- 1Laboratório de Ecologia e Cultivo de Fitoplâncton Marinho, Departamento de Oceanografia Biológica, Faculdade de Oceanografia, Universidade do Estado do Rio de Janeiro, Rio de Janeiro, Brazil
- 2Laboratório de Oceanografia Química, Departamento de Oceanografia Química, Faculdade de Oceanografia, Universidade do Estado do Rio de Janeiro, Rio de Janeiro, Brazil
- 3Brazilian Ocean Acidification Network, Rio Grande, Brazil
- 4Instituto de Biologia, Universidade Federal do Rio de Janeiro, Rio de Janeiro, Brazil
- 5Departamento de Ciências do Mar, Instituto do Mar, Universidade Federal de São Paulo, Santos, Brazil
Environmental gradients can provide habitat-specific scenarios for community functional diversity (FD) that determine the composition of populations on both spatial and temporal scales. The western shelf of the Antarctic Peninsula has experiencing increasing air temperatures while the climate is transitioning to a warm-humid sub-Antarctic-type of climate. As a consequence, abiotic changes are leading to alterations in the trophic web. Microphytoplankton FD was analyzed across environmental gradients of sea surface temperature, salinity, meltwater percentage and nutrient availability in Admiralty Bay, South Shetland Islands, Western Antarctic Peninsula. Samples were collected during the austral summer from 2009 to 2011 and from 2013 to 2015, at Admiralty Bay for which FD indices were calculated based on species traits. The amount of meltwater (MW) present in Admiralty Bay groups microphytoplankton into communities according to physiological and ecological tolerances, thus leading to a greater FD. When meltwater dominated the bay (>2.25% MW scenarios iii - 2013-14 and iv - 2014-15), diatoms and dinoflagellates were codominant. An increase in the dinoflagellate fraction of microplankton, notably with auxotrophic and mixotrophic nutrition mode, can be considered a trigger for changes in the structure of the Antarctic food web. Our results suggest using Admiralty Bay as a model for studies on changes in microphytoplankton community composition and FD.
Introduction
The climate of the western shelf of the Antarctic Peninsula (WAP) is undergoing a transition from a cold-dry polar-type climate to a warm-humid sub-Antarctic–type climate (Montes-Hugo et al., 2009). Air temperature in WAP has increased in relation to global variation (Abram et al., 2013), making this region one of the most rapidly warming on Earth over the last 50 years, with an increase of 0.56°C decade–1 over the years analyzed (Turner et al., 2005) and a 2°C increase in annual mean temperature (Ducklow et al., 2007). This regional increase has consequences for the dynamics of shelf and sea ice (Moline et al., 2004; Rozema et al., 2017; Aracena et al., 2018).
Since climate change has also consequences for the dynamics of chemical and physical properties of seawater, it can also induce changes in the phytoplankton community structure in WAP (Kopczyńska, 2008; Piquet et al., 2011). Abiotic factors are capable of constraining species establishment or permanence and establishing communities in certain sites (Kraft et al., 2015) by acting as environmental filters. This, in turn, can be reflected throughout all the trophic levels of the regional trophic web (Moline et al., 2004; Schofield et al., 2010; Lange et al., 2014; Saba et al., 2014). Climate change in WAP (e.g., increase in temperature and environmental variables) has driven modifications in phytoplankton size (Barrera-Alba et al., 2012, 2015; Lange et al., 2014; Vanzan et al., 2015), composition, biomass and community structure (Kopczyńska, 2008; Montes-Hugo et al., 2009; Piquet et al., 2011; Lange et al., 2014; Tenório et al., 2015; Mendes et al., 2018; Russo et al., 2018).
The use of classification schemes based on functional groups to determine the ecological dynamics of phytoplankton is a growing theme in environmental sciences (Kruk et al., 2002; Litchman et al., 2010; Moser et al., 2014; Roselli and Basset, 2015; Moser et al., 2017; Roselli et al., 2017). Such schemes can help explain the distribution of species along environmental gradients, as well as phytoplankton diversity and community structure (Litchman et al., 2010; Naselli-Flores et al., 2007). Associations are based on physiological, morphological and ecological attributes of species that potentially and alternatively can dominate or co-dominate the system. A reasonable hypothesis is that all species can potentially grow, but their establishment and development are conditioned by environmental variables and community responses, such as the ability to sustain processes of net population growth loss (Kruk et al., 2002, 2010). Morphological traits of species are essential properties that influence growth rate, resource use efficiency (e.g., nutrients, light) and susceptibility to herbivory under different environmental conditions. They can also predict the effects of climatic variability over time (Padisák et al., 2003; Salmaso, 2003). This means that species-specific strategic adaptations can be selectively favored in certain environments (Reynolds, 1980), since the cell morphology of phytoplankton and their adaptive strategies are under different selection pressures due to the fact that aquatic environments differ in many physical and chemical characteristics (Weithoff et al., 2014).
Traits of phytoplankton can be used to evaluate community functional diversity (FD) using a matrix in which community traits and species are combined and compared (Petchey and Gaston, 2007). Trait-based approaches aids to community ecology can help to explain species distribution along environmental gradients and changes in diversity and community structure (Litchman et al., 2010). Morpho-functional traits include physiological, morphological and phenological characteristics that drive the ecological functions of organisms (McGill et al., 2006; Violle et al., 2007) and can be used to evaluate FD since trait variation among phytoplankton species expresses FD (Vallina et al., 2017). FD has been identified as a key component of ecosystem functioning and sustainability (Mouillot et al., 2014). Furthermore, loss of biodiversity can be related to functional loss, since the extinction of a species or a community in a system can decrease its resilience or productivity (Mouchet et al., 2008). For all methods based on traits, a high number of diverging lineages reflects higher FD, while converging lineages represent the opposite (Martin, 2002). Morpho-functional trait diversity within communities responds to environmental parameters, which influence specific traits or trait groups, even during different periods (Verberk et al., 2013; Weithoff and Gaedke, 2016).
The present study focuses on microphytoplankton community structure and function during eight sampling campaigns of the austral summer over four different years in five regions of Admiralty Bay (AB), South Shetland Islands, Antarctica. Our goal was to perform a trait-based evaluation of changes to microphytoplankton communities by characterizing functional groups, FD and yearly variation throughout early and late summer in AB. We hypothesized that: (i) environmental gradients are the main drivers of temporal variation in microphytoplankton from year-to-year and from the early- to late-summer; and (ii) FD, characterized by functional evenness (FEve), functional richness, (FRic) and functional dispersion (FDis), varies under stressful environmental conditions. The results are expected to provide insight into patterns of microphytoplankton FD in a changing polar environment.
Materials and Methods
Study Area
Covering an area of 122 km2 and reaching approximately 500 m depth (Pruszak, 1980; Rakusa-Suszczewski, 1980), Admiralty Bay (AB) (Figure 1) is the largest bay of the South Shetland Islands. Water exchange with the adjacent Bransfield Strait is favored by wind-driven surface currents and tidal exchanges of deep-water masses (Pruszak, 1980). Winds can be an important factor driving the level of horizontal accumulation through advection in semi-enclosed bays like AB, since interactions between physical factors favors significant biomass accumulation in some cases (e.g., phytoplankton blooms in summer) (Schloss et al., 2014).
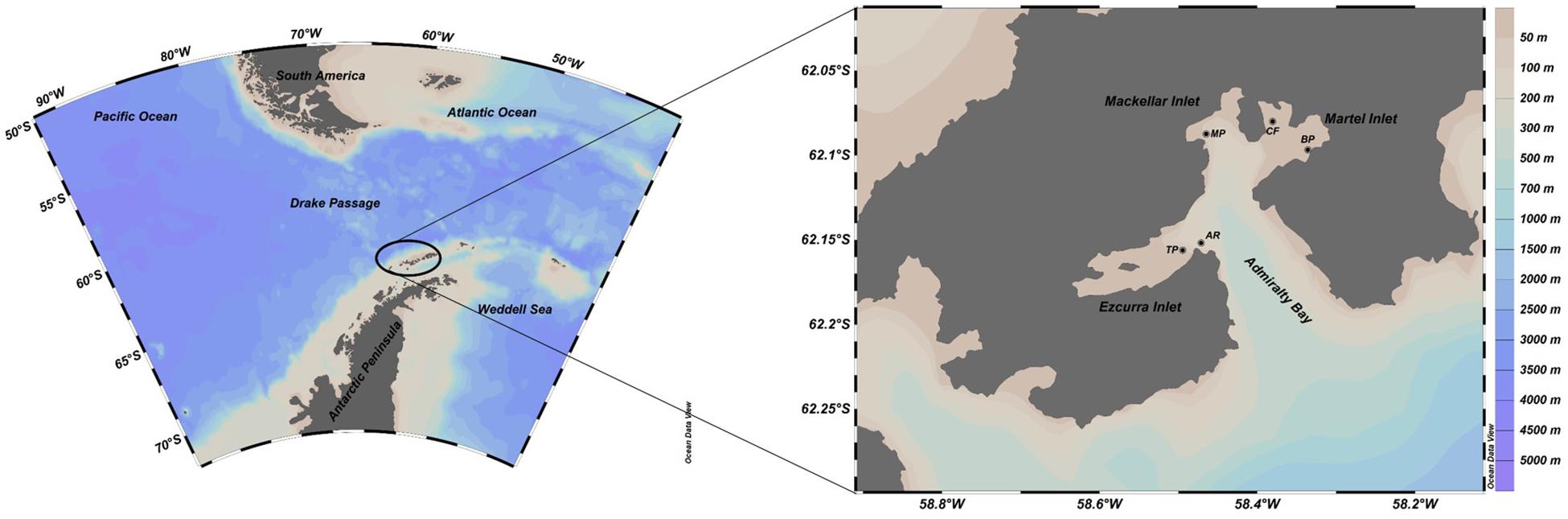
Figure 1. Study area in Admiralty Bay (enlargement) off the King George Island in South Shetland Islands, Antarctica. Black dots denote sampling stations. CF, Comandante Ferraz; BP, Botany Point; MP, Machu Picchu; TP, Thomas Point; AR, Arctowski.
Sampling Methods and Analysis
Admiralty Bay was surveyed during eight sampling periods: early and late summer 2009–2010, early and late summer 2010–2011, early and late summer 2013–2014 and early and late summer 2014–2015.
Sampling and data collection were conducted according to sampling period (I and II, early and late summer, respectively) at five stations: Comandante Ferraz (CF), Botany Point (BP), Machu Picchu (MP), Thomas Point (TP), and Arctowski (AR) (Figure 1) at three depths [surface (1 m), mid-water (15 m) and bottom (23–30 m)]. Vertical profiles of temperature and salinity were recorded with a Valeport® mini-CTD probe. Water samples were collected with 3-L Niskin bottles for laboratory analysis of phytoplankton biomass and composition and dissolved macronutrients.
To evaluate dissolved inorganic macronutrients, 0.7 μm aliquots of filtered water were preserved at −20°C until laboratory analysis according to the methods described at Aminot and Chaussepied (1983) and Grasshoff et al. (1983).
To evaluate possible impacts of sea ice melting on phytoplankton community structure, meltwater percentage (% MW) was calculated from the difference between salinity values at the surface (S surf) and the bottom (S btm), assuming 6 to be the average salinity value of marine ice (Ackley et al., 1979) (See Equation 1). To classify the environment according to %MW (Mendes et al., 2018), two scenarios were proposed: (i) stations under influence of melting sea ice (>2.25% MW); and (ii) stations not under the influence of melting sea ice (<2.25% MW).
Laboratory Methods for Phytoplankton
Chlorophyll a was determined by the spectrofluorometric method (Varian Cary Eclipse®) described in Neveux and Lantoine (1993) and modified by Tenório et al. (2005).
Phytoplankton classification and counts were performed on samples fixed in 2% formaldehyde solution following the Utermöhl method (Lund et al., 1958; Utermöhl, 1958; Rott, 1981). Phytoplankton were analyzed under an inverted microscope (Nikon® Eclipse TS 100) at 200× magnification. Phytoplankton taxa were classified according to keys edited by Hustedt (1930), Cupp (1943), Medlin and Priddle (1990), Round et al. (1990), Hasle and Syvertsen (1996), Tenenbaum et al. (2004), and Scott and Marchant (2005).
Functional traits of the most frequent and abundant taxa (criteria described in Lobo and Leighton, 1986) were selected considering the proposal of Litchman et al. (2010), microscopic observations and the literature. Functional traits used to evaluate the functionality of the phytoplankton community included morphological, physiological, behavioral and life-cycle traits. Morphological traits included greatest axial linear dimension (GALD), which is considered a master trait, and was obtained by measuring between 20 and 40 cells of each taxa with relative frequency above 10%. According to Litchman et al. (2010), classification of GALD as <35 μm and >35 μm reflects predation pressure. Other morphological traits (i) determined from microscopic analyses were surface to volume ratio (s/v), presence of large vacuoles, presence of silica structure (use of silica in the constitution of frustules and other structures), coloniality or chain formation, and presence of raphe. Physiological traits (ii) included the capacity for forming harmful algal bloom (HABs) (Moestrup et al., 2009) and nutrition mode – autotrophy, heterotrophy or mixotrophy were evaluated through the literature. The behavioral trait (iii) considered was mobility, which was determined by the presence of flagella during microscopic observation and a priori knowledge, while the life-cycle trait (iv) considered was resting stages, was also determined through the literature. For plastic traits, such as colony and chain formation, HAB formation capacity, mixotrophy and resting stages, trait values refer to a potential function, and not whether it was expressed in any particular community.
Data Analysis
Testing Environmental Patterns
Biotic and abiotic data were submitted to analysis of variation (ANOVA) and Tukey’s pairwise test (Software Paleontological Statistical 3 – PAST, Hammer et al., 2001). Comparisons were made among each survey year, early and late summer and sampling stations.
Calculating Functional Diversity Indices
Functional diversity indices aim to describe how much of the multifunctional space is filled and how the abundance of a community is distributed within this functional space. These indices have positive values, with higher values representing greater FD of the component they scale (Villéger et al., 2008).
Functional richness (FRic) represents a multidimensional measure of amount the of community-filled functional space and corresponds to the volume of a convex hull in the functional space (Cornwell et al., 2006; Villéger et al., 2008). There is no limit as it quantifies an absolute filled volume (Villéger et al., 2008). Functional equitativity (FEve) describes the evenness of the distribution of abundance in the space of functional traits, that is, it corresponds to a measure of regularity the space between species along the gradient of functional traits and regularity in the weighted distribution of their abundance. This measure decreases when abundance is less evenly distributed among species or when functional distances between species are not consistent. It is restricted to values between 0 and 1 (Villéger et al., 2008). FDis is the weighted mean, in the multidimensional space of traits, of the distance that each species is to the centroid of all species within a community. This index considers both traits and abundance of each species, where weights correspond to the relative abundance of each species, and there is no upper limit to its value (Laliberté and Legendre, 2010).
Functional groups were tested by applying a cluster analysis (Euclidian Distance combined with Unweighted Pair-Groups Method - UPGMA), to a data matrix (binary data: absence 0 and presence 1) (Supplementary Table 1) of selected traits described in Section “Microphytoplankton Response to Environmental Gradients.” Difference among groups were tested by applying ANOSIM to the resulting distance matrix, with p-values lower than 0.05 indicating significant differences. All these procedures were performed using the Vegan package of R software (version 3.5.1 – R Core Team, 2018) (Oksanen et al., 2017).
The functional traits matrix (binary data) and species abundances were used to evaluate community functional diversity (FD) in the resulting groups, using multidimensional indices based on Euclidian distance and non-parametric multidimensional scaling (NMDS) of traits to measure different aspects of diversity, FRic, FEve, and FDis. These metrics were calculated using dbFD function of FD package (Laliberté and Legendre, 2010) (R software, version 3.5.1 – R Core Team, 2018).
Relationships Between Species and Environment
To determine which environmental variables are correlated with phytoplankton assemblages, a Canonical Correspondence Analysis (CCA) was applied to log-transformed matrices of species abundance and environmental variables (temperature, salinity, nitrogen, phosphate, N:P ratio, silicate and chlorophyll a). Normality was tested by the Shapiro–Wilk test (Software Paleontological Statistical 3 – PAST, Hammer et al., 2001), while the significance of canonical axes was tested by a Monte Carlo permutation test.
Relationships Between Functional Indices and Environment
In order to correlate functional and environmental variables and assess which variables drive variation in FD, a Pearson Correlation Test was applied to a matrix containing the resulting indices and the environmental variables (temperature, salinity, meltwater, nitrogen, phosphate, N:P ratio, silicate and chlorophyll a) (Software Paleontological Statistical 3 – PAST, Hammer et al., 2001). Correlations with p values lower than 0.05 were considered significant.
Identification of Functional Groups
Functional groups were identified by considering species that grouped together in the following steps: cluster analysis, NMDS and CCA, as well as by analysis of functional indices.
Results
Environmental Patterns
Spatial Variation
The abiotic variables and chlorophyll a (Table 1) differed among sampling stations, although not significantly (ANOVA – Tukey Test p > 0.05), indicating that the spatial distribution of abiotic variables does not influence the response of the microphytoplankton community with regard to biomass (chlorophyll a).
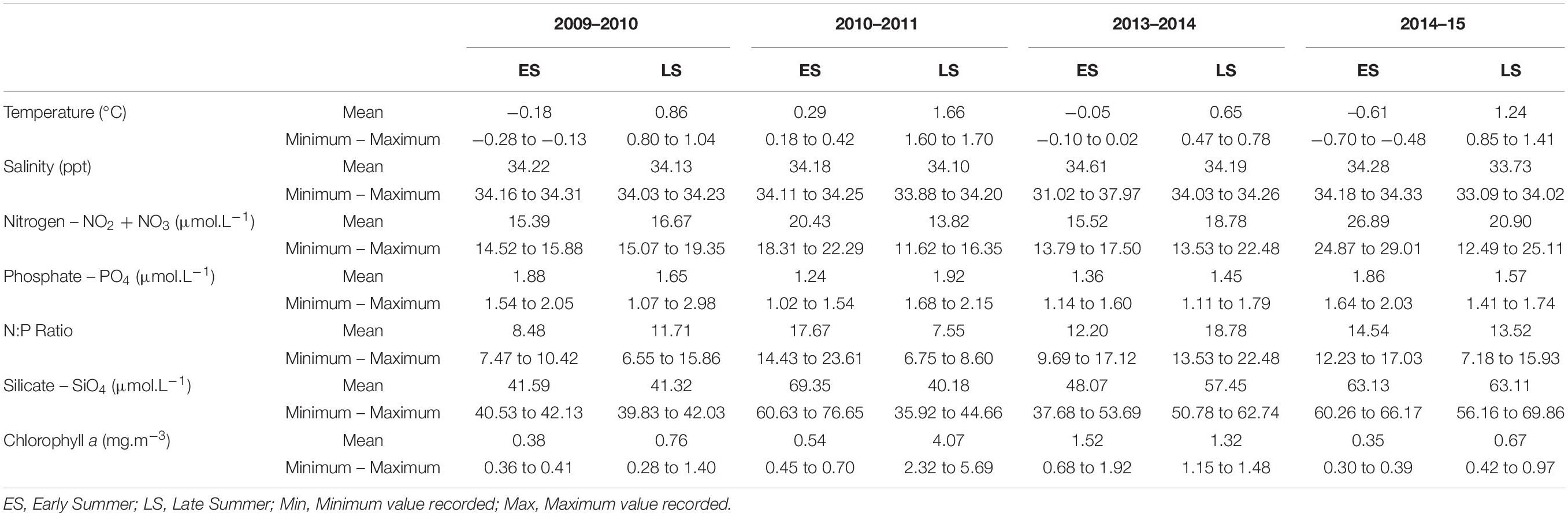
Table 1. Chemical and biological characteristics from Admiralty Bay (Mean, minimum and maximum values).
The sampling stations influenced by meltwater (% MW; Figure 2) were BP during early summer of 2010–2011 (2.66%), all stations during early summer in 2013–2014 (maximum of 20.73% for BP), and CF, MP and TP during late summer 2014–2015 (maximum of 10.08% for TP).
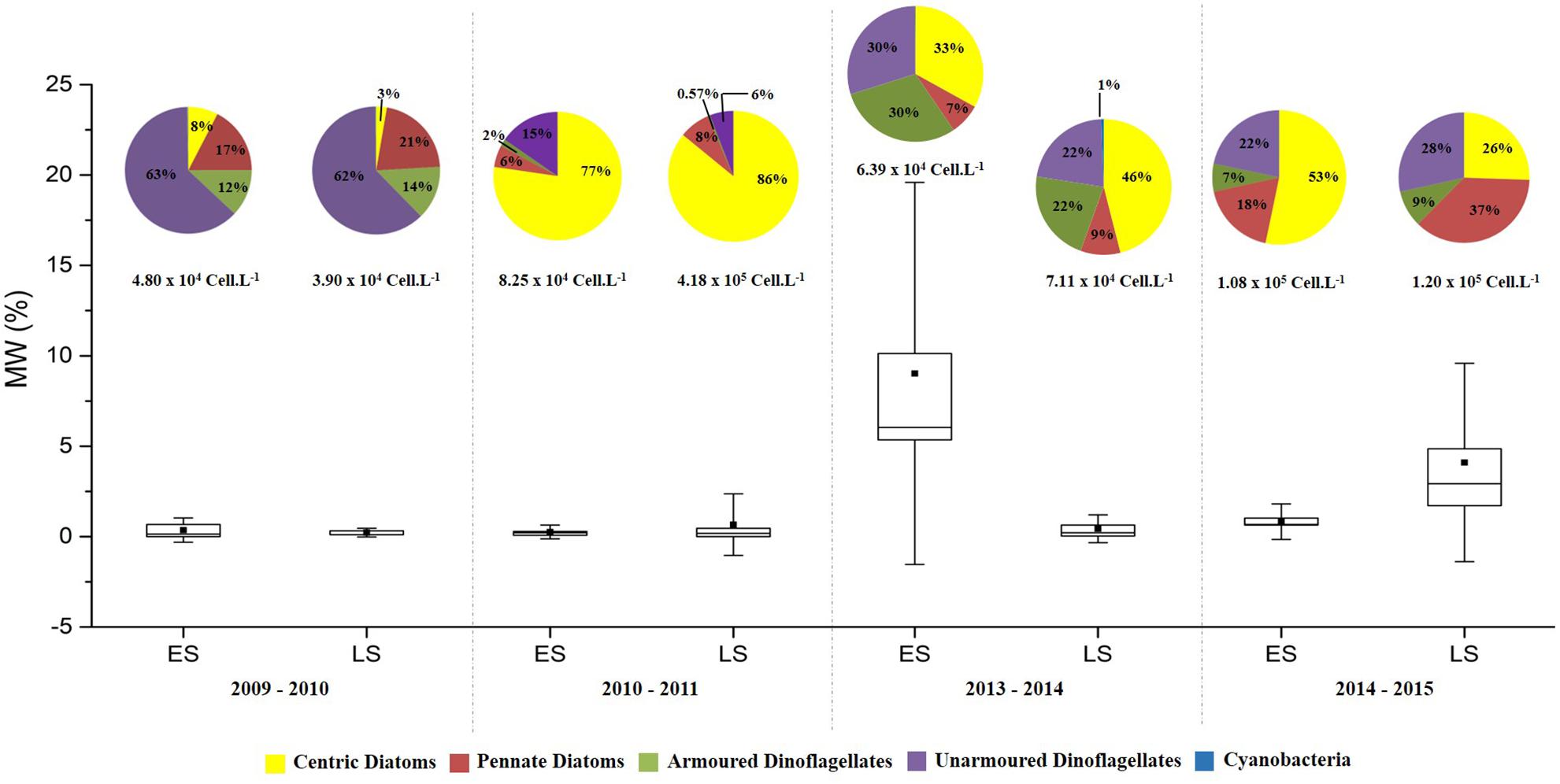
Figure 2. Contribution (%) of microphytoplanktonic groups, total abundance (Cell.L–1) and Meltwater Percentage – MW% (Standard deviation; Percentile 25,75; ■ Mean). Es, Early Summer; LS, Late Summer.
Temporal Variation
Temperature varied significantly among sampling periods (ANOVA – Tukey Test p < 0.05), with the highest averages being for 2010–2011 (1.66°C) and 2014–2015 (1.24°C), both at the end of summer, and the minimum averages being for 2009-2010 (−0.18°C) and 2014–2015 (−0.61°C) in early summer (Table 1).
The highest salinity was observed during early summer 2013–2014 (mean 34.61), while the lowest occurred during late summer, 2014–2015 (mean 33.73). However, salinity was the only variable that did not differ significantly (ANOVA – Tukey Test p > 0.05) among sampling periods.
Nitrogen compounds, phosphate and silicate varied widely (Table 1). The concentrations of dissolved nutrients were sufficient to support phytoplankton biomass for all the surveys, with the end of summer 2010–2011 having sufficient nitrate and silicate to support an abundance of large diatoms. The maximum concentrations of nitrogen compounds occurred during both early and late summer 2014–2015 (29.01 and 25.11 μmol.L–1, respectively; ANOVA – Tukey Test p < 0.05). High phosphate concentrations occurred throughout 2009–2010 (2.98 μmol.L–1) and 2010–2011 (2.15 μmol.L–1), the first years of sampling, both in late summer (ANOVA – Tukey Test p < 0.05). Regarding N:P ratio, high ratios (ANOVA – Tukey Test p < 0.05) were observed during early summer 2010–2011 (maximum of 23.61) and late summer 2013–2014 (maximum of 22.48) (Table 1). The highest concentrations for silicate (ANOVA – Tukey Test p < 0.05) occurred in early summer in 2010–2011 (76.65 μmol.L–1) and late summer 2014–2015 (69.86 μmol.L–1).
Chlorophyll a concentration varied between 0.28 and 5.69 mg.m–3 (ANOVA – Tukey Test p < 0.05), with higher concentrations being found in late summer 2010–2011 (mean 4.07 mg.m–3), followed by early summer 2013–2014 (mean of 1.52 mg.m–3) with a maximum of 1.92 mg.m–3 (Table 1).
The% MW (Figure 2 and Supplementary Table 2) indicated that the last 2 years of sampling (early summer 2013–2014 and late summer 2014–2015) were influenced by melting sea ice (p < 0.05). All stations had MW values > 2.25% in early summer 2013–2014 with a maximum of 20.73% in BP, along with low salinity (31.02) for the same station. The average temperature during this period was −0.05°C. During late summer 2014–2015, three points (CF, MP, and TP) had MW values > 2.25%, with a maximum of 10.08% in TP. During this period melting was associated with the lowest salinity values observed (varying from 33.09 to 34.02) and an average temperature of 1.24°C. It is worth mentioning that during late summer 2010–2011, BP had a MW value of 2.25%; however, because it was only a punctual record, the sampling was not classified as under the influence of melting sea ice.
Microphytoplankton Response to Environmental Gradients
The CCA highlighted the response of the microphytoplankton community to %MW and the environmental variables (Figure 3). Interannual variation was represented by four scenarios with different communities: (i) flagellates during 2009–10 and early summer 2010–2011; (ii) large diatoms during late summer 2010–2011; and two scenarios influenced by meltwater (>2.25% MW), both with co-dominance between dinoflagellates and diatoms: (iii) centric diatoms and armored dinoflagellates during 2013–2014; and (iv) pennate diatoms and unarmored dinoflagellates during 2014–2015.
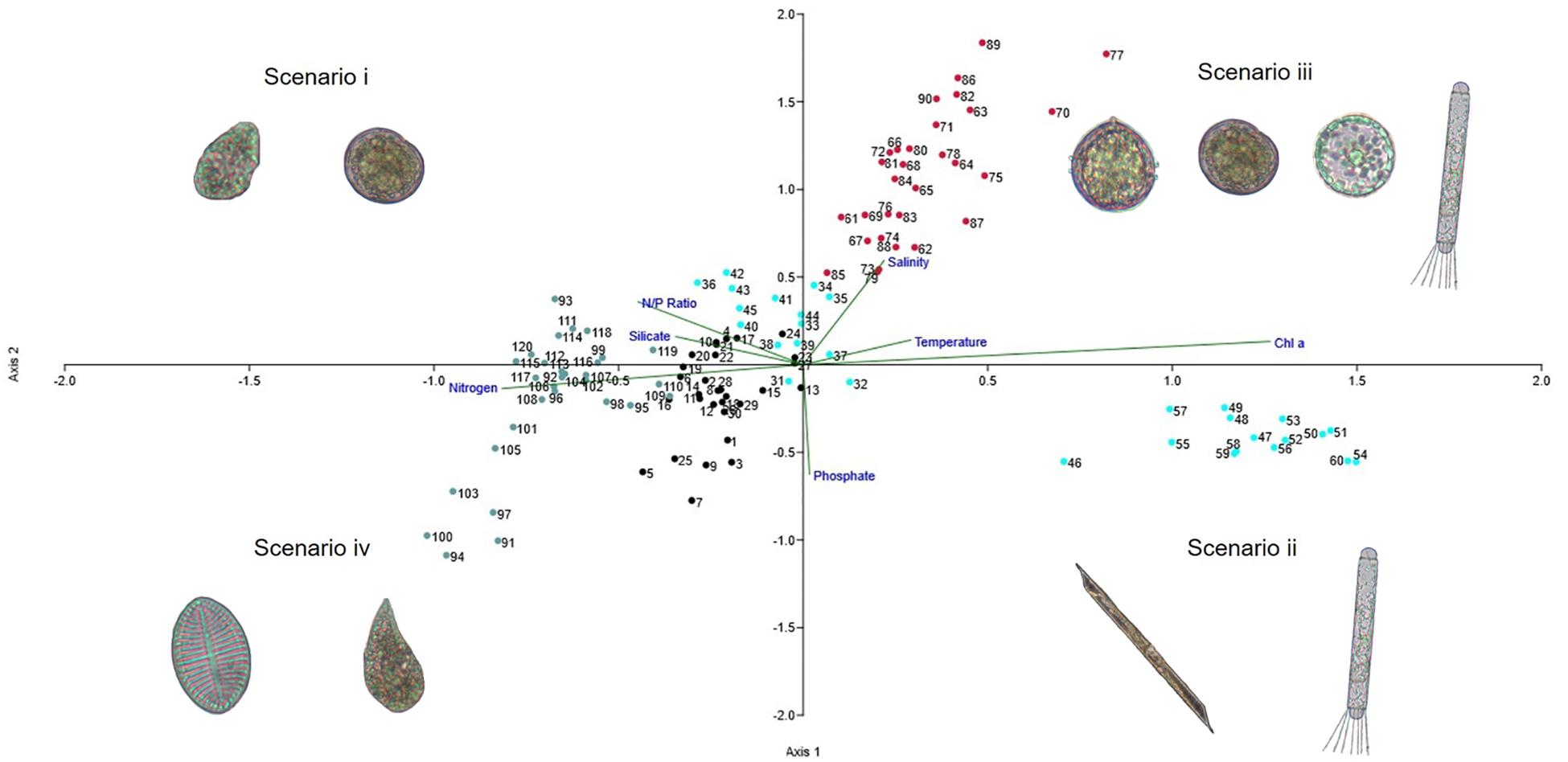
Figure 3. Canonical correlation analysis and abundant species representing the respective scenarios. Numbers and colors denote sampling stations and periods (black – 1 to 30: 2009–2010; blue – 31 to 60: 2010–2011; red – 61 to 90: 2013–2014; gray – 91 to 120: 2014–2015).
Axis 1 of the CCA explained 45% of environmental variation, with the availability of nitrogen compounds, N:P ratio and silicate being associated with scenario i. As well as with greater species richness with a predominance of dinoflagellates (5.80 × 104 cell.L–1; 76% of total cell numbers in 2009–2010), particularly Prorocentrum spp. (P. cf. compressum), Protoperidinium spp. (P. cf. defectum, P. cf. nanum), Amphidinium spp. (A. hadai), Gymnodinium spp. (G. soyai), and Gyrodinium spp. (G. glaciale) and diatoms (6.83 × 104 cell.L–1; 83% of total cell numbers in early summer 2010–2011), such as Corethron pennatum and Thalassiosira spp. Scenario ii, on the other hand, was characterized by decreases in the concentration of silicate and nitrogen concentrations and by the predominance of diatoms (3.59 × 105 cell.L–1; 86% of total cell numbers), with large organisms such as Coretrhon pennatum, Rhizosolenia spp. (R. hebetata, R. setigera) and Odontella spp. (O. litigious, O. weissiflogi) (Figure 2 and Supplementary Table 3).
Axis 2 explained 22% of environmental variation, with salinity, a variable related to melting sea ice, being associated with scenarios iii and iv, with differences in each context. In scenario iii, there was co-dominance between dinoflagellates (6.92 × 104 cell.L–1; 52%), represented mainly by species of the genus Protoperidinium spp. (P. curtum and P. cf. areolatum) and Prorocentrum spp., and centric diatoms (6.53 × 104 cell.L–1; 48%), mostly Corethron pennatum and Thalassiosira cf. antarctica. Scenario iv was characterized by the predominance of diatoms (1.52 × 105 cell.L–1 – 67%), with emphasis on pennate and raphid tychoplanktonic diatoms, such as Cocconeis spp. (C. costata and C. fasciolata), and a great contribution of unarmored dinoflagellates (5.74 × 104 cell.L–1; 25%), especially Gyrodinium spp. (G. glaciale and G. lachryma) and Gymnodinium spp. (G. soyai and G. guttula) (Figure 2 and Supplementary Table 3).
Functional Groups and Functional Diversity
Cluster analysis (Figure 4) revealed seven groups formed by organisms with distinct functional traits (ANOSIM: 0.734 – p < 0.05; cophenetic correlation coefficient: 0.897). Groups a and b were mainly comprised of dinoflagellates: group a mostly by unarmored dinoflagellates (Gyrodinium sp. and Gymnodinium sp.) and group b by armored dinoflagellates (Protoperidinium spp. and Prorocentrum spp.). Group c comprised filament-forming Cyanophyceae; group d raphid and chain-forming pennate diatoms (e.g., Pseudo-nitzschia spp.); groups e and f centric and pennate diatoms; and group g essentially large-sized pennate diatoms (Pseudogomphonema spp., Fragilariopsis spp. and Nitzschia spp.).
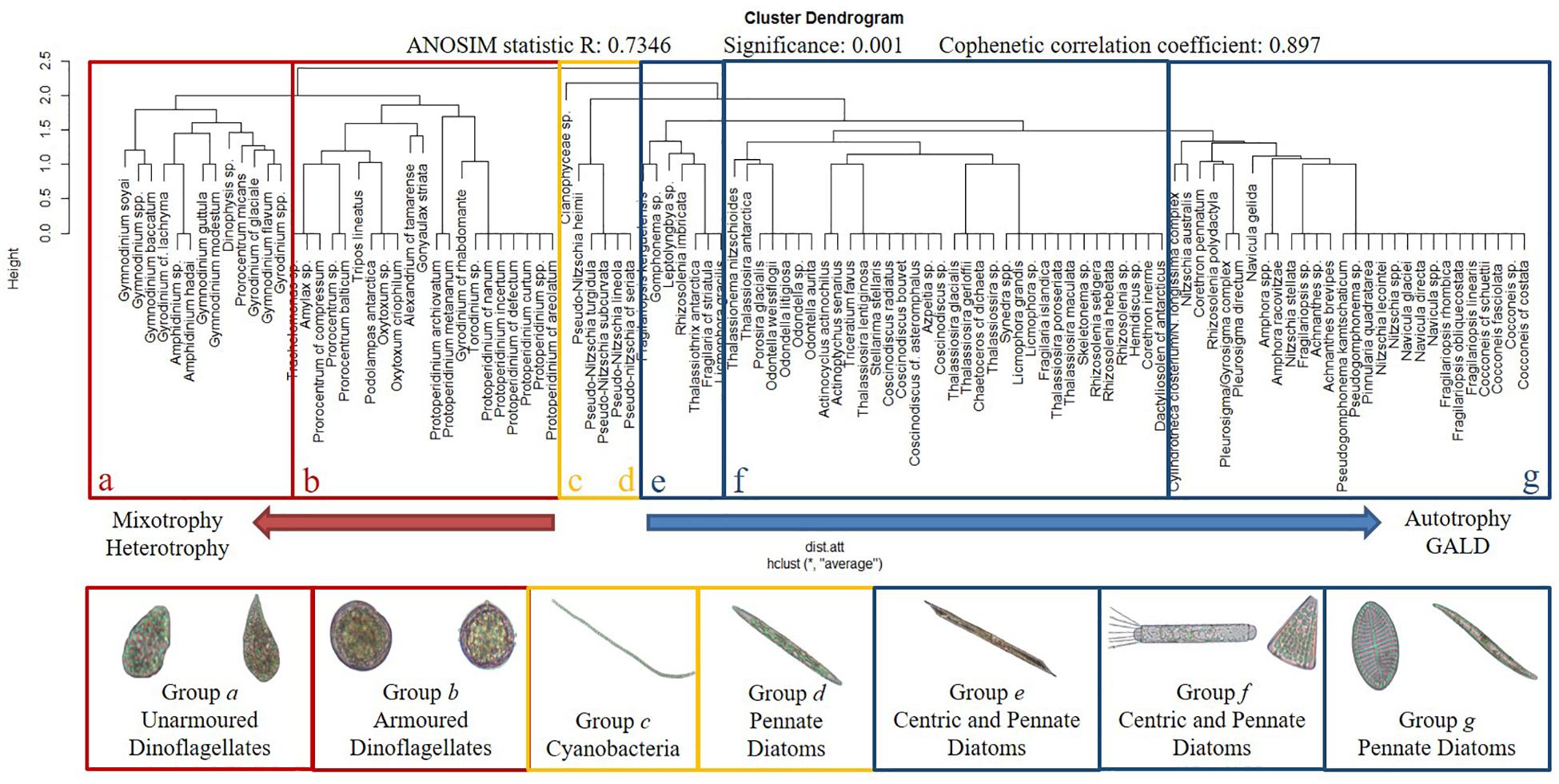
Figure 4. Dendrogram showing the functional groups formed by the presence or absence of the traits and species representing the respective groups. Letters and colors highlighted species groups.
Non-parametric multidimensional scaling (NMDS) (Figure 5) revealed relationships between species groups and three sets of functional traits. Groups a and b, both comprising dinoflagellates, were associated with set one: trophic traits (mixotrophy and heterotrophy), resting stage, vacuole presence, flagella presence, and potential for forming harmful algal bloom. Groups c and d were associated with set two: coloniality/chain formation, the presence of raphe and high s/v ratio. Groups e, f and g were associated with set three: GALD (>35 μm), autotrophy and the presence of silica.
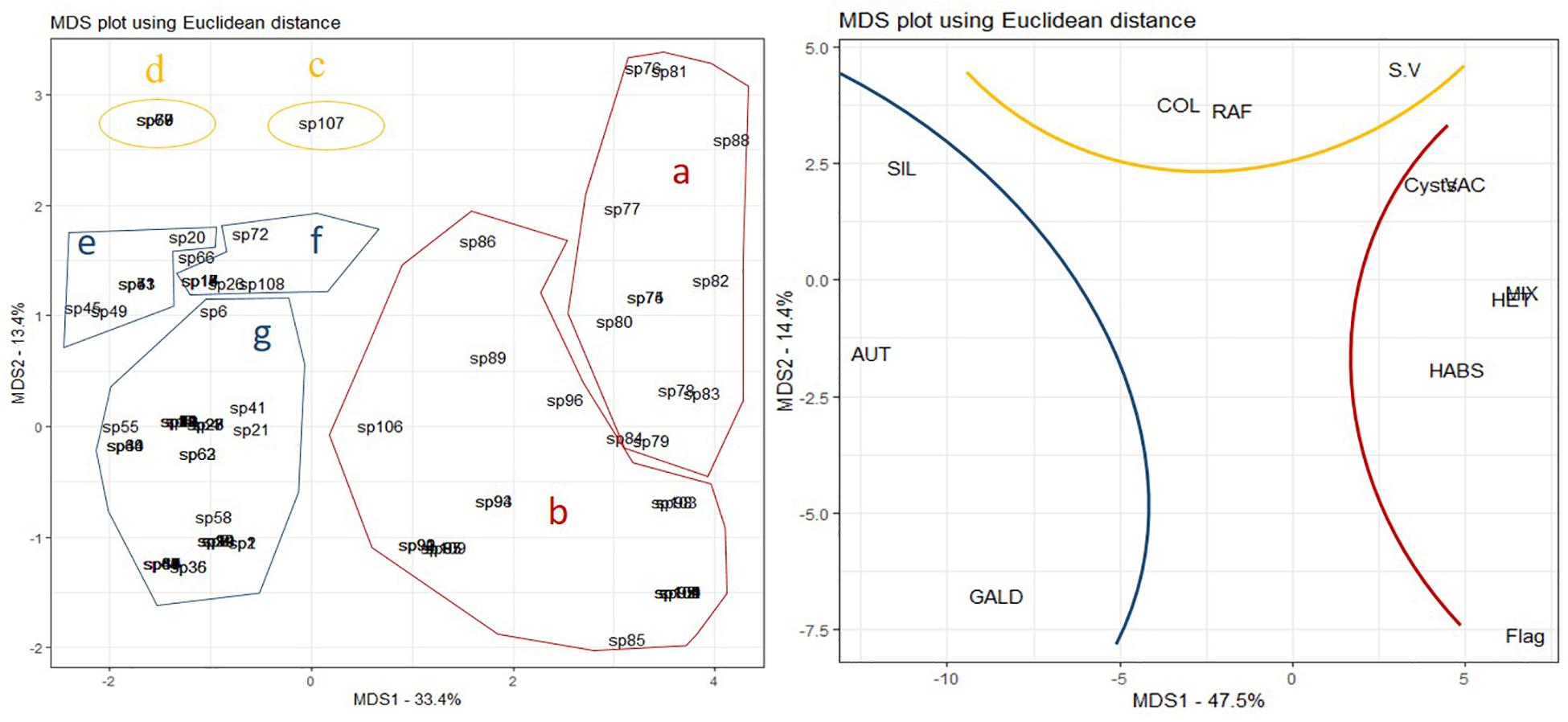
Figure 5. Non-Parametric Multidimensional Scaling (NMDS). Letters denote species groups and colors highlighted traits. GALD, greatest axial linear dimension; S.V, surface to volume ratio; VAC, presence of large vacuoles; SIL, presence of silica structure; COL, coloniality or chain formation; RAF, presence of raphe; HABs, Harmful algal bloom formation capacity; AUT, autotrophy; HET, heterotrophy; MIX, mixotrophy; CYST, resting stages; FLAG, presence of flagella.
These groups related to the three sets of traits (Figure 5) were also observed in the CCA analysis as correlated with environmental variables/scenarios (Figure 3): scenario i – groups a and b correlated with N:P and silica; group c and d correlated with phosphate; scenario iii – groups e and some species of groups f and b correlated with temperature and salinity; scenario ii groups g and some species of group f correlated with chlorophyll a; scenario iv – some species of group g and some species of group a. As revealed above, variation in salinity and the amount of meltwater favor the codominance of dinoflagellate and diatom species.
Species of groups a and b, c and d and e, f and g grouped together (95% of the species) in the Cluster, NMDS and CCA analyses, comprising different sets of traits, thus they were considered functional groups. A Pearson Correlation Test was performed to correlate environmental scenarios/functional groups and with FD indices (Supplementary Table 4).
Higher FEve was observed in scenarios iii and iv (Table 2). FDis ranged from 0.10 (scenario ii) to 0.28 (scenarios i, iii, and iv) while FRic was higher in scenario i. According to the values of these indices, scenarios iii and iv had high FD.
Pearson linear correlation revealed FDis to be negatively correlated with temperature (−0.999, p < 0.05) and chlorophyll a (−0.968, p < 0.05) and positively correlated with N/P ratio (0.940, p < 0.05) (Table 4 from Supplementary Material). According to the CCA analysis, scenario ii (without MW influence) shows the correlation between vectors for temperature and chlorophyll a on axis 1 and the highest relative abundance of diatom species (groups d to g); precisely in this scenario there is a reduction in FDis values (Table 2 - functional indices). The highest functional richness index (FRic) was for scenario i associated with the greater availability of nitrogen compounds and silicate, as revealed by the CCA (Figure 3). In fact, the NMDS revealed a lower number of traits associated with autotrophy (e.g., presence of silica and GALD > 35 μm), with the high relative abundance of species presenting these traits decreasing the distance to the centroid, thus decreasing values for FDis (Figure 5 – MDS).
Increases in nitrogen and N:P ratio favored dinoflagellates, as represented in scenario i in the negative portion of the CCA (Figure 3). It is noteworthy that although the trait mixotrophy had a higher weight for relative abundance, there was greater distribution and abundance weight for the other traits (presence of vacuoles, cysts, presence of flagella, HABs) in relation to the centroid and, thus, greater FDis. FEve was positively correlated (Pearson correlation) with MW (0.957, p < 0.05) and negatively (−0.905, p < 0.05) correlated with FRic. According to the CCA analysis, scenario iii (with the influence of MW) shows greater FEve, reflecting codominance of diatom and dinoflagellate species and, therefore, in the trophic traits of autotrophy and myxotrophy, respectively. These trophic functional traits had the highest scores on axis 1 of the NMDS. The relative abundance of species with autotrophy and myxotrophy was functionally more equitative in the scenarios under the influence of MW.
Functional richness was not significantly correlated with any environmental variable. Autotrophic and mixotrophic organisms occurred in all scenarios, and the area in the multidimensional space of traits was similar (Figure 5). However, there was a negative correlation between trait richness and FEve (−0.905, p < 0.05), due to the higher relative abundance associated with autotrophy or mixotrophy, as in scenarios i and ii (without the influence of MW).
Discussion
Environmental Patterns in Admiralty Bay in the Context of the Western Antarctic Peninsula
Hydrographic conditions in southern Ocean coastal systems are influenced by seasonal reductions in sea ice cover, the melting of glaciers and the breaking of ice shelves (Aracena et al., 2018). These coastal ecosystems are extremely sensitive to climatic variation due to the seasonality of ice (Ducklow et al., 2007); fluctuations in meltwater can affect biological production due to increased flow of freshwater in surface waters (Aracena et al., 2018; Kim et al., 2018). These conditions have become more prevalent due to the warming tendency in the region (Dierssen et al., 2002; Moline et al., 2004; Rozema et al., 2017). Over the last 30 years, the warming of water in the northern region of WAP, including the Bransfield Strait, has resulted in increased cloud cover and wind speed and reduced extent of ice cover (Montes-Hugo et al., 2009).
Seawater temperature has increased around King George Island (Clarke et al., 2005; Schloss et al., 2014), which supports the hypothesis of regional heating and potential threat to the local biota (Gutt et al., 2011). Considering the variation of environmental variables (e.g., temperature, salinity, ice cover) among the different areas of the Antarctic Peninsula, we subdivided WAP into 11 regions for comparison with the local environmental conditions of Admiralty Bay (Figure 6): Sector 1 – Admiralty Bay; Sector 2 – King George Island; Sector 3 – South Shetland Islands; Sector 4 – Bransfield Strait; Sector 5 – Weddell Sea; Sector 6 – Drake Passage; Sector 7 – Weddel-Scotia Confluence Zone; Sector 8 – Near James Ross Island; Sector 9 – Strait of Gerlache; Sector 10 – Marguerite Bay; and Sector 11 – Antarctic Peninsula.
Average temperature values above 1°C were observed in all WAP sectors (Table 3), especially sector 2, King George Island (Figure 6), with a minimum of –2.0°C and a maximum of 3.0°C (Lange et al., 2018), as observed in the present study (Table 1). Interannual differences were also observed, with higher temperatures at the end of the summer. Salinity exhibited little variation, with values higher than 34.0, except at the end of summer 2014–2015 when average values were lower, as observed in the literature both for AB (sector 1) and King George Island (sector 2) (Lange et al., 2018). Lange et al. (2014) observed higher salinities during the beginning than at the end of the summer, while Tenenbaum et al. (2010) reported reduced salinity for neritic regions, possibly due to the presence of glaciers in the region. In this context, Mendes et al. (2018) found lower values for salinity (mean 33.68) in areas near ice-covered regions and higher values (mean 34.14) in regions with little or no influence of ice cover seasonality for sector 7 (Weddel-Scotia Confluence Zone), thus correlating low salinity with water column stability. Additionally, Wasiłowska et al. (2015) associated low salinities (<34) with increased water column stability and a shallow (<2 m) mixture layer in sector 1. Similarly, Mendes et al. (2017) related high values of salinity with reduced water column stability and, consequently, the presence of a deeper mixing layer in sector 9 (Gerlache Strait).
Sampling under greater influence of meltwater occurred in early summer 2013–2014 (scenario iii) and late summer 2014–2015 (scenario iv), as also observed by Mendes et al. (2018) in Weddell-Scotia Confluence Zone in 2013 (sector 7). Despite the high water temperatures recorded in 2010–2011 (average 1.66°C during late summer) only one station, Botany Point, exhibited an influence from meltwater. Vanzan et al. (2015) suggested an increase in the effect of glacial melt in sector 1, near Botany Point, due to high water temperatures and low salinity values in surface waters during summer.
Another factor controlling the phytoplankton community is the availability of dissolved inorganic nutrients, which seems not to be limiting phytoplankton growth in WAP (this study, Lange et al., 2014; Schloss et al., 2014; Kim et al., 2016; Arrigo et al., 2017). In AB, temporal variation in inorganic macronutrients is correlated with biogeochemical and physical processes, such as terrigenous contribution, luminous incidence, marine and glacial melting water, influx of oceanic water masses and water column instability (Nedzarek and Rakusa-Suszczewski, 2004; Lange et al., 2007; Kim et al., 2016).
The temporal variation in average nutrient concentrations recorded in Admiralty Bay (present study) follows an increasing trend over recent years. The lowest N:P (<10) ratios were associated with higher phosphate concentrations in early summer (1.92 μmol.L–1), while higher ratios (N:P > 10) were associated with increased supply of nitrogenous compounds during late summer (maximum of 26.89 μmol.L–1) (Table 1). Lange et al. (2007) also documented differences in N:P ratios related to increased inorganic nitrogen and low phosphate concentrations at the end of the summer in the same sector. However, even with differences in the N:P ratio of 16:1 (Redfield, 1958), the elemental composition of phytoplankton was not constant due to the taxonomic variety (Falkowski, 2000; Geider and La Roche, 2002; Tenenbaum et al., 2015) that essentially occurs in coastal regions (Clarke et al., 2008; Henley et al., 2017; Kim et al., 2016). The same pattern of temporal increase was also observed for silicate concentrations, with high values related to a greater influence (%) of meltwater (scenarios iii and iv). Extensive variation in the concentration of dissolved nutrients was observed in the present study, as was reported in the literature for all sectors of WAP (Table 3). The lowest average concentrations of silicate (40.18 μmol.L–1) and nitrogen compounds (13.82 μmol.L–1), and lowest N:P ratio (7.55), were observed in association with higher diatom densities (94% relative abundance, chlorophyll concentration at 4.07 mg.m–3) during periods of low MW% (scenario ii). When lower macronutrient concentrations were observed in WAP, phytoplanktonic cell densities increased, revealing no limitation to the densities of autotrophic organisms.
In the last century, polar regions have exhibited a tendency for decreased chlorophyll a (Boyce et al., 2010). Marguerite Bay (sector 10) experienced a significant decrease in total biomass over the past 15 years (Rozema et al., 2017), due to increases in temperature (Boyce et al., 2010; Schloss et al., 2014). The chlorophyll a data of the present study follow this trend for some regions of WAP (Table 3), with values around 0.5–2.0 mg.m–3 (Lange et al., 2007; Wasiłowska et al., 2015). Exceptions were in cases of blooms (e.g., Wasiłowska et al., 2015 sector 1; Schloss et al., 2014 sector 2; Lange et al., 2018 sector 2; Aracena et al., 2018 sector 3; Table 3). Great variation was found for other sectors of WAP, including 4 (Bransfield Strait), 5 (Weddell Sea), 6 (Drake Passage) and 8 (James Ross Island), in association with water column stratification (e.g., seasonal heating of the sea water) (Mendes et al., 2012, 2018).
Since the life cycles of the main polar organisms are directly related to sea ice seasonality, the changes observed in WAP food webs are driven by increased water temperature and the consequent decline in ice cover (Moline et al., 2004; Montes-Hugo et al., 2009; Deppeler and Davidson, 2017; Rozema et al., 2017; and the present study). Changes in ice-free areas and micronutrient inputs generate environmental gradients of temperature, salinity and water column stability at WAP (Mendes et al., 2012), which lead to succession of the phytoplankton community, with consequences to the structure of the entire food web.
The Relationship Between Microphytoplankton Community Structure, Meltwater and Resuspension
Considering the observed abiotic environment and variation in phytoplanktonic biomass discussed in the previous section, WAP bays can be considered natural experiments for studying aquatic productivity in response to the effects of local climate and seasonal melting of sea ice, as also discussed by Aracena et al. (2018). Interannual changes in environmental conditions were observed in Admiralty Bay (sector 1). Furthermore, spatial and seasonal variation in meltwater percentage (Mendes et al., 2013; Arrigo et al., 2017; Mendes et al., 2018) lead to changes in both composition and density (103 cell.L–1 to 106 cell.L–1 – Table 3) of phytoplankton communities.
The present study documented a dominance of dinoflagellates or diatoms in the periods without influence from ice melt, represented by scenarios i and ii, respectively, and the codominance of these groups during periods with influence from ice melt (scenarios iii and iv). Such differences in taxonomic composition have been described for several sectors of the Antarctic Peninsula, such as Weddell-Scotia Confluence (sector 7 – Mendes et al., 2018) and Gerlache Strait (sector 9 – Mendes et al., 2017; Russo et al., 2018), but not for Admiralty Bay (sector 1). In AB (sector 1), the predominance of diatoms in the microphytoplankton community during summer is frequent (Lange et al., 2007; Kopczyńska, 2008; Barrera-Alba et al., 2012, 2015). In particular, the region is influenced by microphytobenthic species derived from the resuspension of sediments or the contribution of meltwater (Lange et al., 2007; Kopczyńska, 2008), as also observed in the present study. This dominance was also recorded in other sectors of WAP under the influence of sea ice melt (Schloss et al., 2014; Lange et al., 2018 - sector 2; Aracena et al., 2018 – sector 3; Mendes et al., 2012 – sectors 4, 5, 6, and 8; Rozema et al., 2017 – sector 10; Arrigo et al., 2017 – sector 11).
The decrease in cell size of microphytoplanktonic organisms in relation to hydrological conditions is noteworthy (e.g., Tenenbaum et al., 2010; Barrera-Alba et al., 2015), and associated with changes in dominance of taxonomic groups of phytoplankton from diatoms to dinoflagellates (Tenenbaum et al., 2010; Lange et al., 2014). This was seen in scenarios iii and iv, periods under the influence of meltwater, when there was codominance between diatoms (e.g., Corethron pennatum, Thalassiosira cf. antarctica, Cocconeis spp.) and dinoflagellates (Prorocentrum spp., Protoperidinium spp., Amphidinium spp., Gyrodinium spp., Gymnodinium spp.). The high species richness, especially for the diatom both planktonic and benthic, contribution, observed in sector 1 (Lange et al., 2018 – review; this study), has previously been recorded in the water column and correlated with the resuspension and/or sea ice melting hypothesis (Tenenbaum et al., 2011).
According to Lange et al. (2007), alternation in dominance of centric and pennate diatoms suggests an influence from temporal variation in sea ice cover and phytoplankton communities. In this sense, the remarkable contribution of benthic diatoms in scenarios iii and iv, periods under the influence of meltwater, represented mainly by Cocconeis spp. (C. costata and C. fasciolata), eponymous diatoms such as Fragilariopsis spp. (e.g., F. rhombica), and chain-forming diatoms, mainly Pseudo-nitzschia spp. (e.g., Pseudo-nitzschia subcurvata), reinforce the influence that sea ice melting has on the microphytoplankton community of AB. Ticopelagic diatoms in the water column can be associated with advection processes of water masses driven by continental ice melting (Pichlmaier et al., 2004) and/or coastal upwelling events generate by winds (Schloss et al., 2002; Schloss et al., 2014). Otherwise, centric diatoms (Thalassiosira spp. and Corethron pennatum) observed in scenario ii, without sea ice melt, were possibly transported by currents from Bransfield strait (Kopczyńska, 2008; Wasiłowska et al., 2015).
It should be noted that although the phytoplankton community and events of Antarctic blooms are commonly dominated by diatoms, other taxa are also important components of the trophic web (Henley et al., 2019). Increases in cryptophytes and other flagellates have previously been reported (e.g., Phaeocystis antarctica and Gymnodiniales), as well as the substitution of diatoms for these flagellates in WAP, due to the influence of sea ice melt (Moline et al., 2004; Mendes et al., 2013; Lange et al., 2014; Mendes et al., 2017), as observed in the WAP sectors of the present study (Figure 3): sector 1 (Wasiłowska et al., 2015), sector 4 (Mendes et al., 2012, 2013), oceanic regions of sectors 5 (Mar de Weddell) and 6 (Passagem de Drake) (Mendes et al., 2012), as well as sectors 7 (Mendes et al., 2018), 9 (Gerlache Strait) (Mendes et al., 2017; Russo et al., 2018) and 11 (Arrigo et al., 2017).
Another important consequence of sea ice melting is an increase in turbidity due to suspension of bottom sediments (Pichlmaier et al., 2004). Thus, conditions of high turbidity may have favored the increase in dinoflagellates, and consequently the codominance observed in the scenarios under the influence of meltwater, since the mixotrophy of the majority of these organisms allow them to grow in low light conditions.
Since phytoplankton support the marine trophic web and play a key role in the resilience of marine ecosystems of the Antarctic Peninsula, changes in the abundance and composition of phytoplankton groups can affect interactions in trophic levels and generate a direct effect throughout the regional ecosystem (Haberman et al., 2003; Mendes et al., 2013; Schofield et al., 2017; Mendes et al., 2018). In this context, Antartic krill (e.g., Euphausia superba), which are an important link in the trophic web of the region (Corbisier et al., 2004; Saba et al., 2014), assimilate diatoms more efficiently than cryptophytes (Haberman et al., 2003) and dinoflagellates, with benthic diatoms accounting for 46% of their diet in coastal zones, as well as in Admiralty Bay, sector 1 (Ligowski, 2000).
Small microalgae, such as cryptophytes and other flagellates, including dinoflagellates, are preferred by salps and other suspensivores, as they are not palatable to herbivores such as krill (Moline et al., 2004; Montes-Hugo et al., 2009). In addition, these nannoplanktonic microalgae are generally less efficient in the assimilation and exportation of carbon, when compared to a community dominated by diatoms (Schloss et al., 2007; Weston et al., 2013; Schofield et al., 2017; Da Cunha et al., 2018). Therefore, a decrease in the size of phytoplankton can cause a significant change in the local trophic web and in biogeochemical cycles (Rozema et al., 2017; Aracena et al., 2018; Da Cunha et al., 2018). It is possible that changes in the phytoplankton community and the seasonality of krill lead to population decreases in penguins (Sander et al., 2007), changes in the benthic community (Montes-Hugo et al., 2009) and variation in the period and frequency of migratory movements of humpback whales (Johnston et al., 2012; Andrews-Goff et al., 2018).
The observed increase in the dinoflagellate contribution (e.g., Gymnodinium spp., Protoperidinium spp. and Prorocentrum spp.) (Kopczyńska, 2008; Tenenbaum et al., 2010; Lange et al., 2014, 2018; this study) indicates a change in phytoplankton species composition (Lange et al., 2018). This supports the findings of other studies in AB (Sector 1) and King George Island (sector 2), that highlighted shifts in biota due to environmental changes (increase in solar radiation and temperature, thus increasing the sea ice melting) (Kopczyńska, 2008; Schloss et al., 2012; Campos et al., 2013; Mendes et al., 2013, 2018; Saba et al., 2014; Schofield et al., 2017). These observations were not restricted to coastal areas in WAP. Changes in phytoplankton and the consequences to the trophic web in oceanic areas (Bransfield Strait – sector 4; Drake Passage – sector 6) were previously reported in the literature (Kopczyńska, 2008; Mendes et al., 2012; Lange et al., 2014).
Sea Ice Melt Drives Changes in Microphytoplankton Functional Groups and Functional Diversity
Cell morphology and adaptive strategies of phytoplankton are under different selective pressures due to the physico-chemical environmental characteristics to which they are subjected (Litchman et al., 2009; Weithoff et al., 2014). The influence of sea ice melting in WAP promoted environmental gradients, which can function as filters and restrict diversity, or create niches and promote competition among species, through selecting traits (Lebrija-Trejos et al., 2010; Roselli and Basset, 2015; Weithoff and Gaedke, 2016). Three sets of functional traits were classified in the present study, which varied from scenario i to scenario iv along an environmental gradient of % MW.
The first set of functional traits, composed of dinoflagellates (groups a and b), was selected by trophic traits (heterotrophy and mixotrophy); resistance stages; presence of vacuoles and flagella; and potential HABs formation. Heterotrophy and mixotrophy confer advantages to dinoflagellates under conditions of low nutrient concentrations (Litchman and Klausmeier, 2008) and, associated with the motility of these organisms, represent unique strategies for competing in stratified environments (Bellinger and Sigee, 2011; Klais et al., 2016; Roselli et al., 2017). When compared to heterotrophic competitors, mixotrophy confers the ability to compete and overcome both strictly autotrophic and heterotrophic organisms by using nutrients more efficiently (Leles et al., 2018). In addition, the presence of vacuoles, associated mainly with mixotrophic dinoflagellates, may be indicative of a digestive function for these organelles and the consequent occurrence of phagotrophy by these organisms (Onuma and Horiguchi, 2013; Almada et al., 2017). Another selected trait was the production of resistance stages, which is a survival strategy among dinoflagellates that favors the reintroduction of species into the water column and eventually HAB formation (McQuoid et al., 2002; Ellegaard and Ribeiro, 2018).
It is worth noting that no HAB events were reported for the studied region; however, HAB forming algae exhibit trait plasticity, which may favor the development of these species in different environmental conditions (Litchman et al., 2010). Most harmful events reduce diversity and threaten the fitness of local ecosystems; such effects can be intensified under a climate change scenario (Paerl and Huisman, 2009).
The second set of traits was composed of filamentous cyanobacteria (group c) and pennate diatoms with raphe (group d). Coloniality (trait that includes a group of cells forming colonies, filaments and chains) was the trait that characterized these groups. Coloniality in both cyanobacteria and diatoms confers ecological advantages to these organisms because it modifies their effective size by altering their interaction with the environment and possible predators (Thingstad et al., 2005; Barton et al., 2013), reduces losses by processes of sedimentation (Brasil and Huszar, 2011), and regulates buoyancy allowing migration to depths of optimal irradiance and/or nutrient availability (Brookes and Ganf, 2001; Tamulonis et al., 2011). Besides coloniality, diatoms of group d also possess higher s/v ratios and the presence of raphe. An additional function of diatom chains is to keep cells together in order to prevent dispersion in turbulent environments (Crawford and Sims, 2008; Wadt et al., 2017), such as the case with Pseudo-nitzschia spp., a dominant genus of group d. Since higher s/v ratios allow nutrients to be absorbed more effectively, they favor diatoms in light-limiting conditions (Roselli et al., 2017).
The third set of traits mostly combined centric large-sized diatoms (groups e, f, and g) selected by autotrophy, GALD and silica presence. The observed diatoms are strictly autotrophic and characterized in the literature as having high photosynthetic efficiency (e.g., Roberts et al., 2007), which confers a competitive advantage in environments with high light and nutrient availability (Litchman et al., 2009). Large-sized cells (e.g., GALD > 35 μm; Litchman et al., 2010), such as those observed in this set, can store nutrients, even in conditions of high availability or in environments with fluctuations in the availability of this resource (Thingstad et al., 2005; Litchman et al., 2009). Another advantage of large diatoms (GALD > 35 μm) is their reduced palatability (Kruk and Segura, 2012), which is reflected in high predation pressure on small sized fractions (<35 μm) (Litchman et al., 2010). Additionally, the presence of silica in their constitution is one of the main traits of this group, while the presence of ornaments and processes on the frustule also confer advantages against certain types of predation (Hamm et al., 2003; Barton et al., 2013).
This diversity of traits discussed above translated into the higher FD indices observed in the periods influenced by meltwater, namely scenarios iii and iv, in which the codominance between diatoms and dinoflagellates was recorded. The high contribution of sea ice melt in 2013–2014 and 2014–2015 (scenarios iii and iv) can be considered an intermediate disturbance, which allowed the coexistence of several morphotypes with different traits and/or strategies, thereby potentializing functional niche diversity (Weithoff et al., 2014). Competition for resources may be a key process for shaping phytoplankton community structure by characterizing and clustering organisms in their niche, and thus directing ecosystem functioning, resulting in species coexistence (Mayfield and Levine, 2010; Segura et al., 2013). It is probable that competition among functional groups selected species/types that differed most from each other in the use of resources, resulting in the codominance observed during periods of meltwater, scenarios iii and iv, since competition entails ecological differentiation of coexisting species (Cornwell et al., 2006). Abiotic factors of scenarios iii and iv, associated with % MW, did not act as environmental filters because the organisms exhibited tolerance to this disturbance, which was reflected in greater diversity of the phytoplanktonic community.
The greatest FRic with no influence of meltwater was observed in early summer 2009–2010 and 2010–2011 (scenario i), and was not accompanied by greater expression of FD in the analyzed indices. An increase in taxonomic diversity and lower values of FD may indicate that, although richness is greater, the functional traits that allowed the species to be successful were selected and the community is composed of only functionally similar species, since the taxonomic diversity does not always reflect FD (Weithoff et al., 2014). Thus, FD is lower because different phytoplanktonic species can be functionally neutral (Roselli et al., 2017), that is ecologically equivalent, and so there is no distribution in trait based factorial plan (Vallina et al., 2017), which is evidenced by the dominance of dinoflagellates in this scenario.
While scenarios iii and iv (2013–2014, 2014–2015), characterized by high %MW, had lower FRic than scenario i (2009–2010, 2010–20110), they had high trait diversity, with emphasis on functional equitability (e.g., Villéger et al., 2008; Cardoso et al., 2017). It is likely that the use of contrasting ecological strategies, directed by biotic and abiotic processes as a trade-off between form and size, key features for the selection and interaction of organisms, allowed the coexistence of species (Roselli et al., 2017). This in turn determined changes in the dynamics and functioning of phytoplankton communities (Pomati et al., 2013), and was expressed in the highest values of FD among the scenarios influenced by meltwater.
Conclusion
The hydrographic conditions, as well as the nutrient availability and variation in phytoplankton biomass, observed in this study resemble the gradients and variation reported in the literature for WAP at various temporal scales. The influence of meltwater associated with increasing water temperatures has been highlighted by recent studies as the main environmental stressor for microphytoplankton biomass. The functional groups of the present study reflect different adaptive strategies produced by the divergence of traits. These different adaptive strategies are responsible for the codominance of diatoms and dinoflagellates and the structuring of the microphytoplankton community and FD in scenarios dominated by meltwater. FD increased in relation to a gradient of %MW from summer 2009–2010 to summer 2014–2015. The increased contribution of dinoflagellates in scenarios of meltwater may be a trigger for changes in the structure of the Antarctic trophic web reported in literature. In this sense, we suggest that Admiralty Bay be used as a model for studies on changes to microphytoplankton community composition and FD, since they are relevant descriptors for evaluating potential changes to the local ecosystem at different scales.
Data Availability Statement
All datasets generated for this study are included in the manuscript/Supplementary Files.
Author Contributions
GM, JB-A, and DL conceived the experimental design. DT and JB-A organized the database. DL, MC, MT, and TC performed the sample analysis. DL, GM, FP, and LD performed the statistical analysis and wrote the first draft of the manuscript. All authors contributed to the manuscript revision, read and approved the submitted version.
Funding
This work integrates the National Institute of Science and Technology Antarctic Environmental Research (INCT-APA), which receives scientific and financial support from the National Council for Research and Development (CNPq Grant No. 574018/2008-5) and the Research Support Foundation of the State of Rio de Janeiro (FAPERJ Grant No. E-16/170.023/2008).
Conflict of Interest
The authors declare that the research was conducted in the absence of any commercial or financial relationships that could be construed as a potential conflict of interest.
Acknowledgments
The authors acknowledge the support of the Brazilian Ministries of Science, Technology and Innovation (MCTI), of Environment (MMA), Inter-Ministry Commission for Sea Resources (CIRM), and the National Institute of Science and Technology Antarctic Environmental Research (INCT-APA). The authors would like to thank the Marine Organic Chemical Laboratory of the Oceanographic Institute of São Paulo University (LabQOM-IOUSP) for physical (2009 – 2011 period) and chemical analysis (2009 – 2011 and 2013 – 2014 periods) and Ph.D. Ricardo C. G. Pollery of the Biology Institute of Federal University of Rio de Janeiro (UFRJ) for chemical analysis (2014 – 2015 period).
Supplementary Material
The Supplementary Material for this article can be found online at: https://www.frontiersin.org/articles/10.3389/fmars.2019.00638/full#supplementary-material
References
Abram, N. J., Mulvaney, R., Wolff, E. W., Triest, J., Kipfstuhl, S., Trusel, D., et al. (2013). Acceleration of snow melt in an Antarctic Peninsula ice core during the twentieth century. Nat. Geosci. 6, 404–411. doi: 10.1038/NGEO1787
Ackley, S. F., Buck, K. R., and Taguchi, S. (1979). Standing crop of algae in the sea ice of the Weddell Sea region. Deep Sea Res. 26A, 269–281. doi: 10.1016/0198-0149(79)90024-4
Almada, E. V. C., Carvalho, W. F. C., and Nascimento, S. M. (2017). Investigation of phagotrophy in natural assemblages of the benthic dinoflagellates Ostreopsis, Prorocentrum and Coolia. Braz. J. Oceanogr. 65, 392–399. doi: 10.1590/s1679-87592017140706503
Aminot, A., and Chaussepied, M. (1983). Manuel Des Analyses Chimiques en Milieu Marin. Brest: C.N.E.X.O., 395.
Andrews-Goff, V., Bestley, S., Gales, N. J., Laverick, S. M., Paton, D., Polanowski, A. M., et al. (2018). Humpback whale migrations to Antarctic summer foraging grounds through the southwest Pacific Ocean. Sci. Rep. 8, 1–14. doi: 10.1038/s41598-018-30748-4
Aracena, C., González, H. E., Garcés-Vargas, J., Lange, C. B., Pantoja, S., Muñoz, F., et al. (2018). Influence of summer conditions on surface water properties and phytoplankton productivity in embayments of the South Shetland Islands. Polar Biol. 41, 2157–2158. doi: 10.1007/s00300-018-2338-x
Arrigo, K. R., van Dijken, G. L., Alderkamp, A. C., Erickson, Z. K., Lewis, K. M., Lowry, K. E., et al. (2017). Early spring phytoplankton dynamics in the western Antarctic Peninsula. J. Geophys. Res. 12, 1–20. doi: 10.1002/2017JC013281
Barrera-Alba, J. J., Tenenbaum, D. R., Vanzan, M., and Tenório, M. M. B. (2015). Composition, density and size-structure of the autotrophic plankton community in a shallow coastal zone at King George Island, West Antarctic Peninsula (WAP), during early summer 2010. Braz. J. Aquat. Sci. Technol. 19, 9–17. doi: 10.14210/bjast.v19n3.4731
Barrera-Alba, J. J., Vanzan, M., Tenório, M. M. B., and Tenenbaum, D. R. (2012). “Plankton structure of shallow coastal zone at admiralty bay, king george island, west antarctic peninsula (wap) during early summer/2010: pico, ultra and microplankton and chlorophyll biomass,” in Annual Activity Report of National Institute of Science and Technology Antarctic Environmental Research (INCT-APA) - 2011, eds Y. Y. Valentin, A. G. Dalto, and H. P. Lavrado, (São Carlos: Cubo), 109–114. doi: 10.4322/apa.2014.071
Barton, A. D., Pershing, A. D., Litchman, E., Record, N. R., Edwards, K. F., Finkel, Z. V., et al. (2013). The biogeography of marine plankton traits. Ecol. Lett. 16, 522–534. doi: 10.1111/ele.12063
Bellinger, E. G., and Sigee, D. C. (2011). “Dinoflagellates,” in Freshwater Algae: Identification and Use as Bioindicators, eds E. G. Bellinger, and D. C. Sigee, (Chichester: John Wiley & Sons), 25–28.
Boyce, D. G., Lewis, M. R., and Worm, B. (2010). Global phytoplankton decline over the past century. Nature 466, 591–596. doi: 10.1038/nature09268
Brasil, J., and Huszar, V. L. M. (2011). O papel dos traços funcionais na ecologia do fitoplânton continental. Oec. Austr. 15, 799–834. doi: 10.4257/oeco.2011.1504.04
Brookes, J. D., and Ganf, G. G. (2001). Variations in the buoyancy response of Microcystis aeruginosa to nitrogen, phosphorus and light. J. Plankton Res. 23, 1399–1411. doi: 10.1093/plankt/23.12.1399
Campos, L. S., Barboza, C. A. M., Bassoi, M., Bernardes, M., Bromberg, S., Corbisier, T. N., et al. (2013). “Environmental processes, biodiversity and changes in admiralty bay, king george island, antarctica,” in Adaptation and Evolution in Marine Environments: v. 2: The Impacts of Global Change on Biodiversity, eds C. Verde and G. Di Prisco, (Berlin, DE: Springer Heidelberg), 127–156.
Cardoso, S. J., Nabout, J. C., Farjalla, V. F., Lopes, P. M., Bozelli, R. L., Huszar, V. L. M., et al. (2017). Environmental factors driving phytoplankton taxonomic and functional diversity in Amazonian floodplain lakes. Hydrobiologia 802, 115–130. doi: 10.1007/s10750-017-3244-x
Clarke, A., Barnes, D. K. A., and Hodgson, D. A. (2005). How isolated is Antarctica? Trends Ecol. Evol. 20, 1–3. doi: 10.1016/j.tree.2004.10.004
Clarke, A., Meredith, M. P., Wallace, M. I., Brandon, M. A., and Thomas, D. N. (2008). Seasonal and interannual variability in temperature, chlorophyll and macronutrients in northern Marguerite Bay, Antarctica. Deep Sea Res. II 55, 1988–2006. doi: 10.1016/j.dsr2.2008.04.035
Corbisier, T. N., Petti, M. A. V., Skowronski, R. S. P., and Brito, T. A. S. (2004). Trophic relationships in the nearshore zone of martel inlet (King George Island, Antarctica): δ13C stable-isotope analysis. Polar Biol. 27, 75–82. doi: 10.1007/s00300-003-0567-z
Cornwell, W. K., Schwilk, D. W., and Ackerly, D. D. (2006). A trait-based test for habitat filtering: convex hull volume. Ecology 87, 1465–1471. doi: 10.1890/0012-9658(2006)87
Crawford, R. M., and Sims, P. A. (2008). Some principles of chain formation as evidenced by the early diatom fossil record. Nova Hedwigia 133, 171–186.
Cupp, E. E. (1943). Marine Plankton Diatoms of the West of North America. California, CA: University of California Press.
Da Cunha, L. C., Hamacher, C., Farias, C. O., Kerr, R., Mendes, C. R. B., and Mata, M. M. (2018). Contrasting end summer distribution of organic carbon along the gerlache strait, Northern Antarctic Peninsula: bio-physical interactions. Deep Sea Res. II 149, 206–219. doi: 10.1016/j.dsr2.2018.03.003
Deppeler, S. L., and Davidson, A. T. (2017). Southern ocean phytoplankton in a changing climate. Front. Mar. Sci. 4:40. doi: 10.3389/fmars.2017.00040
Dierssen, H. M., Smith, R. C., and Vernet, M. (2002). Glacial meltwater dynamics in coastal waters west of the Antarctic Peninsula. Proc. Natl. Acad. Sci. U.S.A. 99, 1790–1795. doi: 10.1073/pnas.032206999
Ducklow, H. W., Baker, K., Martinson, D. G., Quetin, L. B., Ross, R. M., Smith, R. C., et al. (2007). Marine pelagic ecosystems: the west Antarctic Peninsula. Phil. Trans. R. Soc. B 362, 67–94. doi: 10.1098/rstb.2006.1955
Ellegaard, M., and Ribeiro, S. (2018). The long-term persistence of phytoplankton resting stages in aquatic ‘seed banks’. Biol. Rev. 93, 166–183. doi: 10.1111/brv.12338
Falkowski, P. G. (2000). Rationalizing elemental ratios in unicellular algae. J. Phycol. 36, 3–6. doi: 10.1046/j.1529-8817.2000.99161.x
Geider, R. J., and La Roche, J. (2002). Redfield revisited: variability of C:N:P in marine microalgae and its biochemical basis. Eur. J. Phycol. 37, 1–17. doi: 10.1017/S0967026201003456
Grasshoff, K., Ehrhardt, M., and Kremling, K. (1983). Methods of Seawater Analysis, 2nd Edn. New York, NY: Verlag Wienhien Chemie, 419.
Gutt, J., Barratt, I., Domack, E. C., D’acoz, C. U., Dimmler, W., Gre’Mare, A., et al. (2011). Biodiversity change after climate-induced ice-shelf collapse in the Antarctic. Deep Sea Res. II 58, 74–83. doi: 10.1016/j.dsr2.2010.05.024
Haberman, K. L., Ross, R. M., and Quetin, L. B. (2003). Diet of the Antarctic krill (Euphausia superba Dana): II. Selective grazing in mixed phytoplankton assemblages. J. Exp. Mar. Biol. Ecol. 283, 97–113. doi: 10.1016/S00220981(02)00467-7
Hamm, C. E., Merkel, R., Springer, O., Jurkojc, P., Maier, C., Prechtel, K., et al. (2003). Architecture and material properties of diatom shells provide effective mechanical protection. Nature 421, 841–843. doi: 10.1038/nature01416
Hammer, Ø, Harper, D. A. T., and Ryan, P. D. (2001). PAST: paleontological statistics software package for education and data analysis. Palaeontologia Electronica 4, 1–9.
Hasle, G. R., and Syvertsen, E. E. (1996). “Marine diatoms,” in Identifying Marine Phytoplankton, ed. C. R. Tomas, (San Diego, CA: Academic Press), 5–385. doi: 10.1016/b978-012693015-3/50005-x
Henley, S. F., Schofield, O. M., Hendry, K. R., Schloss, I. R., Steinberg, D. K., Moffat, C., et al. (2019). Variability and change in the west Antarctic Peninsula marine system: research priorities and opportunities. Prog. Oceanogr. 173, 208–237. doi: 10.1016/j.pocean.2019.03.003
Henley, S. F., Tuerena, R. E., Annett, A. L., Fallick, A. E., Meredith, M. P., Venables, H. J., et al. (2017). Macronutrient supply, uptake and recycling in the coastal ocean of the west Antarctic Peninsula. Deep Sea Res. II 139, 58–76. doi: 10.1016/j.dsr2.2016.10.003
Hustedt, F. (1930). “Die Kieselalgen deutschlands, österreichs und der Schweiz,” in Kryptogamenflora von Deutschland, Österreichs und der Schweiz, ed. L. Rabenhorst, (Leipzig: Akademische Verlagsgesellschaft m.b.H.), 1–608.
Johnston, D. W., Friedlaender, A. S., Read, A. J., and Nowacek, D. P. (2012). Initial density estimates of humpback whales Megaptera novaeangliae in the inshore Waters of the western Antarctic Peninsula during the late autumn. Endang. Species Res. 18, 63–71. doi: 10.3354/esr00395
Kim, H., Doney, S. C., Iannuzzi, R. A., Meredith, M. P., Martinson, D. G., and Ducklow, H. W. (2016). Climate forcing for dynamics of dissolved inorganic nutrients at Palmer Station, Antarctica: An interdecadal (1993-2013) analysis. J. Geophys. Res. Biogeosci. 121, 2369–2389. doi: 10.1002/2015jg003311
Kim, H., Ducklow, H. W., Abele, D., Barlett, E. M. R., Buma, A. G. J., Meredith, M. P., et al. (2018). Inter-decadal variability of phytoplankton biomass along the coastal West Antarctic Peninsula. Phil. Trans. R. Soc. A 376, 1–21. doi: 10.1098/rsta.2017.0174
Klais, R., Norros, V., Lehtinen, S., Tamminen, T., and Olli, K. (2016). Community assembly and drivers of phytoplankton functional structure. Funct. Ecol. 31, 760–767. doi: 10.1111/1365-2435.12784
Kopczyńska, E. E. (1996). Annual study of phytoplankton in Admiralty Bay, King George Island, Antarctica. Pol. Polar Res. 17, 151–164.
Kopczyńska, E. E. (2008). Phytoplankton variability in admiralty bay, king George island, south Shetland islands: six years of monitoring. Pol. Polar Res. 29, 117–139.
Kraft, N. J. B., Adler, P. B., Godoy, O., James, E. C., Fuller, S., and Levine, J. M. (2015). Community assembly, coexistence and the environmental filtering metaphor. Funct. Ecol. 29, 592–599. doi: 10.1111/1365-2435.12345
Kruk, C., Huszar, V. L. M., Peeters, E. T. H. M., Bonilla, S., Costa, L., Lürling, M., et al. (2010). A morphological classification capturing functional variation in phytoplankton. Freshw. Biol. 55, 614–627. doi: 10.1111/j.1365-2427.2009.02298.x
Kruk, C., Mazzeo, N., Lacerot, G., and Reynolds, C. S. (2002). Classification schemes for phytoplankton: a local validation of a functional approach to the analysis of species temporal replacement. J. Plankton Res. 24, 901–912. doi: 10.1093/plankt/24.9.901
Kruk, C., and Segura, A. M. (2012). The habitat template of phytoplankton morphology-based functional groups. Hydrobiologia 698, 191–202. doi: 10.1007/s10750-012-1072-6
Laliberté, E., and Legendre, P. (2010). A distance-based framework for measuring functional diversity from multiple traits. Ecology 91, 299–305. doi: 10.1890/08-2244.1
Lange, P. K., Ligowski, R., and Tenenbaum, D. R. (2018). Phytoplankton in the embayments of King George island (Antarctic Peninsula): a review with emphasis on diatoms. Polar Rec. 54, 1–18. doi: 10.1017/S0032247418000232
Lange, P. K., Tenenbaum, D. R., Braga, E. S., and Campos, L. S. (2007). Microphytoplankton assemblages in shallow waters at Admiralty Bay (King George island, Antarctica) during the summer 2002–2003. Polar Biology 30, 1483–1492. doi: 10.1007/s00300-007-0309-8
Lange, P. K., Tenenbaum, D. R., Tavano, V. M., Paranhos, R., and Campos, L. S. (2014). Shifts in microphytoplankton species and cell size at Admiralty Bay, Antarctica. Antarctic Sci. 27, 225–239. doi: 10.1017/S0954102014000571
Lebrija-Trejos, E., Pérez-García, E. A., Meave, J. A., Bongers, F., and Poorter, L. (2010). Functional traits and environmental filtering drive community assembly in a species-rich tropical system. Ecology, 91, 386–398. doi: 10.1890/08-1449.1
Leles, S. G., Polimene, L., Bruggeman, J., Blackford, J., Ciavatta, S., Mitra, A., et al. (2018). Modelling mixotrophic functional diversity and implications for ecosystem function. J. Plankton Res. 40, 627–642. doi: 10.1093/plankt/fby044
Ligowski, R. (2000). Benthic feeding by krill, Euphausia superba dana, in coastal waters off West Antarctica and in Admiralty Bay, South Shetland islands. Polar Biol. 23, 619–625. doi: 10.1007/s003000000131
Litchman, E., and Klausmeier, C. A. (2008). Trait-based community ecology of phytoplankton. Annu. Rev. Ecol. Evol. Syst. 39, 615–639. doi: 10.1146/annurev.ecolsys.39.110707.173549
Litchman, E., Klausmeier, C. A., and Yoshiyama, K. (2009). Contrasting size evolution in marine and freshwater diatoms. Proc. Natl. Acad. Sci. U.S.A. 106, 2665–2670. doi: 10.1073/pnas.0810891106
Litchman, E., Pinto, P. T., Klausmeier, C. A., Thomas, M. K., and Yoshiyama, K. (2010). Linking traits to species diversity and community structure inphytoplankton. Hydrobiologia 653, 15–28. doi: 10.1007/s10750-010-0341-5
Lobo, E., and Leighton, G. (1986). Estructuras comunitárias de lãs fitocenosis planctônicas de los sistemas de desembocaduras de rios y esteros de la zona central de Chile. Rev. Biol. Mar. 22, 1–29.
Lund, J. W., Kipling, G., and Le Cren, E. D. (1958). The invertedmicroscope method of estimating algae numbers andthe statistical basis of estimation by counting. Hydrobiologia 11, 143–170. doi: 10.1007/bf00007865
Martin, A. P. (2002). Phylogenetic approaches for describing and comparing the diversity of microbial communities. Appl. Environ. Microbiol. 68, 3673–3682. doi: 10.1128/AEM.68.8.3673-3682.2002
Mayfield, M. M., and Levine, J. M. (2010). Opposing effects of competitive exclusion on the phylogenetic structure of communities. Ecol. Lett. 13, 1085–1093. doi: 10.1111/j.1461-0248.2010.01509.x
McGill, B. J., Enquist, B. J., Weiher, E., and Westoby, M. (2006). Rebuilding community ecology from functional traits. Trends Ecol. Evolut. 21, 178–185. doi: 10.1016/j.tree.2006.02.002
McQuoid, M., Godhe, A., and Nordberg, K. (2002). Viability of phytoplankton resting stages in the sediment of a coastal Swedish fjord. Eur. J. Phycol. 37, 191–201. doi: 10.1017/S0967026202003670
Medlin, L. K., and Priddle, J. (1990). Polar Marine Diatoms. Cambridge: British Antarctic Survey, Natural Environment Research Council.
Mendes, C. R. B., de Souza, M. S., Garcia, V. M. T., Leal, M. C., Brotas, V., Garcia, C. A. E., et al. (2012). Dynamics of phytoplankton communities during late summer around the tip of the Antarctic Peninsula. Deep Sea Res. I 65, 1–14. doi: 10.1016/j.dsr.2012.03.002
Mendes, C. R. B., Tavano, V. M., Dotto, T. S., Kerr, R., de Souza, M. S., Garcia, C. A. E., et al. (2017). New insights on the dominance of cryptophytes in Antarctic coastal waters: a case study in Gerlache Strait. Deep Sea Res. II 149, 161–170. doi: 10.1016/j.dsr2.2017.02.010
Mendes, C. R. B., Tavano, V. M., Kerr, R., Dotto, T. S., Maximiano, T., and Secchi, E. R. (2018). Impact of sea ice on the structure of phytoplankton communities in the northern Antarctic Peninsula. Deep Sea Res. II 149, 111–123. doi: 10.1016/j.dsr2.2017.12.003
Mendes, C. R. B., Tavano, V. M., Leal, M. C., de Souza, M. S., Brotas, V., and Garcia, C. A. E. (2013). Shifts in the dominance between diatoms and cryptophytes during three late summers in the Bransfield Strait (Antarctic Peninsula). Polar Biol. 36, 537–547. doi: 10.1007/s00300-012-1282-4
Moestrup, Ø, Akselmann-Cardella, R., Fraga, S., Hoppenrath, M., Iwataki, M., Komárek, J., et al. (2009). IOC-UNESCO Taxonomic Reference List of Harmful Micro Algae. Available at: http://www.marinespecies.org/hab (accessed August 10, 2018).
Moline, M. A., Claustre, H., Frazer, T. K., Schofield, O., and Vernet, M. (2004). Alteration of the food web along the Antarctic Peninsula in response to a regional warming trend. Glob. Chang. Biol. 10, 1973–1980. doi: 10.1111/j.1365-2486.2004.00825.x
Montes-Hugo, M., Doney, S. C., Ducklow, H. W., Fraser, W., Martinson, D., Stammerjohn, S. E., et al. (2009). Recent changes in phytoplankton communities associated with rapid regional climate change along the Western Antarctic Peninsula. Science 323, 1470–1473. doi: 10.1126/science.1164533
Moser, G. A. O., Piedras, F. R., Oaquim, A. B. J., Souza, D. S., Leles, S. G., Lima, D. T., et al. (2017). Tidal effects on phytoplankton assemblages in a near-pristine estuary: a trait-based approach for the case of a shallow tropical ecosystem in Brazil. Mar. Ecol. Evol. Persp. 38, 1–18. doi: 10.1111/maec.12450
Moser, G. A. O., Takanohashi, R. A., Braz, M. C., Lima, D. T., Kirsten, F. V., Guerra, J. V., et al. (2014). Phytoplankton spatial distribution on the continental shelf off rio de janeiro, from paraíba do sul river to cabo Frio. Hydrobiologia 728, 1–21. doi: 10.1007/s10750-013-1791-3
Mouchet, F., Landois, P., Sarremejean, E., Bernard, G., Pascal, P., Pinelli, E., et al. (2008). Characterisation and in vivo ecotoxicity evaluation of double-wall carbon nanotubes in larvae of the amphibian Xenopus laevis. Aquat. Toxicol. 87, 127–137. doi: 10.1016/j.aquatox.2008.01.011
Mouillot, D., Villéger, S., Parravicini, V., Kulbicki, M., Arias-González, J. E., Bender, M., et al. (2014). Functional over-redundancy and high functional vulnerability in global fish faunas on tropical reefs. PNAS 111, 13757–13762. doi: 10.1073/pnas.1317625111
Naselli-Flores, L., Padisak, J., and Albay, M. (2007). Shape and size in phytoplankton ecology: do they matter? Hydrobiologia 578, 157–161. doi: 10.1007/s10750-006-2815z
Nedzarek, A., and Rakusa-Suszczewski, S. (2004). Decomposition of macroalgae and the release of nutrients in Admiralty Bay, King George Island, Antarctica. Polar Biol. 17, 26–35.
Neveux, J., and Lantoine, F. (1993). Spectrofluorometric assay of chlorophylls and phaeopigments using the least- squares approximation technique. Deep Sea Res. I 40, 1747–1765. doi: 10.1016/0967-0637(93)90030-7
Oksanen, J., Blanchet, F. G., Friendly, M., Kindt, R., Legendre, P., McGlinn, D., et al. (2017). Vegan: Community Ecology Package. R package version 2.4-3. Avaiable at: https://CRAN.R-project.org/package=vegan (accessed December 10, 2017).
Onuma, R., and Horiguchi, T. (2013). Morphological Transition in Kleptochloroplasts after Ingestion in the Dinoflagellates Amphidinium poecilochroum and Gymnodinium aeruginosum (Dinophyceae). Protist 164, 622–642. doi: 10.1016/j.protis.2013.06.003
Padisák, J., Soróczki-Pintér, É, and Rezner, Z. (2003). Sinking properties of some phytoplankton shapes and the relation of form resistance to morphological diversity of plankton – an experimental study. Hydrobiologia 500, 243–257. doi: 10.1023/a:1024613001147
Paerl, H. W., and Huisman, J. (2009). Climate change: a catalyst for global expansion of harmful cyanobacterial blooms. Environ. Microbiol. Rep. 1, 27–37. doi: 10.1111/j.1758-2229.2008.00004.x
Petchey, O. L., and Gaston, K. J. (2007). Dendrograms and measuring functional diversity. Oikos 116, 1422–1426. doi: 10.HH/j.2007.0030-1299.158
Pichlmaier, M., Aquino, F. E., Da-Silva, C. S., and Braun, M. (2004). Suspended sediments in Admiralty Bay, King George island (Antarctica). Braz. Antarctic Res. 4, 77–85.
Piquet, A. M. T., Bolhuis, H., Meredith, M. P., and Buma, A. G. J. (2011). Shifts in coastal Antarctic marine microbial communities during and after melt water related surface stratification. FEMS Microb. Ecol. 76, 413–427. doi: 10.1111/j.1574-6941.2011.01062.x
Pomati, F., Kraft, N. J. B., Posch, T., Eugster, B., Jokela, J., and Ibelings, B. W. (2013). Individual cell based traits obtained by scanning flow-cytometry show selection by biotic and abiotic environmental factors during a phytoplankton spring bloom. PLoS One 8:e71677. doi: 10.1371/journal.pone.0071677
Pruszak, Z. (1980). Current circulation in the water of Admiralty Bay (region of Arctowski station on King George Island). Pol. Polar Res. 1, 55–74.
R Core Team (2018). R: A Language and Environment for Statistical Computing. Vienna: R Foundation for Statistical Computing.
Rakusa-Suszczewski, S. (1980). Environmental conditions and the functioning of Admiralty Bay (South Shetland Islands) as part of the near shore Antarctic ecosystem. Polar Res. 1, 11–27.
Redfield, A. C. (1958). The biological control of chemical factors in the environment. Am. Sci. 46, 205–221.
Reynolds, C. S. (1980). Phytoplankton associations and their periodicity in stratifying lake systems. Holarct. Ecol. 3, 141–159. doi: 10.1111/j.1600-0587.1980.tb00721.x
Roberts, K., Granum, E., Leegood, R. C., and Raven, J. A. (2007). C3 and C4 pathways of photosynthetic carbon assimilation in marine diatoms are under genetic, not environmental, control. Plant Physiol. 145, 230–235. doi: 10.1104/pp.107.102616
Roselli, L., and Basset, A. (2015). Decoding size distribution patterns in marine and transitional water phytoplankton: from community to species level. PLoS One 10:e0127193. doi: 10.1371/journal.pone.0127193
Roselli, L., Litchman, E., Stanca, E., Cozzoli, F., and Basset, A. (2017). Contribution to the themed section: phytoplankton traits, functional groups and community organization. J. Plankton Res. 39, 577–588. doi: 10.1093/plankt/fbx001
Rott, E. (1981). Some results from phytoplankton counting intercalibrations. Schweiz. Z. Hydrol. 43, 34–62. doi: 10.1007/bf02502471
Round, F. E., Crawford, R. W., and Mann, D. G. (1990). The Diatoms, Biology & Morphology of the Genera. Cambridge: Cambridge University Press.
Rozema, P. D., Venables, H. J., van de Poll, W. H., Clarke, A., Meredith, M. P., and Buma, A. G. J. (2017). Interannual variability in phytoplankton biomass and species composition in northern Marguerite Bay (West Antarctic Peninsula) is governed by both winter sea ice cover and summer stratification. Limnol. Oceanogr. 62, 235–252. doi: 10.1002/lno.10391
Russo, A. D. P. G., Souza, M. S., Mendes, C. R. B., Tavano, V. M., and Garcia, C. A. E. (2018). Spatial variability of photophysiology and primary production rates of the phytoplankton communities across the western Antarctic Peninsula in late summer 2013. Deep Sea Res. II 149, 99–110. doi: 10.1016/j.dsr2.2017.09.021
Saba, G. K., Fraser, W. R., Saba, V. S., Iannuzzi, R. A., Coleman, K. E., and Doney, S. C. (2014). Winter and spring controls on the summer food web of the coastal West Antarctic Peninsula. Nat. Commun. 5, 4318. doi: 10.1038/ncomms5318
Salmaso, N. (2003). Life strategies, dominace patterns and mechanisms promoting species coexistence in phytoplankton communities along complex environmental gradients. Hydrobiologia 502, 13–36. doi: 10.1023/B:HYDR.0000004267.64870.85
Sander, M., Balbão, T. C., Polito, M. J., Costa, E. S., and Carneiro, A. P. B. (2007). Recent decrease in chinstrap penguin (Pygoscelis antarctica) populations at two of Admiralty Bay’s islets on King George Island, South Shetland Islands, Antarctica. Polar Biol. 30, 659–661. doi: 10.1007/s00300-007-0259-1
Schloss, I. R., Abele, D., Moreau, S., Demers, S., Bers, A. V., González, O., et al. (2012). Response of phytoplankton dynamics to 19-year (1991– 2009) climate trends in Potter Cove (Antarctica). J. Mar. Syst. 92, 53–66. doi: 10.1016/j.jmarsys.2011.10.006
Schloss, I. R., Ferreyra, G. A., Ferrario, M. E., Almandoz, G. O., Codina, R., Bianchi, A. A., et al. (2007). Role of plankton communities in sea–air variations in pCO2 in the SW Atlantic ocean. Mar. Ecol. Prog. Ser. 332, 93–106. doi: 10.3354/meps332093
Schloss, I. R., Ferreyra, G. A., and Ruiz-Pino, D. (2002). Phytoplankton biomass in Antarctic shelf zones: a conceptual model based on Potter Cove, King George Island. J. Mar. Syst. 36, 129–143. doi: 10.1016/S0924-7963(02)00183-5
Schloss, I. R., Wasilowska, A., Dumont, D., Almandoz, G. O., Hernando, M. P., Michaud-Tremblay, C. A., et al. (2014). On the phytoplankton bloom in coastal waters of Southern King George Island (Antarctica) in January 2010: An exceptional feature? Limnol. Oceanogr. 59, 195–210. doi: 10.4319/lo.2014.59.1.0195
Schofield, O., Ducklow, H. W., Martinson, D. G., Meredith, M. P., Moline, M. A., and Fraser, W. R. (2010). How do polar marine ecosystems respond to rapid climate change? Science 328, 1520–1523. doi: 10.1126/science.1185779
Schofield, O., Saba, G., Coleman, K., Carvalho, F., Couto, N., Ducklow, H., et al. (2017). Decadal variability in coastal phytoplankton community composition in a changing West Antarctic Peninsula. Deep Sea Res. I 124, 42–54. doi: 10.1016/j.dsr.2017.04.014
Scott, F. J., and Marchant, H. J. (2005). Antarctic Marine Protists. Canberra, ACT: Australian Biological Resources Study.
Segura, A. M., Kruk, C., Calliari, D., García-Rodriguez, F., Conde, D., Widdicombe, C. E., et al. (2013). competition drives clumpy species coexistence in estuarine phytoplankton. Sci. Rep. 3, 1–6. doi: 10.1038/srep01037
Tamulonis, C., Postma, M., and Kaandorp, J. (2011). Modeling filamentous Cyanobacteria reveals the advantages of long and fast trichomes for optimizing light exposure. PLoS One 6:e22084. doi: 10.1371/journal.pone.0022084
Tenenbaum, D. R., Barrera-Alba, J. J., Duarte, R. D., and Tenório, M. M. B. (2011). “Plankton structure of shallow coastal zone at admiralty bay, king george island, west antarctic peninsula (WAP): pico, nano and microplankton and chlorophyll biomass,” in Annual Activity Report of National Institute of Science and Technology Antarctic Environmental Research (INCT-APA) - 2010, eds Y. Y. Valentin, A. G. Dalto, and H. P. Lavrado, (São Carlos: Cubo), 108–114. doi: 10.4322/apa.2014.033
Tenenbaum, D. R., Barrera-Alba, J. J., and Tenório, M. M. B. (2015). “Microplankton community structure of the shallow coastal zone at admiralty bay, antarctica: comparison between two consecutive austral summers,” in Annual Activity Report of National Institute of Science and Technology Antarctic Environmental Research (INCT-APA) - 2013, eds Y. Y. Valentin, A. G. Dalto, and H. P. Lavrado, (São Carlos: Cubo), 75–80. doi: 10.4322/apa.2015.014
Tenenbaum, D. R., Lange, P. K., Fernandes, L. F., Calixto-Feres, M., Barrera-Alba, J. J., and Garcia, V. M. T. (2010). “Plankton structure in a shallow coastal zone at admiralty bay, king george island west antarctic peninsula (WAP): composition of phytoplankton and influence of benthic diatoms,” in Annual Activity Report of National Institute of Science and Technology Antarctic Environmental Research (INCT-APA) - 2010, eds Y. Y. Valentin, A. G. Dalto, and H. P. Lavrado, (São Carlos: Cubo), 121–125. doi: 10.4322/apa.2014.035
Tenenbaum, D. R., Villac, M. C., Viana, S. C., Matos, M., Hatherly, M., Lima, I. V., et al. (2004). Phytoplankton Atlas of Sepetiba Bay, Rio de Janeiro, Brazil. London: IMO.
Tenório, M. M. B., Le Borgne, R., Rodier, M., and Neveux, J. (2005). The impact of terrigeneous inputs on the Bay of Ouinné (New Caledonia) phytoplankton communities: a spectrofluorometric and microscopic approach. Estuar. Coast. Shelf. Sci. 64, 531–545. doi: 10.1016/j.ecss.2005.02.030
Tenório, M. M. B., Pinto, F. A. V., Barrera-Alba, J. J., Neveux, J., and Tenenbaum, D. R. (2015). “Chlorophyll a biomass and accessory chlorophyll pigments in the shallow coastal zone at admiralty bay, antarctica: comparison between two consecutive austral summers,” in Annual Activity Report of National Institute of Science and Technology Antarctic Environmental Research (INCT-APA) - 2013, eds Y. Y. Valentin, A. G. Dalto, and H. P. Lavrado, (São Carlos: Cubo), 81–86. doi: 10.4322/apa.2015.015
Thingstad, T. F., Øvreas, L., Egge, J. K., Løvdal, T., and Heldal, M. (2005). Use of non-limiting substrates to increase size; a generic strategy to simultaneously optimize uptake and minimize predation in pelagic osmotrophs. Ecol. Lett. 8, 675–682. doi: 10.1111/j.1461-0248.2005.00768.x
Turner, J., Colwell, S. R., Marshall, G. J., Lachlan-Cope, T. A., and Carleton, A. M. (2005). Antarctic climate change during the last 50 years. Int. J. Climatol. 25, 279–294. doi: 10.1002/joc.1130
Utermöhl, H. (1958). Perfeccionamento del método cuantitativo del fitoplancton. Comum. Assoc. Int. Limnol. Teor. Apl. 9, 1–89.
Vallina, S. M., Cermeno, P., Dutkiewicz, S., Loreau, M., and Montoya, J. M. (2017). Phytoplankton functional diversity increases ecosystem productivity and stability. Ecol. Model. 361, 184–196. doi: 10.1016/j.ecolmodel.2017.06.020
Vanzan, M., Barrera-Alba, J. J., Tenório, M. M. B., and Tenenbaum, D. R. (2015). Picoplankton and nanoplankton variability in an Antarctic shallow coastal zone (Admiralty Bay) during the austral summer of 2010/2011. Polar Biol. 38, 1–18. doi: 10.1007/s00300-015-1692-1
Verberk, W. C. E. P., van Noordwijk, C. D. E., and Hildrew, A. G. (2013). Delivering on a promise: integrating species traits to transform descriptive community ecology into a predictive science. Freshw. Sci. 32, 531–547. doi: 10.1899/12-092.1
Villéger, S., Mason, N. W. H., and Mouillot, D. (2008). New multidimensional functional indices for a multifaceted framework in functional ecology. Ecology 89, 2290–2301. doi: 10.1890/07-1206.1
Violle, C., Navas, M. L., Vile, D., Kazakou, E., Fortunel, C., Hummel, I., et al. (2007). Let the concepto f trait be functional! Oikos 116, 882–892. doi: 10.1111/j.0030-1299.2007.15559.x
Wadt, P. R., Mafra, L. L. Jr., Tavares, C. P. S. T., Fernandes, L. F., and Proença, L. A. O. (2017). Growth, chain formation, and toxin production by Southern Brazilian Pseudo-nitzschia isolates under laboratory conditions. Environ. Monit. Assess. 189, 1–15. doi: 10.1007/s10661-017-6301-z
Wasiłowska, A., Kopczyńska, E. E., and Rzepecki, M. (2015). Temporal and spatial variation of phytoplankton in Admiralty Bay, South Shetlands: the dynamics of summer blooms shown by pigment and light microscopy analysis. Polar. Biol. 38, 1–17. doi: 10.1007/s00300-015-1691-2
Weithoff, G., and Gaedke, U. (2016). Mean functional traits of lakephytoplankton reflect seasonal and inter-annual changes in nutrients, climate and herbivory. J. Plankton. Res. 39, 509–517. doi: 10.1093/plankt/fbw072
Weithoff, G., Rocha, M. R., and Gaedke, U. (2014). Comparing seasonal dynamics of functional and taxonomic diversity reveals the driving forces underlying phytoplankton community structure. Freshw. Biol. 60, 758–767. doi: 10.1111/fwb.12527
Keywords: functional diversity, microphytoplankton, Antarctic, abiotic variation, meltwater
Citation: Lima DT, Moser GAO, Piedras FR, Da Cunha LC, Tenenbaum DR, Tenório MMB, Campos MVPB, Cornejo TO and Barrera-Alba JJ (2019) Abiotic Changes Driving Microphytoplankton Functional Diversity in Admiralty Bay, King George Island (Antarctica). Front. Mar. Sci. 6:638. doi: 10.3389/fmars.2019.00638
Received: 25 June 2019; Accepted: 30 September 2019;
Published: 17 October 2019.
Edited by:
Chiara Piroddi, Joint Research Centre (Italy), ItalyReviewed by:
Alex J. Poulton, The Lyell Centre, United KingdomYong Jiang, Ocean University of China, China
Copyright © 2019 Lima, Moser, Piedras, Da Cunha, Tenenbaum, Tenório, Campos, Cornejo and Barrera-Alba. This is an open-access article distributed under the terms of the Creative Commons Attribution License (CC BY). The use, distribution or reproduction in other forums is permitted, provided the original author(s) and the copyright owner(s) are credited and that the original publication in this journal is cited, in accordance with accepted academic practice. No use, distribution or reproduction is permitted which does not comply with these terms.
*Correspondence: Gleyci Aparecida Oliveira Moser, Z2xleWNpbW9zZXJAZ21haWwuY29t