- 1Laboratory of Marine Biogeochemistry, Department of Biological Oceanography, Oceanographic Institute, University of São Paulo, São Paulo, Brazil
- 2Laboratory of Paleomagnetism, Oceanographic Institute, University of São Paulo, São Paulo, Brazil
The autotrophic plankton assemblage along the subtropical coastal embayments of the South Brazil Bight is dominated by the cyanobacterium Synechococcus. This investigation aims to assess its contribution to the total carbon metabolism within the planktonic system of Mamanguá Ría, a sub-system of the Bay of Ilha Grande, southeast Brazilian coast. We hypothesized that photosynthetic carbon fixation by cyanobacteria does not support the plankton metabolism inside the Ría. Net community production (NCP) was calculated from differences between gross community production (GCP) and dark community respiration rates measured by in situ incubations using the oxygen light-and-dark technique. Our results reveal the carbon budget inside the Ría is not balanced by autotrophic production. The deficit of net ecosystem production throughout the year ranged from 0.5 to 1.5 mg m–2 d–1 below what is necessary to sustain local plankton metabolism. We argue that the offset between daily GCP and total community respiration may be balanced by grazing of nano-heterotrophs on heterotrophic bacteria. Our conclusions apply to the majority of the meso-oligotrophic Brazilian inner shelf waters away from estuarine plumes and upwelling systems.
Introduction
Coastal waters constitute less than 10% of the total oceanic area, yet account for ca 25% of the global oceanic primary production (Berger et al., 1989). Part of this primary production is transferred to the nearshore sediment carbon pool, which may increase in volume in the next few decades due to coastal eutrophication worldwide (Selman and Greenhalgh, 2009; Selman et al., 2009). Better assessment of the balance between production and loss of organic carbon from coastal ecosystems would provide a more complete picture of their roles in the global carbon cycle. The main processes behind gains and losses in the water column carbon budget are, respectively, phytoplankton photosynthesis and respiration by heterotrophic bacteria (Williams, 1981), using dissolved organic matter of low molecular weight excreted throughout the planktonic foodweb system (Fuhrman, 1987; Lignell, 1990; Jahnke and Craven, 1995). The carbon biomass stocked in heterotrophic bacteria may return to the classic food chain through bacterivory by microheterotrophs (e.g., flagellates and ciliates) which in turn may be predated by larger zooplankton. This alternate trophic pathway, a microbial loop (sensu Azam et al., 1983), drives back the excreted dissolved organic matter toward the classic food chain. Depending on local hydrodynamics, detritus sedimentation, and/or advection are additional losses of organic carbon that are more difficult to assess quantitatively.
The oligotrophic southwestern Atlantic boundary current limits phytoplankton production, lower than in eastern upwelling systems by at least one order of magnitude (Longhurst, 2006). The overall shortage of nitrate, the main driver of new production (sensu Dugdale and Goering, 1967), along ca 8,500 km of the Brazilian continental margin (4°N – 28.5°S) limits primary production to rates between 0.02 and 4 gC m–2 day–1 and mean chlorophyll concentrations to between 0.2 and 0.4 mg m–3, usually less than 0.05 gC m–2 day–1 (Brandini, 1990; Teixeira and Gaeta, 1991; Smith and DeMaster, 1996; Gaeta et al., 1999; Medeiros et al., 1999; Castro et al., 2006). New nutrients are scarce throughout this oligotrophic coastal zone, which is dominated by the adjacent nutrient-poor open shelf waters. Here the net ecosystem production is negative because heterotrophic carbon consumption is substantially higher than autotrophic production in the classical food chain. Except near estuarine and mouths local upwelling systems (Valentin et al., 1987; Gonzalez-Rodriguez et al., 1992; Odebrecht and Djurfeldt, 1996; Brandini, 2018) where a diatom-based autotrophic ecosystem dominates, point sources of nutrients tend to be washed out by tidal currents and diluted alongshore by barotropic circulation; coastal waters in the South Brazil Bight (hereafter SBB) typically have chlorophyll concentrations of <1 μg L–1 (Castro et al., 2006).
The autotrophic plankton assemblage is dominated mostly by picoplankton cells, represented mostly by the cyanobacterium Synechococcus (prokaryote) and, to a lesser extent, by pico-eukaryotes (Gérikas Ribeiro et al., 2016; Moser et al., 2016; Bergo et al., 2017). This study assessed planktonic carbon metabolism in the Saco do Mamanguá Ría (Figure 1A), an inlet subsystem of the Bay of Ilha Grande (hereafter BIG, Lat 23 – 23.5°S; Long 43.5 – 44.5°W) that is representative of most of the alongshore Brazilian cyanobacteria-dominated oligotrophic coastal ecosystem. The BIG is a conspicuous feature of the SBB coastline (Figure 1C) therefore subjected to oligotrophic conditions as described above. The Ría is ∼11 km long and ∼2 km wide inlet running northeast-southwest and connected to the ocean only on the northeast side (Figure 1A). It is characterized by shallow waters with mean water depth about 5 m, reaching a maximum of 20 m at the outer área, and steep coastlines made of old crystalline rocks (Rodelli et al., 2019).
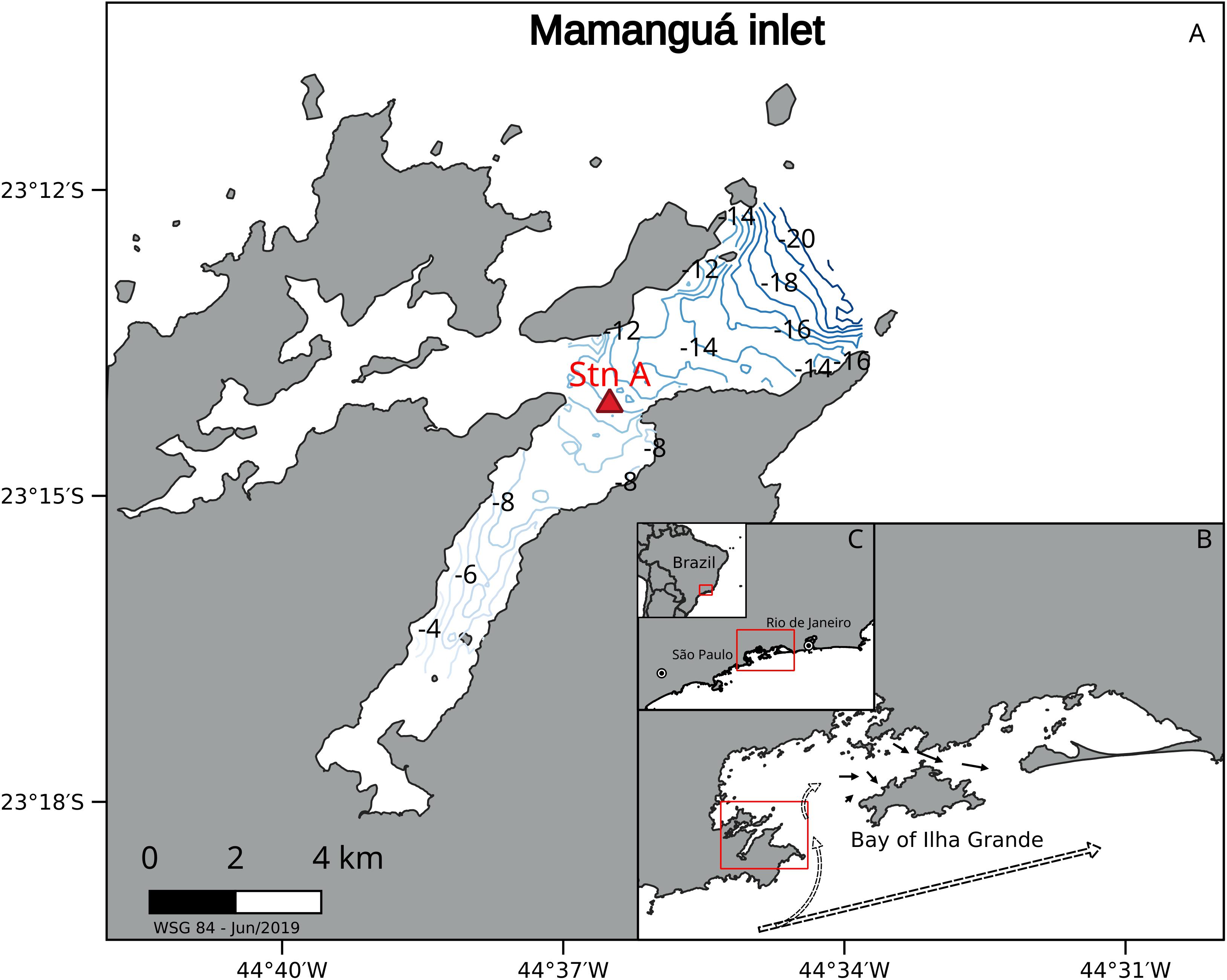
Figure 1. Sampling station (#A) position in Mamanguá Ría (A), Bay of Ilha Grande (B), and southern Brazil (C). Contour lines show the bathymetry inside the Ría. Main circulation is clockwise as shown by small arrows (apud Signorini, 1980). Dashed arrows represents wind-driven barotropic coastal currents.
Here we hypothesized that net community production (NCP), the difference between gross community production (GCP), and dark community respiration (DCR), is not sufficient to sustain the carbon flow metabolism within the planktonic system of the Ría and ultimately along most subtropical Brazilian coastal environments that are far from estuarine plumes and upwelling systems.
Materials and Methods
Study Area
The Ría is sheltered against strong SW and SE winds that are common in winter seasons (June-August), resulting in weak currents inside the western portion of the BIG (∼10 cm s–1) that circulate clockwise due to a combination of tidal-, wind- and density-driven forces (Ikeda and Stevenson, 1980; Signorini, 1980). Coastal waters along northern São Paulo typically move northeastward by wind-driven barotropic currents, enter the western mouth of the BIG, flow clockwise with a mean 10 cm s–1 around the western side of the bay, and out to the ocean through the eastern opening (Figure 1B). Hence, physical-chemical features tend to be similar to those of the northern coast of São Paulo (e.g., Ubatuba, São Sebastião), with minor differences that are attributed to local geomorphology and continental drainage. Air temperature ranges from 14.4 to 38.8°C with an annual mean of 24.9°C, and predominant winds are from the northeast. Mean monthly precipitation over a 20-year period (1979–1999) varied from 64.1 to 170.5 mm as reported by Brazil’s National Hydrometeorological Network operated by the Geological Survey of Brazil1. The innermost and shallower inlets of the Bay (e.g., Saco do Mamanguá Ría; Benites et al., 2015) are rapidly affected by the local wind field and by continental freshwater drainage from surrounding coastal-plain watersheds, particularly the Paraty Mirim river. Drainage is usually low, however, even during rainy summer seasons, so the year-round salinity fluctuations are slight, varying from 34 to 35 (Signorini, 1980).
Meteorological Data
Meteorological data were measured with the automatic meteorological station of Ubatuba coastal research laboratory located near the experiment site over the whole study period. Wind field, daily and monthly average of air temperature, solar radiation, and daily and monthly precipitation were then obtained from the LabDados database at http://labdados.io.usp.br/. Due to technical problems precipitation was not measured between September 2014 and January 2015. To complete the seasonal trend of precipitation during these periods, we used data from the CIIAGRO online database2. The Supplementary Figure S1 reveals a good agreement (r2 = 0.765) between both meteorological stations. Daily solar radiation data during the incubation experiments was obtained every 15 min from CS300 Cambbell Scientific pyranometer installed in the coastal research laboratory of the Oceanographic Institute in Ubatuba, approximately 50 km southward of the field site. Data was made available by Oceanographic Data Laboratory (LabDados3).
Sampling Procedure and Water Column Properties
Inside Saco do Mamanguá, Bay of Ilha Grande, in situ primary production incubations of light-and-dark bottles were performed on 8 occasions at irregular intervals between January 2014 and July 2016 at station A, which had a 14-m bottom depth at high tide (Figure 1). Despite the irregularity of field experiments, we were able to cover sufficiently the seasonal scale in which main differences may be accounted by the extremes in hydrographic conditions of summer (December to February) and winter (June to August) periods.
Gross community production and dark community respiration were measured by in situ incubations of a modified light and dark bottle oxygen method (Gaarder and Gran, 1927; Strickland and Parsons, 1972). We collected a vertical profile of water samples the night preceding an incubation with a Niskin bottle: at the surface, 2, 4, 5, 6, 7, and 8 meters irrespective of tidal state. Prior to incubation, 5 L of water from each sampling depth was filtered through a 200-μm nylon mesh to remove the larger macro-zooplankton and kept in coolers for at least 8 h to allow community respiration, reducing the concentration of dissolved oxygen to levels below saturation. This procedure was necessary to avoid loss of oxygen via bubbles when bottles were open for oxygen flux determinations. Before sunrise, water from each depth was poured into paired 300 mL light and dark Borosilicate glass bottles. These bottles were re-suspended at their original depths until midday, an incubation period of approximately 6 h.
An optical Pro-ODO oxygen probe (YSI Inc.) determined initial and final oxygen concentrations in the incubation bottles with an accuracy of 0.001 mg O2 L–1. The probe was pre-calibrated prior to each experiment at the nearby shore laboratory of the Oceanographic Institute’s research station using 100% water-saturated air under the stable ambient temperature of approximately 23°C provided by air-conditioning.
Daily GCP was determined from the difference between final oxygen concentrations in light and dark bottles and multiplied by the ratio of total integrated daily solar radiation to the integrated solar radiation during the incubating period. Daily DCR was calculated multiplying the DCR during the incubation by 24 h over the incubating period. NCP was then calculated as the difference between GCP and DCR. Mass oxygen fluxes were then converted into mass carbon fluxes using a CO2/O2 molar ratio of 0.375 (=12 mg C/32 mg O2) assuming a photosynthetic quotient (PQ) of 1.28 irrespective of nitrogen sources or phytoplankton composition as reported by Wielgat-Rychert et al. (2017).
Aliquots of the same samples used for incubation were poured into Falcon tubes and kept frozen at −20°C until lab analyses of Phosphate-P, Nitrate (+Nitrite)-N, and Ammonium-N using spectrophotometry according to Grashoff et al. (1999). Water column distributions of temperature, salinity, dissolved oxygen, turbidity, chlorophyll, and phycoerythrin fluorescence were obtained with an EXO2 multi-parametric probe (YSI Inc., United States). Sensors were 2-point calibrated (except dissolved oxygen) with deionized water and the following environment-range concentrated solutions: 1000 μS cm–1 standard solution (salinity), 124 NTU standard solution (turbidity), and 625 and 25 μg L–1 Rhodamine WT solutions for chlorophyll-a and phycoerythrin, respectively. The oxygen probe was calibrated with water saturated air. The light regime at each incubating depth was estimated with the light extinction coefficient k as determined from Secchi disk readings according to Poole and Atkins (1929).
Analyses of the Plankton Community
The water column microbial community was simultaneously investigated from May 2015 to February 2016 with cytometric analyses with one additional sampling obtained in August 2016 without further incubation experiments. Aliquots (1.5 ml) of the different water depth samples were poured into cryotubes, fixed with 0.1% glutaraldehyde (final concentration), and kept frozen (−80°C) in liquid nitrogen until later flow cytometry analysis using a BD AccuriTM C6 cytometer. Cell densities of Synechococcus, Prochlorococcus, pico- (<2 μm) and nano- (between 2 and 20 μm) phytoeukaryotes were determined according to Marie et al. (1999) and converted into carbon biomass based on cell-to-carbon conversion factors of 255 fgC cell–1 for Synechococcus, 36 fgC cell–1 for Prochlorococcus, and 2,590 fgC cell–1 for pico-eukaryotes (Buitenhuis et al., 2012). We used the factor of 132,005 fgC cell–1, considering the mean biomass of dinoflagellates smaller than 20 μm from Menden-Deuer and Lessard (2000), to convert cell densities of the nano-autotrophs into cell carbon.
Results
Environmental Properties
The Ría of Saco do Mamanguá is shielded from wind stress in adjacent open waters. Hence, its hydrographic regime is directly linked to local meteorology and poor freshwater discharge from the local minor hydrographic basin in the adjacent coastal plain. Southeast winds become more frequent in winter seasons from April to August, entering the Ría through its innermost section (Figure 2). These winds push surface waters to the outer section of the Ría, causing turbulence and vertical water column mixing. Seasonal trends of mean monthly air temperatures ranged from 21 to 28°C, closely following seasonal trends of total solar radiation. Mean monthly precipitation varied from 5 mm in winter periods (June to August of 2015 and 2016) up to 558 mm in January 2016.
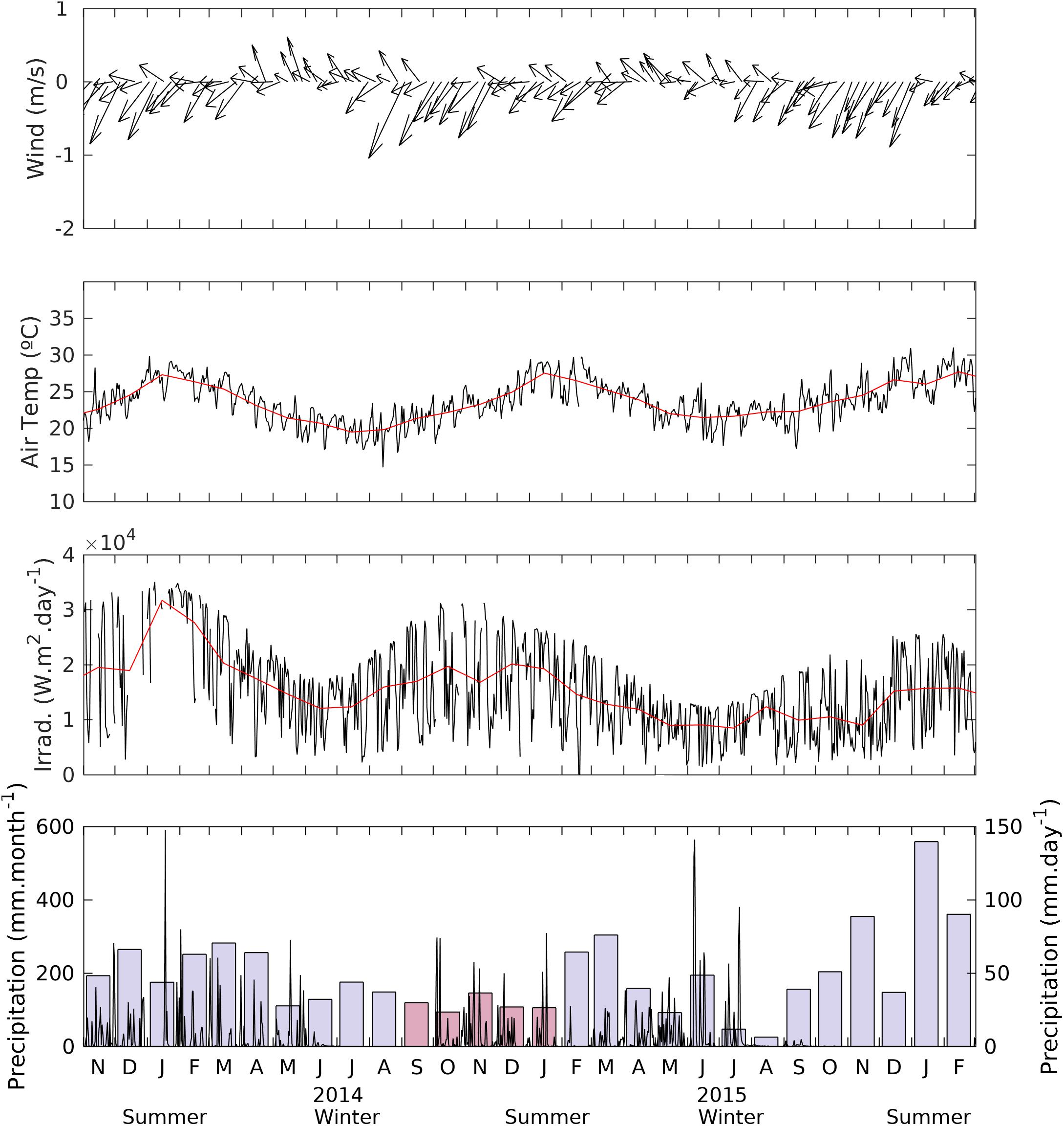
Figure 2. Meteorological data obtained from the Ubatuba coastal research station near the experiment site over the whole study period, wind field (intensity and direction) daily (black lines), and monthly average (red lines) of air temperature and solar radiation. Daily (vertical lines) and monthly (bars) precipitation were obtained from the LabDados database at http://labdados.io.usp.br/). Exceptions are between September 2014 and January 2015 (shown as pink bars) when the precipitation regime was completed with data from the CIIAGRO online database (see text footnote 2). The Supplementary Figure S1 shows a good Pearson’s coefficient of determination (r2 = 0.765; a = 1.0101) between both databases.
Water Column Properties
The vertical distribution of water column properties depicted an unclear seasonal pattern. It alternated between physical stratification and homogeneity (Figure 3) depending on the local wind field and daily radiation from the previous days. Calm winds and high insolation predominated during the summer seasons when surface temperature increased up to 28–30°C (e.g., January 2014 and January/February 2016), decreasing to 21–22°C in colder periods. Even in summer, strong southeast winds may blow sporadically and rapidly homogenize the water column properties, as observed in February 2015. Total solar radiation, along with surface water temperature, decreases during winter becoming vertically homogeneous due to tidal and wind forcing, as seen in April and August 2014, and May 2015. Light extinction coefficients estimated with the Secchi disk revealed light conditions never limited photosynthetic rates at any of the depths where bottles were re-suspended, with bottom intensities of approximately 15% incident surface light throughout the study period. Salinity is usually lower at the surface than in bottom layers due to constant freshwater runoff entering the inlet from the Paraty Mirim drainage basin; it ranged from 30.9 to 34.7 PSU at the surface and from 33.7 to 36.9 PSU at the bottom. The inlet is well ventilated, storing high amounts of dissolved oxygen, ranging between 6 and 7 mg L–1 at the surface and decreasing with depth to between 5 and 6 mg L–1 during stratified periods. Thus, the biochemical demand for dissolved oxygen inside the inlet never offsets atmospheric and biological sources. Even bottom layers tend to be well ventilated by deeper coastal waters entering the inlet during high tides (Figure 3).
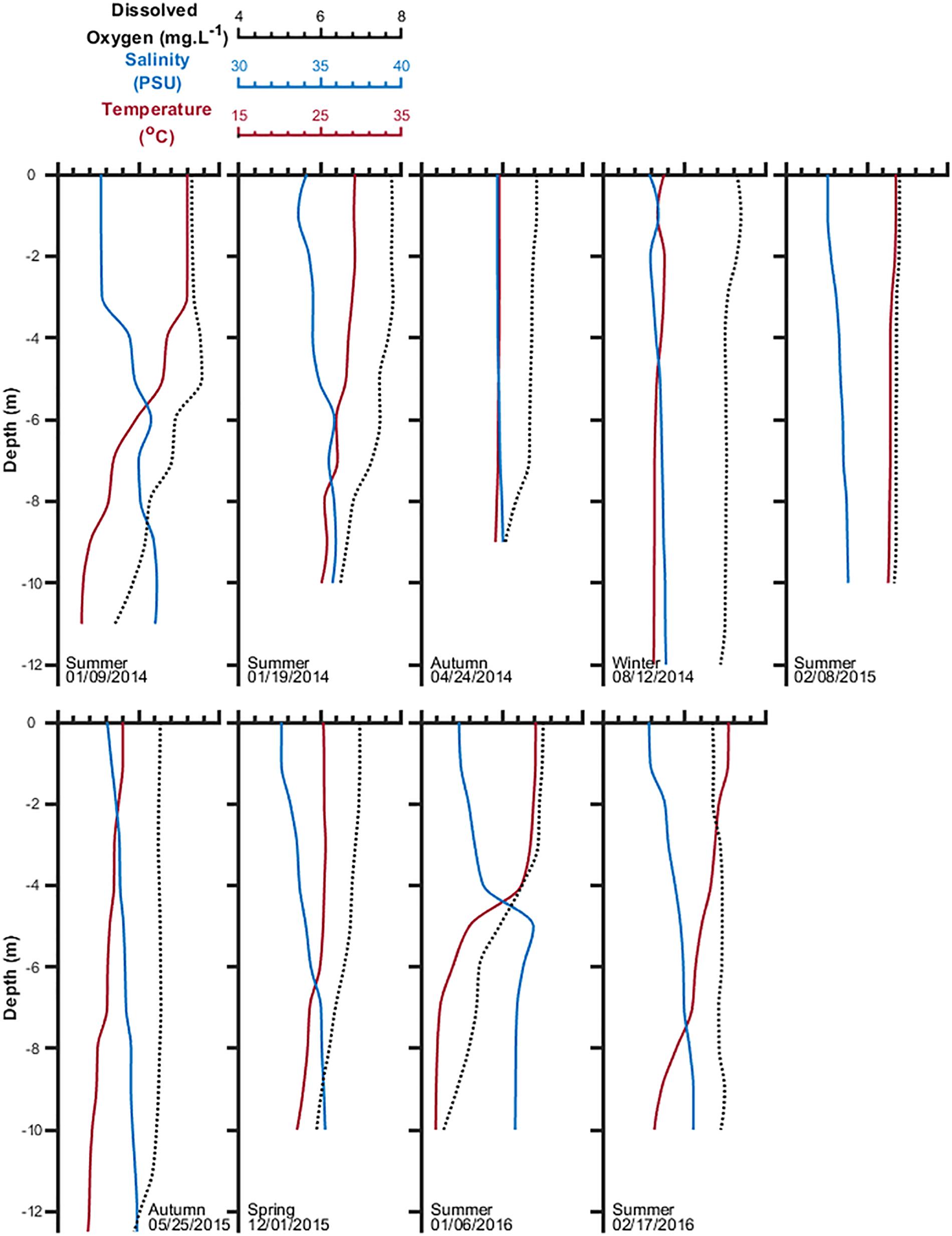
Figure 3. Temperature, salinity, and dissolved oxygen distribution in the water column of the experiment site inside Mamanguá Ría, Bay of Ilha Grande, between January 2014 and February 2016.
Mean Nitrate (+Nitrite)-N and Phosphate-P concentrations in the water column (Table 1) ranged from 0.19 to 0.61 and from 0.17 to 0.44 μM, respectively. Reliable results of ammonium were obtained only in May 2015 (autumn) and January 2016 (summer) when water column concentrations varied, respectively, from 0.04 to 0.13 μM (mean of 0.11 μM) and from 0.4 to 0.85 μM (mean of 0.60 μM). The lowest concentration of Phosphate-P, <0.1 μM, was measured at the surface under highly stratified conditions in January 2014 (summer) (Figure 4), increasing steadily bottomward up to 0.3 μM. Concentrations were higher (0.4–0.5 μM) and homogeneously distributed in the water column in August 2014 (winter) and May 2015 (autumn) under unstratified conditions. The vertical distribution was also irregular in April 2014 (spring) and December 2015 (summer) under weakly stratified conditions depicting concentrations between 0.2 and 0.4 μM in the upper half of the water column, increasing or decreasing downward in April 2014 and December 2015, respectively. Nitrate+Nitrite-N concentration was lowest, between 0.1 and 0.2 μM, in April 2014 and May 2015, increasing bottomward under unstratified conditions. In January and August 2014 and December 2015, concentrations were higher though still limiting to enhance GCP, ranging between 0.3 and 0.5 μM in the upper half of the water column and increasing irregularly bottomward up to 0.5 and 0.7 μM. The relationship between nutrient concentrations and seasonal stratifications was not clear as the lowest and highest concentrations of both Phosphate and Nitrate+Nitrite did not correlate with the seasonal period or stratified conditions. Overall inputs of nutrients to the Ría are constantly low even in summer in spite of increasing continental runoff due to higher precipitation (see Figure 2).
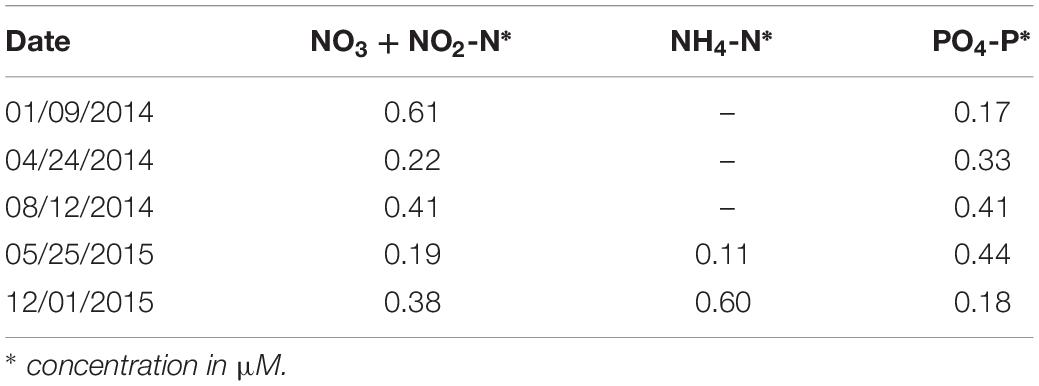
Table 1. Mean (n = 8 except for ammonium) water column concentration of macronutrients at the Mamanguá Ría (Bay of Ilha Grande) experiment site, southeast Brazilian coast, in different seasonal periods.
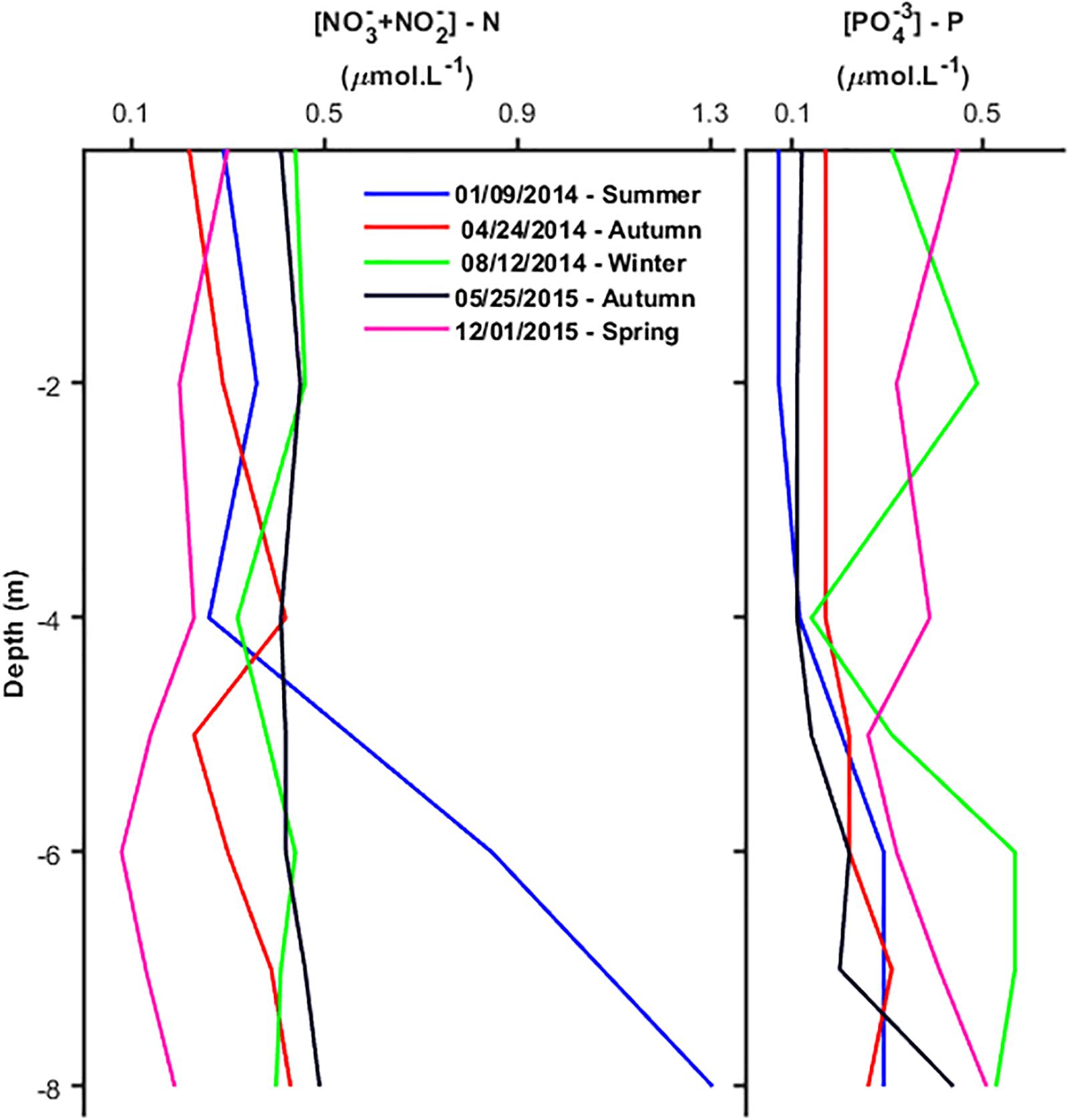
Figure 4. Vertical distribution of Phosphate-P and Nitrate (+Nitrite) – N in the water column of the sampling station inside Mamanguá Ría, Bay of Ilha Grande, in different seasonal periods.
Photosynthetic Pigments and Turbidity
Turbidity ranged from almost 0 to 9 FNU with low values in the upper layers increasing up to one order of magnitude in the near bottom due to particle sedimentation and/or sediment resuspension by tidal currents independently on the seasonal period (Figure 5). However, an opposing trend was observed in early January 2014 (summer) during the first survey of this investigation and, to a lesser extent, later in April (autumn) when turbidity was much higher in surface layers, decreasing downward. The vertical distribution of chlorophyll depicted a regular pattern, with low chlorophyll concentrations of usually <0.5 μg L–1 in surface layers (0–4 m), decreasing with depth, then increasing to much higher concentrations in the near bottom, up to a maximum of 8–9 μg L–1 measured on May 2015 (autumn). The vertical distribution of phycoerythrin, the pigment indicator of Synechococcus, depicted a similar trend confirming the dominance of this coccoid pico-cyanobacterium in the photoautotrophic plankton community. No clear seasonal pattern of pigments and turbidity was observed.
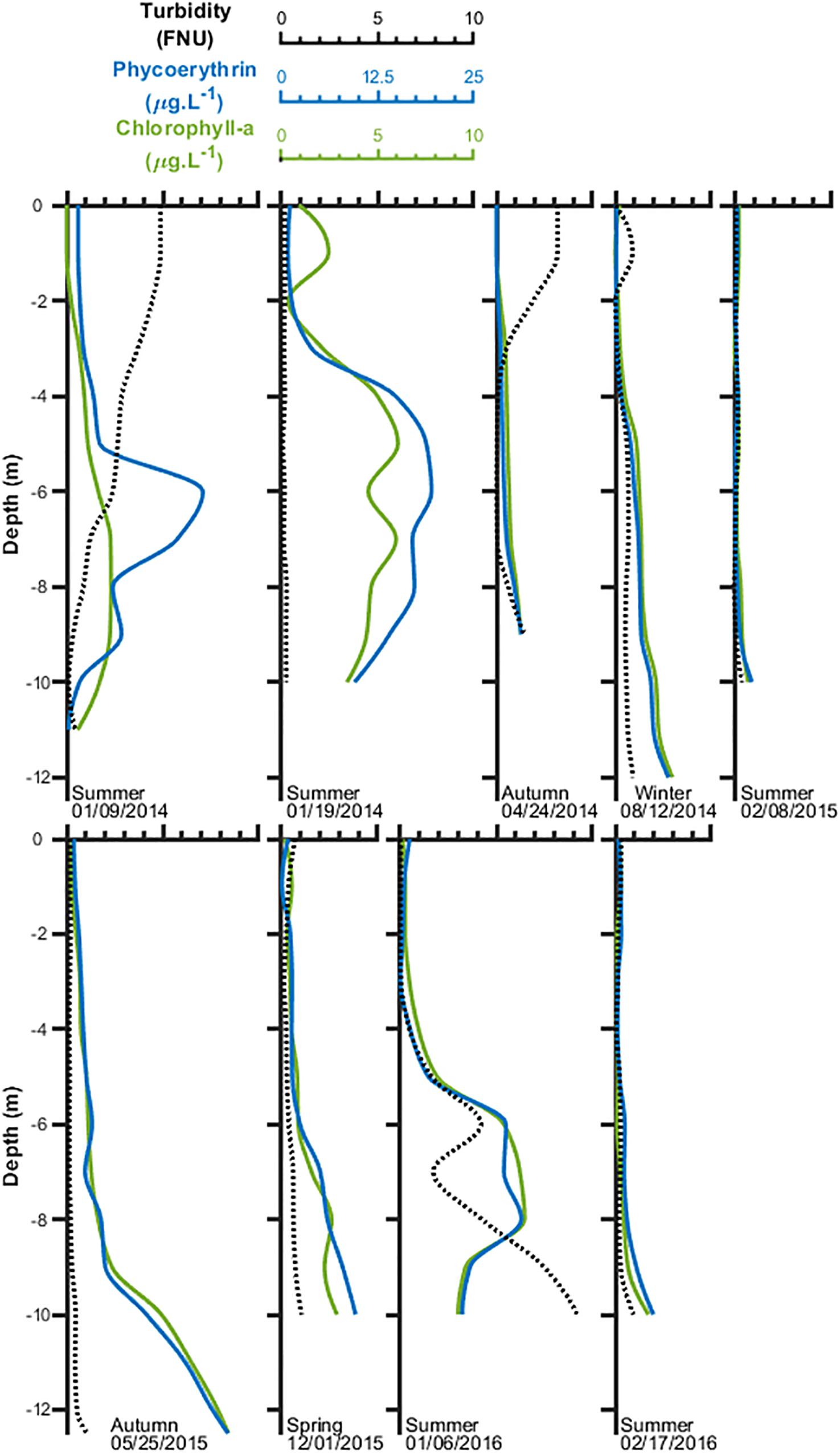
Figure 5. Vertical distribution of turbidity and photosynthetic pigments in the water column of the experiment site inside Mamanguá Ría, Bay of Ilha Grande, between January 2014 and February 2016.
Phytoplankton Density and Carbon Biomass
Cytometric analyses of the nano- and pico-phytoplankton community revealed the numerical dominance of Synechococcus (Table 2) with densities ranging between 99,420 to 192,349 cells mL–1 (water column mean of 157,557 cells mL–1), with the maximum in the surface layers (1–5 m) decreasing with depth. Another frequent autotrophic pico-cyanobacterium was Prochlorococcus, though always in very low concentrations of <20,000 cells mL–1. Pico-eukaryotes were also frequent in low densities ranging from 11,721 to 19,301 cells mL–1. Densities of nano-eukaryotes were lowest among the phytoplankton community though in the same order of magnitude of the pico-eukaryotes, ranging from 2,450 to 6,792 cells mL–1. In spite of low densities, the bulk of the planktonic carbon biomass was concentrated within this nano-sized group, ranging from 118 to 176 μgC L–1 whereas the contribution of Synechococcus to the planktonic carbon biomass was much smaller, varying from <25 to 50 μgC L–1 with the tendency of decreasing with depth during the experimental surveys.

Table 2. Mean density (as cells mL–1) and biomass (between parenthesis as μgC L–1) of pico- and nano-sized planktonic photo-autotrophs measured cytometrically at different depth ranges of the Mamanguá Ría (Bay of Ilha Grande), southeast Brazilian coast, between January 2014 and August 2016.
GCP, DCR, and NCP
Gross community production rates in the Ría (Figure 6) varied from 2 to 31.5 mgC m–3 h–1, highest in upper layers in January 2014. In all other experiments, GCP did not change significantly with depth. Rates at the surface did not differ significantly from those obtained in the deepest layers, ranging from ca 1.1 to 19.3 mgC m–3 h–1. DCR ranged from 0.7 to 25 mgC m–3 h–1, with the minimum in surface layers during colder seasons and maximum near bottom in warmer seasons (e.g., January 2014). NCP varied from −19 to 22 mgC m–3 h–1 with minimum and maximum at the bottom and surface in January and April 2014, respectively, when the planktonic system became autotrophic. In all other experiments, NCP was just slightly positive in surface layers and frequently negative at the bottom.
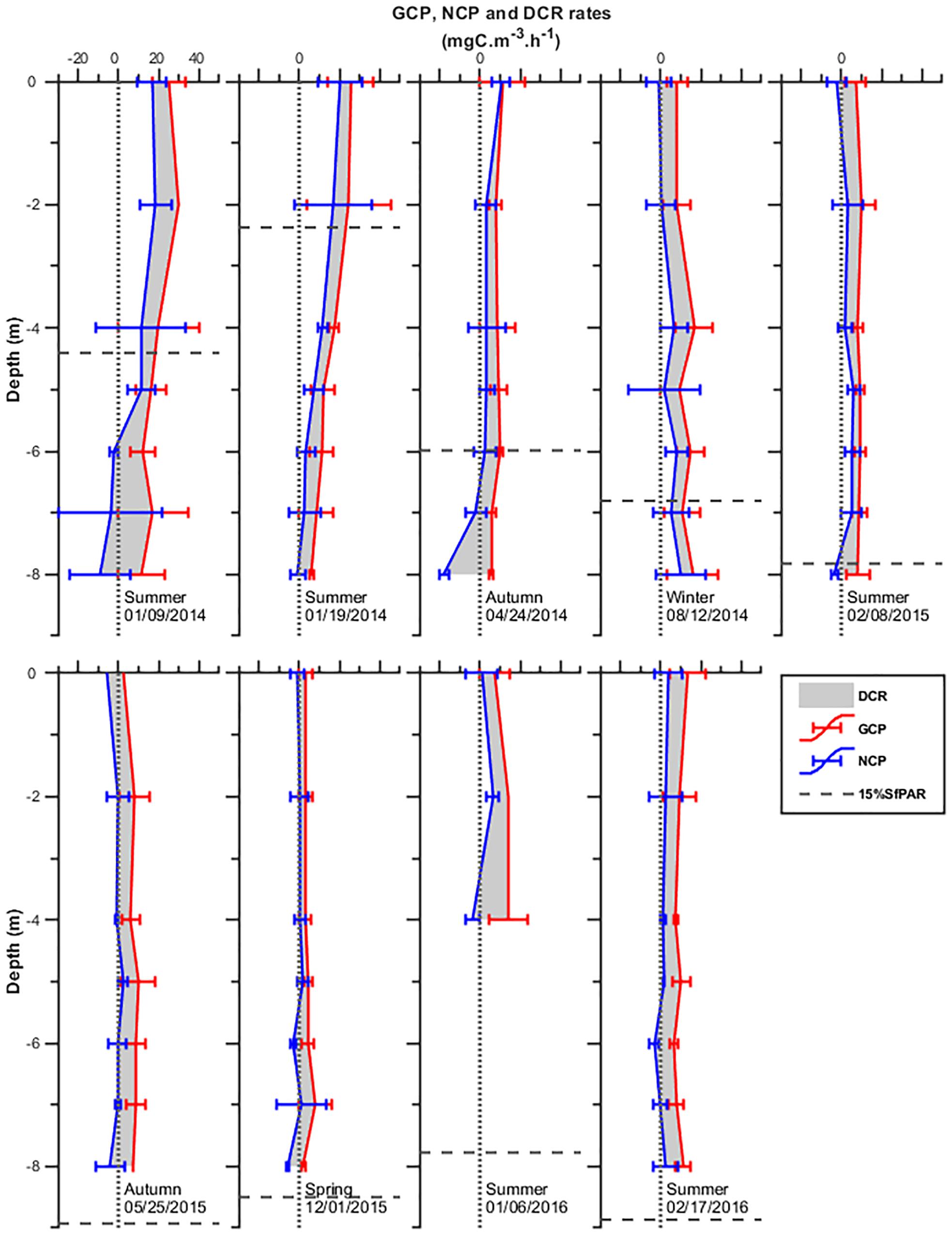
Figure 6. Vertical distribution of gross community production (GCP), dark community respiration (DCR), and net community production (NCP) in the water column inside Mamanguá Ría, Bay of Ilha Grande, between January 2014 and February 2016. The black horizontal dotted line shows the 15% surface light level.
The water column integrated GCP varied from 0.5 to 2.4 gC m–2 d–1 with the highest value on the 9th and 19th of January 2014. In all other periods, it fluctuated between 0.5 and slightly above 1 gC m–2 d–1 with no clear seasonal pattern. DCR was usually higher than GCP, ranging from ca 1.0 to 2.4 gC m–2 d–1 and NCP was positive only in January 2014 (Table 3).
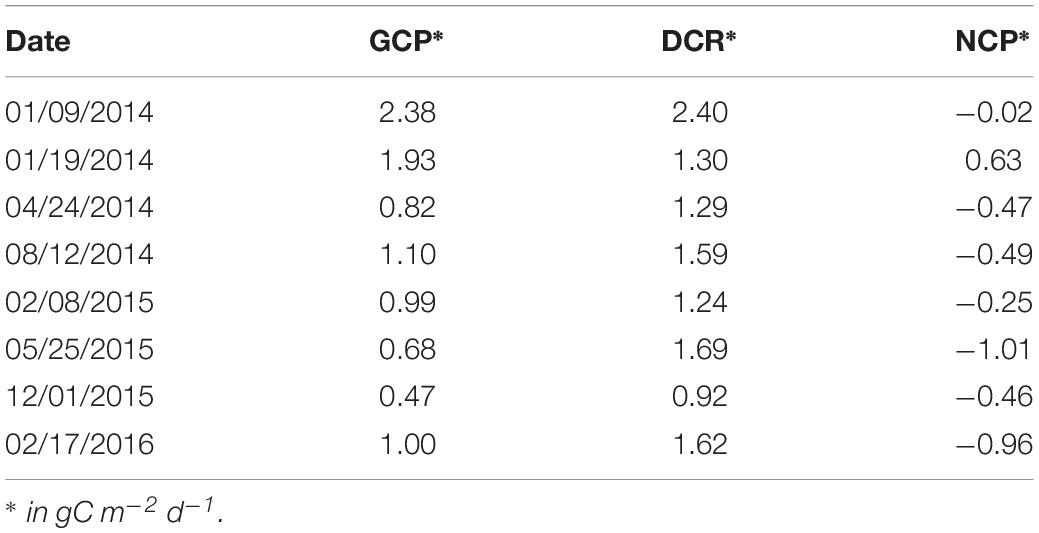
Table 3. Water column integrated gross community production (GCP), net community production (NCP), and dark community respiration (DCR) in the Mamanguá Ría, Bay of Ilha Grande, southeast Brazilian coast, between January 2014 and February 2016.
Discussion
Overall, the seasonal pattern of all hydrographic and biological results was unclear. Inputs of inorganic nitrogen were not sufficient to change the carbon budget of the Ría toward a more autotrophic system. That is the reason why the system is net heterotrophic relying mostly on the carbon pathways of the microbial loop. There were hitherto no previous community production and respiration measurements in the BIG to which our results may be compared. C-14 uptake experiments were conducted in the Bay of Ubatuba (Teixeira, 1973; Teixeira and Gaeta, 1991; Gaeta et al., 1995, 1999) south of the Mamanguá Ría, which according to Ikeda and Stevenson (1980) is dynamically connected with the BIG through coastal water circulation.
These earlier C-14 experiments have reported daily and seasonal variations of photosynthetic rates similar to those obtained here with the oxygen light-and-dark technique. Our range of GCP by water volume (<2 to 31.5 mgC m–3 h–1) are similar to the 0.24 to 28.1 mgC m–3 h–1 reported by Teixeira (1973). On an area basis, our range of 0.46–2.38 gC m–2 d–1 is similar to the range 0.40–1.24 gC m–2 d–1 reported by Gaeta et al. (1999). Exceptional peaks in GCP (1.93 and 2.38 gC m–2 d–1) were measured in January 2014 as the result of chlorophyll-rich coastal waters advected westward from the upwelling system of Cape Frio (Figure 6) by the persistence of strong northeasterly winds (see Figure 2). This chlorophyll-rich water directly affected the biogeochemical conditions of the whole BIG, including the innermost sections.
Gaeta et al. (1999) also performed fractionated filtration of water samples that supports our findings regarding mass contribution of pico-sized cells to the total carbon uptake; while their original study did not include specific taxonomic identification, we now know that their picoplankton community was mostly the cyanobacterium Synechococcus. This organism is ubiquitous and numerically dominates the planktonic photoautotrophs in the global coastal zone (Olson et al., 1990; Partensky et al., 1999). It has been reported as the main pico-autotroph along the subtropical continental margin of the SBB and on eastern shelves (Susini-Ribeiro, 1999; Moser et al., 2014; Gérikas Ribeiro et al., 2016; Bergo et al., 2017) along with a minor contribution from pico-eukaryotes (Gérikas Ribeiro et al., 2016). The hydrographic features encountered inside the Ría are similar to those along the coastal zone and the open inner shelf of the SBB, thus its primary autotrophic carbon biomass also accumulates in Synechococcus cells. In the absence of significant physical input of new nutrients, nitrogen sources for the growth of these cyanobacteria and pico-sized eukaryotes rely mostly on the uptake of ammonium originated from excretion and microbial regeneration within the planktonic metabolism. Indeed, ammonium may reach peak concentrations in the Ría much above the other inorganic nitrogen sources as measured in December 2015 (Table 1). Pico-eukaryotes also contribute to primary production in tropical oligotrophic waters (Partensky et al., 1999; Flombaum et al., 2013; Kirkham et al., 2013) and may be directly transferred to micro-heterotrophs in the second trophic link (Sherr and Sherr, 1984; Kuosa, 1991; Ducklow, 1992; Yun-Chi et al., 2009) of the microbial food web (sensu Azam et al., 1983). Here they were not as abundant as Synechococcus cells, except in April 2016 (autumn) when they contributed with >36% of the total planktonic autotrophs in surface layers. The carbon biomass of heterotrophic bacteria was not estimated in this study, but it has been reported to be at least two orders of magnitude higher than pico-cyanobacteria in the Ubatuba coastal area near our experiment site (Mesquita, 1993; Mesquita and Fernandes, 1996) and within the adjacent shelf system (Gérikas Ribeiro et al., 2016). GCP rates were equally low in either stratified or homogeneously mixed periods, and not directly linked to fertilization in the euphotic zone.
Availability of new nutrients was always low in spite of the wind and tidal-driven water column mixing and lateral advection of nutrient-rich bottom waters from Cape Frio upwelling entering the BIG as observed in January 2014 (Figure 7). The same conclusion was reached by Teixeira and Gaeta (1991) and Gaeta et al. (1999) for the Flamengo inlet in Ubatuba. They also did not find a significant correlation between the temporal dynamics (daily and seasonal) of photosynthesis and nutrient concentrations. Both Flamengo inlet and Mamanguá Ría lack steady nitrogen sources to shift the widespread heterotrophic-dominated ecosystem along the coastal zone of the SBB toward the diatom-dominated autotrophic planktonic system, as seems to be the rule in the geographically limited upwelling and estuarine plumes (Valentin et al., 1987; Fernandes and Brandini, 2004). GCP was also not limited by the water column light regime since light extinction coefficients indicated at least 15% of surface light reaches the bottom layers (Figure 6). The increase of photosynthetic pigments in the bottom layers may be related to photo-adaptation of cells during stratified summer periods, as in January 2014 and January 2016. This photo-adaptation explains why these chlorophyll-rich layers at the lower half of the euphotic zone may not indicate a concomitant rise in GCP (see Figures 5, 6). Vertical distribution of GCP tends to be homogeneous, with no relation to the vertical distribution of chlorophyll. This indicates that photo-adaptation keep the same level of carbon uptake comparing to the surface. Yet pigment-rich particles also accumulated near the bottom even in non-stratified periods (e.g., May 25, 2015) possibly due to sediment resuspension. Northeasterly winds, the most frequent during the study period (Figure 2), blow parallel to the main axis of the Ría and tend to push surface water from the BIG toward the innermost side of the Ría. Wind and tidal current interactions cause turbulence in bottom layers that resuspends chlorophyll-rich sediments.
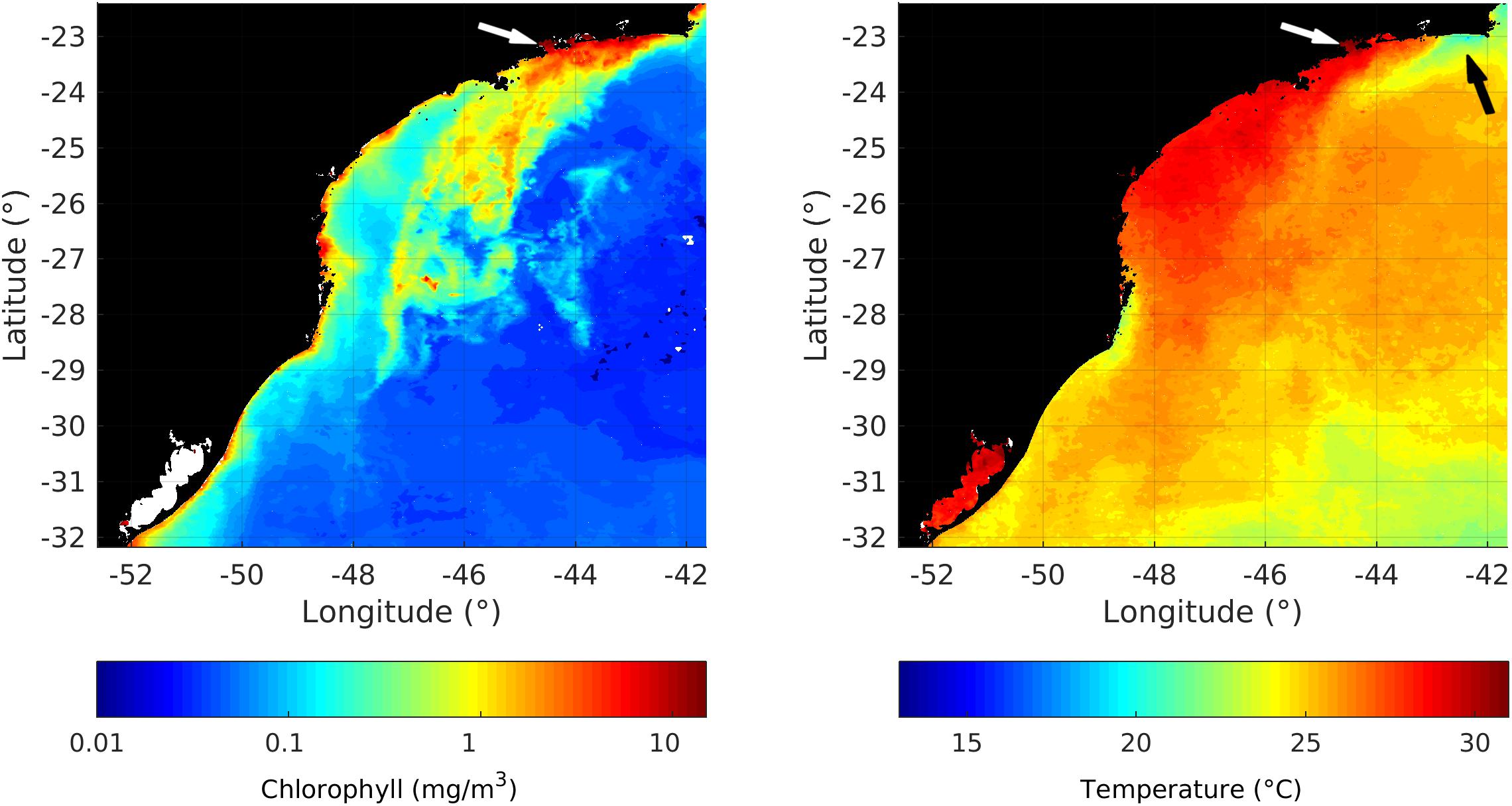
Figure 7. Satellite images of surface chlorophyll and temperature over the southeastern Brazilian margin in January 2014 showing upwelling off Cabo Frio (black arrow) and its associated chlorophyll-rich water advected westward, invading the BIG system (white arrow) (monthly mean from https://giovanni.gsfc.nasa.gov/giovanni/).
There is a highly diverse grazer nanoplankton community in the southeast Brazilian coastal embayments formed mostly by mixotrophic dinoflagellates (Kutner and Sassi, 1979; Sassi and Kutner, 1982; Domingos and Menezes, 1998; Tenenbaum et al., 2006; Villac et al., 2008; Moser et al., 2017) which are possibly the main consumers of Synechococcus, as reported elsewhere (Jeong et al., 2005, and references herein). Indeed, plankton studies in coastal areas of the SBB reported the dominance of heterotrophic nanoflagellates for the carbon biomass (Gomes et al., 2007; Santos et al., 2007). This planktonic structure reflects the meso- oligotrophic conditions of the coastal zone along the SBB where the usual N to P ratios are around 1, limiting the dominance of the primary producers to small photosynthetic cells such as Synecococcus that may rely on sub-micromolar concentrations of nitrogen (Zwirglmaier et al., 2008). With less availability of macro-nutrients, the carbon flow is driven through the heterotrophic bacteria as an alternate (possibly primary) carbon source as usual in oligotrophic plankton metabolism.
Our results suggest that DCR inside Mamanguá Ría is not balanced by the GCP. Net ecosystem production by itself, usually ranging between −0.5 and −1.0 g m–2 d–1, does not sustain the energy flow at low trophic levels. Hence, the offset between daily GCP and DCR, may be balanced by other sources of organic carbon. Nano-eukaryotes contributed with 61.3% of the carbon biomass estimated in the water column of our experiment site. According to our results, they cannot be fully supported solely from the photosynthetic production of cyanobacteria. Mesquita and Fernandes (1996) suggested a predator-prey interaction between nano-heterotrophs and heterotrophic bacteria in the coastal embayments of Ubatuba. Accordingly, the mean water column carbon biomass accumulated in the nano-sized chlorophyll bearing cells we measured cytometrically was 3 times higher than the biomass of the autotrophic pico-prokaryotes mostly represented by Synechococcus (Table 2). We argue that to sustain heterotrophy at the second plankton trophic level, at least three times the carbon biomass is needed over what is available in the cyanobacteria carbon pool. We did not measure heterotrophic eukaryotes; if they were to be included in the carbon budget of the Ria it would take even more carbon biomass to sustain the actual planktonic system. We conclude the planktonic carbon budget in the plankton system of the Saco do Mamanguá Ría is not balanced by autotrophic production. Carbon fixation by Synechococcus, the dominant pico-autotroph, is not enough to sustain DCR on a daily basis. Other sources of carbon, possibly heterotrophic bacteria, must supply the trophic demands of this small subtropical ecosystem. This might be the basic planktonic metabolic scenario encountered in similar hydrographic and meso-oligotrophic conditions, hence representative of the majority of the Brazilian inner shelf waters outside of estuarine plumes and upwelling systems.
Author Contributions
FB conceived the presented idea. LM aided in interpreting the results, worked on the manuscript, carried out the field work, and helped to prepare the figures and tables. GF, GC, and MC carried out the field work, verified the analytical methods, and performed the computations. LJ supervised the project and contributed to the final version of the manuscript.
Funding
This study was financially supported by the Fundação de Amparo à Pesquisa do Estado de São Paulo (FAPESP) project “Avaliação da Produtividade Primária Marinha através do Estudo das Bactérias Magnetotáticas em Sedimentos” (Proc. 2011/22018-3 and Proc. 2018/17061-6).
Conflict of Interest Statement
The authors declare that the research was conducted in the absence of any commercial or financial relationships that could be construed as a potential conflict of interest.
Acknowledgments
We thank the crew members of the R/V Veliger and Alpha Delphini of the Oceanographic Institute of São Paulo University for the helpful support during all field surveys in Saco do Mamanguá Ría. We also thank Linda Waters for her help to improve the grammatical style of the text. We also thank the reviewers for their suggestions that substantially improved the manuscript.
Supplementary Material
The Supplementary Material for this article can be found online at: https://www.frontiersin.org/articles/10.3389/fmars.2019.00584/full#supplementary-material
FIGURE S1 | Pearson coefficient of determination between precipitation data obtained from the LabDados database (http://labdados.io.usp.br/) against the CIIAGRO online database (http://www.ciiagro.sp.gov.br/rede.html).
Footnotes
References
Azam, F., Fenchel, T., Field, J. G., Gray, J. S., Meyerreil, L. A., and Thingstad, F. (1983). The ecological role of water-column microbes in the sea. Mar. Ecol. Prog. Ser. 10, 257–263. doi: 10.3354/meps010257
Benites, M., Pavani, D. A., de los Santos Maly, M., and Jovane, L. (2015). Shallow gas occurence in a Brazilian Ría (Saco do Mamanguá, Rio de Janeiro), inferred from high-resolution seismic data. Cont. Shelf Res. 108, 89–96. doi: 10.1016/j.csr.2015.08.022
Berger, W. H., Smetacek, V. S., and Wefer, G. (eds). (1989). Productivity of the Ocean: Present and Past. Dahlem Workshop Reports, vol. 44, New York, NY: Wiley Interscience. 471.
Bergo, N. M., Signori, C. N., Amado, A. M., Brandini, F. P., and Pellizari, A. H. (2017). The partitioning of carbon biomass among the pico- and nano-plankton community in the South Brazilian bight during a strong summer intrusion of South Atlantic central water. Front. Mar. Sci. 4:238 doi: 10.3389/fmars.2017.00238
Brandini, F. P. (1990). Hydrography and characteristics of the phytoplankton in shelf and oceanic waters off southeastern Brazil during winter (July/August 1982) and summer (February/March 1984). Hydrobiologia 196, 111–148. doi: 10.1007/BF00006105
Brandini, F. P. (2018). “Phytoplankton Assemblages of the Subtropical South West?Atlantic: composition and dynamics in relation to physical?and chemical processes,” in Plankton Ecology of the Southwestern Atlantic – From the Subtropical to the Subantarctic Realm, Part iii - Plankton of Shelf and Boundary Systems, M. S. Hoffmeyer, M. E. Sabatini, F. P. Brandini, D. Calliari and N. H. Santinelli (Berlin: Springer International Publishing), 129–148. doi: 10.1007/978-3-319-77869-3_7
Buitenhuis, E. T., Li, W. K. W., Lomas, M. W., Karl, D. M., Landry, M. R., and Jacquet, S. (2012). Bacterial biomass distribution in the global ocean. Earth Syst. Sci. Data Discuss. 5, 301–315. doi: 10.5194/essdd-5-301-2012
Castro, B. M., Brandini, F. P., Pires-Vanin, A. M. S., and Miranda, L. B. (2006). “Multidisciplinary oceanographic processes on the western Atlantic continental shelf between 4°N and 34°S,” in The Global Coastal Ocean, eds A. R. Robinson and K. H. Brink (Cambridge, MA: Harvard University Press), 259–293.
Domingos, P., and Menezes, M. (1998). Taxonomic remarks on planktonic phytoflagellates in a hypertrophic tropical lagoon (Brazil). Hydrobiologia 369, 297–313. doi: 10.1007/978-94-017-2668-9_26
Ducklow, H. W. (1992). Factors regulating bottom-up control of bacteria bio- mass in open ocean plankton communities. Arch. Hydrobiol. Beih. 37, 207–217.
Dugdale, R. C., and Goering, J. J. (1967). Uptake of new and regenerated forms of nitrogen in marine production. Limnol. Oceanogr. 12, 196–206. doi: 10.4319/lo.1967.12.2.0196
Fernandes, L. F., and Brandini, F. P. (2004). Diatom associations in shelf waters off Paraná State, southern Brazil: annual variation in relation to environmental factors. Braz. J. Oceanogr. 52, 19–34. doi: 10.1590/s1679-87592004000100003
Flombaum, P., Gallegos, J. L., Gordillo, R. A., Rincón, J., Zabalam L. L., and Jiao, N. (2013). Present and future global distributions of the marine cyanobacteria Prochlorococcus and Synechococcus. Proc. Natl. Acad. Sci. U.S.A. 110, 9824–9829. doi: 10.1073/pnas.1307701110
Fuhrman, J. (1987). Close coupling between release and uptake of xsssolved free amoni acids in seawater studied by an isotope dilution approach. Mar. Ecol. Prog. Ser. 37, 45–52. doi: 10.3354/meps037045
Gaarder, T., and Gran, H. H. (1927). Investigation of the production of plankton in the Oslo fjord. Rapp. P.V. Réun. Cons. Perm. Int. Explor. Mer, 42, 1–8.
Gaeta, S. A., Susini Ribeiro, S. M., Metzler, P. M., Francos, M. S., and Abe, D. S. (1999). Environmental forcing on phytoplankton biomass and primary productivity of the coastal ecosystem in Ubatuba region, southern Brazil. Rev. Bras. Oceanogr. 47, 11–27.
Gaeta, S. A., Teixeira, C., Abe, D. S., Lopes, R. M., and Susini, S. M. (1995). Size-fractionation of primary production and phytoplankton biomass on inshore waters of the Ubatuba region, Brazil. Publ. Esp. Inst. Oceanogr. 11, 153–162.
Gérikas Ribeiro, C., Santos, A. L., Marie, D., Pellizari, V. H., Brandini, F. P., and Vaulot, D. (2016). Pico and nanoplankton abundance and carbon stocks along the Brazilian bight. PeerJ 4:e2587. doi: 10.7717/peerj.2587
Gomes, E. A. T., Santos, V. S., Tenenbaum, D. R., and Villac, M. C. (2007). Protozooplankton characterization of two contrasting sites in a tropical coastal ecosystem (Guanabara Bay, RJ). Braz. J. Oceanogr. 55, 29–38. doi: 10.1590/s1679-87592007000100004
Gonzalez-Rodriguez, E., Valentin, J. L., André, D. L., and Jacob, S. A. (1992). Upwelling and downwelling at Cabo Frio (Brazil): comparison of biomass and primary production responses. J. Plankt. Res. 14, 289–306. doi: 10.1093/plankt/14.2.289
Grashoff, K., Kremling, K., and Ehrhardt, M. (eds). (1999). Methods of Seawater Analysis, 3rd Edn. Weinheim: Wiley-VCH Weinhein, 632.
Ikeda, Y., and Stevenson, M. (1980). Determination of the circulation and short period fluctuation in Ilha Grande Bay (RJ), Brazil. Bolm. Inst. Oceanog. 29, 89–98.
Jahnke, R. A., and Craven, D. B. (1995). Quantifying the role of heterotrophic bacteria in the carbon cycle: a need for respiration rate measurements. Limnol. Oceanogr. 40, 436–441. doi: 10.4319/lo.1995.40.2.0436
Jeong, J. H., Park, J. Y., and Nho, J. H. (2005). Feeding by red-tide dinoflagellates on the cyanobacterium Synechococcus. Aquat. Microb. Ecol. 41, 131–143. doi: 10.3354/ame041131
Kirkham, A., Lepère, C., Jardillier, L., and Not, F. (2013). A global perspective on marine photosynthetic picoeukaryote community structure. ISME J. 7, 922–936. doi: 10.1038/ismej.2012.166
Kuosa, H. (1991). Picoplanktonic algae in the northern Baltic Sea: seasonal dynamics and flagellate grazing. Mar. Ecol. Prog. Ser. 73, 269–276. doi: 10.3354/meps073269
Kutner, M. B. B., and Sassi, R. (1979). DinoflagelIates ftom the Ubatuba region (Lat. 230)0’S-Long. 45°06’W) Brazil,” in Toxic Dinoflageliates Blooms, eds D.L. Taylor and H. Seliger (New York, NY: Elsevier), 169–172.
Lignell, R. (1990). Excretion of organic carbon by phytoplankton: its relation to algal biomass, primary productivity and bacterial secondary productivity in the Baltic Sea. Mar. Ecol. Prog. Ser. 68, 85–99. doi: 10.3354/meps068085
Longhurst, A. R. (2006). Ecological Geography of the Sea, 2nd Edn. San Diego, CA: Academic Press, doi: 10.1016/B978-012455521-1/50012-7
Marie, D., Brussaard, C., Partensky, F., Vaulot, D., and Wiley, J. (1999). Flow cytometric analysis of phytoplankton, bacteria and viruses. Curr. Protoc. Cytom. 11, 1–15.
Medeiros, C., Macedo, S. J., Feitosa, F. A. N., and Koening, M. L. (1999). Hydrography and phytoplankton biomass and abundance of North-East Brazilian waters. Arch. Fish. Mar. Res. 47, 133–151.
Menden-Deuer, S., and Lessard, E. J. (2000). Carbon to volume relationships for dinoagellates, diatoms, and other protist plankton. Limnol. Oceanogr. 45, 569–579. doi: 10.4319/lo.2000.45.3.0569
Mesquita, H. S. L., and Fernandes, A. J. (1996). Variação de curta escala temporal de bactérias, picofitoplâncton e nanoheterótrofos na região de Ubatuba -SP, Brasil. Rev. Bras. Oceanogr. 44, 47–56. doi: 10.1590/s1413-77391996000100005
Mesquita, H. S. L. (1993). Densidade e distribuição do bacterioplâncton nas águas de Ubatuba (23°S 45°W), Estado de São Paulo. Publção Esp. Inst. Oceanogr. 10, 45–63.
Moser, G. A. O., Piedras, F. R., Joaquin, A. B., Dousa, D. S. Leles, S. G., and de Lima, D. T. (2017). Tidal effects on phytoplankton assemblages in a near-pristine estuary: a trait-based approach for the case of a shallow tropical ecosystem in Brazil. Mar. Ecol. 38:e12450. doi: 10.1111/maec.12450
Moser, G. A. O., Castro, N. O., Takanohashi, R. A., Fernandez, A. M., Pollery, R. C. G., and Tenenbaum, D. R. (2016). The influence of surface low-salinity Waters and cold subsirface water masses on picoplankton and ultraplankton distribution in the continental shelf off Rio de Janewiro, SE Brazil. Cont. Shelf Res. 120, 82–95. doi: 10.1016/j.csr.2016.02.017
Moser, G. A. O., Takanohashi, R. A., Chagas Braz, M., Lima, D. T., Kirsten, F. V., and Guerra, J. V. (2014). Phytoplankton spatial distribution on the Continental Shelf off Rio de Janeiro, from Paraíba do Sul River to Cabo Frio. Hydrobiologia 728, 1–21. doi: 10.1007/s10750-013-1791-3
Odebrecht, C., and Djurfeldt, L. (1996). The role of nearshore mixing on phytoplankton size structure of Cape Santa Marta Grande, southern Brazil (Spring 1989). Arch. Fish. Mar. Res. 43, 217–230.
Olson, R. J., Zettler, E. R., Armbrust, E., and Chisholm, S. W. (1990). Pigment, size and distribution of Synechococcus in the North Atlantic and Pacific oceans. Limnol. Oceanogr. 35, 45–58. doi: 10.4319/lo.1990.35.1.0045
Partensky, F., Blanchot, J., and Vaulot, D. (1999). “Differential distribution and ecology of Prochlorococcus and Synechococcus in oceanic waters: a review,” in Marine Cyanobacteria, Vol. 19, eds L. Charpy and A. Larkum (Monaco: Musée Océanographique), 457–475.
Poole, H. H., and Atkins, W. R. G. (1929). Photo-electric measurements of submarine illumination throughout the year. J. Mar. Biol. Ass. 16, 297–324. doi: 10.1017/s0025315400029829
Rodelli, D., Jovane, L., Giorgino, M., Rego, E. S., Cornaggia, M., Benites, M., et al. (2019). Diagenetic fate of biogenic soft and hard magnetite in chemically stratified sedimentary environments of Mamanguá Ría, Brazil. JGR Solid Earth 124, 2313–2330. doi: 10.1029/2018jb016576
Santos, V. S., Villac, M. C., Tenenbaum, D. R., and Paranhos, R. (2007). Auto- and heterotrophic nanoplankton and filamentous bacteria of Guanabara Bay (RJ, Brazil): estimates of cell/filament numbers versus carbon contente. Braz. J. Oceanogr. 55, 133–143. doi: 10.1590/s1679-87592007000200006
Sassi, R., and Kutner, M. B. B. (1982). Variação sazonal do fitoplâncton da região do Saco da Ribeira (Lat, 23°30′; Long, 45°07′W), Ubatuba, Brasil. Bolm. Inst. Oceanogr. 31, 29–42. doi: 10.1590/s0373-55241982000200005
Selman, M., and Greenhalgh, S. (2009). Eutrophication: Sources and Drivers of Nutrient Pollution. Washington, DC: World Resources Institute.
Selman, M., Greenhalgh, S., Branosky, E., Jones, C., and Guiling, J. (2009). Water Quality Trading: An International Overview. Washington, DC: World Resources Institute.
Sherr, B. F., and Sherr, E. B. (1984). “Role of heterotrophic protozoa in carbon and energy flow in aquatic ecosystems,” in Current Perspectives in Microbial Ecology, eds M. J. Klug and C. A. Reddy (Washington, DC: American Society for Microbiology), 413–423.
Signorini, S. R. (1980). A study of the circulation in Bay of Ilha Grande and Bay of Sepetiba Part II. A survey of the circulation based on experimental field data. Bolm. Inst. Oceanogr. 29, 41–55.
Smith, W. O. Jr., and DeMaster, D. J. (1996). Phytoplankton biomass and productivity in the Amazon River plume: correlation with seasonal river discharge. Cont. Shelf Res. 16, 291–319. doi: 10.1016/0278-4343(95)00007-n
Strickland, J. D. H., and Parsons, T. R. (1972). A Practical Handbook of Seawater Analysis, 2nd Edn. Otawa, ON: Bulletin of the Fisheries Research Board of Canada, 310.
Susini-Ribeiro, S. M. (1999). Biomass distribution of pico, nano, and microplankton on the continental shelf of Abrolhos, East Brazil. Arch. Fish. Mar. Res. 47, 271–284.
Teixeira, C., and Gaeta, S. A. (1991). Variação nictemeral da clorofila-a, produção primária do fitoplâncton e fatores ambientais da região de Ubatuba (Lat.23°30′5 Long.45°06’W). Bolm. Insl. Oceanogr. 39, 15–24. doi: 10.1590/s0373-55241991000100002
Teixeira, C. (1973). Preliminary studies of primary production in the Ubatuba region (Lat. 23°30′5 - Long. 45 0 06’W), Brazil. Bolm. Inst. Oceanogr. 22, 49–58. doi: 10.1590/s0373-55241973000100003
Tenenbaum, D.R.T., Gomes, E.A.T., and Guimaraes, G.P. (2006). “Microorganismos planctônicos: Pico, Nano e Micro,” in Características hidrobiológicas da região central da zona econômica exclusiva brasileira, ed. J. L. Valentin (Salvador: Editora Ideal), 83–124.
Valentin, J. L., Andre, D. L., and Jacob, S. A. (1987). Hydrobiology in the Cabo Frio (Brazil) upwelling: two-dimensional structure and variability during a wind cycle. Cont. Shelf Res. 7, 77–88. doi: 10.1016/0278-4343(87)90065-3
Villac, M. C., Cabral-Noronha, V. A., and Oliveira Pinto, T. (2008). The phytoplankton biodiversity of the coast of the state of São Paulo, Brazil. Biota Neotrop. 8, 151–173. doi: 10.1590/s1676-06032008000300015
Wielgat-Rychert, M., Rychert, K., Zbigniew-Witek, Z., and Zalewski, M. (2017). Calculation of the photosynthetic quotient (PQ) in the Gulf of Gdanìsk (southern Baltic). J. Ecol. Prot. Coastline 21, 51–60. doi: 10.3750/aip2005.35.1.07
Williams, P. J. L. (1981). Incorporation of microheterotrophic processes into the classical paradigm of the planktonic food web. Kieler Meeresforsch. Sonderh. 5, 1–28.
Yun-Chi, L., An-Yi, T., and Kuo-Ping, C. (2009). Trophic coupling between Synechococcus and pigmented nanoflagellates in the coastal waters of Taiwan, Western Subtropical Pacific. J. Oceanogr. 65, 781–789. doi: 10.1007/s10872-009-0065-1
Keywords: plankton metabolism, gross primary production, net community respiration, Mamanguá Ria, Bay of Ilha Grande, southeastern Brazil
Citation: Brandini F, Michelazzo LS, Freitas GR, Campos G, Chuqui M and Jovane L (2019) Carbon Flow for Plankton Metabolism of Saco do Mamanguá Ría, Bay of Ilha Grande, a Subtropical Coastal Environment in the South Brazil Bight. Front. Mar. Sci. 6:584. doi: 10.3389/fmars.2019.00584
Received: 11 January 2019; Accepted: 03 September 2019;
Published: 18 September 2019.
Edited by:
Michelle Jillian Devlin, Centre for Environment, Fisheries and Aquaculture Science (CEFAS), United KingdomReviewed by:
Punyasloke Bhadury, Indian Institute of Science Education and Research Kolkata, IndiaSuzanne Jane Painting, Centre for Environment, Fisheries and Aquaculture Science (CEFAS), United Kingdom
Copyright © 2019 Brandini, Michelazzo, Freitas, Campos, Chuqui and Jovane. This is an open-access article distributed under the terms of the Creative Commons Attribution License (CC BY). The use, distribution or reproduction in other forums is permitted, provided the original author(s) and the copyright owner(s) are credited and that the original publication in this journal is cited, in accordance with accepted academic practice. No use, distribution or reproduction is permitted which does not comply with these terms.
*Correspondence: Frederico Brandini, YnJhbmRpbmlAdXNwLmJy