- 1University of Cologne-Centre for Accelerator Mass Spectrometry, University of Cologne, Cologne, Germany
- 2Organic Geochemistry Laboratory, Department of Geological Sciences, Institute of Arctic and Alpine Research, University of Colorado Boulder, Boulder, CO, United States
- 3Skidaway Institute of Oceanography, University of Georgia, Savannah, GA, United States
We investigated the origin of sedimentary bacteriohopanepolyols (BHPs) in low-density (<1.6 g cm–3), mesodensity (1.6–2.0 and 2.0–2.5 g cm–3) and high-density fractions (>2.5 g cm–3) as well as unfractionated bulk samples in a highly dynamic coastal setting along a transect spanning from the Mississippi Delta into the Gulf of Mexico (GOM). We observe selective partitioning of BHPs among density fractions both in total abundance and structural diversity. BHPs primarily accumulate in the low-density fraction at all sites. Good correlation with other particle properties [surface area-normalized organic carbon loadings (OC/SA), C/N ratios, Δ14C values, and lignin phenol abundances] and the spatial distribution of absolute and relative BHP abundances suggests that a significant fraction of BHPs are terrestrially sourced and entrained in the OC pool that is stabilized on clay particles or associated with plant fragments. Only a small subset of BHPs seems to have a significant autochthonous origin at the station furthest offshore and they are associated with the low and mesodensity fractions. While provenance and hydrodynamic sorting of particles across the shelf seem to primarily determine BHP inventories along the transect, the samples also harbor an unusual diversity of amino-functionalized BHPs. A subset of these BHPs appears to derive from aerobic methane oxidizing bacteria and are likely exported with particles from the coastal swamps in the Mississippi Delta. Other amino-functionalized BHPs seem to derive from aerobic ammonia oxidizing bacteria thriving in the nutrient-rich Mississippi River plume. We find no evidence for anaerobic ammonium oxidizing bacteria related to recurring seasonal hypoxia on the Louisiana Shelf.
Introduction
Bacteriohopanepolyols (BHPs) are pentacyclic triterpenoids mainly synthesized by bacteria. Their physiological role in cell membranes has so far been linked to lipid raft formation (Sáenz, 2010; Sáenz et al., 2012), pH homeostasis (Welander et al., 2009; Schmerk et al., 2011), antibiotic resistance (Schmerk et al., 2011), temperature stress (Doughty et al., 2011), intracytoplasmic membrane formation and cell survival in late stationary phase (Welander and Summons, 2012), and potentially cell curvature generation (Doughty et al., 2014). In addition to improving membrane resistance in the free-living state, BHPs also appear to aid in plant-microbe symbiosis (Silipo et al., 2014; Kulkarni et al., 2015).
Although there is growing evidence that BHPs may be useful biomarkers to trace cell physiological processes, their environmental and taxonomic origin remains largely elusive, particularly in the marine environment. Studies investigating the distribution of BHPs in the marine water column and sediments are still relatively sparse and the global ocean metagenome shows that most BHP-producing bacteria are yet to be characterized taxonomically (Pearson and Rusch, 2009). However, the capability of marine bacteria to produce BHPs, as determined based on squalene-hopene-cyclase (sqhC) gene abundances, seems to be phylogenetically diverse, yet the BHP diversity does not seem to mirror the genetic diversity (Pearson and Rusch, 2009; Kharbush et al., 2013). Generally, BHP diversity seems to be higher under suboxic or anoxic marine conditions in comparison to oxic marine environments (Wakeham et al., 2007; Sáenz et al., 2011b; Kharbush et al., 2013), but both sqhC abundances and BHPs are much more varied in terrestrial settings in comparison to fully marine settings (Pearson et al., 2007, 2009; Sáenz et al., 2011a). While some BHPs have been assigned a primarily terrestrial origin, namely the soil-marker BHPs (Cooke et al., 2008; Zhu et al., 2011), we still lack a comprehensive understanding of the extent to which the diversity of BHPs in marine sediments derives from the continent, whether they are exported with the gross terrestrial organic carbon (OC) pool or by distinct OC sub-pools, and the mechanisms by which they are transported and/or exported within the ocean. Density fractionation of marine sediments may provide a valuable tool to understand these processes (Arnarson and Keil, 2001; Sampere et al., 2008; Wakeham et al., 2009; Wakeham and Canuel, 2016).
Here, we investigate the BHP molecular compositions of bulk sediment and sedimentary density fractions along an export transect from the Mississippi River to the northern Gulf of Mexico (GOM) for which previous studies have revealed selective partitioning of bulk OC and lignin phenols in density fractions (Sampere et al., 2008; Wakeham et al., 2009). In this highly dynamic shelf system, the OC distribution seems to be primarily determined by OC provenance and density-driven hydrodynamic sorting, which includes re-distribution and aging during cross-shelf transport in the benthic boundary layer (Sampere et al., 2008; Wakeham et al., 2009). Terrestrial OC is particularly enriched in the <1.6 g cm–3 density fraction as indicated by high lignin phenol abundances, high C/N ratios, and pronounced δ13C- and Δ14C-depletion. In contrast, the mesodensity fractions (1.6–2.0 and 2.0–2.5 g cm–3) have much lower lignin concentrations, lower C/N ratios, and enriched δ13C and Δ14C values indicative of a significant marine component (Wakeham et al., 2009). In conjunction with these previous data, we aim at deciphering the origin of BHPs (both provenance and biogeochemical sources), the importance of hydrodynamic sorting on sedimentary BHP inventories, and diagenetic effects that might occur during cross-shelf transport or seasonal hypoxia.
Materials and Methods
Three surface sediment samples (0–5 cm) were taken along a transect from the Mississippi River into the GOM during an R/V Pelican cruise in 2003 (Figure 1). Station Miss R (29°15.73′N, 89°20.15′W; 26 m water depth) was sampled within the Mississippi Delta, station GOM 50 (28°55.80′N, 89°30.39′W; 50 m water depth) in the marine prodelta close to the river mouth, and station GOM 540 (28°31.63′N, 89°47.98′W; 540 m water depth) at the head of the Mississippi Canyon. Samples were retrieved using a box corer (50 × 50 × 50 cm), the upper 5 cm of the box core were subsampled and homogenized on board, and samples were stored frozen at −20°C until analysis. Environmental conditions [water depth, salinity, oxygen content, and fluorescence (chlorophyll a)] were collected from each site during the cruise using a CTD (conductivity–temperature–depth) sensor (Waterson and Canuel, 2008).
Samples were extracted in bulk or following partitioning into four density fractions using sodium metatungstate solutions corresponding to <1.6, 1.6–2.0, 2.0–2.5 and >2.5 g cm–3; a detailed description of the density fractionation procedure is provided by Wakeham et al. (2009). Lipids were extracted from freeze-dried bulk sediments (25–40 g dry weight; gdw) and density-fractionated sediments (0.1–12 gdw) using an accelerated solvent extractor (ASE) and dichloromethane:methanol (2:1). Extracts were archived at −20°C until aliquots were taken for this investigation. The TLE aliquots were spiked with 5α-pregnane-3β,20β-diol as internal standard and acetylated using pyridine: acetic acid 1:1 (v:v) at 50°C for 1 h and then left reacting overnight. Afterwards, the samples were filtered over 0.45 μm PTFE filters using 3:2 methanol: propan-2-ol (v:v). BHPs were analyzed using a Thermo Ultimate 3000 HPLC connected to a Q Exactive Focus Orbitrap MS equipped with an APCI ion source in the Organic Geochemistry Laboratory at the University of Colorado Boulder. Chromatographic separation was achieved on an ACE® C18 column (2.1 × 150 mm, 3 μm; Advanced Chromatography Technologies Ltd.) maintained at 25°C. BHPs were chromatographically separated using the solvent gradient described in Talbot et al. (2007) and a flow rate of 0.2 ml/min. APCI settings were optimized for bacteriohopanetetrol (BHT), 2-methyl-BHT (2Me-BHT), aminotriol, adenosylhopane, BHT cyclitol ether, BHT glucosamine, BHT pentose, and BHhexol cyclitol ether (Figure 2) using an in-house standard sample and were as follows: vaporizer temperature 325°C, capillary temperature 155°C, corona discharge current 8, sheath gas flow 40 arbitrary units (AU), aux gas flow 10 AU, S lens RF level 50 AU. BHP concentrations are reported semi-quantitatively. In the absence of commercially available authentic BHP standards, accurate MS response factors between 5α-pregnane-3β,20β-diacetate and BHPs with different functionalities cannot be determined. However, all samples were analyzed with the same Orbitrap MS system, thus, differences in the respective BHP inventories are directly comparable between samples.
Results
Bacteriohopanepolyol diversity (Figure 3) is high in the Mississippi River and GOM samples (7–24 BHPs). Besides various composite and soil-marker BHPs (listed in Figure 3), which have more complex sugar or amino sugar side chain structures, we observe an unusual diversity of amino-functionalized BHPs including N-acylated aminotriol with C16 or C19 moieties, isomers of aminotriol and methylated aminotriol, and aminopentol and aminotetrol alongside some more ubiquitous (other) BHPs. The structural BHP diversity is relatively similar in the bulk sediments and the <1.6, 1.6–2.0, and 2.0–2.5 g cm–3 density fractions, but lower in the high density (>2.5 g cm–3) fractions. Total mass-normalized BHP concentrations (Figure 4) range from 0.6 ng g–1 (GOM 50) to 15.4 ng g–1 (Miss R) in the bulk samples and 0.1 ng g–1 (Miss R > 2.5 g cm–3) to 279.5 ng g–1 (GOM 540 < 1.6 g cm–3) in the density fractions. Total BHP concentrations are highest in the <1.6 g cm–3 fraction at each station and also significantly enriched in the 1.6–2.0 g cm–3 fraction at station GOM 540 (Figure 4). Along the transect, BHP concentrations are highest at station Miss R and lowest at station GOM 50. This pattern is also evident for most individual BHPs except for BHT-CE, BHT glucosamine, 3Me-BHT, BHT, BHT II, and anhydroBHT, which show highest concentrations at station GOM 540 (Figure 5). We observe good correlation between total BHP concentrations (Figure 4) and individual BHP concentrations (Figure 5) (Pearson correlation coefficient r > 0.57) for all BHPs except for those that were detected in a few samples only (e.g., 2Me-BHT pentose, BHT pentose, aminotriol II, 2Me-adenosylhopane-type 3).
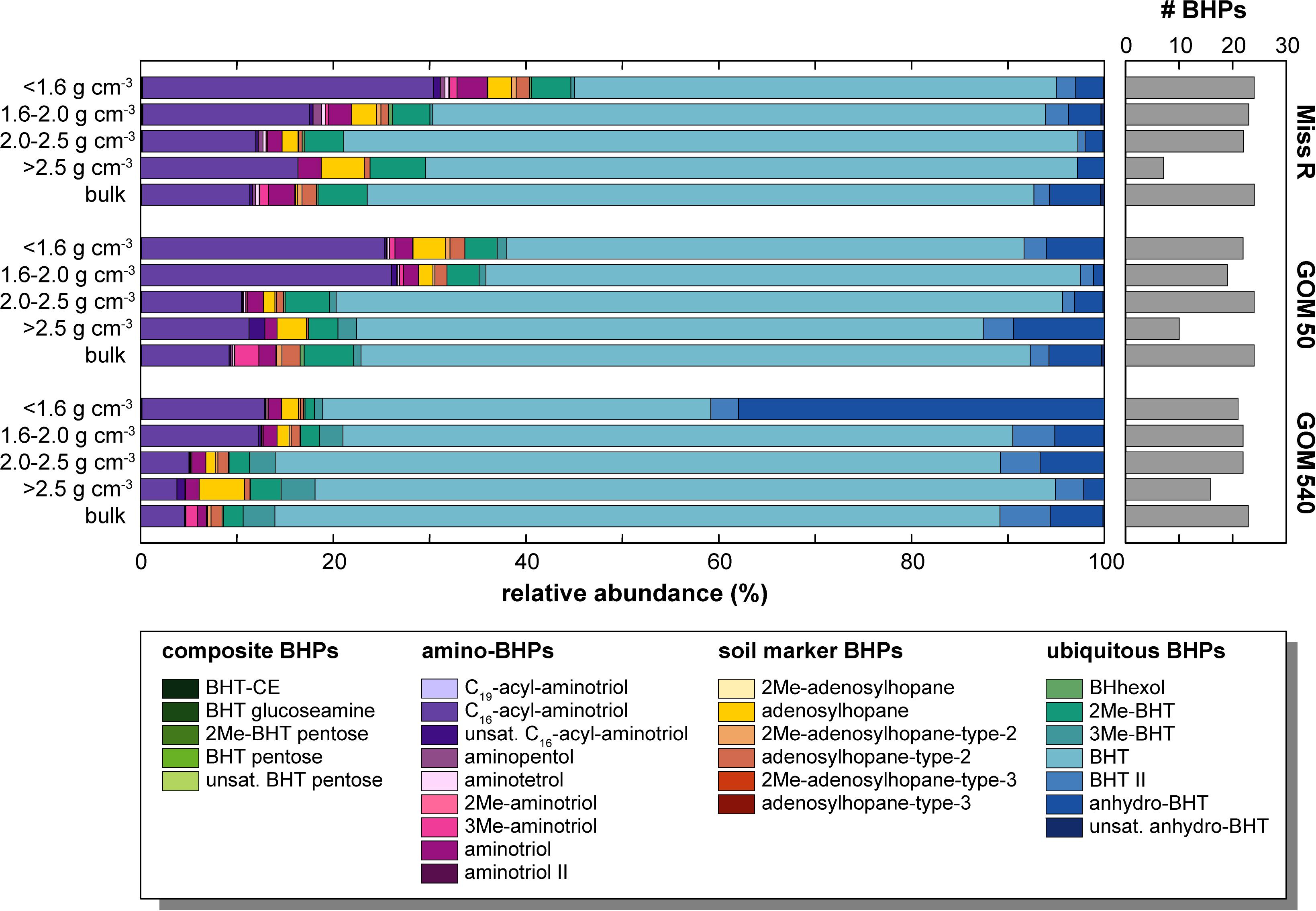
Figure 3. Relative abundance and structural diversity of BHPs in density fractions and bulk sediment samples along the Miss R – GOM transect. Note that relative abundances <1% are not well resolved graphically, we refer to Supplementary Table S1 for details.
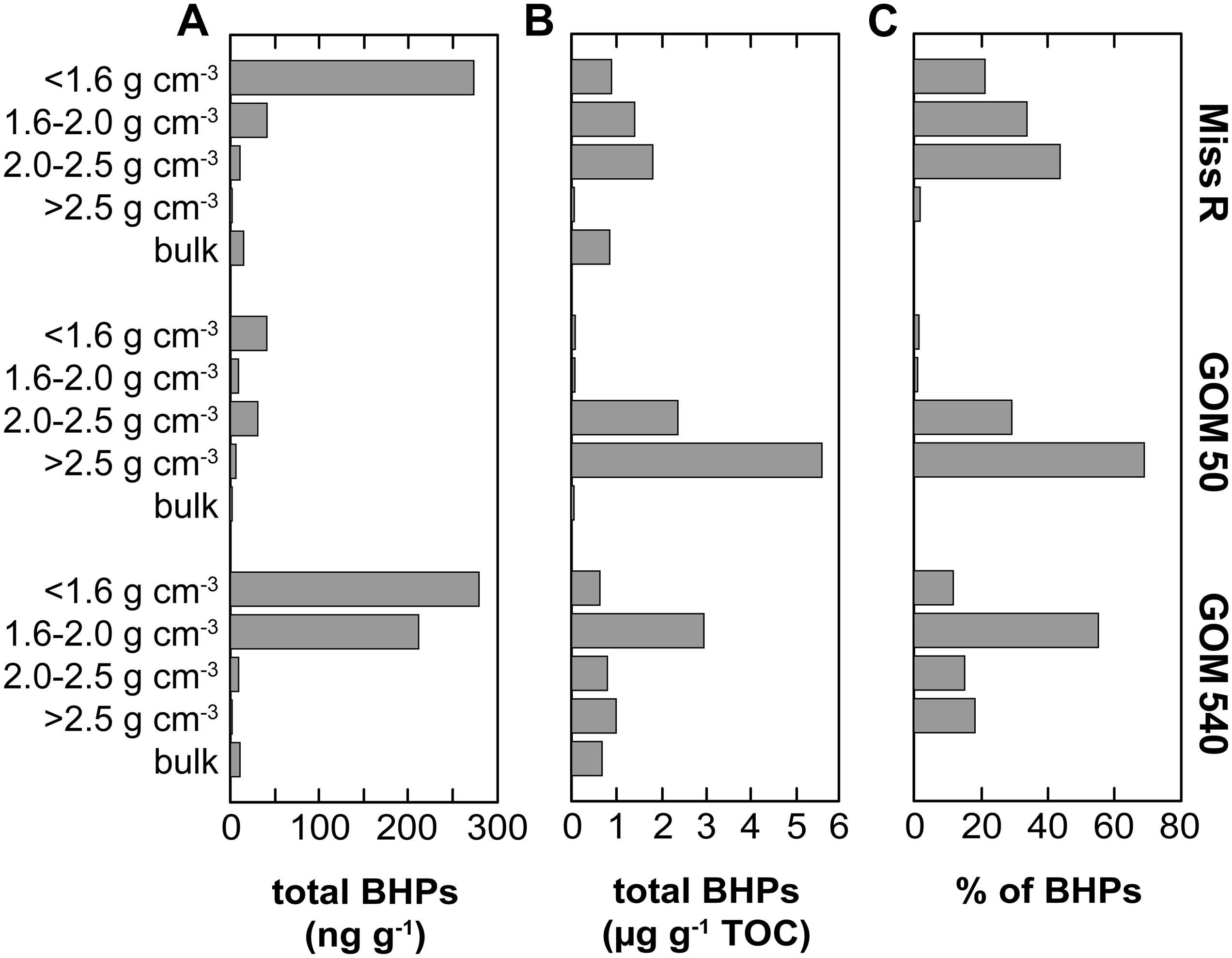
Figure 4. Abundance of BHPs in density fractions and bulk sediment samples along the Miss R – GOM transect. (A) Mass-normalized absolute BHP abundance and (B) TOC-normalized absolute BHP abundance calculated based on the data of Wakeham et al. (2009). (C) Relative abundance of BHPs among density fractions.
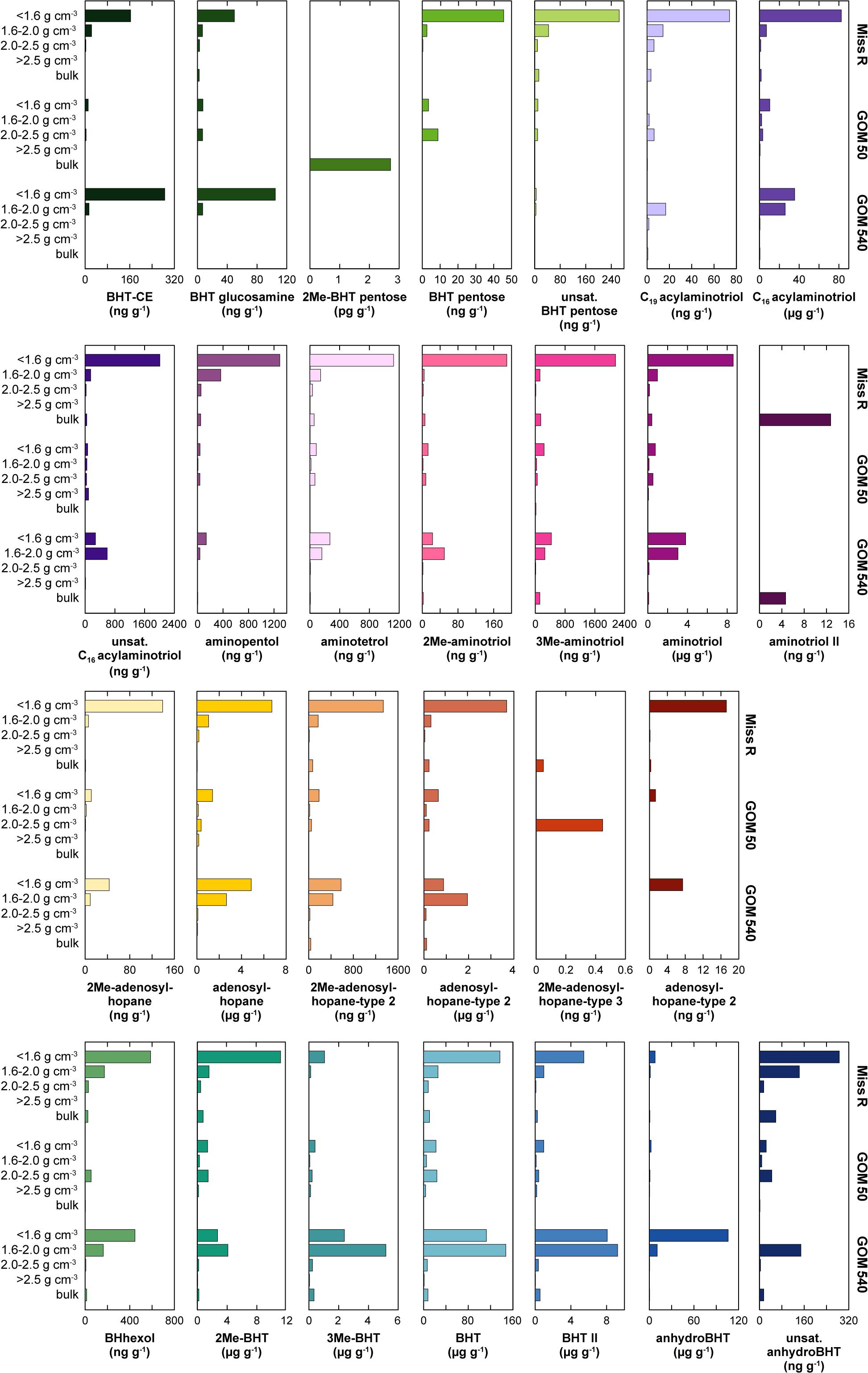
Figure 5. Absolute abundance of individual BHPs in density fractions and bulk sediment samples along the Miss R – GOM transect. Individual BHP abundances are mass-normalized to better reflect the physical partitioning of particles during cross shelf transport. Low abundances are not well resolved graphically, we refer to Supplementary Table S1 for details. Also note different scales.
Bacteriohopanetetrol is the most abundant BHP (40.3–76.8% of total BHPs) in all samples irrespective of density fraction (Figures 3, 5), thus, primarily determining total BHP concentrations (r = 0.967). However, at all stations, relative BHT abundances are consistently lower (by 8.0–36.5%) in the <1.6 g cm–3 fraction compared to in the other density fractions, all of which agree within a standard deviation (SD) of 5%. C16-acyl-aminotriol is the second most abundant BHP (3.8–30.2%) in the majority of samples and is particularly abundant in the <1.6 and 1.6–2.0 g cm–3 density fractions. Adenosylhopane, 2Me-BHT, 3Me-BHT, and BHT II also occur in high relative abundance in all samples as well as anhydroBHT, which is particularly abundant in the <1.6 g cm–3 fraction at station GOM 540.
Overall, soil-marker BHP relative abundances are low compared to BHT abundances, resulting in low Rsoil indices [soil-marker BHPs/(soil-marker BHPs + BHT) (Zhu et al., 2011)], which are <0.1 in all samples. Rsoil indices are highest in the <1.6 and >2.5 g cm–3 fractions at each station (Figure 6). Moreover, relative abundances among soil-marker BHPs differ between density fractions and bulk samples. While adenosylhopane is the most abundant soil-marker BHP (42.1–93.7% of that subset) in all density fractions except the 2.0–2.5 g cm–3 fraction at station GOM 540, it only represents 2.5–8.8% of the soil-marker BHPs in the bulk samples (Figure 6). Among soil-marker BHPs, bulk sediment samples are characterized by high abundances of adenosylhopane-type-2 (69.3–74.8%).
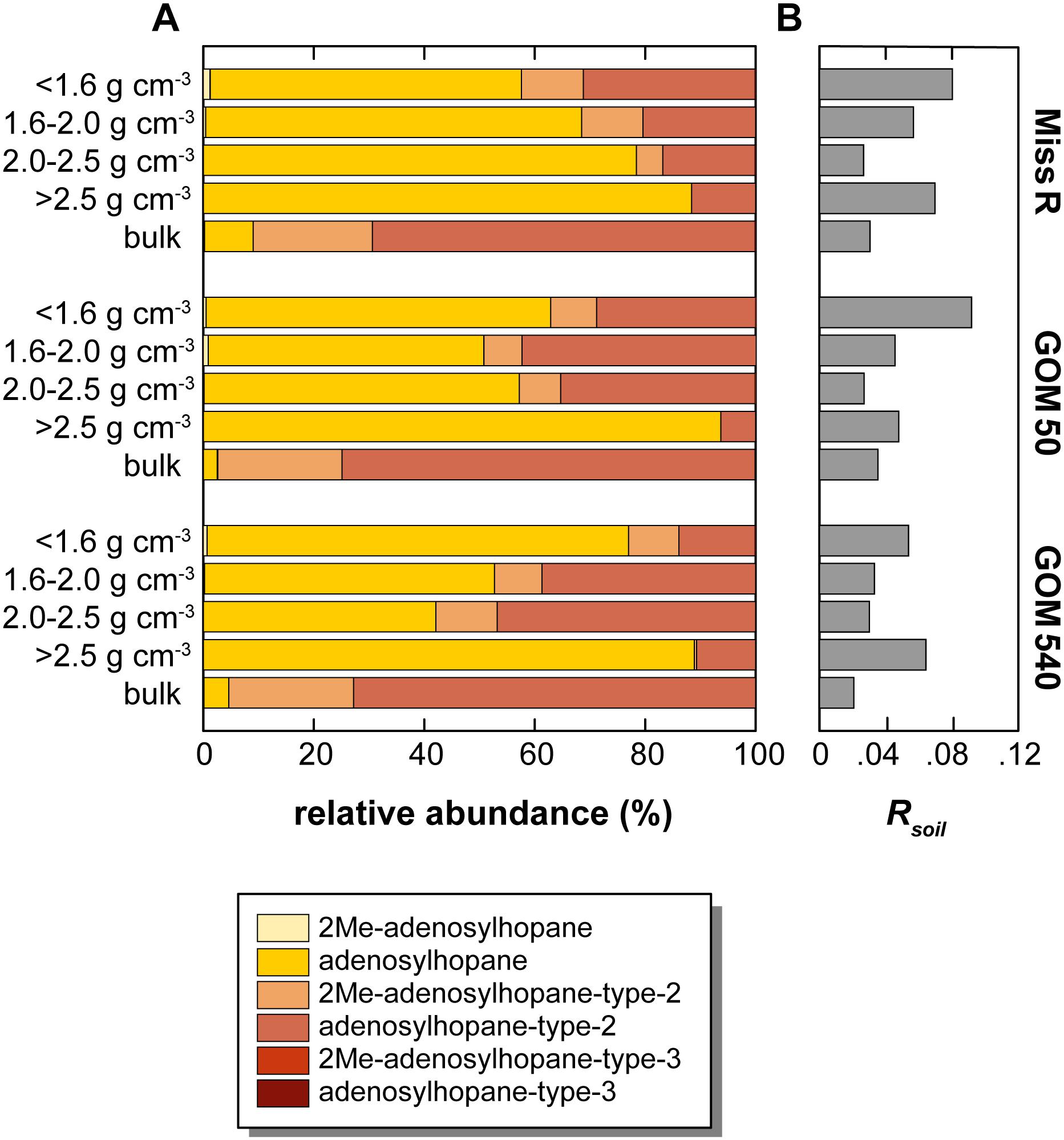
Figure 6. Relative abundance of soil-marker BHPs in density fractions and bulk sediment samples along the Miss R – GOM transect. (A) Relative abundance of soil-marker BHPs, (B) Rsoil values. Note that relative abundances <1% are not well resolved graphically, we refer to Supplementary Table S1 for details.
Discussion
Density Partitioning of BHPs
Organic carbon is partitioned among the different density fractions of the three sediments at stations Miss R, GOM 50, and GOM 540 (Wakeham et al., 2009). OC is particularly enriched in the <1.6 g cm–3 density fraction, which has the highest OC/SA loadings, while the >2.5 g cm–3 fraction is OC-poor, primarily consists of unaggregated mineral grains, and has low OC/SA loadings (Figure 7). Moreover, C/N ratios decrease with increasing density and the <1.6 g cm–3 density fractions are significantly enriched in lignin phenols (Figure 7). Carbon isotope data reveal that the <1.6 g cm–3 fraction is also more δ13C- and Δ14C-depleted while the mesodensity fractions (1.6–2.5 g cm–3) are generally most isotopically enriched (Figure 7). These observations were primarily attributed to selective transport (hydrodynamic sorting), selective preservation (clay mineral association), and/or provenance of the OC (Hedges et al., 1997; Baldock and Skjemstad, 2000; Arnarson and Keil, 2001).
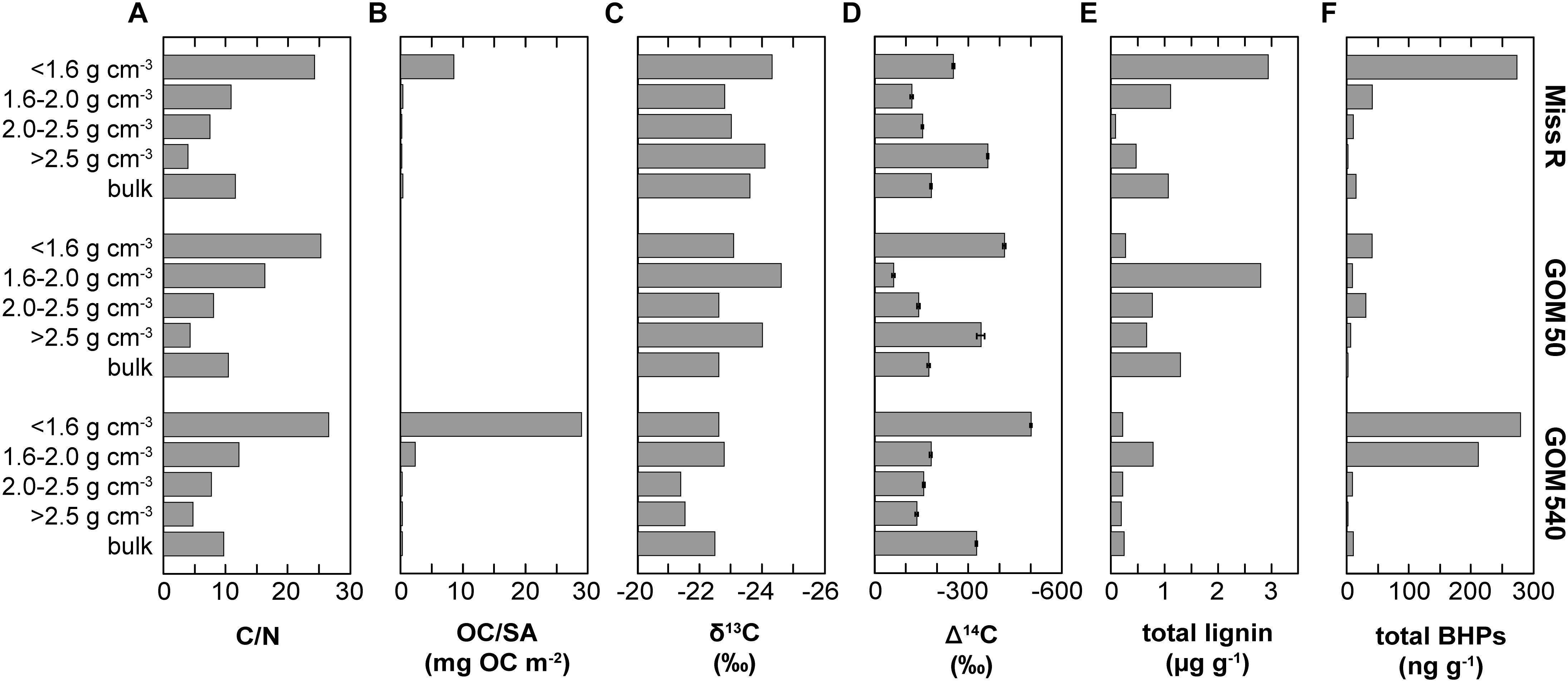
Figure 7. Relationship between sedimentary parameters and BHP abundances in density fractions and bulk sediment samples along the Miss R – GOM transect. (A) C/N ratios, (B) surface area-normalized organic carbon loadings (OC/SA), (C) δ13C of OC, (D) Δ14C of OC, (E) total lignin phenol concentrations, and (F) mass-normalized absolute BHP abundances. Data displayed in panels (A) through (E) were previously published by Wakeham et al. (2009).
Overall, our BHP data agree well with these previous results (Figure 7). BHPs generally are enriched in the <1.6 g cm–3 density fractions and total BHP concentrations correlate particularly well with OC/SA ratios (r = 0.760). These features could simply reflect a preference of aquatic heterotrophic bacteria (both in the water column and in the sediment) for those particles with high OC/SA loadings (i.e., high substrate availability) irrespective of particle origin. However, total BHP concentrations also correlate with C/N ratios (r = 0.697) and, to a lesser extent, with Δ14C values (r = −0.369), and soil-marker BHP concentrations correlate positively with lignin phenol concentrations (r = 0.447). This rather suggests that significant amounts of BHPs are indeed terrestrially sourced and entrained in the OC pool that is stabilized on clay particles or associated with plant fragments (Arnarson and Keil, 2001; Wakeham et al., 2009; Wakeham and Canuel, 2016). Accordingly, the processes governing general OC properties likely also influence BHP abundances, including selective transport and preservation and/or OC provenance.
The relative abundances of BHPs in the different density fractions (Figure 3), as well as the bulk samples, also vary. The relative abundance of composite, soil-marker, and amino-BHPs is highest in the <1.6 and 1.6–2.0 g cm–3 fractions and shows a decreasing trend with distance from the Mississippi River consistent with changing provenance of BHPs across the shelf. However, soil-marker BHP relative abundances reveal a substantial difference between bulk sediment samples and density fractions (Figure 6). While adenosylhopane is the dominant soil-marker BHP in the density fractions (42.1–93.7% of soil marker BHPs), its relative abundance is significantly lower in the bulk samples (2.5–8.8%), which have distinctly higher adenosylhopane-type-2 (69.3–74.8%) and 2Me-adenosylhopane-type-2 (21.6–22.6%) abundances. Accordingly, the bulk sample relative BHP distribution cannot mathematically be explained (as per mass balance) by any admixture of the different density fractions. Unfortunately, we cannot determine whether the different distributions represent true environmental heterogeneity of soil-marker BHPs or whether they are due to analytical effects stemming from the density fractionation procedure. Nevertheless, differences between relative BHP abundances in bulk samples and density fractions appear to be less pronounced for composite, amino, and “other” (rather ubiquitous) BHPs, although bulk samples show much higher 3Me-aminotriol abundances than the respective density fractions (Figures 3, 5).
Soil-marker BHP concentrations agree well with total BHP concentrations (r = 0.933) and are highest in the <1.6 g cm–3 fractions of stations Miss R and GOM 540, while they are notably lower at station GOM 50. Other individual BHP concentrations follow this trend except BHT-CE, BHT glucosamine, 3Me-BHT, BHT, BHT II, and anhydroBHT, which have highest concentrations in the <1.6 g cm–3 and/or 1.6–2.0 g cm–3 fractions at station GOM 540 (Figure 5). This pattern is not evident for lignin phenols, thus, unlikely results from solely hydrodynamic sorting of terrestrial particles across the shelf. Instead it suggests additional input of BHPs at station GOM 540, implying that both sources and provenance determine BHP abundances spatially and among density fractions.
BHP Sources and Provenance
Autochthonous vs. Allochthonous Input
The Mississippi Delta and adjacent shelf represents a highly dynamic environment that receives input from various autochthonous and allochthonous OC sources (Goñi et al., 1997; Waterson and Canuel, 2008; Wakeham et al., 2009). Previous analyses of our samples revealed that C/N ratios decrease along the transect and bulk samples as well as long-chain fatty acids and the <1.6 g cm–3 density fraction are characterized by increasing δ13C and decreasing Δ14C values along the transect (Figure 7; Wakeham et al., 2009). These trends indicate an increasing proportion of marine OC with distance offshore and aging of terrestrial OC during cross-shelf transport. This general pattern is also mirrored in the relative abundances of autochthonous and allochthonous fatty acids as well as sterols in the same (bulk) samples (Waterson and Canuel, 2008), while lignin phenols only display this trend in the <1.6 g cm–3 density fractions (Sampere et al., 2008; Wakeham et al., 2009).
Data relevant to transport of BHPs from continent to ocean in other regions show high BHP diversity in terrestrial settings in comparison to low diversity in marine settings (Pearson et al., 2009; Sáenz et al., 2011a; Zhu et al., 2011). Accordingly, the consistently high BHP diversity in our samples suggests a substantial terrestrial BHP component in all of our samples. Soil-marker BHPs and the associated Rsoil index are the primary tool to trace the input of terrestrial BHPs into marine systems (Handley et al., 2010; Zhu et al., 2011; Doğrul Selver et al., 2015; De Jonge et al., 2016), although adenosylhopane has also been shown to be an intermediate in BHP side chain synthesis (Bradley et al., 2010). The good correlation of soil-marker BHP concentrations with C/N ratios (r = 0.715) and lignin phenol concentrations (r = 0.447) in our samples suggests that the majority of soil-marker BHPs is indeed sourced from the continent. These results agree well with the dataset of Waterson and Canuel (2008), which shows clustering of bacterial branched fatty acids with terrestrial C29 sterols and long-chain fatty acids. Rsoil indices also anticorrelate (r = −0.412) with Δ14C values (Wakeham et al., 2009), suggesting that the majority of soil-marker BHPs is exported as part of a stabilized pre-aged sediment fraction that experiences further aging during cross-shelf transport. However, Rsoil indices are low and do not show a pronounced decreasing trend along the transect irrespective of density fraction (Figure 6) as could be expected due to increasing contribution of marine OC with distance offshore. This trend likely results from a high relative contribution of riverine and/or marine BHT at all locations, subduing the soil-marker BHP contribution and consequently lowering Rsoil index values. Accordingly, Rsoil does not seem to be a good proxy to quantitatively trace terrestrial input into the GOM. However, the Rsoil-derived spatial pattern of terrestrial OC input differs from the spatial pattern evident from absolute soil-marker BHP abundances. Rsoil shows similar values for station Miss R and GOM 50 and slightly lower values at station GOM 540, whereas absolute soil-marker BHP abundances are highest at stations Miss R and GOM 540 and significantly lower at stationGOM 50. As stated above, with a few exceptions most BHPs show this same pattern (Figure 5), including C-2 methylated BHPs such as 2Me-BHT, which could be sourced, among others, from plant-associated bacteria (Ricci et al., 2014; Silipo et al., 2014; Kulkarni et al., 2015). The low BHP abundance at station GOM 50 results from low BHP contribution to total OC in the <1.6 and 1.6–2.0 g cm–3 density fractions (Figure 4). This likely results from dilution with other compounds such as sterols and pigments that are particularly enriched at station GOM 50 in comparison to stations Miss R and GOM 540 (Sampere et al., 2008; Waterson and Canuel, 2008).
Although TOC-normalized BHP concentrations are very high in the 2.0–2.5 and >2.5 g cm–3 fractions (Figure 4B), their low %TOC (Wakeham et al., 2009) results in low mass-normalized BHP abundances (Figure 4A) at station GOM 50. Accordingly, we attribute the low BHP abundances at station GOM 50 to dilution with another molecular OC pool rather than a significant inversion of relative BHP sources (allochthonous vs. autochthonous), in agreement with the spatial Rsoil index pattern. As stated above, along the transect individual BHT-CE, BHT glucosamine, 3Me-BHT, BHT, BHT II, and anhydroBHT concentrations are higher in the <1.6 g cm–3 and/or 1.6–2.0 g cm–3 fractions at station GOM 540 than at station Miss R (Figure 5). Particularly high abundances of these BHPs in the 1.6–2.0 g cm–3 fraction suggest that they may have an additional marine source (in the water column or sediment) accumulating in the Δ14C-enriched mesodensity fraction. In contrast, the exported terrestrial BHP component seems to be primarily associated with the <1.6 g cm–3 density fraction as expected due to hydrodynamic sorting. Whereas BHT-CE, BHT glucosamine, and BHT are rather ubiquitous and have been identified in a range of environments and bacterial cultures (Talbot et al., 2008, 2016; Pearson et al., 2009; Sáenz et al., 2011a, b; Eickhoff et al., 2014; Höfle et al., 2015; Spencer-Jones et al., 2015), other BHPs such as anhydroBHT, 3Me-BHT, and BHT II potentially carry additional information about source organisms and/or redox conditions or diagenesis (see below).
Aminopolyol Sources
Our samples show an unusually high diversity of aminopolyols. Aminopentol and aminotetrol have been primarily detected in proteobacterial Type I and Type II methanotrophs, respectively (Rohmer et al., 1984; Talbot et al., 2001; van Winden et al., 2012) and their presence in near-shore marine sediments has been attributed to the supply of continental wetland-derived OC off, for example, the Amazon and Congo rivers (Talbot et al., 2014; Wagner et al., 2014). Although aminopentol has thus far been regarded as the most taxonomically specific BHP methanotrophy proxy, Rush et al. (2016) recently challenged its proficiency as proxy for Type I aerobic methanotrophy except in the case of terrestrial wetland methanotrophy (Rush et al., 2016). We observe a general decrease of both aminopentol and aminotetrol abundances from station Miss R to station GOM 540 (Figure 8). Both aminotetrol and aminopentol have been identified in cultures of several sulfate reducing bacteria belonging to the genus Desulfovibrio and this source seems to be discernible by characteristically low ratios of aminopentol: aminotriol (∼1:1350) and aminotetrol: aminotriol (1:20–1:100) (Rush et al., 2016 and references therein). Accordingly, high aminopentol: aminotriol ratios (1:3–1:133) and aminotetrol: aminotriol ratios (1:5–1:45) suggest overall negligible contributions from Desulfovibrio except in the 2.0–2.5 g cm–3 (aminotetrol:aminotriol 1:21) and >2.5 g cm–3 density fraction (aminotetrol:aminotriol 1:45) at station GOM 540. In our investigation, aminopentol and aminotetrol abundances are highest in the <1.6 g cm–3 fraction at station Miss R and correlate strongly with soil-marker BHPs (r = 0.889) suggesting that aminopentol and aminotetrol primarily derivefrom continental sources, possibly the anaerobic sediments found in coastal swamps in the Mississippi Delta (DeLaune et al., 1986). This result is consistent with the observations of Bianchi et al. (2011), who demonstrated that coastal swamps/marshes are a significant and previously under-estimated contributor to the sedimentary OC on the Louisiana shelf. Using a three-component endmember model based on sedimentary δ13C values, lignin concentrations, and C/N ratios, these authors calculated that coastal marshes supply up to ∼34% of the OC deposited during springtime. Similarly, Goñi et al. (1997) showed that C3 plant-derived lignin from coastal forests and swamps primarily accumulates in sediments off the Mississippi river mouth, while C4 plant-derived lignin from grassland soils of the Mississippi catchment is transported much further offshore. Interestingly, aminopentol: aminotriol and aminotetrol: aminotriol ratios decrease with distance offshore, which may indicate the increasing presence of Desulfovibrio. Alternatively, the lower ratios could also result from aminotriol production by some other marine source since aminotriol also shows a relative enrichment in the 1.6–2.0 g cm–3 fraction (in comparison to station Miss R) that is likely associated with in situ production.
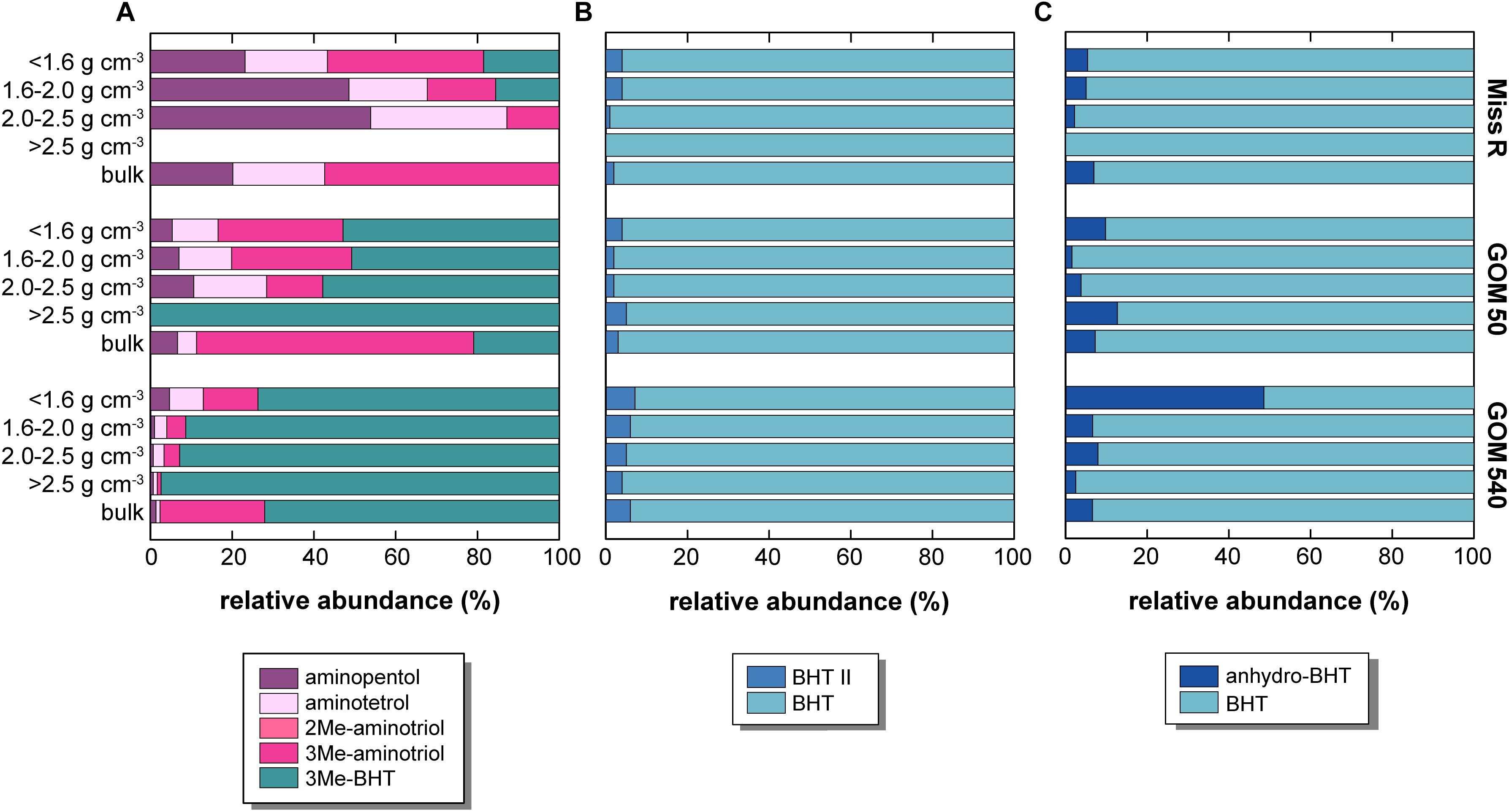
Figure 8. Relative abundance of selected BHP sub-pools in density fractions and bulk sediment samples along the Miss R – GOM transect. (A) Relative abundance of putative methanotrophic BHPs, (B) relative abundance of BHT II and BHT (BHT II ratio), and (C) relative abundances of anhydro-BHT and BHT.
Our samples contain additional BHPs that may have a methanotrophic source. C-3 methylation of amino-BHPs as well as BHT (Neunlist and Rohmer, 1985; Zundel and Rohmer, 1985; Rush et al., 2016) has been detected in various Type I methanotrophic bacteria, although 3Me-BHT has also been identified in acetic acid bacteria (Zundel and Rohmer, 1985). The hopanoid C-3 methylase (hpnR) is phylogenetically diverse among bacteria (Welander and Summons, 2012). More recently, 3Me-aminotriol was identified in haloalkaliphilic (Type I) Methylomicrobium alcaliphilum (Banta et al., 2015; Rush et al., 2016) and was also detected in a Sphagnum peat (Talbot et al., 2016). In the latter, 3Me-aminotriol abundances mirrored the depth profile of aminopentol, providing circumstantial evidence that 3Me-aminotriol could be produced by other Type I methanotrophs. In our samples, aminopentol and aminotetrol absolute abundances (Figure 5) correlate well with 3Me-aminotriol (r = 0.981) but do not correlate with 3Me-BHT abundances (r = 0.164). The contribution of 3Me-BHT increases with distance offshore, particularly in the 1.6–2.0 g cm–3 fraction (Figure 8), suggesting 3Me-BHT may also have a marine origin in the GOM which may or may not include methanotrophic bacteria. Methylomirabilis oxyfera (Kool et al., 2014) is currently the only known non-thermophilic source organism for BHhexol (the only other confirmed source being Alicyclobacillus acidoterrestris; Řezanka et al., 2011), but its origin is less well constrained. BHhexol was also identified in a marine sponge (likely representing bacterial symbionts; Shatz et al., 2000), however, De Jonge et al. (2016) suggested a terrestrial origin due to its near-shore presence in the Kara Sea as well as other shelf systems and soils. Analogous to 3Me-aminotriol, absolute BHhexol abundances correlate well (r = 0.858) with aminopentol and aminotetrol, indicating that BHhexol seems to be associated with the terrestrial BHP pool, but a methanotrophic source cannot be elucidated based on our data.
In addition to the isomers of aminotriol [which seems to correspond to the isomer identified at a nearby station FCC-1 (29.16°N, 89.20°; 27 m water depth) by Kusch et al., 2018] and methylated aminotriol, we detected three N-acylaminotriols. N-acylaminotriols have so far been detected in purple non-sulfur bacteria as well as Nitrosomonas europaea (Seemann et al., 1999; Talbot et al., 2007). N. europaea is a chemolithoautotrophic aerobic ammonium oxidizing bacterium (AOB) that is widespread in nitrogen-rich terrestrial ecosystems. N-acylaminotriol abundances correlate well with soil-marker BHPs (r = 0.991) and aminopentol and aminotetrol (r = 0.934) abundances suggesting a primarily terrestrial source. Nonetheless, these BHPs have so far not been reported from soils. N. europaea has also been shown to oxidize a range of hydrocarbons (Keener and Arp, 1994). Thus, high N supply in the Mississippi River plume (Pakulski et al., 2000), natural seepage of hydrocarbons in the northern GOM, and anthropogenic inputs from the Mississippi River itself (MacDonald et al., 2015) could provide conditions under which N. europaea may thrive in the water column or sediments. To our knowledge, N. europaea has not been directly detected off the Mississippi River. However, it is normally abundant in coastal environments and under high ammonia concentrations, and AOB related to N. europaea were identified in NW GOM sediments off the Texas coast in conjunction with aerobic ammonium oxidizing archaea (Flood et al., 2014). Archaeal ammonia monooxygenase (amoA) genes, typically much more abundant than bacterial amoA genes (Wuchter et al., 2006), are also frequently identified in high abundance in the water column in or near the mouth of the Mississippi River and are associated with low salinity as well as seasonal hypoxia (Gillies et al., 2015; Mason et al., 2016).
Diagenetic and Redox Influence on BHP Distributions
Since the 1950s, the inner shelf areas of the northern GOM experience recurring summer bottom water hypoxia. Hypoxia is driven by the high freshwater input and nutrient loads from the Mississippi and Atchafalaya Rivers causing strong thermohaline stratification, eutrophication, and intense microbial respiration and oxygen consumption (Dagg et al., 2007; Bianchi et al., 2010; Rabalais et al., 2010). This hypoxia influences the microbial community structures (Gillies et al., 2015; Gillies Campbell et al., 2019) and BHP assemblages have been shown to vary with redox conditions elsewhere (Wakeham et al., 2007; Sáenz et al., 2011b; Kharbush et al., 2013; Ricci et al., 2014; Rush et al., 2014; Höfle et al., 2015; Matys et al., 2017). BHT II accumulates under suboxic and anoxic conditions (Sáenz et al., 2011b; Matys et al., 2017) and, more recently, it has been used as a proxy for anammox bacteria when present in high abundance relative to BHT [BHT II ratios; BHT II/(BHT + BHT II)] (Rush et al., 2014, 2019; Matys et al., 2017). Support for an anammox origin of BHT II comes from its significantly δ13C-depleted values (–50.6%) in a Mediterranean Sea sediment (Hemingway et al., 2018). Hypoxia usually extends along the continental shelf to the west of our study area (e.g., in 2002)1 although it is generally less well documented directly off the Mississippi River mouth. At times, bottom waters in the northern GOM can become anoxic (Rabalais et al., 2010); thus, anammox may occur in bottom waters, at the sediment-water interface, or in sediments. Thus far, however, conclusive evidence for anammox in the area of our study is missing. McCarthy et al. (2015) used 15N isotope labeling and nitrite reductase (nirS) gene microarrays (from K. stuttgartiensis) in sediments from the northern GOM including our study area. While isotope labeling did not permit differentiating 29N2 production from denitrification or anammox, the authors concluded that the detection of nirS from K. stuttgartiensis confirmed the presence of anammox and estimated that it may be a significant N sink (potentially as high as 29%) in the northern GOM. However, “Candidatus Scalindua” spp. is the typical marine anammox clade (Oshiki et al., 2016) rather than the freshwater K. stuttgartiensis, and C. Scalindua has been shown to produce significant amounts of BHT II (Rush et al., 2014). Accordingly, BHT II ratios may aid at identifying whether anammox is present at our stations and whether BHT II is linked to specific density fractions. The latter would support observations that larger aggregates may hold oxygen-depleted micro-niches, which could harbor anammox bacteria under otherwise inhibitive external O2 concentrations (Woebken et al., 2007). BHT II ratios in our samples are consistently low, ranging from 0 to 0.07 in the Miss R, GOM 50, and GOM 540 samples irrespective of density fraction (Figure 8), which is consistent with surface sediment data from the nearby station FCC-1 (Kusch et al., 2018). We observe a slight increase of BHT II ratio offshore, however, ratios are still well below those observed in a “Candidatus Scalindua profunda” enrichment culture or in upwelling-induced oxygen minimum zones (>0.5) (Rush et al., 2014; Matys et al., 2017). We also do not observe association of BHT II with a specific density fraction at any site as the observed difference of BHT II ratios between density fractions is well within analytical reproducibility (±0.02 SD; repeated analyses of an in-house standard sample). Either anammox abundances are too low to result in significant BHT II concentrations in our samples or, more likely, O2 concentrations during seasonal hypoxia still exceed the tolerance levels of anammox (Kalvelage et al., 2011; Bristow et al., 2016). Bianchi et al. (2010) highlighted that stratification and hypoxia show strong spatial and temporal variability not only on a seasonal basis but even on a daily basis. Thus, depending on the timing of O2 measurements during a monitoring campaign, waters may be classified hypoxic, but this “snapshot” hypoxia may not persist locally and consequently such locations would in general not support anoxic metabolisms such as anammox.
If the water column and surface sediments at our stations are primarily oxic for most of the year, BHPs may likely be affected by in situ degradation during cross-shelf transport and after deposition in sediments. Lignin phenols in our samples show an increase of vanillyl acid to aldehyde ratios with density fraction, indicating increased oxidation of those phenols associated with the high density, primarily mineral-rich fractions (Sampere et al., 2008; Wakeham et al., 2009). AnhydroBHT is interpreted to represent a degradation product of BHPs (Handley et al., 2010; Eickhoff et al., 2014). Its high abundance in the <1.6 g cm–3 density fraction at station GOM 540 suggests enhanced diagenetic alteration of BHPs in this sample, which agrees well with its strongly Δ14C-depleted values (Figure 7) assuming OC degradation primarily depends on oxygen exposure time (Hartnett et al., 1998). Indeed, anhydroBHT has a significantly lower abundance in the <1.6 g cm–3 fraction at station Miss R (more than one order of magnitude; Figure 5). BHT-normalized anhydroBHT abundances [anhydroBHT/(BHT + anhydroBHT)] increase in the <1.6 g cm–3 fractions along the transect (Figure 8), from 0.05 (Miss R) to 0.10 (GOM 50) and 0.49 (GOM 540), supporting a diagenetic origin of anhydroBHT during cross-shelf transport. Interestingly, unsat. anhydroBHT (Figure 5) shows a very different pattern both spatially and among density fractions; it correlates very well with soil-marker BHPs (r = 0.785) and aminopentol and aminotetrol (r = 0.867) abundances indicating that unsat. anhydroBHT derives from the continent. If unsat. anhydroBHT is a diagenetic product of unsaturated BHPs, unsat. BHT pentose and unsat. C16-acylaminotriol represent possible precursor compounds. Strong correlation of unsat. anhydroBHT with unsat. BHT pentose (r = 0.856) and unsat. C16-acylaminotriol (r = 0.883) may support this argument, although it could also simply mirror provenance rather than source. Overall, the provenance of BHPs seems to have a much stronger imprint on BHP inventories in our samples than water column and sediment redox conditions.
Summary and Conclusion
Surface sediments along a transect spanning from the Mississippi Delta into the GOM were fractionated into four density fractions and BHPs were analyzed to investigate their origin. For comparison, BHPs were also analyzed in bulk samples. Our results show that BHP abundances are highest in the <1.6 g cm–3 fraction and correlate well with high OC/SA ratios, C/N ratios, and lignin phenol abundances as well as low Δ14C values in this fraction. This combined evidence suggests that the majority of BHPs in these sediments have a terrestrial origin and are subjected to hydrodynamic sorting during cross-shelf transport. This trend is not quantitatively reflected by the Rsoil proxy, which does not seem to be a good proxy for terrestrial input in this setting. A small sub-set of BHPs including BHT-CE, BHT glucosamine, 3Me-BHT, BHT, BHT II, and anhydroBHT seem to have an additional source offshore and are abundant in both the <1.6 and 1.6–2.0 g cm–3 fractions. These BHPs likely derive from autochthonous in situ production in the water column and/or degradation of BHPs during transport across the shelf. Moreover, we observe an unusually high diversity of amino-functionalized BHPs that are likely synthesized by aerobic methane oxidizing bacteria and exported from the Mississippi Delta swamps as well as by aerobic ammonia oxidizing bacteria in the Mississippi River plume. In contrast, anaerobic ammonium oxidizing bacteria do not seem to be present during recurring seasonal hypoxia on the shelf.
Our data helps deconvolute the origin of BHPs in a highly dynamic shelf system exposed to a large gradient of organic matter sources. Our results also highlight the need for additional culture-based constraints on the diversity of BHPs produced by different bacteria under different environmental conditions. This is particularly obvious for BHPs such as N-acylaminotriols, for which known source organisms cannot be matched to published metagenomic data off the Mississippi River. In addition, the marked difference between soil-marker BHP relative abundances in bulk samples and density fractions warrants further investigation to determine whether this is an analytical artifact or a “real” signal and, in case of the latter, which mechanisms explain this observation.
Data Availability Statement
All datasets generated for this study are included in the manuscript/Supplementary Files.
Author Contributions
SK, JS, and SW designed the study. SK and SW performed the analyses and all authors contributed to the data interpretation. SK wrote the manuscript with input from all co-authors.
Funding
SK was supported by the University of Cologne via a “Network Exchange – NetEx” grant. JS thanks the University of Colorado Boulder for funding. SW acknowledges support by NSF grants OCE-0223226 and DEB-0454741. The Gulf of Mexico project was a collaboration with Thomas S. Bianchi and Elizabeth A. Canuel whose participation was supported by NSF grants OCE-0223334 (TSB) and OCE-0223295 (EAC). The authors would like to thank the Hanse-Wissenschaftskolleg Delmenhorst, Germany, for sponsoring the “Marine Organic Biogeochemistry” workshop in April 2019. The workshop was funded by the Deutsche Forschungsgemeinschaft (DFG, German Research Foundation) – project number: 422798570.
Conflict of Interest
The authors declare that the research was conducted in the absence of any commercial or financial relationships that could be construed as a potential conflict of interest.
Acknowledgments
The authors thank Nadia Dildar for laboratory assistance in the Organic Geochemistry Laboratory at the University of Colorado Boulder. The authors also thank the captain and crew of R/V Pelican for aid in sample collection, and colleagues Troy Sampere, Elizabeth Lerberg (née Waterson), Brent McKee, Meade Allison, and Reide Corbett for their participation in this program. This is a contribution to the Frontiers in Marine Science Marine Biogeochemistry Research Topic emanating the “Marine Organic Biogeochemistry” workshop.
Supplementary Material
The Supplementary Material for this article can be found online at: https://www.frontiersin.org/articles/10.3389/fmars.2019.00729/full#supplementary-material
Footnotes
References
Arnarson, T. S., and Keil, R. G. (2001). Organic-mineral interactions in marine sediments studied using density fractionation and X-ray photoelectron spectroscopy. Org. Geochem. 32, 1401–1415. doi: 10.1016/s0146-6380(01)00114-0
Baldock, J. A., and Skjemstad, J. O. (2000). Role of the soil matrix and minerals in protecting natural organic materials against biological attack. Org. Geochem. 31, 697–710. doi: 10.1016/s0146-6380(00)00049-8
Banta, A. B., Wei, J. H., and Welander, P. V. (2015). A distinct pathway for tetrahymanol synthesis in bacteria. Proc. Natl. Acad. Sci. U.S.A. 112, 13478–13483. doi: 10.1073/pnas.1511482112
Bianchi, T. S., DiMarco, S. F., Cowan, J. H. Jr., Hetland, R. D., Chapman, P., Day, J. W., et al. (2010). The science of hypoxia in the Northern Gulf of Mexico: a review. Sci Total Environ. 408, 1471–1484. doi: 10.1016/j.scitotenv.2009.11.047
Bianchi, T. S., Wysocki, L. A., Schreiner, K. M., Filley, T. R., Corbett, D. R., and Kolker, A. S. (2011). Sources of terrestrial organic carbon in the Mississippi Plume region: evidence for the importance of coastal marsh inputs. Aquat. Geochem. 17, 431–456. doi: 10.1007/s10498-010-9110-3
Bradley, A. S., Pearson, A., Sáenz, J. P., and Marx, C. J. (2010). Adenosylhopane: the first intermediate in hopanoid side chain biosynthesis. Org. Geochem. 41, 1075–1081. doi: 10.1016/j.orggeochem.2010.07.003
Bristow, L. A., Dalsgaard, T., Tiano, L., Mills, D. B., Bertagnolli, A. D., Wright, J. J., et al. (2016). Ammonium and nitrite oxidation at nanomolar oxygen concentrations in oxygen minimum zone waters. Proc. Natl. Acad. Sci. U.S.A. 113, 10601–10606. doi: 10.1073/pnas.1600359113
Cooke, M. P., Talbot, H. M., and Farrimond, P. (2008). Bacterial populations recorded in bacteriohopanepolyol distributions in soils from Northern England. Org. Geochem. 39, 1347–1358. doi: 10.1016/j.orggeochem.2008.05.003
Dagg, M. J., Ammerman, J. W., Amon, R. M. W., Gardner, W. S., Green, R. E., and Lohrenz, S. E. (2007). A review of water column processes influencing hypoxia in the northern Gulf of Mexico. Estuaries Coast. 30, 735–752. doi: 10.1007/bf02841331
De Jonge, C., Talbot, H. M., Bischoff, J., Stadnitskaia, A., Cherkashov, G., and Sinninghe Damsté, J. S. (2016). Bacteriohopanepolyol distribution in Yenisei River and Kara Sea suspended particulate matter and sediments traces terrigenous organic matter input. Geochim. Cosmochim. Acta 174, 85–101. doi: 10.1016/j.gca.2015.11.008
DeLaune, R. D., Smith, C. J., and Patrick, W. H. (1986). Methane production in Mississippi river deltaic plain peat. Org. Geochem. 9, 193–197. doi: 10.1016/0146-6380(86)90069-0
Doğrul Selver, A., Sparkes, R. B., Bischoff, J., Talbot, H. M., Gustafsson, Ö., Semiletov, I. P., et al. (2015). Distributions of bacterial and archaeal membrane lipids in surface sediments reflect differences in input and loss of terrestrial organic carbon along a cross-shelf Arctic transect. Org. Geochem. 83-84, 16–26. doi: 10.1016/j.orggeochem.2015.01.005
Doughty, D. M., Coleman, M. L., Hunter, R. C., Sessions, A. L., Summons, R. E., and Newman, D. K. (2011). The RND-family transporter, HpnN, is required for hopanoid localization to the outer membrane of Rhodopseudomonas palustris TIE-1. Proc. Natl. Acad. Sci. U.S.A. 108, E1045–E1051. doi: 10.1073/pnas.1104209108
Doughty, D. M., Dieterle, M., Sessions, A. L., Fischer, W. W., and Newman, D. K. (2014). Probing the subcellular localization of hopanoid lipids in bacteria using NanoSIMS. PLoS One 9:e84455. doi: 10.1371/journal.pone.0084455
Eickhoff, M., Birgel, D., Talbot, H. M., Peckmann, J., and Kappler, A. (2014). Diagenetic degradation products of bacteriohopanepolyols produced by Rhodopseudomonas palustris strain TIE-1. Org. Geochem. 68, 31–38. doi: 10.1016/j.orggeochem.2014.01.002
Flood, M., Frabutt, D., Floyd, D., Powers, A., Ezegwe, U., Devol, A., et al. (2014). Ammonia-oxidizing bacteria and archaea in sediments of the Gulf of Mexico. Environ. Technol. 36, 124–135. doi: 10.1080/09593330.2014.942385
Gillies, L. E., Thrash, J. C., deRada, S., Rabalais, N. N., and Mason, O. U. (2015). Archaeal enrichment in the hypoxic zone in the northern Gulf of Mexico. Environ. Microbiol. 17, 3847–3856. doi: 10.1111/1462-2920.12853
Gillies Campbell, L., Thrash, J. C., Rabalais, N. N., and Mason, O. U. (2019). Extent of the annual Gulf of Mexico hypoxic zone influences microbial community structure. PLoS One 14:e209055. doi: 10.1371/journal.pone.0209055
Goñi, M. A., Ruttenberg, K. C., and Eglinton, T. I. (1997). Source and contribution of terrigenous organic carbon to surface sediments in the Gulf of Mexico. Nature 389, 275–278. doi: 10.1038/38477
Handley, L., Talbot, H. M., Cooke, M. P., Anderson, K. E., and Wagner, T. (2010). Bacteriohopanepolyols as tracers for continental and marine organic matter supply and phases of enhanced nitrogen cycling on the late Quaternary Congo deep sea fan. Org. Geochem. 41, 910–914. doi: 10.1016/j.orggeochem.2010.04.016
Hartnett, H. E., Keil, R. G., Hedges, J. I., and Devol, A. H. (1998). Influence of oxygen exposure time on organic carbon preservation in continental margin sediments. Nature 391, 572–574.
Hedges, J. I., Keil, R. G., and Benner, R. (1997). What happens to terrestrial organic matter in the ocean? Org. Geochem. 27, 195–212. doi: 10.1016/s0146-6380(97)00066-1
Hemingway, J. D., Kusch, S., Walter, S. R. S., Polik, C. A., Elling, F. J., and Pearson, A. (2018). A novel method to measure the 13C composition of intact bacteriohopanepolyols. Org. Geochem. 123, 144–147. doi: 10.1016/j.orggeochem.2018.07.002
Höfle, S. T., Kusch, S., Talbot, H. M., Mollenhauer, G., Zubrzycki, S., Burghardt, S., et al. (2015). Characterisation of bacterial populations in Arctic permafrost soils using bacteriohopanepolyols. Org. Geochem. 88, 1–16. doi: 10.1016/j.orggeochem.2015.08.002
Kalvelage, T., Jensen, M. M., Contreras, S., Revsbech, N. P., Lam, P., Günter, M., et al. (2011). Oxygen sensitivity of anammox and coupled N-Cycle processes in oxygen minimum zones. PLoS One 6:e29299. doi: 10.1371/journal.pone.0029299
Keener, W. K., and Arp, D. J. (1994). Transformations of aromatic compounds by Nitrosomonas europaea. Appl. Environ. Microbiol. 60, 1914–1920.
Kharbush, J. J., Ugalde, J. A., Hogle, S. L., Allen, E. E., and Aluwihare, L. I. (2013). Composite bacterial hopanoids and their microbial producers across oxygen gradients in the water column of the California Current. Appl. Environ. Microbiol. 79, 7491–7501. doi: 10.1128/AEM.02367-13
Kool, D. M., Talbot, H. M., Rush, D., Ettwig, K., and Sinninghe Damsté, J. S. (2014). Rare bacteriohopanepolyols as markers for an autotrophic, intra-aerobic methanotroph. Geochim. Cosmochim. Acta 136, 114–125. doi: 10.1016/j.gca.2014.04.002
Kulkarni, G., Busset, N., Molinaro, A., Gargani, D., Chaintreuil, C., Silipo, A., et al. (2015). Specific hopanoid classes differentially affect free-living and symbiotic states of bradyrhizobium diazoefficiens. mBio 6:e1251-15. doi: 10.1128/mBio.01251-15
Kusch, S., Walter, S. R. S., Hemingway, J. D., and Pearson, A. (2018). Improved chromatography reveals multiple new bacteriohopanepolyol isomers in marine sediments. Org. Geochem. 124, 12–21. doi: 10.1016/j.orggeochem.2018.07.010
MacDonald, I. R., Garcia-Pineda, O., Beet, A., Daneshgar Asl, S., Feng, L., Graettinger, G., et al. (2015). Natural and unnatural oil slicks in the Gulf of Mexico. J. Geophys. Res. 120, 8364–8380.
Mason, O. U., Canter, E. J., Gillies, L. E., Paisie, T. K., and Roberts, B. J. (2016). Mississippi river plume enriches microbial diversity in the Northern Gulf of Mexico. Front. Microbiol. 7:1048. doi: 10.3389/fmicb.2016.01048
Matys, E. D., Sepúlveda, J., Pantoja, S., Lange, C. B., Caniupán, M., Lamy, F., et al. (2017). Bacteriohopanepolyols along redox gradients in the humboldt current system off northern Chile. Geobiology 15, 844–857. doi: 10.1111/gbi.12250
McCarthy, M. J., Newell, S. E., Carini, S. A., and Gardner, W. S. (2015). Denitrification dominates sediment nitrogen removal and is enhanced by bottom-water hypoxia in the Northern Gulf of Mexico. Estuaries Coast. 38, 2279–2294. doi: 10.1007/s12237-015-9964-0
Neunlist, S., and Rohmer, M. (1985). Novel hopanoids from the methylotrophic bacteria Methylococcus capsulatus and Methylomonas methanica - (22S)-35-aminobacteriohopane-30,31,32,33,34-pentol and (22S)-35-amino-3-beta-methylbacteriohopane-30,31,32,33,34-pentol. Biochem. J. 231, 635–639. doi: 10.1042/bj2310635
Oshiki, M., Satoh, H., and Okabe, S. (2016). Ecology and physiology of anaerobic ammonium oxidizing bacteria. Environ. Microbiol. 18, 2784–2796. doi: 10.1111/1462-2920.13134
Pakulski, J. D., Benner, R., Whitledge, T., Amon, R., Eadie, B., Cifuentes, L., et al. (2000). Microbial metabolism and nutrient cycling in the mississippi and atchafalaya river plumes. Estuarine Coast. Shelf Sci. 50, 173–184. doi: 10.1006/ecss.1999.0561
Pearson, A., Flood Page, S. R., Jorgenson, T. L., Fischer, W. W., and Higgins, M. B. (2007). Novel hopanoid cyclases from the environment. Environ. Microbiol. 9, 2175–2188. doi: 10.1111/j.1462-2920.2007.01331.x
Pearson, A., Leavitt, W. D., Sáenz, J. P., Summons, R. E., Tam, M. C. M., and Close, H. G. (2009). Diversity of hopanoids and squalene-hopene cyclases across a tropical land-sea gradient. Environ. Microbiol. 11, 1208–1223. doi: 10.1111/j.1462-2920.2008.01817.x
Pearson, A., and Rusch, D. B. (2009). Distribution of microbial terpenoid lipid cyclases in the global ocean metagenome. ISME J. 3, 352–363. doi: 10.1038/ismej.2008.116
Rabalais, N. N., Diaz, R. J., Levin, L. A., Turner, R. E., Gilbert, D., and Zhang, J. (2010). Dynamics and distribution of natural and human-caused hypoxia. Biogeosciences 7, 585–619. doi: 10.5194/bg-7-585-2010
Řezanka, T., Siristova, L., Melzoch, K., and Sigler, K. (2011). N-acylated bacteriohopanehexol-mannosamides from the thermophilic bacterium Alicyclobacillus acidoterrestris. Lipids 46, 249–261. doi: 10.1007/s11745-010-3482-4
Ricci, J. N., Coleman, M. L., Welander, P. V., Sessions, A. L., Summons, R. E., Spear, J. R., et al. (2014). Diverse capacity for 2-methylhopanoid production correlates with a specific ecological niche. ISME J. 8, 675–684. doi: 10.1038/ismej.2013.191
Rohmer, M., Bouvier-Nave, P., and Ourisson, G. (1984). Distribution of hopanoid triterpenes in prokaryotes. Microbiology 130, 1137–1150. doi: 10.1099/00221287-130-5-1137
Rush, D., Osborne, K. A., Birgel, D., Kappler, A., Hirayama, H., Peckmann, J., et al. (2016). The bacteriohopanepolyol inventory of novel aerobic methane oxidising bacteria reveals new biomarker signatures of aerobic methanotrophy in marine systems. PLoS One 11:e165635. doi: 10.1371/journal.pone.0165635
Rush, D., Sinninghe Damsté, J. S., Poulton, S. W., Thamdrup, B., Garside, A. L., González, J. A., et al. (2014). Anaerobic ammonium-oxidising bacteria: a biological source of the bacteriohopanetetrol stereoisomer in marine sediments. Geochim. Cosmochim. Acta 140, 50–64. doi: 10.1016/j.gca.2014.05.014
Rush, D., Talbot, H. M., van der Meer, M., Hopmans, E. C., Douglas, B., and Sinninghe Damsté, J. S. (2019). Biomarker evidence for the occurrence of anaerobic ammonium oxidation in the eastern mediterranean sea during quaternary and pliocene sapropel formation. Biogeosciences 16, 2467–2479. doi: 10.5194/bg-16-2467-2019
Sáenz, J. P. (2010). Hopanoid enrichment in a detergent resistant membrane fraction of Crocosphaera watsonii: implications for bacterial lipid raft formation. Org. Geochem. 41, 853–856. doi: 10.1016/j.orggeochem.2010.05.005
Sáenz, J. P., Eglinton, T. I., and Summons, R. E. (2011a). Abundance and structural diversity of bacteriohopanepolyols in suspended particulate matter along a river to ocean transect. Org. Geochem. 42, 774–780. doi: 10.1016/j.orggeochem.2011.05.006
Sáenz, J. P., Wakeham, S. G., Eglinton, T. I., and Summons, R. E. (2011b). New constraints on the provenance of hopanoids in the marine geologic record: bacteriohopanepolyols in marine suboxic and anoxic environments. Org. Geochem. 42, 1351–1362. doi: 10.1016/j.orggeochem.2011.08.016
Sáenz, J. P., Sezgin, E., Schwille, P., and Simons, K. (2012). Functional convergence of hopanoids and sterols in membrane ordering. Proc. Natl. Acad. Sci. U.S.A. 109, 14236–14240. doi: 10.1073/pnas.1212141109
Sampere, T. P., Bianchi, T. S., Wakeham, S. G., and Allison, M. A. (2008). Sources of organic matter in surface sediments of the louisiana continental margin: effects of major depositional/transport pathways and Hurricane Ivan. Cont. Shelf Res. 28, 2472–2487. doi: 10.1016/j.csr.2008.06.009
Schmerk, C. L., Bernards, M. A., and Valvano, M. A. (2011). Hopanoid production is required for Low-pH tolerance, antimicrobial resistance, and motility in Burkholderia cenocepacia. J. Bacteriol. 193, 6712–6723. doi: 10.1128/JB.05979-11
Seemann, M., Bisseret, P., Tritz, J.-P., Hooper, A. B., and Rohmer, M. (1999). Novel bacterial triterpenoids of the hopane series from Nitrosomonas europaea and their significance for the formation of the C35 bacteriohopane skeleton. Tetrahedron Lett. 40, 1681–1684. doi: 10.1016/s0040-4039(99)00064-7
Shatz, M., Yosief, T., and Kashman, Y. (2000). Bacteriohopanehexol, a new triterpene from the marine sponge Petrosia species. J. Nat. Prod. 63, 1554–1556. doi: 10.1021/np000190r
Silipo, A., Vitiello, G., Gully, D., Sturiale, L., Chaintreuil, C. E. M., Fardoux, J., et al. (2014). Covalently linked hopanoid-lipid A improves outer-membrane resistance of a Bradyrhizobium symbiont of legumes. Nat. Comms. 5, 1–11. doi: 10.1038/ncomms6106
Spencer-Jones, C. L., Wagner, T., Dinga, B. J., Schefuß, E., Mann, P. J., Poulsen, J. R., et al. (2015). Bacteriohopanepolyols in tropical soils and sediments from the Congo River catchment area. Org. Geochem. 89-90, 1–13. doi: 10.1016/j.orggeochem.2015.09.003
Talbot, H. M., Handley, L., Spencer-Jones, C. L., Dinga, B. J., Schefuß, E., Mann, P. J., et al. (2014). Variability in aerobic methane oxidation over the past 1.2 Myrs recorded in microbial biomarker signatures from Congo fan sediments. Geochim. Cosmochim. Acta 133, 387–401. doi: 10.1016/j.gca.2014.02.035
Talbot, H. M., McClymont, E. L., Inglis, G. N., Evershed, R. P., and Pancost, R. D. (2016). Origin and preservation of bacteriohopanepolyol signatures in Sphagnum peat from Bissendorfer Moor (Germany). Org. Geochem. 97, 95–110. doi: 10.1016/j.orggeochem.2016.04.011
Talbot, H. M., Rohmer, M., and Farrimond, P. (2007). Rapid structural elucidation of composite bacterial hopanoids by atmospheric pressure chemical ionisation liquid chromatography/ion trap mass spectrometry. Rapid Commun. Mass Spectrom. 21, 880–892. doi: 10.1002/rcm.2911
Talbot, H. M., Summons, R. E., Jahnke, L. L., Cockell, C. S., Rohmer, M., and Farrimond, P. (2008). Cyanobacterial bacteriohopanepolyol signatures from cultures and natural environmental settings. Org. Geochem. 39, 232–263. doi: 10.1016/j.orggeochem.2007.08.006
Talbot, H. M., Watson, D. F., Murrell, J. C., Carter, J. F., and Farrimond, P. (2001). Analysis of intact bacteriohopanepolyols from methanotrophic bacteria by reversed-phase high-performance liquid chromatography-atmospheric pressure chemical ionisation mass spectrometry. J. Chromatogr. A 921, 175–185. doi: 10.1016/s0021-9673(01)00871-8
van Winden, J. F., Talbot, H. M., Kip, N., Reichart, G.-J., Pol, A., McNamara, N. P., et al. (2012). Bacteriohopanepolyol signatures as markers for methanotrophic bacteria in peat moss. Geochim. Cosmochim. Acta 77, 52–61. doi: 10.1016/j.gca.2011.10.026
Wagner, T., Kallweit, W., Talbot, H. M., Mollenhauer, G., Boom, A., and Zabel, M. (2014). Microbial biomarkers support organic carbon transport from methane-rich Amazon wetlands to the shelf and deep sea fan during recent and glacial climate conditions. Org. Geochem. 67, 85–98. doi: 10.1016/j.orggeochem.2013.12.003
Wakeham, S. G., Amann, R., Freeman, K. H., Hopmans, E. C., Jørgensen, B. B., Putnam, I. F., et al. (2007). Microbial ecology of the stratified water column of the Black Sea as revealed by a comprehensive biomarker study. Org. Geochem. 38, 2070–2097. doi: 10.1016/j.orggeochem.2007.08.003
Wakeham, S. G., and Canuel, E. A. (2016). The nature of organic carbon in density-fractionated sediments in the Sacramento-San Joaquin River Delta (California). Biogeosciences 13, 567–582. doi: 10.5194/bg-13-567-2016
Wakeham, S. G., Canuel, E. A., Lerberg, E. J., Mason, P., Sampere, T. P., and Bianchi, T. S. (2009). Partitioning of organic matter in continental margin sediments among density fractions. Mar. Chem. 115, 211–225. doi: 10.1016/j.marchem.2009.08.005
Waterson, E. J., and Canuel, E. A. (2008). Sources of sedimentary organic matter in the Mississippi River and adjacent Gulf of Mexico as revealed by lipid biomarker and δ13CTOC analyses. Org. Geochem. 39, 422–439. doi: 10.1016/j.orggeochem.2008.01.011
Welander, P. V., Hunter, R. C., Zhang, L., Sessions, A. L., Summons, R. E., and Newman, D. K. (2009). Hopanoids play a role in membrane integrity and pH homeostasis in Rhodopseudomonas palustris TIE-1. J. Bacteriol. 191, 6145–6156. doi: 10.1128/JB.00460-09
Welander, P. V., and Summons, R. E. (2012). Discovery, taxonomic distribution, and phenotypic characterization of a gene required for 3-methylhopanoid production. Proc. Natl. Acad. Sci. U.S.A. 109, 12905–12910. doi: 10.1073/pnas.1208255109
Woebken, D., Fuchs, B. M., Kuypers, M. M. M., and Amann, R. (2007). Potential interactions of particle-associated anammox bacteria with bacterial and archaeal partners in the namibian upwelling system. Appl. Environ. Microbiol. 73, 4648–4657. doi: 10.1128/aem.02774-06
Wuchter, C., Abbas, B., Coolen, M. J. L., Herfort, L., van Bleijswijk, J., Timmers, P., et al. (2006). Archaeal nitrification in the ocean. Proc. Natl. Acad. Sci. U.S.A. 103, 12317–12322. doi: 10.1073/pnas.0600756103
Zhu, C., Talbot, H. M., Wagner, T., Pan, J.-M., and Pancost, R. D. (2011). Distribution of hopanoids along a land to sea transect: implications for microbial ecology and the use of hopanoids in environmental studies. Limnol. Oceangr. 56, 1850–1865. doi: 10.4319/lo.2011.56.5.1850
Keywords: bacteriohopanepolyols, BHPs, density fraction, Gulf of Mexico, Mississippi River, methanotrophy
Citation: Kusch S, Sepúlveda J and Wakeham SG (2019) Origin of Sedimentary BHPs Along a Mississippi River–Gulf of Mexico Export Transect: Insights From Spatial and Density Distributions. Front. Mar. Sci. 6:729. doi: 10.3389/fmars.2019.00729
Received: 30 September 2019; Accepted: 08 November 2019;
Published: 27 November 2019.
Edited by:
Selvaraj Kandasamy, Xiamen University, ChinaReviewed by:
Yuan-Pin Chang, National Sun Yat-sen University, TaiwanThomas S. Bianchi, University of Florida, United States
Copyright © 2019 Kusch, Sepúlveda and Wakeham. This is an open-access article distributed under the terms of the Creative Commons Attribution License (CC BY). The use, distribution or reproduction in other forums is permitted, provided the original author(s) and the copyright owner(s) are credited and that the original publication in this journal is cited, in accordance with accepted academic practice. No use, distribution or reproduction is permitted which does not comply with these terms.
*Correspondence: Stephanie Kusch, c3RlcGhhbmllLmt1c2NoQHVuaS1rb2Vsbi5kZQ==