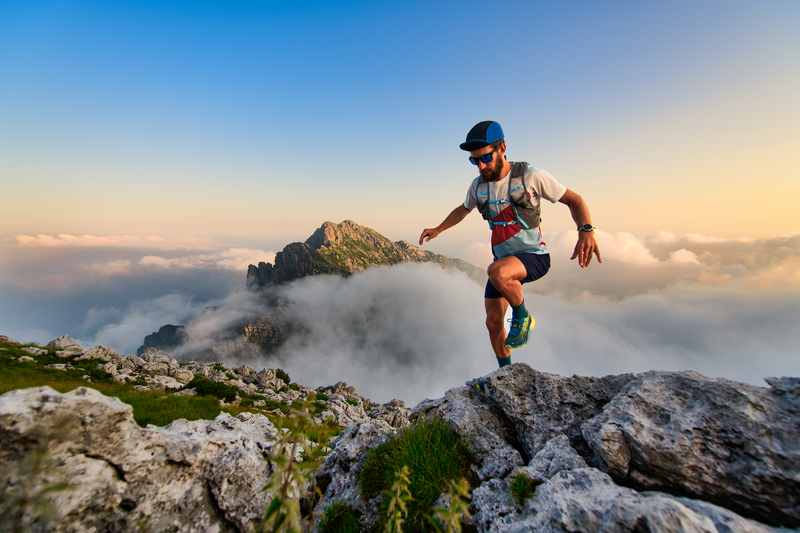
94% of researchers rate our articles as excellent or good
Learn more about the work of our research integrity team to safeguard the quality of each article we publish.
Find out more
ORIGINAL RESEARCH article
Front. Mar. Sci. , 21 January 2020
Sec. Marine Ecosystem Ecology
Volume 6 - 2019 | https://doi.org/10.3389/fmars.2019.00809
This article is part of the Research Topic Submarine Groundwater Discharge: Impacts on Coastal Ecosystem by Hidden Water and Dissolved Materials View all 11 articles
Submarine groundwater discharge (SGD), the direct discharge of groundwater into the sea, is abundant around the globe. Fresh SGD can occur as focused flow in submarine springs. However, little is known on the impact of submarine springs on marine organisms. For a better understanding of the interaction between SGD and its surrounding organisms, the impact of SGD on the abundance of fish was investigated in a coastal lagoon of Tahiti, French Polynesia. The study is based on the assumption of an enhanced biological production due to increased amounts of nutrient input caused by terrestrial groundwater supply into the sea. Biofouling processes and zooplankton samples were used as indicators for elevated nutrient input due to submarine springs. The main objective was to investigate the effect on the abundance of fish assuming a higher fish abundance possibly caused by a bottom-up control. Presented data show a significantly higher abundance around a submarine spring as well as significantly larger growth of algal turfs exposed to groundwater discharge. Zooplankton evaluations suggest slightly higher abundances around the submarine spring. The results suggest elevated nutrient concentrations transmitted by submarine springs may cause a bottom-up control resulting in a higher abundance of fish around the investigated submarine spring.
Scientific interest in submarine groundwater discharge (SGD), the direct discharge of groundwater to the coastal waters, has strongly increased over the past years, highlighting its influence on coastal nutrient budgets (Moore, 2010; Moosdorf et al., 2015), global water budgets (Burnett et al., 2003; Kwon et al., 2014), and global biogeochemical cycles (Slomp and Van Cappellen, 2004; Cole et al., 2007; Beusen et al., 2013; Cho et al., 2018). Its effect on marine flora and fauna in turn has been studied rarely (e.g., Fujita et al., 2019; Lilkendey et al., 2019). These recent studies point towards a bottom-up influence of SGD on fish abundance and fitness.
We present a detailed photographic study of fish at a submarine spring site located in a coastal lagoon of Tahiti, French Polynesia accompanied by observations on nutrients, primary (biofouling) and secondary production (zooplankton).
Due to their location between continental and marine ecosystems, coastal lagoons are often described as boundary environments (Sarno et al., 1993). Their prevailing sedimentary deposition in tropical zones is often characterized by the accumulation of calcium carbonate remains and calcium carbonate producing organisms (Duck and da Silva, 2012). Determined by the local climate conditions and the degree of hydrological access salinity values can alternate from freshwater to hypersaline (Kjerfve, 1986). Coastal lagoons are highly productive ecosystems with water depths that rarely exceed a few meters (Kjerfve, 1986). The coastal lagoon presented in this study (Trou de Lagon) exhibits several SGD spots of which we used two for our investigations.
Submarine groundwater discharge consists of two main fractions: recirculated seawater and terrestrial groundwater discharging into the ocean (Burnett et al., 2003). The latter, here called “fresh SGD” referring to the fresh fraction of the often brackish actual discharge, is an important pathway of terrestrial pollutants including nutrients, trace metals, pharmaceuticals, various pollutants and other terrestrial substances into the coastal ocean (Knee and Paytan, 2011). Fresh SGD can discharge diffusely, e.g., from a coarse grained coastal sediment, in a focused way from conduits (here called “submarine springs”) (Slomp and Van Cappellen, 2004). Submarine springs generally influence their surrounding environment, by changing salinity, temperature, nutrient and sediment concentrations (Miller and Ullman, 2004; Oehler et al., 2018) or by creating morphological features on the sea floor (Stieglitz and Ridd, 2000). Ecological impacts of SGD were mostly derived from postulating an impact from elevated nutrient content (e.g., Johannes, 1980; Miller and Ullman, 2004; Slomp and Van Cappellen, 2004; Tait et al., 2014).
Direct impacts of terrestrial groundwater inflow through elevated nutrient concentrations was reported to enhance biological production as well as species diversity of coastal ecosystems in a limited number of studies (Lecher and Mackey, 2018). However, fishermen in many regions all over the world observe higher fish abundance around submarine springs and attribute the flourishing influence to the freshwater (Moosdorf and Oehler, 2017). This effect was scientifically confirmed only in locations in Japan reporting significantly higher abundances or biomass of fish (Utsunomiya et al., 2015; Hata et al., 2016; Shoji and Tominaga, 2018; Fujita et al., 2019). In Mauritius, a positive effect of fresh SGD on fitness of juvenile fish was observed (Lilkendey et al., 2019).
As changes in ecosystems are often based on alterations in nutrient fluxes, Johannes (1980) argued that ignoring SGD may lead to profound misinterpretation of ecological data in terms of coastal pollution, benthic zonation and productivity. His data indicated that SGD locally delivers several times more nitrate to coastal waters at the assessed location than river runoff does. This is supported by other authors, partly associated with harmful algae blooms, at various locations (e.g., Laroche et al., 1997; Paerl, 1997; Gobler and Sañudo-Wilhelmy, 2001; Smith and Swarzenski, 2012; Liu et al., 2017). Paytan et al. (2006) reported enhanced anthropogenic nutrient loads from SGD is also likely to contribute to reef degradation.
Few authors reported on nutrient fluxes delivered by SGD on marine biota. For example, macrophyte tissue reflecting δ15N of SGD showing higher nitrogen concentrations, larger leaves and blades, reduced macrophytes biodiversity and higher biomass (Kamermans et al., 2002; Umezawa et al., 2002; Lapointe et al., 2005; Amato et al., 2016). Studies lacking δ15N data but conducted in known SGD areas also show these tendencies as e.g., in the most investigated green macroalgae Ulva. Williams and Carpenter (1988) worked on the productivity of small micro filamentous epilithic algae, so called “algal turfs”, resulting in a significantly higher primary production per unit chlorophyll-a of ammonium treated algal turfs. The bryozoan Pentapora fascialis also showed changes of colony growth, size, and mortality around SGD (Novosel et al., 2005; Cocito et al., 2006).
According to Liu et al. (2017) environmental factors influencing zooplankton abundance and diversity are mainly chlorophyll-a concentration, temperature and salinity. As SGD influences these factors and as zooplankton is highly depending on primary producers, zooplankton is here used as an indicator for SGD and its influence on higher trophic levels. In the present study, primarily mesozooplankton will be investigated. Based on the key role of zooplankton in early life history of fish Liu et al. (2017) pointed out that changes in zooplankton abundance as well as in its composition have significant impacts on the recruitment and dynamics of fish.
The present study investigates the effect of a submarine spring on the abundance of fish in a coastal lagoon of Tahiti, French Polynesia, assuming a bottom-up control caused by elevated nutrient concentrations from a submarine spring.
To investigate the abundance of fish a stationary photo sampling was performed. To visualize the nutrient input and emphasize the effect on primary- and secondary production a short-term in situ biofouling experiment as well as a zooplankton sampling of small sampling size were conducted. These two parameters were used as indicator of elevated nutrient concentrations released by the submarine groundwater spring at Trou de Lagon.
Experiments were performed from 20th February until 3rd March 2017. Tahiti is a volcanic island located at 17° 24′ S and 149° 07′ W (Figure 1). It is part of the so called Society Island archipelago, with a surface of 1042 km2 (Rougerie et al., 1997). The island is composed mainly of basaltic material (Frouin, 2000) and surrounded by an almost continuous barrier reef disconnected from the coastline by a narrow lagoon of 100 to 800 m width and a rather small tide-range of about ±0.15 m (Rougerie et al., 1997).
The climate of Tahiti Island is influenced by the Southern Oscillation. Trade winds blow primarily from the northeast during summer (rainy season) and southeast during winter. The mean rainfall is 150 cm y–1 and the air temperature generally between 20–33°C. Tahiti is located in the western area of the south pacific gyre with sea surface temperatures between 26–30°C and sea surface salinity values of 35.5–36.3. The high salinity values are caused by the negative P-E differentials leading to increasing surface salinity whereas values may decrease during rainy season (Delcroix and Hénin, 1991; Rougerie et al., 1997).
Tahiti generally consists of two major volcanoes called Tahiti-Nui (the bigger part of the island) and Tahiti-Iti (the smaller part of the island) which are aligned in NW-SW (Duncan et al., 1994). The study site is called Trou du Lagon which is located on Tahiti-Iti in a low populated area. One of the main food production in form of agriculture and livestock cultivation is located in the region of Afaahiti-Taravao, Papeari, and Vairao at the isthmus of the island which is the transition from Tahiti-Nui to Tahiti-Iti (Cunningham, 1961). The study site is located directly within this area which leads to the assumption that the elevated nutrient concentrations are caused by the agriculture and livestock production.
Trou de Lagon is an oval basin in shape (Figure 1A) with depths from two to thirty meters enclosed in a shallow water body connected to the lagoon. The sampling area ranges about 200 m × 200 m around the sampling sites with three submarine springs in 11.6, 5.5, and 3.5 m water depth. The springs are of small size and do not exhibit any expression at the water surface. The distance to the shore line is about 850 m, the distance to the reef about 800 m. As Tahiti is a volcanic island, the substrate is basalt, which is mostly covered by corals, due to the oligotrophic water conditions, the lack of sediments and the nearby barrier reef. The in situ biofouling and photo sampling experiments were performed at two different springs at the same study site during the same time. While no direct geochemical characterization of the springs is available, given the geology of the island and the SGD from Tahiti in general (cf. Haßler et al., 2019) two springs that close to each other can be expected to originate from the same aquifer and behave similarly. The sampling was performed at 17° 46‘S and 149° 19’W in about 130 m distance to each other. The study site exhibits very little disturbances due to divers or fishermen.
To demonstrate the presence of submarine discharge, water samples were taken directly from the discharge spot using falcon tubes. The sampling was performed daily during the stationary photo sampling and once during the biofouling experiment. The sampling solely provided salinity data as temperature adapted too quickly to the ambient seawater temperature. To estimate the groundwater discharge temperature of the submarine spring at Trou de Lagon temperature was measured manually at different accessible groundwater discharge spots within the eulittoral zone around the island. These measurements were performed by using a rod-thermometer. Nitrate and phosphate concentrations of the spring at Trou de Lagon were taken from Haßler et al. (2019), who performed a field study in January 2016.
In order to indicate the assumed elevated primary production a short-term in situ experiment was conducted. A total of 26 plastic panels of ten by ten centimeters size were strung on a rope and tied around corals in 3.5 m depth, through the submarine spring (Figures 2, 3). The objective was to expose the central panels to the submarine spring to monitor the biofouling process caused by the spring, and observe the range of influence to both sides of the spring. The panels on the outer parts of the line exposed to fully marine seawater were used as control panels. For identification purposes, the groundwater exposed panels were marked with cable ties. For further evaluations, all panels were distinguished in spring and control panels.
Figure 2. Experimental design of the 10-day in situ biofouling experiment in 3.5 m water depth. In total 26 settlement panels were used, five panels exposed to the submarine spring and twenty-one to seawater.
Figure 3. Settlement panels (10 × 10 cm) stringed on a rope and fastened across the submarine spring (↓).
After 10 days, the panels were taken out of the water and dried for several days not exposed to direct sunlight. To analyze, front and back side of each panel were scanned by using an Epson Perfection V330 photo scanner. All scans were cropped at a height of seven centimeters to cut out the rope influenced part of the panels. Within this part most of the settlement took place but the focus of analysis was on the panels’ surfaces. The cropping and all further analyses were performed by using the software ImageJ (Version 1.51 f). To finally quantify the amount of settlement as percentage of covered area, a scale was entered using the Set Scale function. Therefore, a line of known distance on one of the panels was used as scale reference. The Type of Image was set on RGB Color and the Color Threshold function was used. To define the areas of settlement the Color Space was set on HSB, which are the three properties to describe a color (Hue, Saturation and Brightness). On an interval of 0–255, given by ImageJ, thresholds were set by trial and error and applied for all panels equally. The hue was set from 0 to 115, saturation from 26 to 255 and brightness from 115 to 255. Applying these settings, the areas of investigation were defined in red (Figure 4, right) to finally determine the settled areas in percent by using the function Analyze Particles. The front and back sides of all 26 panels were evaluated separately to gain two separate datasets which can be considered as replicates. In total, five panels where marked as groundwater discharge exposed and eleven as seawater exposed (control).
Figure 4. One of 26 settlement panels exposed to the submarine spring (left: settled areas in green, yellowish; right: identical areas in red to quantify the proportion of settlement in percent by using ImageJ).
As a further indicator of elevated nutrient concentrations, a zooplankton sampling of small sampling size was performed. The sampling was carried out by using an APSTEIN plankton net of 55 μm mesh size and a unscrewable net beaker (sampling container) within a water column of 5 m. A total of twelve hauls was performed, six hauls around the submarine spring within a radius of about 3 m and six at the control site. Each haul was performed from the boat in the same way. After each haul, the inside of the net was rinsed with filtered sea water (55 μm) in order to collect the entire sample in the sampling container. After rinsing, the container was unscrewed and the content transferred in 50 ml Kautex container by using a funnel and a squeeze bottle with filtered sea water (55 μm). Each sample was preserved in 60% alcohol and stored in a fridge at six degrees Celsius until analysis.
To analyze the zooplankton abundance the hardware Hydroptic ZooScan ZSCA02 together with the software Vuescan (Version 8.4.57), ZooProcess and Plankton Identifier was used, following the procedure described by Gorsky et al. (2010). To determine the zooplankton abundance each sample was first transferred into a sieve of 55 μm mesh size to separate it from the alcohol. To scan the isolated sample, it was poured directly into a transparent frame (15 cm × 24 cm) inserted in the scanning cell. The frame includes a 5-mm step. De-ionized water was poured up to the edge of the step beforehand to avoid forming a meniscus on the periphery of the image. To obtain one vignette (image) of each single individual overlapping organisms and organisms touching the side of the frame were physically separated from each other or from the frame before digitizing. Organisms touching the sides of the frame are automatically removed from the data set (Gorsky et al., 2010). The density of individuals was below 1000–1500 organisms within the scanning area which is sufficient to reduce overlapping organisms (Gorsky et al., 2010). To recheck that organisms are not overlapping, each scan was reviewed and overlapping individuals separated manually by using the software ImageJ. Each 16-bit raw image was normalized and converted into an 8-bit full gray scale image and processed by subtracting the background and removing the frame edges. The rolling ball method (Sternberg, 1983) provided by the ZooProcess software was used for background subtraction.
For automatic classification (Plankton Identifier) of objects across all scanned images into major groups a learning file of selected categories was used. The learning file contained vignettes of single objects of different taxonomic groups. Each folder within the learning file represented one taxonomic group. Additional folders of abiotic objects as e.g., fibers, bubbles as well as blurry objects were also part of the learning file. To reduce the error rates all automatically classified objects were re-validated manually, and any misidentified objects were moved to the appropriate taxonomic group. The final images are digitized with 96-dpi and pixels of 24284 – 15296 in size with a pixel resolution of 10.58 μm. These 8-bit gray level images require about 350 MB storage and can be handled easily by regular PC ìs (Figure 5). Finally, the zooplankton was classified into six major groups above genus level (Copepods, Appendicularia, Chaetognatha, Malacostraca, Polychaeta, and fish eggs). The classification on a species level is not feasible using the software Plankton Identifier. The abundance was calculated for each haul per site as well as for each of the six groups per site given in the number of individuals per volume of water (Ind. L–1).
Figure 5. Examples of scanned objects (vignettes) (A) Ostracoda (Oligostraca), (B) Calanoida (Copepoda), (C) Zoea (Decapoda), (D) Zoea (Decapoda), (E) Egg, (F) Appendicularia (Tunicata), (G) Chaetognatha (Metazoa), (H) Zoea (Decapoda), (I) Harpacticoida (Copepod), (J) Polychaeta (Annelida), (K) Fish Larvae.
To investigate the effect of the submarine spring on the abundance of fish, a 10-day stationary photo sampling was implemented. Two GoProHero4 cameras were mounted on the bottom of the reef, one camera in about one meter distance to the submarine spring in 5.5 m depth recording the presence of fish around the submarine spring. The second camera was installed in 5.4 m depth as a control. The control site was chosen in a way that the surrounding area was ideally of the same structure and certainly not influenced by the submarine spring. The distance between both cameras was 56 m. In order to install the cameras two small camera mounts were built to guarantee a secure hold as well as to reduce possible thievery (Figure 6). Recorded were images instead of videos in order to enhance the battery capacity. The cameras were set to take images at an interval of one shot per minute. The change of batteries and memory cards took place once a day at 7:30 AM. In consequence, the daily recording started at the same time and ended with the complete battery discharge. Status lights and sounds were deactivated. In order to extend the batteries capacity each camera was equipped with one GoPro battery BacPac. By using the battery packs, images were taken up to 10 h per day, resulting in about 400 to 600 pictures per day. To evaluate the photo sampling the number of fish at the spring and control site images was counted manually. To visualize a potential difference in quantity straightforward as well as to compare the obtained data easier, the number of fish recorded in the images were grouped in categories. For example, one up to five fish, six up to ten fish. Species were not identified. Distant fish in the very depth of the images were neglected during the counting process. In total 7206 Images were evaluated with 3603 images per site (Figure 7).
Figure 6. Installation built for the stationary photo sampling (left: metal loops fixed onto a metal plate to interlock the GoPro camera housing; right: mounting process of the metal construction as fixation for the 10-day photo sampling).
Figure 7. Example of higher fish abundance regarding three-dimensionality and different substrate manifestation in the depth of the images [left: spring site with less substrate, right: control site with more pronounced substrate and a higher number of fish in the depth of the image (orange circle)].
The Statistical analysis was carried out by using the software R-Studio (Version 1.0.143). The assumptions of normal distributed data and homoscedasticity were tested by graphical and numeric methods. The type of distribution was investigated by plotting normal q-q plots, histograms as well as by performing the Shapiro–Wilk test. Homoscedasticity was verified by the F-test. Log transformations were applied when the assumptions of normal distribution and homoscedasticity were not fulfilled. The subsequent hypotheses testing was carried out either by using the parametric two sampled T-test or the non-parametric Mann–WhitneyU-test depending on whether the assumptions were fulfilled. The frequencies of counted categorized fish were analyzed by applying the Chi–squared Test of homogeneity. Therefore, the data was displayed in a contingency table. Based on this table the test evaluates the distribution of frequencies compared to the expected frequency (Köhler et al., 2012). The test was applied on the same number of images at both sites at the respective days. The data set consists of one factor (site) with two factor levels (spring and control) and one response variable depending on which data set was tested (settlement, abundance, counts).
The manually measured groundwater spring temperatures at different submarine springs within the eulittoral zone around the island were between 23.0 and 24.0°C whereas at Trou de Lagon the temperature at the control site (seawater) of the photo sampling was between 29.2 and 30.2°C. The salinity values at the photo sampling and biofouling experiment resulted in salinities between 18.5 and 26.5 (still mixed with seawater) at the submarine spring and between 35.4 and 35.9 at the control site. The nutrient concentration of the submarine spring at Trou de Lagon was measured in 2016, resulting in concentrations of 5.7 mmol m–3 NOx and 3.7 mmol m–3 PO4. Concentrations in seawater sampled at a control site were below 0.4 mmol m–3 PO4 and below 0.3 mmol m–3 NOx (Haßler et al., 2019).
The settlement of algal turf on the groundwater exposed settlement panels was significantly higher compared to the seawater exposed panels (non-parametric Mann–Whitney U-test, p = 0.004 and p = 0.030 on panel front and back sides, respectively). The panels exposed to the submarine spring exhibit elevated settlement compared to the seawater exposed control panels. The largest amount of settlement in area percent of the submarine spring exposed panels is located above the total amount of settlement of the seawater exposed panels (Figure 8). The groundwater exposed panels displayed a median settlement of 6.5% whereas the seawater exposed panels exhibit a median settlement of 2.7%. The panel front sides were settled more intensively (medians 10.3 and 3.6% for groundwater and seawater exposed panels, respectively) than the back sides (medians 3.7 and 2.1% on groundwater and seawater exposed panels). The median settlement area thus increased by factor 2.4 of the groundwater exposed panels (2.8 at the front sides, 1.8 at the back sides). From Figure 9 it can be seen that related to the center of the spring the increasing biofouling process exhibited a shift to the right of front and back sides, respectively.
Figure 8. Biofouling processes of settlement panels exposed to a submarine spring and a control section of seawater.
Figure 9. Biofouling processes of 26 settlement panels exposed to a submarine spring and the seawater control section. The biofouling process on the front and the back sides of the settlement panels are illustrated separately. Each data point is one panel in the same distance to the submarine spring as in the field experiment.
The total zooplankton abundance of six hauls at Trou de Lagon was slightly higher around the submarine spring (2.57 Ind. L–1 ± 0.10 Ind. L–1) than at the control site (2.36 Ind. L–1 ± 0.16 Ind. L–1). The median abundance was with 0.46 Ind. L–1 around the submarine spring and 0.33 Ind. L–1 at the control site slightly higher around the spring. The most abundant taxa at both sites were Copepoda followed by Appendicularia, Chaetognatha, Malacostraca, Polychaeta, and fish eggs. Copepoda were taken here as a proxy for elevated nutrient concentrations. The total copepod abundance was 1.97 Ind. L–1 ± 0.09 Ind. L–1 around the submarine spring and 1.82 Ind. L–1 ± 0.14 Ind. L–1 at the control site. The median copepod abundance of six hauls was 0.35 Ind. L–1 around the submarine spring and 0.25 Ind. L–1 at the control site. The evaluation of Copepod abundances per haul (Figures 10, 11) resulted in slightly higher abundances around the submarine spring in four out of six hauls (two, three, five, and six) compared to the control sites. However, the applied non-parametric Mann–Whitney U-test did not result in a significantly higher copepod abundance (p = 0.7) around the submarine spring compared to the control site.
Figure 10. Copepod abundances sampled within a diameter of six meters around a submarine spring and a seawater control site.
Figure 11. Copepod abundances sampled at the submarine spring site within a diameter of six meters around the submarine spring and the seawater control site.
With the stationary photo sampling we analyzed the number of fish around a submarine spring and a control site. The number of fish, here presented in frequencies of counted categories, declined stronger at the control site compared to the submarine spring site (Figure 12). Five out of seven categories were counted more frequent at the submarine spring site compared to the control site. Categories of zero fish and six until ten fish were counted more frequent at the control site. The frequency distribution of counted categories (Figure 12) follows more or less a Poisson distribution. The factor results of spring and control site presents the increase in categories of higher number of fish (Figure 12, secondary axis). Category 0 was counted more often by factor 8.2, category 1–5 by factor 1.0, category 6–10 by factor 0.7, category 11–15 by factor 1.0, category 16–20 by factor 1.9, category 21–25 by factor 5.4, category 26–30 by factor 4.2, category 31 → by factor 11.7. Despite the eight times more frequent category of zero fish and the similar outcome of category 1–5, 6–10, and 11–15 fish the evaluation resulted in a higher presence of fish at the submarine spring site. According to the Chi–Square test of homogeneity the frequencies of counted categories significantly differ (p < 0.001) from one another in favor of the submarine spring site.
Figure 12. Categorized frequencies of counted fish around a submarine spring and a seawater control site. The secondary axis depicts the ratio Spring/Control. Note that the left (Frequency) axis is on logarithmic scale while the right (Ratio) axis is linear.
The presence of the submarine springs was confirmed visually, in salinity and temperature measurements. The temperatures of the submarine springs at Trou de Lagon are assumed to be equivalent to the manually measured temperatures at the different groundwater discharge spots within the eulittoral zone around the island. The salinity differences between the submarine spring site and control site underscore the effect of the submarine spring on the environmental parameters. The remaining low salinity values of the spring site are caused by the immediate mixing of ground and seawater whilst discharging. Nitrogen and phosphorus concentrations were elevated at the submarine spring sites compared to samples of seawater at a control site (Haßler et al., 2019). The reduced salinity within the spring as well as the nutrient concentrations support our hypothesis of fresh SGD as a source of nutrient enrichment at the groundwater discharge site.
Data regarding species diversity of Tahiti lagoons are scarce. Studies on macrobenthic communities were mostly undertaken of soft bottom communities at the most populated region of the island nearby Papeete or Faaa Airport (e.g., Frouin and Hutchings, 2001). The authors described the resulting biomass and species diversity as low in comparison with most other areas within the South Pacific Ocean. Some fish species identified in the context of this study are: different types of Holothuroidea; Chaetodontidae: Chaetodon ornatissmus, Chaetodon mertensii, Chaetodon unimaculatus, Focipiger flavissimus, Forcipiger flavissimus; Balistidae: Odonus niger, Balistapus undulatus, Rhinecanthus aculeatus, Balistoides viridescens; Scaridae: Scarus oviceps; Aulostomidae: Aulostomus chinensis; Pomacanthidae: Centropyge flavissima, Pygoplites diacanthus; Pomacentridae: Chromis margaritifer; Labridae: Thalassoma hardwicke, Halichoeres trimaculatus; Acanthuridae: Zebrasoma scopas, Acanthurus triostegus the latter is often found grazing on hard substrate near freshwater discharge where certain algae grow (Kuiter and Tonozuka, 2001).
The frequency of counted categories of larger fish groups was significantly higher at the submarine groundwater spring site. Our hypothesis of a higher presence of fish around submarine springs can thus be confirmed for the site of the present study. While categories including small groups of fish were counted in similar frequencies at both sites, categories of larger groups were counted significantly more often at the submarine spring site (Figure 12). Despite the possibility, that factors such as wind stress or waves may have certain influence, that we could not control or measure in this study, we conclude that the main cause for the increased abundance of fish around the spring is the elevated nutrient inflow by groundwater discharge enhancing primary production leading to a higher availability of prey.
An adaptation to changing environmental conditions as e.g., the seahorse Hippocampus guttulatus in Tiralongo and Baldacconi (2014) is not considered as the nutrient input at Trou de Lagon is punctual and thus avoidable. Fish at Trou de Lagon are assumed to prefer the proximity of the spring due to higher availability of nutrients and prey. Tiralongo and Baldacconi (2014) worked on a H. guttulatus population found in the Mar Piccolo of Taranto (Ionian Sea) known for its substantial pollution and oscillations of environmental parameters. This declining and endangered species, inhabit preferably seagrass and macroalgal meadows. Yet, the population is well adapted to the highly polluted environment showing a clear preference to artificial hard substrate over the usual seagrass. The authors’ assumption of the abundant population is lacking fishing pressure and good availability of food caused by the artificial eutrophication. However, it is questionable whether this result can be linked to the present study. Fish at Trou de Lagon inhabit a general oligotrophic environment being attracted by a submarine spring carrying higher nutrient concentrations, whereas H. guttulatus inhabit a highly eutrophic environment but mainly found in the western part of the lagoon, which is in direct contact with the open sea allowing a water exchange.
Apart from nutrient input also temperature should be considered to effect the distribution of fish around the study sites. Groundwater discharge temperatures in lower latitudes generally tends to be lower than its ambient seawater (Shaban et al., 2005; Ka’eo Duarte et al., 2006; Johnson et al., 2008). As dissolved oxygen (DO) increases with lower temperature and salinity values, here caused by the submarine spring, it might also influence the abundance of fish around submarine springs although the observed flow rate at Trou de Lagon is low. Salinity and temperature values could not be measured precisely as ground and seawater mixed immediately during the sampling.
A variability in refuge possibilities due to higher manifestation of substrate between sites was tried to rule out by the camera set up. Recorded were image sections with approximately the same amount of substrate within close proximity to the camera at both sites (spring and control). However, a difference in substrate manifestation took place at the very far back of the control site images.
A higher abundance of fish due to recruitment after spawning depending on seasonal variations of temperature, inducing algal blooms in spring or autumn, were excluded as the climate is tropical with only two distinct seasons. However, it is worth mentioning that instead of seasonal variations in temperature as in higher latitudes other variations as rain and dry season do have an effect on reproduction (Flecker and Feifarek, 1994) and may influence processes. Furthermore, seasonal variation is not assumed to cause such selective higher fish abundance.
The significantly higher abundance of fish is suggested to be the result of nutrient inflow by the groundwater discharge enhancing primary production as presented in the short-term biofouling experiment.
Lapointe (1997) found epilithic algal communities such as algal turfs as well as macroalgae and coralline algae increasing with increasing nutrient concentrations. During our short-term biofouling experiment, a visible settlement of small filamentous sessile algae took place, which was significantly higher on the groundwater compared to the seawater exposed panels. The biofouling process on the front sides was higher compared to the back sides of the settlement panels. The latter might be a result of the light exposure with a higher light irradiation on the front sides caused by the alignment to the sun. The back sides of the panels were aligned in south-eastern direction receiving light irradiation for a fractional amount of the day, whereas the front sides where aligned more in direction north-west receiving light irradiation during almost the entire daytime. The extent of biofouling along the transect (Figure 9) exhibited a shift of elevated settlement to the right which might be caused by slight currents moving the mixing plume to the right or the freshwater exposed panels have not been marked precisely.
The result of the biofouling experiment supports the previously assumed higher productivity due to elevated nutrient concentrations around SGD sites (e.g., Williams and Carpenter, 1988; Lapointe, 1997; Novosel et al., 2005; Cocito et al., 2006). Factors such as wave energy, exposure to winds or regional advection as causes of the different manifestation in settlement between ground and seawater exposed panels are excluded. These factors would in contrast cause a mixing of fresh and seawater during short or long term events neutralizing the groundwater effect rather leading to a homogeneous algal turf manifestation.
The total zooplankton abundance at the groundwater discharge site was just slightly and insignificantly higher compared to the control site. The overall abundance was very low at both sites, based on the in general oligotrophic tropic waters of low production (Dias et al., 2015). An even lower total abundance of 0.068 Ind. L–1 was found by Carleton and Doherty (1998) in a lagoon of Tuamotu Archipelago, located within the same archipelago as Tahiti. As expected the most abundant taxon at both sampling sites were Copepods which are the most important grazer of nano- and microplankton and the main diet of planktivorous fish.
The outcome of the present work is in line with results from Obama Bay, Japan, showing a significantly higher abundance of fish at a groundwater discharge site (Utsunomiya et al., 2015). Also, a significantly higher abundances of turban snail, hermit crabs and gammarids were reported at the groundwater discharge site (Utsunomiya et al., 2015). The authors assumed the higher abundance of fish might be the result of the 18 times higher gammarid abundance being the major prey for these fish. The higher abundance of fish around the submarine spring at Trou de Lagon may thus be the result of a bottom-up control as hypothesized.
Diversity of fish species was not evaluated in the frame of this work, as a proper identification of species could not be performed by using the recorded images. Obstacles were e.g., the distance of fish to the camera, turbidity or the salinity gradient blurring the sight. The proportion of juvenile and adult fish could not be estimated on the basis of the implemented sampling. Therefore, a 3D video-based technique (Neuswanger et al., 2016) should be considered for further investigations.
Difficulties which occurred during the image evaluation should be taken into account for further investigations. Difficulties in counting fish occurred for example due to the three-dimensionality of the water body, changing image sections caused by the daily change of batteries, different manifestations of substrate as well as rapidly changing turbidity. The three-dimensionality of the water body made it difficult to count as the distances to the actual study site cannot be estimated precisely. In addition with different substrate complexity the counting involves subjective decisions. More substrate causes more shelter and nutrition for fish. A varying amount of substrate between two sites may also influence the abundance of fish (McClanahan, 1994; Gratwicke and Speight, 2005) which may be an additional variable besides the submarine spring. The cameras were set up in a way to record the same amount of substrate image sections at both sites. A little higher substrate complexity occurred in the distance of the control site images. To overcome this obstacle distant fish in the very depth of the images were neglected during the counting process as they were basically out of the study site clearly evident by very small sizes and blurriness caused by the distance. Double counting of fish was minimized by recounting.
While there are abundant research questions that need answering regarding the impact of submarine springs on flora and fauna, the here presented results are the first to show that submarine springs do attract fish in tropical settings. This result is assumed to be induced by the nutrient inflow of the submarine spring as presented in our in situ biofouling experiment.
The datasets generated for this study can be found as Starke et al. (2018).
CS planned and carried out the experiments, analyzed the data, and wrote the manuscript with support of WE and NM. WE and NM supervised the project and provided critical feedback and helped to shape the research, analysis, and manuscript.
This study was funded through German Federal Ministry of Education and Research (BMBF) Grant #01LN1307A to NM.
The authors declare that the research was conducted in the absence of any commercial or financial relationships that could be construed as a potential conflict of interest.
We would like to thank Kathrin Haßler from Leibniz Centre for Tropical Marine Research (ZMT), Bremen, Germany for providing us with the nutrient concentration data of Trou de Lagon, Lionel Hertrich, and Isabelle Klein from Tahiti Iti Diving Centre for their support during the in situ implementation process. Also, Till Oehler and Stefanie Bröhl (ZMT) for their support in organizational and technical issues.
Amato, D. W., Bishop, J. M., Glenn, C. R., Dulai, H., and Smith, C. M. (2016). Impact of submarine groundwater discharge on marine water quality and Reef Biota of Maui. PLoS One 11:e0165825. doi: 10.1371/journal.pone.0165825
Beusen, A. H. W., Slomp, C. P., and Bouwman, A. F. (2013). Global land-ocean linkage: direct inputs of nitrogen to coastal waters via submarine groundwater discharge. Environ. Res. Lett. 8:034035. doi: 10.1088/1748-9326/8/3/034035
Burnett, W. C., Bokuniewicz, H., Huettel, M., Moore, W. S., and Taniguchi, M. (2003). Groundwater and pore water inputs to the coastal zone. Biogeochemistry 66, 3–33. doi: 10.1023/B:BIOG.0000006066.21240.53
Carleton, J. H., and Doherty, P. J. (1998). Tropical zooplankton in the highly-enclosed lagoon of Taiaro Atoll (Tuamotu Archipelago, French Polynesia). Coral Reefs 17, 29–35. doi: 10.1007/s003380050090
Cho, H.-M., Kim, G., Kwon, E. Y., Moosdorf, N., Garcia-Orellana, J., and Santos, I. R. (2018). Radium tracing nutrient inputs through submarine groundwater discharge in the global ocean. Sci. Rep. 8:2439. doi: 10.1038/s41598-018-20806-2
Cocito, S., Novosel, M., Pasarić, Z., and Key, M. M. (2006). Growth of the bryozoan Pentapora Fascialis (Cheilostomata, Ascophora) around submarine freshwater springs in the Adriatic Sea. Linzer Biol. Beitr. 38, 15–24.
Cole, J. J., Prairie, Y. T., Caraco, N. F., McDowell, W. H., Tranvik, L. J., Striegl, R. G., et al. (2007). Plumbing the global carbon cycle: integrating inland waters into the terrestrial carbon budget. Ecosystems 10, 172–185. doi: 10.1007/s10021-006-9013-8
Delcroix, T., and Hénin, C. (1991). Seasonal and interannual variations of sea surface salinity in the Tropical Pacific Ocean. J. Geophys. Res. 96, 22135–22150. doi: 10.1029/91JC02124
Dias, C. O., Araujo, A. V., Vianna, S. C., Loureiro Fernandes, L. F., Paranhos, R., Suzuki, M. S., et al. (2015). Spatial and temporal changes in biomass, production and assemblage structure of mesozooplanktonic copepods in the Tropical South-West Atlantic Ocean. J. Mar. Biol. Assoc. United Kingdom 95, 483–496. doi: 10.1017/S0025315414001866
Duck, R. W., and da Silva, J. F. (2012). Coastal lagoons and their evolution: a hydromorphological perspective. Estuar. Coast. Shelf. Sci. 110, 2–14. doi: 10.1016/j.ecss.2012.03.007
Duncan, R. A., Fisk, M. R., White, W. M., and Nielsen, R. L. (1994). Tahiti: geochemical evolution of a French Polynesian Volcano. J. Geophys. Res. 99, 24341–24357. doi: 10.1029/94jb00991
Flecker, A. S., and Feifarek, B. (1994). Disturbance and the temporal variability of invertebrate assemblages in two andean streams. Freshw. Biol. 31, 131–142. doi: 10.1111/j.1365-2427.1994.tb00847.x
Frouin, P. (2000). Effects of anthropogenic disturbances of tropical soft-bottom benthic communities. Mar. Ecol. Prog. Ser. 194, 39–53. doi: 10.3354/meps194039
Frouin, P., and Hutchings, P. (2001). Macrobenthic communities in a tropical lagoon (Tahiti, French Polynesia, central Pacific). Coral Reefs 19, 277–286. doi: 10.1007/PL00006961
Fujita, K., Shoji, J., Sugimoto, R., Nakajima, T., Honda, H., Takeuchi, M., et al. (2019). Increase in fish production through bottom-up trophic linkage in coastal waters induced by nutrients supplied via submarine groundwater. Front. Environ. Sci. 7.
Gobler, C. J., and Sañudo-Wilhelmy, S. A. (2001). Temporal variability of groundwater seepage and brown tide blooms in a long island embayment. Mar. Ecol. Prog. Ser. 217, 299–309. doi: 10.3354/meps217299
Gorsky, G., Ohman, M. D., Picheral, M., Gasparini, S., Stemmann, L., Romagnan, J. B., et al. (2010). Digital zooplankton image analysis using the zooscan integrated system. J. Plankt. Res. 32, 285–303. doi: 10.1093/plankt/fbp124
Gratwicke, B., and Speight, M. R. (2005). The relationship between fish species richness, abundance and habitat complexity in a range of shallow tropical marine habitats. J. Fish Biol. 66, 650–667. doi: 10.1111/j.1095-8649.2005.00629.x
Hata, M., Sugimoto, R., Hori, M., Tomiyama, T., and Shoji, J. (2016). Occurrence, distribution and prey items of juvenile marbled sole Pseudopleuronectes Yokohamae around a submarine groundwater seepage on a tidal flat in Southwestern Japan. J. Sea Res. 111, 47–53. doi: 10.1016/j.seares.2016.01.009
Haßler, K., Dähnke, K., Kölling, M., Sichoix, L., Nickl, A. L., and Moosdorf, N. (2019). Provenance of nutrients in submarine fresh groundwater discharge on Tahiti and Moorea, French Polynesia. Appl. Geochem. 100, 181–189. doi: 10.1016/j.apgeochem.2018.11.020
Johannes, R. E. (1980). The ecological significance of the submarine discharge of groundwater. Mar. Ecol. Prog. Ser. 3, 365–373. doi: 10.3354/meps003365
Johnson, A. G., Glenn, C. R., Burnett, W. C., Peterson, R. N., and Lucey, P. G. (2008). Aerial infrared imaging reveals large nutrient-rich groundwater inputs to the ocean. Geophys. Res. Lett. 35:L15606. doi: 10.1029/2008GL034574
Ka’eo Duarte, T., Hemond, H. F., Frankel, D., and Frankel, S. (2006). Assessment of submarine groundwater discharge by handheld aerial infrared imagery: case study of Kaloko Fishpond and Bay, Hawai’i. Limnol. Oceanogr. 4, 227–236. doi: 10.4319/lom.2006.4.227
Kamermans, P., Hemminga, M. A., Tack, J. F., Mateo, M. A., Marbà, N., Mtolera, M., et al. (2002). Groundwater effects on diversity and abundance of lagoonal seagrasses in Kenya and on Zanzibar Island (East Africa). Mar. Ecol. Prog. Ser. 231, 75–83. doi: 10.3354/meps231075
Kjerfve, B. (1986). “Comparative oceanography of coastal lagoons,” in Estuarine Variability, ed. D. A. Wolfe (New York, NY: Academic Press), 63–81. doi: 10.1016/B978-0-12-761890-6.50009-5
Knee, K. L., and Paytan, A. (2011). Submarine Groundwater Discharge. Treatise on Estuarine and Coastal Science. Amsterdam: Elsevier, 205–233. doi: 10.1016/B978-0-12-374711-2.00410-1
Köhler, W., Schachtel, G., and Voleske, P. (2012). “Biostatistik,” in Eine Einführung für Biologen und Agrarwissenschaftler, Berlin: Springer-Verlag.
Kuiter, R. H., and Tonozuka, T. (2001). “Pictorial guide to indonesian reef fishes. Part 3. Jawfishes - Sunfishes, Opistognathidae - Molidae,” in Zoonetics. Australia, Ref. No. 48637, 623–893.
Kwon, E. Y., Kim, G., Primeau, F., Moore, W. S., Cho, H.-M., DeVries, T., et al. (2014). Global estimate of submarine groundwater discharge based on an observationally constrained radium isotope model. Geophys. Res. Lett. 41, 8438–8444. doi: 10.1002/2014GL061574
Lapointe, B. E. (1997). Nutrient thresholds for bottom-up control of macroalgal blooms on Coral Reefs in Jamaica and Southeast Florida. Limnol. Oceanogr. 42, 1119–1131. doi: 10.4319/lo.1997.42.5_part_2.1119
Lapointe, B. E., Barile, P. J., Littler, M. M., and Littler, D. S. (2005). Macroalgal blooms on southeast florida coral reefs. Harmful Algae 4, 1106–1122. doi: 10.1016/j.hal.2005.06.002
Laroche, J., Nuzzi, R., Waters, R., Wyman, K., Falkowski, P., and Wallace, D. (1997). Brown tide blooms in long island’s coastal waters linked to interannual variability in groundwater flow. Global Change Biol. 3, 397–410. doi: 10.1046/j.1365-2486.1997.00117.x
Lecher, A., and Mackey, K. R. M. (2018). Synthesizing the effects of submarine groundwater discharge on marine biota. Hydrology 5:60. doi: 10.3390/hydrology5040060
Lilkendey, J., Pisternick, T., Neumann, S. I., Dumur Neelayya, D., Bröhl, S., Neehaul, Y., et al. (2019). Fresh submarine groundwater discharge augments growth in a reef fish. Front. Mar. Sci. 6.
Liu, J., Su, N., Wang, X., and Du, J. (2017). Submarine groundwater discharge and associated nutrient fluxes into the southern yellow sea: a case study for semi-enclosed and oligotrophic seas-implication for green tide bloom. J. Geophys. Res. Oceans 122, 139–152. doi: 10.1002/2016JC012282
McClanahan, T. R. (1994). Kenyan coral reef lagoon fish: effects of fishing, substrate complexity, and Sea Urchins. Coral Reefs 13, 231–241. doi: 10.1007/BF00303637
Miller, D. C., and Ullman, W. J. (2004). Ecological consequences of ground water discharge to Delaware Bay, United States. Ground Water 42, 959–970. doi: 10.1111/j.1745-6584.2004.tb02635.x
Moore, W. S. (2010). The effect of submarine groundwater discharge on the ocean. Annu. Rev. Mar. Sci. 2, 59–88. doi: 10.1146/annurev-marine-120308-081019
Moosdorf, N., and Oehler, T. (2017). Societal use of fresh submarine groundwater discharge: an overlooked water resource. Earth Sci. Rev. 171, 338–348. doi: 10.1016/j.earscirev.2017.06.006
Moosdorf, N., Stieglitz, T., Waska, H., Dürr, H. H., and Hartmann, J. (2015). Submarine groundwater discharge from tropical islands: a review. Grundwasser 20, 53–67. doi: 10.1007/s00767-014-0275-3
Neuswanger, J. R., Wipfli, M. S., Rosenberger, A. E., and Hughes, N. F. (2016). Measuring fish and their physical habitats: versatile 2D and 3D video techniques with user-friendly software. Can. J. Fish. Aquat. Sci. 73, 1861–1873. doi: 10.1139/cjfas-2016-0010
Novosel, M., Olujic, G., Cocito, S., and Pozar-Domac, A. (2005). “Submarine freshwater springs in the adriatic sea: a unique habitat for the Bryozoan Pentapora fascialis,” in Proceedings of the 13th International Conference of the International Bryozoology Association (Chile: IBA).
Oehler, T., Eiche, E., Putra, D., Adyasari, D., Hennig, H., Mallast, U., et al. (2018). Seasonal variability of land-ocean groundwater nutrient fluxes from a Tropical Karstic Region (Southern Java, Indonesia). J. Hydrol. 565, 662–671. doi: 10.1016/j.jhydrol.2018.08.077
Paerl, H. W. (1997). Coastal eutrophication and harmful algal blooms: importance of atmospheric deposition and groundwater as ‘new’ nitrogen and other nutrient sources. Limnol. Oceanogr. 42, 1154–1165. doi: 10.4319/lo.1997.42.5_part_2.1154
Paytan, A., Shellenbarger, G. G., Street, J. H., Gonneea, M. E., Davis, K., Young, M. B., et al. (2006). Submarine groundwater discharge: an important source of new inorganic nitrogen to coral reef ecosystems. Limnol. Oceanogr. 51, 343–348. doi: 10.4319/lo.2006.51.1.0343
Rougerie, F., Fichez, R., and Déjardin, P. (1997). Geomorphology and hydrogeology of selected islands of French Polynesia: Tikehau (Atoll) and Tahiti (Barrier Reef). Geology and hydrogeology of carbonate islands. Dev. Sedimentol. 54, 475–502. doi: 10.1016/s0070-4571(04)80037-2
Sarno, D., Zingone, A., Saggiomo, V., and Carrada, G. (1993). Phytoplankton biomass and species composition in a Mediterranean coastal lagoon. Hydrobiologia 271, 27–40. doi: 10.1007/BF00005692
Shaban, A., Khawlie, M., Abdallah, C., and Faour, G. (2005). Geologic controls of submarine groundwater discharge: application of remote sensing to North Lebanon. Environ. Geol. 47, 512–522. doi: 10.1007/s00254-004-1172-3
Shoji, J., and Tominaga, O. (2018). “Relationships between submarine groundwater discharge and coastal fisheries as a water-food Nexus,” in The Water-Energy-Food Nexus, eds A. Endo, and T. Oh (Singapore: Springer), 117–131. doi: 10.1007/978-981-10-7383-0_9
Slomp, C. P., and Van Cappellen, P. (2004). Nutrient inputs to the coastal ocean through submarine groundwater discharge: controls and potential impact. J. Hydrol. 295, 64–86. doi: 10.1016/j.jhydrol.2004.02.018
Smith, C. G., and Swarzenski, P. W. (2012). An investigation of submarine groundwater-borne nutrient fluxes to the west florida shelf and recurrent harmful algal blooms. Limnol. Oceanogr. 57, 471–485. doi: 10.4319/lo.2012.57.2.0471
Starke, C., Ekau, W., and Moosdorf, N. (2018). “Impact of submarine groundwater discharge on fish and zooplankton abundances and on early biofouling processes in a coastal la goon of Tahiti, French Polynesia,” in Leibniz-Zentrum für Marine Tropenforschung, Bremen, Germany: PANGAEA. Available at: https://doi.org/10.1594/PANGAEA.888416.
Sternberg, S. R. (1983). Biomedical image processing. Computer 16, 22–34. doi: 10.1109/MC.1983.1654163
Stieglitz, T., and Ridd, P. (2000). “Submarine groundwater discharge from paleochannels?: ‘Wonky Holes’ on the Inner Shelf of the Great Barrier Reef, Australia,” in Proceedings of the international conference on Hydro 2000: Interactive Hydrology (Barton: Institution of Engineers).
Tait, D. R., Erler, D. V., Santos, I. R., Cyronak, T. J., Morgenstern, U., and Eyre, B. D. (2014). The influence of groundwater inputs and age on nutrient dynamics in a Coral Reef Lagoon. Mar. Chem. 166, 36–47. doi: 10.1016/j.marchem.2014.08.004
Tiralongo, F., and Baldacconi, R. (2014). A conspicuous population of the long-snouted seahorse, Hippocampus guttulatus (Actinopterygii: Syngnathiformes: Syngnathidae), in a highly polluted mediterranean coastal lagoon. Acta Ichthyol. Piscatoria 44, 99–104. doi: 10.3750/AIP2014.44.2.02
Umezawa, Y., Miyajima, T., Yamamuro, M., Kayanne, H., and Koike, I. (2002). Fine-scale mapping of land-derived nitrogen in coral reefs by δ 15 N in Macroalgae. Limnol. Oceanogr. 47, 1405–1416. doi: 10.4319/lo.2002.47.5.1405
Utsunomiya, T., Hata, M., Sugimoto, R., Honda, H., Kobayashi, S., Miyata, Y., et al. (2015). Higher species richness and abundance of fish and benthic invertebrates around submarine groundwater discharge in Obama Bay, Japan. J. Hydrol. Reg. Stud. 11, 139–146. doi: 10.1016/j.ejrh.2015.11.012
Keywords: submarine groundwater discharge, in situ primary productivity, nutrient input, fish abundance, bottom-up control
Citation: Starke C, Ekau W and Moosdorf N (2020) Enhanced Productivity and Fish Abundance at a Submarine Spring in a Coastal Lagoon on Tahiti, French Polynesia. Front. Mar. Sci. 6:809. doi: 10.3389/fmars.2019.00809
Received: 16 January 2019; Accepted: 16 December 2019;
Published: 21 January 2020.
Edited by:
Makoto Taniguchi, Research Institute for Humanity and Nature, JapanReviewed by:
Francesco Tiralongo, Ente Fauna Marina Mediterranea (EFMM), ItalyCopyright © 2020 Starke, Ekau and Moosdorf. This is an open-access article distributed under the terms of the Creative Commons Attribution License (CC BY). The use, distribution or reproduction in other forums is permitted, provided the original author(s) and the copyright owner(s) are credited and that the original publication in this journal is cited, in accordance with accepted academic practice. No use, distribution or reproduction is permitted which does not comply with these terms.
*Correspondence: Claudia Starke, c3RhcmtlY2xhdWRpYTgyQGdtYWlsLmNvbQ==
Disclaimer: All claims expressed in this article are solely those of the authors and do not necessarily represent those of their affiliated organizations, or those of the publisher, the editors and the reviewers. Any product that may be evaluated in this article or claim that may be made by its manufacturer is not guaranteed or endorsed by the publisher.
Research integrity at Frontiers
Learn more about the work of our research integrity team to safeguard the quality of each article we publish.