- 1Department of Medicine, Duke University, Durham, NC, United States
- 2Department of Orthopaedics, Duke University, Durham, NC, United States
- 3Duke Cancer Institute, Duke University, Durham, NC, United States
- 4Department of Biological Sciences, North Carolina State University, Raleigh, NC, United States
- 5Division of Marine Science and Conservation, Nicholas School of the Environment, Duke University, Durham, NC, United States
Plastic waste has reached epidemic proportions worldwide, and the production of plastic continues to rise steadily. Plastic represents a diverse array of commonly used synthetic polymers that are extremely useful as durable, economically beneficial alternatives to other materials; however, despite the wide-ranging utility of plastic, the increasing accumulation of plastic waste in the environment has had numerous detrimental impacts. In particular, plastic marine debris can transport invasive species, entangle marine organisms, and cause toxic chemical bioaccumulation in the marine food web. The negative impacts of plastic waste have motivated research on new ways to reduce and eliminate plastic. One unique approach to tackle the plastic waste problem is to turn to nature’s solutions for degrading polymers by leveraging the biology of naturally occurring organisms to degrade plastic. Advances in metagenomics, next generation sequencing, and bioengineering have provided new insights and new opportunities to identify and optimize organisms for use in plastic bioremediation. In this review, we discuss the plastic waste problem and possible solutions, with a focus on potential mechanisms for plastic bioremediation. We pinpoint two key habitats to identify plastic-biodegrading organisms: (1) habitats with distinct enrichment of plastic waste, such as those near processing or disposal sites, and (2) habitats with naturally occurring polymers, such as cutin, lignin, and wax. Finally, we identify directions of future research for the isolation and optimization of these methods for widespread bioremediation applications.
Introduction
The Plastic Epidemic
Plastics are an engineering and economic marvel. These synthetic, malleable polymers are cheap and easily manufactured, with diverse material properties and versatile applications. Since their popularization in the 1950s, plastic products ranging from nylon parachutes to polyethylene terephthalate (PET) water bottles have provided societal health, safety, and energy benefits (Andrady and Neal, 2009). While initially lauded for their resistance to degradation, this very property of recalcitrance also manifests in an epidemic of unmanaged waste. Plastics make up the fourth largest category for global solid waste (Hoornweg and Perinaz, 2012). In the first global analysis of all mass-produced plastics ever made, researchers presented staggering statistics: Over 5.8 trillion kilograms of plastic waste have been produced since 1950, with half of that occurring between 2004 and 2017 (Geyer et al., 2017). Perhaps more significantly, the rapid acceleration in production and waste trends suggest that 12 trillion kilograms of plastic waste will accumulate in landfills and the natural environment by 2050 (Neufeld et al., 2016; Geyer et al., 2017).
In the ocean, plastic products enter the ocean at a staggering rate, estimated at between 4.8 and 12.7 million metric tons per year (Jambeck et al., 2015). In total, one study estimates that 5.25 trillion particles weighing 268,940 tons are floating in the world’s ocean (Eriksen et al., 2014). This debris, ranging in size from microbeads less than one millimeter in diameter to large fishing nets (Law, 2017), spreads throughout water columns and across the oceans, impacting all ecological systems (Rochman et al., 2016; Cózar et al., 2017; Chiba et al., 2018). For instance, debris can entangle marine organisms (Gregory, 2009), transport invasive species (Gregory, 2009; Carlton et al., 2017) and cause toxic chemical bioaccumulation up the food chain through ingestion of plastic waste (Eriksson and Burton, 2003; Rochman et al., 2013; Setälä et al., 2014). In fact, a recent study estimates that the average American consumes at least 39,000–52,000 microplastic particles just from their drinking water (Cox et al., 2019). Plastics have also been associated with a range of health hazards in humans. For example, exposure to vinyl chloride, the monomer of polyvinyl chloride (PVC), has long been linked with increased cancer risk for workers in PVC manufacturing plants (Infante et al., 1976; Bosetti et al., 2003; Lopez et al., 2003; Kumar et al., 2013). High levels of vinyl chloride have been found near waste disposal sites (Brandt-Rauf et al., 2012). Additionally, Bisphenol A (BPA) is a carcinogenic compound found in plastics (Wang et al., 2017). BPA is an endocrine disrupter, and exposure to it can contribute to breast cancer development (Rubin, 2011; Wang et al., 2017). Despite its potential hazards, plastic demand and disposal continues to increase world-wide, outpacing all other manufactured materials and continuing to put human and environmental health at risk (Geyer et al., 2017). According to the International Agency for Research on Cancer, over 40 compounds used in plastic manufacturing have proven or suspected carcinogenicity in humans (Table 1). Among these compounds are monomers, hardeners, curing agents, chemical intermediates, and others. While none of the polymers themselves are carcinogenic, the reagents used during plastic manufacturing have the potential to enter the environment and impact surrounding ecological systems (International Agencey for Research on Cancer, 1977, 1986, 1990, 1993, 1994, 1995, 1999a,b, 2000, 2002, 2006, 2008, 2010, 2012a,b, 2013, 2014, 2017, 2018).
Nature’s Circular Degradation Economy
Biodegradation refers to the disintegration of materials by biological means. In nature, vastly diverse ecosystems of biodegrading macro- and microorganisms are vital to ecosystem health (Prosser et al., 2007). As these organisms metabolize complex organic matter, including waste and dead plants and animals, these degraders return nutrients to the environment where they can be used by other organisms (Cromack, 1981). With their ubiquity and ability to adapt to even the most extreme environments, microbes facilitate a global “circular economy” of organic resources (Horner-Devine et al., 2004). This stands in stark contrast to the plastic industry’s established linear model of “make, use, dispose,” with the consumer having the option to add “recycle.”
Plastic Biodegradation Is a Complex, Step-Wise Process
Just as with the biodegradation of naturally occurring polymers (Austin and Vivanco, 2006), biodegradation of plastics depends on complex interactions between environmental and biotic factors at multiple stages (Figure 1). In general, abiotic factors like photo-oxidation, UV-irradiation (Orr et al., 2004; Esmaeili et al., 2013; Gewert et al., 2015), and temperature (Lucas et al., 2008) impact the molecular structure of the plastic, affecting susceptibility to microbial attack. Meanwhile, different biotic factors, such as enzymes or microorganisms, can act at each of the three stages of biodegradation: biodeterioration, biofragmentation, and assimilation (Lucas et al., 2008). During biodeterioration, polymer properties are modified for colony and biofilm growth. This step can involve secretion of adhesives or biosurfactants (Koutny et al., 2009) or rapid adaptation of organisms to the plastic niche (Tribedi et al., 2015). During biofragmentation, the polymer is converted to oligomers and monomers, often by secreted enzymes. Finally, assimilation may occur when an organism metabolizes the fragments or transforms them into carbon dioxide and water.
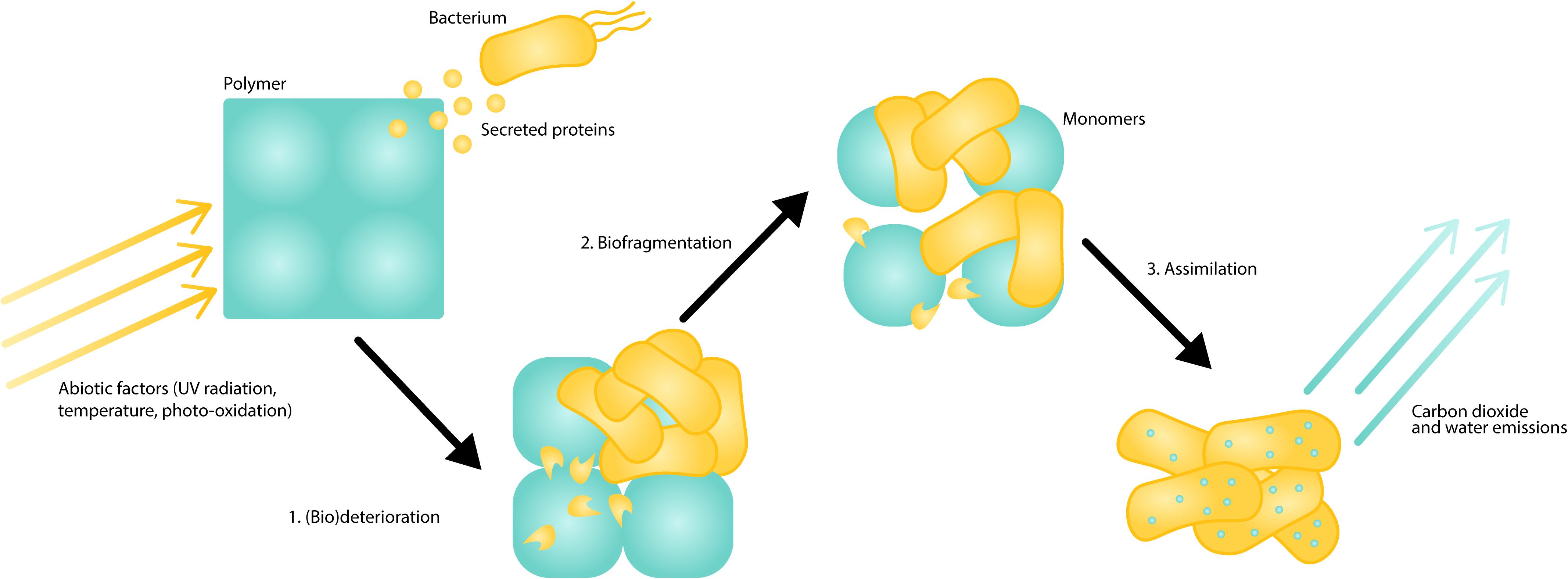
Figure 1. Schematic illustration of plastic biodegradation as a complex, step-wise pathway. First, during biodeterioration (1), abiotic and biotic factors, like light and secreted hydrophobins, modify the polymer surface and increase its surface area. Next, during biofragmentation (2), organisms secrete enzymes that break the polymer down into its monomer components. Finally, during assimilation (3), the organism metabolizes the monomers into biomass, carbon dioxide, and water.
Some industries have begun experimenting with cradle-to-cradle design inspired by natural resource cycling (McDonough, 2002), developing “biodegradable” or “compostable” plastic alternatives that may be degraded by the action of microorganisms (Song et al., 2009). While inventing new materials is a critical aspect for the future reduction of plastic pollution, it is not effective at eradicating the currently-existing plastic waste (Thompson et al., 2009). In addition, biodegradable petroleum-based plastics may have their own harmful environmental impacts: if they ultimately degrade anaerobically in landfills instead of aerobically in compost, they release methane instead of carbon dioxide, thereby contributing to climate change (Gómez and Michel, 2013). Although the design of fully biodegradable plastics will be advantageous, there is an urgent need to develop sustainable, effective, and convenient methods to remediate plastic waste.
As evident by the scale of plastic pollution, current options are not sufficient. Incineration releases detrimental greenhouse gases (Astrup et al., 2009) and other toxic substances (Gilpin et al., 2005). Landfills accumulate staggering quantities of plastic (Barnes et al., 2009), leach contaminants into the environment (Teuten et al., 2009), and emit greenhouse gases when exposed to ambient solar radiation (Royer et al., 2018). Recycling addresses only a limited selection of plastics, leading to costly collection and sorting challenges (Hopewell et al., 2009). For example, in the United States, only 9% of all recyclable plastics are actually recycled (Geyer et al., 2017), and just 5% of plastics are retained in the recycling stream (Neufeld et al., 2016). Therefore, the development and implementation of new biodegradation and bioremediation technologies have unrealized potential as more effective, less impactful pathways to improve existing methods of plastic disposal and recycling efforts. One of the most promising recent discoveries is the presence of organisms capable of degrading and transforming plastics of different kinds. These organisms represent powerful new tools in the fight to reduce and ultimately eliminate plastic waste. Below, we review the existing literature on plastic-degrading organisms and highlight areas of focus for future discovery and bioengineering efforts.
Looking Where They Are Most Expected: Plastic-Degrading Organisms Are Enriched in Specific Habitats
Designing a biodegradation system that accounts for dozens of interacting, variable environmental and polymer factors presents an immense challenge. Luckily, nature offers an elegant solution: a multitude of fungi and bacteria, along with several associated enzymes, that have been isolated and characterized to biodegrade recalcitrant, conventional plastics (Figure 2 and Supplementary Table S1). These components are extremely valuable as starting points for both the building blocks for unique combinations of organisms or enzymes and for bioengineering-based optimization strategies. However, review of the existing literature reveals that the search for naturally occurring, plastic-biodegrading organisms may have only scratched the surface of microbial biodiversity, with isolations thus far occurring almost exclusively in India and Japan (Figure 3). Yet, despite the potentially limited nature of the current search for these organisms, the available data pinpoint two habitats in which to focus for the identification of new plastic-degrading species or strains: (1) habitats that contain natural polymers; and (2) habitats that are enriched with plastic waste.
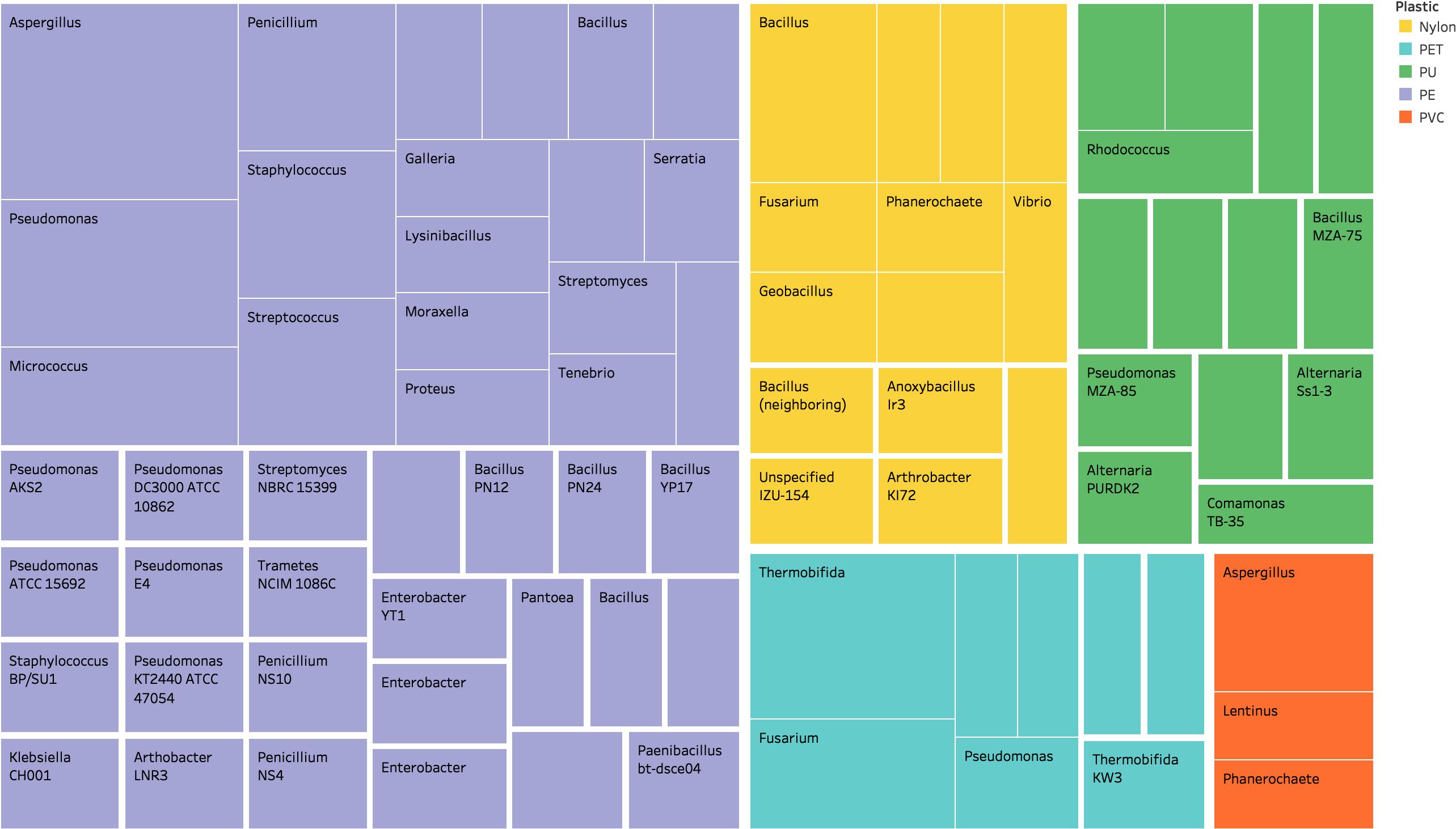
Figure 2. Many organisms have been isolated that have the potential to biodegrade specific plastics. The distribution of these organisms by genus is represented above, with the size of each block corresponding to the number of strains and color corresponding to the plastic it can degrade. For a comprehensive listing of organisms, refer to Supplementary Table S1.
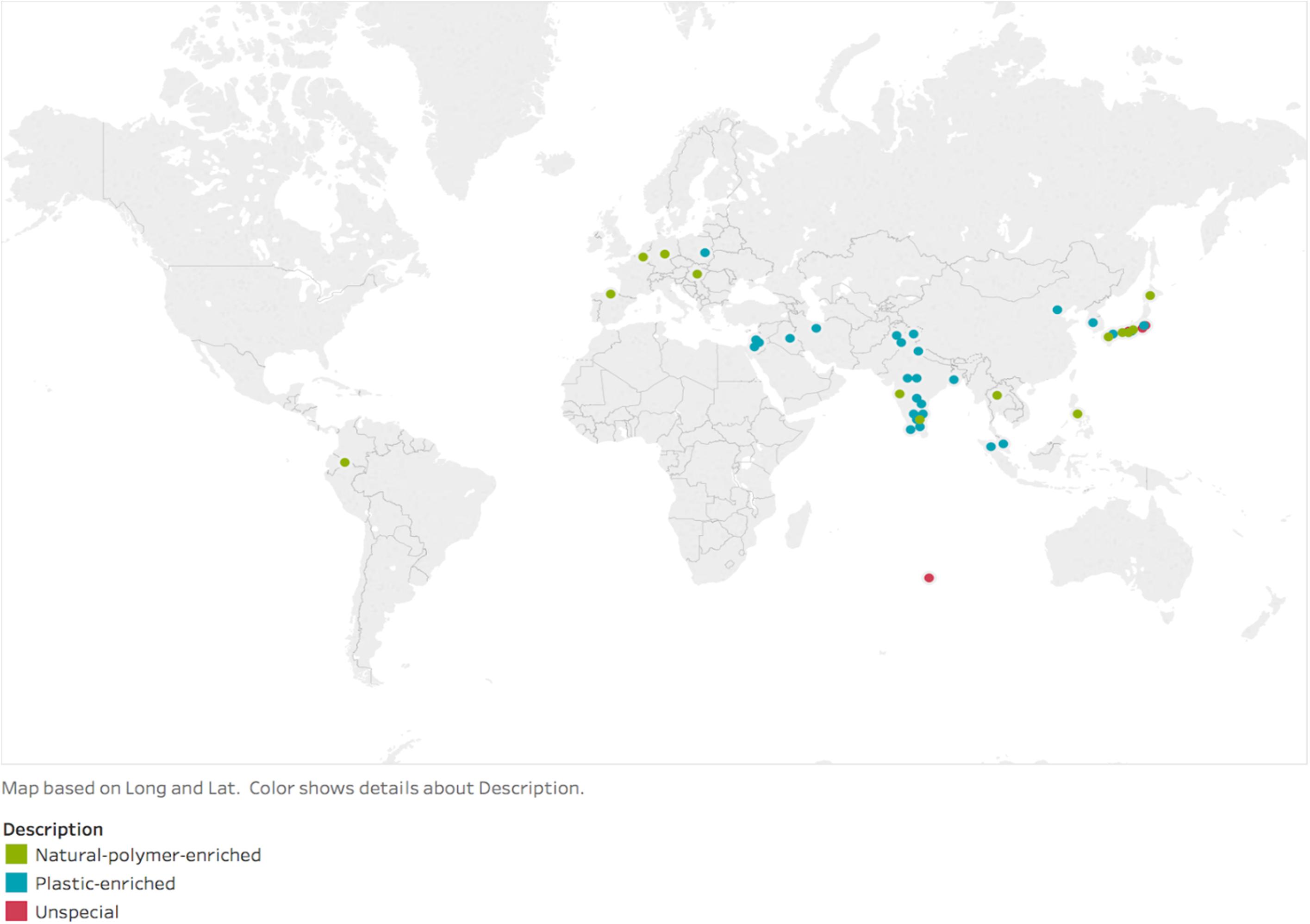
Figure 3. The exploration of plastic-biodegrading organisms is concentrated in Japan and India. Much of the world remains unexplored.
Naturally Occurring Activity From Enzymes That Degrade Natural Polymers
A growing body of literature supports the hypothesis that organisms evolved to metabolize naturally occurring polymers may also possess an ability to degrade plastic. The similarities in molecular structures between certain natural and synthetic polymers allow enzymes with broad substrate specificity to also degrade plastics. So far, similar degradation mechanisms appear to take place for three polymer pairs: (1) polyethylene terephthalate (PET) and cutin, a waxy biopolyester coating plant cuticles; (2) polyamide (nylon) and lignin, a woody complex polymer found in plant cell walls; and (3) polyethylene (PE) and beeswax.
First, research shows that PET degradation is a hydrolytic process catalyzed by cutinases, enzymes that typically hydrolyze ester bonds found in cutin (Müller et al., 2005; Ronkvist et al., 2009; Sulaiman et al., 2012; Kawai et al., 2014). Cutin is a waxy, water-repellent, fatty acid polymer that makes up the cuticle of plants (Fich et al., 2016). Several cutinases isolated from bacteria located around decomposing plant matter have also shown activity for degrading PET (Silva et al., 2011; Herrero Acero et al., 2013; Shirke et al., 2018). In one example, Wei et al. (2016) engineered a double mutation of Thermobifida fusca cutinase TfCut2 based on the leaf and branch compost cutinase (LCC), a PET-degrading cutinase isolated from leaf compost (Sulaiman et al., 2012). The TfCut2-LCC hybrid mutant expressed a 2.7-fold increase in degradation activity compared to the wild type enzyme, at lower temperatures than LCC requires: it caused over 42% weight loss of PET films after only 50 h of treatment (Wei et al., 2016). Cutinase-catalyzed PET-degradation has also been enhanced by targeting polymer-cutinase interactions through the addition of hydrophobins, small hydrophobic proteins produced by filamentous fungi (Espino-Rammer et al., 2013; Ribitsch et al., 2015), and surfactants (Eberl et al., 2009).
Second, both nylon and lignin are susceptible to degradation by white-rot fungi (Deguchi et al., 1997, 1998; Fujisawa et al., 2001; Klun et al., 2003; Friedrich et al., 2007). Lignin is a highly hydrophobic, aromatic compound found in the cell walls of plants. It is highly recalcitrant to degradation, just like many plastics. While natural mechanisms of lignin degradation are still being uncovered, white-rot fungi are among the few organisms known to break down lignin in natural environments (Leonowicz et al., 1999; Dashtban et al., 2010). Evidence suggests that lignin degradation by white-rot fungi takes place by the secretion of extracellular oxidative enzymes, such as laccase and manganese peroxidase (Deguchi et al., 1998; Klun et al., 2003; Friedrich et al., 2007). Furthermore, lignin biodegradation has been the subject of optimization efforts by the biofuel industry by adding a “mediator” reagent to enhance laccase activity (Christopher et al., 2014; Plácido and Capareda, 2015). By using the lignin degradation system adapted by white-rot fungi, Fujisawa et al. (2001) effectively applied a laccase-mediator system to polyethylene and nylon-6,6 (Fujisawa et al., 2001). With the added mediator, a laccase isolated from Trametes versicolor fungi could more easily oxidize the non-phenolic structures of nylon, reducing its average molecular weight by over five fold in 3 days. This result highlights the potential in further applying lignin-degrading systems to nylon biodegradation. The use of laccase-mediator systems has since not focused on plastic, but on bioremediation of other compounds such as perfluoroctanesulfonate, carbamazepine, and non-steroidal anti-inflammatory drugs (NSAIDs) (Luo et al., 2018; Naghdi et al., 2018; Apriceno et al., 2019).
Third, several recent studies highlight PE biodegradation potential in the ecological niche of wax-degrading organisms and their microbiota (Hadad et al., 2005; Yang et al., 2014; Bombelli et al., 2017). Yang et al. (2014) identified strains Bacillus sp. YP1 and Enterobacter asburiae YT1 as PE-degrading bacteria from Plodia interpunctella larvae, or Indian meal moths (Yang et al., 2014). These bacteria independently degraded six to 10% by weight of a low-density polyethylene (LDPE) film in 60 days. Bombelli et al. (2017) reported even more rapid biodegradation of PE by another wax moth larvae, Galleria mellonella, into ethylene glycol: larvae homogenate caused a 13% weight loss in PE film after just 14 h (Bombelli et al., 2017). The biodegradation mechanism of these wax moth larvae is hypothesized to originate in the insect’s natural adaptation to parasitize bee hives and use beeswax as a nutrient source (Kong et al., 2019), which has a similar saturated, aliphatic structure to polyethylene (Tulloch, 1971). The wax-degrading thermophilic bacterium Brevibacillus borstelensis has also been shown to have PE-degrading potential, reducing gravimetric weight by 11% in 30 days (Hadad et al., 2005).
Adaptation to Plastic as a Carbon Source Where Plastic Is Plentiful
In addition to harnessing the biodegradation activity from organisms that evolved to degrade naturally occurring polymers, another promising location to identify plastic-degrading organisms may be within plastic-enriched locations. Multiple studies have reported on the characterization of plastic-degrading organisms isolated from plastic processing facilities, recycling plants, plastic waste dump sites, and other hydrocarbon-contaminated soils (Satlewal et al., 2008; Ibrahim et al., 2009; Tachibana et al., 2010; Mukherjee et al., 2011; Gyung Yoon and Jeong Jeon, 2012; Esmaeili et al., 2013; Shah et al., 2013, 2016; Kowalczyk et al., 2016; Skariyachan et al., 2016, 2017; Yoshida et al., 2016; Ojha et al., 2017). High plastic concentrations in these locations may exert eco-toxilogical selective pressures different than natural selective pressures, leading to the evolution of biodegradation mechanisms as a means to utilize plastic as an additional carbon source. Notably, the bacterium Ideonella sakaiensis 201-F6, which was isolated from a PET debris–contaminated environmental sample, could completely degrade a PET film in six weeks (Yoshida et al., 2016). The microbe’s two-step mechanism of PET-degradation into ethylene glycol and terephthalic acid consists of two enzymes: PETase and MHETase (Yoshida et al., 2016). Interestingly, analysis of PETase reveals sequence homology and structural similarity to the PET-degrading cutinases discussed above. Both contain a α/β helicase fold with a Ser-Asp-His catalytic triad, a well-defined domain capable of performing nucleophilic attacks on ester bonds (Austin et al., 2018). PETase, however, has different substrate specificity than its cutinase-like homolog: PETase exhibits higher activity against PET (Han et al., 2017). Despite the increased activity of PETase for PET, extensive research is underway to optimize the enzyme. For example, converting through mutagenesis the wide active-site cleft of PETase into a narrower one that more closely resembles that of cutinases significantly increases PET degrading activity (Austin et al., 2018). Building on nature’s design in this way may allow PETase and other enzymes to be optimized for biodegradation activity (Austin et al., 2018; Joo et al., 2018).
Plastic-enriched sites yield promising prospects in the form of bacterial consortia. For example, a crafted consortium of the three most effectively degrading strains of bacteria isolated from a garbage processing facility caused over 81% weight loss of LDPE films after a 120-day incubation (Skariyachan et al., 2016). The same researchers produced similar results on both LDPE and HDPE films with another consortium of four thermophilic bacteria isolated from plastic-contaminated cow dung (Skariyachan et al., 2017). Several of the strains in the latter study could not independently degrade the films, but their effectiveness was significantly improved when combined with the others. Likewise, the bacterium Bacillus subtilis MZA-75, isolated from waste dumping sites in India, was initially shown to mineralize polyester polyurethane alone (Shah et al., 2013), but was later revealed to work more effectively in a naturally adapted consortium with Pseudomonas aeruginosa MZA-85 (Shah et al., 2016). These findings suggest indigenous consortia could maximize the potential for degradation as compared to single microbial species or strains.
Future Research Directions
As the plastic epidemic’s impacts on the environment intensify, there will be a growing need to identify and harness organisms and enzymes that can biodegrade plastics. Below, we highlight directions for future exploration and optimization of plastic biodegradation mechanisms.
Engineering and Optimizing Systems
The discovery of organisms with natural biodegradation activity allows researchers to apply established and emerging technologies in bioengineering for optimization and practical application. In addition to protein engineering of enzymes, such as PETase (Austin et al., 2018; Joo et al., 2018), other promising efforts aim to develop systems that enhance enzymatic activity. For example, in order to relieve product inhibition of enzymes degrading PET, researchers employed a dual enzyme system of a cutinase and carboxylesterase to remove this intermediate degradation product via an ultrafiltration system (Barth et al., 2015, 2016). By viewing naturally adapted organisms and enzymes as building blocks to optimize and combine into systems for specific uses, natural biodegradation activity can be channeled into actionable, effective bioremediation applications.
Locations to Explore
The presence of plastic-degrading organisms in locations enriched with natural polymers hints at unexplored potential in other richly biodiverse ecosystems, such as rainforests and the deep sea. First, rainforests are enriched with enormous varieties of natural polymers in the form of plant life (Dirzo and Raven, 2003) and microorganisms that live in close proximity to those polymers (Tripathi et al., 2016). One particularly promising subset of these microorganisms is endophytes, organisms that live inside of plants (Nair and Padmavathy, 2014). In fact, several organisms able to degrade polyurethane as a sole carbon source were identified from a small sample of endophytes from Ecuadorian rainforests (Russell et al., 2011), suggesting there is unexplored biodegradation potential in the dense biodiversity of rainforests and their microorganisms.
Similar to rainforests, the marine environment is an uncharted ecosystem enriched with microbial biodiversity (Sogin et al., 2006) and naturally occurring polymers like cross-linked proteins in mussels and sea cucumbers (Scott, 2015), in addition to anthropogenic hydrocarbons like plastic waste and petroleum (National Research Council, 2003; Chiba et al., 2018). While most biodegrading organisms have been isolated from terrestrial locations (Figure 3 and Supplementary Table S1), the marine ecosystem has been largely unexplored. In one study, however, a marine bacterial culture from the Indian Ocean demonstrated degradation of several nylon varieties (Sudhakar et al., 2007), highlighting the ocean’s potential for identifying new plastic-degrading species (Alava, 2019). Furthermore, marine bacteria that exclusively feed on hydrocarbons have been found to degrade oil from oil spills, suggesting that they could adapt to other man-made hydrocarbons like plastic as well (Brooijmans et al., 2009). Additionally, interactions between larger marine organisms and their microbiota may result in biodegradation analogous to the terrestrial case of wax moth larvae: a prime example is the polychaete Marphysa sanguinea, a type of marine worm that has recently been found to fragment polystyrene into microplastics (Jang et al., 2018). As mentioned above, laccase mediator systems have the capability to degrade nylon (Thompson et al., 2009). This creates opportunities for further expansion, perhaps with the marine fungal isolate Trematosphaeria mangrovei, which has high laccase activity (Tulloch, 1971). These initial observations in marine environments suggest the ocean environment could contain undiscovered potential for plastic biodegradation.
Finally, medical environments are target locations; selective pressures resulting from their sterile conditions result in microorganism adaptation. For example, two Enterococcus species–E. faecalis and E. faecium–have extended survival on fabrics and plastics; their survival has been attributed to an ability to degrade and metabolize the synthetic plastics they colonize (Neely and Maley, 2000). While undesirable in hospital settings, microorganisms able to survive in such environments have likely evolved mechanisms of biodegradation that hold great value for plastic biodegradation efforts.
Conclusion
As plastic waste accumulates, so does the critical need for efficient, sustainable remediation. Drawing inspiration from nature’s well-tuned resource cycling and constant evolutionary selection, biodegradation has serious potential as a novel and creative pathway to manage the plastic epidemic. To identify these new potential sources of plastic remediation, researchers should turn to two locations: environments enriched with naturally occurring polymers, and sites with high concentrations of plastic waste. Ultimately, these ongoing identification and optimization efforts may facilitate the integration of a “circular economy” of resources into the plastic industry by providing effective mechanisms for the breakdown of plastics and by mitigating the many hazards of plastic waste accumulation. The hunt for naturally occurring plastic-degrading organisms, while currently only scratching the surface of Earth’s biodiversity, provides hope for what may be awaiting discovery.
Author Contributions
MS, SK, ES, WE, and JS contributed to conception and design of the study. MS, SK, ES, and EM organized the tables and data. MS, SK, ES, and JS wrote the first draft of the manuscript. EM, SH, MD-D, AR, and TS wrote sections of the manuscript. All authors contributed to manuscript revision, read, and approved the submitted version.
Conflict of Interest
The authors declare that the research was conducted in the absence of any commercial or financial relationships that could be construed as a potential conflict of interest.
Funding
JS is supported by the Department of Defense (W81XWH-18-1-0189), the Seaworld & Busch Gardens Conservation Fund, Meg and Bill Lindenberger, the Paul and Shirley Friedland Fund, the Triangle Center for Evolutionary Medicine, and funds raised in memory of Muriel E. Rudershausen (riding4research.org).
Supplementary Material
The Supplementary Material for this article can be found online at: https://www.frontiersin.org/articles/10.3389/fmars.2019.00624/full#supplementary-material
TABLE S1 | Organisms that degrade plastic.
References
Alava, J. J. (2019). “Ocean pollution and warming oceans: towards ocean solutions and natural marine bioremediation,” in Predicting Future Oceans: Sustainability of Ocean and Human Systems amidst Global Environmental Change, eds W. Cheung, A. Cisneros-Montemayor, and Y. Ota, (Amsterdam: Elsevier).
Andrady, A. L., and Neal, M. A. (2009). Applications and societal benefits of plastics. Philos. Trans. R. Soc. Lond. B Biol. Sci. 364, 1977–1984. doi: 10.1098/rstb.2008.0304
Apriceno, A., Astolfi, M. L., Girelli, A. M., and Scuto, F. R. (2019). A new laccase-mediator system facing the biodegradation challenge: insight into the NSAIDs removal. Chemosphere 215, 535–542. doi: 10.1016/j.chemosphere.2018.10.086
Astrup, T., Moller, J., and Fruergaard, T. (2009). Incineration and co-combustion of waste: accounting of greenhouse gases and global warming contributions. Waste Manag. Res. 27, 789–799. doi: 10.1177/0734242X09343774
Austin, A. T., and Vivanco, L. (2006). Plant litter decomposition in a semi-arid ecosystem controlled by photodegradation. Nature 442, 555–558. doi: 10.1038/nature05038
Austin, H. P., Allen, M. D., Donohoe, B. S., Rorrer, N. A., Kearns, F. L., Silveira, R. L., et al. (2018). Characterization and engineering of a plastic-degrading aromatic polyesterase. Proc. Natl. Acad. Sci. U.S.A. 115, E4350–E4357. doi: 10.1073/pnas.1718804115
Barnes, D. K. A., Galgani, F., Thompson, R. C., and Barlaz, M. (2009). Accumulation and fragmentation of plastic debris in global environments. Philos. Trans. R. Soc. B Biol. Sci. 364, 1985–1998. doi: 10.1098/rstb.2008.0205
Barth, M., Honak, A., Oeser, T., Wei, R., Belisário-Ferrari, M. R., Then, J., et al. (2016). A dual enzyme system composed of a polyester hydrolase and a carboxylesterase enhances the biocatalytic degradation of polyethylene terephthalate films. Biotechnol. J. 11, 1082–1087. doi: 10.1002/biot.201600008
Barth, M., Oeser, T., Wei, R., Then, J., Chmidt, J., and Zimmermann, W. (2015). Effect of hydrolysis products on the enzymatic degradation of polyethylene terephthalate nanoparticles by a polyester hydrolase from Thermobifida fusca. Biochem. Eng. J. 93, 222–228. doi: 10.1016/j.bej.2014.10.012
Bombelli, P., Howe, C. J., and Bertocchini, F. (2017). Polyethylene bio-degradation by caterpillars of the wax moth Galleria mellonella. Curr. Biol. 27, R292–R293. doi: 10.1016/j.cub.2017.02.060
Bosetti, C., La Vecchia, C., Lipworth, L., and McLaughlin, J. K. (2003). Occupational exposure to vinyl chloride and cancer risk: a review of the epidemiologic literature. Eur. J. Cancer Prevent. 12, 427–430. doi: 10.1097/00008469-200310000-00012
Brandt-Rauf, P. W., Li, Y., Long, C., Monaco, R., Kovvali, G., and Marion, M. (2012). Plastics and carcinogenesis: the example of vinyl chloride. J. Carcinog. 11:5. doi: 10.4103/1477-3163.93700
Brooijmans, R. J., Pastink, M. I., and Siezen, R. J. (2009). Hydrocarbon-degrading bacteria: the oil-spill clean-up crew. Microb. Biotechnol. 2, 587–594. doi: 10.1111/j.1751-7915.2009.00151.x
Carlton, J. T., Chapman, J. W., Geller, J. B., Miller, J. A., Carlton, D. A., McCuller, M. I., et al. (2017). Tsunami-driven rafting: transoceanic species dispersal and implications for marine biogeography. Science 357, 1402–1406. doi: 10.1126/science.aao1498
Chiba, S., Saito, H., Fletcher, R., Yogi, T., Kayo, M., Miyagi, S., et al. (2018). Human footprint in the abyss: 30 year records of deep-sea plastic debris. Mar. Policy 96, 204–212. doi: 10.1016/j.marpol.2018.03.022
Christopher, L. P., Yao, B., and Ji, Y. (2014). Lignin biodegradation with laccase-mediator systems. Front. Energy Res. 2:12. doi: 10.3389/fenrg.2014.00012
Cox, K. D., Coverton, G. A., Davies, H. L., Dower, J. F., Juanes, F., and Dudas, S. E. (2019). Human consumption of microplastics. Environ. Sci. Technol. 53, 7068–7074. doi: 10.1021/acs.est.9b01517
Cózar, A., Marti, E., Duarte, C. M., García-de-Lomas, J., van Sebille, E., Ballatore, T. J., et al. (2017). The Arctic Ocean as a dead end for floating plastics in the North Atlantic branch of the thermohaline circulation. Sci. Adv. 3:e1600582. doi: 10.1126/sciadv.1600582
Dashtban, M., Schraft, H., Syed, T. A., and Qin, W. (2010). Fungal biodegradation and enzymatic modification of lignin. Int. J. Biochem. Mol. Biol. 1, 36–50.
Deguchi, T., Kakezawa, M., and Nishida, T. (1997). Nylon biodegradation by lignin-degrading fungi. Appl. Environ. Microbiol. 63, 329–331.
Deguchi, T., Kitaoka, Y., Kakezawa, M., and Nishida, T. (1998). Purification and characterization of a nylon-degrading enzyme. Appl. Environ. Microbiol. 64, 1366–1371.
Dirzo, R., and Raven, P. H. (2003). Global state of biodiversity and loss. Annu. Rev. Environ. Resour. 28, 137–167.
Eberl, A., Heumann, S., Brückner, T., Araujo, R., Cavaco-Paulo, A., Kaufmann, F., et al. (2009). Enzymatic surface hydrolysis of poly(ethylene terephthalate) and bis(benzoyloxyethyl) terephthalate by lipase and cutinase in the presence of surface active molecules. J. Biotechnol. 143, 207–212. doi: 10.1016/j.jbiotec.2009.07.008
Eriksen, M., Lebreton, L. C. M., Carson, H. S., Theil, M., Moore, C. J., Borerro, J. C., et al. (2014). Plastic pollution in the world’s oceans: more than 5 trillion plastic pieces weighing over 250,000 Tons Afloat at Sea. PLoS One 9:e111913. doi: 10.1371/journal.pone.0111913
Eriksson, C., and Burton, H. (2003). Origins and biological accumulation of small plastic particles in fur seals from Macquarie Island. AMBIO 32, 380–384. doi: 10.1579/0044-7447-32.6.380
Esmaeili, A., Pourbabaee, A. A., Alikhani, H. A., Shabani, F., and Esmaeili, E. (2013). Biodegradation of low-density polyethylene (LDPE) by mixed culture of Lysinibacillus xylanilyticus and Aspergillus niger in Soil. PLoS One 8:e71720. doi: 10.1371/journal.pone.0071720
Espino-Rammer, L., Ribitsch, D., Przylucka, A., Marold, A., Greimel, K. J., Herrero Acero, E., et al. (2013). Two novel class II hydrophobins from Trichoderma spp. stimulate enzymatic hydrolysis of poly(ethylene terephthalate) when expressed as fusion proteins. Appl. Environ. Microbiol. 79, 4230–4238. doi: 10.1128/AEM.01132-13
Fich, E. A., Segerson, N. A., and Rose, J. K. (2016). The plant polyester cutin: biosynthesis, structure, and biological roles. Annu. Rev. Plant Biol. 67, 207–233. doi: 10.1146/annurev-arplant-043015-111929
Friedrich, J., Zalar, P., Mohorčič, M., Klun, U., and Kržan, A. (2007). Ability of fungi to degrade synthetic polymer nylon-6. Chemosphere 67, 2089–2095. doi: 10.1016/j.chemosphere.2006.09.038
Fujisawa, M., Hirai, H., and Nishida, T. (2001). Degradation of polyethylene and nylon-66 by the laccase-mediator system. J. Poly. Environ. 9, 103–108.
Gewert, B., Plassmann, M. M., and MacLeod, M. (2015). Pathways for degradation of plastic polymers floating in the marine environment. Environ. Sci. Process. Impacts 17, 1513–1521. doi: 10.1039/c5em00207a
Geyer, R., Jambeck, J. R., and Law, K. L. (2017). Production, use, and fate of all plastics ever made. Sci. Adv. 3:e1700782. doi: 10.1126/sciadv.1700782
Gilpin, R. K., Wagel, D. J., and Solch, J. G. (2005). “Production, distribution, and fate of polychlorinated Dibenzo-p-Dioxins, Dibenzofurans, and related organohalogens in the environment,” in Dioxins and Health, eds A. Schecter and T. A. Gasiewicz, (Hoboken, NJ: Wiley).
Gómez, E., and Michel, F. (2013). Biodegradability of conventional and bio-based plastics and natural fiber composites during composting, anaerobic digestion and long-term soil incubation. Polym. Degrad. Stabil. 98, 2583–2591. doi: 10.1016/j.polymdegradstab.2013.09.018
Gregory, M. R. (2009). Environmental implications of plastic debris in marine settings–entanglement, ingestion, smothering, hangers-on, hitch-hiking and alien invasions. Philos. Trans. R. Soc. Lond. B Biol. Sci. 364, 2013–2025. doi: 10.1098/rstb.2008.0265
Gyung Yoon, M., and Jeong Jeon, H. (2012). Biodegradation of polyethylene by a soil bacterium and AlkB cloned recombinant cell. J. Bioremed. Biodegrad. 3:4.
Hadad, D., Geresh, S., and Sivan, A. (2005). Biodegradation of polyethylene by the thermophilic bacterium Brevibacillus borstelensis. J. Appl. Microbiol. 98, 1093–1100. doi: 10.1111/j.1365-2672.2005.02553.x
Han, X., Liu, W., Huang, J. W., Ma, J., Zhen, Y., Ko, T. P., et al. (2017). Structural insight into catalytic mechanism of PET hydrolase. Nat. Commun. 8:2106. doi: 10.1038/s41467-017-02255-z
Herrero Acero, E., Ribitsch, D., Dellacher, A., Zitzenbacher, S., Marold, A., Steinkellner, G., et al. (2013). Surface engineering of a cutinase from Thermobifida cellulosilytica for improved polyester hydrolysis. Biotechnol. Bioeng. 110, 2581–2590. doi: 10.1002/bit.24930
Hoornweg, D., and Perinaz, B. T. (2012). What a Waste: a Global Review of Solid Waste Management. Urban Development Series;Knowledge Papers no. 15, Vol. 15. Washington, DC: World Bank, 87–88.
Hopewell, J., Dvorak, R., and Kosior, E. (2009). Plastics recycling: challenges and opportunities. Philos. Trans. R. Soc. Lond. B Biol. Sci. 364, 2115–2126. doi: 10.1098/rstb.2008.0311
Horner-Devine, M. C., Carney, K. M., and Bohannan, B. J. M. (2004). An ecological perspective on bacterial biodiversity. Proc. R. Soc. Lond. Ser. B Biol. Sci. 271, 113–122.
Ibrahim, I. N., Maraqa, A., Hameed, K. M., Saadoun, I. M., Maswadeh, H. M., and Nakajima-Kambe, T. (2009). Polyester-polyurethane biodegradation by Alternaria solani, isolated from northern Jordan. Adv. Environ. Biol. 3, 162–170.
Infante, P. F., Wagoner, J. K., and Waxweiler, R. J. (1976). Carcinogenic, mutagenic and teratogenic risks associated with vinyl chloride. Mutat. Res. 41, 131–141. doi: 10.1016/0027-5107(76)90083-x
International Agencey for Research on Cancer (1977). Asbestos. IARC Monographs on the Evaluation of Carcinogenic Risks to Humans, Vol. 14. Lyon: International Agencey for Research on Cancer, 29–32.
International Agencey for Research on Cancer (1986). Some chemicals used in plastics and elastomers. IARC Monogr. Eval. Carcinog. Risks Hum. 39, 347–368.
International Agencey for Research on Cancer (1990). Some flame retardants and textile chemicals, and exposures in the textile manufacturing industry. IARC Monogr. Eval. Carcinog. Risks Hum. 48, 45–72.
International Agencey for Research on Cancer (1993). Beryllium, cadmium, mercury, and exposures in the glass manufacturing industry. IARC Monogr. Eval. Carcinog. Risks Hum. 58, 41–238.
International Agencey for Research on Cancer (1994). Some Industrial Chemicals. IARC Monographs on the Evaluation of Carcinogenic Risks to Humans. 60. Lyon: International Agencey for Research on Cancer, 215–496.
International Agencey for Research on Cancer (1995). Dry cleaning, some chlorinated solvents and other industrial chemicals. IARC monographs on the evaluation of carcinogenic risks to humans, 63. Cancer Caus. Control 7, 289–291. doi: 10.1007/bf00051307
International Agencey for Research on Cancer (1999a). Re-evaluation of Some Organic Chemicals, Hyddrazine and Hydrogen Peroxide. IARC Monographs on the Evaluation of Carcinogenic Risks to Humans. Lyon: International Agencey for Research on Cancer.
International Agencey for Research on Cancer (1999b). Some Chemicals that Cause Tumours of the Kidney or Urinary Bladder in Rodents and Some Other Substances. IARC Monographs on the Evaluation of Carcinogenic Risks to Humans. Lyon: International Agencey for Research on Cancer, 131–338.
International Agencey for Research on Cancer (2000). Some Industrial Chemicals. IARC Monographs on the Evaluation of Carcinogenic Risks to Humans, 77. Lyon: International Agencey for Research on Cancer, 41–266.
International Agencey for Research on Cancer (2002). Some traditional herbal medicines, some mycotoxins, napthalene and styrene. IARC Monogr. Eval. Carcinog. Risks Hum. 82, 437–550.
International Agencey for Research on Cancer (2006). Formaldehyde, 2-Butoxyethanol and 1-tert-Butoxypropan-2-ol. IARC Monogr. Eval. Carcinog. Risks Hum. 88, 37–326.
International Agencey for Research on Cancer (2008). 1,3-Butadiene, Ethylene Oxide and Vinyl Halides (Vinyl Fluoride, Vinyl Chloride and Vinyl Bromide). IARC Monogr. Eval. Carcinog. Risks Hum. 97, 311–458.
International Agencey for Research on Cancer (2010). Some Aromatic Amines, Organic Dyes, and Related Exposures. IARC Monographs on the Evaluation of Carcinogenic Risks to Humans. 99. Lyon: International Agencey for Research on Cancer, 325–368.
International Agencey for Research on Cancer (2012a). Arsenic, Metals, Fibres and Dusts. IARC Monographs on the Evaluation of Carcinogenic Risks to Humans 100C. Lyon: International Agencey for Research on Cancer, 219–310.
International Agencey for Research on Cancer (2012b). Chemical Agents and Related Occupations. IARC Monographs on the Evaluation of Carcinogenic Risks to Humans 100F. Lyon: International Agencey for Research on Cancer, 309–478.
International Agencey for Research on Cancer (2013). Some Chemicals Present in Industrial and Consumer Products, Food and Drinking-water. IARC Monographs on the Evaluation of Carcinogenic Risks to Humans 101. Lyon: International Agencey for Research on Cancer, 141–148.
International Agencey for Research on Cancer (2014). Trichloroethylene, Tetrachloroethylene, and Some Other Chlorinated Agents. IARC Monographs on the Evaluation of Carcinogenic Risks to Humans, 106. Lyon: International Agencey for Research on Cancer, 35–352.
International Agencey for Research on Cancer (2017). Some Chemicals Used as Solvents and in Polymer Manufacture. IARC Monographs on the Evaluation of Carcinogenic Risks to Humans, Vol. 110. Geneva: World Health Organization, 111–273.
International Agencey for Research on Cancer (2018). Some Industrial Chemicals. IARC Monographs on the Evaluation of Carcinogenic Risks to Humans. Lyon: International Agencey for Research on Cancer, 191–246.
Jambeck, J. R., Geyer, R., Wilcox, C., Siegler, T. R., Perryman, M., Andrady, A., et al. (2015). Marine pollution. Plastic waste inputs from land into the ocean. Science 347, 768–771. doi: 10.1126/science.1260352
Jang, M., Shim, W. J., Han, G. M., Song, Y. K., and Hong, S. H. (2018). Formation of microplastics by polychaetes (Marphysa sanguinea) inhabiting expanded polystyrene marine debris. Mar. Pollut. Bull. 131, 365–369. doi: 10.1016/j.marpolbul.2018.04.017
Joo, S., Cho, I. J., Seo, H., Son, H. F., Sagong, H. Y., Shin, T. J., et al. (2018). Structural insight into molecular mechanism of poly(ethylene terephthalate) degradation. Nat Commun. 9:382. doi: 10.1038/s41467-018-02881-1
Kawai, F., Oda, M., Tamashiro, T., Waku, T., Tanaka, N., Yamamoto, M., et al. (2014). A novel Ca(2)(+)-activated, thermostabilized polyesterase capable of hydrolyzing polyethylene terephthalate from Saccharomonospora viridis AHK190. Appl. Microbiol. Biotechnol. 98, 10053–10064. doi: 10.1007/s00253-014-5860-y
Klun, U., Friedrich, J., and Kržan, A. (2003). Polyamide-6 fibre degradation by a lignolytic fungus. Polym. Degrad. Stab. 79, 99–104. doi: 10.1016/s0141-3910(02)00260-4
Kong, H. G., Kim, H. H., Chung, J.-H., Jun, J., Lee, S., Kim, H.-M., et al. (2019). The Galleria mellonella hologenome supports microbiota-independent metabolism of long-chain hydrocarbon beeswax. Cell Rep. 26, 2451–2464. doi: 10.1016/j.celrep.2019.02.018
Koutny, M., Amato, P., Muchova, M., Ruzicka, J., and Delort, A. (2009). Soil bacterial strains able to grow on the surface of oxidized polyethylene film containing prooxidant additives. Int. Biodeterior. Biodegradation 63, 354–357. doi: 10.1016/j.ibiod.2008.11.003
Kowalczyk, A., Chyc, M., Ryszka, P., and Latowski, D. (2016). Achromobacter xylosoxidans as a new microorganism strain colonizing high-density polyethylene as a key step to its biodegradation. Environ. Sci. Pollut. Res. Int. 23, 11349–11356. doi: 10.1007/s11356-016-6563-y
Kumar, A. K., Balachandar, V., Arun, M., Ahamed, S. A., Kumar, S. S., Balamuralikrishnan, B., et al. (2013). A comprehensive analysis of plausible genotoxic covariates among workers of a polyvinyl chloride plant exposed to vinyl chloride monomer. Arch. Environ. Contam. Toxicol. 64, 652–658. doi: 10.1007/s00244-012-9857-1
Leonowicz, A., Matuszewska, A., Luterek, J., Ziegenhagen, D., Wojtaś-Wasilewska, M., Cho, N. S., et al. (1999). Biodegradation of lignin by white rot fungi. Fungal Genet. Biol. 27, 175–185.
Lopez, V., Chamoux, A., Tempier, M., Thiel, H., Ughetto, S., Trousselard, M., et al. (2003). The long-term effects of occupational exposure to vinyl chloride monomer on microcirculation: a cross-sectional study 15 years after retirement. BMJ Open 3:e002785. doi: 10.1136/bmjopen-2013-002785
Lucas, N., Bienaime, C., Belloy, C., Queneudec, M., Silvestre, F., and Nava-Saucedo, J. E. (2008). Polymer biodegradation: mechanisms and estimation techniques. Chemosphere. 73, 429–442. doi: 10.1016/j.chemosphere.2008.06.064
Luo, Q., Yan, X., Lu, J., and Huang, Q. (2018). Perfluorooctanesulfonate degrades in a laccase-mediator system. Environ. Sci. Technol. 52, 10617–10626. doi: 10.1021/acs.est.8b00839
McDonough, W. (2002). ). Cradle to Cradle : Remaking the Way We Make Things, 1st Edn. New York, NY: North Point Press, 2002.
Mukherjee, K., Tribedi, P., Chowdhury, A., Ray, T., Joardar, A., Giri, S., et al. (2011). Isolation of a Pseudomonas aeruginosa strain from soil that can degrade polyurethane diol. Biodegradation. 22, 377–388. doi: 10.1007/s10532-010-9409-1
Müller, R.-J., Schrader, H., Profe, J., Dresler, K., and Deckwer, W. D. (2005). Enzymatic degradation of poly(ethylene terephthalate): rapid hydrolyse using a hydrolase from T. fusca. Macromol. Rapid Commun. 26, 1400–1405. doi: 10.1002/marc.200500410
Naghdi, M., Taheran, M., Brar, S. K., Kermanshahi-pour, A., Verma, M., and Surampalli, R. Y. (2018). Biotransformation of carbamazepine by laccase-mediator system: kinetics, by-products and toxicity assessment. Process Biochem. 67, 147–154. doi: 10.1016/j.procbio.2018.02.009
Nair, D. N., and Padmavathy, S. (2014). Impact of endophytic microorganisms on plants, environment and humans. Sci. World J. 2014: 250693.
National Research Council (2003). Oil in the Sea III: Inputs, Fates, and Effects. Washington, DC: National Academies Press.
Neely, A. N., and Maley, M. P. (2000). Survival of enterococci and staphylococci on hospital fabrics and plastic. J. Clin. Microbiol. 38, 724–726.
Neufeld, F. S., Sheppard, R., and Gilman, T. (2016). The New Plastics Economy: Rethinking the Future of Plastics. 10/20 2017]. Available at: http://www3.weforum.org/docs/WEF_The_New_Plastics_Economy.pdf (accessed July 1, 2018).
Ojha, N., Pradhan, N., Singh, S., Barla, A., Shrivastava, A., Khatua, P., et al. (2017). Evaluation of HDPE and LDPE degradation by fungus, implemented by statistical optimization. Sci. Rep. 7:39515. doi: 10.1038/srep39515
Orr, I. G., Hadar, Y., and Sivan, A. (2004). Colonization, biofilm formation and biodegradation of polyethylene by a strain of Rhodococcus ruber. Appl. Microbiol. Biotechnol. 65, 97–104.
Plácido, J., and Capareda, S. (2015). Ligninolytic enzymes: a biotechnological alternative for bioethanol production. Bioresour. Bioprocess. 20, 1–12.
Prosser, J. I., Bohannan, B. J., Curtis, T. P., Ellis, R. J., Firestone, M. K., Freckleton, R. P., et al. (2007). The role of ecological theory in microbial ecology. Nat. Rev. Microbiol. 5, 384–392.
Ribitsch, D., Herrero Acero, E., Przylucka, A., Zitzenbacher, S., Marold, A., Gamerith, C., et al. (2015). Enhanced cutinase-catalyzed hydrolysis of polyethylene terephthalate by covalent fusion to hydrophobins. Appl. Environ. Microbiol. 81, 3586–3592. doi: 10.1128/AEM.04111-14
Rochman, C. M., Browne, M. A., Underwood, A. J., van Franeker, J. A., Thompson, R. C., and Amaral-Zettler, L. A. (2016). The ecological impacts of marine debris: unraveling the demonstrated evidence from what is perceived. Ecology 97, 302–312. doi: 10.1890/14-2070.1
Rochman, C. M., Hoh, E., Kurobe, T., and Teh, S. J. (2013). Ingested plastic transfers hazardous chemicals to fish and induces hepatic stress. Sci. Rep. 3:3263. doi: 10.1038/srep03263
Ronkvist, A., Xie, W., Lu, W., and Gross, R. A. (2009). Cutinase-catalyzed hydrolysis of poly(ethylene terephthalate). Macromolecules 42, 5128–5138. doi: 10.1021/ma9005318
Royer, S. J., Ferrón, S., Wilson, W. T., and Karl, D. M. (2018). Production of methane and ethylene from plastic in the environment. PLoS One 13:e0200574. doi: 10.1371/journal.pone.0200574
Rubin, B. S. (2011). Bisphenol A: an endocrine disruptor with widespread exposure and multiple effects. J. Steroid Biochem. Mol. Biol. 127, 27–34. doi: 10.1016/j.jsbmb.2011.05.002
Russell, J. R., Huang, J., Anand, P., Kucera, K., Sandoval, A. G., Dantzler, K. W., et al. (2011). Biodegradation of polyester polyurethane by endophytic fungi. Appl. Environ. Microbiol. 77, 6076–6084. doi: 10.1128/AEM.00521-11
Satlewal, A., Soni, R., Zaidi, M., Shouche, Y., and Goel, R. (2008). Comparative biodegradation of HDPE and LDPE using an indigenously developed microbial consortium. J. Microbiol. Biotechnol. 18, 477–482.
Setälä, O., Fleming-Lehtinen, V., and Lehtiniemi, M. (2014). Ingestion and transfer of microplastics in the planktonic food web. Environ. Pollut. 185, 77–83. doi: 10.1016/j.envpol.2013.10.013
Shah, Z., Gulzar, M., Hasan, F., and Shah, A. A. (2016). Degradation of polyester polyurethane by an indigenously developed consortium of Pseudomonas and Bacillus species isolated from soil. Poly. Degrad. Stab. 134, 349–356. doi: 10.1016/j.polymdegradstab.2016.11.003
Shah, Z., Hasan, F., Kruhmholz, L., Aktas, D. F., and Shah, A. A. (2013). Degradation of polyester polyurethane by a newly isolated soil bacterium, Bacillus subtilis strain MZA-75. Biodegradation 24, 865–877. doi: 10.1007/s10532-013-9634-5
Shirke, A. N., White, C., Englaender, J. A., Zwarycz, A., Butterfoss, G. L., Linhardt, R. J., et al. (2018). Stabilizing leaf and branch compost cutinase (LCC) with glycosylation: mechanism and effect on PET hydrolysis. Biochemistry 57, 1190–1200. doi: 10.1021/acs.biochem.7b01189
Silva, C., Da, S., Silva, N., Matamá, T., Araújo, R., Martins, M., et al. (2011). Engineered Thermobifida fusca cutinase with increased activity on polyester substrates. Biotechnol. J. 6, 1230–1239. doi: 10.1002/biot.201000391
Skariyachan, S., Manjunatha, V., Sultana, S., Jois, C., Bai, V., and Vasist, K. S. (2016). Novel bacterial consortia isolated from plastic garbage processing areas demonstrated enhanced degradation for low density polyethylene. Environ. Sci. Pollut. Res. Int. 23, 18307–18319. doi: 10.1007/s11356-016-7000-y
Skariyachan, S., Setlur, A. S., Naik, S. Y., Naik, A. A., Usharani, M. E., and Vasist, K. S. (2017). Enhanced biodegradation of low and high-density polyethylene by novel bacterial consortia formulated from plastic-contaminated cow dung under thermophilic conditions. Environ. Sci. Pollut. Res. Int. 24, 8443–8457. doi: 10.1007/s11356-017-8537-0
Sogin, M. L., Morrison, H. G., Huber, J. A., Welch, D. M., Huse, S. M., Neal, P. R., et al. (2006). Microbial diversity in the deep sea and the underexplored “rare biosphere”. Proc. Natl. Acad. Sci. U.S.A. 103:12115. doi: 10.1073/pnas.0605127103
Song, J. H., Murphy, R. J., Narayan, R., and Davies, G. B. (2009). Biodegradable and compostable alternatives to conventional plastics. Philos. Trans. R. Soc. B Biol. Sci. 364:2127. doi: 10.1098/rstb.2008.0289
Sudhakar, M., Priyadarshini, C., Doble, M., Murthy, P. S., and Venkatesan, R. (2007). Marine bacteria mediated degradation of nylon 66 and 6. Int. Biodeterior. Biodegrad. 60, 144–151. doi: 10.1016/j.ibiod.2007.02.002
Sulaiman, S., Yamato, S., Kanaya, E., Kim, J. J., Koga, Y., Takano, K., et al. (2012). Isolation of a novel cutinase homolog with polyethylene terephthalate-degrading activity from leaf-branch compost by using a metagenomic approach. Appl. Environ. Microbiol. 78, 1556–1562. doi: 10.1128/AEM.06725-11
Tachibana, K., Hashimoto, K., Yoshikawa, M., and Okawa, H. (2010). Isolation and characterization of microorganisms degrading nylon 4 in the composted soil. Polymer Degradation and Stability, 95, 912–917. doi: 10.1016/j.polymdegradstab.2010.03.031
Teuten, E. L., Saquing, J. M., Knappe, D. R. U., Barlaz, M. A., Johnsson, S., Björn, A., et al. (2009). Transport and release of chemicals from plastics to the environment and to wildlife. Philos. Trans. R. Soc. Lond. B Biol. Sci. 364, 2027–2045. doi: 10.1098/rstb.2008.0284
Thompson, R. C., Moore, C. J., vom Saal, F. S., and Swan, S. H. (2009). Plastics, the environment and human health: current consensus and future trends. Philos. Trans. R. Soc. B Biol. Sci. 364, 2153–2166. doi: 10.1098/rstb.2009.0053
Tribedi, P., Gupta, A. D., and Sil, A. K. (2015). Adaptation of Pseudomonas sp. AKS2 in biofilm on low-density polyethylene surface: an effective strategy for efficient survival and polymer degradation. Bioresour. Bioprocess. 2:14.
Tripathi, B. M., Song, W., Slik, J. W. F., Sukri, R. S., Jaafar, S., Dong, K., et al. (2016). Distinctive tropical forest variants have unique soil microbial communities, but not always low microbial diversity. Front. Microbiol. 7:376. doi: 10.3389/fmicb.2016.00376
Tulloch, A. P. (1971). Beeswax: structure of the esters and their component hydroxy acids and diols. Chem. Phys. Lipids 6, 235–265. doi: 10.1016/0009-3084(71)90063-6
Wang, Z., Liu, H., and Liu, S. (2017). Low-dose bisphenol a exposure: a seemingly instigating carcinogenic effect on breast cancer. Adv. Sci. 4:1600248. doi: 10.1002/advs.201600248
Wei, R., Oeser, T., Schmidt, J., Meier, R., Barth, M., Then, J., et al. (2016). Engineered bacterial polyester hydrolases efficiently degrade polyethylene terephthalate due to relieved product inhibition. Biotechnol. Bioeng. 113, 1658–1665. doi: 10.1002/bit.25941
Yang, J., Yang, Y., Wu, W. M., Zhao, J., and Jiang, L. (2014). Evidence of polyethylene biodegradation by bacterial strains from the guts of plastic-eating waxworms. Environ. Sci. Technol. 48, 13776–13784. doi: 10.1021/es504038a
Keywords: bioremediation, PETase, polymer, degradation, pollution
Citation: Sheth MU, Kwartler SK, Schmaltz ER, Hoskinson SM, Martz EJ, Dunphy-Daly MM, Schultz TF, Read AJ, Eward WC and Somarelli JA (2019) Bioengineering a Future Free of Marine Plastic Waste. Front. Mar. Sci. 6:624. doi: 10.3389/fmars.2019.00624
Received: 07 January 2019; Accepted: 23 September 2019;
Published: 11 October 2019.
Edited by:
James Scott Maki, Marquette University, United StatesReviewed by:
Zongze Shao, Third Institute of Oceanography, ChinaJuan Jose Alava, The University of British Columbia, Canada
Copyright © 2019 Sheth, Kwartler, Schmaltz, Hoskinson, Martz, Dunphy-Daly, Schultz, Read, Eward and Somarelli. This is an open-access article distributed under the terms of the Creative Commons Attribution License (CC BY). The use, distribution or reproduction in other forums is permitted, provided the original author(s) and the copyright owner(s) are credited and that the original publication in this journal is cited, in accordance with accepted academic practice. No use, distribution or reproduction is permitted which does not comply with these terms.
*Correspondence: Jason A. Somarelli, amFzb24uc29tYXJlbGxpQGR1a2UuZWR1
†These authors have contributed equally to this work