- Mediterranean Institute of Oceanography, UM 110, Aix-Marseille Université, CNRS, IRD, Marseille, France
The oceanic biological carbon pump (BCP) regulates the Earth carbon cycle by transporting part of the photosynthetically fixed CO2 into the deep ocean. Suppressing this mechanism would result in an important increase of atmospheric CO2 level. The BCP occurs mainly in the form of (1) organic carbon (OC) particles sinking out the surface ocean, of (2) neutrally buoyant OC (dissolved or particulate) entrained by downward water masses movements and/or mixing, and of (3) active transport of OC by migrating animals such as zooplankton and fishes. These various pools of OC differ in size since their sinking, production and decomposition rates vary spatially and temporally. Moreover, the OC transported to depths via these various export pathways as well as their decomposition pathways all have different ecological origins and therefore may response differently to climate changes. Currently, most ocean biogeochemical models do not resolve these various of OC pathways explicitly; rather, they imply that OC is therein created and destroyed equally. In addition, the organic composition of these various pools is largely unknown, especially at depths below 500 m. Here, known processes of OC export from the surface ocean to the mesopelagic zone (100–1000 m) are briefly reviewed. Three OC export pathways and some of their sub-categories are considered. I refer to published studies of OC fluxes associated with the specific downward export pathways and identify gaps that need to be addressed to better understand the OC fluxes associated with the BCP.
Introduction
The oceanic biological carbon pump (BCP) is a complex mechanism regulating the Earth carbon (C) cycle by sequestrating part of the photosynthetically fixed CO2 into the deep ocean and the seafloor. Suppressing this mechanism would increase the current levels of atmospheric CO2 level by about 50% (Sarmiento et al., 2004; Parekh et al., 2006). Currently, the variability in the proportion of surface primary production (PP) leaving the euphotic zone and the amount of organic carbon (OC) exported to a certain depth via the BCP are challenging to explain. This prevents robust predictions of how C cycle, and hence climate, will change in the future.
Three major forms of the BCP are distinguished: (i) gravitational, (ii) mixing and (iii) migration. The gravitational pump (i) involves downward sinking of the OC contained in various particles (POC) of planktonic origin from the surface ocean to depth due to their large size and/or high density (Siegel et al., 2016). This sinking POC is termed “marine snow” and can be made of single phytoplankton cells (DiTullio et al., 2000; Rembauville et al., 2016; Leblanc et al., 2018), various types of aggregates resulting from coagulation processes (Alldredge and Jackson, 1995; Riley et al., 2012; Laurenceau-Cornec et al., 2015) and zooplankton fecal pellets (FP) (Turner, 2002; Cavan et al., 2015). The mixing pump (ii) occurs when neutrally buoyant OC is transported to depth by downward-moving water masses and/or mixing. This OC is either dissolved (DOC) or entrained in particles which are too small in size or positively buoyant relative to seawater to sink on their own (Hansell et al., 2009; Le Moigne et al., 2016). The physical mechanisms that transport the OC downward can be either diapycnal with DOC diffusing out of the surface waters, or isopycnal, when water mass movements eddies and/or mixed layer bring DOC and/or suspended particles to depth (Hansell et al., 2009; Omand et al., 2015; Dall’Olmo et al., 2016). (iii) Diurnal vertical migration (DVM) of zooplankton and fish is a common feature in oceanic ecosystems (Longhurst, 1991; Steinberg, 2000; Landry and Calbet, 2004). In order to avoid predation, swimmers feed at night in surface waters and defecate deeper in the mesopelagic during the day. Doing so, they actively transport OC into the ocean’s interior.
The presence, absence, and dominance of each of these different export pathways are controlled by biological (Piontek et al., 2014; Agusti et al., 2015; Le Moigne et al., 2016), chemical (Edwards et al., 2015), ecological (Cavan et al., 2015; Guidi et al., 2016), and physical (Levy et al., 2013; Taucher et al., 2014; Dall’Olmo et al., 2016) processes. They all vary in time and space and at depth. Most of these processes, however, are still poorly understood which hinders mechanistic predictions of the magnitude of the downward OC fluxes. At the base of the euphotic zone, the sinking of particles (termed gravitational flux) dominate the amount of OC (Giering et al., 2014). However, in the end of winter, the OC flux is induced by the so-called mixed layer pump which is driven by the seasonal entrainment of surface waters in deeper layers (Gardner et al., 1995) and can supply a substantial amount of OC to the deep ocean. Also, while globally the proportion of OC transported by migrating zooplankton and larger organisms relative to total OC flux is <20%, this active flux can occasionally dominate the total OC flux [(Aumont et al., 2018) and references therein].
These examples demonstrate the need for more systematic and simultaneous knowledge (ecological, biological, and geochemical) of the various pathways of OC export and at a given location and time. As such, the various individual export pathways (Siegel et al., 2016) for POC sinking from the euphotic zone to the mesopelagic and below have to be quantified. This would, for example, improve our understanding of the imbalance between supply and metabolic demand of OC (Steinberg et al., 2008; Burd et al., 2010; Collins et al., 2015) and the discrepancy between observed and modeled O2 concentration in the mesopelagic zone (Gutknecht and Perruche, 2016). Currently our knowledge of the processes occurring in of the mesopelagic zone is incomplete despite its importance for the air sea carbon balance (Kwon et al., 2009). In this mini-review, I list the OC fluxes associated to the specific downward export pathways published in literature. Missing knowledge in estimates of specific export pathways that need to be addressed to better understand the OC fluxes associated with the BCP are identified.
The Gravitational Pump Flux
As stated above, the gravitational flux of OC occurs in the form of various particles of planktonic origin sinking from the surface ocean due to their large size and/or high density. The gravitational pump includes particles that are single phytoplankton cells, various types of aggregates, and zooplankton dejections termed fecal pellets.
Fecal Pellets Flux
Specific OC flux associated with zooplankton fecal pellets is relatively easy to measure. Fecal pellets are compact and have distinctive shapes and thus can easily identified and picked out from samples collected using different types of sediment traps (Refaeli et al., 2008; Turner, 2015). However, some pellets can experience degradation in moored sediment traps cups and are not being counted as such (Wilson et al., 2008). This may result in underestimation of the flux of fecal pellets. The OC flux associated with fecal pellets in the global ocean from 0 to 3500 m depth (n = 374) are shown in Figure 1 and also compiled in Supplementary Table S2. The fluxes classified by depth are presented in Figures 1A–C. Note that Figure 1 only presents the values that were readily available in the literature. I consider that the factors used by the authors to convert fecal pellets count into OC are correct. Counts of fecal pellets observed in various sediment traps if they were not converted to OC fluxes are not reported.
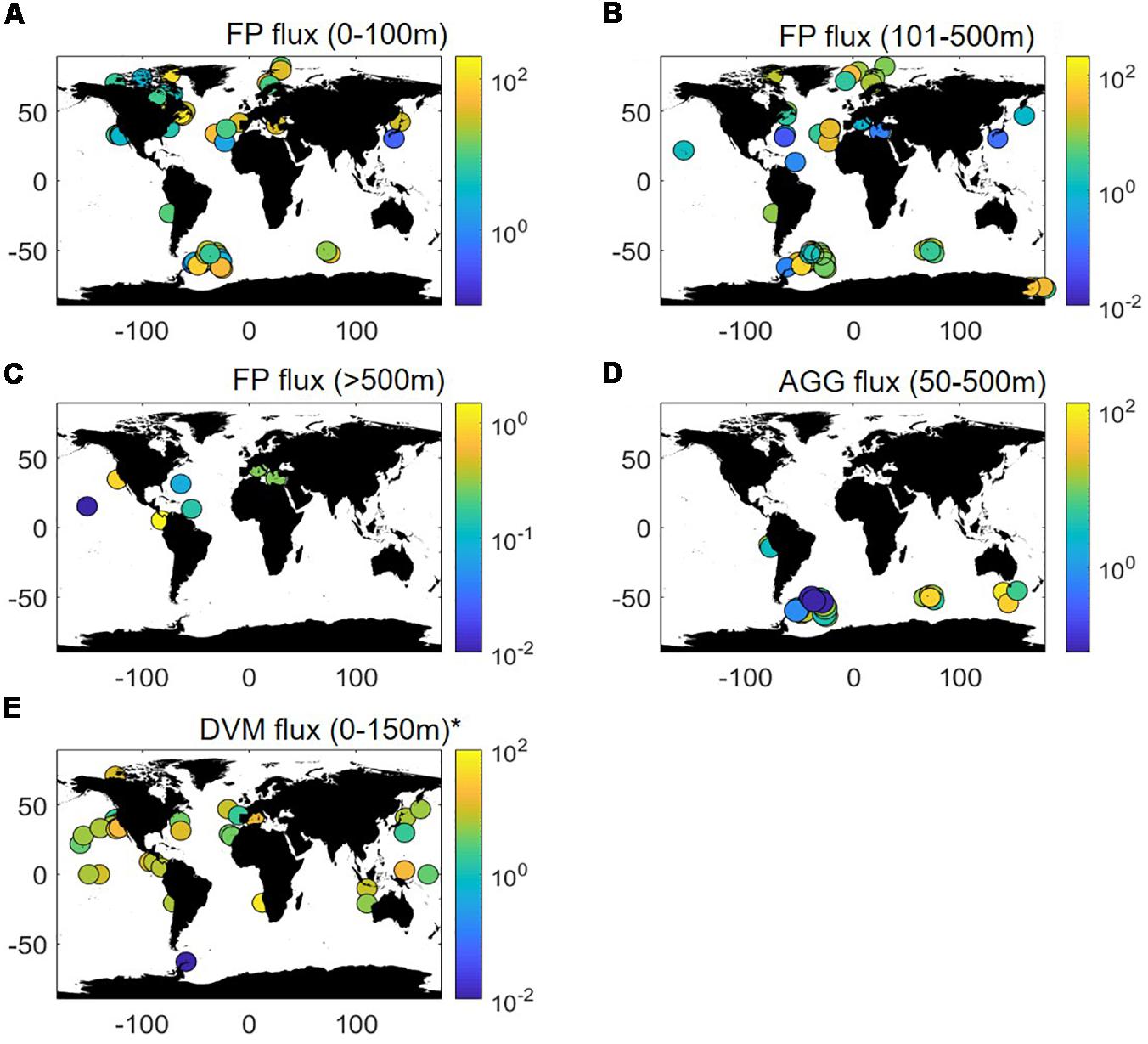
Figure 1. Global distribution of downward flux of organic carbon (OC; units are mg of OC m–2 d–1) associated to various export pathways (see main text). Panel (A) represents the flux associated to fecal pellets (FP) collected between 0–100 m; (B) 101–500 m and (C) below 500 m. Panel (D) represents the flux of aggregates collected between 50–500 m. Panel (E) represents the flux associated to vertically migrating organisms. ∗Replotted after Aumont et al. (2018). All references/sources are provided in the main text and in Supplementary Tables S1–S3.
Figure 1A shows the OC flux from 0 to 100 m depth. The OC flux varies from 0–218 mg C m–2 d–1 with the large OC fecal pellets fluxes recorded in high latitudes [e.g., Kerguelen Island in the Southern Ocean (Ebersbach and Trull, 2008; Laurenceau-Cornec et al., 2015)] and upwelling zones (Le Moigne et al., 2018). Yet, some studies also report absence of fecal pellets in high latitudes regions [e.g., (Cavan et al., 2015)]. Fecal pellets fluxes are significantly lower in mesotrophic and oligotrophic regions (Carroll et al., 1998; Shatova et al., 2012). In the upper mesopelagic zone (100–500 m; Figure 1B), the OC flux of fecal pellets ranges from 0 to 71 mg C m–2 d–1 and on regional scale broadly follows the pattern observed in the upper ocean. At depths below 500 m (Figure 1C), fecal pellet fluxes are generally low (0 to 1.5 mg C m–2 d–1). No reports of the OC flux below 500 m exist for the high latitude ocean. Fecal pellets sink fast and resist degradation well even under high hydrostatic pressure (Turley and Mackie, 1994; Tamburini et al., 2006; Riou et al., 2017) and thus their flux is expected to be high below 500 m. Coprorhexy (fragmentation of pellets by copepods) and/or coprophagy (ingestion of fecal pellets by copepods) could, however, lower the abundance of fecal pellets and hence the associated OC flux in the lower mesopelagic (Iversen and Poulsen, 2007). Additionally, such a trend could also result from fish and large zooplankton species (such as siphonophores) feeding on the pellet flux (Proud et al., 2019).
Phytoplankton Cells Flux
Turbulent mixing and buoyancy regulation tend to retain single phytoplankton single cells tend in the surface ocean. However, despite of their small Reynolds number [describes the nature of the surrounding flow and its fall velocity (Stokes, 1851)], some intact cells (not grazed or aggregated) are found in sediment traps. This can happen to single live or dead phytoplankton cells, resting spores or cysts, and colonies.
Phytoplankton cells sink into the ocean’s interior as single cells which are live, senescent and/or dead. DiTullio et al. (2000) observed single cells of Phaeocystis antarctica in the lower mesopelagic zone (500–600 m) in the Ross sea and concluded that they were exported rapidly and alive following a large Phaeocystis antarctica bloom. However, the mechanism delivering these cells down to the mesopelagic zone is unclear. Although physical processes could entrain surface cells to depth (see following sections), the cells observed at 500 m were still photosynthesizing, which suggests that their export to depth was likely too rapid to be induced by a physical process. Phytoplankton cells also sink dead. For example (Salter et al., 2007), observed dead cells of large diatoms Eucampia antarctica being selectively exported relative to the surface phytoplankton community in the Southern Ocean. These diatoms were exported rapidly owing to their heavily silicified, and thus dense, frustule (Salter et al., 2007). Phytoplankton resting spores and/or cysts are also exported from the surface ocean down to bathypelagic/abyssal depths (>1000 m). They are formed strategically by some phytoplankton in response to nutrient limitation and contain significant amounts of OC. In sediment traps located within an iron-fertilized bloom in the Southern Ocean near the Kerguelen Islands, Rembauville et al. (2016) measured OC fluxes associated with resting spores of diatoms Chaetoceros Hyalochaete or Thalassiosira Antarctica. The resting spores of a single species can account for up to 90% of the total gravitational OC flux (Rembauville et al., 2016). Acantharian cysts (unicellular organisms related to Radiolaria producing celestite, the densest biomineral in the ocean) were abundant in deep sediment trap material in the Iceland Basin (Martin et al., 2010) and the Southern Ocean (Belcher et al., 2018). High cyst flux was restricted to only 2 weeks but contributed up to half of the total gravitational OC flux during this period (Martin et al., 2010; Decelle et al., 2013). The Acantarian cysts are made of dense celestite which ensures their fast sedimentation to the bathypelagic depths. Phytoplankton cell colonies and chains are also observed in sediment traps samples. Laurenceau-Cornec et al. (2015) found chains of the pennate diatoms Fragilariopsis sp. in the Southern Ocean, while Pabortsava et al. (2017) collected intact colonies of nitrogen-fixing Trichodesmium species in the deep traps (3000 m) collecting in the North Atlantic gyre.
However, all published sediment trap studies do not necessarily report the OC flux associated with phytoplankton cell export. This is because phytoplankton enumeration methods require a substantial amount of cells to be identified in order to provide robust estimates. This is not always possible, and counting is often performed only on a small area imaged under microscopes (Leblanc et al., 2018). Quantification of the cell size (or biovolume) to OC content for a given species is also not straightforward (Gosselain et al., 2000) and varies substantially depending on upper ocean nutrients regime (Davidson and Marchant, 1992). Grouping phytoplankton cell flux in an ecologically meaningful way might be especially advantageous for numerical models as providing the OC flux associated with sinking cells of individual phytoplankton species is computationally inefficient. Future effort may require grouping phytoplankton cell flux functionally based on size, nutrients affinity, and ecological/physiological traits. Therefore, no global maps of the OC flux associated to sinking phytoplankton cells are provided here.
Aggregate Flux
Evaluating OC flux associated with aggregates is challenging as their structure is often fragile and erratic (Alldredge, 1998). In moored sediment traps, marine aggregates lose their shapes. Unlike fecal pellets (see section above), aggregates in the bulk sediment trap material are clumped and thus difficult to pick out individually. The use of polyacrylamide gels as preservative in the sediment traps collection tubes/cups has, however, enabled significant improvements of estimating the aggregates flux (Lundsgaard, 1995; Waite et al., 2000). These highly viscous gels preserve the morphological characteristics of marine particles as they sink through the water column and into collection cups. Gels provide a slow deceleration of sinking particles and isolate particles allowing for quantitative image analysis (Ebersbach and Trull, 2008).
Only few studies quantified aggregate-associated OC flux to date. Most estimates are from the Southern Ocean (Ebersbach and Trull, 2008; Cavan et al., 2015; Laurenceau-Cornec et al., 2015) and upwellings (Le Moigne et al., 2018). Largest OC fluxes (110 mg m–2 d–1) were recorded in the Sub-Antarctic zone south of Tasmania (Ebersbach et al., 2011). Large fluxes were also observed in the vicinity of the Kerguelen islands (Laurenceau-Cornec et al., 2015) and in the South Georgia bloom (Cavan et al., 2015) (Supplementary Table S1). Vertically, the flux of aggregates rarely shows a clear decrease with depth indicating significant changes in aggregates dynamics with depth. This highlights the importance of disaggregation and aggregation processes. Although overall disaggregation vastly dominates particles dynamics below the surface ocean (Stemmann et al., 2004; Lam and Marchal, 2015), aggregation by differential settling (Lam and Marchal, 2015) does occur in the mesopelagic zone. In addition, while the ratio of disaggregation to aggregation increases with depth, it tends to decrease with increasing PP (Lam and Marchal, 2015). This may partly explain why the vertical fluxes of aggregates presented here show limited decrease with depth.
Quantification of the aggregate-associated OC flux requires the knowledge of the relationship between aggregates biovolume to OC content, which is not straightforward (Alldredge and Crocker, 1995; Alldredge and Jackson, 1995). Laurenceau-Cornec et al. (2015) report particle-specific relationships compiled in the literature from which the OC content of aggregates can be estimated (Alldredge, 1998; Ebersbach and Trull, 2008). However, the database on the aggregates biovolume to OC content has to be significantly expanded to improve the statistical confidence of this relationship and also considering more diverse aggregates types. For example, tools like the Marine Snow Catcher (Riley et al., 2012; Cavan et al., 2015) could be used to collect particles and quantify their size, geochemical content and biological characteristics. The amount of particles collected per deployment can be small with the amount of OC entrained being too low to measure analytically (McDonnell et al., 2015). This device also likely misses the largest particles but pulling particles from multiple deployments or using devices of a larger volume (Cavan et al., 2017) could, however, resolve this issue.
The Mixing Flux
Several ocean physical processes transport slow-sinking, neutrally buoyant or dissolved OC (DOC) from the surface where they are produced to the mesopelagic zone. Recently, “mixing fluxes” gained more importance (see following references). This is mainly due the development of semi-automated platforms and devices which enabled high frequency sizing of particles (Picheral et al., 2010; Claustre et al., 2011). Large-scale ocean circulation is also one of the processes transporting particles produced in the surface to ocean’s interior. For instance, regions of deep water formation are hotspots for such transport (Levy et al., 2013).
The mixed layer pump is another process that redistributes dissolved OC, neutrally buoyant or slow-sinking organic matter down the water column (Dall’Olmo et al., 2016). This process occurs during transient stratifications at the start of the productive season when suspended POC first accumulates in surface, and then redistributed throughout the water column by deep mixing. Globally, the mixed layer pump is responsible for a flux of 0.5 Gt C yr–1. This process supplies POC mainly to locations with deep winter mixing such as the North Atlantic Ocean or the Southern Ocean (Dall’Olmo et al., 2016).
The water mass subduction induced by eddies also pumps POC into the mesopelagic zone. Omand et al. (2015) quantified the amount of suspended POC sinking following eddy driven subduction in the North Atlantic. In essence, suspended POC produced in surface sink along tilted isopycnals. Globally, eddy-driven subduction of POC is responsible for an export flux of ∼1 Gt C yr–1 (Omand et al., 2015). Altogether, these processes may export up to 2 Gt of POC yr–1, nearly a third of the gravitational flux (Boyd et al., 2019).
Large-scale circulation, mixing and eddies subduction also transport DOC beneath the surface ocean. Examining large scale variability of DOC concentrations, Hansell et al. (2009) estimated that DOC export represents 20% that of gravitational POC export flux in the global ocean. However, the amount of DOC transported by processes such as eddy driven subduction and mixed layer pump (see references above) have poor spatial coverage both on local and global scales. Using an artificial neural network, Roshan and DeVries (2017) reconstructed global DOC concentrations distribution. Here, DOC production and export fluxes were estimated by coupling reconstructed DOC concentrations to a global ocean circulation model (DeVries and Primeau, 2011). Large annual DOC export rates were shown in the tropics (50–80 mg of OC m–2 d–1) and low in high latitudes (0–40 mg of OC m–2 d–1), contrary to findings by Dall’Olmo et al. (2016) and Omand et al. (2015). Their model, however, extrapolated DOC concentrations measured during summer to a global scale. This may have limited the effect of the aforementioned physical processes. More work is required to estimate the annual flux of DOC from the surface ocean.
The global maps of the OC flux associated to the various mixing fluxes are not shown here but are available in the literature cited above.
The Active Migration Flux
The concept of active flux by migrating organisms (also known as “swimmers”) in the mesopelagic is based on widespread observations of DVM of zooplankton and fish caught in nets (Longhurst, 1991; Steinberg, 2000; Landry and Calbet, 2004). In essence, to avoid predation swimmers feed at night in surface waters and defecate deeper in the mesopelagic during the day (Steinberg and Landry, 2017). Inverted DVM and desynchronized vertical migration do exist (Neill, 1990; Cohen and Forward, 2016), however, typical DVM as described above are more common in marine systems. This actively supplies OC to depth with minimizing decomposition. To date, estimates of DVM have mostly been focused on mesozooplankton because they are readily caught in multiple opening/closing towed nets (Tarling et al., 2002). DVM is a difficult process to model (Hansen and Visser, 2016), however, this field has made significant progresses recently (Aumont et al., 2018; Archibald et al., 2019; Gorgues et al., 2019).
The active transport of OC by migrating mesozooplankton can contribute up to 40% of the gravitational flux (Stukel et al., 2013) with global estimates ranging from 0.2 to 71 mg m–2 d–1 (Aumont et al., 2018; Figure 1E and Supplementary Table S3). Most studies of mesozooplankton associated OC flux were conducted in coastal and upwelling regions, while data from high latitudes and polar waters are scarce (Figure 1E). The largest flux is recorded in the Benguela upwelling system while the smallest are observed near the Antarctic peninsula. Large DVM fluxes in upwelling system is counterintuitive given that these waters are productive but often poorly oxygenated below surface. However, some migrating organisms can survive with little oxygen concentrations by adopting adaptive metabolic strategies (Bianchi et al., 2013, 2014; Kiko et al., 2015). Excluding the extreme values of 0.2 and 71 mg m–2 d–1, the OC fluxes associated with migratory organisms appear to be relatively homogeneous and in the order of 9.8 ± 7.3 mg C m–2 d–1.
The OC flux associated to DVM of both macrozooplankton and fishes are, however, more challenging to estimate. These organisms are not always quantitatively represented in net catchments (Sameoto et al., 2007). Acoustics techniques are promising for estimating these (Lebourges-Dhaussy and Josse, 2008; Lebourges-Dhaussy et al., 2014) and dedicated surveys performed on large scales are starting to emerge (Bianchi et al., 2013; Irigoien et al., 2014). Most of the available observations involve mesozooplankton and/or micronekton. The whole community is, however, represented only by a limited number of datapoints (Aumont et al., 2018). Moreover, these fluxes mostly represent the active flux between the surface and the upper mesopelagic zone. DVM OC fluxes between surface and the lower mesopelagic zone are more rarely estimated. This is despite the presence of migrating species deeper down in the mesopelagic zone (Sutton, 2013; D’Elia et al., 2016).
Conclusion and Perspectives
In the last decade, numerous studies highlighted the importance and the complexity of the BCP. As our understanding of the BCP advances, new research directions into the functioning of the BCP also emerged building on knowledge about the chemical composition of the bulk export fluxes. For instance, the importance of the types, transport pathways, particles associated communities, and fates of sinking particles have become apparent thanks to technological breakthroughs, and thus need further research. Known processes of OC export from the surface ocean to the mesopelagic zone (100–1000 m) were reviewed. I provided a list of the published OC fluxes associated to the specific downward export pathways involving zooplankton fecal pellets, aggregates, and by vertical migrating organisms. However, while data on phytoplankton cell flux exist, estimates of the OC flux associated to sinking single cells are challenging. Moreover, processes responsible for the sinking of phytoplankton single cells are unclear. I report global estimates of the physically driven processes that supply slow sinking and/or neutrally buoyant and dissolved OC to the dark ocean. However, locally, their contribution relative to the total gravitational flux is often missing, especially in high latitude regions. In addition, most estimates of vertical migration concern only part of the community and organisms migrating deeper are overlooked.
Author Contributions
The author confirms being the sole contributor of this work and has approved it for publication.
Funding
This work was funded by the Deutsche Forschungsgemeinschaft (DFG, German Research Foundation) – Project Number: 422798570 and by the Hanse-Wissenschaftskolleg, Delmenhorst.
Conflict of Interest
The author declares that the research was conducted in the absence of any commercial or financial relationships that could be construed as a potential conflict of interest.
Acknowledgments
I would like to thank the Cindy Lee, Stuart Wakeham, Kai-Uwe Hinrichs, Carol Arnosti, Anne Pearson, and Thorsten Dittmar for organizing the Marine Organic Biogeochemistry workshop held at the Hanse-Wissenschaftskolleg, Delmenhorst, Germany, April 27 to 30th 2019. I thank Emmanuel Laurenceau-Cornec (University of Tasmania, Institute for Marine and Antarctic Studies, Hobart, Australia), Katsiaryna Pabortsava (NOC, Southampton, United-Kingdom), and Morten Iversen (MARUM, AWI, Bremerhaven, Germany) for advices on aggregates fluxes, Olivier Aumont (IRD, LOCEAN, Paris, France) for sharing data on migration fluxes and the two reviewers for their constructive comments.
Supplementary Material
The Supplementary Material for this article can be found online at: https://www.frontiersin.org/articles/10.3389/fmars.2019.00634/full#supplementary-material
References
Agusti, S., González-Gordillo, J. I., Vaqué, D., Estrada, M., Cerezo, M. I., Salazar, J., et al. (2015). Ubiquitous healthy diatoms in the deep sea confirm deep carbon injection by the biological pump. Nat. Commun. 6:7608. doi: 10.1038/ncomms8608
Alldredge, A. (1998). The carbon, nitrogen and mass content of marine snow as a function of aggregate size. Deep. Res. I 45, 529–541. doi: 10.1016/s0967-0637(97)00048-4
Alldredge, A. L., and Crocker, K. M. (1995). Why do sinking mucilage aggregates accumulate in the water column. Sci. Total Environ. 165, 15–22. doi: 10.1016/0048-9697(95)04539-d
Alldredge, A. L., and Jackson, G. A. (1995). Aggregation in marine systems - preface. Deep. Res. Part II Top. Stud. Oceanogr. 42, 1–7.
Archibald, K. M., Siegel, D. A., and Doney, S. C. (2019). Modeling the impact of zooplankton diel vertical migration on the carbon export flux of the biological pump. Global Biogeochem. Cycles 33, 181–199. doi: 10.1029/2018gb005983
Aumont, O., Maury, O., Lefort, S., and Bopp, L. (2018). Evaluating the potential impacts of the diurnal vertical migration by marine organisms on marine biogeochemistry. Global Biogeochem. Cycles 32, 1622–1643. doi: 10.1029/2018GB005886
Belcher, A., Manno, C., Thorpe, S., and Tarling, G. (2018). Acantharian cysts: high flux occurrence in the bathypelagic zone of the Scotia Sea. Southern Ocean. Mar. Biol. 165:117. doi: 10.1007/s00227-018-3376-1
Bianchi, D., Babbin, A. R., and Galbraith, E. D. (2014). Enhancement of anammox by the excretion of diel vertical migrators. Proc. Natl. Acad. Sci. U.S.A. 111, 15653–15658. doi: 10.1073/pnas.1410790111
Bianchi, D., Galbraith, E. D., Carozza, D. A., Mislan, K. A. S., and Stock, C. A. (2013). Intensification of open-ocean oxygen depletion by vertically migrating animals. Nat. Geosci. 6, 545–548. doi: 10.1038/ngeo1837
Boyd, P. W., Claustre, H., Levy, M., Siegel, D. A., and Weber, T. (2019). Multi-faceted particle pumps drive carbon sequestration in the ocean. Nature 568, 327–335. doi: 10.1038/s41586-019-1098-2
Burd, A., Hansell, D. A., Steinberg, D. K., Anderson, T. R., Aristegui, J., Baltar, F., et al. (2010). Assessing the apparent imbalance between geochemical and biochemical indicators of meso- and bathypelagic biological activity: what the @$# is wrong with present calculations of carbon budgets? Deep sea Res. II 57, 1557–1571. doi: 10.1016/j.dsr2.2010.02.022
Carroll, M. L., Miquel, J. C., and Fowler, S. W. (1998). Seasonal patterns and depth-specific trends of zooplankton fecal pellet fluxes in the northwestern Mediterranean Sea. Deep. Res. Part I Oceanogr. Res. Pap. 45, 1303–1318. doi: 10.1016/S0967-0637(98)00013-2
Cavan, E., Le Moigne, F. A. C., Poulton, A. J., Daniels, C. J., Fragoso, G., and Sanders, R. J. (2015). Zooplankton fecal pellets control the attenuation of particulate organic carbon flux in the Scotia Sea, Southern Ocean. Geophys. Res. Lett. 42, 821–830. doi: 10.1002/2014GL062744
Cavan, E., Trimmer, M., Shelley, F., and Sanders, R. (2017). Remineralization of particulate organic carbon in an ocean oxygen minimum zone. Nat. Commun. 8:14847. doi: 10.1038/ncomms14847
Claustre, H., Antoine, D., Boehme, L., Boss, E., D’Ortenzio, F., Fanton, D., et al. (2011). “Guidelines towards an integrated ocean observation system for ecosystems and biogeochemical cycles,” in Proceedings of the OceanObs’09: Sustained Ocean Observations and Information for Society, Vol. 1, (Venice: ESA Publication), 21–25.
Cohen, J. H., and Forward, R. B. (2016). Zooplankton diel vertical migration - a review of proximate control. Oceanogr. Mar. Biol. 47, 77–109. doi: 10.1201/9781420094220.ch2
Collins, J. R., Edwards, B. R., Thamatrakoln, K., Ossonlinski, J. E., DiTullio, G. R., Bidle, K. D., et al. (2015). The multiple fates of sinking particles in the North Atlantic Ocean. Global Biogeochem. Cycles 29, 1471–1494. doi: 10.1002/2014gb005037
Dall’Olmo, G., Dingle, J., Polimene, L., Brewin, R. J. W., and Claustre, H. (2016). Substantial energy input to the mesopelagic ecosystem from the seasonal mixed-layer pump. Nat. Geosci. 9, 820–823. doi: 10.1038/NGEO2818
Davidson, A. T., and Marchant, H. J. (1992). Protist abundance and carbon concentration during a phaeocystis-dominated bloom at an antarctic coastal site. Polar Biol. 12, 387–395.
Decelle, J., Martin, P., Pabortsava, K., Pond, D. W., Tarling, G., Mahe, F., et al. (2013). Diversity, ecology and biogeochemistry of cyst-forming acantharia (Radiolaria) in the oceans. PLoS One 8:e53598. doi: 10.1371/journal.pone.0053598
D’Elia, M., Warren, J. D., Rodriguez-Pinto, I., Sutton, T. T., Cook, A., and Boswell, K. M. (2016). Diel variation in the vertical distribution of deep-water scattering layers in the Gulf of Mexico. Deep. Res. Part I Oceanogr. Res. Pap. 115, 91–102. doi: 10.1016/j.dsr.2016.05.014
DeVries, T., and Primeau, F. (2011). Dynamically and observationally constrained estimates of water-mass distributions and ages in the global ocean. J. Phys. Oceanogr. 41, 2381–2401. doi: 10.1175/jpo-d-10-05011.1
DiTullio, G. R., Grebmeier, J. M., Arrigo, K. R., Lizotte, M. P., Robinson, D. H., Leventer, A., et al. (2000). Rapid and early export of Phaeocystis antarctica blooms in the Ross Sea, Antarctica. Nature 404, 595–598. doi: 10.1038/35007061
Ebersbach, F., and Trull, T. W. (2008). Sinking particle properties from polyacrylamide gels during the kerguelen ocean and plateau compared study (KEOPS): zooplankton control of carbon export in an area of persistent natural iron inputs in the Southern Ocean. Limnol. Oceanogr. 53, 212–224. doi: 10.4319/lo.2008.53.1.0212
Ebersbach, F., Trull, T. W., Davies, D. M., and Bray, S. G. (2011). Controls on mesopelagic particle fluxes in the Sub-Antarctic and Polar Frontal Zones in the Southern Ocean south of Australia in summer – Perspectives from free-drifting sediment traps. Deep Sea Res. II 58, 2260–2276. doi: 10.1016/j.dsr2.2011.05.025
Edwards, B. R., Bidle, K. D., and Van Mooy, B. A. S. (2015). Dose dependent regulation of microbial activity on sinking particles by polyunsaturated aldehydes: implications for the carbon cycle. PNAS 112, 5909–5914. doi: 10.1073/pnas.1422664112
Gardner, W. D., Chung, S. P., Richardson, M. J., and Walsh, I. D. (1995). The oceanic mixed-layer pump. Deep Sea Res. Part II Top. Stud. Oceanogr. 42, 757–765.
Giering, S. L. C., Sanders, R. J., Lampitt, R. S., Anderson, T. R., Tamburini, C., Boutrif, M., et al. (2014). Reconciliation of the carbon budget in the ocean’s twilight zone. Nature 507, 480–483. doi: 10.1038/nature13123
Gorgues, T., Aumont, O., and Memery, L. (2019). Simulated changes in the particulate carbon export efficiency due to diel vertical migration of zooplankton in the North Atlantic. Geophys. Res. Lett. 46, 5387–5395. doi: 10.1029/2018GL081748
Gosselain, V., Hamilton, P. B., and Descy, J. P. (2000). Estimating phytoplankton carbon from microscopic counts: an application for riverine systems. Hydrobiologia. 438, 75–90. doi: 10.1023/A:1004161928957
Guidi, L., Chaffron, S., Bittner, L., Eveillard, D., Larhlimi, A., Roux, S., et al. (2016). Plankton networks driving carbon export in the oligotrophic ocean. Nature 532, 465–470. doi: 10.1038/nature16942
Gutknecht, E., and Perruche, C. (2016). “Evaluation de la configuration globale Mercator Océan à partir des données Bio-Argo,” in Paper presented at the Annuel Meeting of the NAOS 21-22/09/2016, Belgium.
Hansell, D. A., Carlson, C. A., Repeta, D. J., and Schlitzer, R. (2009). Dissolved organic matter in the ocean: a controversy stimulates New Insights. Oceanography 22, 202–211. doi: 10.5670/oceanog.2009.109
Hansen, A. N., and Visser, A. W. (2016). Carbon export by vertically migrating zooplankton: an optimal behavior model. Limnol. Oceanogr. 61, 701–710. doi: 10.1002/lno.10249
Irigoien, X., Klevjer, T. A., Røstad, A., Martinez, U., Boyra, G., Acuña, J. L., et al. (2014). Large mesopelagic fishes biomass and trophic efficiency in the open ocean. Nat. Commun. 5:3271. doi: 10.1038/ncomms4271
Iversen, M. H., and Poulsen, L. K. (2007). Coprorhexy, coprophagy, and coprochaly in the copepods Calanus helgolandicus, Pseudocalanus elongatus, and Oithona similis. Mar. Ecol. Prog. Ser. 350, 79–89. doi: 10.3354/meps07095
Kiko, R., Hauss, H., Dengler, M., Sommer, S., and Melzner, F. (2015). The squat lobster Pleuroncodes monodon tolerates anoxic “dead zone” conditions off Peru. Mar. Biol. 162, 1913–1921. doi: 10.1007/s00227-015-2709-6
Kwon, E. Y., Primeau, F., and Sarmiento, J. L. (2009). The impact of remineralization depth on the air-sea carbon balance. Nat. Geosci. 2, 630–635. doi: 10.1038/ngeo612
Lam, P. J., and Marchal, O. (2015). Insights into particle cycling from thorium and particle data. Ann. Rev. Mar. Sci. 7, 159–184. doi: 10.1146/annurev-marine-010814-015623
Landry, M. R., and Calbet, A. (2004). Microzooplankton production in the oceans. ICES J. Mar. Sci. 61, 501–507. doi: 10.1016/j.icesjms.2004.03.011
Laurenceau-Cornec, E. C., Trull, T. W., Davies, D. M., Bray, S. G., Doran, J., Planchon, F., et al. (2015). The relative importance of phytoplankton aggregates and zooplankton fecal pellets to carbon export: insights from free-drifting sediment trap deployments in naturally iron-fertilised waters near the Kerguelen Plateau. Biogeosciences 12, 1007–1027. doi: 10.5194/bg-12-1007-2015
Leblanc, K., Quéguiner, B., Diaz, F., Cornet, V., Michel-Rodriguez, M., Durrieu De Madron, X., et al. (2018). Nanoplanktonic diatoms are globally overlooked but play a role in spring blooms and carbon export. Nat. Commun. 9: 953. doi: 10.1038/s41467-018-03376-9
Lebourges-Dhaussy, A., Huggett, J., Ockhuis, S., Roudaut, G., Josse, E., and Verheye, H. (2014). Zooplankton size and distribution within mesoscale structures in the Mozambique Channel: a comparative approach using the TAPS acoustic profiler, a multiple net sampler and ZooScan image analysis. Deep. Res. Part II Top. Stud. Oceanogr. 100, 136–152. doi: 10.1016/j.dsr2.2013.10.022
Lebourges-Dhaussy, A., and Josse, E. (2008). Acoustical methods that provide an integrated view of the marine ecosystem. J. Acoust. Soc. Am. 123:2992. doi: 10.1121/1.2932534
Le Moigne, F. A. C., Cisternas-Novoa, C., Laurenceau-Cornec, E. C., and Engel, A. (2018). “Pathways of gravitational particle export in the peruvian OMZ,” in Proceedings of the Ocean Deoxygenation Conference, (Kiel: Kiel University).
Le Moigne, F. A. C., Henson, S. A., Cavan, E., Georges, C., Pabortsava, K., Achterberg, E. P., et al. (2016). What causes the inverse relationships between primary production and export efficiency in the southern ocean? Geophys. Res. Lett. 43, 4457–4466. doi: 10.1002/2016GL068480
Levy, M., Bopp, L., Karleskind, P., Resplandy, L., Ethe, C., and Pinsard, F. (2013). Physical pathways for carbon transfers between the surface mixed layer and the ocean interior. Global Biogeochem. Cycles. 27, 1001–1012. doi: 10.1002/gbc.20092
Longhurst, A. R. (1991). Large marine ecosystems - patterns, processes and yields. Mar. Policy 15, 377–378.
Lundsgaard, C. (1995). “Use of a high viscosity medium in studies of aggregates,” in Sediment trap studies in the Nordic countries 3. Proceedings of the Symposium on Seasonal Dynamics of Planktonic Ecosystems and Sedimentation in Coastal Nordic Waters, eds S. Floderus, A. S. Heisakanen, M. Oleson, and P. Wassman, (Helsinki: Finnish Environment Agency), 141–152.
Martin, P., Allen, J. T., Cooper, M. J., Johns, D. G., Lampitt, R. S., Sanders, R., et al. (2010). Sedimentation of acantharian cysts in the Iceland Basin: strontium as a ballast for deep ocean particle flux, and implications for acantharian reproductive strategies. Limnol. Oceanogr. 55, 604–614. doi: 10.4319/lo.2009.55.2.0604
McDonnell, A. M. P., Lam, P. J., Lamborg, C. H., Buesseler, K. O., Sanders, R., and Riley, J. S. (2015). The oceanographic toolbox for the collection of sinking and suspended marine particles. Prog. Oceanogr. 133, 17–31. doi: 10.1016/j.pocean.2015.01.007
Neill, W. E. (1990). Induced vertical migration in copepods as a defence against invertebrate predation. Nature 345, 524–526. doi: 10.1038/345524a0
Omand, M. M., D’asaro, E., Lee, C., Perry, M. J., Briggs, N., Cetini, I., et al. (2015). Eddy-driven subduction exports particulate organic carbon from the spring bloom. Science 348, 222–225. doi: 10.1126/science.1260062
Pabortsava, K., Lampitt, R. S., Benson, J., Crowe, C., McLachlan, R., Le Moigne, F. A. C., et al. (2017). Carbon sequestration in the deep Atlantic enhanced by Saharan dust. Nat. Geosci. 10, 189–194. doi: 10.1038/ngeo2899
Parekh, P., Dutkiewicz, S., Follows, M. J., and Ito, T. (2006). Atmospheric carbon dioxide in a less dusty world. Geophys. Res. Lett. 33:L03610. doi: 10.1029/2005GL025098
Picheral, M., Guidi, L., Stemmann, L., Karl, D. M., Iddaoud, G., and Gorsky, G. (2010). The underwater vision profiler 5: an advanced instrument for high spatial resolution studies of particle size spectra and zooplankton. Limnol. Oceanogr. Methods 8, 462–473. doi: 10.4319/lom.2010.8.462
Piontek, J., Sperling, M., Nothig, E. M., and Engel, A. (2014). Regulation of bacterioplankton activity in Fram Strait (Arctic Ocean) during early summer: the role of organic matter supply and temperature. J. Mar. Syst. 132, 83–94. doi: 10.1016/j.jmarsys.2014.01.003
Proud, R., Handegard, N. O., Kloser, R. J., Cox, M. J., and Brierley, A. S. (2019). From siphonophores to deep scattering layers: uncertainty ranges for the estimation of global mesopelagic fish biomass. ICES J. Mar. Sci. 76, 718–733. doi: 10.1093/icesjms/fsy037
Refaeli, Y., Young, R. M., Turner, B. C., Duda, J., Field, K. A., and Bishop, J. M. (2008). The B cell antigen receptor and overexpression of MYC can cooperate in the genesis of B cell lymphomas. PLoS Biol. 6:e152. doi: 10.1371/journal.pbio.0060152
Rembauville, M., Manno, C., Tarling, G. A., Blain, S., and Salter, I. (2016). Strong contribution of diatom resting spores to deep-sea carbon transfer in naturally iron-fertilized waters downstream of South Georgia. Deep Sea Res. I 115, 22–35. doi: 10.1016/j.dsr.2016.05.002
Riley, J., Sanders, R., Marsay, C., Le Moigne, F. A. C., Achterberg, E., and Poulton, A. (2012). The relative contribution of fast and slow sinking particles to ocean carbon export. Global Biogeochem. Cycles 26:GB1026. doi: 10.1029/2011GB004085
Riou, V., Para, J., Garel, M., Guigue, C., Al Ali, B., Santinelli, C., et al. (2017). Biodegradation of Emiliania huxleyi aggregates by natural prokaryotic communities under increasing hydrostatic pressure. Prog. Oceanogr. 163, 271–281. doi: 10.1016/j.pocean.2017.01.005
Roshan, S., and DeVries, T. (2017). Efficient dissolved organic carbon production and export in the oligotrophic ocean. Nat. Commun. 8:2036. doi: 10.1038/s41467-017-02227-3
Salter, I., Lampitt, R. S., Sanders, R., Poulton, A., Kemp, A. E. S., Boorman, B., et al. (2007). Estimating carbon, silica and diatom export from a naturally fertilised phytoplankton bloom in the Southern Ocean using PELAGRA: a novel drifting sediment trap. Deep. Res. Part Ii-Topical Stud. Oceanogr. 54, 2233–2259. doi: 10.1016/j.dsr2.2007.06.008
Sameoto, D., Wiebe, P., Runge, J., Postel, L., Dunn, J., Miller, C., et al. (2007). “Collecting zooplankton,” in ICES Zooplankton Methodology Manual, eds R. Harris, P. Wiebe, J. Lenz, H. R. Skjoldal, and M. Huntley, (San Diego, CA: Academic Press).
Sarmiento, J. L., Slater, R., Barber, R., Bopp, L., Doney, S. C., Hirst, A. C., et al. (2004). Response of ocean ecosystems to climate warming. Global Biogeochem. Cycles 18:GB3003. doi: 10.1029/2003GB002134
Shatova, O., Koweek, D., Conte, M. H., and Weber, J. C. (2012). Contribution of zooplankton fecal pellets to deep ocean particle flux in the Sargasso Sea assessed using quantitative image analysis. J. Plankton Res. 34, 905–921. doi: 10.1093/plankt/fbs053
Siegel, D. A., Buesseler, K. O., Behrenfeld, M. J., Benitez-Nelson, C. R., Boss, E., Brzezinski, M. A., et al. (2016). Prediction of the export and fate of global ocean net primary production: the EXPORTS science plan. Front. Mar. Sci. 3:22. doi: 10.3389/fmars.2016.00022
Steinberg, D. K. (2000). Zooplankton vertical migration and the active transport of dissolved organic and inorganic carbon in the Sargasso Sea. Deep Sea Res. Part I Oceanogr. Res. Pap. 47, 137–158. doi: 10.1016/s0967-0637(99)00052-7
Steinberg, D. K., and Landry, M. R. (2017). Zooplankton and the ocean carbon cycle. Ann. Rev. Mar. Sci. 9, 413–444. doi: 10.1146/annurev-marine-010814-015924
Steinberg, D. K., Van Mooy, B. A. S., Buesseler, K. O., Boyd, P. W., Kobari, T., and Karl, D. M. (2008). Bacterial vs. zooplankton control of sinking particle flux in the ocean’s twilight zone. Limnol. Oceanogr. 53, 1327–1338. doi: 10.4319/lo.2008.53.4.1327
Stemmann, L., Jackson, G. A., and Ianson, D. (2004). A vertical model of particle size distributions and fluxes in the midwater column that includes biological and physical processes - Part I: model formulation. Deep. Res. Part I Oceanogr. Res. Pap. 51, 885–908. doi: 10.1016/j.dsr.2004.03.001
Stokes, G. G. (1851). On the effect of the internal friction of fluids on the motion of pendulums. Trans. Cambridge Philos. Soc. 9, 8–106. doi: 10.1017/cbo9780511702266.002
Stukel, M. R., Ohman, M. D., Benitez-Nelson, C. R., and Landry, M. R. (2013). Contributions of mesozooplankton to vertical carbon export in a coastal upwelling system. Mar. Ecol. Prog. Ser. 491, 47–65. doi: 10.3354/meps10453
Sutton, T. (2013). Vertical ecology of the pelagic ocean: classical patterns and new perspectives. J. Fish Biol. 83, 1508–1527. doi: 10.1111/jfb.12263
Tamburini, C., Garcin, J., Gregori, G., Leblanc, K., Rimmelin, P., and Kirchman, D. L. (2006). Pressure effects on surface mediterranean prokaryotes and biogenic silica dissolution during a diatom sinking experiment. Aquat. Microb. Ecol. 43, 267–276. doi: 10.3354/ame043267
Tarling, G. A., Jarvis, T., Emsley, S. M., and Matthews, J. B. L. (2002). Midnight sinking behaviour in Calanus finmarchicus: a response to satiation or krill predation? Mar. Ecol. Prog. Ser. 240, 183–194. doi: 10.3354/meps240183
Taucher, J., Bach, L., Riebesell, U., and Oschlies, A. (2014). The viscosity effect on marine particle flux: a climate relevant feedback mechanism. Global Biogeochem. Cycles 28, 415–422. doi: 10.1002/2013GB004728
Turley, C. M., and Mackie, P. J. (1994). Biogeochemical significance of attached and free-living bacteria and the flux of particles in the NE Atlantic Ocean. Mar. Ecol. Prog. Ser. 115, 191–203. doi: 10.3354/meps115191
Turner, J. T. (2002). Zooplankton fecal pellets, marine snow and sinking phytoplankton blooms. Aquat. Microb. Ecol. 27, 57–102. doi: 10.3354/ame027057
Turner, J. T. (2015). Zooplankton fecal pellets, marine snow, phytodetritus and the ocean’s biological pump. Prog. Oceanogr. 130, 205–248. doi: 10.1016/j.pocean.2014.08.005
Waite, A. M., Safi, K. A., Hall, J. A., and Nodder, S. D. (2000). Mass sedimentation of picoplankton embedded in organic aggregates. Limnol. Oceanogr. 45, 87–97. doi: 10.4319/lo.2000.45.1.0087
Wilson, S. E., Steinberg, D. K., and BUesseler, K. O. (2008). Changes in fecal pellet characteristics with depth as indicators of zooplankton repackaging of particles in the mesopelagic zone of the subtropical and subarctic North Pacific Ocean. Deep Sea Res. II 55, 1636–1647. doi: 10.1016/j.dsr2.2008.04.019
Keywords: plankton, biogeochemical processes, carbon cycle, ecological process, biological carbon pump
Citation: Le Moigne FAC (2019) Pathways of Organic Carbon Downward Transport by the Oceanic Biological Carbon Pump. Front. Mar. Sci. 6:634. doi: 10.3389/fmars.2019.00634
Received: 02 July 2019; Accepted: 27 September 2019;
Published: 09 October 2019.
Edited by:
Stuart Wakeham, University of Georgia, United StatesReviewed by:
Emma Louise Cavan, University of Tasmania, AustraliaAdrian Benedict Burd, University of Georgia, United States
Copyright © 2019 Le Moigne. This is an open-access article distributed under the terms of the Creative Commons Attribution License (CC BY). The use, distribution or reproduction in other forums is permitted, provided the original author(s) and the copyright owner(s) are credited and that the original publication in this journal is cited, in accordance with accepted academic practice. No use, distribution or reproduction is permitted which does not comply with these terms.
*Correspondence: Frédéric A. C. Le Moigne, ZnJlZGVyaWMubGVtb2lnbmVAbWlvLm9zdXB5dGhlYXMuZnI=