- 1Smithsonian Marine Station, Fort Pierce, FL, United States
- 2Department of Biomedical Sciences, Carlson College of Veterinary Medicine, Oregon State University, Corvallis, OR, United States
- 3School of Ocean Sciences, Bangor University, Bangor, United Kingdom
- 4Department of Soil and Water Sciences, University of Florida, Gainesville, FL, United States
An outbreak of stony coral tissue loss disease (SCTLD), emerged on reefs off the coast of southeast Florida in 2014 and continues to spread throughout Florida’s Reef Tract. SCTLD is causing extensive mortality of multiple coral species and disease signs vary among affected coral species with differences in rates of tissue loss (acute and subacute), lesion morphology (adjacent bleached zone or not) and lesion occurrence (focal and multi-focal). We examined the virulence, transmission dynamics and response to antibiotic treatment of coral species exhibiting different types of tissue loss lesions from two regions in Florida. Montastraea cavernosa with subacute tissue loss lesions in the southeast Florida region near Fort Lauderdale was compared to corals (multiple species) with acute tissue loss lesions in the Middle Keys. Corals from both regions showed progressive tissue loss but the in situ rate of mortality was significantly higher in tagged colonies in the Keys. Aquaria studies showed disease transmission occurred through direct contact and through the water column for corals from both regions. However, transmission success was higher for corals with acute vs. subacute lesions. There was 100% transmission for both test species, M. cavernosa and Meandrina meandrites, touching acute lesions. Among the three species touching subacute lesions, the disease transmitted readily to Orbicella faveolata (100%) followed by M. cavernosa (30%) with no transmission occurring with Porites astreoides. Diseased fragments of all species tested responded to antibiotic treatment with a cessation or slowing of the disease lesions suggesting that bacteria are involved in disease progression. Mortality was higher for in situ corals with acute lesions and transmission was higher in M. cavernosa exposed to acute lesions compared to subacute lesions, suggesting that different microbes may be involved with the two lesion types. However, since in situ mortality of M. cavernosa was not measured in the Middle Keys, we cannot completely rule out that a common pathogen is involved but is less virulent within M. cavernosa.
Introduction
Outbreaks of coral disease, especially tissue loss diseases (white syndromes), have damaged coral reefs worldwide, with the first outbreaks reported from the Caribbean in the 1970s (Dustan, 1977). Since that time, diseases have become a serious threat to corals with increases in numbers of diseases, coral species affected, and geographic extent (Sutherland et al., 2004; Ward and Lafferty, 2004; Sokolow et al., 2009). Ocean warming and changes in weather patterns associated with global climate change exacerbate the problem through impacts on the coral hosts and pathogens with potential increases in disease prevalence and frequency of disease outbreaks (Harvell et al., 2007; Maynard et al., 2015). Florida’s reefs have been especially hard hit by diseases, which have contributed to significant declines in coral cover (Aronson and Precht, 1997; Richardson et al., 1998a; Patterson et al., 2002; Williams and Miller, 2005; Brandt et al., 2012). The tissue loss diseases are the most virulent (highest host mortality), and Florida’s reefs have had multiple outbreaks. Some disease outbreaks were caused by apparently non-host specific pathogens that affected multiple coral species (white plagues) (Dustan, 1977; Richardson et al., 1998a; Brandt et al., 2012), whereas others (white pox, white band) appeared to exclusively impact acroporids (Aronson and Precht, 2001; Patterson et al., 2002; Williams and Miller, 2005). Pathogenic bacteria were found to underlie some of these outbreaks, e.g., white plague (Richardson et al., 1998b; Denner et al., 2003) and white pox (Patterson et al., 2002).
The Florida Reef Tract is a predominantly continental reef system extending approximately 577 km along south Florida and into the Florida Keys. It is the 3rd largest barrier reef ecosystem in the world but has been heavily impacted by humans with densely populated coastlines, high visitor numbers, polluted terrestrial run-off and overfishing, among other problems (Jackson et al., 2014). Florida’s coral reefs have declined significantly over the past several decades due to anthropogenic impacts and numerous disease outbreaks (Gardner et al., 2003; Jackson et al., 2014). Currently, there is another outbreak of tissue loss disease occurring on the reefs of Florida affecting over 20 coral species (Precht et al., 2016; Walton et al., 2018). It is a tissue loss disease of unknown etiology, affecting only reef corals (e.g., not soft corals), and so the name stony coral tissue loss disease (SCTLD) was decided upon by group consensus1, and so should be considered similar to “white syndrome” commonly used to describe tissue loss diseases of unknown etiology in corals in the Indo-Pacific (Bourne et al., 2014). Fortunately, a case definition has been developed for SCTLD2, which will be useful in discriminating this disease outbreak from other tissue loss diseases. Outbreak levels of the disease were first observed near Virginia Key, Florida in 2014 and have since spread north and south along the Florida Reef Tract. On affected reefs, some impacted species have been reduced to <3% of their initial population densities (Precht et al., 2016), with regional declines reported in coral densities and live tissue as a result of SCTLD (Walton et al., 2018). The emergence of infectious disease outbreaks often occurs following a change in host-parasite ecology such as introduction of novel pathogens in naïve host populations, emergence of newly evolved pathogens or changes in the environment fostering increased pathogen virulence or rates of transmission (Daszak et al., 2000, 2001). The first reports of high levels of SCTLD occurred in 2014 coincident with summer bleaching events across the Florida Reef Tract in 2014 and 2015 (Manzello, 2015; Walton et al., 2018) and dredging operations between 2013 and 2015 in the channel at Port of Miami, FL, that resulted in massive sedimentation near the initial site of the outbreak (Miller et al., 2016). It is likely that these two concurrent stresses, bleaching, and sedimentation, both of which can degrade coral health contributed to the emergence of this disease. Most outbreaks of tissue loss diseases on coral reefs are localized in spatial extent and ephemeral in nature (e.g., Williams and Miller, 2005; Brandt et al., 2012; Aeby et al., 2016). Unfortunately, the current Florida outbreak is continuing to spread and diseased colonies have now appeared as far south as Key West in the Florida Keys3. This is producing an unprecedented amount of coral mortality on these already degraded coral reefs.
The current Florida disease outbreak is unique, in that the disease signs vary within and among affected coral species with differences in rates of tissue loss (acute and subacute), lesion morphology (adjacent bleached zone or not) and lesion occurrence (focal and multi-focal). Regional differences in lesion morphology were also evident with corals in some regions predominantly presenting with subacute tissue loss lesions, frequently with bleached borders, whereas corals in other regions had acute tissue loss lesions without bleached borders. Differences in disease signs could reflect different diseases, differences in host response or different stages of the same disease. However, little was known about the ecology or pathogenesis of SCTLD. We examined the disease ecology of coral species exhibiting different types of tissue loss lesions from two regions in Florida: corals with subacute lesions in the southeast Florida region near Fort Lauderdale and corals with acute lesions in the Middle Florida Keys. We arbitrarily defined subacute tissue loss as lesions with bare white skeleton less than 5 cm along the lesion edge and acute tissue loss as lesions with greater than 5 cm bare white skeleton along the lesion edge (Figure 1). We also use degree of algae colonization of the bare skeleton and signs of sediment accumulation or skeletal erosion to discriminate between different rates of tissue loss. The objectives of this study were to examine, for each lesion type, (1) in situ disease virulence (degree of colony mortality) and prevalence of tagged colonies through time; (2) transmissibility and differences in susceptibility among species; and (3) whether bacteria were involved in disease progression.
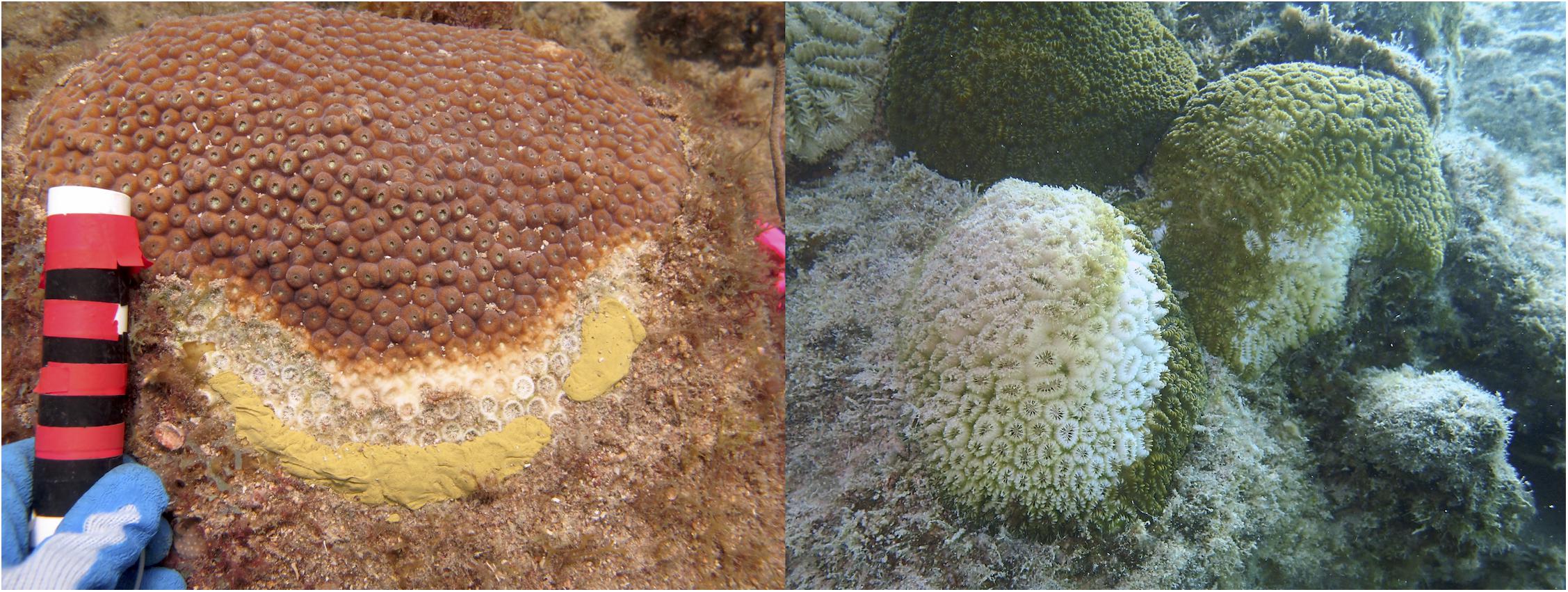
Figure 1. Representative pictures showing Montastraea cavernosa with a subacute tissue loss lesion on the left and Dichocoenia stokesii with acute tissue loss lesions on the right.
Materials and Methods
Disease Virulence and Prevalence of Tagged Colonies Through Time
To determine rates of in situ tissue loss, we tagged and tracked individual colonies. One set of tagged colonies focused on corals in the Fort Lauderdale region, specifically Montastraea cavernosa displaying subacute tissue loss lesions with bleached zones. The other set of tagged corals was in the Middle Keys near Long Key where the coral species Meandrina jacksoni, Pseudodiploria strigosa, Diploria labyrinthiformis, and Dichocoenia stokesii displayed acute tissue loss lesions. Unfortunately, no M. cavernosa occurred on this patch reef for a more direct comparison with the tagged corals in the Fort Lauderdale region.
For the first set, 20 M. cavernosa colonies with subacute tissue loss were mapped, tagged and photographed at a site off Fort Lauderdale (N26° 08.9151′′W80° 05.7549′′) in July 2017. No M. cavernosa colonies with acute tissue loss were noted at the Fort Lauderdale site at the start of our study. The site was approximately 8 m in depth with low coral cover (visual estimate < 5%). To help visualize tissue loss, bands of marine epoxy (Z-Spar A-788 Splash Zone Epoxy) were placed on the dead part of the colonies, parallel and approximately 5 cm away from lesion edges. Colonies were resurveyed and photographed on approximately monthly intervals until July 2018. The three-dimensional structure of the coral colonies and the presence of widespread multi-focal lesions complicated the use of digital image analysis for calculating the rates of tissue loss. Instead, a semi-quantitative estimate of tissue loss was used whereby the proportion of each colony that was healthy, dead, or with recent tissue loss was recorded. Similar methods have been used successfully to estimate coral tissue loss in other coral disease studies (Aeby et al., 2010, 2016; Walton et al., 2018). Visual estimates of colony health (proportion of colony dead, diseased, or healthy) were assessed from photo review. We determined the rate of tissue loss by visually estimating the proportion of overall loss for each colony by month. Degree of colony mortality (proportion of tissue loss) and disease prevalence [(# of tagged colonies with lesions/total # live tagged colonies) × 100] were followed through time, and case fatality rate [(# colonies with complete tissue loss/total # colonies) × 100] was calculated for the study period.
The amount of mortality from tissue loss diseases can vary among coral colonies and could be affected by a number of variables such as colony size, stage of the disease and/or disease duration. To determine which factors might best predict disease outcome, we examined the relationship between colony mortality after 1 year and (1) colony size, specifically the initial area of healthy tissue on the colony, (2) proportion of the colony with active disease lesions when first observed, and (3) duration of the disease through time, defined as the proportion of months with active disease signs until 100% mortality or the end of the current study period. The relationship between total colony mortality and each of these variables was examined using a non-parametric Spearman–Rank correlation test. Then the proportion of variability in colony mortality explained by a combination of these three variables was investigated using a permutational distance-based linear model (DISTLM) (McArdle and Anderson, 2001). DISTLM carries out a partitioning of variation in a data set described by a resemblance matrix according to a regression (or multiple regression) model. Predictor variables can be categorical or continuous and the technique makes no prior assumptions about the nature of the response variable distribution, meaning that normality does not have to be satisfied. The model was based on 9999 random permutations of the raw data and a Euclidean distance matrix. The predictors were first investigated for collinearity by calculating their pairwise Pearson’s correlation values (r ≤ 0.6 in all cases), and therefore all three predictors were included in the model fitting exercise. During the model fitting process, all possible candidate models (i.e., all possible unique combinations of the predictors) were fitted. Importantly, when more than one predictor was included, its ability to capture variation in the response variable was conditional on the performance of other predictors included in the model. The final optimal model (balancing performance against model complexity) was identified using Akaike’s Information Criterion (Akaike, 1973) with a second-order bias correction applied (AICc) to help account for the relatively low sample sizes (Hurvich and Tsai, 1989; Burnham and Anderson, 2004). This was chosen as the method to identify the most parsimonious model as it adds a ‘penalty’ for increases in the number of predictor variables. We report all model summaries to highlight the change in AICc values with changing model complexity.
The second set of corals was tagged in December 2017 at the Long Key Bridge Pilings off Long Key in the Middle Keys (N24° 44.002′′W80° 49.742′′) where the disease had just emerged as evidenced by the presence of numerous coral colonies having lesions of bare white skeleton lacking algal colonization. The site was approximately 8 m in depth with higher coral cover than the Fort Lauderdale site (visual estimate > 50% cover). Colony density was high enough that we were able to tag both healthy and diseased colonies within our dive time limits. At this site, all diseased colonies (n = 13) were located, tagged and photographed, and an additional 8 apparently healthy colonies were tagged and photographed as time allowed. Five coral species with acute tissue loss lesions were tagged and included Pseudodiploria strigosa (n = 5), Diploria labyrinthiformis (n = 5), Dichocoenia stokesii (n = 2), and Meandrina jacksoni (n = 1). Tagged healthy colonies included Pseudodiploria strigosa (n = 1), Diploria labyrinthiformis (n = 6), and Porites astreoides (n = 1). The site was revisited in January 2018 and again in July 2018. As described above, visual estimates on colony health (percent of colony dead, diseased, or healthy) were recorded in situ and from photo review. From these data, we measured disease virulence, prevalence and incidence [(# new tagged infected colonies/initial # tagged healthy colonies) × 100], through time and case fatality rate.
For the tagged colonies at the Middle Keys site, calculating a monthly loss was not useful as the rate of tissue loss was too fast (high mortality within a month). Therefore, we estimated daily rate of tissue loss only for the first month of the study. Tagged colonies had a circular or elliptical colony shape, so the measured length and width of each colony was used to calculate the initial colony size including live and dead portions of the colony (cm2). We calculated the initial area of live tissue by multiplying the total colony area by the estimated proportion of healthy tissue at the start of the study. In the same manner, we estimated rates of tissue loss by calculating the change in proportion of healthy tissue and transcribing that into cm2 loss.
Disease Virulence of Tagged Colonies in Fort Lauderdale vs. Middle Keys
Lesion morphology differed in corals between regions, so to examine whether lesion differences might be indicative of different stages of the same disease vs. different diseases altogether, we compared the daily rate of tissue loss between tagged colonies from the Fort Lauderdale site to the Middle Keys site. Unfortunately, there were no coral species overlapping within these two regions preventing a direct comparison of disease virulence. However, we felt it would still be informative to document differences in rates of tissue loss between species and regions. For the Fort Lauderdale colonies, we calculated the daily rate of tissue loss for the month with the highest average rate of colony loss during the year (November to December 2017). For the Middle Keys site, we used the first month of the study (December 2017 to January 2018). Data were not normally distributed and sample sizes were unequal between regions, so a non-parametric Wilcoxon two-sample test was used to examine differences in rates of tissue loss (cm2 day–1) among regions.
Health of the Zooxanthellae-Coral Symbiosis in M. cavernosa
Diseased M. cavernosa in the Fort Lauderdale region had a distinct bleached zone along the lesion edge suggesting the coral-zooxanthellae symbiosis was compromised before tissue loss. Pulse Amplitude Modulated Fluorometry (Model: Walz Diving-PAM; LED emission maximum 650 nm) was used to measure the in situ photochemical efficiency of healthy and diseased M. cavernosa colonies. Effective quantum yield (ΔF/Fm′) was measured by administering repeated saturation pulses across the upper coral surface along a linear transect. Saturation pulses were preceded by 15 s of actinic illumination by the fiber optic probe. For healthy colonies, the linear transect was positioned haphazardly across healthy tissue. For diseased colonies, the linear transect was positioned such that the saturation pulses transitioned from the disease front (at cm 0), toward tissue healthy in appearance (cm 2–6) (see Figure 1). During the linear transect, the probe was moved at 1 cm intervals. The fiber optic tip (5.5 mm active diameter) was standardized to a distance of 10 mm from the coral surface, and initial fluorescence values (Fo) were between 300–500 units. Internal settings on the diving-PAM were as follows (measuring intensity = 6, saturation intensity = 8; saturation width = 0.8 s; actinic width = 0.15; actinic intensity = 4). All measurements were taken during the hours of 0730 – 1100, with ambient PAR values ranging from 60 to 450 μmol photons m–2 s–1. Order of the selected colonies (healthy vs. diseased) was haphazard with respect to time. Initial measurements were taken in July 2017 (diseased n = 8; healthy n = 6), and additional measurements on different colonies were taken again in October 2017 (diseased n = 8; healthy n = 8). Repeated measures analysis of variance (RM-ANOVA) was used to assess yield differences along probe transects. Separate RM-ANOVAs were run for healthy and diseased colonies (for a total of n = 16 diseased colonies and n = 14 healthy colonies), with probe location (cm) serving as the within-subjects factor, and measurement date (July or October) serving as the between-subjects factor. Post hoc analysis of the within-subject factor was conducted with a Sidak test (α < 0.05 was considered significant). Prior to analysis, all data were checked for normality using the Kolmogorov–Smirnov test. Assumptions of variance homogeneity were not met (tested with Mauchly’s sphericity test), so a Greenhouse–Geisser correction was applied to appropriately adjust the degrees of freedom (Grieve, 1984).
Transmissibility, Mode of Transmission and Interspecific Variability to Infection
Manipulative aquaria studies were set up to determine whether the tissue loss disease was transmissible, and, if so, whether there were interspecific differences in susceptibility to disease. Three sets of transmission experiments were conducted. The first experiment was conducted July 2017 using fragments of diseased (subacute tissue loss lesions) Montastraea cavernosa and apparently healthy M. cavernosa and Orbicella faveolata as the test corals. The second experiment was conducted Aug 2017 using fragments of diseased (subacute tissue loss lesions) M. cavernosa and apparently healthy M. cavernosa and Porites astreoides as the test corals. The third experiment was conducted June 2018 using fragments of diseased (acute tissue loss lesions) Colpophyllia natans and healthy M. cavernosa and Meandrina meandrites as the test corals. Experiments were conducted outdoors under natural sunlight with shade cloth covers that reduced ambient light by ∼50%. Individual 5 L aquaria were placed in larger temperature-controlled water tables with circulating freshwater adjusted with a cooling and heating system to maintain water temperatures at summer ambient levels (28–29°C). Aquaria were filled with seawater filtered through a 0.22 μm pore filter (FSW) and a bubbler placed to create water motion.
Experiments were conducted using a block design of four aquaria. Within each block, there were 2 aquaria (experimental and control) used for each test species and two test species were used in each trial for a total of 4 aquaria/block (Figure 2). In the experimental tanks, an infected fragment with a distinct tissue loss lesion was placed in direct contact with a healthy fragment (direct transmission) and another healthy fragment was placed ∼10 cm away (waterborne transmission). The same set up was used in control aquaria, except the diseased fragment was replaced with a healthy fragment of the same species to control for lesions created by coral to coral aggressive interactions. Partial water changes (∼2 L) were conducted daily to maintain water quality and coral fragments were photographed and examined for development of new tissue loss lesions. For all three trials, some interspecific aggression was noted between the corals in direct contact in control tanks. Therefore, to discriminate between lesions caused by aggression vs. a transmissible disease, any corals that developed lesions in the experimental or control tanks were removed from contact and observed for signs of lesion progression or recovery until the end of the experiment. Lesions that progressed following removal from contact were considered indicative of disease transmission. Lesions that failed to progress or healed were considered indicative of coral-to-coral aggression.
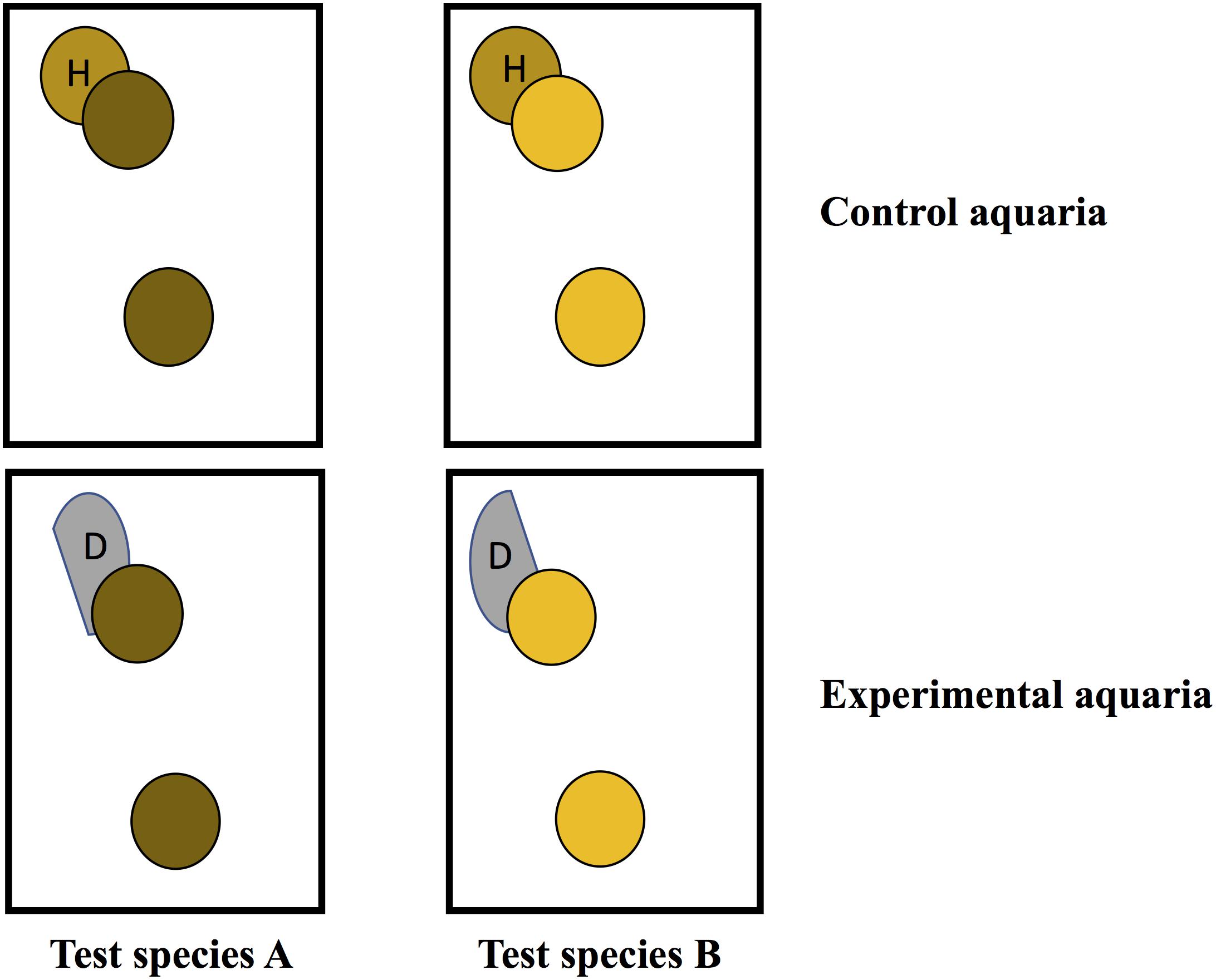
Figure 2. Schematic showing experimental design for transmission studies in aquaria. Circles with the same color indicate coral fragments were collected from the same colony.
For experiments, diseased coral fragments were collected from reefs off Fort Lauderdale (M. cavernosa) or the Florida Keys (Colpophyllia natans). Apparently healthy fragments for testing were obtained from the NOAA in situ coral nursery at Key West, Florida Keys (M. cavernosa, O. faveolata, P. astreoides) and the Florida Keys (Meandrina meandrites, Colpophyllia natans). However, to meet permit requirements and minimize damage to uninfected C. natans on the reef, visually “healthy” fragments of C. natans were collected from affected colonies but as far from the disease front as feasibly possible. Although not ideal, these were the only samples available and none of the fragments in the control aquaria developed lesions, without contact, confirming the non-disease state of the fragments. For corals collected in the field, each coral was photographed, and then the collected fragment was placed in an individual Ziploc bag at depth and transported to the laboratory in coolers. All test fragments were cut into four pieces with a rock saw within 1–2 days after collection. This allowed for the use of clonemates in the paired control and experimental aquaria controlling for intraspecific variability among test corals. Test fragments ranged from 8 to 16 cm2 in size depending on the species. Diseased fragments were cut in half and used for the comparative study between intra- and inter-specific rates of transmission, ensuring that each test species was exposed to a similar level of infectiousness from the diseased coral.
The first experiment comparing the rate of transmission in M. cavernosa to M. cavernosa (n = 10) vs. M. cavernosa to O. faveolata (n = 10) was run for 12 days. The second experiment comparing the rate of transmission in M. cavernosa to M. cavernosa (n = 8) vs. M. cavernosa to P. astreoides (n = 8) was run for 11 days. The third experiment comparing the rate of transmission in C. natans to M. cavernosa (n = 10) vs. C. natans to M. meandrites (n = 10) was run for 6 days. The disease lesions on C. natans in this last experiment progressed faster than on the M. cavernosa in the first two experiments, so the experiment was continued until test corals developed progressive tissue loss lesions or when the lesion on the diseased C. natans progressed until no live tissue remained.
A Mantel–Cox test was used to examine species differences in susceptibility to infection in the transmission trials. A Mantel–Cox test is a non-parametric test comparing the survival distributions of two groups and examines the outcome of the trial (transmission or no transmission) and the time to the outcome. In experiment 1, transmission to M. cavernosa was compared to O. faveolata, experiment 2 compared M. cavernosa to P. astreoides and experiment 3 compared M. cavernosa to M. meandrites. Touching and non-touching fragments were analyzed separately for each species pair.
Therapeutic Diagnosis With Antibiotic Treatment of Corals
Initial microscopy on diseased coral fragments (M. cavernosa) ruled out pathogenic fungi or ciliates at the disease lesions so the etiological agent was hypothesized to be a virus or bacterium. To discriminate between bacterial vs. viral infections, a diagnostic approach was taken. If tissue loss is significantly slowed or arrested by antibiotic treatment, then that would suggest bacteria are involved with disease progression. Fragments of diseased M. cavernosa with subacute tissue loss lesions (Fort Lauderdale) as well as C. natans and M. meandrites fragments with acute tissue loss lesions (Florida Keys), were collected from the field as described above. For each experimental pair, a coral fragment with a disease lesion was cut in half with a rock saw so that each fragment had a relatively equal area of the disease lesion and living tissue. One fragment from each pair was left untreated as a control, while the experimental fragments were treated with antibiotics dissolved in the aquarium water. Each fragment was individually housed in an aquarium with filtered (0.22 μm) seawater (FSW) and a bubbler for water motion. All aquaria were maintained in larger water tables with circulating freshwater adjusted with a cooling and heating system to maintain water temperatures between 28 and 29°C. Coral fragments were photographed and partial water changes conducted daily. For the experimental fragments, water was replaced with FSW pre-mixed with the corresponding concentration of antibiotics. The antibiotic selected for each species was based upon initial testing to find the antibiotic, or combination of antibiotics, which had the least observable negative effects on the corals and had the predicted widest range of activity. Healthy representatives of M. cavernosa and C. natans were first tested with amoxicillin, kanamycin, chloramphenicol, sulfathiazole, and nalidixic acid but extra M. meandrites fragments were not available for initial testing.
A combination of amoxicillin (50 μg/ml of tank water) and kanamycin (50 μg/ml of tank water) was chosen for M. cavernosa and M. meandrites fragments. A dual-antibiotic treatment would inhibit a wider range of any potential bacterial pathogens (Klastersky and Zinner, 1982; Hooton et al., 1984). We used a combination of a beta-lactam (amoxicillin) and aminoglycoside (kanamycin) antibiotic, which can also have a synergistic effect (Hewitt et al., 1966). C. natans did not tolerate the same combination or an amoxicillin-only treatment and displayed an obvious stress response within an hour after exposure to this antibiotic treatment (i.e., increased mucus sloughing and tank water discoloration from zooxanthellae expulsion). Therefore, the diseased C. natans were instead treated with the broad-spectrum antibiotic nalidixic acid (50 μg/ml of tank water).
A total of 21 sets of diseased fragments from M. cavernosa were tested; of which, 13 sets were run in July 2017 and experiments lasted 11 days while the remaining eight sets were run in November 2017 for 14 days. Only pairs of fragments whose controls displayed progressive tissue loss were used in the analysis (n = 13). The other 8 control fragments did not progress, so comparisons between controls and treatments could not be made. Tests with M. meandrites (n = 3) and C. natans (n = 8) were conducted in July 2018 and were run for 11 days. All controls had progressive tissue loss, so all pairs were used in the analysis. To examine the effect of antibiotic treatment on lesion progression a McNemar’s test for paired nominal data was used. Test fragments were scored as positive or negative for lesion progression.
Results
Disease Virulence and Prevalence of Tagged Colonies Through Time
Fort Lauderdale: M. cavernosa With Subacute Tissue Loss Lesions
Nineteen of the 20 tagged colonies had progressive tissue loss and the total amount of tissue lost by colonies during the 1-year study period averaged 34% (SE ± 8.7%). The average tissue loss per month for those 12 months was 2.83% per colony. However, change in live tissue varied among colonies and ranged from +1% (colony healed) to −100% (complete mortality) (Figure 3). Two colonies lost all remaining tissue by December 2017 followed by a third colony in February 2018 (case fatality rate = 15%).
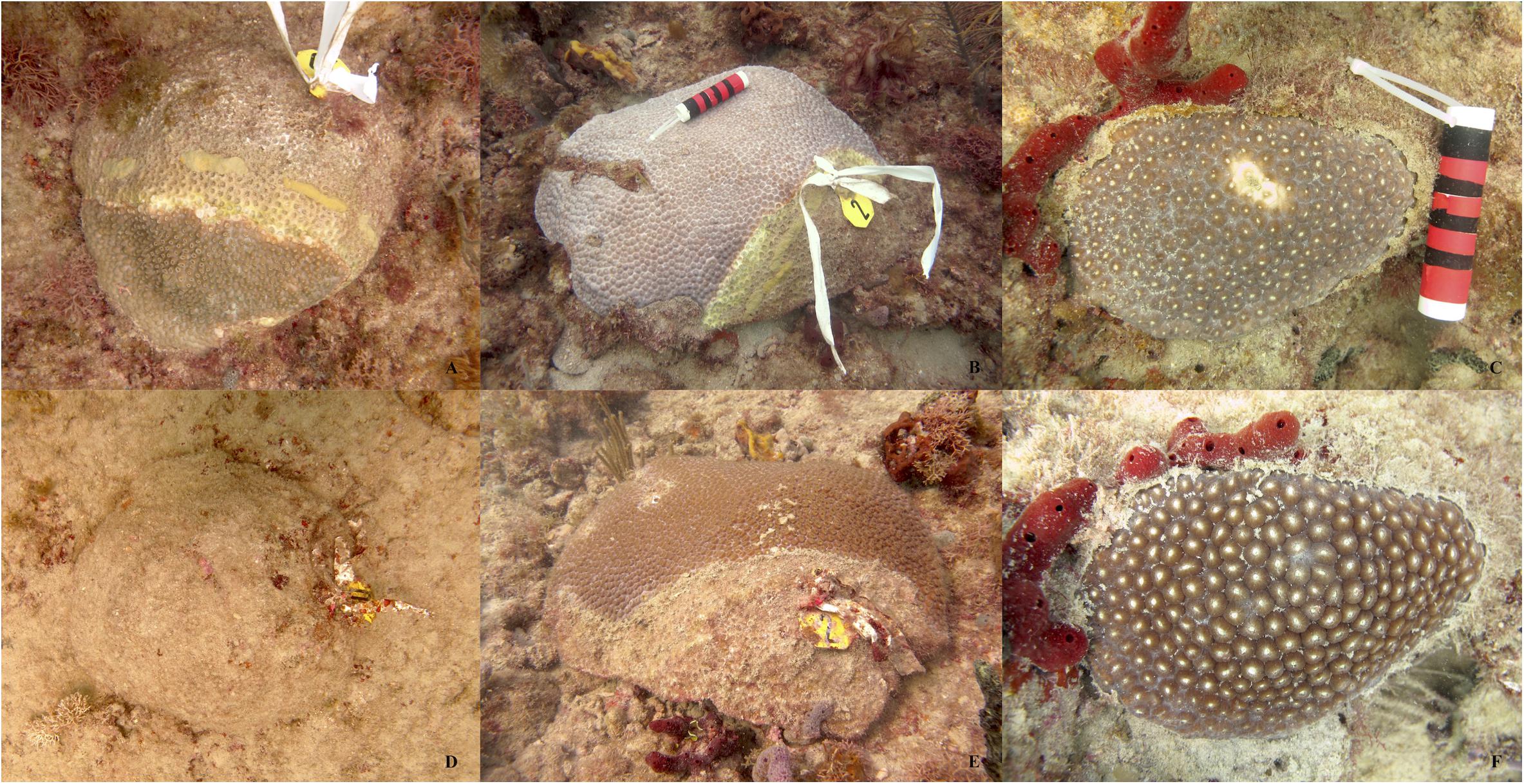
Figure 3. Representative pictures showing differences in degree of mortality among diseased colonies of Montastraea cavernosa. Top pictures (A–C) are from July 2017 and bottom pictures (D–F) are the same colonies in July 2018.
All 20 tagged colonies had active disease lesions at the start of the study in July 2017 (prevalence = 100%) and the disease remained active on 19 of the 20 colonies through November 2017. Disease prevalence started to decline in December and by March 2018 only 4 colonies had active lesions. Disease prevalence remained below 30% for the remainder of the study (Figure 4A). For disease progression, we found a similar temporal pattern with a higher rate of tissue loss on colonies through November 2017 and then a lower rate of loss for the remainder of the study (Figure 4B).
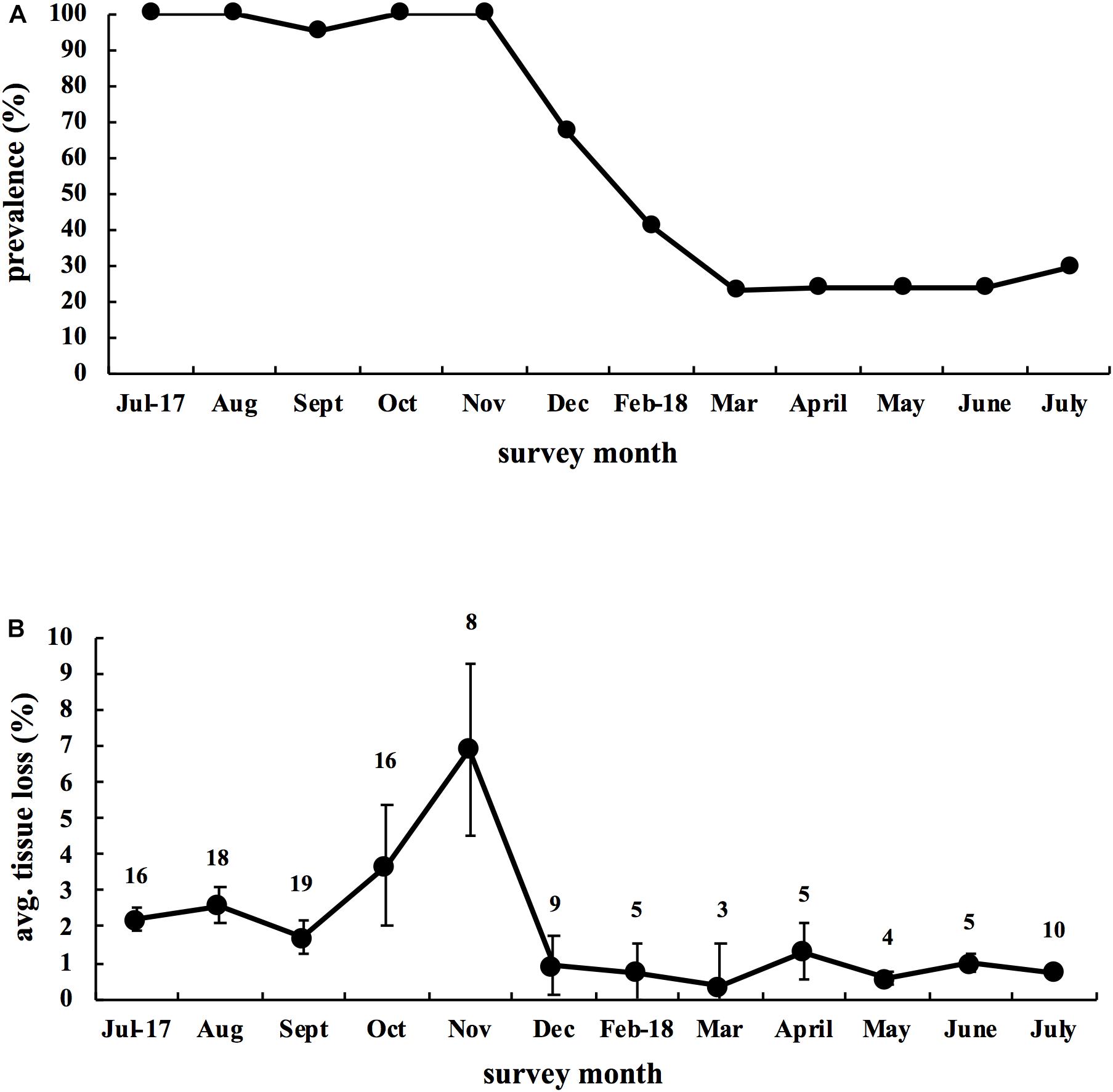
Figure 4. Disease dynamics of 20 infected Montastraea cavernosa colonies tagged in July 2017 and followed for 1 year. (A) Disease prevalence of M. cavernosa colonies through time. (B) Average monthly rate of tissue loss for M. cavernosa through time. Data reflect the mean ± SE. Calculation of monthly tissue loss was based upon colonies having active lesions the month prior. Number above each month indicates the number of active colonies used for each month’s calculation.
Mortality from disease differed among colonies and so we examined factors that might explain disease outcome. We found no significant relationship between starting colony size and mortality (Spearman-rank, ρ = −0.33, p = 0.16; Figure 5A), or between initial degree of affected tissue on colonies at the start of the study and mortality (Spearman-rank, ρ = −0.05, p = 0.83; Figure 5B), and a significant positive relationship between duration of the infection and mortality (Spearman-rank, ρ = 0.65, p = 0.002; Figure 5C). Of all the possible models containing unique combinations of these three predictor variables, the optimal model comprised the proportion of months with active lesions alone, with this model explaining 31.7% of the variability surrounding total amount of tissue lost by colonies (DISTLM, Pseudo-F = 8.38, p = 0.01; Table 1).
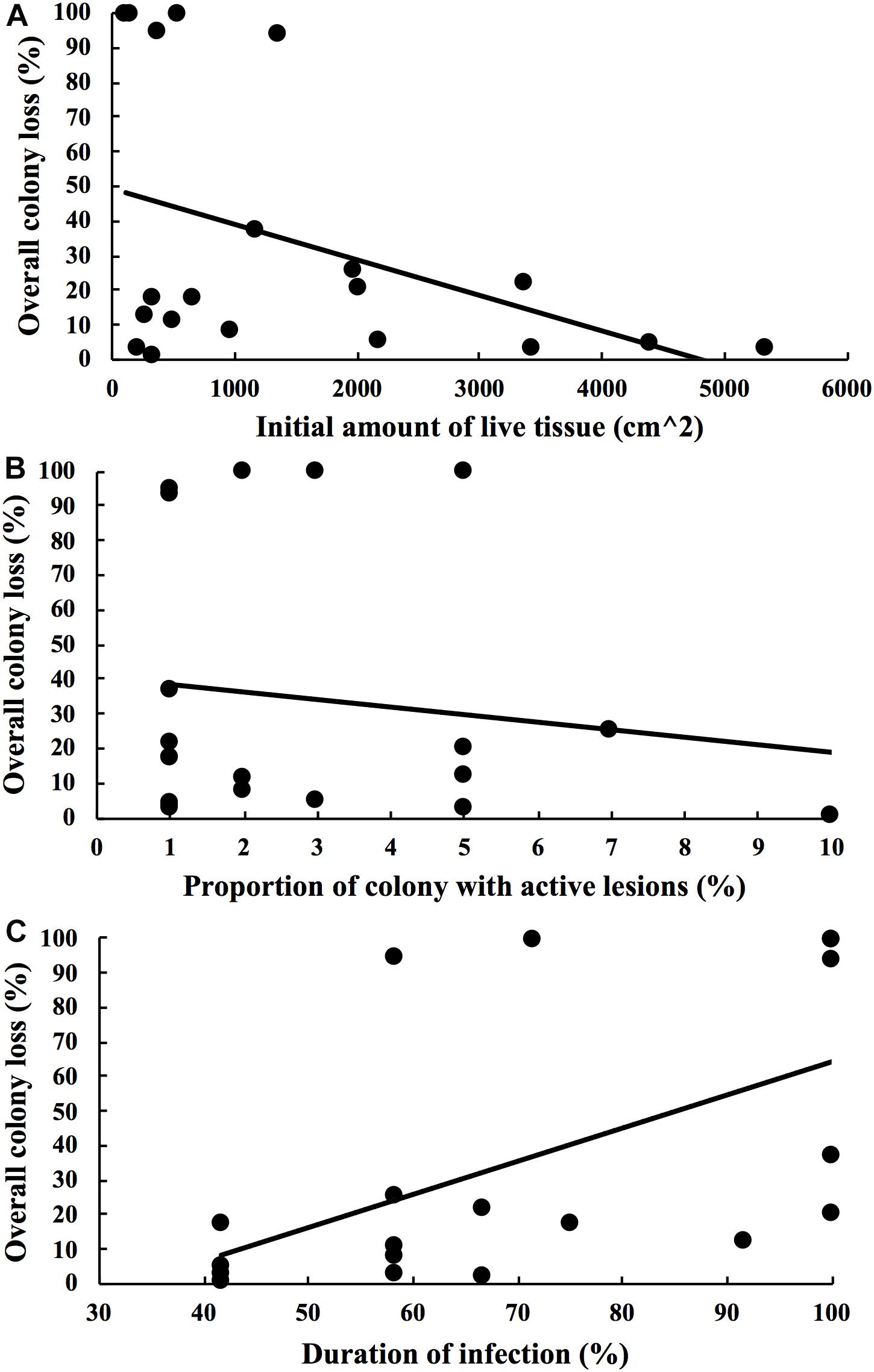
Figure 5. Scattergrams showing the relationship between the overall colony loss from disease after 1 year and (A) initial amount of health tissue on colonies at the start of the study, (B) proportion of colony with active lesions at the start of the study and (C) duration of the infection during the 1 year study period. 20 Montastrea cavernosa colonies with SCTLD were tagged and followed through time for 1 year.
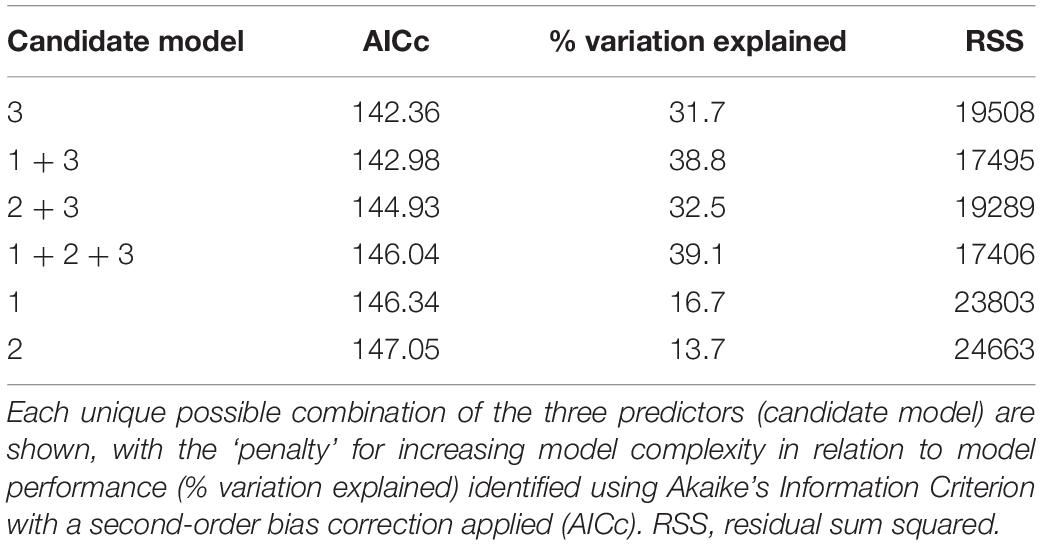
Table 1. Distance-based linear models relating colony size (predictor 1), initial stage of the disease (predictor 2), and duration of the disease through time (predictor 3) to the degree of colony mortality.
Middle Keys: Coral Species With Acute Tissue Loss Lesions
In December 2017, disease prevalence of tagged colonies (all species pooled) was 62% with 13 tagged diseased colonies and 8 tagged healthy colonies. In January 2018, we were able to find and photograph 11 of the 13 diseased colonies and all 8 healthy colonies (total = 19 of the 21 colonies). Eight of the 11 diseased colonies had complete mortality (73%), 3 of the prior healthy colonies had developed lesions (disease incidence = 15.8%) and overall disease prevalence was 54.5% (6 of 11 remaining live colonies). In July 2018, we found 21 colonies, of which, there was 100% mortality in 19 of the colonies (case fatality rate = 90.5%). Two colonies remained healthy, one P. astreoides and one D. labyrinthiformis, giving a final incidence rate on tagged colonies of 75% (6 of 8 healthy colonies developed lesions).
Disease Virulence of Tagged Colonies in Fort Lauderdale vs. Middle Keys
To examine how fast the disease was progressing on Middle Keys corals as compared to Fort Lauderdale corals, we calculated the daily tissue loss for the Middle Keys corals for the first month of the study, between December 2017 and January 2018 (all species pooled). For the Fort Lauderdale coral colonies, we chose the month with the highest average percent colony loss (November–December 2017) and calculated the average daily loss of tissue for that month. Middle Keys corals had a significantly faster rate of tissue loss (mean ± SE) of 4.2 ± 0.8 cm2 day–1 (n = 11) compared to Fort Lauderdale corals (0.82 ± 0.28 cm2 day–1, n = 20) (Wilcoxon two-sample test, Z = 3.63, df = 1, p < 0.0003). For corals within the Middle Keys, we also compared the rate of tissue loss for the two species with adequate sample sizes: D. labyrinthiformis (n = 5) and P. strigosa (n = 5). D. labyrinthiformis colonies had tissue loss rates of 3.3 ± 1.6 cm2 day–1, while P. strigosa had rates of 5.3 ± 0.73 cm2 day–1 (Wilcoxon two-sample test, Z = 1.04, df = 1, P = 0.3).
Health of the Zooxanthellae-Coral Symbiosis in M. cavernosa
Effective quantum yields (mean ± SE) displayed minor variation across probe transects within healthy colonies (RM-ANOVA, n = 14, p = 0.39), and overall averaged 0.42 ± 0.01 and 0.48 ± 0.01 in July (n = 6) and October (n = 8), respectively (Figure 6). While yields were slightly higher in October (RM-ANOVA, p < 0.01), there was no significant interaction between measurement date and probe distance (RM-ANOVA, p = 0.09). In contrast, for diseased colonies, yields across probe transects varied widely, ranging from 0.09–0.47 in July (n = 8), and 0.03 – 0.67 in October (n = 8). While yields were again higher in October for the diseased colonies (RM-ANOVA, p = 0.03), there was no significant interaction between measurement date and probe location (RM-ANOVA, p = 0.54). When combined across measurement dates, yields in diseased colonies were 0.22 ± 0.03 (at the disease front), 0.40 ± 0.02 (at 1 cm from lesion), and 0.46 ± 0.02 (at 2 cm from lesion). For all measurement dates, yields were significantly lower at the lesion front relative to healthy tissues beyond the bleached lesion (Figure 7, RM-ANOVA, n = 16, p < 0.001). Furthermore, there was no significant difference in yields between tissues adjacent (1 cm) and distant (2 cm) from the disease front (Sidak post hoc test, p = 0.20). Thus, tissue directly adjacent to the disease front displayed similar symbiont health relative to tissue further removed from the front.
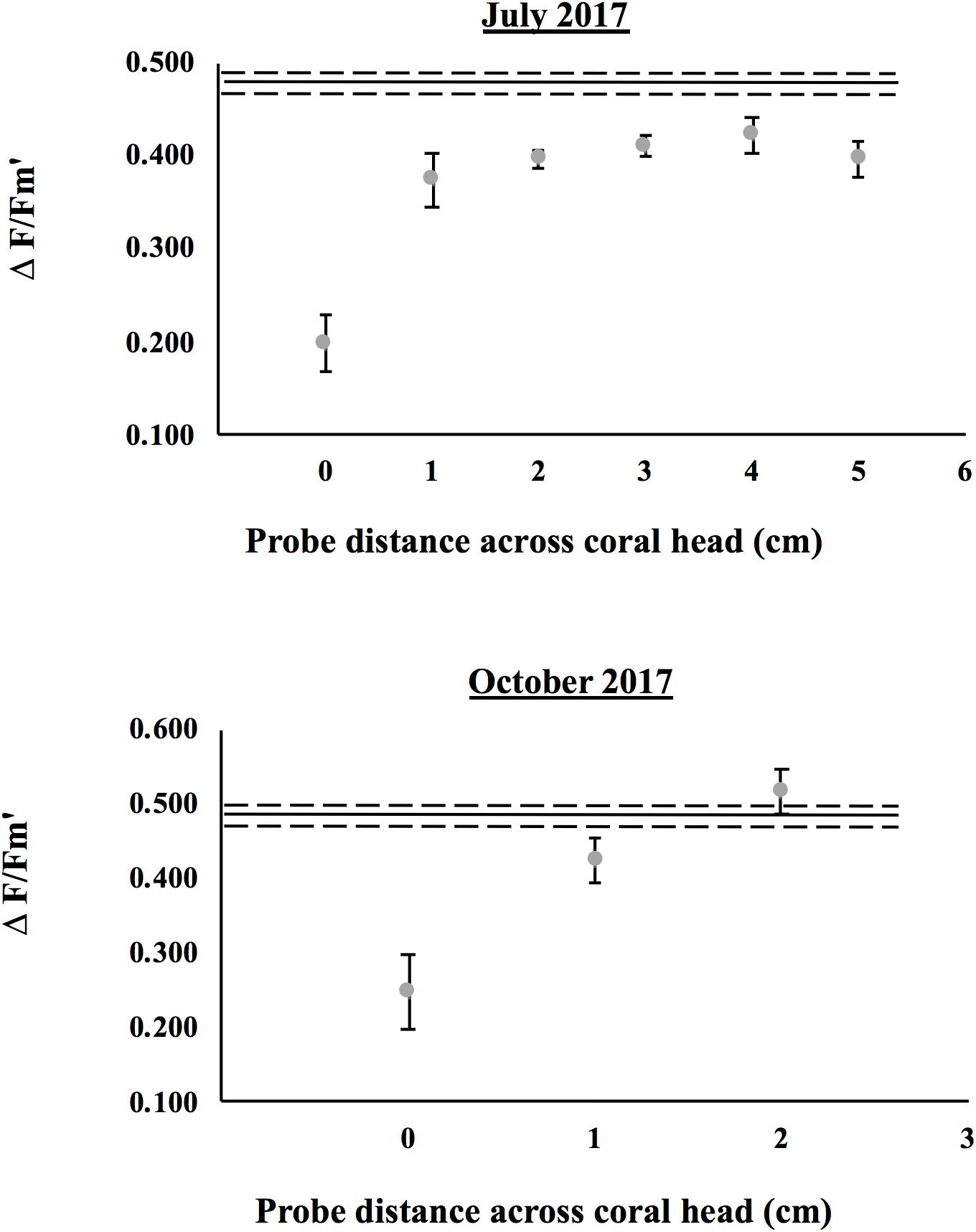
Figure 6. Comparisons of effective quantum yield (ΔF/Fm’) across colonies of Montastraea cavernosa in July and October 2017. The solid line represents the mean yield of healthy colonies across the probe transect (dashed lines represent the standard error). For healthy corals, yield transects were initiated at a haphazard location on the upper surface of the colony. Gray symbols represent individual yields (means ± SE) for diseased colonies along the linear transect. For diseased corals, yield measurements started at the bleached lesion front (0 cm) and progressed along a linear transect toward tissue healthy in appearance (2–6 cm away).
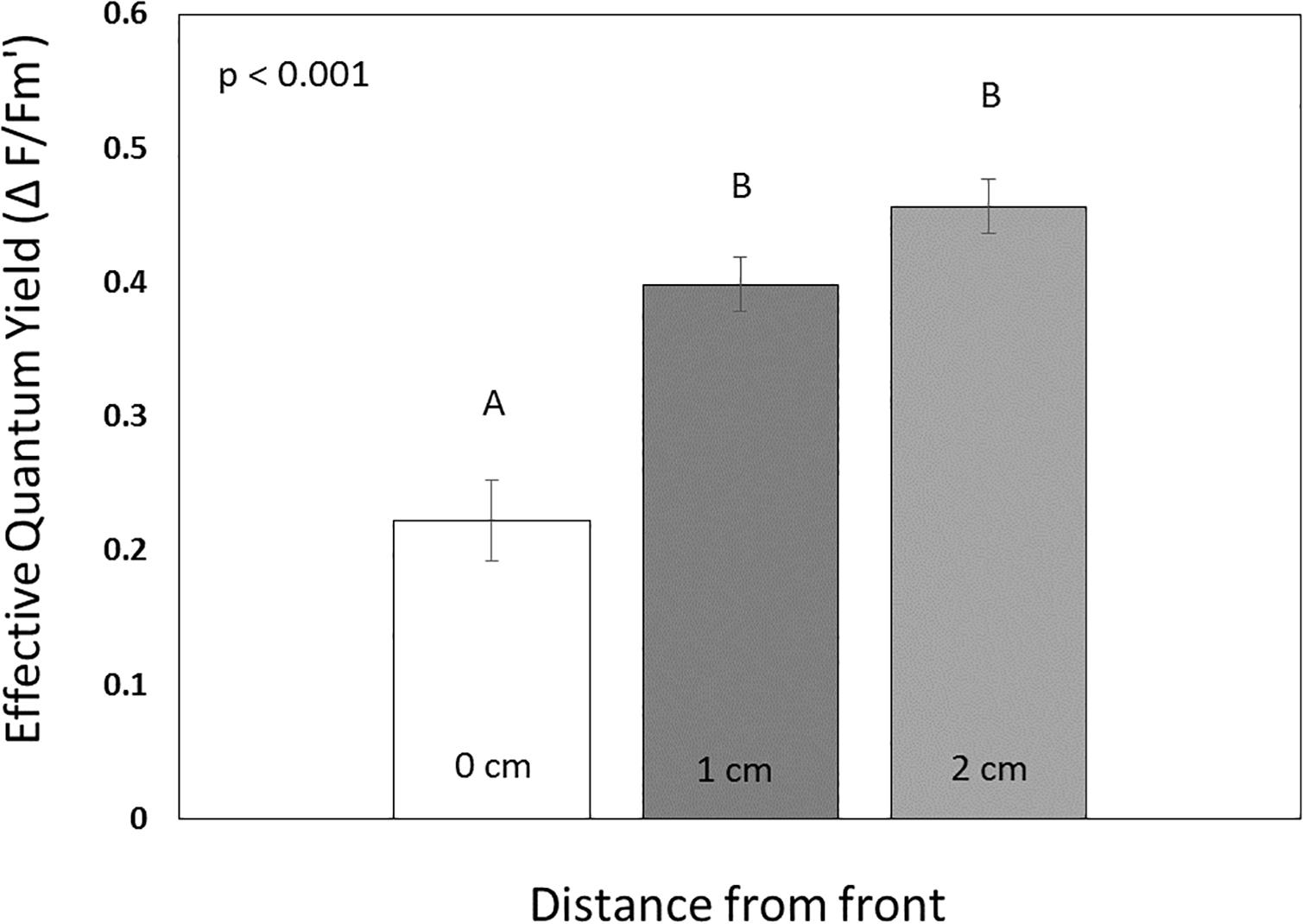
Figure 7. Comparisons of effective quantum yields (means ± SE, n = 16) for tissue at (0 cm removed), adjacent (1 cm), and removed (2 cm) from the disease front in Montastraea cavernosa. Statistical results of a repeated measures ANOVA are displayed. Bars with distinct lettering are significantly different (Sidak post hoc test).
Transmissibility, Mode of Transmission and Interspecific Variability to Infection
M. cavernosa vs. O. faveolata
When healthy M. cavernosa and O. faveolata were exposed to diseased M. cavernosa (subacute tissue loss lesions) we found successful disease transmission (development of a lesion) for both species upon direct contact and through the water column (Table 2). Three of the 10 healthy M. cavernosa fragments touching a diseased M. cavernosa developed lesions after an average of 3.5 days (range = 2–6 days), and one of 10 non-contact M. cavernosa developed a lesion after 9 days. Transmission to O. faveolata was more successful with 10 out of 10 of the contact fragments developing a lesion after an average of 4.6 days (range = 2–12 days) and 4 of 10 of the non-contact fragments developing lesions after an average of 10.3 days (range = 8–12 days). None of the control fragments developed lesions. Both species developed disease lesions but contact transmission to O. faveolata was more successful compared to M. cavernosa (Mantel–Cox, X2 = 9.4, df = 1, p = 0.002). In both species, non-contact transmission was lower than when in direct contact and was not significantly different among species (Mantel–Cox, X2 = 2.1, df = 1, p = 0.15).
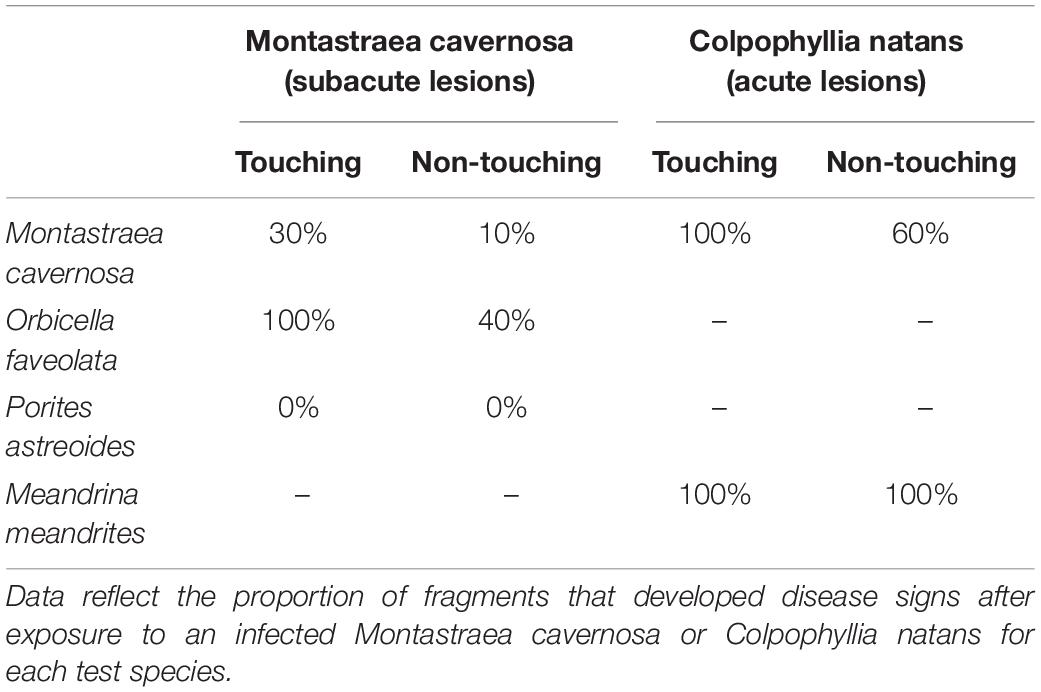
Table 2. Summary of results for aquaria studies examining the transmission of Montastraea cavernosa with subacute lesions and Colpophyllia natans with acute lesions to different test species.
M. cavernosa vs. P. astreoides
Successful transmission occurred between infected and healthy M. cavernosa fragments but no transmission (touching or non-touching) was found between infected M. cavernosa and healthy P. astreoides (Table 2). Two of the eight M. cavernosa touching diseased fragments developed lesions after an average of 5.3 days (range = 4–6) that progressed after contact was discontinued. In contrast, all of the P. astreoides fragments in contact with infected M. cavernosa also developed lesions within 1–2 days but all fragments showed signs of healing once contact was removed, indicating that the lesions were likely due to contact aggression and not disease. All of the P. astreoides fragments touching healthy fragments of M. cavernosa in control aquaria also developed lesions, which started healing once contact was terminated. No lesions developed on touching M. cavernosa fragments in control aquaria. None of the non-contact fragments developed lesions in either species.
M. cavernosa vs. M. meandrites
When healthy M. cavernosa and M. meandrites were exposed to C. natans fragments with acute lesions, disease transmission occurred in both species and at a faster rate compared to the transmission studies using M. cavernosa with subacute lesions (Table 2). There was 100% transmission for both test species (M. cavernosa and M. meandrites) for fragments directly touching the C. natans lesions (10 out of 10) and no significant difference in contact transmission between the two species (Mantel–Cox, X2 = 1.2, df = 1, p = 0.26). M. cavernosa fragments developed tissue loss lesions after an average of 3.8 days (range 3–5 days) and M. meandrites developed lesions after an average of 3.4 days (ranges 3–5 days). Lesions in both species progressed after contact was discontinued. The non-contact fragments in each species also developed tissue loss lesions in 6 of the 10 fragments of M. cavernosa after an average 4.7 days (range 4–5 days) and in 10 of 10 fragments in M. meandrites after an average of 4.3 days (range 3–6 days) (Mantel–Cox, X2 = 6.7, df = 1, p = 0.0094).
There was aggression between coral species in the control aquaria with one control M. cavernosa touching C. natans developing a tissue loss lesion 5 days after the start of the experiment and 7 of the M. meandrites developing lesions 3 days after contact. However, none of the lesions progressed once contact between corals was discontinued. It was also noted that there was more aggression and resultant tissue loss directed at C. natans by both of the test species than vice versa. All 10 control C. natans fragments in contact with M. cavernosa developed tissue loss lesions 1–2 days after contact and all 10 control C. natans developed lesions 1 day after contact with M. meandrites. Lesions on control C. natans did not progress after contact between corals was discontinued. Non-contact control fragments did not develop tissue loss lesions.
Therapeutic Diagnosis With Antibiotic Treatment
Fort Lauderdale: M. cavernosa With Subacute Tissue Loss Lesions
Disease lesions of the 13 M. cavernosa control fragments progressed during the experiment (Figure 8), with six of the controls showing 100% tissue loss after 10 days. For the experimental fragments, treatment with amoxicillin and kanamycin, completely arrested disease progression in 12 of the 13 fragments (McNemar’s test, p < 0.01). For the one experimental fragment with a lesion that progressed during treatment, tissue loss was initially arrested until day four of the experiment, whereas the untreated control had progressive tissue loss throughout the experiment. Among the 8 M. cavernosa sets without progressive tissue loss, four sets displayed signs of re-pigmentation of the bleached areas at the disease lesion of their control and treated fragments, but this was not quantified.
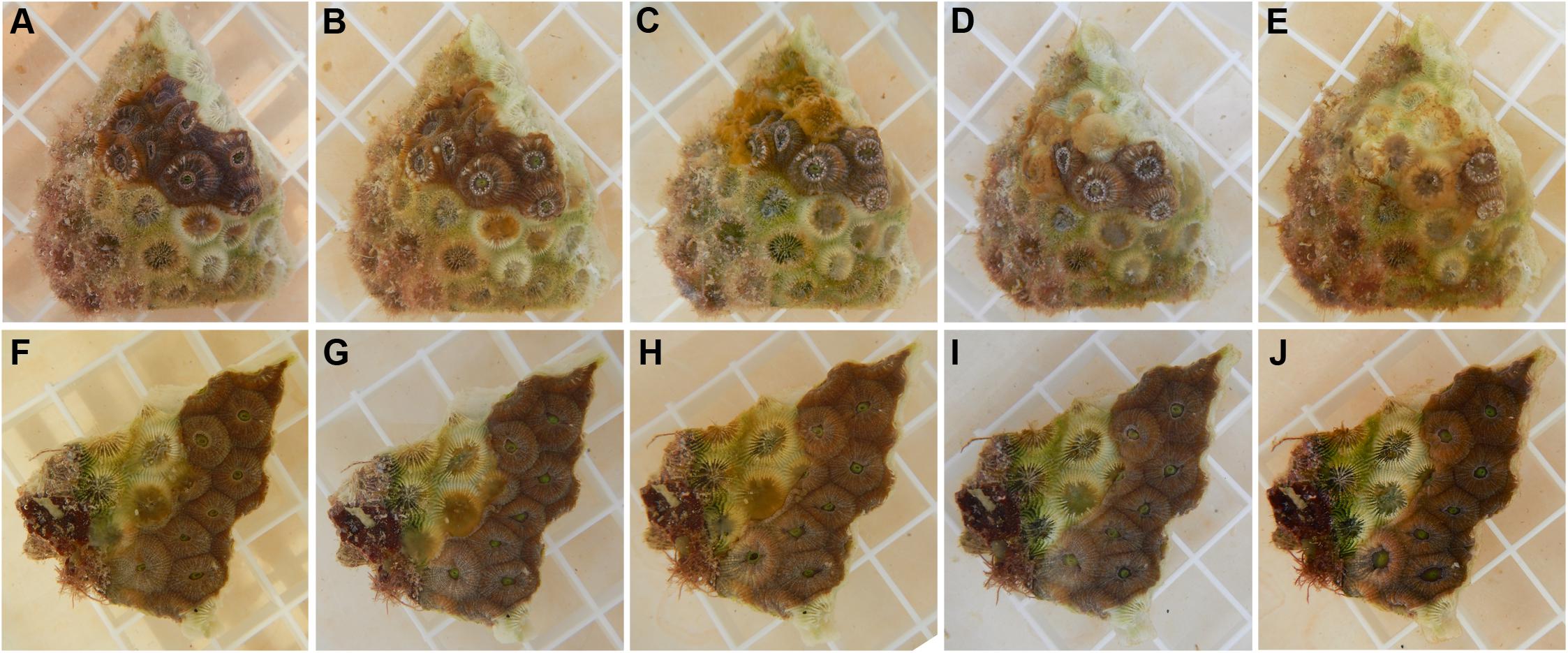
Figure 8. Antibiotic treatment of diseased Montastraea cavernosa. (A) Through (E) photos of a control fragment without antibiotic treatment (A) before the start of the experiment, (B) after 24 h, (C) 48 h, (D) 72 h and (E) 96 h. (F) Through (J) photos of an antibiotic treated fragment (F) before treatment, (G) after 24 h, (H) 48 h, (I) 72 h, and (J) 96 h. Each square on the grid is 1 × 1 cm.
Florida Keys: Coral Species With Acute Tissue Loss Lesions
All of the controls for the C. natans (n = 8) and M. meandrites (n = 3) progressed throughout the experiment resulting in 100% tissue loss within 4–5 days for C. natans and 4–9 days for M. meandrites. Treatment with amoxicillin and kanamycin completely arrested tissue loss for all three treated M. meandrites fragments (Figure 9). The combination antibiotic treatment or amoxicillin alone was not tolerated by C. natans, so a single broad-spectrum antibiotic, nalidixic acid, was used instead. Treatment of C. natans with nalidixic acid was less effective at preventing disease progression as compared to the corals treated with the amoxicillin and kanamycin combination. However, five of the eight diseased C. natans fragments had lesions that were stopped (n = 2) or slowed (n = 3) by antibiotic treatment (Figure 9), which was significantly different compared to the non-treated controls (Mantel–Cox’s test, p < 0.01, n = 8).
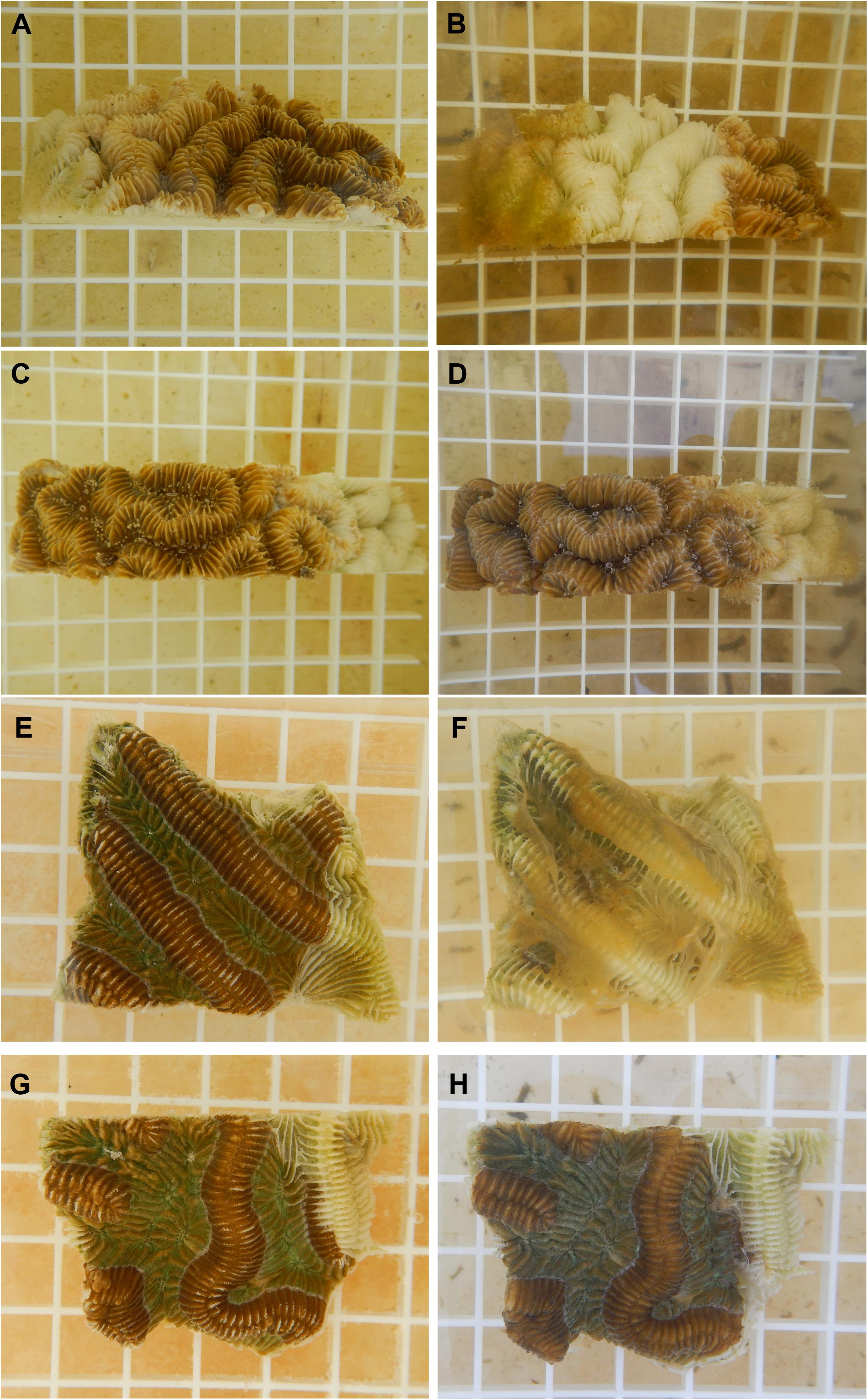
Figure 9. Antibiotic treatment of diseased Meandrina meandrites and Colpophyllia natans. (A) A diseased non-treated M. meandrites fragment at the start of the experiment and (B) the same fragment after 8 days. (C) A diseased M. meandrites fragment before treatment with amoxicillin and kanamycin, and (D) the same fragment 8 days after treatment. (E) A diseased non-treated C. natans fragment at the start of the experiment, and (F) the same fragment after 4 days. (G) A diseased C. natans fragment before treatment with nalidixic acid, and (H) the same fragment 8 days after treatment. Each square on the grid is 1 × 1 cm.
Discussion
We examined the disease ecology of coral species exhibiting different types of tissue loss lesions from two regions in Florida: corals with subacute lesions near Fort Lauderdale and corals with acute lesions in the Middle Keys. This study demonstrates that corals with both types of lesions are progressive tissue loss diseases, and the average rates of tissue loss differ between species and regions. Manipulative experiments demonstrate that diseased corals with both types of lesions (subacute and acute) are transmissible through direct contact and through the water column and disease susceptibility varies among species. Most diseased fragments of all species tested, whether with subacute or acute lesions, responded to antibiotic treatment with a cessation or slowing of the disease lesions suggesting that bacteria may be involved in disease progression. However, the degree of colony mortality and transmissibility exhibited by the two lesion types differs dramatically and so the involvement of multiple pathogens cannot be ruled out.
At our Fort Lauderdale site, all tagged M. cavernosa colonies were initially infected but the outcome from disease varied in terms of amount of mortality and duration of infection. Some colonies died, others had minimal tissue loss, and one colony tagged in the early stages of the disease, with only a bleached lesion, completely healed. Duration of the infection also varied among colonies. For four M. cavernosa colonies, the disease persisted throughout the 12-month study while two other colonies lost all disease signs for months and then again showed active lesions. However, for most M. cavernosa colonies (12 colonies), disease signs disappeared and colonies remained lesion-free for the remainder of the study. Other tissue loss diseases show a similar pattern of lesions waxing and waning such as Montipora white syndrome on corals in Hawaii (Work et al., 2012). In addition, during our aquaria studies lesions on some of the M. cavernosa fragments failed to progress, and instead bleached lesion margins started re-pigmenting, suggesting the fragment was healing from disease.
We hypothesized that factors such as colony size, stage of the disease at the onset of a study or differences in disease duration would all contribute to disease outcome. It logically follows and has been found in other studies (Nugues, 2002) that a smaller colony, with less tissue to lose, would suffer higher mortality or that colonies in a more active disease state, e.g., larger lesions at the start of study, would die faster. However, we found the best predictor of total colony mortality was exclusively duration of the infection through time, e.g., colonies that had defensive traits that allowed them to overcome the disease survived. It is also possible that multiple pathogens are involved in disease in M. cavernosa at our site and may be contributing to differences in mortality among tagged colonies. Corals have a suite of defense mechanisms to protect themselves from potential pathogens, including the production, release, and biochemical properties of mucus, mucus-associated bacterial communities, phagocytic cells that can engulf and destroy micro-organisms, and antimicrobial chemical defenses (Mullen et al., 2004; Ritchie, 2006; Bourne et al., 2009; Mydlarz et al., 2010). Differences in types and levels of defense vary among families, genera, and species and at the level of the individual colony (Mullen et al., 2004; Gochfeld and Aeby, 2008; Shore-Maggio et al., 2015). These differences might enable particular populations, species, or genotypes to have an advantage over others in resisting or overcoming invasion by pathogens. The high variability we found in survival among infected M. cavernosa colonies, suggests that this population of corals possess significant differences in defense capacity and this offers a nugget of hope for coral populations devastated by SCTLD.
Currently, there is an emphasis on restoration of reefs in Florida4 and so colony-level variability in disease resistance should be considered when choosing which genotypes to culture. The next step would be to discover the critical defense mechanisms enabling corals to survive SCTLD and target colonies possessing those traits for restoration efforts. It should also be noted that disease resistance can come at a cost to other traits such as bleaching resistance (Shore-Maggio et al., 2015) and so careful consideration should be given to which genotypes and species are selected for restoration.
For diseased M. cavernosa, the lesion margin usually, but not always, had a bleached border (avg. ∼2 cm in width), suggesting the health of the coral-zooxanthellae symbiosis was compromised before actual tissue loss occurred. This was confirmed using in situ pulse-amplitude modulated fluorometry, with the photosynthetic efficiency of the active front (bleached zone) found to be significantly reduced compared to healthy tissue. Impairment of the coral-zooxanthellae symbiosis was also documented for Montastraea spp. with yellow band disease (Cervino et al., 2004) and Sussman et al. (2009) found photoinactivation of endosymbiotic zooxanthellae and eventual tissue loss in corals when exposed to proteins produced in cultures of bacterial pathogens responsible for white syndromes on the Great Barrier Reef. In our study, tissue within 1–2 cm beyond the bleached border displayed normal effective quantum yields suggesting that the effect of disease on photosynthetic efficiency is generally localized to the immediate lesion. Effective quantum yields were significantly higher in October as compared to July 2017. Because effective yields, and not maximum quantum yields (requiring dark adaptation), were measured, it is likely that variation in short-term light history was responsible for differences in yields across dates. But, for both time periods, there was a consistent pattern of reduced photosynthetic efficiency only at the bleached edge of the lesion. Currently, the lesion occlusion method to arrest disease (covering the lesion with antibiotics or marine epoxy) is being tested in Florida for the ongoing SCTLD outbreak5, and in this context, it is important to know that for diseased M. cavernosa, the tissue does not appear to be affected (photosynthetically compromised) much beyond the bleached border.
On most M. cavernosa colonies, the lesion maintained a bleached edge, but some lesions did shift through time. Several colonies started with a bleached border, shifted to tissue loss adjacent to healthy tissue on the next survey, and then went back to a bleached border on the following month. On lesions that became inactive, we did not see much re-sheeting of tissue along lesion edges. Dead areas of colonies, instead, became covered with sediment, algae and invasive sponges, which hampers coral recovery and has been found in other studies involving tissue loss from disease (Nugues, 2002; Brandt et al., 2012).
Many coral diseases have been linked to warmer temperatures including yellow band disease (Cervino et al., 2004), black band disease (Edmunds, 1991; Sato et al., 2009) and white syndromes in the Indo-Pacific (Bruno et al., 2007; Heron et al., 2010; Maynard et al., 2010; Williams et al., 2011). However, we found no consistent pattern with SCTLD related to temperature. We initially tagged infected M. cavernosa colonies in the summer of 2017 and most colonies remained with active lesions through November 2017. Prevalence started to decline at the December 2017 survey, hit a low by March 2018 and remained low for the duration of the study, which extended through July 2018. The rate of tissue loss on colonies showed a similar pattern with percent colony loss higher through November 2017 with a subsequent decline that remained low until the end of the study. Sea surface temperatures near Fort Lauderdale average 22–23°C in the winter months with temperatures starting to rise in April and May and obtain highs of 28–29°C in July and August6. However, neither disease prevalence nor rate of tissue loss on tagged colonies increased in 2018 as water temperatures warmed. In addition, disease did not emerge on the reef in the Middle Keys until December 2017 when winter water temperatures had already dropped. The changes in disease levels we found among our tagged colonies suggests a shift in some component of the pathogen-host ecology yet water temperature does not appear to be the main driving factor. Environmental factors contribute to host-pathogen dynamics in many disease systems including corals (Harvell et al., 2007), but the underlying factors allowing the emergence and continued spread of SCTLD are not yet understood. An important step for Florida in managing this ongoing disease outbreak will be to determine what environmental parameters are contributing to the ongoing disease outbreak on Florida’s reefs.
Lesion progression on the tagged colonies in the Middle Keys was noticeably faster compared to the colonies around Fort Lauderdale. Tagged colonies in the Middle Keys, regardless of species, had a high rate of mortality with most of the colonies dead after 7 months. Additionally, the acute lesions showed no bleaching along lesion margins, which could be attributed to either the zooxanthellae-coral symbiosis not being impacted or tissue loss being too rapid for bleaching to be observed. The duration of the disease event was also much shorter for the Middle Keys with tagged colonies either dead or disease-free by month seven compared to the Fort Lauderdale corals where a disease was still ongoing after a year. Unfortunately, no M. cavernosa colonies were available for tagging at our site in the Middle Keys so no direct comparisons of mortality can be made between regions within our study. However, studies in other regions on this disease did find high mortality in M. cavernosa (Precht et al., 2016).
It must be noted that most of the tagged colonies in the Middle Keys were smaller (avg. max. diameter = 17.6 cm) compared to the Fort Lauderdale colonies (avg. max. diameter = 53.5 cm). It is likely that smaller colonies, once infected, would have higher mortality rates as has been reported for other disease outbreaks (Nugues, 2002), although this was not found for diseased M. cavernosa at Fort Lauderdale. Regardless of colony sizes, we found that the daily rate of tissue loss in the Middle Keys colonies was significantly higher than the highest rate observed in the Fort Lauderdale colonies. The amount of rapid disease-induced mortality found in the Middle Keys is similar to what has been reported for this current outbreak in other regions (Precht et al., 2016; Walton et al., 2018) as well as for numerous other disease outbreaks across Florida (Richardson et al., 1998a; Williams and Miller, 2005; Brandt et al., 2012).
At the Middle Keys site, we found four species heavily impacted by SCTLD. The pathogen(s) responsible for this ongoing outbreak appear to be host generalists, but do not appear to affect all coral species as there were species with no disease signs at our sites. This was confirmed by our aquaria studies which found successful disease transmission between some, but not all species. Precht et al. (2016) and Walton et al. (2018) also report multiple coral species affected but with differences among species in susceptibility and prevalence. At our study site in the Middle Keys, disease spread within the site with numerous initially healthy tagged colonies developing disease signs but two of the eight healthy colonies (one P. astreoides and one D. labyrinthiformis) remained disease-free after 7 months. Newly infected colonies were not in contact with infected ones suggesting the mode of transmission to be either water or vector-borne. Snails (Williams and Miller, 2005), fireworms (Sussman et al., 2003) and reef fish (Aeby and Santavy, 2006; Raymundo et al., 2009) have all been identified as potential coral disease vectors at within reef scales. The disease has already spread within and across reefs within the Florida Reef Tract (Precht et al., 2016; Walton et al., 2018) potentially indicative of multiple modes of transmission. The manipulative experiments presented in this study show that SCTLD is a waterborne disease, where the pathogen(s) could be carried by ocean currents. Transmission between reefs via ocean currents was proposed for black band disease by Bruckner et al. (1997) who found the spread of the disease along reefs was in the direction of the prevailing ocean currents. The etiological agents could also be transferred via fomite or pelagic vectors (plankton) that may be carried by ocean currents. Aeby et al. (2016) hypothesized that the spread of acute Montipora white syndrome between reefs in Kaneohe Bay, Oahu might have been facilitated by phytoplankton and zooplankton blooms associated with storm runoff and eutrophication. SCTLD has spread widely across the Florida Reef Tract and more research is needed to understand the multiple modes of transmission underlying disease spread both within and between reefs.
Our aquaria studies confirmed differences in transmission rates among coral species. When M. cavernosa with subacute lesions was tested with healthy M. cavernosa, O. faveolata and P. astreoides, O. faveolata was found to be the most susceptible species and P. astreoides appeared to be fairly disease resistant. There was significant aggression noted between P. astreoides and M. cavernosa and both species developed tissue loss lesions at the point of contact. This may have affected transmission success with P. astreoides, but one might hypothesize that a coral’s defenses, likely compromised by a lesion, would have resulted in increased disease transmission. In all cases, once contact between species was terminated the lesions on P. astreoides (experimental and control aquaria) began to heal. Porites spp. are often thought to be less susceptible to tissue loss diseases based on field patterns of disease prevalence (Willis et al., 2004; Aeby et al., 2011, 2015) including in Florida species (Porter et al., 2001; Santavy et al., 2001), and with direct inoculation of known coral pathogens in the laboratory (Ushijima et al., 2014). However, there are regional exceptions, such as the Philippines (Raymundo et al., 2005) and the Persian Gulf (Riegl et al., 2012; Alidoost Salimi et al., 2017), where Porites sp. display comparatively higher prevalence of tissue loss diseases in the field.
When we examined transmission dynamics of C. natans with acute lesions a very different pattern emerged. In these experiments, M. cavernosa and M. meandrites each had 100% successful transmission after contact with diseased C. natans. This was surprising, as in the field, M. meandrites is considered one of the most susceptible species to SCTLD, whereas M. cavernosa has an intermediate level of susceptibility7. Yet, under controlled experimental conditions M. meandrites and M. cavernosa were equally susceptible upon direct contact. Both test species also had high rates of transmission among the non-contact fragments, but M. meandrites did have a significantly higher rate of waterborne transmission than did M. cavernosa. This suggests that there are factors, other than just innate immunity, that determines susceptibility and disease patterns in the field. Coral species differ in their abilities to rid themselves of sediment and other settling organisms such as potential pathogens and colony morphology contributes to those differences (Stafford-Smith and Ormond, 1992; Duckworth et al., 2017). M. cavernosa is a massive coral whereas M. meandrites is a brain coral with deep grooves. Sediment or other material, including potential pathogens, can get trapped in concave areas on colonies so perhaps differences in colony morphology are affecting field patterns of infection among coral species. Conversely, most species of corals are active heterotrophs ingesting a variety of prey items from bacteria to zooplankton (Houlbreque and Ferrier-Pages, 2009) as well as feeding on particulate matter (Anthony, 1999). Feeding strategies also differ among coral species with some species feeding by tentacle capture, others feed via mucus nets or mucus filaments, and some use a combination of the two strategies (Lewis and Price, 1975). Depending on whether the pathogen is carried directly in the water, clinging to particulate matter or attached to zooplankton vectors, then initial infection might be dependent upon a coral species feeding strategy. Understanding the multiple modes of transmission potentially used by this pathogen would help shed light on the observed field patterns of infection among species.
The other interesting finding from these transmission experiments was the higher transmission rate, contact and non-contact, for the test coral M. cavernosa, when exposed to corals with acute lesions compared to corals with subacute lesions. We also reviewed photographs of M. cavernosa fragments in all three transmission experiments and found that lesion sizes differed on M. cavernosa in contact with subacute vs. acute lesions. Visual estimates showed that M. cavernosa touching diseased M. cavernosa had smaller lesions (avg. percent tissue loss = 17.4% of the fragment, n = 5) compared to when touching diseased C. natans (avg. percent tissue loss = 54% of the fragment, n = 10). This opens up the possibility that distinct pathogens may be underlying these different lesion types. Similarly, in Kaneohe Bay, Oahu the reef Montipora capitata displays chronic and acute tissue loss lesions (Aeby et al., 2010, 2016) that were found to be caused by different bacterial pathogens (Ushijima et al., 2012, 2014; Beurmann et al., 2017).
For all of the transmission studies, it must be kept in mind that these experiments were conducted under artificial conditions (aquaria with filtered water, all experimental fragments (healthy and diseased) cut into pieces), which likely affected the rates of transmission. As example, the acute lesions on C. natans progressed rapidly usually within 6 days and so even with daily partial water changes sloughing tissue remained in the water, which might have contributed to the high transmission success of non-contact fragments. Therefore, although the conclusions drawn about whether the disease is transmissible or not and differences among species are supported, the actual rates may differ from transmission occurring under natural conditions.
The transmission experiments demonstrated that disease lesions were caused by an infectious agent, however, there are a plethora of microorganisms commonly present in tissue loss lesions (Work et al., 2012). Sweet et al. (2014) used antibiotic treatments to determine if white band disease in Acropora cervicornis could be caused by a bacterial pathogen and we took a similar approach using antibiotic treatment for diagnostic purposes. Diseased fragments of all species tested, whether with subacute or acute lesions, responded to antibiotic treatment with a cessation or slowing of the disease lesions, suggesting the involvement of pathogenic bacteria in lesion progression. This was not surprising as bacterial pathogens have been identified for many other coral tissue loss diseases (Richardson et al., 1998b; Patterson et al., 2002; Ben-Haim and Rosenberg, 2002; Denner et al., 2003; Sussman et al., 2008; Ushijima et al., 2012, 2014, 2016; Beurmann et al., 2017). Although, whether the different lesion types, subacute on M. cavernosa and acute on M. meandrites and C. natans, involve the same pathogenic bacteria is not yet understood as host-pathogen interactions are typically complex and difficult to unravel. For example, although the antibiotic treatments suggest the involvement of a pathogenic bacterium, there are caveats. First, there may be a consortium of bacteria required for an infection to occur. This scenario is exemplified by yellow band disease and black band disease, which both require a mixture of multiple bacterial strains to initiate disease (Richardson, 2004; Cervino et al., 2008). Alternatively, there could be a succession of multiple bacterial infections. For example, Beurmann et al. (2017) found that the coral pathogen Pseudoalteromonas piratica can infect healthy Montipora capitata (33% infection after an average of 22 days post-inoculation) but is much more successful at infecting M. capitata already compromised by a chronic tissue loss disease (56% infection after an average of 8 days post-inoculation). Finally, there could be an underlying viral infection affecting the corals in Florida, which could be making them more susceptible to secondary bacterial infections. Such is the case with OsHV-1 viral infections of Pacific oysters (Crassostrea gigas), where some families of oysters do not develop disease signs after infection by OsHV-1, however, the viral infection suppresses their immune system making them more susceptible to secondary bacterial infections (de Lorgeril et al., 2018).
One of the key questions about the ongoing disease outbreak on Florida’s reefs is whether the different lesion types observed on corals are indicative of different pathogens, different stages of the same disease, differential host response to a common pathogen or a combination of all. Our study provides some clues by examining the similarities and differences in disease ecology of M. cavernosa with subacute tissue loss and other species displaying acute tissue loss lesions. We found some similarities among lesion types (transmissible, responds to antibiotic treatment) but also differences (degree of colony mortality, transmission rate). We examined lesion types on different coral species from different regions so perhaps these differences merely indicate species or regional differences in response to a common pathogen. However, Precht et al. (2016) monitored tagged colonies with SCTLD in the Miami-Dade region between September 2014, when the disease first emerged, and July 2015 and they found that all diseased colonies died regardless of species, including M. cavernosa. Further evidence, suggesting they may be different diseases, comes from our findings that healthy M. cavernosa responded differently when exposed to corals with subacute lesions (low rate of transmission) vs. corals with acute lesions (high rate of transmission). As such, it appears there is something beyond species response that is different in the pathogenesis of these diseases.
Management of emerging infectious diseases, such as SCTLD, requires a good understanding of the ecology and pathogenesis of the disease. Prior outbreaks of acute tissue loss disease affecting multiple coral species have occurred in Florida and were called white plagues (Dustan, 1977; Richardson et al., 1998a; Brandt et al., 2012). One outbreak that emerged in Florida in 1995 was also widespread in spatial (∼200 km of Florida Reef Tract) and temporal (3 years) extent (Richardson et al., 1998a) similar to the current outbreak. However, the pathogen, Aurantimonas coralicida, identified from the 1995 white plague event (Richardson et al., 1998b; Denner et al., 2003), was not identified in lesions from the multiple coral species examined in this outbreak (Meyer et al., 2019) nor was it found in studies of past white plague outbreaks (Sunagawa et al., 2009). This highlights that tissue loss diseases cannot be diagnosed based on field signs alone (Work et al., 2012; Bourne et al., 2014). A pathogen has not yet been identified from the current outbreak either, hence it is unclear whether the past outbreaks or current outbreaks are caused by the same, different or multiple pathogens. This highlights the need for more research, using a multi-disciplinary approach, to understand the complex pathogen-coral-environment dynamics influencing the current disease outbreak.
Data Availability Statement
The datasets generated for this study are available on request to the corresponding author.
Author Contributions
GA, BU, VP, JM, and CH conceived the study. GA, VP, and SJ conducted the fieldwork. GA and BU conducted the transmission and antibiotic trials. JC examined the zooxanthellae-coral symbiosis in M. cavernosa. GW contributed to the statistical analyses. All authors were involved in writing the manuscript.
Funding
This research was supported by National Science Foundation award IOS-1728002 (VP, CH), EPA Wetlands Program Grant CD00D66917 (JM, VP, CH), the Florida Coastal Office – Southeast Region, within the Florida Department of Environmental Protection (VP, BU, GA), and the Mote Marine Laboratory Protect our Reefs Grant Program POR 2016-21 (VP).
Conflict of Interest
The authors declare that the research was conducted in the absence of any commercial or financial relationships that could be construed as a potential conflict of interest.
Acknowledgments
We thank Woody Lee and Kendall Lee for help setting up and maintaining the water tables and Jay Houk and Sharon Thompson for numerous hours in the microbiology laboratory. Thanks also to Ken Banks, Audrey Looby, Cindy Lewis, and Angel Rivera for logistical support in the field and Jennifer Sneed for support and valuable discussions. Field collections were authorized under Florida Fish and Wildlife Conservation Commission Special Activity Licenses SAL-16-1702A-SRP and SAL-17-1702-SRP and Florida Keys National Marine Sanctuary permit # FKNMS-2017-128-A2. The manuscript was improved by suggestions from the three reviewers. This is contribution # 1124 from the Smithsonian Marine Station and contribution # 168 from the Center for Coastal Oceans in the Institute of Water and Environment at Florida International University.
Footnotes
- ^ https://floridakeys.noaa.gov/coral-disease/disease.html
- ^ https://nmsfloridakeys.blob.core.windows.net/floridakeys-prod/media/docs/20181002-stony-coral-tissue-loss-disease-case-definition.pdf
- ^ https://floridadep.gov/rcp/coral/content/florida-reef-tract-coral-disease-outbreak
- ^ https://floridadep.gov/rcp/coral/content/restoration-trials-team
- ^ https://floridadep.gov/fco/aquatic-preserve/documents/sefl-large-diseased-coral-colony-intervention-summary
- ^ https://www.seatemperature.org/north-america/united-states/fort-lauderdale.htm
- ^ https://nmsfloridakeys.blob.core.windows.net/floridakeys-prod/media/docs/20181002-stony-coral-tissue-loss-disease-case-definition.pdf
References
Aeby, G., Callahan, S., Cox, E., Runyon, C., Smith, A., Stanton, F., et al. (2016). Emerging coral diseases in Kâne‘ohe Bay, O‘ahu, Hawai‘i (USA): two major disease outbreaks of acute Montipora white syndrome. Dis. Aquat. Organ 119, 189–198. doi: 10.3354/dao02996
Aeby, G., and Santavy, D. (2006). Factors affecting susceptibility of the coral Montastraea faveolata to black-band disease. Marine Ecology Progress Series 318, 103–110. doi: 10.3354/meps318103
Aeby, G., Tribollet, A., Lasne, G., and Work, T. (2015). Assessing threats from coral and crustose coralline algae disease on the reefs of New Caledonia. Marine and Freshwater Research 67, 455–465. doi: 10.1071/MF14151
Aeby, G. S., Ross, M., Williams, G. J., Lewis, T. D., and Work, T. M. (2010). Disease Dynamics of Montipora White Syndrome within Kaneohe Bay, Oahu, Hawaii: Distribution, Seasonality, Virulence, and Transmissibility. Diseases of Aquatic Organisms 91, 1–8. doi: 10.3354/dao02247
Aeby, G. S., Williams, G. J., Franklin, E. C., Kenyon, J., Cox, E. F., Coles, S., et al. (2011). Patterns of Coral Disease across the Hawaiian Archipelago: Relating Disease to Environment. PLoS ONE 6:e20370. doi: 10.1371/journal.pone.0020370
Akaike, H. (1973). Maximum likelihood identification of Gaussian autoregressive moving average models. Biometrika 60, 255–265. doi: 10.1093/biomet/60.2.255
Alidoost Salimi, M., Mostafavi, P., Fatemi, S., and Aeby, G. (2017). Health status of corals surrounding Kish Island, Persian Gulf. Dis. Aquat. Organ 124, 77–84. doi: 10.3354/dao03105
Anthony, K. (1999). Coral suspension feeding on fine particulate matter. J Experimental marine biology and ecology 232, 85–106. doi: 10.1016/s0022-0981(98)00099-9
Aronson, R. B., and Precht, W. (1997). Stasis, Biological Disturbance, and Community Structure of a Holocene Coral Reef. Paleobiology 23, 326–346. doi: 10.1017/s0094837300019710
Aronson, R. B., and Precht, W. F. (2001). “White-band disease and the changing face of Caribbean coral reefs,” in The Ecology and Etiology of Newly Emerging Marine Diseases, ed. J. W. Porter, (Dordrecht: Springer), 25–38. doi: 10.1007/978-94-017-3284-0-2
Ben-Haim, Y., and Rosenberg, E. (2002). A novel Vibrio sp. pathogen of the coral Pocillopora damicornis. Marine Biology 141, 47–55. doi: 10.1007/s00227-002-0797-6
Beurmann, S., Ushijima, B., Videau, P., Svoboda, C. M., Smith, A. M., Rivers, O. S., et al. (2017). Pseudoalteromonas piratica strain OCN003 is a coral pathogen that causes a switch from chronic to acute Montipora white syndrome in Montipora capitata. PLoS ONE 12:e0188319. doi: 10.1371/journal.pone.0188319
Bourne, D. G., Ainsworth, T. D., Pollock, F. J., and Willis, B. L. (2014). Towards a better understanding of white syndromes and their causes on Indo-Pacific coral reefs. Coral Reefs 34, 233–242. doi: 10.1007/s00338-014-1239-x
Bourne, D. G., Garren, M., Work, T. M., Rosenberg, E., Smith, G. W., and Harvell, C. D. (2009). Microbial disease and the coral holobiont. Trends in Microbiology 17, 554–562. doi: 10.1016/j.tim.2009.09.004
Brandt, M. E., Ruttenberg, B. I., Waara, R., Miller, J., Witcher, B., Estep, A. J., et al. (2012). Dynamics of an Acute Coral Disease Outbreak Associated with the Macroalgae Dictyota spp. in Dry Tortugas National Park, Florida, USA. Bulletin of Marine Science 88, 1035–1050. doi: 10.5343/bms.2011.1104
Bruckner, A. W., Bruckner, R. J., and Williams, E. H. (1997). Spread of a black band disease epizootic through the coral reef system in St. Ann’s Bay, Jamaica. Bulletin of Marine Science 61, 919–928.
Bruno, J. F., Selig, E. R., Casey, K. S., Page, C. A., Willis, B. L., Harvell, C. D., et al. (2007). Thermal Stress and Coral Cover as Drivers of Coral Disease Outbreaks. PLoS Biol. 5:e124. doi: 10.1371/journal.pbio.0050124
Burnham, K., and Anderson, D. (2004). Multimodel Inference: Understanding AIC and BIC in Model Selection. Sociological Methods & Research 33, 261–304. doi: 10.1177/0049124104268644
Cervino, J. M., Hayes, R., Goreau, T. J., and Smith, G. W. (2004). Zooxanthellae Regulation in Yellow Blotch/Band and Other Coral Diseases Contrasted with Temperature Related Bleaching: In Situ Destruction vs Expulsion. Symbiosis 37, 63–85.
Cervino, J. M., Thompson, F. L., Gomez-Gil, B., Lorence, E. A., Goreau, T. J., Hayes, R. L., et al. (2008). The Vibrio core group induces yellow band disease in Caribbean and Indo-Pacific reef-building corals. J. Appl. Microbiol. 105, 1658–1671. doi: 10.1111/j.1365-2672.2008.03871.x
Daszak, P., Cunningham, A. A., and Hyatt, A. D. (2000). Emerging Infectious Diseases of Wildlife– Threats to Biodiversity and Human Health. Science 287, 443–449. doi: 10.1126/science.287.5452.443
Daszak, P., Cunningham, A. A., and Hyatt, A. D. (2001). Anthropogenic environmental change and the emergence of infectious diseases in wildlife. Acta Trop. 78, 103–116. doi: 10.1016/s0001-706x(00)00179-0
de Lorgeril, J., Lucasson, A., Petton, B., Toulza, E., Montagnani, C., Clerissi, C., et al. (2018). Immune-suppression by OsHV-1 viral infection causes fatal bacteraemia in Pacific oysters. Nature Communications 9, 4215. doi: 10.1038/s41467-018-06659-3
Denner, E. B. M., Smith, G. W., Busse, H. J., Schumann, P., Narzt, T., Polson, S. W., et al. (2003). Aurantimonas coralicida gen. nov., sp. nov., the causative agent of white plague type II on Caribbean scleractinian corals. International Journal of Systematic and Evolutionary Microbiology 53, 1115–1122. doi: 10.1099/ijs.0.02359-0
Duckworth, A., Giofre, N., and Jones, R. (2017). Coral morphology and sedimentation. Mar. Pollut. Bull. 125, 289–300. doi: 10.1016/j.marpolbul.2017.08.036
Dustan, P. (1977). Vitality of reef coral populations off Key Largo. Florida: Recruitment and mortality. Environmental Geology 2, 51–58. doi: 10.1007/BF02430665
Edmunds, P. J. (1991). Extent and effect of black band disease on a Caribbean reef. Coral Reefs 10, 161–165. doi: 10.1007/BF00572175
Gardner, T., Cote, I., Gill, J., Grant, A., and Watkinson, A. (2003). Long-term region-wide declines in Caribbean corals. Science 301, 958–960. doi: 10.1126/science.1086050
Gochfeld, D., and Aeby, G. (2008). Antibacterial chemical defenses in Hawaiian corals provide possible protection from disease. Marine Ecology Progress Series 362, 119–128. doi: 10.3354/meps07418
Grieve, A. P. (1984). Tests of sphericity of normal distributions and the analysis of repeated measures designs. Psychometrika 49, 257–267. doi: 10.1007/bf02294176
Harvell, C. D., Jordan-Dahlgren, E., Merkel, S., Rosenberg, E., Raymundo, L., Smith, G., et al. (2007). Coral disease, environmental drivers and the balance between coral and microbial associates. Oceanography 20, 172–195. doi: 10.5670/oceanog.2007.91
Heron, S. F., Willis, B. L., Skirving, W. J., Eakin, C. M., Page, C. A., and Miller, I. R. (2010). Summer Hot Snaps and Winter Conditions: Modelling White Syndrome Outbreaks on Great Barrier Reef Corals. PLoS ONE 5:e12210. doi: 10.1371/journal.pone.0012210
Hewitt, W. L., Seligman, S. J., and Deigh, R. A. (1966). Kinetics of the synergism of penicillin-streptomycin and penicillin-kanamycin for enterococci and its relationship to L-phase variants. Journal of Laboratory and Clinical Medicine 67, 792–807.
Hooton, T. M., Blair, A. D., Turck, M., and Counts, G. W. (1984). Synergism at clinically attainable concentrations of aminoglycoside and β-lactam antibiotics. Antimicrob. Agents Chemother. 26, 535–538. doi: 10.1128/aac.26.4.535
Houlbreque, F., and Ferrier-Pages, C. (2009). Heterotrophy in tropical scleractinian corals. Biological Reviews 84, 1–17. doi: 10.1111/j.1469-185x.2008.00058.x
Hurvich, C. M., and Tsai, C. L. (1989). Regression and time series model selection in small samples. Biometrika 76, 297–307. doi: 10.1093/biomet/76.2.297
Jackson, J., Donovan, M., Cramer, K. L., and Lam, V. V. (eds) (2014). Status and Trends of Caribbean Coral Reefs: 1970-2012 Global Coral Reef Monitoring Network. Gland: IUCN.
Klastersky, J., and Zinner, S. H. (1982). Synergistic combinations of antibiotics in gram-negative bacillary infections. Reviews of Infectious Diseases 4, 294–301. doi: 10.1093/clinids/4.2.294
Lewis, J. B., and Price, W. S. (1975). Feeding mechanisms and feeding strategies of Atlantic reef corals. J Zoology 176, 527–544. doi: 10.1111/j.1469-7998.1975.tb03219.x
Manzello, D. P. (2015). Rapid recent warming of coral reefs in the Florida Keys. Scientific Reports 5, 1–10. doi: 10.1038/srep16762
Maynard, J., van Hooidonk, R., Eakin, C. M., Puotinen, M., Garren, M., Williams, G., et al. (2015). Projections of climate conditions that increase coral disease susceptibility and pathogen abundance and virulence. Nature Climate Change 5, 688–694. doi: 10.1038/nclimate2625
Maynard, J. A., Anthony, K. R. N., Harvell, C. D., Burgman, M. A., Beeden, R., Sweatman, H., et al. (2010). Predicting outbreaks of a climate-driven coral disease in the Great Barrier Reef. Coral Reefs 30, 485–495. doi: 10.1007/s00338-010-0708-0
McArdle, B., and Anderson, M. (2001). Fitting multivariate models to community data: a comment on distance-based redundancy analysis. Ecology 82, 290–297. doi: 10.1890/0012-9658(2001)082%5B0290:fmmtcd%5D2.0.co;2
Meyer, J. L., Castellanos-Gell, J., Aeby, G. S., Hase, C. C., Ushijima, B., and Paul, V. J. (2019). Microbial community shifts associated with the ongoing stony coral tissue loss disease outbreak on the Florida Reef Tract. Front. Microbiol. 10, 2244. doi: 10.3389/fmicb.2019.02244
Miller, M. W., Karazsia, J., Groves, C. E., Griffin, S., Moore, T., Wilber, P., et al. (2016). Detecting sedimentation impacts to coral reefs resulting from dredging the Port of Miami, Florida USA. PeerJ 4, e2711. doi: 10.7717/peerj.2711
Mullen, K. M., Peters, E. C., and Harvell, C. D. (2004). Coral Resistance to Disease, in: Rosenberg, E., Loya, Y. (Eds.), Coral Health and Disease. Berlin: Springer.
Mydlarz, L. D., McGinty, E. S., and Harvell, C. D. (2010). What are the physiological and immunological responses of coral to climate warming and disease? Journal of Experimental Biology 213, 934–945. doi: 10.1242/jeb.037580
Nugues, M. (2002). Impact of a coral disease outbreak on coral communities in St. Lucia: What and how much has been lost? Marine Ecology Progress Series 229, 61–71. doi: 10.3354/meps229061
Patterson, K. L., Porter, J. W., Ritchie, K. B., Polson, S. W., Mueller, E., Peters, E. C., et al. (2002). The etiology of white pox, a lethal disease of the Caribbean elkhorn coral, Acropora palmata. Proc. Natl. Acad. Sci. U.S.A. 99, 8725–8730. doi: 10.1073/pnas.092260099
Porter, J. W., Dustan, P., Jaap, W. C., Patterson, K. L., Kosmynin, V., Meier, O. W., et al. (2001). “Patterns of spread of coral disease in the Florida Keys,” in The Ecology and Etiology of Newly Emerging Marine Diseases, ed. J. W. Porter, (Dordrecht: Springer), 1–24. doi: 10.1007/978-94-017-3284-0-1
Precht, W. F., Gintert, B. E., Robbart, M. L., Fura, R., and van Woesik, R. (2016). Unprecedented disease-related coral mortality in southeastern Florida. Scientific Reports 6, 31374. doi: 10.1038/srep31374
Raymundo, L., Rosell, K., Reboton, C., and Kaczmarsky, L. (2005). Coral diseases on Philippine reefs: genus Porites is a dominant host. Dis. Aquat. Organ 64, 181–191. doi: 10.3354/dao064181
Raymundo, L. J., Halford, A. R., Maypa, A. P., and Kerr, A. M. (2009). Functionally diverse reef-fish communities ameliorate coral disease. PNAS 106, 17067–17070. doi: 10.1073/pnas.0900365106
Richardson, L. (2004). Black Band Disease, in: Rosenberg, E., Loya, Y. (Eds.), Coral Health and Disease. Berlin: Springer.
Richardson, L., Goldberg, W., Carlton, R., and Halas, J. (1998a). Coral disease outbreak in the Florida Keys: Plague Type II. Rev. Biol. Trop. 46, 187–198.
Richardson, L., Goldberg, W., Kuta, K., Aronson, R., Smith, G., Ritchie, K., et al. (1998b). Florida’s mystery coral-killer identified. Nature 392, 557–558. doi: 10.1038/33302
Riegl, B. M., Bruckner, A. W., Samimi-Namin, K., and Purkis, S. J. (2012). “Diseases, harmful algae blooms (HABs) and their effects on Gulf coral populations and communities,” in Coral Reefs of the Gulf, eds B. M. Riegl, and S. J. Purkis (Dordrecht: Springer), 107–125. doi: 10.1007/978-94-007-3008-3-7
Ritchie, K. B. (2006). Regulation of microbial populations by coral surface mucus and mucus-associated bacteria. Mar. Ecol. Prog. Ser. 322, 1–14. doi: 10.3354/meps322001
Santavy, D. L., Mueller, E., Peters, E. C., MacLaughlin, L., Porter, J. W., Patterson, K. L., et al. (2001). “Quantitative assessment of coral diseases in the Florida Keys: strategy and methodology,” in The Ecology and Etiology of Newly Emerging Marine Diseases, eds A. Porter, and W. James (Dordrecht: Springer), 39–52. doi: 10.1007/978-94-017-3284-0-3
Sato, Y., Bourne, D. G., and Willis, B. L. (2009). Dynamics of seasonal outbreaks of black band disease in an assemblage of Montipora species at Pelorus Island (Great Barrier Reef, Australia). Proceedings of the Royal Society B: Biological Sciences 276, 2795–2803. doi: 10.1098/rspb.2009.0481
Shore-Maggio, A., Runyon, C. M., Ushijima, B., Aeby, G. S., and Callahan, S. M. (2015). Differences in bacterial community structure in two color morphs of the Hawaiian reef coral Montipora capitata. Appl. Environ. Microbiol. 81, 7312–7318. doi: 10.1128/AEM.01935-15
Sokolow, S. H., Foley, P., Foley, J. E., Hastings, A., and Richardson, L. L. (2009). Disease dynamics in marine metapopulations: modelling infectious diseases on coral reefs. Journal of Applied Ecology 46, 621–631. doi: 10.1111/j.1365-2664.2009.01649.x
Stafford-Smith, M., and Ormond, R. (1992). Sediment-rejection mechanisms of 42 species of Australian scleractinian corals. Mar. Freshw. Res. 43, 683–705.
Sunagawa, S., DeSantis, T., Piceno, Y., Brodie, E., DeSalvo, M., Voolstra, C., et al. (2009). Bacterial diversity and white plague disease-associated community changes in the Caribbean coral Montastraea faveolata. Int. Soc. for Microbial Ecology 3, 512–521. doi: 10.1038/ismej.2008.131
Sussman, M., Loya, Y., Fine, M., and Rosenberg, E. (2003). The marine fireworm Hermodice carunculata is a winter reservoir and spring-summer vector for the coral-bleaching pathogen Vibrio shiloi. Environ. Microbiol. 5, 250–255. doi: 10.1046/j.1462-2920.2003.00424.x
Sussman, M., Mieog, J. C., Doyle, J., Victor, S., Willis, B. L., and Bourne, D. G. (2009). Vibrio zinc-metalloprotease causes photoinactivation of coral endosymbionts and coral tissue lesions. PLoS ONE 4:e4511. doi: 10.1371/journal.pone.0004511
Sussman, M., Willis, B. L., Victor, S., and Bourne, D. G. (2008). Coral Pathogens Identified for White Syndrome (WS) Epizootics in the Indo-Pacific. PLoS ONE 3:e2393. doi: 10.1371/journal.pone.0002393
Sutherland, K., Porter, J., and Torres, C. (2004). Disease and immunity in Caribbean and Indo-Pacific zooxanthellate corals. Marine Ecology Progress Series 266, 273–302. doi: 10.3354/meps266273
Sweet, M. J., Croquer, A., and Bythell, J. C. (2014). Experimental antibiotic treatment identifies potential pathogens of white band disease in the endangered Caribbean coral Acropora cervicornis. Proceedings of the Royal Society B: Biological Sciences 281, 20140094–20140094. doi: 10.1098/rspb.2014.0094
Ushijima, B., Smith, A., Aeby, G. S., and Callahan, S. M. (2012). Vibrio owensii induces the tissue loss disease Montipora white syndrome in the Hawaiian reef coral Montipora capitata. PLoS ONE 7:e46717. doi: 10.1371/journal.pone.0046717
Ushijima, B., Videau, P., Burger, A. H., Shore-Maggio, A., Runyon, C. M., Sudek, M., et al. (2014). Vibrio coralliilyticus strain OCN008 is an etiological agent of acute Montipora white syndrome. Appl. Environ. Microbiol. 80, 2102–2109. doi: 10.1128/AEM.03463-13
Ushijima, B., Videau, P., Poscablo, D., Stengel, J. W., Beurmann, S., Burger, A. H., et al. (2016). Mutation of the toxR or mshA genes from Vibrio coralliilyticus strain OCN014 reduces infection of the coral Acropora cytherea: Virulence genes in Vibrio coralliilyticus. Environ. Microbiol. 18, 4055–4067. doi: 10.1111/1462-2920.13428
Walton, C. J., Hayes, N. K., and Gilliam, D. S. (2018). Impacts of a Regional, Multi-Year, Multi-Species Coral Disease Outbreak in Southeast Florida. Frontiers in Marine Science 5:323. doi: 10.3389/fmars.2018.00323
Ward, J. R., and Lafferty, K. D. (2004). The Elusive Baseline of Marine Disease: Are Diseases in Ocean Ecosystems Increasing? PLoS Biology 2:e120. doi: 10.1371/journal.pbio.0020120
Williams, D., and Miller, M. (2005). Coral disease outbreak: pattern, prevalence and transmission in Acropora cervicornis. Marine Ecology Progress Series 301, 119–128. doi: 10.3354/meps301119
Williams, G. J., Knapp, I. S., Work, T. M., and Conklin, E. J. (2011). Outbreak of Acropora white syndrome following a mild bleaching event at Palmyra Atoll, Northern Line Islands, Central Pacific. Coral Reefs 30, 621. doi: 10.1007/s00338-011-0762-2
Willis, B. L., Page, C. A., and Dinsdale, E. A. (2004). “Coral disease on the Great Barrier Reef,” in Coral Health and Disease, eds E. Rosenberg, and Y. Loya (Berlin: Springer), 69–104. doi: 10.1007/978-3-662-06414-6-3
Keywords: Florida Reef Tract, coral disease, transmission, virulence, antibiotic diagnostics, acute lesions, subacute lesions, SCTLD
Citation: Aeby GS, Ushijima B, Campbell JE, Jones S, Williams GJ, Meyer JL, Häse C and Paul VJ (2019) Pathogenesis of a Tissue Loss Disease Affecting Multiple Species of Corals Along the Florida Reef Tract. Front. Mar. Sci. 6:678. doi: 10.3389/fmars.2019.00678
Received: 22 August 2019; Accepted: 18 October 2019;
Published: 01 November 2019.
Edited by:
Michael Sweet, University of Derby, United KingdomReviewed by:
Ernesto Weil, University of Puerto Rico at Mayagüez, Puerto RicoJennifer Moore, NOAA Fisheries Southeast Regional Office, United States
Erinn Muller, MOTE Marine Laboratory & Aquarium, United States
Copyright © 2019 Aeby, Ushijima, Campbell, Jones, Williams, Meyer, Häse and Paul. This is an open-access article distributed under the terms of the Creative Commons Attribution License (CC BY). The use, distribution or reproduction in other forums is permitted, provided the original author(s) and the copyright owner(s) are credited and that the original publication in this journal is cited, in accordance with accepted academic practice. No use, distribution or reproduction is permitted which does not comply with these terms.
*Correspondence: Greta S. Aeby, Z3JldGFAaGF3YWlpLmVkdQ==; Valerie J. Paul, cGF1bEBzaS5lZHU=
†Present address: Blake Ushijima, Smithsonian Marine Station, Fort Pierce, FL, United States; Justin E. Campbell, Department of Biological Sciences, Florida International University, Miami, FL, United States