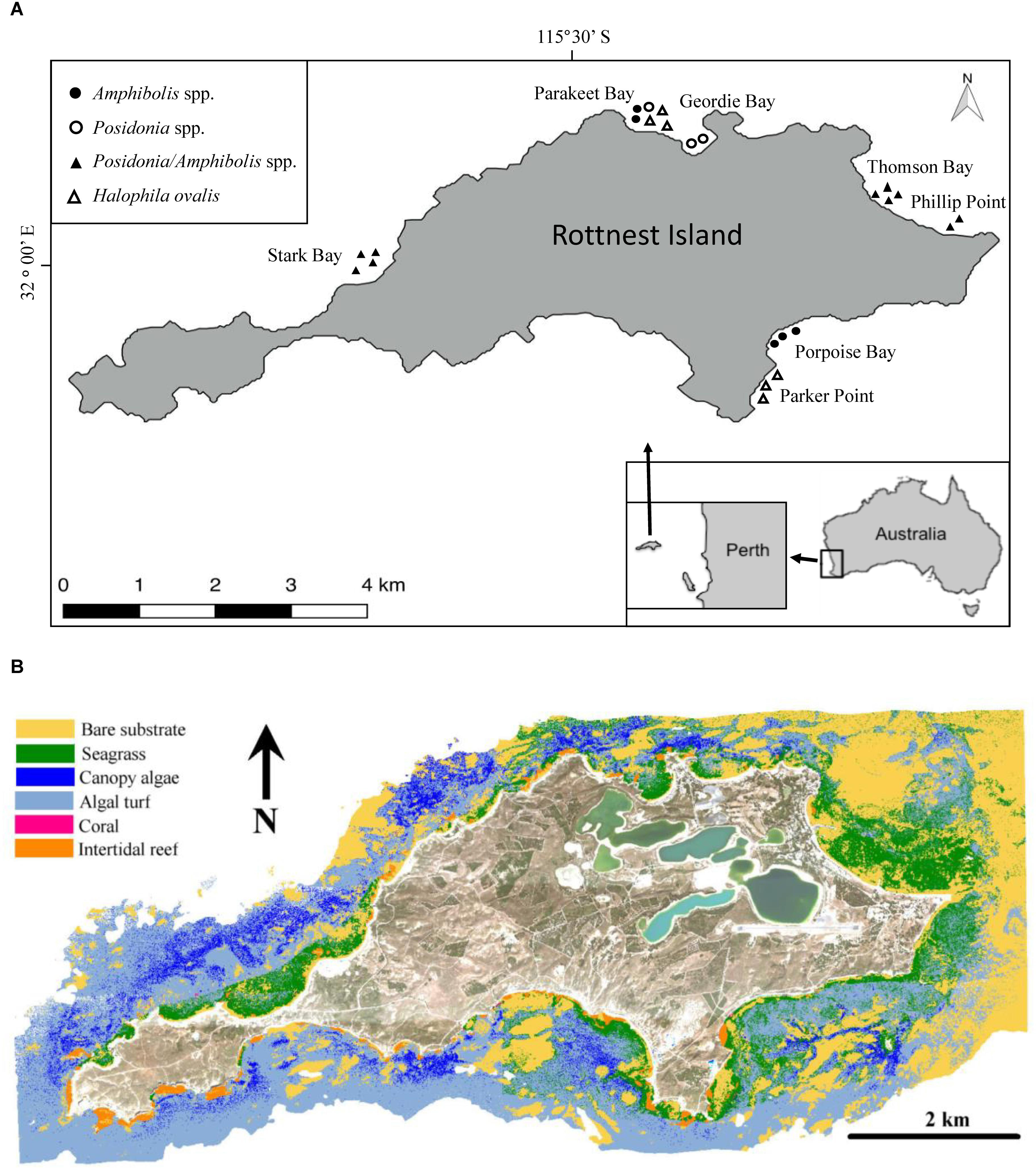
Figure 1. (A) Rottnest Island is located 19 km off the coast of Perth, Western Australia. The symbols depict the coring locations and the species composition of the meadows sampled. (B) Benthic habitat map for Rottnest Island using three HyMap flight lines and limited to regions <15 m water depth. Seagrass habitats represents Posidonia spp. and Amphibolis spp. meadows. Macroalgae habitats represent those dominated by Ecklonia radiata and Sargassum spp.
To assess seagrass soil Corg stocks and accumulation rates, in 2014 we sampled soil cores (N = 24) from extensive seagrass meadows comprising mono-specific meadows of Halophila ovalis (6 cores), mixed meadows of P. australis and P. sinuosa (3 cores), mixed meadows of A. antarctica and A. griffithii (5 cores), and mixed Posidonia spp. and Amphibolis spp. meadows (10 cores) along the north and southeast shores of the island (i.e., Thomson Bay, Stark Bay, Phillip Point, Porpoise Bay, Parakeet Bay, Parker Point, and Geordie Bay; Figure 1 and Supplementary Tables 1). The sites were chosen to be representative of seagrass meadows from a variety of habitats, including differences in biotic (e.g., species composition) and abiotic (e.g., hydrodynamic energy) settings. The cores were sampled within extensive seagrass meadows, and meadow edges were located > 50 m away in all cases.
Undisturbed soil cores were randomly sampled within continuous meadows using PVC pipes (70 mm in diameter, 75 cm long) that were gently hammered into the seafloor at 2–4 m water depth while being rotated to assist with penetration. The corers were sealed before retrieval (i.e., using lids and PVC tape) to avoid losing the unconsolidated sediments contained within the corers. Following retrieval, the core samples were cut into 1 cm thick slices. Each slice was weighed before and after oven drying at 60°C until constant weight [dry weight (DW)] to estimate the dry bulk density (DBD). Then, every second slice was divided into two subsamples by quartering. One subsample was milled and analyzed for Corg and stable carbon isotope composition (δ13C), while the other subsample was used for sediment grain-size analyses.
For Corg and δ13C analyses, 1 g of ground sample was acidified with HCl 4% until bubbling stopped to remove inorganic carbon, centrifuged (5 min at 3,400 r/min), and the supernatant removed carefully by pipette. Then, the sample was washed with Milli-Q water, centrifuged, and the supernatant removed. The residual samples were re-dried and then encapsulated for Corg analysis using a Micro Cube elemental analyzer (Elementar Analysensysteme GmbH, Hanau, Germany) interfaced to a PDZ Europa 20-20 isotope ratio mass spectrometer (Sercon Ltd., Cheshire, United Kingdom) at the University of California Davis. Carbon isotope ratios are expressed as δ values in parts per thousand (‰) relative to the Vienna PeeDee Belemnite. Replicate assays and standards indicated measurement errors of 0.01% for Corg content and 0.06‰ for δ13C. Content of Corg was calculated for bulk (pre-acidified) samples.
For sediment grain-size analysis, a Mastersizer 2000 laser-diffraction particle analyzer was used following digestion of the samples with 10% hydrogen peroxide to remove organic matter. Sediments were classified as coarse sand (<1 and >0.5 mm) medium sand (<0.5 and >0.25 mm), fine sand (<0.25 and > 0.125 mm), and very fine sand plus mud (<0.125 mm), according to a scale adapted from Brown and McLachlan (2010).
Data Analyses
The length of core barrel inserted into the soil and the length of retrieved soil were recorded in order to correct the core lengths for compression effects. The corrected core lengths ranged from 22 to 68 cm, and all variables studied here are referred to the corrected, uncompressed depths. Corg density (g Corg cm–3) was calculated for each soil depth in each core by multiplying the DBD (g cm–3) by the Corg concentration (%). For soil depths where Corg content (%) was not analyzed, we extrapolated the %Corg (i.e., by averaging the %Corg between above and below depths) and multiplied the %Corg by the DBD to obtain Corg density (g Corg cm–3). To allow direct comparisons among sites, the standing stocks per unit area (cumulative stocks; kg Corg m–2) were standardized to 50 cm thick deposits, which involved extrapolating linearly integrated values of cumulative Corg stocks with depth in 12 out of the 24 cores sampled (i.e., from 30 to 50 cm; r2 = 0.98, P < 0.001; Supplementary Figures 1). Accumulation rates of Corg were estimated by dividing the inventories in the 50 cm thick soils by the mean soil accretion rate estimated for seagrass meadows at Rottnest Island (i.e., based on 210Pb dating) by Serrano et al. (2016d).
The proportion of autochthonous and allochthonous Corg in the seagrass soil Corg pool was estimated using Stable Isotope Mixing Models in R (“simmr” and “rjags” packages; Parnell et al., 2010). The average δ13C values within the top 50 cm of each core were analyzed for the probability of relative organic matter contribution to soil stocks using a one-isotope three-source mixing model (Zencich et al., 2002; Phillips and Gregg, 2003). The δ13C signatures of potential autochthonous and allochthonous Corg sources (mean ± SD; −10.8 ± 1.6‰ for seagrass, −17.2 ± 1.1‰ for epiphytes plus macroalgae and microphytobenthos, and −24.2 ± 0.6‰ for seston) were obtained from Waite et al. (2007), Ricart et al. (2015), and Serrano et al. (2016c).
All statistical analyses were performed using univariate general linear mixed model (GLMM) procedures in SPSS v. 14.0. GLMMs were used to accommodate the potential non-independence of samples taken at different depths within the same core, since depth is a proxy for time in the cores, and the unbalanced sampling design. The GLMMs were performed to test for significant effects of species composition (Amphibolis spp., Posidonia spp., H. ovalis, and Posidonia/Amphibolis spp.) on DBD (g cm–3), Corg concentration (%), Corg content (mg cm–3), δ13C signatures, and sediments < 0.125 mm. Species composition and soil depth (cm) were treated as fixed factors, and study site (Thomson Bay, Stark Bay, Phillip Point, Porpoise Bay, Parakeet Bay, Parker Point, and Geordie Bay) was treated as a random factor (probably distribution: normal; link function: identity). A separate GLMM was run to test the effect of species composition (fixed factor) on cumulative Corg stocks (kg Corg m–2). All response variables (DBD, Corg concentration, content and stock, δ13C signatures, and sediment grain size fractions) were square-root transformed prior to analyses and had homogenous variances. Normality and homoscedasticity of model residuals were determined by visual estimation. Pearson correlation analysis was used to test for significant relationships among the variables studied.
Estimates of Seagrass Area and Corg Storage at Rottnest Island
Three flight lines of HyMap hyperspectral data were flown over Rottnest Island by HyVista Corporation in April 2004 with a ground resolution of 3.5 m. The data were corrected to remove sun-glitter and the influence of the atmosphere and water column, using the Modular Inversion and Processing System (MIPS; Heege and Fischer, 2004; Pinnel, 2007). MIPS uses a physically based process to extract information from the data on the water constituents, bathymetry, bottom cover type, and bottom reflectance, with no external inputs. The hyperspectral data were corrected using three generic bottom cover types: bare sediment, and light and dark submerged aquatic vegetation represented by spectral signatures extracted directly from the image.
The output of MIPS provides the spatial distribution of bare sediment and vegetated regions < 15 m depth within the Rottnest Island Marine Park boundaries. The vegetated regions were further classified to identify seagrass meadows and macroalgae using a hierarchical spectral separation classification algorithm in conjunction with a library of spectral reflectance signatures, measured in situ, describing the dominant benthic habitat components for Rottnest Island (Harvey, 2009). The classification algorithm calculated the probability of each benthic habitat component (e.g., Posidonia spp.) being the dominant component for each image pixel and assigning the pixel to the component with the highest probability. The results of this classification required an additional step using the abiotic variables of mean sea level and wave exposure to systematically correct areas misclassified as seagrass as either intertidal reef (areas above mean sea level) or macroalgae (areas highly exposed to wave action). The seagrass meadows were further classified to those dominated by Posidonia spp. or Amphibolis spp., and macroalgae habitats were classified into those dominated by Ecklonia radiata or Sargassum spp. It should be noted it was not possible to identify Halophila spp. meadows using the data obtained due to its sparse growth and size, which means its spectral reflectance at a pixel level is dominated by the bare substrate. The extent of each habitat type was calculated using the number of pixels multiplied by the area of each pixel. Regional seagrass soil Corg stocks and accumulation rates at Rottnest Island were estimated considering inter-species variability, by multiplying the average Corg stocks and accumulation rates of Posidonia spp., Amphibolis spp., and mixed Posidonia/Amphibolis spp. by their respective extents around the Island.
Results
The total area of seagrass within the Rottnest Island Marine Park was estimated to be 755 ha, with Posidonia spp. occupying 113 ha, Amphibolis spp. 257 ha, and mixed Posidonia/Amphibolis spp. meadows 385 ha. The sediments under seagrass meadows at Rottnest Island were mainly composed of medium and fine sands (70–80% of DW). The DBD ranged from 0.5 to 2.1 g cm–3, while the Corg content (%) of the soils ranged from 0.05 to 3.8% and the δ13C signatures of soil organic matter ranged from −8.6 to −24.5‰. The soil Corg stocks and accumulation rates (in 50 cm thick deposits) across the 24 meadows studied ranged 200-fold from 0.05 to 12.9 kg Corg m–2 and 0.22 to 58.9 kg Corg m–2 year–1 (mean ± SE, 5.1 ± 0.7 kg Corg m–2 and 23.2 ± 3.2 g Corg m–2 year–1, respectively; Table 1).
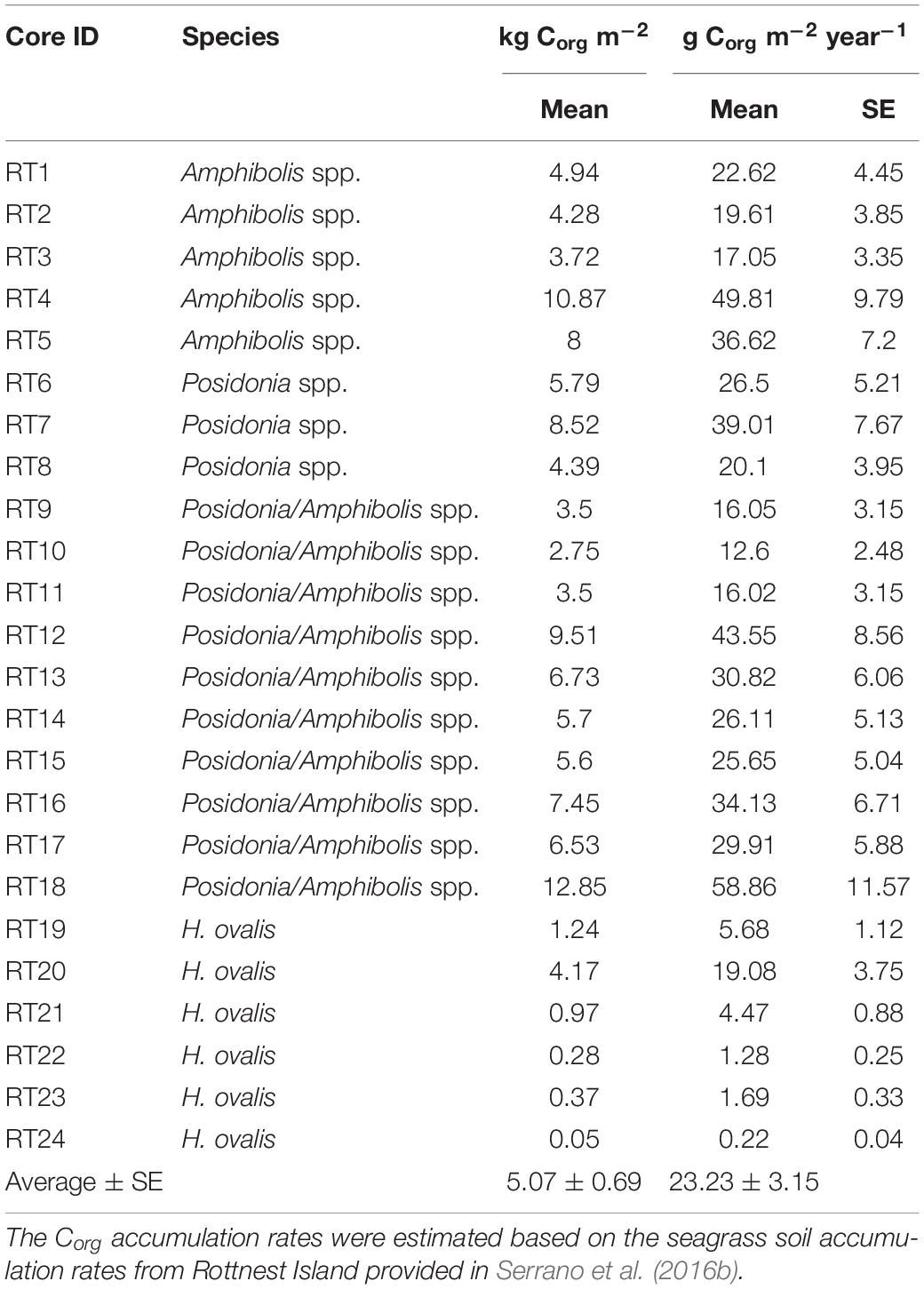
Table 1. Cumulative organic carbon (Corg) stocks [kg Corg m–2; mean ± standard error (SE)] and accumulation rates (g Corg m–2 year–1) in 50 cm thick soil deposit in seagrass meadows at Rottnest Island, Western Australia.
The DBD, proportion of sediment particles < 0.125 mm, and δ13C signatures increased with soil depth within the top 10 cm of Amphibolis spp. and H. ovalis cores, and were relatively constant or declined in the Posidonia spp. and mixed Posidonia/Amphibolis cores (Supplementary Figures 2). Below 10 cm soil depth, DBD, sediment particles < 0.125 mm and δ13C signatures remained constant or slightly declined down-core in all meadows, except for the increase in sediment particles < 0.125 mm and δ13C signatures after 30 cm depth in H. ovalis cores. The soil Corg content (%) in Posidonia spp. and Amphibolis spp. showed no clear trends with soil depth, but Corg content declined in Posidonia/Amphibolis spp. and H. ovalis cores below 20 cm depth. Overall, there were no significant relationships (P > 0.05) between %Corg and the proportion of sediment particles < 0.125 mm (R2 = 0.02), nor between %Corg and δ13C signatures (R2 = 0.07), except for Halophila cores that showed a weak but statistically significant positive relationship between %Corg and δ13C signatures (R2 = 0.37; Supplementary Figures 3).
Amphibolis spp. meadows had significantly higher DBD (1.3 ± 0.03 g cm–3) than Posidonia spp., Posidonia/Amphibolis spp., and H. ovalis meadows (ranging from 1.0 ± 0.03 to 1.1 ± 0.02 g cm–3; P < 0.001; Figure 2A and Table 2). The Corg content was significantly lower in H. ovalis meadows (0.4 ± 0.1% Corg and 1.4 ± 0.2 mg Corg cm–3) compared to the other meadows (ranging from 0.9 ± 0.004 to 1.5 ± 0.1% Corg and 11.0 ± 0.5 and 15.6 ± 0.6 mg Corg cm–3; P < 0.001), while mixed Posidonia/Amphibolis spp. meadows contained higher Corg content (1.5 ± 0.1% Corg and 15.6 ± 0.6 mg Corg cm–3) than mono-specific Amphibolis spp. meadows (0.9 ± 0.03% Corg and 11.0 ± 0.6 mg Corg cm–3; P < 0.001; Figures 2B,C). The δ13C values of organic matter in Posidonia spp. soils were significantly lower (−16.7 ± 0.6‰) than in Posidonia/Amphibolis spp., Amphibolis spp., and H. ovalis meadows (−13.8 ± 0.2, −13.7 ± 0.3, and −15.0 ± 0.3‰, respectively; P < 0.001; Figure 2E and Table 2). Therefore, the contribution of seagrass-derived organic matter to the soil Corg pool was relatively lower in Posidonia spp. (33 ± 2%) compared to the other meadows (ranging from 53 to 66%; Figure 3 and Supplementary Tables 2). The proportion of sediment particles < 0.125 mm was significantly higher in Amphibolis spp. and Posidonia/Amphibolis spp. (14.6 and 13.5%, respectively) than in Posidonia spp. and H. ovalis meadows (7.3 and 8.7%, respectively; P < 0.001; Figure 2F and Table 2).
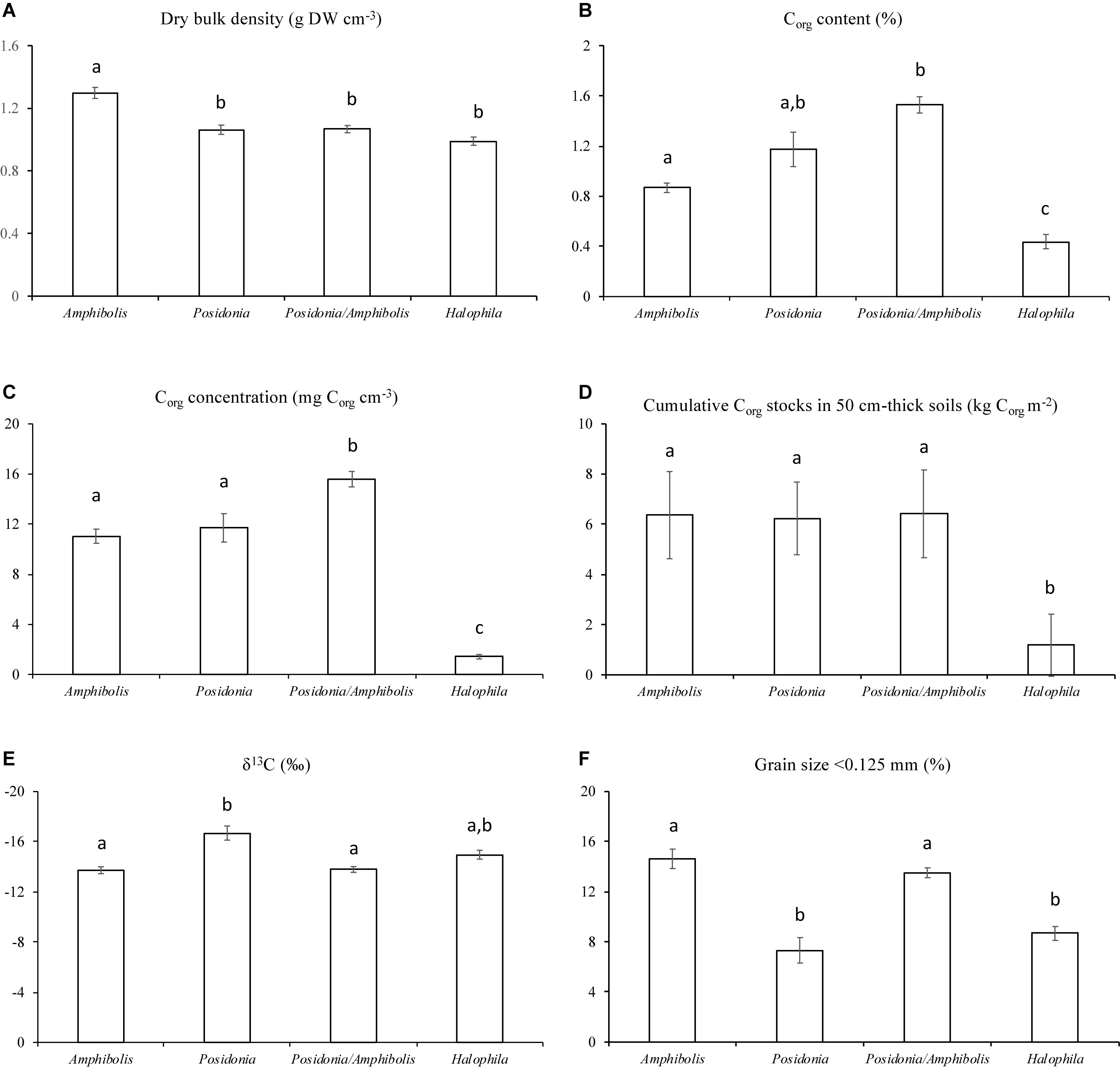
Figure 2. Mean ± SE of (A) dry bulk density (g DW cm–3), (B) Corg content (%), (C) Corg concentration (mg cm–3), (D) Corg inventories (kg m–2), (E) δ13C values (‰), and (F) fine sediment content (% < 0.125 mm) among seagrass habitats (mono-specific Amphibolis spp., Posidonia spp. and H. ovalis, and mixed Posidonia/Amphibolis spp. meadows).
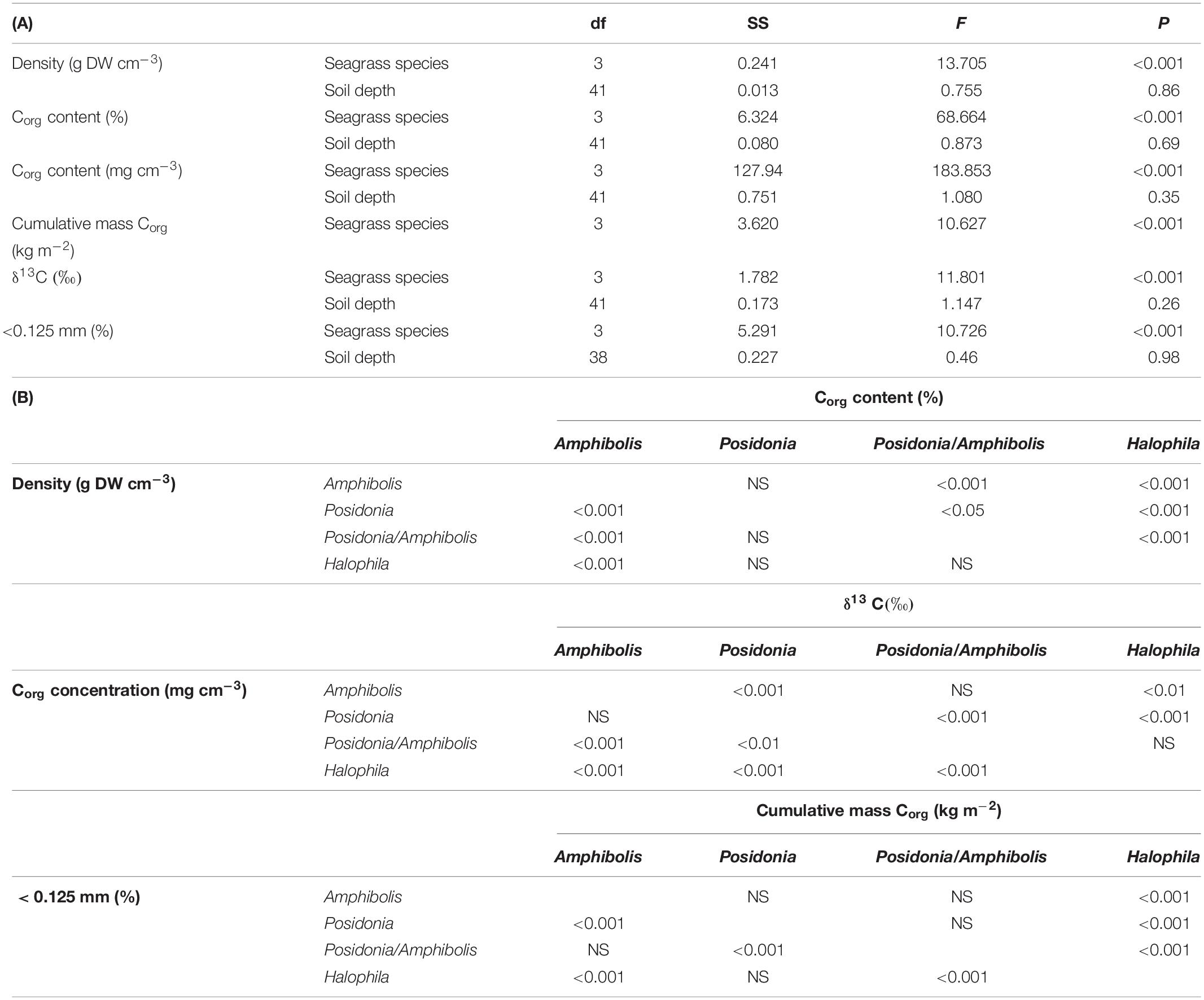
Table 2. (A) Results of statistical testing (GLMMs) for significant effects of seagrass species and soil depth (cm) on the dry bulk density (g DW cm–3), organic carbon content (%Corg and g Corg cm–3), cumulative soil Corg stocks at 50 cm depth (kg m–2), stable carbon isotope values (δ13C), and the content of soil particles < 0.125 mm. (B) Post hoc tests showing differences in the variables studied among seagrass species.
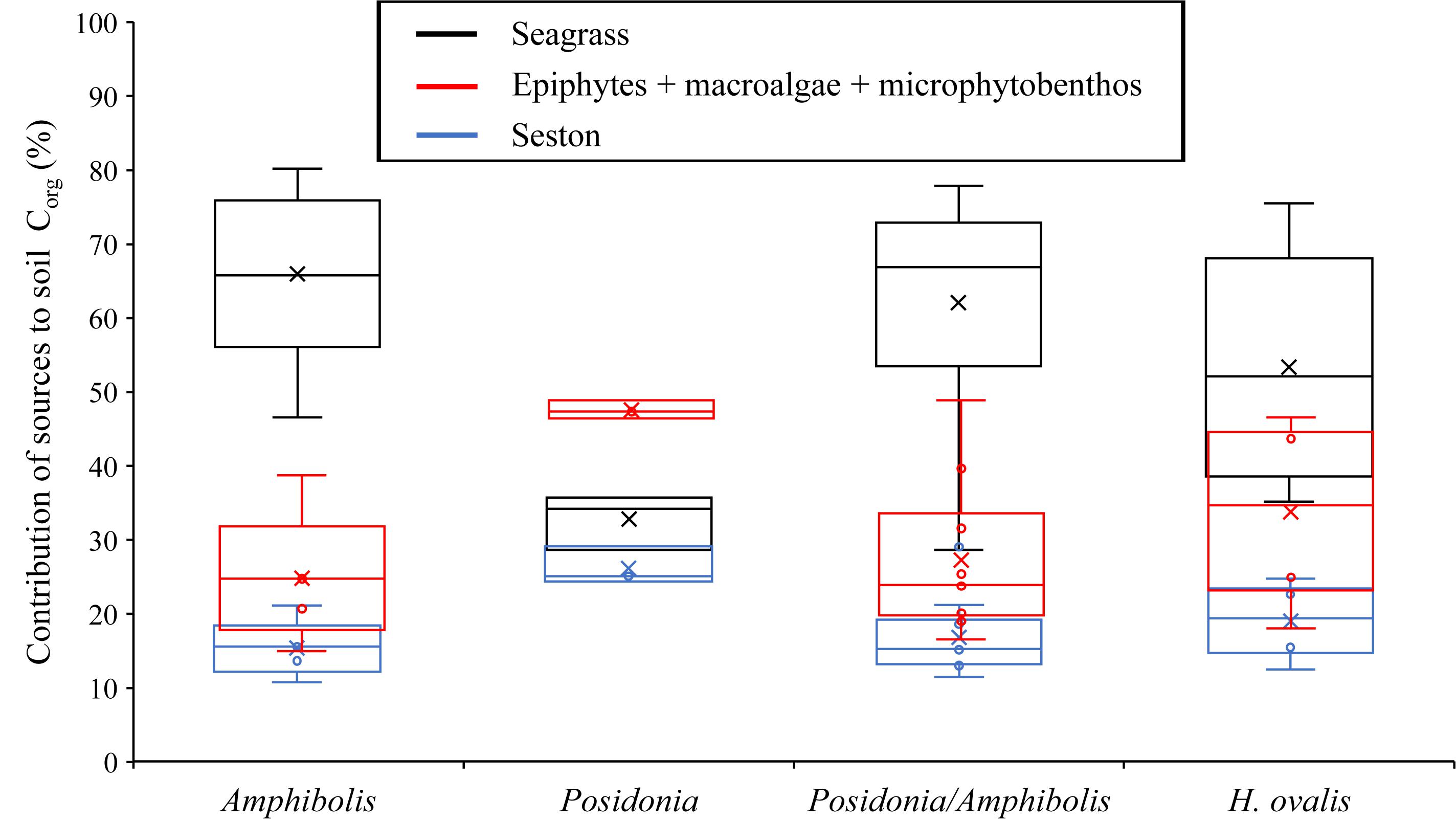
Figure 3. Boxplot displaying the outcomes of the stable isotopic mixing models. Proportion (25, 50, and 75% quantiles) of autochthonous (seagrass) and allochthonous (i.e., epiphytes plus macroalgae plus microphytobenthos, and seston) Corg to the seagrass soil Corg pool (top 50 cm) based on seagrass species (Amphibolis spp., Posidonia spp., Posidonia/Amphibolis spp., and Halophila ovalis).
The Corg stocks and accumulation rates in H. ovalis meadows (1.2 ± 0.6 kg Corg m–2 and 5.4 ± 0.4 g Corg m–2 year–1) were up to fivefold lower than in Posidonia/Amphibolis spp. (6.4 ± 1.0 kg Corg m–2 and 29.4 ± 1.8 g Corg m–2 year–1), Amphibolis (6.4 ± 1.3 kg Corg m–2 and 29.1 ± 2.6 g Corg m–2 year–1), and Posidonia (6.2 ± 1.2 kg Corg m–2 and 28.5 ± 3.2 g Corg m–2 year–1) meadows (P < 0.001), which were similar among them (Figure 2D and Table 1). The total seagrass Corg stocks in 0.5 m thick soils in Rottnest Island, accounting for inter-species variability, were estimated to be 48.1 ± 8.5 Gg Corg. Of this total stock, 34% was contained within monospecific Amphibolis spp. meadows, 14% in monospecific Posidonia spp. meadows, and the remaining 51% in mixed meadows of Posidonia/Amphibolis spp. These contributions are directly proportional to the area of each seagrass habitat. The seagrass meadows in Rottnest Island were estimated to have accumulated 220 ± 17 Mg Corg year–1 over the last decades. As with Corg stocks, the contribution of each habitat to the total annual sequestration was in direct proportion to their area. The seagrass Corg stocks in Rottnest Island are equivalent to 177 ± 31 Gg CO2 captured at a rate of 0.81 ± 0.06 Gg CO2 year–1 (Table 3).
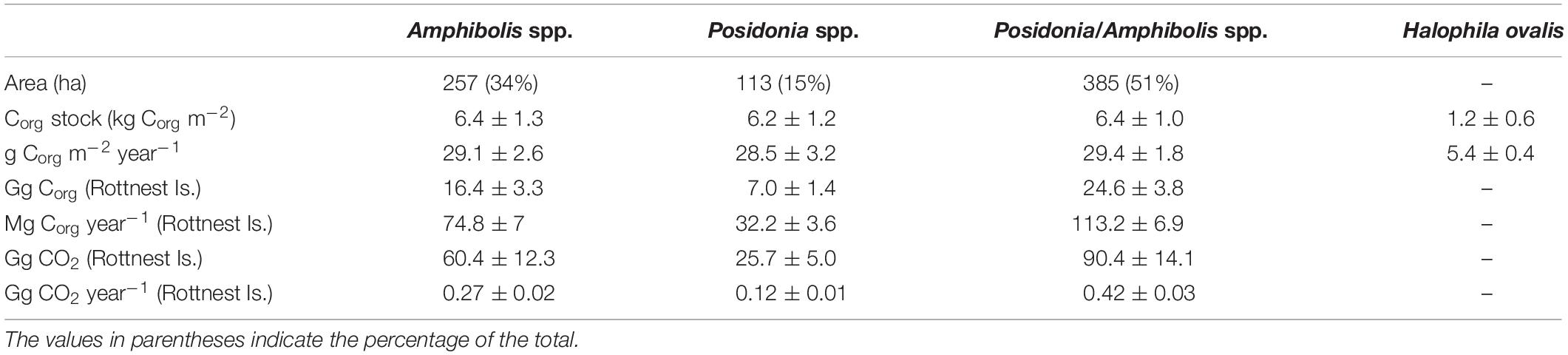
Table 3. Differences in area (ha), organic carbon (Corg) stocks (in 0.5 m thick soils; kg Corg m–2) and accumulation rates (g Corg m–2 year–1) per unit area, Corg stocks (in 0.5 m thick soils; Gg Corg), and Corg accumulation rates (Mg Corg year–1) and CO2–eq (Gg CO2, Gg CO2 year–1) among seagrass habitats at Rottnest Island.
Discussion
Variability in Seagrass Corg Storage Among Habitats
The soil %Corg content in Posidonia spp. (0.9–1.4%), Amphibolis spp. (0.6–0.9%), Halophila spp. (0.05–1.4%), and mixed Posidonia/Amphibolis spp. (0.7–2.3%) meadows measured in this study (Figure 2B) are comparable to global values from meadows dominated by the seagrass P. sinuosa and P. australis (0.1–2.1%), A. antarctica and A. griffithii (0.4–3%), and Halophila spp. (0.6–1.2%; Fourqurean et al., 2012b; Lavery et al., 2013; Serrano et al., 2014; Campbell et al., 2015). The relatively low soil Corg content in Rottnest Island seagrasses (1.15% Corg) compared to the global average (2% Corg; Fourqurean et al., 2012a) resulted mainly from the low Corg content in Halophila spp. meadows and to the fact that global estimates are likely biased by extremely high Corg storage values from some temperate seagrass meadows, especially Posidonia oceanica.
The seagrass soil Corg stocks at Rottnest Island (0.05–12.9 kg Corg m–2 in 0.5 m thick soils) are in the low range compared to global estimates (ranging from 12 to 83 kg Corg m–2 in 1 m thick soils; Fourqurean et al., 2012a). However, the global estimates were derived from a limited data set biased by the extremely high Corg content of soils from Mediterranean P. oceanica meadows (Fourqurean et al., 2012a). Recent estimates of soil Corg stocks in seagrass meadows encompassed by low biomass species (e.g., Halodule uninervis, H. ovalis, Halophila stipulacea, Zostera spp., and Thalassia hemprichii) ranged from 0.1 to 5.4 kg Corg m–2 (in 0.5 m thick deposits; estimated from Campbell et al., 2015; Macreadie et al., 2015; Serrano et al., 2018), which are within the range of Corg stocks estimated for low biomass H. ovalis meadows at Rottnest Island (ranging from 0.05 to 4.2 kg Corg m–2). In addition, the Corg stocks in meadows with high biomass species (P. australis and P. sinuosa) at Rottnest Island (ranging from 2.7 to 12.9 kg Corg m–2) is in the higher range of other meadows with similar species composition (e.g., ranging from 0.9 to 3.6 kg Corg m–2; estimated for 0.5 m thick soils from Rozaimi et al., 2016 and Serrano et al., 2016b), but fourfold higher than previous studies on Amphibolis spp. meadows (1.6 kg Corg m–2 in 0.5 m thick soils; estimated from Lavery et al., 2013). Hence, despite Corg stocks in Rottnest Island seagrass soils tending to be in the lower range of global estimates, the comparisons above highlight the need to update the global estimate of Corg content in seagrass soils using a more balanced geographical distribution of seagrass meadows, but also accounting for habitat variability (i.e., diversity of morphological traits across species and geomorphology; Mazarrasa et al., 2018).
Seagrass biomass and net primary production have been found to play a key role in Corg storage, with dense meadows formed by large species storing larger amounts of Corg in their soils (Fourqurean et al., 2012a; Lavery et al., 2013; Serrano et al., 2016c). The results obtained in this study support this generalization, with up to fivefold higher Corg stocks in Posidonia spp. and Amphibolis spp. meadows compared to H. ovalis meadows. Seagrass meadows of the genera Posidonia (P. australis and P. sinuosa) and Amphibolis (A. antarctica and A. grifficiae) are composed of large and long-living species with high above- and below-ground biomass (averaging 500 and 1,000 g DW m–2, respectively), whereas H. ovalis is a small and fast-growing species which forms relatively low biomass meadows (76 g DW m–2; Duarte and Chiscano, 1999). These differences in biomass likely contributed to the higher Corg stocks in Posidonia/Amphibolis spp. meadows compared to H. ovalis meadows. Another factor that may contribute to higher Corg stocks in monospecific and mixed Posidonia/Amphibolis spp. meadows is their capacity to form bulky roots and rhizomes that can penetrate deep into the soil, helping to more rapidly bury dead roots and rhizomes in deeper, anoxic soils, which favors their preservation (Mateo et al., 2006), further enhanced by the higher recalcitrance of these tissues (Trevathan-Tackett et al., 2017). The higher Corg content (% and mg Corg cm–3) in mixed Posidonia/Amphibolis spp. meadows compared to monospecific meadows could be related to the higher functional performance (e.g., productivity) of meadows formed by multiple species (Duarte, 2000), as observed in mangroves where monotypic forests have lower Corg stocks than those comprising multiple species (Atwood et al., 2017).
Mud content is a good predictor of soil Corg content in Halophila spp. meadows but not in mixed Posidonia/Amphibolis spp. meadows (Serrano et al., 2016a), where the larger contributions of seagrass matter to soil Corg pools dominates the contributing factors. Consequently, the higher δ13C values (typical of seagrasses compared to other source of carbon) in meadows of larger seagrass species have been associated with higher Corg storage (Serrano et al., 2016a). The higher contribution of seagrass-derived Corg to the soil Corg pool in Amphibolis spp. and Posidonia/Amphibolis spp. meadows (ranging from 62 to 66%) support this hypothesis (Figure 3). However, the relatively higher soil Corg stocks in Posidonia spp. meadows were not linked to relatively high seagrass-Corg contributions (33% on average), while the relatively high contribution of seagrass-derived Corg in H. ovalis meadows (averaging 53%) did not entail relatively higher soil Corg stocks. The significant positive relationship between %Corg and δ13C values in Halophila spp. meadows is consistent with the previous observation that soil Corg stocks increase with increasing contribution of seagrass-derived Corg in the soil pool (Serrano et al., 2016a). However, the lack of significant relationships between %Corg and particles < 0.125 mm, and between %Corg and δ13C within monospecific and mixed Posidonia spp. and Amphibolis spp. meadows precludes any generalizable conclusion about the influence of sediment grain-size and seagrass-detritus contribution on soil Corg storage. Other habitat characteristics known to play a role in seagrass soil Corg storage (e.g., differences in hydrodynamic energy, plant cover, density, and biomass; Mazarrasa et al., 2018) among study sites, which were not considered in this study, may help to explain the observations.
The Corg accumulation rates in Rottnest Island seagrass (23.1 ± 3.2 g Corg m–2 year–1 on average) are much lower than global estimates (138 ± 38 g Corg m–2 year–1; Duarte et al., 2013). However, the Corg accumulation rates in Posidonia spp. meadows at Rottnest Island (28 ± 3 g Corg m–2 year–1) are similar to previous estimates for Posidonia spp. meadows in Australia (12 ± 7 to 26 ± 0.8 g Corg m–2 year–1; Marbà et al., 2015; Serrano et al., 2016b), but lower than previous estimates for P. oceanica meadows in the Mediterranean Sea (84 ± 20 g Corg m–2 year–1), which are the highest in the world (Serrano et al., 2016b). The low accumulation rates in Halophila meadows (5.4 ± 0.4 g Corg m–2 year–1), are similar to previous estimates for low biomass and fast-growing seagrass species (i.e., Zostera spp., T. hemprichii, Enhalus acoroides, and Cymodocea serrulata) from Japan, ranging from 2.1 to 10.1 g Corg m–2 year–1 (Miyajima et al., 2015).
Contribution of Seagrasses Toward Carbon Neutrality at Rottnest Island
Seagrasses are widely distributed along the coast at Rottnest Island, occupying 755 ha within the Marine Park (excluding meadows formed by small seagrasses such as Halophila spp.), and store considerable amounts of Corg in their soils. In this study, it was not possible to incorporate inter-species variability in Corg storage (i.e., higher Corg stocks in Posidonia and Amphibolis spp. compared to H. ovalis meadows) because of mapping constrains for Halophila spp. meadows. Thus, there is a need to improve seagrass mapping (i.e., including extent of small and large seagrasses) to robustly estimate seagrass blue carbon ecosystem service at Rottnest Island and globally.
Accounting for Corg storage, we estimated that seagrass meadows at Rottnest Island store ∼48 ± 8 Gg Corg within the top 0.5 m soils, which is equivalent to roughly 48 years of local CO2 emissions at 2015 rates (3,650 Mg CO2 year–1; RIA, 2015). Soil Corg stocks at Rottnest Island have been accumulating at a rate of 0.81 ± 0.06 Gg Corg year–1 over the last decades, thereby sequestering Corg at a rate equivalent to ∼22% of current annual CO2 emissions from the Island (ranging from 20 to 24%). This highlights the significant role of seagrass meadows in sequestering CO2.
The estimates of total Corg storage and CO2 sequestration in Rottnest Island provided here are overall conservative because: (1) we did not incorporate Corg storage within small meadows such as those formed by H. ovalis due to the lack of knowledge on their extent, (2) we restricted our estimates within the 755 ha of mapped seagrass area within the Rottnest Marine Park (up to 15 m depth), and (3) our estimates only considered the Corg stocks within the top 50 cm of soil, while some habitats may contain deeper seagrass Corg stocks. In addition, the large extent of macroalgae at Rottnest Island (190 ha for canopy-forming macroalgae and 1,192 ha for turf algae; Harvey, 2009) could also contribute to Corg sequestration in marine sediments and the deep ocean (Krause-Jensen and Duarte, 2016). In particular, our results showed that macroalgae (plus epiphytes) contributed 12–45% (28% on average) to the seagrass soil Corg pool, suggesting that the export of macroalgae biomass largely contributes to seagrass blue carbon (Kennedy et al., 2010).
Seagrass ecosystems around Rottnest Island were under threat and declining due to anchoring damage (Hastings et al., 1995), which lead to the loss of seagrass, and their associated carbon sink capacity, while eroding the carbon stocks (Serrano et al., 2016d). The deployment of 893 moorings along the coast of Rottnest Island since 1950s has resulted in the loss of 4.8 ha of seagrass meadows (RIA, 2015), which led to the erosion of existing sedimentary Corg stocks and the lack of further accumulation of Corg (Serrano et al., 2016d). Assuming an average loss of 48 Mg Corg ha–1 following mooring deployment (Serrano et al., 2016d), we estimate cumulative emissions of 845 Mg CO2–eq from soil Corg stocks (within 4.8 ha of seagrass loss), and a lack of sequestration of 3.9 Mg CO2–eq ha–1 year–1 (cumulative lack of sequestration over 60 years estimated at 235 Mg CO2–eq year–1). Seagrass conservation and restoration measures resulting in enhanced Corg sequestration and/or avoided emissions at Rottnest Island can help offset CO2 emissions by human activities in the island. For example, the revegetation of the 4.8 ha mooring scar extent could result in enhanced sequestration of 19 Mg CO2–eq year–1, which would offset 0.5% of annual CO2 emissions at Rottnest Island (3,650 Mg CO2 year–1; RIA, 2018).
Conclusion
Rottnest Island is a popular tourism destination, with approximately half a million visitors per year, with 150,000 arriving by private vessel (Smallwood and Beckley, 2008). The RIA is committed to increasing the environmental, social, and economic sustainability of the Island through increasing the placement of renewable energy, increasing energy efficiency and actions to promote carbon sequestration through potential revegetation programs. This is reflected in the lower CO2 emissions at Rottnest Island (0.007 Mg CO2 per capita1) compared to the average emissions in Australia (16 Mg CO2 per capita). Therefore, through the incorporation of seagrass Corg sequestration in national greenhouse gas inventories (IPCC, 2003), Rottnest Island is well positioned to improve its carbon neutral policy. The CO2 sequestration capacity of seagrass ecosystems provides one means of valuing these ecosystems and would provide economic and development opportunities for coastal communities in Rottnest Island and around the world, thereby potentially generating economic income through carbon trading schemes based on the conservation and restoration of seagrass meadows resulting in enhanced Corg sequestration and/or avoided CO2 emissions (Kelleway et al., 2017). For instance, the replacement of existing moorings at Rottnest Island for environmental-friendly moorings could contribute to minimize seagrass fragmentation and enhance recolonization of bare areas (Demers et al., 2013), thereby enhancing Corg sequestration and reducing CO2 emissions.
Despite the low Corg stocks of Halophila meadows it is important not to neglect their contribution to ecosystem dynamics. As they are small pioneer species, in temperate regions they are extremely important in colonizing the gaps and scars created by human and natural disturbances, facilitating the establishment of long-living and large biomass seagrasses such as Posidonia spp. and Amphibolis spp. after disturbance (Duarte et al., 1997; Duarte, 2000). Our results showed that there is a need to incorporate inter-habitat variability in regional estimates of seagrass blue carbon resource, in particular accounting for differences between large and long living seagrass such as Posidonia spp. and Amphibolis spp. vs. small and short living species such as H. ovalis.
Our results contribute to the existing global dataset on seagrass soil Corg storage. Furthermore, the results show a significant potential of seagrass to sequester CO2, which are particularly relevant in the frame of achieving carbon neutral in environmentally-friendly tourist destinations. Indeed, conservation actions aimed to enhance Corg sequestration and/or avoid CO2 emissions from disturbed seagrass ecosystems could contribute to offset CO2 emissions at Rottnest Island, and it will also help to protect one of the most valuable ecosystems in the world considering the ecological services they provide (Costanza et al., 1997).
Data Availability Statement
All datasets generated for this study are included in the article/Supplementary Material.
Ethics Statement
The Ethics Committee of Edith Cowan University has approved this study.
Author Contributions
OS designed the study. CB, OS, MH, and CD carried out the field and laboratory measurements. MH mapped habitat types. CB and OS analyzed the data and drafted the first version of the manuscript. All authors contributed to the writing and editing of the manuscript.
Funding
This work was supported by the ECU Faculty Research Grant Scheme. CB was supported by Brazilian Scholarship Program Science Without Borders. OS was supported by an ARC DECRA (DE170101524) and Edith Cowan University Collaboration Enhancement Scheme.
Conflict of Interest
The authors declare that the research was conducted in the absence of any commercial or financial relationships that could be construed as a potential conflict of interest.
Acknowledgments
The authors are grateful to R. Czarnik, Y. Olsen, I. Hendricks, and C. Salinas for their help in field and laboratory tasks.
Supplementary Material
The Supplementary Material for this article can be found online at: https://www.frontiersin.org/articles/10.3389/fmars.2020.00001/full#supplementary-material
FIGURES 1 | Relationship between extrapolated and measured organic carbon stocks (a) from 30 cm to 50 cm in seagrass soil cores ≥ 50 cm depth.
FIGURES 2 | Changes along soil depth (mean ± SE) in the variables studied based on seagrass species at Rottnest Island.
FIGURES 3 | Biplots showing the relationships among the variables studied in the seagrass cores based on seagrass species.
TABLES 1 | Details of the seagrass cores sampled, including the % compression during coring operations. Core length recovered (cm compressed) and core length corrected for compression (cm decompressed).
TABLES 2 | Mean (±SE) isotopic carbon values (‰) in seagrass soil organic matter. Percentage (%; Mean ± SE) contribution of potential organic sources (i.e., seagrass, epiphytes & macroalgae & microphytoenthos, and seston) into seagrass soils.
TABLES 3 | Dataset used to produce this manuscript.
Footnotes
References
Arias-Ortiz, A., Serrano, O., Masqué, P., Lavery, P. S., Mueller, U., Kendrick, G. A., et al. (2018). A marine heatwave drives massive losses from the world’s largest seagrass carbon stocks. Nat. Clim. Chang. 8, 338–344.
Atwood, T. B., Connolly, R. M., Almahasheer, H., Carnell, P. E., Duarte, C. M., Lewis, C. J. E., et al. (2017). Global patterns in mangrove soil carbon stocks and losses. Nat. Clim. Chang. 7, 523–528.
Cambridge, M. L., and McComb, A. J. (1984). The loss of seagrasses in Cockburn Sound, Western Australia. I. The time course and magnitude of seagrass decline in relation to industrial development. Aquat. Bot. 20, 229–243.
Campbell, J. E., Lacey, E. A., Decker, R. A., Crooks, S., and Fourqurean, J. W. (2015). Carbon storage in seagrass beds of Abu Dhabi, United Arab Emirates. Estuaries Coast. 38, 242–251.
Carmen, B., Krause-Jensen, D., Alcoverro, T., Marbà, N., Duarte, C. M., van Katwijk, M. M., et al. (2019). Recent trend reversal for declining European seagrass meadows. Nat. Commun. 10:3356. doi: 10.1038/s41467-019-11340-4
Carruthers, T. J. B., Dennison, W. C., Kendrick, G. A., Waycott, M., Walker, D. I., and Cambridge, M. L. (2007). Seagrasses of south–west Australia: a conceptual synthesis of the world’s most diverse and extensive seagrass meadows. J. Exp. Mar. Biol. Ecol. 350, 21–45.
Costanza, R., d’Arge, R., De Groot, R., Farber, S., Grasso, M., Hannon, B., et al. (1997). The value of the world’s ecosystem services and natural capital. Nature 387, 253–260.
De Falco, G., Ferrari, S., Cancemi, G., and Baroli, M. (2000). Relationship between sediment distribution and Posidonia oceanica seagrass. Geo Mar. Lett. 20, 50–57. doi: 10.1016/j.scitotenv.2019.05.418
Demers, M. C. A., Davis, A. R., and Knott, N. A. (2013). A comparison of the impact of ‘seagrass-friendly’boat mooring systems on Posidonia australis. Mar. Environ. Res. 83, 54–62. doi: 10.1016/j.marenvres.2012.10.010
Duarte, C. M. (2000). Marine biodiversity and ecosystem services: an elusive link. J. Exp. Mar. Biol. Ecol. 250, 117–131.
Duarte, C. M., and Chiscano, C. L. (1999). Seagrass biomass and production: a reassessment. Aquat. Bot. 65, 159–174.
Duarte, C. M., Losada, I. J., Hendriks, I. E., Mazarrasa, I., and Marbà, N. (2013). The role of coastal plant communities for climate change mitigation and adaptation. Nat. Clim. Chang. 3, 961–968. doi: 10.1371/journal.pone.0044727
Duarte, C. M., Middelburg, J. J., and Caraco, N. (2005). Major role of marine vegetation on the oceanic carbon cycle. Biogeosci. Discuss. 1, 659–679.
Duarte, C. M., Terrados, J., Agawin, N. S., Fortes, M. D., Bach, S., and Kenworthy, W. J. (1997). Response of a mixed Philippine seagrass meadow to experimental burial. Mar. Ecol. Prog. Ser. 147, 285–294.
Fourqurean, J. W., Duarte, C. M., Kennedy, H., Marbà, N., Holmer, M., Mateo, M. A., et al. (2012a). Seagrass ecosystems as a globally significant carbon stock. Nat. Geosci. 5, 505–509. doi: 10.1002/eap.1489
Fourqurean, J. W., Kendrick, G. A., Collins, L. S., Chambers, R. M., and Vanderklift, M. A. (2012b). Carbon, nitrogen and phosphorus storage in subtropical seagrass meadows: examples from Florida Bay and Shark Bay. Mar. Freshw. Res. 63, 967–983.
Gössling, S. (2009). Carbon neutral destinations: a conceptual analysis. J. Sustain. Tour. 17, 17–37.
Gullström, M., Lyimo, L. D., Dahl, M., Samuelsson, G. S., Eggertsen, M., Anderberg, E., et al. (2018). Blue carbon storage in tropical seagrass meadows relates to carbonate stock dynamics, plant–sediment processes, and landscape context: insights from the Western Indian Ocean. Ecosystems 21, 551–566.
Harvey, M. (2009). Development of Techniques to Classify Marine Benthic Habitats Using Hyperspectral Imagery in Oligotrophic, Temperate Waters. Doctoral dissertation, Murdoch University, Perth, WA.
Hastings, K., Hesp, P., and Kendrick, G. A. (1995). Seagrass loss associated with boat moorings at Rottnest Island, Western Australia. Ocean Coast. Manag. 26, 225–246.
Heege, T., and Fischer, J. (2004). Mapping of water constituents in Lake Constance using multispectral airborne scanner data and a physically based processing scheme. Can. J. Remote Sens. 30, 77–86.
Hendriks, I. E., Sintes, T., Bouma, T. J., and Duarte, C. M. (2008). Experimental assessment and modeling evaluation of the effects of the seagrass Posidonia oceanica on flow and particle trapping. Mar. Ecol. Prog. Ser. 356, 163–173.
IPCC (2003). Good Practice Guidance for Land Use, Land−Use Change and Forestry (IPCC National Greenhouse Gas Inventories Programme). Kanagawa: The Institute for Global Environmental Strategies.
Keil, R. G., and Hedges, J. I. (1993). Sorption of organic matter to mineral surfaces and the preservation of organic matter in coastal marine sediments. Chem. Geol. 107, 385–388.
Kelleway, J., Serrano, O., Baldock, J., Cannard, T., Lavery, P., Lovelock, C. E., et al. (2017). Technical Review of Opportunities for Including Blue Carbon in the Australian Government’s Emissions Reduction Fund. Canberra, ACT: CSIRO.
Kendrick, G. A., Hegge, B. J., Wyllie, A., Davidson, A., and Lord, D. A. (2000). Changes in seagrass cover on Success and Parmelia Banks, Western Australia between 1965 and 1995. Estuar. Coast. Shelf Sci. 50, 341–353.
Kennedy, H., Beggins, J., Duarte, C. M., Fourqurean, J. W., Holmer, M., Marbà, N., et al. (2010). Seagrass sediments as a global carbon sink: isotopic constraints. Global Biogeochem. Cycles 24:GB4026.
Krause-Jensen, D., and Duarte, C. M. (2016). Substantial role of macroalgae in marine carbon sequestration. Nat. Geosci. 9, 737–742. doi: 10.1007/s13280-016-0849-7
Lavery, P. S., Mateo, M. Á, Serrano, O., and Rozaimi, M. (2013). Variability in the carbon storage of seagrass habitats and its implications for global estimates of blue carbon ecosystem service. PloS One 8:e73748. doi: 10.1371/journal.pone.0073748
Lavery, P. S., and Vanderklift, M. A. (2002). A comparison of spatial and temporal patterns in epiphytic macroalgal assemblages of the seagrasses Amphibolis griffithii and Posidonia coriacea. Mar. Ecol. Prog. Ser. 236, 99–112.
Macreadie, P. I., Trevathan-Tackett, S. M., Skilbeck, C. G., Sanderman, J., Curlevski, N., Jacobsen, G., et al. (2015). Losses and recovery of organic carbon from a seagrass ecosystem following disturbance. Proc. R. Soc. B Biol. Sci. 282:20151537. doi: 10.1098/rspb.2015.1537
Marbà, N., Arias-Ortiz, A., Masqué, P., Kendrick, G. A., Mazarrasa, I., Bastyan, G. R., et al. (2015). Impact of seagrass loss and subsequent revegetation on carbon sequestration and stocks. J. Ecol. 103, 296–302.
Mateo, M., Cebrián, J., Dunton, K., and Mutchler, T. (2006). “Carbon flux in seagrass ecosystems,” in Seagrasses: Biology, Ecology and Conservation, eds A. W. D. Larkum, et al. (Dordrecht: Springer), 159–192.
Mateo, M. A., Romero, J., Pérez, M., Littler, M. M., and Littler, D. S. (1997). Dynamics of millenary organic deposits resulting from the growth of the Mediterranean seagrass Posidonia oceanica. Estuar. Coast. Shelf Sci. 44, 103–110.
Mazarrasa, I., Samper-Villarreal, J., Serrano, O., Lavery, P. S., Lovelock, C. E., Marbà, N., et al. (2018). Habitat characteristics provide insights of carbon storage in seagrass meadows. Mar. Pollut. Bull. 134, 106–117. doi: 10.1016/j.marpolbul.2018.01.059
Mcleod, E., Chmura, G. L., Bouillon, S., Salm, R., Björk, M., Duarte, C. M., et al. (2011). A blueprint for blue carbon: toward an improved understanding of the role of vegetated coastal habitats in sequestering CO2. Front. Ecol. Environ. 9, 552–560. doi: 10.1890/110004
Miyajima, T., Hori, M., Hamaguchi, M., Shimabukuro, H., Adachi, H., Yamano, H., et al. (2015). Geographic variability in organic carbon stock and accumulation rate in sediments of East and Southeast Asian seagrass meadows. Glob. Biogeochem. Cycles 29, 397–415.
Nellemann, C., and Corcoran, E. (eds.) (2009). Blue Carbon: The Role of Healthy Oceans in Binding Carbon: A Rapid Response Assessment. Arendal: Grid-Arendal/UNEP.
Orth, R. J., Carruthers, T. J., Dennison, W. C., Duarte, C. M., Fourqurean, J. W., Heck, K. L., et al. (2006). A global crisis for seagrass ecosystems. Bioscience 56, 987–996. doi: 10.1016/j.tree.2019.04.004
Parnell, A. C., Inger, R., Bearhop, S., and Jackson, A. L. (2010). Source partitioning using stable isotopes: coping with too much variation. PLoSONE 5:5.
Phillips, D. L., and Gregg, J. W. (2003). Source partitioning using stable isotopes: coping with too many sources. Oecologia 136, 261–269.
Pinnel, N. (2007). A Method for Mapping Submerged Macrophytes in Lakes Using Hyperspectral Remote Sensing. Doctoral dissertation, Technische Universität München, Munich.
RIA (ed.) (2018). Sustainability Action Plan 2018-2024. Rottnest Island, WA: Rottnest Island Authority.
Ricart, A. M., York, P. H., Rasheed, M. A., Pérez, M., Romero, J., Bryant, C. V., et al. (2015). Variability of sedimentary organic carbon in patchy seagrass landscapes. Mar. Pollut. Bull. 100, 476–482. doi: 10.1016/j.marpolbul.2015.09.032
Röhr, M. E., Bostrom, C., Canal-Vergés, P., and Holmer, M. (2016). Blue carbon stocks in Baltic Sea eelgrass (Zostera marina) meadows. Biogeosciences 13, 6139–6153.
Rozaimi, M., Lavery, P. S., Serrano, O., and Kyrwood, D. (2016). Long-term carbon storage and its recent loss in an estuarine Posidonia australis meadow (Albany, Western Australia). Estuar. Coast. Shelf Sci. 171, 58–65.
Serrano, O., Almahasheer, H., Duarte, C. M., and Irigoien, X. (2018). Carbon stocks and accumulation rates in Red Sea seagrass meadows. Sci. Rep. 8:15037. doi: 10.1038/s41598-018-33182-8
Serrano, O., Lavery, P. S., Duarte, C. M., Kendrick, G. A., Calafat, A., York, P. H., et al. (2016a). Can mud (silt and clay) concentration be used to predict soil organic carbon content within seagrass ecosystems? Biogeosciences 13, 4915–4926.
Serrano, O., Lavery, P. S., López-Merino, L., Ballesteros, E., and Mateo, M. A. (2016b). Location and associated carbon storage of erosional escarpments of seagrass Posidonia mats. Front. Mar. Sci. 3:42. doi: 10.3389/fmars.2016.00042
Serrano, O., Lavery, P. S., Rozaimi, M., and Mateo, M. Á. (2014). Influence of water depth on the carbon sequestration capacity of seagrasses. Glob. Biogeochem. Cycles 28, 950–961.
Serrano, O., Ricart, A. M., Lavery, P. S., Mateo, M. A., Arias-Ortiz, A., Rozaimi, M., et al. (2016c). Key biogeochemical factors affecting soil carbon storage in Posidonia meadows. Biogeosciences 13, 4581–4594.
Serrano, O., Ruhon, R., Lavery, P. S., Kendrick, G. A., Hickey, S., Masqué, P., et al. (2016d). Impact of mooring activities on carbon stocks in seagrass meadows. Sci. Rep. 6:23193. doi: 10.1038/srep23193
Short, F. T., and Wyllie-Echeverria, S. (1996). Natural and human-induced disturbance of seagrasses. Environ. Conserv. 23, 17–27. doi: 10.1016/j.marenvres.2008.04.004
Smallwood, C. B., and Beckley, L. E. (2008). Benchmarking recreational boating pressure in the Rottnest Island reserve, Western Australia. Tour. Mar. Environ. 5, 301–317.
Torbatinejad, N. M., Annison, G., Rutherfurd-Markwick, K., and Sabine, J. R. (2007). Structural constituents of the seagrass Posidonia australis. J. Agric. Food Chem. 55, 4021–4026.
Trevathan-Tackett, S. M., Macreadie, P. I., Sanderman, J., Baldock, J., Howes, J. M., and Ralph, P. J. (2017). A global assessment of the chemical recalcitrance of seagrass tissues: implications for long-term carbon sequestration. Front. Plant Sci. 8:925. doi: 10.3389/fpls.2017.00925
Waite, A. M., Muhling, B. A., Holl, C. M., Beckley, L. E., Montoya, J. P., Strzelecki, J., et al. (2007). Food web structure in two counter-rotating eddies based on δ15N and δ13C isotopic analyses. Deep Sea Res. Part II Top. Stud. Oceanogr. 54, 1055–1075.
Walker, D. I., Lukatelich, R. J., Bastyan, G., and McComb, A. J. (1989). Effect of boat moorings on seagrass beds near Perth, Western Australia. Aquat. Bot. 36, 69–77.
Waycott, M., Duarte, C. M., Carruthers, T. J., Orth, R. J., Dennison, W. C., Olyarnik, S., et al. (2009). Accelerating loss of seagrasses across the globe threatens coastal ecosystems. Proc. Natl. Acad. Sci. U.S.A. 106, 12377–12381. doi: 10.1073/pnas.0905620106
Wells, F. E., Walker, D. I, Kirkman, H., Lethbridge, R., and Australian Marine Sciences Association, (1993). The Marine Flora and Fauna of Rottnest Island, Western Australia. Perth, WA: Western Australian Museum.
Keywords: organic carbon, coastal vegetated ecosystems, Posidonia, Amphibolis, Halophila, Rottnest Island, Western Australia
Citation: Bedulli C, Lavery PS, Harvey M, Duarte CM and Serrano O (2020) Contribution of Seagrass Blue Carbon Toward Carbon Neutral Policies in a Touristic and Environmentally-Friendly Island. Front. Mar. Sci. 7:1. doi: 10.3389/fmars.2020.00001
Received: 26 June 2019; Accepted: 06 January 2020;
Published: 24 January 2020.
Edited by:
Michelle Jillian Devlin, Centre for Environment, Fisheries and Aquaculture Science (CEFAS), United KingdomReviewed by:
Fernando Tuya, University of Las Palmas de Gran Canaria, SpainSasi Nayar, South Australian Research and Development Institute, Australia
Copyright © 2020 Bedulli, Lavery, Harvey, Duarte and Serrano. This is an open-access article distributed under the terms of the Creative Commons Attribution License (CC BY). The use, distribution or reproduction in other forums is permitted, provided the original author(s) and the copyright owner(s) are credited and that the original publication in this journal is cited, in accordance with accepted academic practice. No use, distribution or reproduction is permitted which does not comply with these terms.
*Correspondence: Oscar Serrano, by5zZXJyYW5vZ3Jhc0BlY3UuZWR1LmF1