- 1Ocean Systems, Royal Netherlands Institute for Sea Research and Utrecht University, Den Burg, Netherlands
- 2Biology Department, Rhode Island College, Providence, RI, United States
- 3German Center for Marine Biodiversity Research, Senckenberg am Meer, Wilhelmshaven, Germany
- 4Biology Department, Woods Hole Oceanographic Institution, Woods Hole, MA, United States
- 5Laboratoire d’Ecogéochimie des Environnements Benthiques, CNRS, Sorbonne Université, Banyuls-sur-Mer, France
- 6Laboratoire d’Océanographie de Villefranche, CNRS, Sorbonne Université, Villefranche-sur-Mer, France
- 7Department of Functional and Evolutionary Ecology, University of Vienna, Vienna, Austria
In 2005/2006, a major volcanic eruption buried faunal communities over a large area of the 9°N East Pacific Rise (EPR) vent field. In late 2006, we initiated colonization studies at several types of post eruption vent communities including those that either survived the eruption, re-established after the eruption, or arisen at new sites. Some of these vents were active whereas others appeared senescent. Although the spatial scale of non-paved (surviving) vent communities was small (several m2 compared to several km2 of total paved area), the remnant individuals at surviving active and senescent vent sites may be important for recolonization. A total of 46 meio- and macrofauna species were encountered at non-paved areas with 33 of those species detected were also present at new sites in 2006. The animals living at non-paved areas represent refuge populations that could act as source populations for new vent sites directly after disturbance. Remnants may be especially important for the meiofauna, where many taxa have limited or no larval dispersal. Meiofauna may reach new vent sites predominantly via migration from local refuge areas, where a reproductive and abundant meiofauna is thriving. These findings are important to consider in any potential future deep-sea mining scenario at deep-sea hydrothermal vents. Within our 4-year study period, we regularly observed vent habitats with tubeworm assemblages that became senescent and died, as vent fluid emissions locally stopped at patches within active vent sites. Senescent vents harbored a species rich mix of typical vent species as well as rare yet undescribed species. The senescent vents contributed significantly to diversity at the 9°N EPR with 55 macrofaunal species (11 singletons) and 74 meiofaunal species (19 singletons). Of these 129 species associated with senescent vents, 60 have not been reported from active vents. Tubeworms and other vent megafauna not only act as foundation species when alive but provide habitat also when dead, sustaining abundant and diverse small sized fauna.
Introduction
Animals at deep-sea hydrothermal vents can be exposed to a very dynamic environment of changing vent fluid conditions and associated productivity regimes. On a second to minute timeframe, they can be exposed to highly variable hydrothermal fluids emissions, with temperatures ranging from 2 to about 40°C, and high sulfide and mineral concentrations at diffuse flow active vent sites (Fisher et al., 2007). On fast spreading centers, on yearly to decadal timescales, local venting features within active sites can become inactive, resulting in the death of large symbiotic fauna. After a vent became inactive, animals are exposed to stable ambient deep-sea temperatures, lack of vent fluid emissions, and loss of primary production through chemosynthesis (Tsurumi and Tunnicliffe, 2003). In addition, communities at hydrothermal vents that are associated with magmatic activity can be exposed to large-scale disturbances by volcanic eruptions that can kill animals by burying them with lava (Tolstoy et al., 2006). At the studied 9°N East Pacific Rise (EPR), animal communities are exposed to all of the above-mentioned stressors and disturbances. The large majority of vent research has focused on fauna at active hydrothermal vents. Megafauna associated with chemoautotrophic symbionts such as tubeworms or mussels depend on active venting, as their symbionts require reduced sulfur species to provide organic carbon to their hosts (Childress and Fisher, 1992). The megafauna act as foundation species and host a high biomass and high abundance, but relatively low diversity macrofaunal community that is adapted to the extreme active vent environment and that is largely restricted to the active hydrothermal vent environment (Van Dover, 2000; Fisher et al., 2007). The meiofaunal communities are overall much less studied than the mega- and macrofaunal, but many species that occur at active vents are also present in the vent periphery (Gollner et al., 2015a; Plum et al., 2017).
Senescent or waning vents are poorly studied. They are characterized by: (1) absence of vent fluid emissions, i.e., no visible shimmering water and a temperature anomaly lower than 0.1°C above the ambient (Van Dover, 2002) and (2) presence of (mostly) dead large symbiotic fauna, i.e., vestimentiferan tubes with no visible branchial plumes (Tsurumi and Tunnicliffe, 2003). Senescent vents are ecotones, i.e., areas of environmental transition, where ecological communities coincide. In the case of senescent vents, a former active hydrothermal vent site or patch loses the energy provided by vent fluid emissions, causing the death of symbiotic megafaunal species such as tubeworms that depend on chemolithoautotrophic bacteria (Van Dover, 2000). The patch/site exposed to stable ambient deep-sea temperatures of ∼2°C transits into a biomass rich area without in situ primary production and without hydrothermal vent fluid emissions with very low/no temperature anomaly and reduced toxicity, as the H2S flow decreases but can persist at low levels as a degradation product of organic matter or sulfide minerals. The biomass degrades until the patch/site finally becomes similar to typical biomass poor hard substrate deep-sea areas that are sustained by lateral fluxes of particle from distant active vent patches and downward flux of organic matter from the ocean’s surface waters (Smith et al., 2008). The very few studies from senescent vents show that communities are characterized by a subset of fauna found at active fields as well as several non-vent deep-sea taxa (Van Dover, 2002; Tsurumi and Tunnicliffe, 2003; Gollner et al., 2013). In contrast to the senescent/waning vents that can be viewed as the latest stage of succession at active vents, at old inactive chimneys no vent symbiotic megafauna remainings are visible. Such old inactive vents typically host sessile taxa such as sponges, corals, hydroids, crabs, and echinoderms (Tunnicliffe et al., 1986; Galkin, 1997; Erickson et al., 2009). Boschen et al. (2015) found that megafaunal assemblages on inactive sulfide-rich chimneys may be distinct from both active vents communities and those on seamounts (Boschen et al., 2015).
Succession of active vents after violent volcanic eruptions was studied at the fast spreading Juan de Fuca Ridge and at the 9°N EPR, and showed recovery of benthic communities at active sites in less than a decade (Shank et al., 1998; Marcus et al., 2009; Gollner et al., 2015b, 2017). After such a major disturbance event at the EPR, colonization of new vent sites by macrofaunal larvae from distant areas was found to be one of the major drivers during recovery (Mullineaux et al., 2010, 2012). Simulations on recoverability of vent fields in the western Pacific Ocean suggested substantial variation in recovery time due to variation in regional connectivity, ranging from ∼6 to 140 years (Suzuki et al., 2018), but comparison to the EPR and Juan de Fuca Ridge is not really possible due to the limited knowledge of the temporal dynamics of habitats and lack of knowledge on adaptation of species to environmental instabilities in this area. Time-series studies showed that vent communities along the slow-spreading Mid-Atlantic and recent revisited vent fields along Back-Arc Basins are very stable on decadal time-scale (Cuvelier et al., 2011; Gollner et al., 2017; Du Preez and Fisher, 2018).
Natural disturbances [sensu destruction or removal of biomass (Grime, 1977; Sousa, 2001)], only rarely completely eliminate existing communities (Sousa, 2001). Instead, post-disturbance landscapes are usually mosaics of patches having different species composition. Individuals that survive disturbance may influence colonization and succession after disturbance. Depending on their life-history traits, these remnant individuals, so called legacies (Franklin et al., 1988), can accelerate or impede recovery to its pre-disturbance state (Platt and Connell, 2003). Mullineaux et al. (2009) experimentally tested the imprint of past environmental regime on the vent community succession at the 9°N EPR (Mullineaux et al., 2009). By using transplant experiments, placing communities from warm to cool environments (simulating waning of vent fluids) and from cool to warm (simulating new vent sites), they found that productivity enhancement may outweigh potential physiological stress in setting limits to distributions of vent macrofauna. Most macrofauna species of established “warm” communities were unable to survive in the cold environment with less productivity. Species limited by primary productivity may survive an increase but not a decrease in vent fluid emission (Mullineaux et al., 2009). The legacies of meiofauna are to date not studied, although the different life-history traits of meio- and macrofauna may influence recovery after disturbance.
The size and associated life-history traits of meio- and macrofauna are fundamentally different. Permanent meiofauna, such as nematodes or copepods, remain small as adults (< 1 mm). Macrofauna, such as gastropods, bivalves, and polychaetes, are > 1 mm when adult. During their larvae and juvenile stages, macrofauna are in the same size class as the permanent meiofauna (< 1 mm) (Warwick, 1984; Giere, 2009). Meio- and macrofauna highly influence each other’s community structure (Ólafsson, 2003). The discrimination of meio- and macrofauna is based on life-history traits that are optimized at certain body size (∼1 mm) and weight (45 μg dry weight). They comprise two separate evolutionary units each with an internally coherent set of biological characteristics, such as development (direct benthic versus planktonic), dispersal (as adults versus planktonic larvae), generation time (less than 1 year versus more than 1 year), growth (reach asymptotic adult size versus continue growth throughout life), or mobility (motile versus sedentary or motile) (Warwick, 1984). At hydrothermal vents, patterns of habitat fidelity differ for the meio- and macrofauna: macrofaunal species are primarily limited to direct contact with venting fluids, while meiofaunal species are distributed across proximate and distant habitats to vent openings and are thus not restricted to vent habitats. The presence of vent meiofauna on basalt may suggest that meiofauna is in contrast to macrofauna rather limited by physico-chemical stress than by productivity (Gollner et al., 2015a). One thus may expect that vent meiofauna is present at senescent vents as well.
Once seen as oases within a barren deep ocean, it is now recognized that vent communities interact with surrounding ecosystems on the sea floor and in the water column. Yet, especially the role of waning vents that provide organic remains of dead symbiotic vent megafauna is to date not quantified (Levin et al., 2016). Here, we take the unique opportunity to: (1) investigate the meio- and macrofauna abundance and diversity patterns at senescent vents and to (2) explore the role of fauna present at non-paved senescent and active vents for recovery processes of new vent sites after volcanic eruptions. We monitored site activity from 2006 to 2009 and present meio- and macrofaunal data on one active site where diffuse venting remained after the volcanic eruption (Bio9 Vent) as well as from two sites where the activity had totally ceased after the eruption probably due to clogging or reconstruction of the main high-temperature subseafloor vent fluid channels during the eruption (Q Vent and East Wall). In addition, we show data from two sites which were covered by lava and were active after the eruption, visible by the presence of new Tevnia tubeworm in 2006, but where patches of tubeworms within vent sites locally ceased due to the progressing clogging of the low-temperature emission network on new basalt in the first years after the eruption (Sketchy and P Vent). We hypothesize that (1) senescent vents at the 9°N EPR support a rich meio- and macrofaunal community. We further hypothesize that (2) active and senescent vents that were not paved by lava may play pivotal roles for recovery after disturbance: they provide remnants, i.e., legacies which may act as source populations for the close by new active vents. In addition, we (3) bring the data into a temporal context by comparing our new results to our previous studies on faunal diversity from active vents before and after the eruption (Gollner et al., 2010, 2015a,b). In the final section of the manuscript, we consider our results in the context of deep-sea mining disturbance.
Materials and Methods
Numerous dives were made with the DSV Alvin in order to explore the 9°N EPR vent field after it was covered by lava due to an eruption in late 2005/early 2006 (Tolstoy et al., 2006; Soule et al., 2007). During a first cruise in October 2006, we deployed settlement substrates at six vent sites: Bio9 Vent (not paved by lava, old active black smoker with Pompeii worms); East Wall and Q Vent (not paved by lava but vent fluid stopped after eruption; senescent vent with dead tubeworms/mussels and dead Alvinella polychaetes); Tica Vent, P Vent, Sketchy (paved with lava by the eruption, new venting activity and Tevnia tubeworms in late 2006) (Figure 1). In addition, we deployed settlement substrates also in the vent periphery of each site. In this manuscript, we use the term “new basalt” for peripheral areas that were covered with lava in 2006, and “old basalt” for areas that were not covered with lava in 2006. All sites were located at ∼2500 m depth in the 9°N region of the EPR. We recovered settlement substrates in December 2006, November 2007, and December 2009 (Table 1 and Figures 1, 2). Each settlement substrate used at new active vent patches consisted of two plastic kitchen sponges with a circular surface area of ∼64 cm2 (Gollner et al., 2015b). Each settlement substrate used on basalt or at senescent vents consisted of four plastic kitchen sponges in order to enhance sample size. The suitability of sponges as settlement substrates for fauna was carefully tested. Throughout the entire sampling period (2006–2009), animals from natural community samples were taken and compared to animals from settlement devices. Statistical tests revealed that the plastic kitchen sponges can be considered efficient to quantitatively sample the mobile meiofauna, as well as mobile and sessile macrofauna (for details on statistical test, please see Chapter 2.1. benthic collections and Supplementary Material in Gollner et al., 2015b).
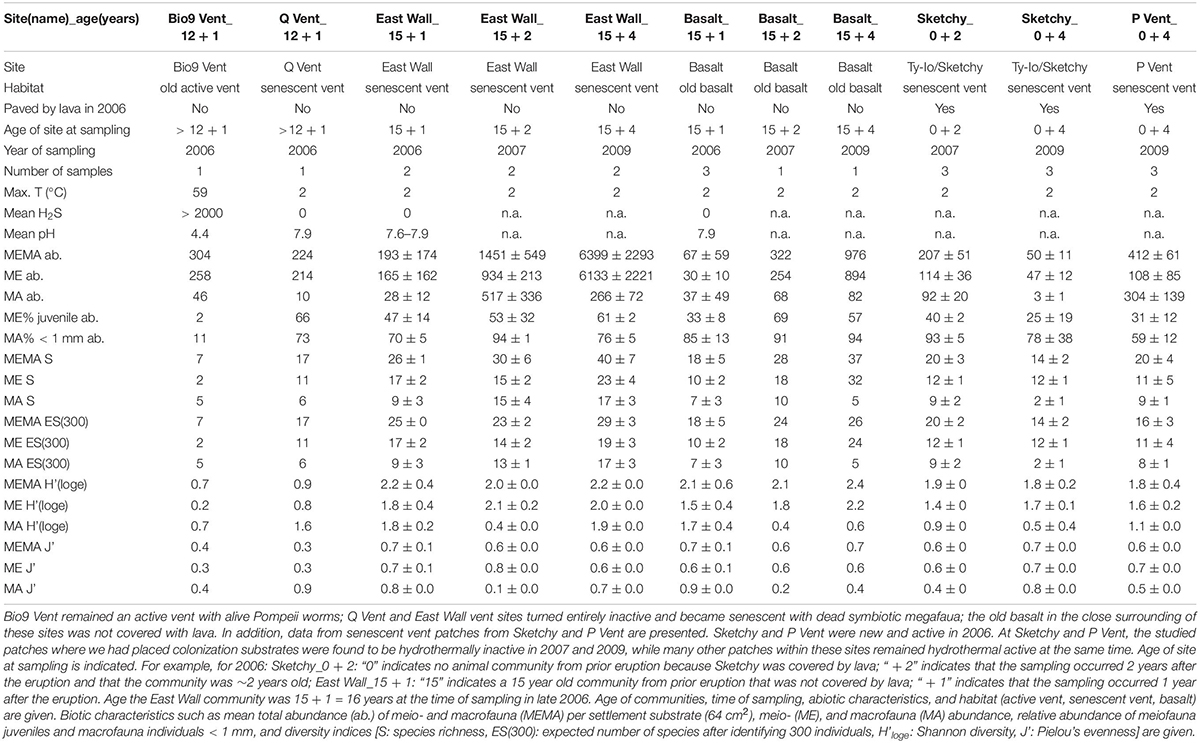
Table 1. Habitat and animal community characteristics of sites that were not covered with lava by the volcanic eruption in 2005/06 (for habitat and animal community characteristics from lava paved sites, including new vent sites and new basalt see Gollner et al., 2015b).
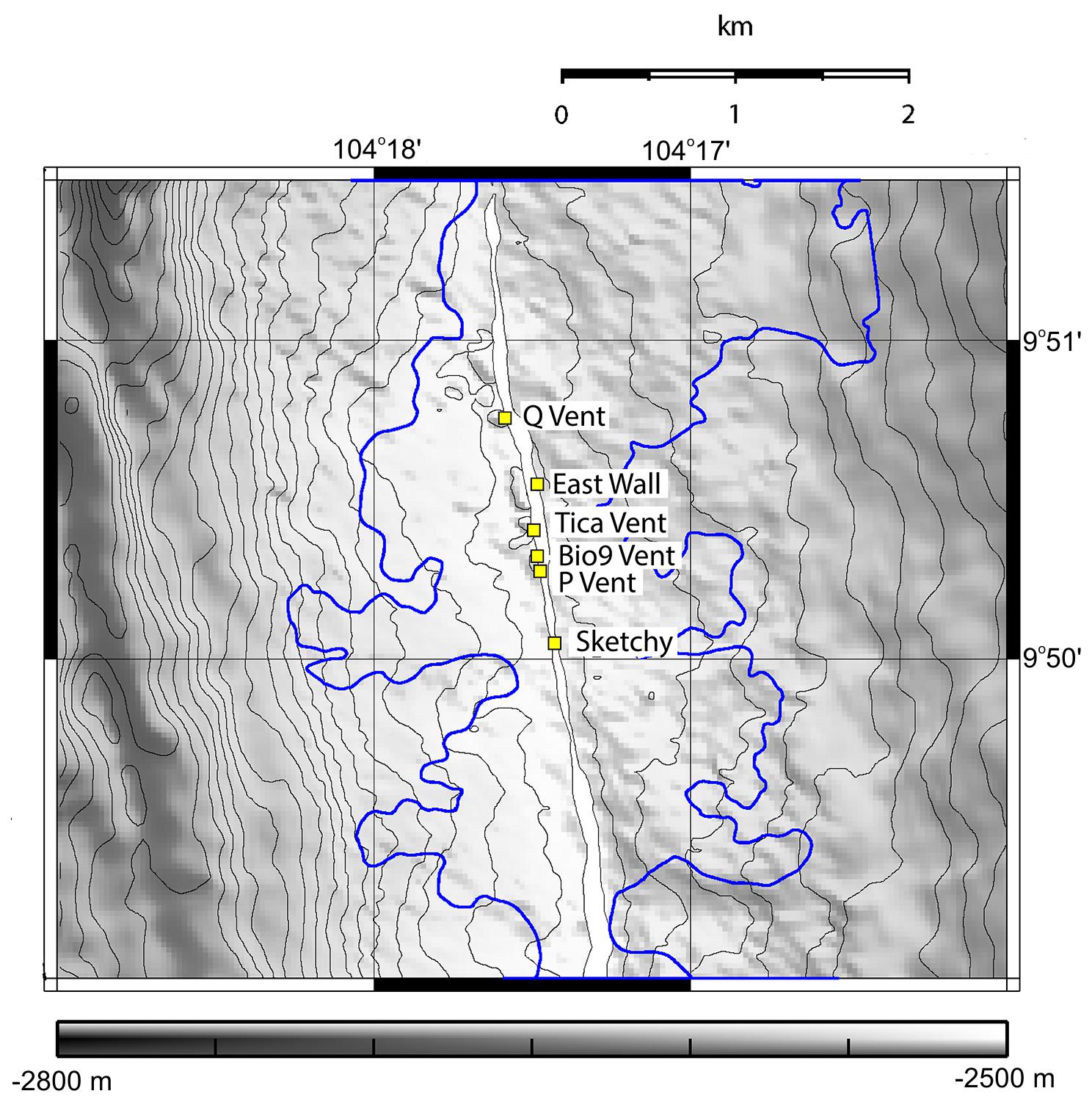
Figure 1. Map showing the studied sites East Wall, Q Vent, Bio9 Vent, Tica Vent, Sketchy patch in the Ty-Io site, and P Vent in the 9°N East Pacific Rise area. Several km2 (blue outline) of the area were flooded with lava during an eruption in 2005/2006. We observed that Bio9 Vent, East Wall and Q Vent were not covered by lava. Tica Vent, Ty-Io/Sketchy, and P Vent were covered by lava. Depth gray scale is given in meters. Map courtesy of Soule et al. (2007).
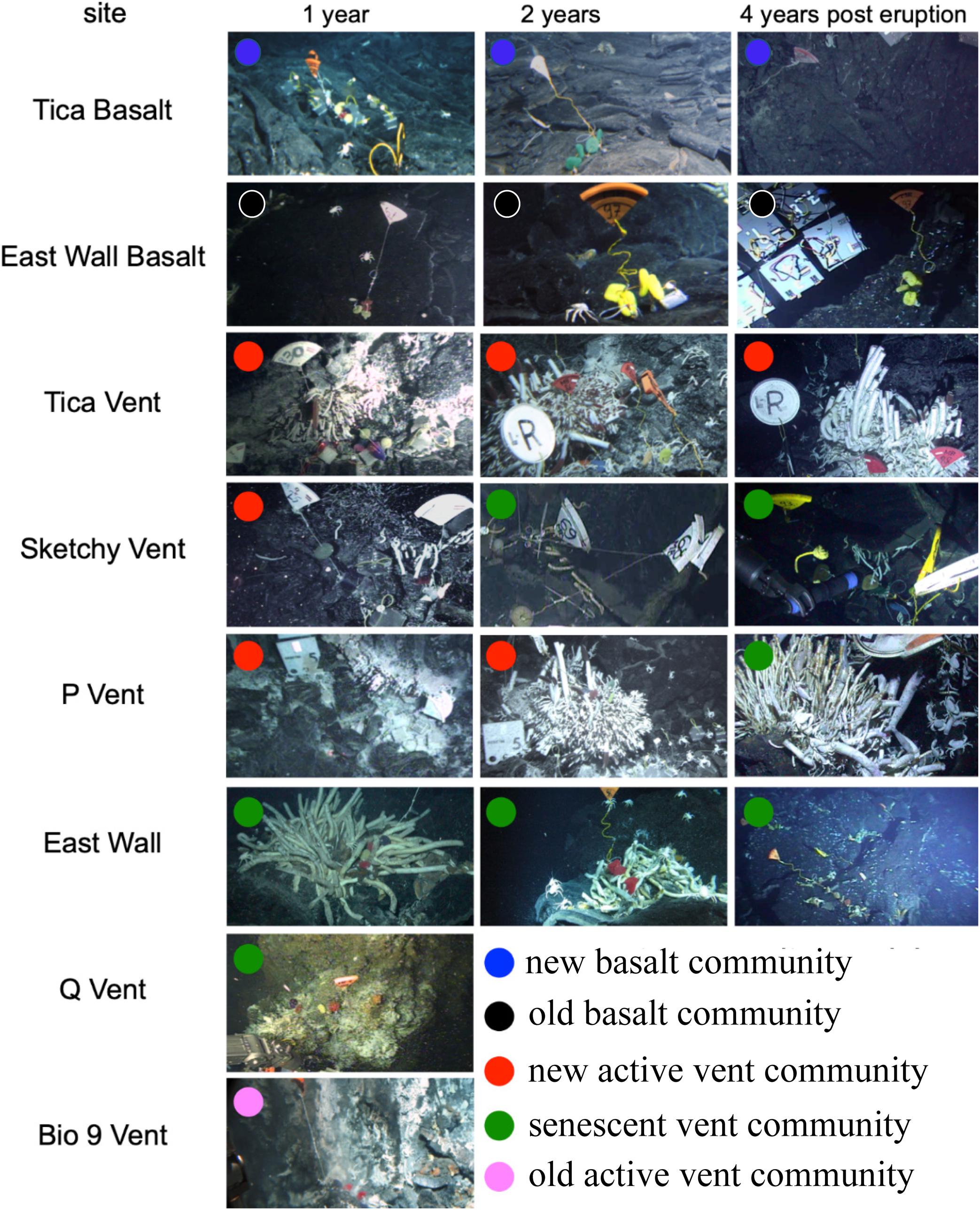
Figure 2. Photographs of studied patches at sites at the 9°N East Pacific Rise from 1 year, 2 years, and 4 years post eruption taken in 2006, 2007, and 2009. Blue dots: new basalt in the periphery of new active vents (new basalt areas covered with lava in 2005/06). Black dots: old basalt not covered with lava. Red dots: new active vents that became active in 2006. Green dots: senescent vents (vent site East Wall and Q Vent were not covered with lava, but no vent fluids in 2006; patches at new Sketchy vent became inactive in the second year post eruption, patches at new P Vent became inactive in the fourth year post eruption). Pink dot: old active vent (active vent site not covered by lava).
All settlement substrates and natural samples were deployed and/or recovered into isolated boxes with the hydraulic arm of the submersible Alvin. Temperature was measured with the temperature probe of the submersible during deployment and recoveries. In situ sulfide and pH measurements were conducted with combined potentiometric pH and sulfide sensors, as described in Mullineaux et al. (2012). To remove animals from settlement substrates, each sponge (consisting of a 55 m long and 1 mm thin plastic thread woven to a loose net, which was furled and fixed with a rubber band) was uncoiled and placed into 37‰ MgCl in water for 10 min to relax the animals (Higgins and Thiel, 1988). Animals were then sieved through a 32 μm and 1 mm net with 32 μm filtered, cold seawater to separate the meio- from the macrofauna. Animals were preserved in 4% buffered formaldehyde (for details see Gollner et al., 2015b). All animals were sorted and counted using a dissecting microscope.
In total ∼96,100 meiofaunal and ∼20,400 macrofaunal individuals were analyzed from senescent vents, from active old Bio9 Vent, and from old basalt for this study (Table 1). In addition, we compare these data to our published datasets of 40,050 meio- and 60,175 macrofauna individuals from new active vent sites and new basalt sites after the eruption from the years 2006–2009 (Gollner et al., 2015b) and to 69,000 meio- and 105,000 macrofauna individuals that had been collected before the eruption in the years 2001–2004 (Gollner et al., 2015a). All three studies exclude abundances of megafauna (Pompeii worms, tubeworms, mussels). All macrofauna > 1 mm were identified to species level. Macrofauna < 1 mm were also identified to species level whenever this was morphologically possible. If this was not possible, they were recorded as juveniles—for example “juvenile gastropods” or “juvenile polychaetes.” For the meiofauna, at least 200 individuals per higher taxon (e.g., copepods, nematodes) were identified per settlement substrate/in situ natural collection to species level unless fewer were found in the sample. All meiofauna specimens were morphologically identified to species level which the exception of juveniles that were grouped into for example “juvenile copepods” or “juvenile nematodes.” Data from meio- and macrofaunal juveniles that could not be identified to species level were included in abundance calculations but excluded from diversity calculations, which are all based on species level identification. All species abundance data are shown in Supplementary Datasheet S1. A detailed faunistic description as well as prokaryote abundances are given in Supplementary Material S1.
Species richness (S obs.), Shannon diversity (H’loge), Pielou’s evenness (J’), and expected number of species after identifying 300 individuals [ES(300)] were calculated from quantitative species abundance data by DIVERSE subroutines in PRIMER Version 6 package (Clarke and Gorley, 2001). ES(300) was chosen because > 300 individuals were identified in samples and in order to compare values to data published by Gollner et al. (2015a, b). For statistical analyses of univariate measurements from prokaryotes and fauna at different sites and years, non-parametric Kruskal–Wallis tests were conducted, followed by multiple pairwise comparisons between groups using the Wilcox test, using the standard package S in the program R studio (Ihaka and Gentleman, 1996). The SIMPROF (similarity profile) test was performed to look for statistically significant differences of clusters of sites without making any a priori hypothesis. This shows in addition to the multi-dimensional-scaling (MDS), the determination of groupings, especially when stress of the MDS is high (Clarke and Warwick, 2001). One-way and two-way crossed analysis of similarity (ANOSIM) and SIMPER (similarity percentage) were used in order to determine (dis)similarity between samples from distinct years (2006, 2007, 2009, pre-eruption) and distinct habitats (active vent, senescent vent, basalt). Two-way analysis was used when testing multiple sites per year. SIMPROF (p < 0.05), SIMPER, ANOSIM, and MDS plots were performed for settlement substrates with PRIMERv6. To demonstrate communities’ time trajectory from distinct sites within the study period, we used overlays in the MDS plots. Bubble plots were used to visualize occurrence of selected species in different habitats and years. To create Bray–Curtis similarity matrices for these analyses, abundances of species were standardized to compensate for varying abundances, and square-root transformed to down-weight the importance of very abundant species without losing the influence of rarer species (Clarke and Gorley, 2001).
Results
Post-disturbance Landscape at the 9°N EPR After the Volcanic Eruption in 2006
In 2006, the 9°N EPR area comprised a very complex environment with three major different habitats (active vent, senescent vent, basalt) and two major different aged communities (old communities that survived the volcanic eruption and new communities that had newly formed after the volcanic eruption). Several sites in the 9°N EPR were visually observed and photographs were taken during a series of Alvin dives in ∼2500 m water depth at the 9°N EPR in October 2006, December 2006, November 2007, and October 2009 (Figures 1, 2 and Table 1).
We observed that the site Bio9 Vent was not covered with lava and the Bio9 black smoker hosted live Pompeii worms (Alvinella pompeiana) and was hydrothermally active in 2006. Bio9 Vent had very hot hydrothermal fluid emissions—the settlement substrates (plastic kitchen sponges) deployed in October 2006 were found to be partly melted after recovery in December 2006, indicating temperature peaks of > 100°C during October and December 2006. Settlement substrates that were intended for recovery from Bio9 Vent in December 2007 were never found back: the smoker had collapsed and eventually buried the settlement substrates and fauna.
The sites Q Vent and East Wall were also not covered with lava but were hydrothermally inactive with no temperature anomalies in 2006 (Table 1 and Figure 2). At Q Vent empty tubes from Pompeii worms were observed. At East Wall empty tubes from Riftia pachyptila and shells from Bathymodiolus thermophilus were found. Q Vent and East Wall are therefore so called senescent vents. Due to dive-plan constraints, Q Vent could only be visited in 2006. At East Wall, we collected samples in 2006, 2007, and 2009. Beside the dead megafauna, also very few individuals (∼ < 10) of alive Bathymodiolus were present at East Wall in 2006 and 2007. At the East Wall site, tubes and shells further degraded in 2007 and were not visually recognizable as tubes and shells anymore in 2009. Only a fluffy organic layer was left on the basalt 4 years after venting had ceased at East Wall (see Figure 2).
Tica Vent, Ty-Io/Sketchy, and P Vent were covered by lava in 2005/2006 and vent fauna that were present pre-eruption such as large Riftia and the associated meio- and macrofauna was disturbed: biomass was removed as it was covered with lava. In late 2006 predominantly new Tevnia tubeworms were established at these sites. Tubeworm communities were visually dominated by Tevnia tubeworms, but Riftia and Oasisia were also present in small numbers (Schimak et al., 2012). Data on active vent communities are published elsewhere (Gollner et al., 2015b). We observed high temporal variation in vent fluid emissions within these active sites during the studied period. Settlement substrates that had been deployed at Sketchy within the Ty-Io area were exposed to no vent activity in 2007 and 2009. The Sketchy patch became a small senescent vent (with dead Tevnia tubes and no temperature anomalies) although the Ty-Io area overall remained active. Similarly, our studied tubeworm patch at P Vent was found to be senescent in 2009, although the site in general remained hydrothermally active. Data on senescent vent patches from Sketchy and P Vent are shown in this manuscript. We also observed similar patch dynamics—tubeworm patches locally become senescent within an active vent site—at the Tica Vent site (Klose et al., 2015).
Faunal Communities at Senescent Vents
At senescent vents, we encountered on average 1145 animals per settlement device (64 cm–2), with 968 meiofauna and 178 macrofauna individuals. Abundances were variable and ranged from 38 to 8020 individuals. The highest animal abundances were observed at the 4 yearlong hydrothermal inactive East Wall site, the lowest abundances at 2 yearlong hydrothermal inactive Sketchy site (Table 1). The mean abundances per settlement device at senescent communities at East Wall and Q Vent, which were active for > 12 years before venting waned, were 2330 ± 2995. Mean abundances at senescent Sketchy and P Vent, which had been active for < 3 years, were 223 ± 162. All encountered abundances at senescent vents were similar to active vents (all p = ns). Active vents had a mean abundance of 869 animals per settlement device (64 cm–2), with 258 meiofauna and 610 macrofauna individuals (data from Gollner et al., 2015b). The mean relative abundance of meiofauna juveniles was high at senescent vents (42%) and was higher, but not significantly compared to active vents (23%) (p = ns). The mean relative abundance of macrofauna individuals < 1 mm was very high with mean 78% at senescent vents, and was lower, although also not significantly (p = ns) at active vent sites (mean 30%).
In our entire sample collections within the Axial Summit Trough (AST) (including vent and basalt samples with ∼175,000 individuals from pre-eruption and ∼217,000 individuals from post eruption; this study and data from Gollner et al., 2010, 2015a), we detected a total of 189 species, including 76 macrofaunal species (13 singletons) and 113 meiofaunal species (42 singletons). Senescent vents contributed significantly to these numbers with ∼100,000 counted individuals, containing 55 macrofaunal species (11 singletons) and 74 meiofaunal species (19 singletons). Of these 129 species, 60 are not reported from active vents.
The macrofaunal singletons, species that were only encountered with one specimen in our sample collections, at senescent vents were mostly unidentified polychaetes, amphipods, hydrozoans, isopods, gastropods, ophiorids and include potentially new species such as for example Archinome sp. nov. 1. Dominant macrofaunal species at senescent vents were Ophyrotrocha akessoni, Lepetodrilus elevatus, Ventiella sulfuris, Prionospio sandersi, Lepetodrilus tevnianus, Amphisamytha galapagensis, Hesospina vestimentifera, and Archinome rosacea. All these species occur also in high abundances at active vents.
The meiofaunal singletons at senescent vents were mostly unidentified nematodes, copepods, and foraminifera species. From the 19 singletons, 16 were distinguished within our own sample collections as distinct species but were not further studied, and three could be classified as known or new species: the copepod Mesocletodes elmari, the copepod Ferregastes sp. nov.1, and the nematode Halomonhystera sp. nov. 2. The species Thalassomonhystera sp. nov. 11 occurred four times and was only detected at the senescent East Wall site. The kinorhynch Desmodasys abyssalis was known from the active Tica Vent pre-eruption and occurred in high abundances (total > 100 individuals) at the senescent East Wall site. Most dominant meiofauna at senescent vents were the foraminifera Abyssotherma pacifica, the harpacticoid copepods Amphiascus aff. varians, Ameira sp. nov. 1, and Tisbe sp. nov.1, the nematodes Neochromadora aff. poecilosoma and Thalassomonhystera fisheri and the ostracod Xylocythere vanharteni. All the dominant species from senescent vents also occur at active vents (in varying abundances). The active vent dominating dirivultid copepods (e.g., Aphotopontius acanthinus) occurred only in low abundances at senescent vents.
All ANOSIM results from senescent vent samples (considering distinct time and sites) were not significant due to low number of permutations and due to high sample variability. ANOSIM results based on groupings per year while neglecting a possible effect of different sites showed a significant change in meiofaunal community at senescent vents in all years (2006–2007: R = 0.549 with p = 0.036; 2007–2009: R = 0.353 with p = 0.026) and a significant change in the marcofauna community only from 2006 to 2007 (2006–2007: R = 0.959 with p = 0.018; 2007–2009: R = 0.137 with p = ns). SIMPER revealed that similarity at senescent vents was ranging from 63 to 75% in distinct years (Table 2). Species contributing most to similarity in 2006 and 2007 were the copepod Amphiascus aff. varians and the polychaete O. akessoni and in 2009, the copepod Ameira sp. 1. and the foraminifera A. pacifica.
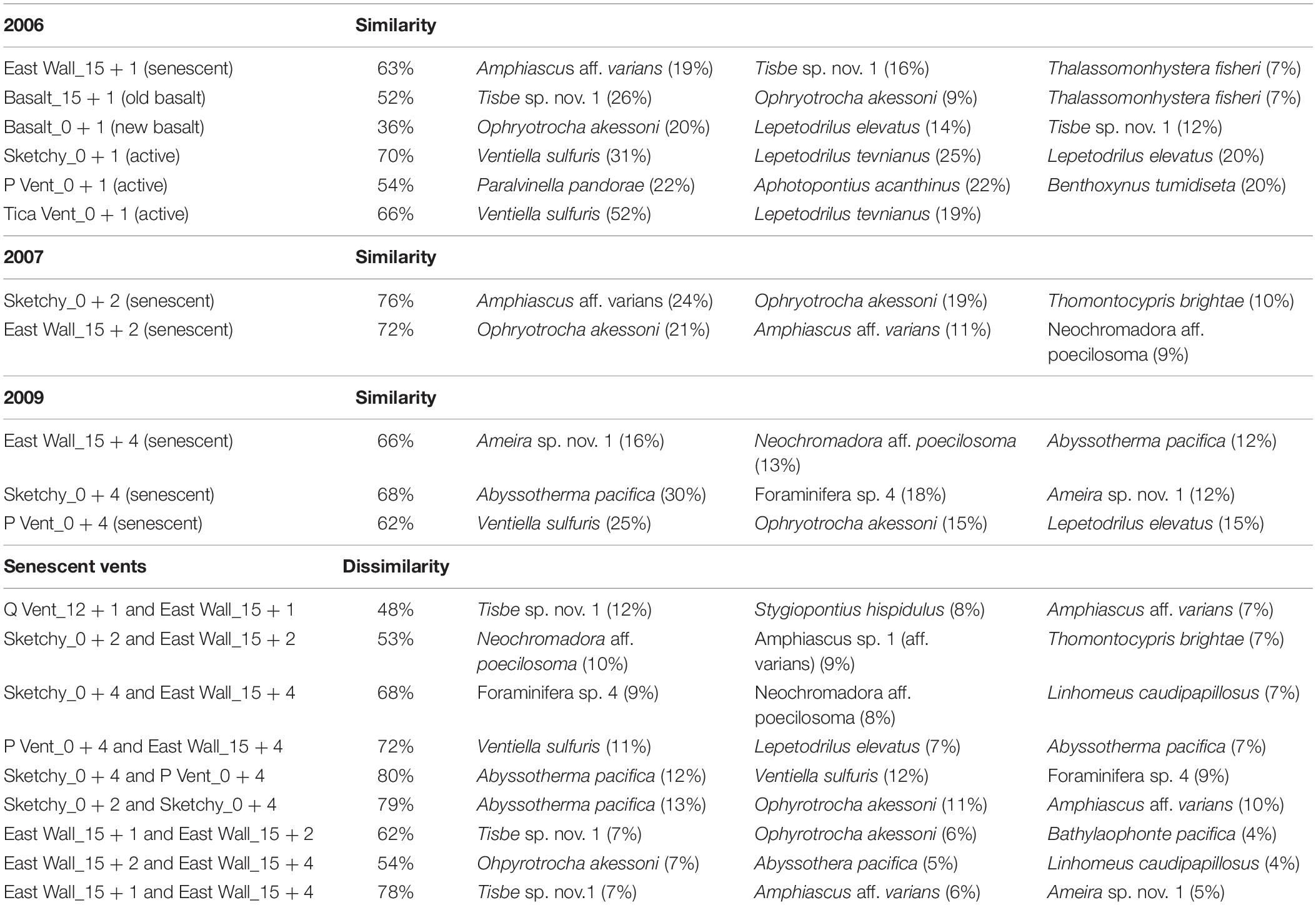
Table 2. SIMPER similarity and dissimilarity including three species that contributed most to (dis)similarity from Q Vent, East Wall, Bio9 Vent, and surrounding old basalt, as well as from senescent patches at Sketchy and P Vent.
Faunal Communities Not Covered by Lava After the Eruption in 2006
The sites that were not covered by lava in early 2006 (old active Bio9 Vent, senescent Q Vent, senescent East Wall, and the neighboring old basalt) harbored between 26 and 315 individuals per 64 cm2 in late 2006. In total 46 species were found in these samples; 13 of these 46 species were not detected at new vent sites or new basalt (Table 3, species in bold); 33 of the 46 species occurred at old and newly formed vent sites and basalt, and most of them were detected in typically high abundances in late 2006 (Table 3, active vent data from Gollner et al., 2015a, b). Several species that were detected in these 2006 samples were not detected in samples from pre-eruption. These included rare species like the copepod M. elmari, but also abundant species like the limpet L. tevnianus or the copepod Tisbe sp. nov.1.
Bubble plots in the nMDS visualize that animals occur in different abundances at distinct sites in 2006 (Figure 3). The vent endemic dirivultid copepod A. acanthinus was highly abundant at old active Bio9 Vent. The meiofauna species X. vanharteni (ostracod) and T. fisheri (nematode) occurred predominantly at senescent vent sites and old basalt. The harpacticoid copepod Amphiascus aff. varians was present at old and new sites. Vent endemic macrofauna species like the limpet L. tevnianus and the amphipod V. sulfuris occurred in high abundance at new vent sites.
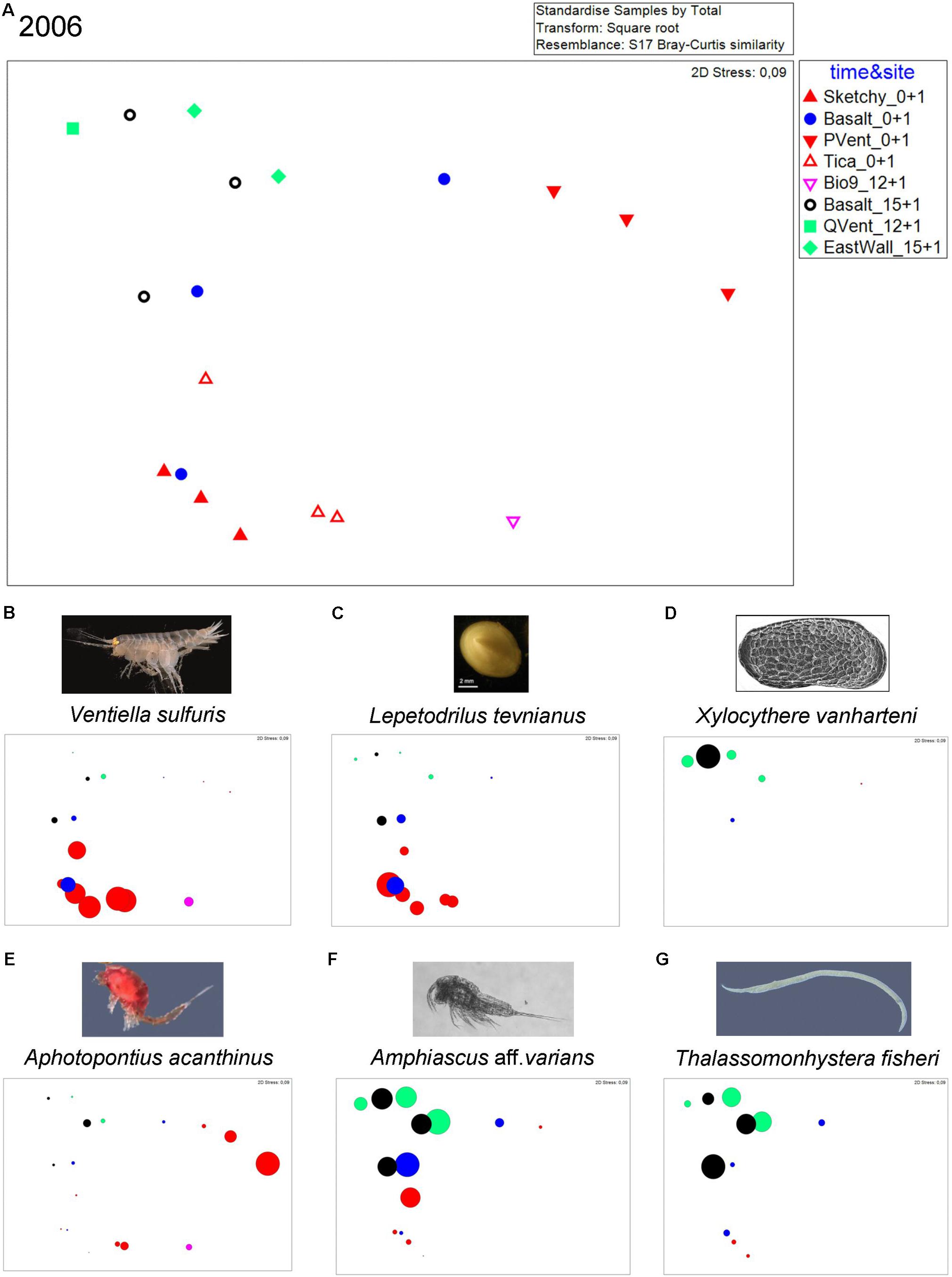
Figure 3. (A) nMDS plot for samples from sites collected in the year 2006: old active Bio9 Vent (pink) that was not directly affected by the volcanic eruption, senescent East Wall and Q Vent (green), old basalt that was not covered by lava (black), new basalt (blue), newly active vent sites Tica Vent, Sketchy and P Vent (red). (B–G) Bubble plots showing relative abundance of species at the different sites. (B) Amphipod Ventiella sulfuris (photograph © Bright), (C) limpet Lepetodrilus tevnianus [image of limpet L. tevnianus reprinted by permission from Springer Nature, Marine Biology, Bayer et al. (2011)], (D) ostracod Xylocythere vanharteni [image provided by Rosalie F. Maddocks (Maddocks, 2005], (E) dirivultid copepod Aphotopontius acanthinus (image Aphotopontius sp. © Bright), (F) harpacticoid copepod Amphiascus aff. varians (image Amphiascus sp. © Gollner), and (G) nematode Thalassomonhystera fisheri (image Thalassomonhystera sp. © Bright). Numbers after site names indicate age of communities: Sketchy 0+1: “0” indicates no animal community from prior eruption because Sketchy was covered by lava; “+ 1” indicates that the sampling occurred 1 year after the eruption and that the community was ∼1 year old; East Wall 15+ 1: “15” indicates that the community from prior eruption that was not paved by lava and was ∼15 years old in 2005; “+ 1” indicates that the sampling occurred 1 year after the eruption. Total age the East Wall community was ∼16 years at the time of sampling in 2006.
In 2006, similarity at the senescent vents and on old and new basalt was low and ranged from 36 to 63% with the meiofaunal species Tisbe sp. nov. 1 contributing most to similarity. Similarity within new active vents ranged from 54 to 70% with macrofaunal species V. sulfuris and L. tevnianus contributing most to similarity (Table 2) (Gollner et al., 2015b). ANOSIM results were not significant (p > 0.1) allowing no interpretation of R values which ranged from 1 (highly different) to 0 (similar). The nMDS plot (Figure 3) shows loose grouping of senescent East Wall, senescent Q Vent, and old basalt, as well as grouping of the new active vents Tica Vent, Sketchy, and new basalt in 2006. Communities from P Vent, and Bio9 Vent which both were exposed to the most extreme temperature and chemical regime of vent fluid emissions also formed own groups.
Meio- and Macrofaunal Trends in Community Succession
Considering all 67 samples from senescent vents, active vents, and basalt from pre-eruption (2001–2004) and from post eruption (2006, 2007, 2009) two large-scale trends emerge when looking at meio- and macrofaunal community patterns related to time and productivity regimes (Figure 4). The overlays shown in the nMDS plot point to a change in meiofaunal communities over time (Figure 4C) and ANOSIM proofed that meiofaunal communities were typically significantly dissimilar in different years (R = 0.42–0.631; Table 4). In contrast, there was no grouping according to habitat for the meiofauna (active vent, senescent vent, basalt; Figure 4A) and ANOSIM revealed that meiofaunal communities from active and senescent vents and basalt are all similar to each other although this was not significant (R = 0.019–0.142; p = 0.083–0.39; Table 4). The macrofaunal communities are grouped according to productivity (active vent versus basalt and senescent vent; Figure 4B) and ANOSIM for macrofaunal communities showed slightly higher R values (more dissimilar) for the factor of habitat (active vent, senescent vent, basalt) and little lower R values (more similar) for the factor time (Table 4). The time overlay in the nMDS plots for macrofauna (Figure 4D) does not show a clear trend of change of macrofaunal communities over years. ANOSIM R values were always higher (thus more dissimilar) for the meiofauna than for the macrofauna when considering the influence of years, while R values were always higher for the macrofauna than for the meiofauna when considering habitat.
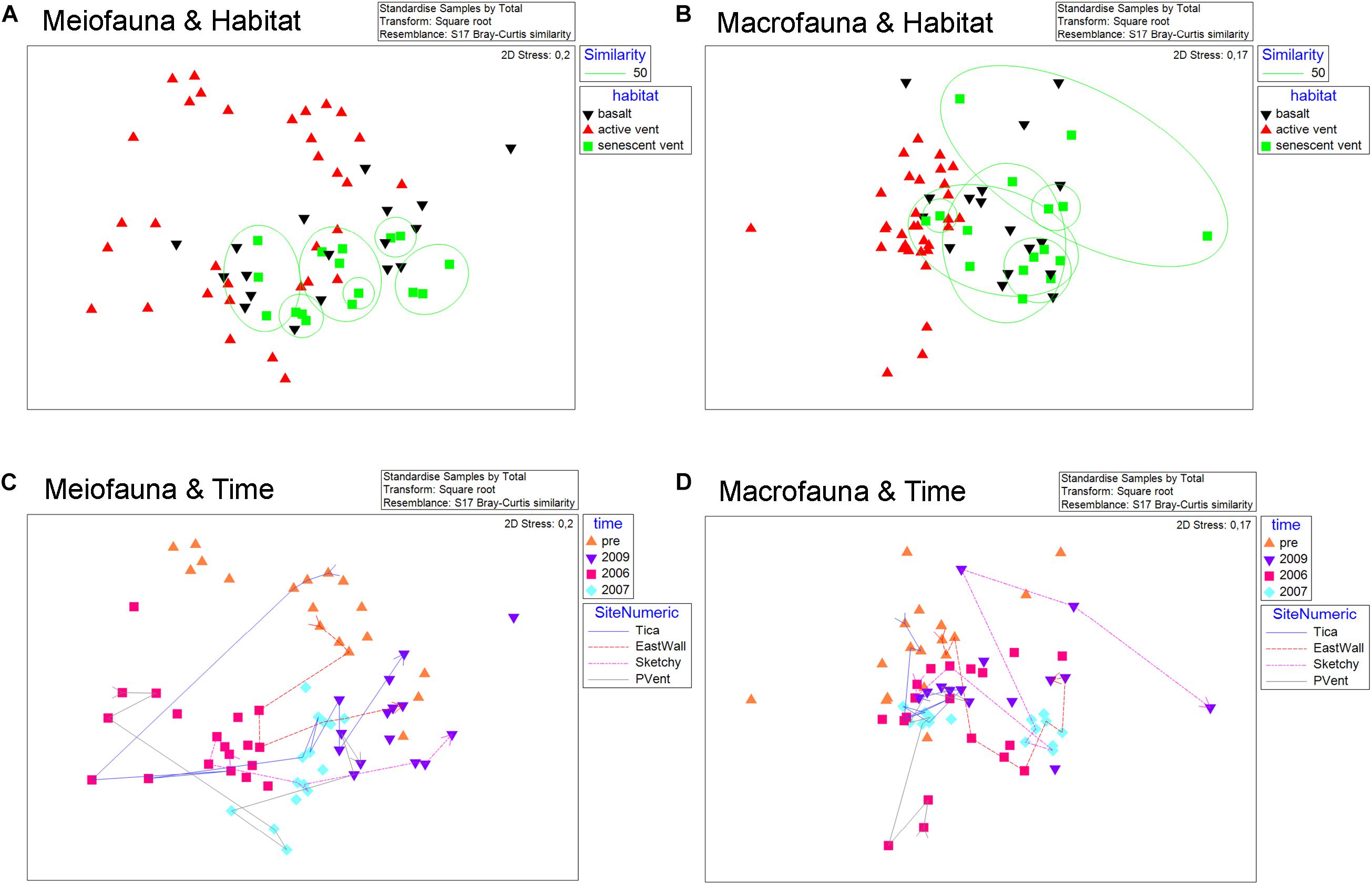
Figure 4. nMDS plots for meiofauna (left) and macrofauna (right) of all active vent, senescent vent, and basalt samples pre- and post eruption analyzed in this study and by Gollner et al. (2015a; b). (A,B) Meiofauna and macrofauna nMDS with color codes according to habitat (active vent, senescent vent, basalt). Overlay for similarity percent (50%) shows no grouping of sites according to habitat for the meiofauna (A) but for the macrofauna (B). (C,D) Meiofauna and macrofauna nMDS with color codes according to years (pre-eruption, 2006, 2007, 2009). Overlay for numeric sites indicates position change (and thus similarity) of distinct sites over years for East Wall, Tica Vent, Sketchy, and P Vent. The meiofauna communities change their position on the MDS rather uniformly from top/left against to clock to the right side of the nMDS, according to year of collection (C). For the macrofauna communities, no such uniform change of similarities over time can be seen (D).
Discussion
Senescent Vents — A Species Rich Ecotone
Senescent vents at the 9°N EPR harbor at least 55 macrofaunal species and 74 meiofaunal species, supporting the idea that ecotones act as taxonomic diversity hotspots (Kark and van Rensburg, 2006). At the senescent vents, we find a biodiversity rich community of typical active vent fauna, and of species that are known from the background. This is in accordance to earlier observations by Tsurumi and Tunnicliffe (2003) who studied fauna from five senescent vent samples at Juan de Fuca Ridge. They detected 49 species of which 20 were non-vent species (Tsurumi and Tunnicliffe, 2003), while at the 9°N EPR, we report 129 species of which 60 were non-vent. From these two studies, one may conclude that about half of species at senescent vents are associated with vent environments and the other half from non-vent environments. Similar, Van Dover (2002) observed a mix of vent and non-vent fauna at a senescent mussel bed. These authors attributed this observation to the decrease in the levels of toxic sulfide that may allow non-vent taxa to invade the senescent vent (Van Dover, 2002). Whether these non-vent fauna are background fauna or fauna restricted to senescent vents cannot be judged currently due to paucity of data from both environments. For example, one non-vent species that was detected in our study at senescent East Wall but never at active vents is the copepod M. elmari, a species with broad abyssal distribution (Menzel, 2011). However, most non-vent species at mid-ocean ridges are new to science and are not yet described.
At our current stage of knowledge, it remains difficult to compare senescent vents, where communities can rely on megafauna biomass previously produced by in situ chemosynthetic primary production, to old inactive vents. Old inactive vents, or hydrothermal sulfide deposits, can be tens of thousands years old and could also produce bacterial biomass by local chemosynthetic activity (Le Bris et al., 2019). To our knowledge, studies at older inactive vents considered only visually observed megafauna. Old inactive chimneys typically host sessile taxa such as sponges, cnidarians including corals, and echinoderms (Tunnicliffe et al., 1986; Galkin, 1997). At Manus Basin, inactive chimneys were characterized by Vulcanolepas cf. parensis, Munidopsis spp., hydroids, Abyssocladia dominalba, and Keratoisis sp. (Erickson et al., 2009). Boschen et al. (2015) found that megafaunal assemblages on inactive, sulfide-rich chimneys may be distinctive to those found on chimneys of active vents and to hard substrates on seamounts with no current or past hydrothermal activity. To our knowledge, meio- and macrofauna associated with these old inactive vent megafauna has to date not been investigated and thus we cannot compare data to senescent vents.
Senescent Vents May Provide Food for an Abundant Small Sized Fauna
Dead megafauna at senescent vents at the 9°N EPR likely provide nutrition for an abundant meio- and macrofauna consumer community. Even in the small (less than a few square meter sized) patches of decaying tubeworm tubes at East Wall, up to more than 6000 mostly meiofaunal individuals could be found on an area of 64 cm2 (size of colonization substrates that have been investigated in this study). In theory, up to one million small sized animals could thus live in 1 square meter of decaying vent megafauna. Interestingly, the highest meiofauna abundances were encountered in the oldest, namely, 4 yearlong hydrothermally inactive, senescent vent, where visually no remaining tubeworm tube could be observed, but instead fluffy organic matter was present (see Figure 2, East Wall site in 2009). Potentially this old more highly degraded material may be used more efficiently by the meiofauna than the younger less degraded tubes. In coastal areas, meiofauna abundance and diversity is known to be influenced by food quality and quantity (van der Heijden et al., 2018). We have not quantified or qualified the nutritional link from the old dead megafauna to the living animals by isotopes but the observed high meiofaunal abundances point out that such a link may be likely.
Other potential pathways of energy transfer include horizontal advection of particulate organic material derived from vents to peripheral areas (Levin et al., 2016) and may have also contributed to the high meiofauna abundances found at senescent vents at the 9°N EPR. Erickson et al. (2009) showed that for example, bamboo corals and other non-vent organisms from old inactive vents at Manus Basin had sulfur isotopic compositions that are consistent with a diet of particulate organic material derived from microbial chemoautotrophy (Erickson et al., 2009). Also anemones and serpulid worms in close vicinity to vents feed on chemoautotrophically derived particulate organic matter (Hessler et al., 1988; Levin et al., 2016). The distance of the nearest active vent site to senescent East Wall was 250 m. We cannot tell with our analyses if horizontal advection plays an important role but it is likely to be significant within the axial valley were bottom currents can be strong and particle export known to be significant (Adams et al., 2011; Thurnherr et al., 2011).
Release of gametes and larvae is a yet unquantified source of export of particulate carbon from chemosynthetic ecosystems, given the high fecundity of many vent taxa with dispersive larval stages (Tyler and Young, 1999; Adams et al., 2011; Levin et al., 2016). Continuous and high presence of vent macrofauna < 1 mm in body size at the studied senescent vents shows that larvae of vent macrofauna can settle and/or grow at senescent vents in high numbers. The low proportion of macrofauna > 1 mm is extraordinary and shows that it is currently not clear if and to what extant/how long typical vent macrofauna can reproduce at senescent vents. There are three potential scenarios: (1) The macrofauna at senescent vents can reproduce and offspring may settle at the same site. (2) There is continuous and abundant transfer of gametes and larvae originating from active vents toward the senescent vents. These larvae can settle and grow only to a limited size/biomass before they die. (3) The senescent vents may act as a nursery area and larger mobile individuals eventually migrate to food-richer areas (active vents) at a late juvenile/young adult age.
Body size related food demands could cause demographic pattern biased toward small sized vent macrofauna at senescent vents, similar to what was suggested for basalt in the periphery of active vents (Gollner et al., 2015a). The specialized vent macrofauna could be food limited at senescent vents and on basalt and must migrate into the active vent environment to develop into adults and reproduce. Meiofaunal species at senescent vents do not have such a strong bias toward juveniles. All live stages including juveniles, and adult females carrying eggs and males carrying spermatophores have been detected. Where the particulate carbon of juvenile macrofauna from senescent vents is used/ending up is currently unclear. Macrofauna may leave senescent vents in the search for more food at active vent sites or may die and become food for meiofauna.
While small macrofauna individuals (< 1 mm) were common at senescent vents, hardly any small juvenile symbiotic megafauna recruits have been detected at senescent vents. Symbiotic megafauna may have very different settlement cues compared to non-symbiotic macro- and meiofauna. Instead of food they mostly rely on hydrothermal fluid emissions for their symbionts (Van Dover, 2000). In total we detected only 10 individuals of Bathymodiolus mussels and tubeworms < 1 mm in all of our senescent samples. This in stark contrast to the thousands of small vent macrofauna individuals (for example, limpets Lepetodrilus). The almost absence of symbiotic megafauna larvae recruits is in accordance with the finding of Van Dover (2002) who observed lack of post-juvenile mussels at the senescent Animal Farm site at the EPR. These findings may point to different settlement cues of symbiotic and non-symbiotic fauna. We speculate that symbiotic fauna may use, for example, chemical cues, while macro- and meiofauna may settle more randomly or use other/additional cues.
Recovery After Disturbance: Local Remnants and Regional Dispersal Networks
Natural disturbances are rarely absolute and not all individuals die. More often, some individuals survive within or near a disturbed area and influence recolonization (Platt and Connell, 2003). This type of historical influence occurs in a variety of terrestrial and marine habitats. Our study has shown that even though the spatial scale of areas not covered by lava was small (several m2 compared to several km2 lava paved area), the importance of remnant fauna in these areas for community recovery cannot be underestimated. The active vent Bio9 Vent and the two senescent vents East Wall and Q Vent were not paved by lava at the 9°N EPR and harbored at least 33 species in 2006 that potentially may have directly colonized the close by emerging vent sites, where the same species were detected. Larval supply and migrants after the volcanic eruption thus very likely came from local as well as regional sources (Figure 5).
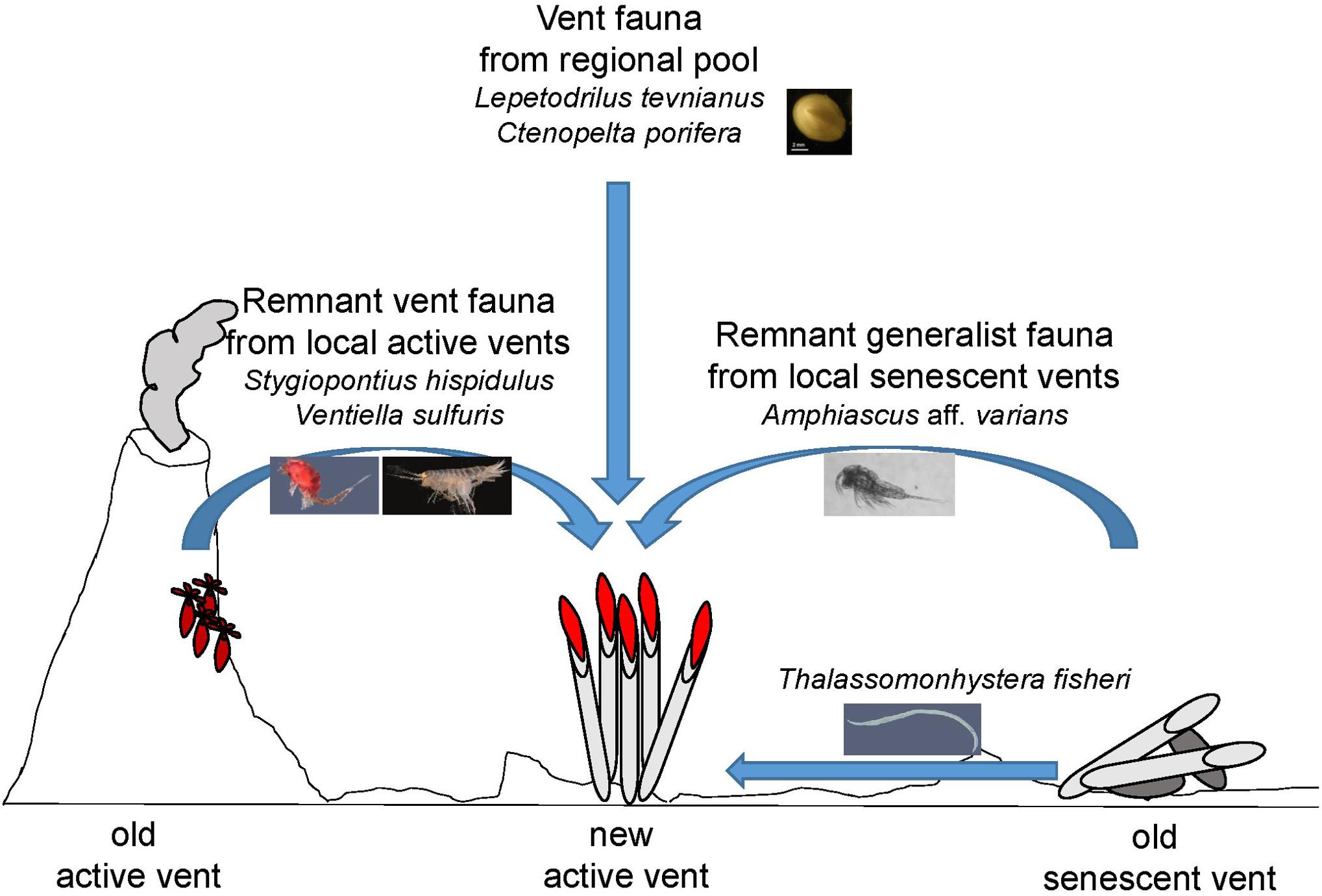
Figure 5. Concept of meio- and macrofauna colonization at new active vent sites after the volcanic eruption from local as well as from regional pool at the 9°N EPR. Vent megafauna like sessile Tevnia and semi-sessile macrofauna like the limpets Lepetodrilus tevnianus and Ctenopelta porifera must have arrived as larvae from a regional pool since these species were not present in the area pre-eruption. Mobile macrofauna like the vent amphipod Ventiella sulfuris and vent specialized dirivultid copepods like Stygiopontius hispidulus were present at the local active Bio9 Vent smoker that was not covered by lava during the eruption. These remnants may have migrated either as larvae and/or free-swimming as adults from this old local active vent source to the new active vent sites. Generalist fauna like harpacticoid copepods such as Amphiascus aff. varians (having nauplii) and like nematodes such as Thalassomonhystera fisheri (lacking larval dispersal stages) were abundant at the senescent vents not covered by lava. The remnant meiofauna may have populated the new vents sites via crawling or swimming/drifting from the local senescent vents and old basalt areas. Many meiofauna species are generalists and can live on basalt, at senescent vents and at active vents. Typical vent macrofauna species are also found on basalt and at senescent vents, but often remain small (and unfertile?) in body size.
The distinct relative abundances of species in our 2006 samples at active and senescent vents (Figure 3) let us speculate that animals may have very different strategies to recover from disturbance. Animals living at hydrothermal vents are characterized by very different life traits such as mode of dispersal or nutritional needs (Gollner et al., 2015a). The absence of the limpet L. tevnianus and C. porifera before the eruption at 9°N EPR and their high abundance at the new vent sites showed that larvae from afar can colonize vents after a volcanic eruption (Mullineaux et al., 2010). Adult limpets have limited mobility and are known to produce high numbers of lecithotrophic larvae that are abundant in the water column above vents (Mullineaux et al., 2005, 2010). The finding of these species at senescent vents in 2006 shows that vent macrofaunal species can settle in any habitat, although in different abundances and/or with different survival rates. Another successful early colonizer was the amphipod V. sulfuris. In contrast to the semi-sessile limpets, this species can swim at a speed of ∼5–10 cm–1 and is a brooder with lecithotrophic larvae and has moderate gene flow (France et al., 1992; Kaartvedt et al., 1994; Vrijenhoek, 2010). Ventiella may have swum from Bio9 Vent toward the new vent sites. Dirivultid copepods, like A. acanthinus or Stygiopontius hispidulus were abundant at new vents. Dirivultids are crawlers and have rather limited swimming capabilities (Heptner and Ivanenko, 2002). Dirivultid adults occur rarely in the water column, but their juvenile copepodite stages are the most abundant specimens among all vent animals encountered in pelagic pump samples with ∼50 ind. per 1000 L at 3 m above vents (Gollner et al., 2015b). Their presence at the sites not covered by lava and their high dispersal abilities may have allowed them to very quickly invade the new vent sites. In contrast to the dirivultid copepods, harpacticoid copepods have more eggs but their copepodites are much less abundant (< 1% relative abundance) in the waters above vents (Gollner et al., 2015b). However, their high abundance at senescent East Wall and Q Vent as well as on old basalt may have helped the harpacticoids to colonize the new vent sites relatively quickly. On top, the leg morphology trait of the most successful harpacticoids, having a prehensile P1 endopod and seven to eight setae on terminal segments of podia (Bell et al., 1987; Thistle and Sedlacek, 2004), show enhanced migration potential for these species. Nematode abundance and richness were extremely low at all studied active sites in late 2006 and increased only slightly by 2009, although nematodes were present at senescent East Wall, Q Vent, and old basalt (Gollner et al., 2013). The limited dispersal abilities of nematodes, including lack of larvae, extremely rare occurrence of adults in the water column above vents, and limited adult mobility might have delayed their establishment at new active vents and new basalt.
To conclude, species at vents may have developed different strategies to cope with extreme disturbance events and patchy distribution of productivity rich but environmentally challenging vent environments (Figure 5). Strategies may include: (1) new vent colonizers arrive as larvae from a regional pool. Such species may be characterized by long-distance dispersal capabilities, as seen for typical vent mega- and macrofauna species as well as dirivultid copepods; (2) remnant vent fauna arrive via migration or larval dispersal from local vents not paved by lava; and (3) remnant fauna with or without larvae migrate from local senescent vents toward new active vents. Many generalist meiofauna species including harpacticoid copepods or nematodes may use this strategy as they have limited larval dispersal.
Meio- and Macrofaunal Trends in Community Succession at the 9°N EPR
Primary succession—the non-seasonal, directional continuous pattern of colonization and extinction (Begon et al., 1990)—is typically initiated by formation of new habitat, such as from lava flow caused by a volcanic eruption. The post-disturbance landscape at the 9°N EPR consists of mosaics of patches, including old and new active vents, senescent vents, and old and new basalt. Metapopulations at vents are shaped by different processes and different scales, including disturbance, local controls (influence of habitat and biological interactions), and regional controls (larval exchange, connectivity) (Mullineaux et al., 2018).
Regional controls, i.e., larval dispersal and supply, have strong influence on the primary succession, because they determine which species are able to arrive first and eventually become established. The arrival of the gastropod pioneer colonists Ctenopelta porifera, an immigrant from possibly more than 300 km away, and L. tevnianus, a species that occurred in low abundance prior eruption, demonstrated that larval supply can change markedly after removal of local source populations at the 9°N EPR (Mullineaux et al., 2009). However, we did not detect a clear directional continuous pattern of colonization for the macrofauna during our study period (see Figure 4D), which may be linked to the fact that larval supply of some species may be continuous while others arrive only episodically (Adams and Mullineaux, 2008; Mullineaux et al., 2012; Mills et al., 2013). In contrast, meiofaunal succession was very likely shaped by dispersal potential of species, with rapid recovery of dispersive copepods and slow recovery of nematodes which lack pelagic dispersal stages (Gollner et al., 2013, 2015b). In this study, we could further show that larval supply after a volcanic eruption is not restricted to regional controls, but local remnant fauna may be crucial for colonization (see section “Recovery After Disturbance: Local Remnants and Regional Dispersal Networks”).
Local environmental conditions at active vents can be extreme, and distribution and zonation of mega-, macro-, and meiofauna are shaped by vent fluid conditions. At the 9°N EPR, with increasing temperature and toxic hydrogen sulfide concentrations and increasing amplitude of variation of these factors, fewer species are able to cope with these extreme conditions, resulting in typically less diverse communities in more extreme habitats (Galkin and Goroslavskaya, 2008; Gollner et al., 2010, 2015a). Changes in community composition after major disturbance coincide with changes in vent flow conditions, as at the 9°N EPR hydrothermal fluid emissions typically wane within a few years after an eruption (Shank et al., 1998; Le Bris et al., 2006) and species diversity tends to increase (Mullineaux et al., 2012; Gollner et al., 2015b). Manipulative colonization experiments at the 9°N EPR showed that successional mechanism of macrofauna varies along a gradient in hydrothermal fluid flux. Inhibition of later colonists by early mobile colonizers was found to be predominant in tubeworms areas with relatively higher vent fluid flux. Facilitation of later colonists by early sessile colonists played an important role in the suspension feeder zone with relatively lower vent fluid flux (Mullineaux et al., 2003). Biological controls are yet unknown from senescent vents and basalt. Facilitation and inhibition for the meiofauna remains to be determined at active and senescent vents as well as on basalt, but in general, it can be expected that mega- and macrofauna do structure meiofaunal communities (and the other way round) (Ólafsson, 2003).
Local environmental conditions at senescent vents may be experienced very differently by meio- and macrofaunal metapopulations as faunae differ in energy requirements. Stopping of vent fluids causes death of symbiont-bearing vent megafauna, as the direct source of energy for the symbionts is cut off. One to 4 years after vent fluids had stopped, we encountered a predominantly juvenile marcofaunal community and a highly abundant and reproductive meiofaunal community at the studied senescent vents (see sections “Senescent Vents—A Species Rich Ecotone” and “Senescent Vents May Provide Food for an Abundant Small Sized Fauna”). Our MDS plot shows that macrofaunal communities are mainly grouped according to distinct productivity regimes (active vent, senescent vent, basalt) and not according to year within our study period (Figures 4B,D). This may be related to macrofaunal habitat fidelity patterns. Macrofaunal species occur primarily at active vents and are typically restricted (endemic) to this habitat (Van Dover, 2000; Gollner et al., 2015a). In contrast, meiofaunal species are not restricted to active and senescent vents but also occur on basalt (Gollner et al., 2015a; Plum et al., 2017). This is shown by our MDS (Figure 4A), where meiofaunal communities at active vents, senescent vents, and basalt are rather similar. The contrasting habitat fidelity patterns of a vent endemic macrofauna and a generalist meiofauna, which may be evolutionary driven by distinct food demands and reproduction strategies inherent to fauna of different sizes (Gollner et al., 2015a), may shape succession of AST communities.
Overall, our study suggests that size class specific traits may influence AST communities’ succession after major disturbance. Meiofaunal communities were predominantly structured over years by the apparent distinct dispersal abilities of meiofauna taxa, with good copepod dispersers and poor nematode dispersers (Gollner et al., 2015b). This was found independent of habitat type in the AST, including basalt, senescent vent, and active vents. Productivity regimes appeared highly distinct in these habitats (see prokaryote abundances in Supplementary Material S1), but we did not detect significant meiofaunal community dissimilarities. In contrast, macrofaunal communities’ dissimilarities were higher between habitats of different productivity regimes than between different years. Productivity may set the limits for vent macrofauna distribution (Mullineaux et al., 2009).
Relevance for Deep-Sea Mining
There is an increasing interest in mining minerals at deep-sea hydrothermal vents. First, mining tests have been carried out in the national waters of Papua New Guinea and Japan and seven contractors have signed contracts for mineral exploration of seafloor massive sulfides in international waters with the International Seabed authority (ISA) (Gollner et al., 2017). The ISA is currently drafting a Mining Code, including environmental regulations, for the exploitation of minerals in international waters. In just a few years’ time, mining in the deep-sea might become a legal reality. Potential long-lasting impacts of seabed mining emphasize the need for effective environmental management plans (Cuvelier et al., 2018; Tunnicliffe et al., 2018). Such plans should include efforts to mitigate deep-sea mining impact such as avoidance and the designation of undisturbed areas (refuges). A recent publication argued that active hydrothermal vent ecosystems shall be excluded from deep-sea mining at all (Van Dover et al., 2018).
Deep-sea mining will cause large-scale disturbances and will kill animals. Our study shows that many species at vents may not be adapted to complete destruction of their populations. Even the large-scale volcanic eruption at the 9°N EPR in 2005/2006 did not pave all areas with lava. The elevated areas—like smoker structures at Bio9 Vent and Q Vent or the elevated East Wall site harbored many remnants that may have played crucial roles for recovery of communities on lava paved areas. Recovery of several (many?) species at new vents and new basalt may depend on survival at local non-disturbed areas and local migration. These findings should be integrated into future models of dispersal networks. Suzuki et al. (2018) demonstrated a population dynamic model estimating resilience of vent communities by selecting for one species with larval dispersal in the West Pacific. Ideally, in the future, such models should also integrate species with non-larval dispersal. To summarize, mining of entire vent sites could have even more dramatic impact than volcanic eruptions. Hydrothermal vent communities may not be adapted to complete disturbance and local remnants may play pivotal roles for recovery after disturbance.
Conclusion
Entirely senescent vent sites like Q Vent and East Wall as well as senescent vent patches within active vent sites like Sketchy and P Vent occur regularly at the 9°N EPR and harbor a species rich mix of species from active vents as well as rare and mostly yet undescribed species. Our findings are in accordance with Mullineaux et al. (2009) whose experimental results indicated that abrupt environmental change at deep-sea vents does not necessarily result in elimination of existing macrofauna and rapid replacement but instead an imprint may persist for many months after the disturbance. Also, Van Dover (2002) and Tsurumi and Tunnicliffe (2003) found vent macrofauna and background fauna at senescent vents. We detected an abundant and reproductive meiofaunal community including adults and juveniles at senescent vents that have not been exposed to hydrothermal venting for up to 4 years. It is not clear yet if and to what extant/how long typical vent macrofauna can reproduce at senescent vents, and if the presence of a predominantly juvenile macrofauna results from continuous settlement from active vents or from in situ reproduction.
After the volcanic eruption in 2005/2006, remnants from small areas that were not paved by lava, including two senescent vent sites and one active vent site, may have provided source populations for the close by new emerging vent sites. In addition, vent animals known as early colonizers originating from remote areas after the volcanic eruptions, such as the limpet L. tevnianus, could settle at senescent vents and new active vents, pointing to settlement processes that do not solely rely on the presence of hydrothermal fluid flux. Meiofaunal community dynamics during early succession at the 9°N EPR seem to be driven by distinct dispersal capabilities of different higher taxa such as copepods and nematodes, while macrofaunal community dynamics may be more linked to productivity/vent fluid regimes. Vent meio- and macrofauna have fundamentally different life traits that may determine their success (in terms of abundance, diversity, and reproduction) to live at senescent vents and on basalt with no direct in situ primary production. Their intrinsic reproduction and dispersal strategies further influence mode of recovery after major disturbance. Remnant individuals after disturbance may be especially important for meiofauna recovery since they have rather limited dispersal abilities but may profit from higher habitat flexibility related to lower food demands, enabling them to live and reproduce at senescent vents and on basalt. Different modes of animal dispersal and habitat flexibility need to be integrated into future spatial management strategies on environmental protection with regard to future deep-sea mining at vents. Also, it has to be considered that hydrothermal vent communities may not be adapted to complete disturbance and mining of entire sites could have even more dramatic impact than volcanic eruptions.
Data Availability Statement
Data are presented in the article and in the Supplementary Material.
Author Contributions
SG designed the project, identified animals, analyzed the data, and wrote the manuscript. MB designed the project and edited the manuscript. BG and PM identified animals. LM provided samples, advised on experimental design, and edited the manuscript. SM and TS provided samples and edited the manuscript. NL measured chemistry and edited the manuscript. MW measured prokaryote abundances.
Funding
We received funding from the Austrian FWF (GrantP20190-B17; MB), the U.S. National Science Foundation (OCE-0424953; to LM, D. McGillicuddy, A. Thurnherr, J. Ledwell, and W. Lavelle; and OCE-1356738 to LM), and the European Union Seventh Framework Programme (FP7/2007-2013) under the MIDAS project, Grant Agreement No. 603418. Ifremer and CNRS (France) supported NL cruise participation and sensor developments. BG was supported by a postdoctoral fellowship from the Deep Ocean Exploration Institute at WHOI (United States). TS was supported by the U.S. National Science Foundation (OCE-0327261 to TS and OCE-0937395 to TS and BG).
Conflict of Interest
The authors declare that the research was conducted in the absence of any commercial or financial relationships that could be construed as a potential conflict of interest.
Acknowledgments
The authors thank the crew of R/V Atlantis and DSV Alvin, and scientists on board for their tremendous support during cruises. Special thanks to Ingrid Kolar and Salvador Espada Hinojosa (technicians involved in numerous parts of this project), Sigrid Katz and Bettina Pflugfelder (help during cruises), and Maria Miljutina (help in nematode identification).
Supplementary Material
The Supplementary Material for this article can be found online at: https://www.frontiersin.org/articles/10.3389/fmars.2019.00832/full#supplementary-material
DATASHEET S1 | Meio- and macrofauna species abundance per 64 cm2 from all samples.
MATERIAL S1 | Details on prokaryote abundances and animal communities.
References
Adams, D., McGillicuddy, D., Zamudio, L., Thurnherr, A., Liang, X., Rouxel, O., et al. (2011). Surface-generated mesoscale eddies transport deep-sea products from hydrothermal vents. Science 332, 580–583. doi: 10.1126/science.1201066
Adams, D. K., and Mullineaux, L. S. (2008). Supply of gastropod larvae to hydrothermal vents reflects transport from local larval sources. Limnol. Oceanogr. 53, 1945–1955. doi: 10.4319/lo.2008.53.5.1945
Bayer, S. R., Mullineaux, L. S., Waller, R. G., and Solow, A. R. (2011). Reproductive traits of pioneer gastropod species colonizing deep-sea hydrothermal vents after an eruption. Mar. Biol. 158, 181–192. doi: 10.1007/s00227-010-1550-1
Begon, M., Harper, J. L., and Townsend, C. R. (1990). Ecology: Individuals Populations and Communities. Oxford: Blackwell.
Bell, S. S., Walters, K., and Hall, M. O. (1987). Habitat utilization by harpacticoid copepods: a morphometric approach. Mar. Ecol. Prog. Ser. 35, 59–64. doi: 10.3354/meps035059
Boschen, R. E., Rowden, A. A., Clark, M. R., Barton, J. S., Pallentin, A., Gardner, J. P. A., et al. (2015). Megabenthic assemblage structure on three New Zealand seamounts: implications for seafloor massive sulfide mining. Mar. Ecol. Prog. Ser. 523, 1–14. doi: 10.3354/meps11239
Childress, J. J., and Fisher, C. R. (1992). The biology of hydrothermal vent animals: physiology, biochemistry and autotrophic symbiosis. Oceanogr. Mar. Biol. Annu. Rev. 30, 337–441.
Clarke, K. R., and Warwick, R. M. (2001). Change in Marine Communities: An Approach to Statistical Analysis and Interpretation. Primer-E Ltd: Plymouth.
Cuvelier, D., Gollner, S., Jones, D. O. B., Kaiser, S., Arbizu, P. M., Menzel, L., et al. (2018). Potential mitigation and restoration actions in ecosystems impacted by seabed mining. Front. Mar. Sci. 5:467. doi: 10.3389/fmars.2018.00467
Cuvelier, D., Sarrazin, J., Colaço, A., Copley, J., Glover, A., Tyler, P., et al. (2011). Community dynamics over 14 years at the eiffel tower hydrothermal edifice on the mid-atlantic ridge. Limnol. Oceanogr. 56, 1624–1640. doi: 10.4319/lo.2011.56.5.1624
Du Preez, C., and Fisher, C. R. (2018). Long-term stability of back-arc basin hydrothermal vents. Front. Mar. Sci. 5:54. doi: 10.3389/fmars.2018.00054
Erickson, K. L., Macko, S. A., and Van Dover, C. L. (2009). Evidence for a chemoautotrophically based food web at inactive hydrothermal vents (Manus Basin). Deep-Sea Res. II 56, 1577–1585. doi: 10.1016/j.dsr2.2009.05.002
Fisher, C. R., Takai, K., and Le Bris, N. (2007). Hydrothermal vent ecosystems. Oceanography 20, 14–23. doi: 10.5670/oceanog.2007.75
France, S. C., Hessler, R. R., and Vrijenhoek, R. C. (1992). Genetic differentiation between spatially-disjunct populations of the deep-sea hydrothermal vent endemic amphipod ventiella sulfuris. Mar. Biol. 114, 551–559. doi: 10.1007/bf00357252
Franklin, J. F., Frenzen, P., and Swanson, F. J. (1988). “Re-creation of ecosystems at Mt. St. Helens: contrasts in artificial and natural approaches,” in Rehabilitating Damaged Ecosystems, vol II, ed. Cairns, J. (Boca Raton: CRC Press), 1–37.
Galkin, S. V. (1997). Megafauna associated with hydrothermal vents in the manus back-arc basin (Bismarck Sea). Mar. Geol. 142, 197–206. doi: 10.1016/s0025-3227(97)00051-0
Galkin, S. V., and Goroslavskaya, E. I. (2008). Bottom fauna associated with mussel beds and alvinellid communities in the hydrothermal field at 9°n of the east pacific rise. Oceanology 48, 509–516. doi: 10.1134/s0001437008040061
Giere, O. (2009). Meiobenthology, the Microscopic Mobile Fauna of Aquatic Sediments, 2nd Edn. Berlin: Springer Verlag.
Gollner, S., Govenar, B., Fisher, C. R., and Bright, M. (2015a). Size matters at deep-sea hydrothermal vents: different diversity and habitat fidelity patterns of meio- and macrofauna. Mar. Ecol. Prog. Ser. 520, 57–66. doi: 10.3354/meps11078
Gollner, S., Govenar, B., Martinez Arbizu, P., Mills, S., Le Bris, N., Weinbauer, M., et al. (2015b). Differences in recovery between deep-sea hydrothermal vent and vent-proximate communities after a volcanic eruption. Deep-Sea Res. I 106, 167–182. doi: 10.1016/j.dsr.2015.10.008
Gollner, S., Kaiser, S., Menzel, L., Jones, D. O. B., van Oevelen, D., Menot, L., et al. (2017). Resilience of benthic deep-sea fauna to mineral mining activities. Mar. Environ. Res. 129, 76–101. doi: 10.1016/j.marenvres.2017.04.010
Gollner, S., Miljutina, M., and Bright, M. (2013). Nematode succession at deep-sea hydrothermal vents after a recent volcanic eruption with the desciption of two dominant species. Organ. Divers. Evol. 13, 349–371. doi: 10.1007/s13127-012-0122-2
Gollner, S., Riemer, B., Martinez Arbizu, P., Le Bris, N., and Bright, M. (2010). Diversity of meiofauna from the 9°50′N east pacific rise across a gradient of hydrothermal fluid emissions. PLoS One 5:e12321. doi: 10.1371/journal.pone.0012321
Grime, J. P. (1977). Evidence for the existence of three primary strategies in the plants and its relevance to ecological and evolutionary theory. Amer. Nat. 111, 1169–1194. doi: 10.1086/283244
Heptner, M. V., and Ivanenko, V. N. (2002). Copepoda (Crustacea) of hydrothmerl ecosystems of the world ocean. Arthropoda Sel. 11, 117–134.
Hessler, R. R., Smithey, W. M., Boudrias, M. A., Keller, C. H., Lutz, R. A., and Childress, J. J. (1988). Temporal change in megafauna at the rose garden hydrothermal vent (Galapagos Rift; eastern tropical Pacific). Deep Sea Res. Part A. Oceanogr. Res. Pap. 35, 1681–1709. doi: 10.1016/0198-0149(88)90044-1
Higgins, P. R., and Thiel, H. (1988). Introduction to the Study of Meiofauna. Washington DC: Smithsonian Insitution Press.
Ihaka, R., and Gentleman, R. (1996). R: a language for data analysis and graphics. J. Comput. Graph. Stat. 5, 299–314.
Kaartvedt, S., Van Dover, C. L., Mullineaux, L. S., Wiebe, P. H., and Bollens, S. M. (1994). Amphipods on a deep-sea hydrothermal treadmill. Deep Sea Res. Part I: Oceanogr. Res. Pap. 41, 179–195. doi: 10.1016/0967-0637(94)90032-9
Kark, S., and van Rensburg, B. J. (2006). Ecotones: marginal or central areas of transition? Isr. J. Ecol. Evol. 52, 29–53. doi: 10.1560/ijee.52.1.29
Klose, J., Polz, M. F., Wagner, M., Schimak, M. P., Gollner, S., and Bright, M. (2015). Endosymbionts escape dead hydrothermal vent tubeworms to enrich the free-living population. Proc. Natl. Acad. Sci. U.S.A. 112, 11300–11305. doi: 10.1073/pnas.1501160112
Le Bris, N., Govenar, B., Le Gall, C., and Fisher, C. R. (2006). Variability of physico-chemical conditions in 9°50′N EPR diffuse flow vent habitats. Mar. Chem. 98, 167–182. doi: 10.1016/j.marchem.2005.08.008
Le Bris, N., Yücel, M., Das, A., Sievert, S., Ponnapakkam, L., and Girguis, P. (2019). Hydrothermal energy transfer and organic carbon production at the deep seafloor. Front. Mar. Sci. 5:531. doi: 10.3389/fmars.2018.00531
Levin, L. A., Baco, A. R., Bowden, D. A., Colaco, A., Cordes, E. E., Cunha, M. R., et al. (2016). Hydrothermal vents and methane seeps: rethinking the sphere of influence. Front. Mar. Sci. 3:72. doi: 10.3389/fmars.2016.00072
Maddocks, R. F. (2005). Three new species of podocopid ostracoda from hydrothermal vent fields at 9°50′n on the east pacific rise. Micropaleontology 51, 345–372. doi: 10.2113/gsmicropal.51.5.345
Marcus, J., Tunnicliffe, V., and Butterfield, D. A. (2009). Post-eruption succession of macrofaunal communities at diffuse flow hydrothermal vents on axial volcano. Juan de Fuca Ridge, Northeast Pacific. Deep-Sea Res. II Top. Stud. Oceanogr. 56, 1586–1598. doi: 10.1016/j.dsr2.2009.05.004
Menzel, L. (2011). First descriptions of copepodid stages, sexual dimorphism and intraspecific variability of mesocletodes sars, 1909 (Copepoda, Harpacticoida, Argestidae), including the description of a new species with broad abyssal distribution. ZooKeys 96, 39–80. doi: 10.3897/zookeys.96.1496
Mills, S. W., Mullineaux, L. S., Beaulieu, S. E., and Adams, D. K. (2013). Persistent effects of disturbance on larval patterns in the plankton after an eruption on the East Pacific Rise. Mar. Ecol. Prog. Ser. 491, 67–76. doi: 10.3354/meps10463
Mullineaux, L. S., Adams, D. K., Mills, S. W., and Beaulieu, S. E. (2010). Larvae from afar colonize deep-sea hydrothermal vents after a ctastrophic eruption. Proc. Natl. Acad. Sci. U.S.A. 107, 7829–7834. doi: 10.1073/pnas.0913187107
Mullineaux, L. S., Le Bris, N., Mills, S. W., Henri, P., Bayer, S. R., Secrist, R. G., et al. (2012). Detecting the influence of initial pioneers on succession at deep-sea vents. PLoS One 7:e50015. doi: 10.1371/journal.pone.0050015
Mullineaux, L. S., Metaxas, A., Beaulieu, S. E., Bright, M., Gollner, S., Grupe, B. M., et al. (2018). Exploring the ecology of deep-sea hydrothermal vents in a metacommunity framework. Front. Mar. Sci. 5:49. doi: 10.3389/fmars.2018.00049
Mullineaux, L. S., Micheli, F., Peterson, C. H., Lenihan, H. S., and Markus, L. (2009). Imprint of past environmental regimes on structure and succession of a deep-sea hydrothermal vent community. Oecologia 161, 387–440. doi: 10.1007/s00442-009-1390-1
Mullineaux, L. S., Mills, S. W., Sweetman, A. K., Beaudreau, A. H., Metaxas, A., and Hunt, H. L. (2005). Vertical, lateral and temporal structure in larval distributions at hydrothermal vents. Mar. Ecol. Prog. Ser. 293, 1–16. doi: 10.3354/meps293001
Mullineaux, L. S., Peterson, C. H., Micheli, F., and Mills, S. W. (2003). Successional mechanism varies along a gradient in hydrothermal fluid flux at deep-sea vents. Ecol. Monogr. 73, 523–542. doi: 10.1890/02-0674
Ólafsson, E. (2003). Do macrofauna structure meiofauna assemblages in marine soft-bottoms? Vie Milieu 53, 249–265.
Platt, W., and Connell, J. H. (2003). Natural disturbances and directional replacement of species. Ecol. Monogr. 73, 507–522. doi: 10.1890/01-0552
Plum, C., Pradillon, F., Fujiwara, Y., and Sarrazin, J. (2017). Copepod colonization of organic and inorganic substrata at a deep-sea hydrothermal vent site on the Mid-Atlantic Ridge. Deep Sea Res. II 137, 335–348. doi: 10.1016/j.dsr2.2016.06.008
Schimak, M. P., Toenshoff, E. R., and Bright, M. (2012). Simultaneous 16S and 18S rRNA fluorescence in situ hybridization (FISH) on LR White sections demonstrated in vestimentifera (Siboglinidae) tubeworms. Acta Histochem. 144, 122–130. doi: 10.1016/j.acthis.2011.03.008
Shank, T. M., Fornari, D. J., Von Damm, K. L., Haymon, R. M., and Lutz, R. A. (1998). Temporal and spatial patterns of biological community development at nascent deep-sea hydrothermal vents (9°50′N, East Pacific Rise). Deep-Sea Res. Part II 45, 465–515. doi: 10.1016/s0967-0645(97)00089-1
Smith, C. R., De Leo, F. C., Bernardino, A. F., Sweetman, A. K., and Martinez Arbizu, P. (2008). Abyssal food limitation, ecosystem structure and climate change. Tree 23, 518–528. doi: 10.1016/j.tree.2008.05.002
Soule, S. A., Fornari, D. J., Perfit, M. R., and Rubin, K. H. (2007). New insights into mid-ocean ridge volcanic processes from the 2005–2006 eruption of the East Pacific Rise, 9°46′N–9°56′N. Geology 35, 1079–1082.
Sousa, W. P. (2001). “Natural disturbance and the dynamcis of marine benthic communities,” in Marine Community Ecology, eds M. D. Bertness, S. D. Gaines, and M. E. Hay, (Sunderland, MA: Sinauer Associates, Inc), 85–130.
Suzuki, K., Yoshida, K., Watanabe, H., and Yamamoto, H. (2018). Mapping the resilience of chemosynthetic communities in hydrothermal vent fields. Sci. Rep. 8:9364. doi: 10.1038/s41598-018-27596-7
Thistle, D., and Sedlacek, L. (2004). Emergent and non-emergent species of harpacticoid copepods can be recognized morphologically. Mar. Ecol. Prog. Ser. 266, 195–200. doi: 10.3354/meps266195
Thurnherr, A. M., Ledwell, J. R., Lavelle, W., and Mullineaux, L. S. (2011). Hydrography and circulation near the crest of the East Pacific Rise between 9° and 10°N. Deep Sea Res. I 58, 365–376. doi: 10.1016/j.dsr.2011.01.009
Tolstoy, M., Cowen, J. P., Baker, E. T., Fornari, D. J., Rubin, K. H., Shank, T. M., et al. (2006). A sea-floor spreading event captured by seismometers. Science 314, 1920–1922. doi: 10.1126/science.1133950
Tsurumi, M., and Tunnicliffe, V. (2003). Tubeworm-associated communities at hydrothermal vents on the juan de fuca ridge, northeast Pacific. Deep-Sea Res. Part I 50, 611–629. doi: 10.1016/s0967-0637(03)00039-6
Tunnicliffe, V., Botros, M., De Burgh, M. E., Dinet, A., Johnson, H. P., and Juniper, S. K. (1986). Hydrothermal vents of explorer ridge, northeast Pacific. Deep Sea Res. Part A. Oceanogr. Res. Pap. 33, 401–412. doi: 10.1016/0198-0149(86)90100-7
Tunnicliffe, V., Metaxas, A., Le, J., Ramirez-Llodra, E., and Levin, L. A. (2018). Strategic environmental goals and objectives: setting the basis for environmental regulation of deep seabed mining. Mar. Policy 95, 388–393.
Tyler, P. A., and Young, C. M. (1999). Reproduction and dispersal at vents and cold seeps. J. Mar. Biol. Associat. 79, 193–208. doi: 10.1017/s0025315499000235
van der Heijden, L. H., Rzeznik-Orignac, J., Asmus, R. M., Fichet, D., Bréret, M., Kadel, P., et al. (2018). How do food sources drive meiofauna community structure in soft-bottom coastal food webs? Mar. Biol. 165:166.
Van Dover, C. L. (2000). The ecology of Hydrothermal Vents. Princeton NJ: Princeton University Press.
Van Dover, C. L. (2002). Community structure of mussel beds at deep-sea hydrothermal vents. Mar. Ecol. Prog. Ser. 230, 137–158. doi: 10.3354/meps230137
Van Dover, C. L., Arnaud-Haond, S., Gianni, M., Helmreich, S., Huber, J. A., Jaeckel, A. L. et al. (2018). Scientific rationale and international obligations for protection of active hydrothermal vent ecosystems from deep-sea mining. Mar. Policy 90, 20–28. doi: 10.1016/j.marpol.2018.01.020
Vrijenhoek, R. C. (2010). Genetic diversity and connectivity of deep-sea hydrothermal vent metapopulations. Mol. Ecol. 19, 4391–4411. doi: 10.1111/j.1365-294X.2010.04789.x
Keywords: senescent vent, biodiversity, volcanic eruption, recovery, meiofauna, macrofaunal, deep-sea mining
Citation: Gollner S, Govenar B, Martinez Arbizu P, Mullineaux LS, Mills S, Le Bris N, Weinbauer M, Shank TM and Bright M (2020) Animal Community Dynamics at Senescent and Active Vents at the 9°N East Pacific Rise After a Volcanic Eruption. Front. Mar. Sci. 6:832. doi: 10.3389/fmars.2019.00832
Received: 30 May 2019; Accepted: 27 December 2019;
Published: 24 January 2020.
Edited by:
Stephen Hammond, Office of Oceanic and Atmospheric Research (NOAA), United StatesReviewed by:
Christoph Plum, University of Oldenburg, GermanyAmanda N. Netburn, NOAA’s Office of Ocean Exploration and Research, United States
Copyright © 2020 Gollner, Govenar, Martinez Arbizu, Mullineaux, Mills, Le Bris, Weinbauer, Shank and Bright. This is an open-access article distributed under the terms of the Creative Commons Attribution License (CC BY). The use, distribution or reproduction in other forums is permitted, provided the original author(s) and the copyright owner(s) are credited and that the original publication in this journal is cited, in accordance with accepted academic practice. No use, distribution or reproduction is permitted which does not comply with these terms.
*Correspondence: Sabine Gollner, c2FiaW5lLmdvbGxuZXJAbmlvei5ubA==