- 1Kiel Marine Science, Kiel University, Kiel, Germany
- 2Environmental Research Division, NOAA Southwest Fisheries Science Center, Monterey, CA, United States
- 3Marine Research Division, AZTI Tecnalia, Pasaia, Spain
- 4Institute of Oceanography, Rio Grande Federal University, Rio Grande, Brazil
- 5NOAA Alaska Fisheries Science Center, Seattle, WA, United States
- 6College of Earth, Ocean, and Atmospheric Sciences, Oregon State University, Corvallis, OR, United States
- 7NOAA National Centers for Environmental Information, Silver Spring, MD, United States
- 8Institute of Marine Science, University of California, Santa Cruz, Santa Cruz, CA, United States
- 9Instituto de Tecnología y Ciencias Marinas, Universidad Simón Bolívar, Caracas, Venezuela
- 10NOAA Southeast Fisheries Science Center, Miami, FL, United States
- 11European Commission Joint Research Centre, Directorate D—Sustainable Resource, Unit D.02 Water and Marine Resources, Ispra, Italy
- 12Norwegian Polar Institute, Fram Centre, Tromsø, Norway
- 13CSIRO Oceans and Atmosphere, Hobart, TAS, Australia
- 14Physical Sciences Division, NOAA Earth System Research Laboratory, Boulder, CO, United States
- 15GEOMAR Helmholtz Centre for Ocean Research Kiel, Kiel, Germany
- 16Lowestoft Laboratory, Centre for Environment, Fisheries and Aquaculture Science, Lowestoft, United Kingdom
- 17Inter-American Tropical Tuna Commission, La Jolla, CA, United States
- 18Laboratório de Oceanografia Biológica and Laboratório de Pesquisa em Monitoramento Ambiental Marinho, Instituto de Geociências, Universidade Federal do Pará, Campus Guamá, Belém, Brazil
- 19Institute for Marine and Antarctic Studies, University of Tasmania, Hobart, TAS, Australia
- 20Departamento de Estudios Ambientales, Universidad Simón Bolívar, Caracas, Venezuela
- 21MARE – Marine and Environmental Sciences Centre, Laboratório Marítimo da Guia, Faculdade de Ciências da Universidade de Lisboa, Lisbon, Portugal
- 22School of Science and Engineering, University of the Sunshine Coast, Maroochydore, QLD, Australia
- 23Laboratoire de Météorologie Dynamique, IPSL, Ecole Normale Supérieure, Paris, France
- 24Department of Microbiology, Department of Civil, Environmental and Geodetic Engineering, and the Byrd Polar and Climate Research Center, The Ohio State University, Columbus, OH, United States
- 25Farallon Institute for Advanced Ecosystem Research, Petaluma, CA, United States
- 26Fisheries Research Division, NOAA Southwest Fisheries Science Center, La Jolla, CA, United States
- 27Department of Oceanography, Dalhousie University, Halifax, NS, Canada
- 28Innovation Research Center, School of Management, Federal University of Rio Grande do Sul, Porto Alegre, Brazil
Advances in ocean observing technologies and modeling provide the capacity to revolutionize the management of living marine resources. While traditional fisheries management approaches like single-species stock assessments are still common, a global effort is underway to adopt ecosystem-based fisheries management (EBFM) approaches. These approaches consider changes in the physical environment and interactions between ecosystem elements, including human uses, holistically. For example, integrated ecosystem assessments aim to synthesize a suite of observations (physical, biological, socioeconomic) and modeling platforms [ocean circulation models, ecological models, short-term forecasts, management strategy evaluations (MSEs)] to assess the current status and recent and future trends of ecosystem components. This information provides guidance for better management strategies. A common thread in EBFM approaches is the need for high-quality observations of ocean conditions, at scales that resolve critical physical-biological processes and are timely for management needs. Here we explore options for a future observing system that meets the needs of EBFM by (i) identifying observing needs for different user groups, (ii) reviewing relevant datasets and existing technologies, (iii) showcasing regional case studies, and (iv) recommending observational approaches required to implement EBFM. We recommend linking ocean observing within the context of Global Ocean Observing System (GOOS) and other regional ocean observing efforts with fisheries observations, new forecasting methods, and capacity development, in a comprehensive ocean observing framework.
Introduction
Fisheries advice and fisheries management have a long history of requiring ocean observing. More than 500 million people rely on fisheries and other harvests from marine ecosystems for protein and livelihoods (FAO 2018 report). Fisheries and marine ecosystems are addressed in at least two of the UN Sustainable Development Goals (SDGs): SDG 2 (hunger) and SDG 14 (life below water). SDG 14 has six targets tied to marine life and associated ecosystem services. What ocean observing capacities and information delivery strategies are required to meet these goals?
Sustained ocean observing to address the needs of fisheries management is complex. It requires integrating observations across biological, physical and biogeochemical domains from the coast to the open ocean, including the deep sea. It must also consider human stressors and the economy, to inform policy and decision makers. Sustained ocean observing includes multiple elements purposefully linked into an “ocean observing supply chain.”
The ocean observing supply chain can best be understood by starting with the element for which society, or an end-user, articulates a need for an ocean-derived product. To properly create such a product, a dialogue needs to be established between end-users and the scientific community. The approach will define the details of the sampling strategy including time, space, parameter, setting, etc. and sampling methods, including different technologies. The collected data then moves to the next element of the supply chain concerned with integration and product generation. Products are then distributed to the end user. Feedback mechanisms help improve data collection and product quality. Organizations like the International Council for the Exploration of the Sea (ICES) in the North Atlantic, and to some extent the North Pacific Marine Science Organization (PICES) in the North Pacific, have developed frameworks to identify observing targets, to coordinate data collection internationally, and to create observing products that inform users while simultaneously identifying gaps and ways to improve the elements and their linkages.
An overarching strategy for ocean observing has been outlined in the “Framework for Ocean Observing” (FOO; Lindstrom et al., 2012). The “Global Ocean Observing System” (GOOS) provides society with information on ocean health and climate for operational services (e.g., ocean forecasts). The FOO is applicable across disciplines, including biology, physics, biogeochemistry and geology. The FOO is divided into three elements: Requirement Processes, Coordination of Observing Elements, and Data Management and Information Products. In order to assess and eventually improve the readiness of the three elements and to address the observing objectives of GOOS, the FOO uses the “Technological Readiness Level” (TRL) approach. The FOO is a strategic document, and despite its intention to structure the many ocean observing efforts that had been presented at the OceanObs09 conference (Hall et al., 2010) leaves aside implementation.
However, the FOO strategy has been important to guide the improvement of ocean observing efforts by defining Essential Ocean Variables (EOVs; Lindstrom et al., 2012). One of the EOVs is “fish abundance and distribution” (Miloslavich et al., 2018a). Ocean observing in the context of GOOS and ocean observing for fisheries advice have been historically separated from one another. Clearly, a closer link will benefit both sides. For GOOS and the implementation strategy for the FOO, seeking advice on ocean observing capacity and needs for fisheries could be a great benefit. Likewise, fisheries observing may benefit from a TRL analysis (as proposed in the FOO) to detect weak links and further improve the respective elements of the ocean observing supply chain. We propose that a major focus in defining EOVs in support of ecosystem-based fisheries management (EBFM) is to identify the needs of fisheries management and to evaluate existing data and link to other ocean observations to obtain a global understanding of fish abundance and distribution in the ecosystem context.
There are known issues with collecting observations for effective fisheries management. There is often a diversity of observing data available, but these are often not integrated to assess fisheries in an ecosystem context. Moreover, not all users of particular ocean spaces are considered, and thus certain societal needs are not addressed.
The Ocean Sciences Meeting (Portland, United States; February 2018) and The Effects of Climate Change on the World’s Oceans Symposium (Washington, DC, United States; June 2018) brought together ocean observing communities to discuss these issues. Topics included: current status in observing climate, ocean, eco- and human system related processes and variables; integration across systems; climate, ocean, socioeconomic objectives and major societal needs that should be addressed; improvements and adaptations to monitoring systems and models to achieve these goals; and identification of the major obstacles and stepping-stones. Building on these meetings and other experiences we summarize the current status, key gaps, and future needs related to building the observational capacity to effectively monitor and manage the world’s marine ecosystems and fisheries.
Ocean Observing to Serve the Needs of Multiple Fisheries and Ecosystems User Groups
Information needs and societal requirements vary with user groups. Recognizing the connections between users and their technology, disciplines, temporal and spatial scales, and institutions is critical to ensure that ocean observing meets the needs of society. Hence, ocean observing must follow an open architecture particularly regarding information sharing. With respect to data, a strategy has been developed branded as “FAIR” (Findable, Accessible, Interoperable, and Reusable; Wilkinson et al., 2016), ideally guiding data collection and use. While data sharing is implicit in many ocean observatories, access to biological data is often more constrained. There is a lack for centralized access and storage. Biological data are collected over different spatial and temporal scales, often with a wide variety of disparate methods and by different agencies and entities. Such lack of uniformity undermines research productivity and the production of information for management.
Communication with end-users will help modify or adapt the ocean observing supply chain according to user needs. These are some of the critical groups that have to be linked in this chain:
Resource users are the stakeholder groups that include commercial, recreational, and indigenous fishers, aquaculture, and eco-tourism operators, fish processors, and retailers. They require information on ocean conditions, resource distribution, and abundance. They generally do not require primary data, but products tailored to specific needs. Thus, a dialogue between data integrators and this user group should include advisory information as well as information about the resource returned to the larger community.
Scientists are a multifaceted stakeholder group. They collect, generate, and use primary observations and products (e.g., indicators, maps). Their goal is to advance basic and applied understanding of the marine system. Scientific knowledge is of critical importance at a number of key places in the ocean observing supply chain: scientific results lay out the observational approach that is used to collect and integrate data to address societal requirements. Data integration techniques, assessments, and prediction all are based on scientific evidence. Scientists unravel natural variability (e.g., climate variability) or anthropogenic influences (e.g., climate change, fisheries, resource extraction). They set experimental conditions, parameterize, test and validate models, initialize ocean forecasts, and characterize hazards and pollution. What is often underestimated is the value of ocean observing to inform other scientific communities including the social sciences and economics.
Collectively, these applications advance our understanding of ocean ecosystems and the key processes relevant to their structure and function. For example, ecosystem models require data for various species groups at a range of trophic levels, and often ontogenetic life stages, to simulate the effect of various ocean pressures (e.g., scenario testing for fisheries impacts). Mid-trophic levels (e.g., zooplankton and forage fish) that link low with high trophic levels are currently underrepresented in sampling efforts. So are standardized biological, physical and chemical data and metadata that help characterize suitable habitat at different life stages for many (most) species. Addressing such gaps will enhance our ability to predict the consequences of current and future actions.
Resource Managers (both for living and non-living resources) are charged with regulating access to marine resources, ensuring its sustainable use. They include fishery managers, conservation managers, and tourism managers. This stakeholder group requires products that assist in defining trade-offs between different management aims, assessment techniques, and data collection programs. Resource managers must base decisions on the best available information. They may operate with imperfect knowledge of regional and local marine ecosystem condition, to address critical societal needs. Managers are in a unique position to inform ocean observation strategies for better societal value.
Policy-makers require short and condensed information about ocean observing results and products. Policy ultimately sets the formal rules and frameworks that regulate resource users, managers, and the operating space for scientists. Information on ocean conditions allows competent reporting on local conditions toward, e.g., SDGs, and drive the establishment of new regulations and guidelines. The development of stronger scientific and user knowledge from ocean observing, including local and traditional knowledge, enables the assessment of ongoing changes in the oceans and their impacts on societies and economies. Scientific evidence derived from ocean observations also provides a means for more informed policy-making, particularly to strengthen environmental governance in the oceans and connect ocean science with societal needs.
The General Public and the Media often seek information on trends and patterns derived from ocean observing. This includes information on the status of ecosystems, including on the sustainability of marine resources, and pollution. They rarely seek access to primary databases but typically need synthesized information [e.g., indices of performance, causes for mass mortality events, harmful algal blooms (HABs), plastic abundance, or Sargassum blooms]. This group plays a major role leading policy makers. It is a priority to promote and facilitate to reliable and well-tailored information to this group.
Data Availability for Fisheries and Ecosystem Studies
Ecosystem science and its application to resource management is interdisciplinary. It involves many different scientific, stakeholder, and user groups. This presents a special impetus toward data sharing and integration. A mature data management system will include components for data transport, preservation, continued quality assurance and quality control (QA/QC), discovery, and access. Generally, each of these components will be supported by community-accepted standards, including criteria for data formats, metadata, and web services. The physical sciences have progressed further than biological sciences in this regard, providing experience that should be leveraged where possible.
The major challenge of incorporating climate (the long-term mean ambient physical and chemical conditions) data into ecosystem study, including modeling and monitoring, is to get measurements from their source to the user in a useful and easy way. Ecosystems include the interaction of biological organisms with these same conditions as well as with each other. The main interest in monitoring climate variables for ecosystem studies is in monitoring their change over time and how organisms react to these changes. The main oceanographic climate variables are represented by the physical and biogeochemistry categories of the EOVs. Some of the variables, such as sea surface temperature and salinity, sea surface elevation, sea ice, and ocean color, can be measured synoptically from satellites and are widely available, though not always of sufficient resolution or accuracy for management of ocean uses. Many observations including subsurface temperature, salinity, oxygen, nutrients, carbon, and other tracers, can be measured from research ships, ships of opportunity, and autonomous platforms (e.g., moored buoys, Argo floats, gliders). The Argo program (Jayne et al., 2017) has developed effective data management plans for getting timely, quality-controlled data to users. Other EOVs have programs for data quality control and dissemination (e.g., the Global Ocean Data Analysis Project for Carbon – GLODAP; Lauvset et al., 2016).
For ecosystem work on continental shelves and in coastal areas, there is some national coordination on particular EOVs. Examples include the Biological and Chemical Oceanography Data Management Office (BCO-DMO1) for the National Science Foundation of the United States, NOAA’s National Centers for Environmental Information (NCEI),2 the Marine Data Portal of the Institut Français de Recherche pour L’exploitation de la Mer3 in France, and the Biochem database of Fisheries and Oceans Canada4.
There needs to be more international coordination on measuring EOVs (Muller-Karger et al., 2018a). International exchange of climate data for ecosystems is important for countries that share ecosystems, but also for an understanding of similar ecosystems globally. The Joint Technical Commission for Oceanography and Marine Meteorology (JCOMM) is attempting to address this lack of data availability with the Marine Climate Data System (MCDS). The MCDS envisions six to ten data areas, where a data area covers one or more EOVs measured by multiple observing systems. Currently there are two areas established, subsurface ocean profile data and ocean trajectory (currents) data, with marine meteorological data area coming soon. In the data areas, the MCDS is setting up a structure of Data Assembly Centers (at the project, institution, national level) which will feed data to a set of Global Data Assembly Centers, which will in turn feed a Center for Marine Meteorology and Oceanographic Climate Data (CMOC). The mission of the CMOC is to ensure global data dissemination of high-quality ocean and marine meteorological climate data. The World Ocean Database (WOD; Boyer et al., 2018) is a CMOC for ocean profile data, which includes many EOVs including temperature, salinity, oxygen, nutrients, and ocean tracers. The WOD contains almost 16 million historic and recent ocean profile sets including many from shelf and coastal areas. Much work needs to be done to complete the setup of the MCDS and ensure the maximum flow of timely data through the system from measurement to user.
Even with the flow of climate data, merging these data with ecosystem data, such as plankton measurements, is still a challenge. Ecosystem measurements are often not part of projects which share their data freely and in a timely manner. There are a few national and international data aggregation projects for biological measurements. The Ocean Biological Information System (OBIS5) and the Coastal and Oceanic Plankton Ecology Production and Observation Database (COPEPOD6) are two examples. Both OBIS and COPEPOD couple biological data with climatological mean physical and chemical EOVs. Marine Mammals Exploring the Oceans Pole to Pole (MEOP; Treasure et al., 2017) and BirdLife International seabird tracking7 are examples for higher level biological organisms. However, to study the effects of climate change on an ecosystem, in situ measurements close in space and time should be coupled with the biological observations. The WOD contains more than 450,000 historic and recent plankton tows and stations, but the focus of WOD is on the physical and chemical variables, not biological. OBIS and WOD have been working together to match concurrent or nearby physical and chemical measurements with the biological observations. This and similar collaborations are necessary to best utilize climate monitoring for ecosystem study. An MCDS area for plankton data would ensure coordination of efforts and dissemination of the maximum amount of ecosystem data.
Another challenge is that standardized data formats and standards of metadata differ among communities. Borrowing from the satellite community, the subsurface physical and chemical EOV community has, to a large extent, adopted Climate-Forecast (CF) compliant netCDF formatting for easy machine-to-machine data exchange. Major projects such as Argo and OceanSITES use this self-describing format. Climate variables are usually less complex than biological variables in terms of metadata and data formatting; thus, biological data are not easily represented in the compact and complete exchange format of netCDF. The WOD has a specific netCDF structure for plankton data which is outside the CDF conventions and of little use in machine-to-machine exchange. Still, it would be useful for the biological EOV observation community to agree standards to facilitate global data exchange. An advantage is access to data exchange and tools which should easily accommodate any data.
To summarize, the formalization of the JCOMM MCDS can facilitate the aggregation and dissemination of climate variables in both the open ocean and in coastal and shelf regions important to ecosystem monitoring and fisheries applications. The synthesis of global collections of plankton (and higher organism) observations with concurrent or close-by climate variables will assist in the study of climate change effects on ecosystems. The standardization of exchange formats and the possible use of netCDF standards and DarwinCore (OBIS-ENV-DATA) standards for biological data in the ecosystem community will reap the benefit not only of easier dissemination of ecosystem data, but of greater access to climate data.
Technology for Fisheries and Ecosystem Studies
Technology has always had a significant role in large-scale ocean observing, but it has become even more vital in recent years. Rudimentary, mechanical and in situ sampling has been exponentially complemented by more powerful, systematic, digital, and remote sampling techniques. The development of more potent computer systems and data storing capacity, custom genetics and robotics, automatic sampling drifting devices, GPS-based electronic tags for marine organisms, and newer and more precise optical sensors for satellites and cameras (Barnes et al., 2019) have opened new horizons for the holistic observation and monitoring of the oceans, fisheries and ecosystems. The oceans and ocean observing systems are now better connected through global satellite cell phone service and seafloor cables allowing key environmental information to be available in real time. Fisheries studies have used satellite data more and more to infer relationships between species and the environment (e.g., Druon et al., 2016), but are now assembling additional information such as genetic data or biologging information that reflect animal movements and habitat use at both individual and population level (e.g., Dragon et al., 2015; Blanco Gonzalez et al., 2016; Druon et al., 2016). Genetic (Rodriguez-Ezpeleta et al., 2017) or chemical biomarkers (Rooker et al., 2014) of natal origin are being applied to understand the spatial distribution and mixing of populations in the ocean (Djurhuus et al., 2018) and inform resource management.
The combination and use of a large variety of devices and techniques has significantly increased the ability of scientists to assess the links between small, medium and large-scale processes occurring in the ocean, their connection with marine ecosystems and its multi-sectoral users. The benefits of integrating multiple data sources for better science and more comprehensive ecosystem advice are unquestioned. However, if the quality of advice is to be improved, we must either have common standards to ensure data harmonization and availability, or we need to consider governance of the data collections not only at the level of the individual monitoring program.
Sea-going fisheries surveys should collect relevant environmental data (EOVs) as marine organism abundances are collected. These data should be organized in common formats and available for regional and global assessments of the state of life in the sea. These measurements could be added to existing expensive, yet already funded, cruises by addition of new, relatively low-cost, small robust sensors (e.g., salinity-temperature-depth, dissolved oxygen, chlorophyll fluorescence) to existing sampling equipment (e.g., Keller et al., 2010). Alternatively, dedicated environmental sampling could be done during non-fisheries sampling on the same vessel, for example during the night watch. Another approach is to coordinate the use of autonomous surface vehicles and underwater gliders to map physical, chemical and bio-optical fields in the region where fisheries data are being collected.
Oceanic features of importance for marine ecosystems such as fronts (Druon et al., 2019) can be of relatively small spatio-temporal dimension (Druon et al., 2005; Abrahms et al., 2018; Scales et al., 2018) requiring high resolution observations. The tremendous ocean observing capacity of satellites was foreseen in the 1960s (Ewing, 1965). Progress has since been regularly reviewed (Barale et al., 2010; Muller-Karger et al., 2013). Technological improvements offer global observation of our oceans with an increasing return frequency and spatial resolution. Satellite remote sensing of ocean color, which provides the primary biological observation from space (surface chlorophyll-a concentration), is currently offering major improvements with sub-hourly frequency from geostationary platforms (GOCI sensor launched in 2010, MeteoSat Third generation satellites in 2020) at regional scale and spatial resolution down to 300 m (Sentinel-3 OLCI sensor since 2016) at global scale (Kwiatkowska et al., 2016). New satellite technologies promise the routine observation of Essential Biodiversity Variables (Muller-Karger et al., 2018b).
Satellites, however, only observe the ocean surface or near-surface and. In situ ocean observing platforms and models are required to measure and interpret the state of the interior of the ocean. Many of observing networks exchange best practices on platform handling, sensor calibration, survey strategies, data quality control and data sharing (Pearlman et al., 2019). This approach should occur on the global scale, for example under the auspices of the JCOMM.
Sampling ships can be divided into two groups: (1) dedicated research and monitoring vessels that will sample according to a well-defined survey plan, and (2) ships of opportunity that follow a route set by commercial (e.g., container ship) or other (e.g., yacht) interest. Research vessels allow for the most sophisticated survey types and are a cornerstone for almost all marine observing programs. As mentioned above, research vessels could be made more efficient by allowing them to concentrate on making measurements that cannot be done from the new autonomous vehicles, for example, net sampling for zooplankton and fish, while coordinating with autonomous vehicles that would supply the subsurface oceanographic fields.
Ships of opportunity have a more limited survey character (acoustics, depth limitations for probes, often only near surface observations such as the Continuous Plankton Recorder) but often operate with a high frequency rate (daily ferry crossing or weekly open ocean surveys). The other big group, the autonomous systems, includes moorings, gliders and free drifting buoys. Free drifting buoys include, since the early 2000s, the Argo network of >3500 devices that profile the upper 2000 m every 10 days, acquiring temperature, salinity, pressure, and mean drift current (at 1500 m). A subset of the Argo array has recently included chemical (pH, pCO2, oxygen and nitrate) and optical sensors (chlorophyll fluorescence, turbidity). Single floats can operate autonomously over a period of more than 3 years. Underwater gliders are observing devices with navigation capabilities that dive currently to 1000 m depth and profile. These can include passive and active acoustics with maximum survey times of 2–6 months. Most underwater gliders measure physical EOVs (temperature, salinity, pressure, depth-averaged velocity) and many sample a subset of biogeochemical EOVs (dissolved oxygen, turbidity, chlorophyll fluorescence, PAR). New sensors include passive (Lembke et al., 2018) and active (e.g., Benoit-Bird et al., 2018) acoustics, although power requirements for the latter usually significantly shorten mission lengths. Moorings (including landers) are installations at a fixed position. They can carry rather heavy instrumentation and thus have even a larger sensor portfolio, for example the same multi-frequency acoustics as carried by fisheries research vessels. However, the sensors are in many cases installed at fixed depth and vertical profiling is only now becoming more routine (e.g., SeaCycler; McLane profilers; shallow 200-m profilers on the U.S. Ocean Observatories Initiative8). Gliders and moorings allow for higher data quality compared to Argo floats because they are recovered after mission.
Today, many of these platforms carry biogeochemical sensors (e.g., pH, pCO2, O2). Some key variables that are not directly measured from satellites, such as surface-water pCO2, can be assimilated and estimated using algorithms combining remotely sensed observations of surface salinity, temperature and chlorophyll (e.g., Chierici et al., 2012). Yet it is clear that the technology exists now to measure actual biological and biodiversity variables that are required to understand ecosystem states in a manner relevant to fisheries. These cannot be inferred with simplistic measures of chlorophyll fluorescence (Boss et al., 2018).
Most of the techniques, systems and devices mentioned above have been originally designed and improved following scientist and monitoring agency needs, whereas other equipment, like fish echo sounders, temperature sensors for fisheries or oil platforms and others, have been developed for non-scientist users. Yet, the latter can also be used for scientific purposes. Satellite linked echo-sounder buoys associated to fish aggregating devices (FAD) used by the tropical tuna fisheries are a notable example. Tens of thousands of these buoys are deployed globally every year (Scott and Lopez, 2014) to provide fishers with accurate geolocation information of the floating object and a rough estimate of the biomass underneath (Lopez et al., 2014). Several authors have noted the advantages these devices can provide to systematically and remotely study the pelagic environment at different levels in a cost-effective manner, including ecological, behavioral and ecosystem investigations (Moreno et al., 2016).
The combination of ocean surface data (satellites) with in situ data (ships and autonomous systems) represents the basis for a constant improvement of the operational 3D data-assimilative ocean models. Marine observation services, such as the Copernicus Marine Environment Monitoring Service9, provide regular and systematic reference information on the physical and biological state, variability and dynamics of the global ocean and planktonic ecosystems (von Schuckmann et al., 2018). There has been impressive success in assimilating satellite surface chlorophyll maps into 4D ocean circulation and biogeochemical models (Song et al., 2016). These operational products and our knowledge of ocean dynamics under climate change and human impact will only be improved if observational capacity continues and increases in spatial and temporal resolution.
The sampling with traditional means of trophic levels higher than plankton in the ocean is even more challenging. The high diversity of marine species, the difficulties to accurately identify them with fisheries-independent data, and the dynamics of their spatio-temporal distribution is affected by complex behavioral, ecological, biological and movement patterns. Technologies such as optical (video monitoring), acoustics (pelagic species), electronic tagging, acoustic tracking of fish, robotics (drones), microchemistry, and genetic methods have begun to provide individual and population level insights on the complex links between prey and predators, spawners and recruits, and population structure (Boss et al., 2018). Each of these ecosystem components are often highly impacted by both climate variability and fishing activities. Electronic tagging in particular provides unique information on movements that can infer animal behavior and its distribution dynamics in complex marine ecosystems (Bograd et al., 2010; Block et al., 2011). Improving electronic tags with better positioning algorithms and systems, particularly for non-GPS systems attached to permanently underwater animals, together with the implementation of physiologic recorders in the tags (levels of hormones and other indicators of ontogenetic and biologic stages), will substantially increase our knowledge of the ecology of marine species (Crossin et al., 2014).
Complementary genetic and microchemistry studies would help in understanding species and ecosystem connectivity at local scales for relatively low mobile species (i.e., below 100 km radius) and at large scale for highly migratory species (Pecoraro et al., 2018). This informs about the connectivity between spawning grounds and nurseries such as by transport of pelagic larvae (Selkoe et al., 2016). This knowledge on essential habitats for high trophic level species and the links with low trophic levels (Druon et al., 2019) will be key to improve the robustness of full ecosystem models and the management of marine ecosystems.
Ecosystem models make attempts to describe bottom-up and top-down controls and relationships of the marine food chains including the effect of climate change and fishing mortality (Kaplan and Marshall, 2016). However, because they are highly data demanding, these models are slowly making progress and are most often used to describe long-term trends and first-order processes. More harmonized and available data collection, and the development of derived products such as forage or high trophic level habitats, will allow increasing robustness of these models and their use in a more comprehensive Ecosystem Based Management (EBM) framework. The development of linked open databases on the marine environment, such as the European Marine Observation and Data Network10, will favor progress of these complex models. In parallel, the use in near-real time of the simpler habitat products in a dynamic fisheries management framework allows additional and immediate benefits. For instance, these habitat maps allow nursery areas or non-target species to be actively avoided by fishing vessels (Hobday and Hartog, 2014; Druon et al., 2015; Lewison et al., 2015). The success of implementing an ecosystem-based management that ensures conservation and a sustainable use of the oceans, seas and marine resources (UN Sustainable Development Goal 14) will largely depend on the availability and quality of data recorded, processed and issued by the advanced technologies. Moreover, it relies on the capacity of the scientific community to integrate these data in their studies and the ability to find synergies between experts in data collection, marine ecology and numerical modeling.
Observational Approaches to Ecosystem Based Management
Single species stock assessment remains the most common source of tactical scientific advice to set fisheries quotas (Hilborn and Walters, 2013). Increasingly, ecosystem information is being incorporated into traditional stock assessments. Ecosystem information is integral to EBFM (Figure 1, Patrick and Link, 2015; Hollowed et al., 2013). As ecosystems change in response to climate variability, continuous ocean observations are needed to reduce uncertainty for ongoing biophysical predictions and, in turn, for different management approaches. For example, long-term time-series of observations are required to inform environmental drivers of stock-recruitment relationships in multi-species stock assessments, in full ecosystem models including predator-prey interactions or habitat analysis. These approaches are complementary, allowing management questions to be addressed more precisely. For example, MSEs can be used to predict the impacts of varying management decisions and, when underlying models include climate interactions, MSEs can be used to evaluate the effects of these decisions under assumptions about changing climate (Weijerman et al., 2016).
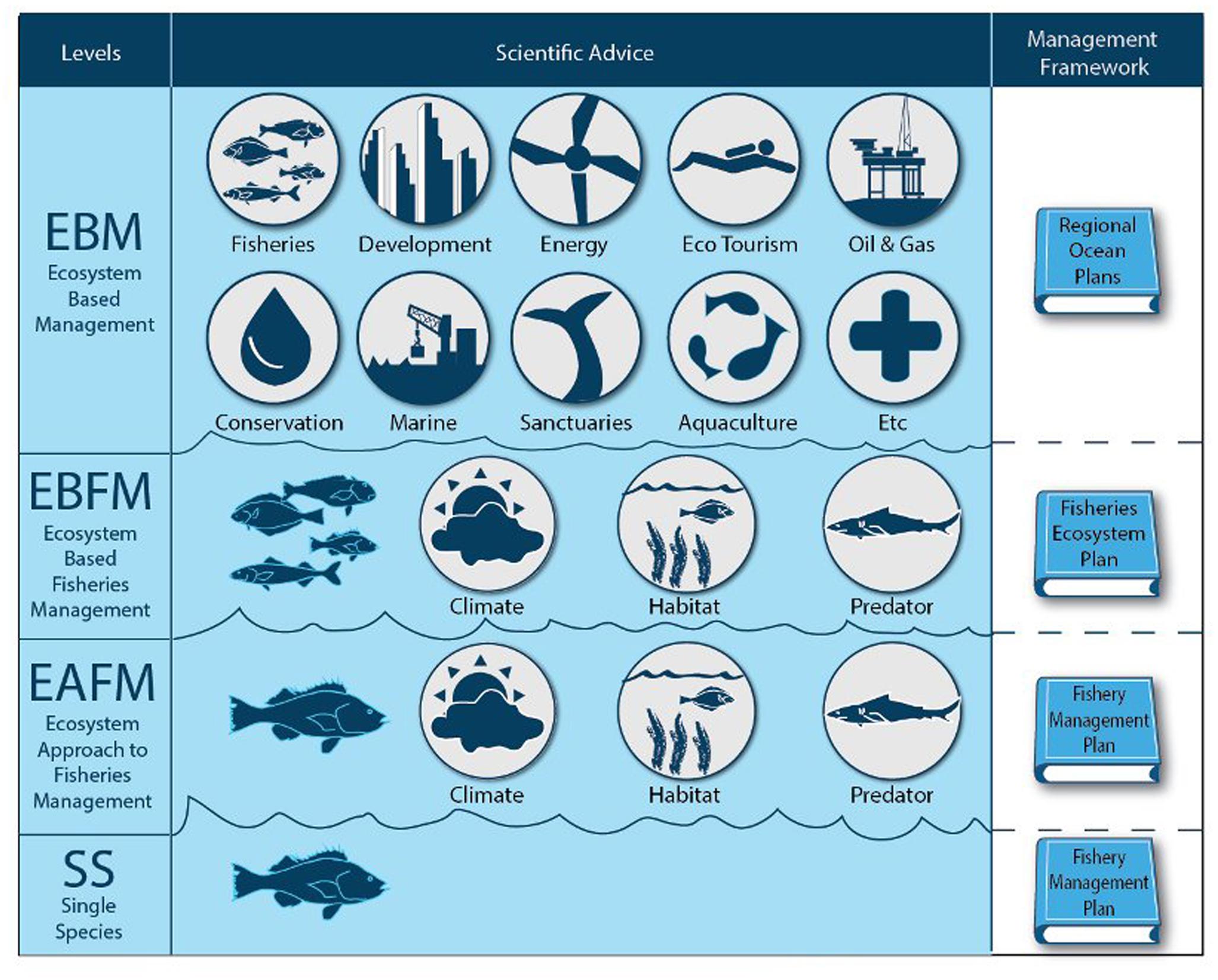
Figure 1. From single stock to Ecosystem Based Management – after Dolan et al. (2016).
In recent years, alternative management strategies have emerged that aim to better integrate ecosystem dynamics to reduce conflict across multi-sectoral ocean users. One such approach is dynamic ocean management (DOM), where management changes in space and time in response to the shifting nature of the ocean and its multi-sectoral users (Maxwell et al., 2015). DOM tools rely on the integration of observational data from biological, oceanographic, or socio-economic sources to better align the spatiotemporal scales between biophysics and ocean users (Lewison et al., 2015; Maxwell et al., 2015; Welch et al., 2018). To date, the emerging field of DOM research has covered a diverse range of biota (e.g., from scallops to tuna and turtles, as summarized in Maxwell et al., 2015), objectives [e.g., conservation outcomes to industry, adaptation to climate variability (e.g., Spillman and Hobday, 2014; Mills et al., 2017; Hazen et al., 2018)] and levels of data availability [e.g., data-poor to fishery-independent to satellite telemetry (e.g., Howell et al., 2008; Hazen et al., 2016; Brodie et al., 2017)].
Dynamic ocean management in the form of ecological forecasts has emerged as a useful tool to understand, predict and respond to ocean changes on a variety of spatiotemporal scales (Payne et al., 2017; Tommasi et al., 2017). Ecological nowcasts and forecasts up to seasonal timescales are being used as decision-support tools to help marine sectors adapt to climate change and variability (Payne et al., 2017; Tommasi et al., 2017; Hobday et al., 2018). For example, ecological forecasts can be disseminated in near real-time for immediate action (e.g., for conservation purposes; Howell et al., 2008; Hazen et al., 2018; Lewison et al., 2018), or on longer timescales (monthly to annually) for diverse applications, including identifying environmental variability (Hobday et al., 2016), hazardous events (e.g., HABs; Stumpf et al., 2009) and fisheries resources (e.g., Burke et al., 2013; Eveson et al., 2015; Kaplan et al., 2016; Mills et al., 2017).
Case Studies of Ocean Observing Applications to Fisheries and Ecosystem Studies
Several case studies illustrate the importance of ocean observing systems to fisheries and ecosystem studies.
North Atlantic Fisheries Management: ICES
International Council for the Exploration of the Sea has focused on the implementation of the ecosystem approach, while maintaining the existing expertise for the provision of advice needed by fisheries management. It has drawn together much of the existing knowledge on North Atlantic ecosystems and developed ecosystem and fisheries overviews that express our understanding of how human activities are influencing the development of ecosystems. Through its transparent and scientifically collaborative process, ICES has tried to link evidence to qualitative and quantitative forms of advice. At the same time ICES recognizes the proliferation of data usage for different purposes and recognizes the need to document data provenance and quality assurance. More recently ICES has recognized the need for much more work in the area of understanding societal demands and priorities in order to improve the utility of its advice (Human Dimensions).
The data sources needed to provide comprehensive ecosystem advice are varied and diverse, but most importantly ICES, like many other organizations, currently has relatively little influence over the development and implementation of monitoring programs beyond fisheries observations and is largely passive in the use of other data sources (with some exceptions11). Even for fisheries information, such as fisheries-independent surveys ICES serves as a coordination body, but ultimately monitoring decisions are taken at the national level which means it is difficult to steer the development of ecosystem advice or to guarantee consistent forms of advice when relying on externally available data.
Sargassum Influx: Impacts, Monitoring, and Trends
The “golden tides” are a new phenomenon in many offshore and coastal regions of the world. Pelagic Sargassum species (Sargassum natans and S. fluitans) have been recorded in new regions since 2011, from the Amazon shelf to the African coast, and the frequency of Sargassum mats has been rising (Smetacek and Zingone, 2013). Sargassum can also introduce non-native species, potentially threatening the existing state of local ecosystems (Macaya et al., 2016). The environmental impacts range from hypoxia, transport of non-native species, to economic losses in tourism and fisheries, making the monitoring of the algal mats an important issue for management and impact mitigation (van Tussenbroek et al., 2018).
The first record of mass occurrence of mats off the northern Brazilian coast was reported in July 2011 (Széchy et al., 2012), while another mass stranding was reported in Western Africa. During April 2014, a 100 tons of Sargassum stranded on Brazilian Amazon beaches, but the highest records were observed in 2015 when an enormous stranding was recorded (April and May), and algal slicks were spotted next to the Brazilian oceanic islands, probably coming from the eastern side of the South Atlantic (Sissini et al., 2017). Sargassum declined in 2016, but increased again in 2017 and reached record biomass estimates in 2018 for the wider Caribbean region (WCR).
Some hypotheses have been raised to explain this phenomenon. Some have implicated nutrient runoff coming from different land-uses like deforestation, mining, cattle farming and agriculture may lead to coastal eutrophication (Gollnow and Lakes, 2014). Pelagic Sargassum is limited mainly by phosphorus (Lapointe, 1995) and at least one hypothesis suggests that coastal eutrophication has led to Sargassum blooms. However, these hypotheses are challenged by the scale of the blooms of Sargassum in the interior of the ocean. Other factors affect brown algae production, including higher SST, acidification, and reduced herbivory. The occurrence in new areas can also be caused by changes in surface circulation patterns in the Atlantic, particularly in the South Equatorial Current (Taylor et al., 2012). The roles of all of these factors needs to be investigated, and a monitoring program should to be implemented, to address the societal need to understand the occurrence of Sargassum and to develop mitigation measures.
The Caribbean Regional Fisheries Mechanism (CRFM) has reported declines in fish production starting in 2014, following a period of increase between 2003 and 2013, a timing that matches the most recent Sargassum blooms. However, more direct effects on fisheries also occur, including clogging of nets and motors. Sargassum also changes the fish species composition as it serves as spawning, nurturing and feeding areas for tuna, Coryphaena hippurus, Rachycentron canadum, Seriola dumerili, and Caranx spp. Thus, while catch of some species might increase, the overall effect on local fisheries may be detrimental.
Negative effects are also observed for tourism in the Caribbean, since tourists avoid the beaches due to the smell released by Sargassum degradation. In addition, dead fish, turtles and other animals are often found in the strandings. Sulfur compounds from decomposing algae are known for mild intoxication of local communities. Alternatively, the algal biomass has already been used as a fertilizer in some Caribbean islands, a possible sustainable solution for the stranded biomass (Hinds et al., 2016).
A growing concern on Sargassum impacts, mainly in the WCR, has led to the development of detection tools and warning systems. Satellite tracked drifters, coupled with oceanographic models, have generated useful results (Gower et al., 2013). Back traces from the Sargassum sightings on the Amazon Shelf since 2009 were made using a high-resolution numerical ocean circulation model which pointed to areas in the North Equatorial Recirculation Region (NERR) off Brazil as regions of Sargassum accumulation and blooming (Johnson et al., 2013).
Early advisory systems have been developed since 2013 (Webster and Linton, 2013), and advanced prediction through analysis of the Sargassum loop system using remote sensing technology has been developed (Dierssen et al., 2015). Additionally, a monthly Sargassum prediction outlook bulletin is already available for the WCR from MODIS observations (Hu et al., 2016; Wang and Hu, 2017). Consultancy agencies like Fulcrum and CLS have also developed early warning systems for some of the Caribbean islands. Tourism dependent islands may now be notified with predictions of arriving Sargassum slicks from the sea, improving management and action planning. As we are reaching satisfactory methods to predict Sargassum mats in some areas, the suite of causes of the events are still unclear. Experimental approaches and interdisciplinary research, coupled with near-real-time monitoring of oceanographic conditions, will be necessary to better understand the phenomenon, improve the predictions, and clarify the possible impacts on fish stocks.
Alaska Cod
The severe marine heatwave which affected the Gulf of Alaska (GOA) from 2014 to 2016 (Bond et al., 2015; Di Lorenzo and Mantua, 2016) provided a shock-test of the management system and EBFM practices currently employed in North Pacific groundfish fisheries. The heatwave precipitated a severe decline (47% in spawning biomass from 2013 to 2017) in the GOA Pacific cod (Gadus macrocephalus) stock resulting in a recommended 77% decrease in the acceptable biological catch (ABC) limit for 2018 from that recommended in 2016. For 2010–2015 the GOA Pacific cod fishery averaged $103 million US in ex-vessel earnings per year, making up 29% of the value of the GOA groundfish fisheries (Fissel et al., 2016). The reduction in allowable catch is a substantial economic hit to a region already experiencing an economic crisis due to reductions in salmon harvests. Although EBFM did not help in the prediction of the decline in GOA Pacific cod, the integration of EBFM with the cooperative and adaptive fisheries management system provided an evidence-based explanation for the decline allowing for a quick and uncontroversial downward adjustment to the final catch limits.
The GOA Pacific cod stock is assessed annually using a single-species age-based stock assessment (Barbeaux et al., 2017). Unique to the North Pacific and key to EBFM has been the annual production of Ecosystem Status Reports (ESRs; Zador et al., 2017). The ESRs informs the North Pacific Fisheries Management Council (NPFMC) of the ecological status and trends within each large marine ecosystem under its purview, as well as global trends. ESRs have been presented to the NPFMC since 2014 (Zador, 2014, 2015; Zador and Yasumiishi, 2016, 2017) prior to presentation of stock assessments. Since 2015, the GOA ESRs have identified substantial impacts of the 2014–2016 marine heatwave. Besides the physical oceanographic indications of warmer than normal conditions, the ESRs pointed to changes in biological conditions including drops in abundance and health of a number of key species (Zador and Yasumiishi, 2017).
In the last week of September 2017 the AFSC summer longline (annual) and bottom trawl survey (biennial) abundance estimates became available to the stock assessment lead author. Both surveys provided precise estimates indicating large drops in abundance of Pacific cod (−53 and −71%, respectively). Including these new data points in the assessment model resulted in a sudden drop in spawning biomass, switching the projected trend from increasing to steeply decreasing. This triggered a scramble by the assessment author to verify the survey results with other data sources and ascertain how the adult population may have decreased in such a sudden fashion. Two other fishery independent surveys previously not examined confirmed a sudden decrease in abundance during the 2014–2016 heatwave. Observer fishery data from the 2017 directed fishery was investigated and drops in catch per unit effort (CPUE) and decreases in Pacific cod fish condition (weight-at-length) were observed throughout the GOA. Bycatch of Pacific cod was also investigated in other non-target fisheries and precipitous decreases in encounter rates of Pacific cod were observed during the heatwave.
A precautionary management approach was implemented for this stock and a higher natural mortality during the heatwave was assumed which resulted in an overall reduction in the estimated spawning population. The 2017 assessment model allowed natural mortality to be fit independently for 2015–2016 resulting in a 45% increase in the natural mortality rate for this period. This new model which was adopted by the NPFMC suggested a severe cut in maximum ABC for 2018 and 2019. The GOA Pacific cod fishery has an additional rule which closes the directed fishery if the stock drops below a threshold to insure adequate Steller sea lion (Eumetopias jubatus) forage.
EBFM in the North Pacific groundfish fisheries relies on the integration of information through both single-species stock assessments and ESRs which in turn rely on data collected and indices summarized from a broad range of platforms and agencies with a shared responsibility among stakeholders. It should be highlighted that no one entity or observation series dictates the success of EBFM in the North Pacific. The lack of objections to the reduction in Pacific cod quotas during NPFMC public testimony by the fishing industry can be attributed to several factors, not least of which is an engaged fishing industry which strongly believes in shared responsibility for fisheries health. This attitude by the fishing industry has been fostered through long-term (1982 to present) co-monitoring of the groundfish resources in Alaska with research surveys conducted on board commercial fishing vessels using fishing captains and crew paired with scientists, a history of on-board observers (1989 to present) recording biological and environmental information of catch funded by the fishing industry, open communication among the industry, managers, and fisheries scientists both formally during public meetings and informally during the fishing season, and co-management of the groundfish stocks through the open NPFMC process. Although the magnitude of the decline in GOA Pacific cod abundance had been surprising, fishers had been reporting poor fishery performance in the spring and summer of 2017, the ESRs presented to the NPFMC had identified emerging ecosystem concerns 2 years previous, and fishers, as well as managers, had been notified in 2016 that a decline in Pacific cod was likely in coming years due to the heatwave impacts on recruitment. The impact of the heatwave on adult Pacific cod, although unexpected, fit within an already well-described narrative of its potential ecosystem impacts.
Brazil Skipjack and Sardine Catch Variability
Sardine and skipjack tuna fisheries along the South West Atlantic Ocean have a high social and economic relevance for fishers, producers, factories and markets. They support local consumption of sardines and the export of canned tuna. However, over the last 26 years, the catches and the biomass of these species have shown significant variability. This variability may not be driven only by changes in fishing effort, but by nutrient supply and SST. It is well known that climate variability can impact local fisheries (Badjeck et al., 2010) and the region including Southern Brazil, Uruguay and Argentina has shown a strong increase in SST over the last 50 years (Bertrand et al., 2018). To support fisheries management in the region, the understanding of climate impacts on fisheries is crucial. However, the Southwestern Atlantic is a data poor region, which challenges regionalized modeling approaches.
To investigate the relationship between sardine, skipjack, nutrients and SST, six pole-and-line vessels have been tracked over a 5 year period together with satellite measurements of SST, chlorophyll, and altimetry, along with oceanographic model data for thermocline, salinity and sub-surface temperature. To extend the time series, SST measurements from 18 virtual stations over the mid-shelf and shelf-break have been assembled for the period 1981–2017 and been correlated with sardine and skipjack catches of Brazil between 1992 and 201712. Sardine and skipjack dynamics show similar trends (Figure 2), suggesting that conditioning factors affecting variability for both might be similar.
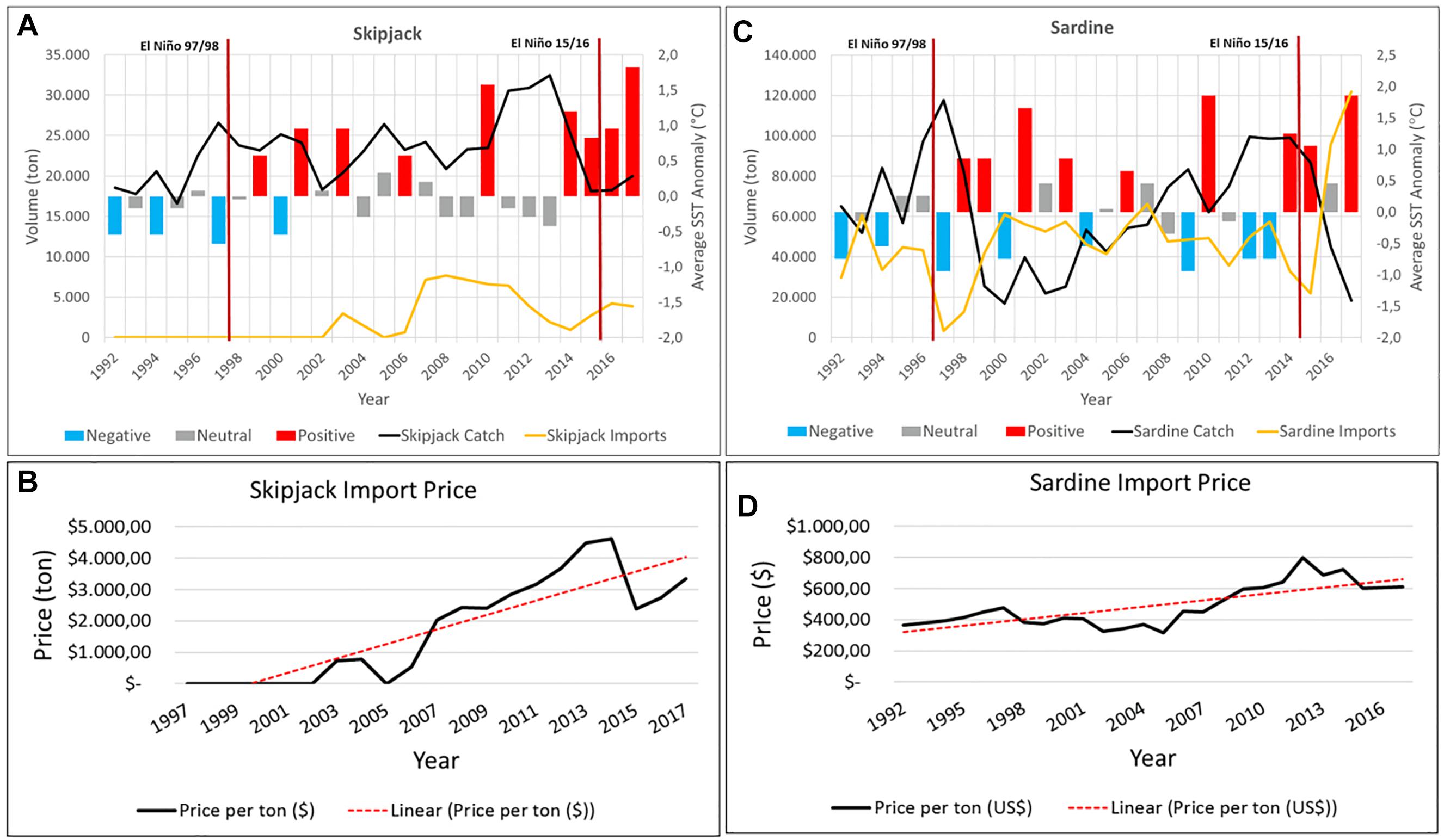
Figure 2. Skipjack (A) and sardine catches (C) from 1992 to 2017 along the south east and south Brazilian coasts associated to SST average annual anomalies from virtual stations over the 50 m and 1000 isobaths. Skipjack (B) and sardine (D) import volumes and prices over the study period.
Both sardine and skipjack catch variability is strongly connected to El Niño and La Niña events and fluctuate under changing environmental conditions (Checkley et al., 2017). Sardine catch along the South-east Brazilian coast varied from 15k to 117k tons between 1992 and 2017. Highest and lowest sardine catch years were 1997 and 2017, respectively, both associated with very strong El Niño events in 1997–1998 and 2015–2016. The 2017 lowest ever sardine catch season was anticipated by the 2015–2016 strong El Niño and by the weak warm 2014–2015 El Niño. Sardine catches started dropping in 2015 to the lowest values in 2017 (Figure 2C). Skipjack catches follow a similar trend in which lower catches were observed in 2015–2016 (Figure 2A). A sequence of positive SST anomalies reduced catches by 85% for sardines and 50% for skipjack. Catches from more recent years suggest that the weak warm 2014–2015 El Niño impact on skipjack catches was exacerbated by the 2015–2016 strong event. These catch fluctuations, and the need to import sardines when catches were low, have had important impacts on the local economies.
A comprehensive monitoring program in the Southwestern Atlantic Ocean, including environmental conditions and status of fish stocks, would support the development of predictive models. These models would improve the management of the resource and economic and social outcomes for the people in the region.
Spanish Sardine Fishery in the Northeastern Venezuelan Upwelling Area
The importance of the Spanish sardine (Sardinella aurita) as a fishery resource in Venezuelan waters has been recognized since the 1950s (Cárdenas, 2003). Since then, total yearly catches had been increasing until settling at an average of 123,773 t/year in the1990s and early 2000s. The Spanish sardine fishery along with other pelagic and demersal fisheries are responsible for characterizing the Venezuela’s eastern shelf as one of the most important fishing grounds in the Caribbean Sea. This area is influenced by coastal seasonal upwelling from November to May, and also by the Orinoco River plume in the rainy season (June–October) (Mendoza, 2015).
Sardine landings, which between the late 1990s and early 2000s represented nearly 40% of the total fisheries catches within Venezuelan territorial waters, have supported the canning industry and all of its associated value chains. Therefore, sardine is highly relevant for the economy of eastern Venezuela’s coastal cities and is an essential and affordable protein source for the population. In the 1970s, the growth of this fishery raised concern about the need for quantitative assessments of the resource. This triggered the first formal evaluations which were derived from primary production, catches and aerial surveys, establishing the catch potential at 200,000 t/year (Ginés, 1972). This initial estimate was followed by intermittent acoustic assessments which reported the following metric tonnes per year: 1,000,000 (Gerlotto and Ginés, 1988), 800,000 (Stroemme and Saetersdal, 1989), 700,000–1,000,000 (Gerlotto, 1993), 800,000–1,300,000 (Cardénas, 1992), 785,600–941,000 (Cárdenas and Achury, 2002), and 81,000–290,000 (Gassman et al., 2012). Despite some technical issues that affected the precision of the 2010s acoustic estimation, this last survey clearly indicated a decrease in the stock from around 900,000 t on average to possibly under 100,000 t.
This biomass reduction was coupled by a downfall of the Sardinella aurita landings between 2005 and 2011 with a minimum of 34.753 t, in contrast to years 1998 and 2004, when the yearly catches were 186,060 t and 200,232, respectively (Figure 3). These exceptionally high landings could have been the result of high effort levels along with an increase in the catchability of the sardines which were concentrated in the much smaller upwelling area characteristic of those years (Rueda-Roa et al., 2017). From 2005, diverging from a characteristic seasonal upwelling cycle, a sequence of reduced upwelling years occurred through 2013, followed by an apparent progressive recovery of sardine landings of 50,000 t in 2014 and 120,000 t in 2016, in parallel with two intense upwelling years in 2014 and 2015 (Figures 3, 4).
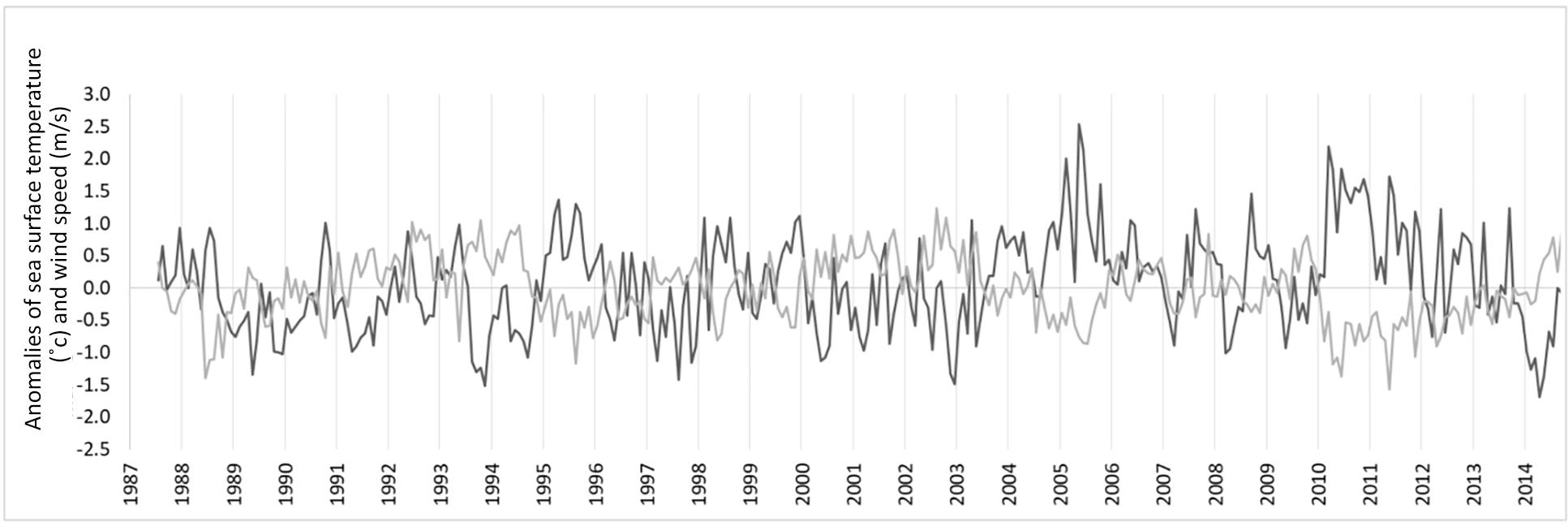
Figure 4. Relationship between the interannual variation of the upwelling intensity (expressed as the SST anomalies; black line) and the wind intensity anomalies (gray line) (data provided by Rueda-Roa, Institute for Marine Remote Sensing, University of South Florida).
One of the most serious problems in managing the Spanish sardine stocks is the weakness of Venezuela’s fisheries statistics, and the lack of ongoing acoustic or any other oceanographic information. Therefore, it is not possible to verify or explain how this biomass recovery of over 150% could occur between 2014 and 2015. Assuming these data are valid and therefore reflecting a stock healing process, the increase in the average SST for 2016 and 2017 (weak upwelling) affecting the sardine habitat conditions (Figure 4), should be a key issue to be considered along with wind anomalies, chlorophyll, and state of the plankton community, to establish fishery regulation policies for this resource. The sardine fishery management in the eastern continental shelf of Venezuela clearly shows the need for regular ocean monitoring and an EBM approach to improve fishing guidelines and benefit the country both ecologically and economically.
Connecting Process Studies With Long-Term Monitoring
Governments have a need for up-to-date process information in order to balance long-term environmental concerns with often short-term societal benefits. When the environmental state changes or is no longer deemed satisfactory by society, process knowledge is needed to assess the impact of potential remedial measures.
Moving forward, information presented in the previous sections should be drawn together into a coherent approach to environmental monitoring and management. We suggest three critical priorities:
First is an initial quantitative understanding of how the ecosystem functions, how it responds to climate change and to future societal demands, and how it is optimally observed. This means building predictive models that can be used to test hypotheses about our current understanding of ecosystem function and using them to assess the value and design of monitoring programs [e.g., through Observation System Simulation Experiments (OSSEs), see e.g., Gasparin et al. (2019) or Integrated Ecosystem Assessments, e.g., Möllmann et al. (2013)]. Future data collection will need to be informed by these models.
Second is full implementation of a data quality management system. Turrell (2018) outlines the necessity of quality control and recognizes the importance of strong governance in this process, though the question of how to objectively balance adaptive change against maintaining time series remains open. It is essential to develop an objective and coherent governance approach that allows all monitoring actors to work together to maximize the benefits of the monitoring efforts as a whole.
Third is a coherent and adaptive way of monitoring and a strong governance group to manage change in observing strategies. Changes in ecosystems and societal needs will challenge existing monitoring programs. However, there is value in stability and the effort that has gone into developing consistent ocean observing systems (SCOR, ICES, IMBER, etc.), and the concomitant scientific consensus cannot be understated. In the absence of a limitless budget, monitoring trade-offs will have to be undertaken and progress should not be limited by focusing too much on developing the perfect monitoring program based on the current conditions. In addition, the value of individual observations, e.g., one-off projects or smaller, time-limited programs, for an overall monitoring system will increase with more harmonization and, if appropriate, standardization of protocols. The UN Environment Statistics group is supporting environment-related statistical standards development and indicator-based environment assessments. Finally, data assimilative models (e.g., Edwards et al., 2015) should be leveraged as a means of incorporating disparate observations into a consistent framework while considering the errors inherent in different measurements, and assessing not only the ocean state but also the monitoring program itself.
Capacity Development to Support Ocean Observations for Fisheries and Ecosystem Studies
Collection of ocean observations must be accompanied by improvements in survey design to ensure gaps are filled and optimal collection occurs. Supplementary observations such as port sampling, on-board sampling, and citizen science initiatives can add to the global knowledge base and can work in a range of situations. These supplemental programs provide an entry point for capacity development too, and add value to global observations, provided consistent sampling protocols can be developed and distributed.
Collectively, analysis and visualization tools need to improve, including training opportunities for users with varying levels of expertise. Without this capacity development, these data will not realize their best value.
We provide examples of several existing programs (not exhaustive) that add value via capacity development for users of ocean data:
• ICES: The ICES13 offers training courses to ensure a high quality of their advisory process. The courses are linked to national institutes and universities and developed and evaluated by an operational training group. Being an international advisory body focused on developing science to support the sustainable use of the marine ecosystem and resources, most of the courses are relevant to fisheries (e.g., stock assessments, modeling for fisheries science and management, geostatistics for fisheries, and genomics in support of fisheries and aquaculture management among others).
• UNEP: The Marine and Coastal Ecosystems Unit in the Division of Environmental Policy Implementation is supporting work on EBM approaches, marine protected areas and ocean governance, marine pollution (litter, nutrients, waste water), and supports the Regional Seas Program and work to protect coral reefs. It specifically supports the Samoa pathway as a means of facilitating achievement of the SDGs (Small Island Developing States).
• OTGA: The Ocean Teacher Global Academy14 provides training through regional centers in several topics. Those relevant to fisheries include courses on ocean observation, data management, coastal and marine spatial planning, marine biodiversity data and HABs. OTGA training has been far reaching, with more than 2500 people trained from 134 IOC member states, more than 4200 registered users, and more than 180 training courses. Regional training centers are currently located in Belgium, Colombia, India, Kenya, Malaysia, Mozambique and Senegal, with courses provided in several languages.
• SCOR: The Scientific Committee for Oceanic Research (SCOR) has supported capacity development activities in oceanography since its foundation in 1957. Support is always targeted to helping scientists from developing countries to engage with SCOR activities, international networks and scientists from developed countries. SCOR supports travel to attend scientific events, visiting scholars and fellows, summer schools, and the establishment of regional graduate networks of oceanography in developing countries15. SCOR working groups also have the responsibility of delivering capacity within their different topics. A current working group with relevant applications on fisheries is WG #149 which studies how biota will respond to a changing ocean by understanding effects of multiple environmental drivers on individual organisms, community and food web responses to ocean change, and the timescales of these biological responses to changes.
• PICES: In a similar way to SCOR but at a regional level, the PICES16 supports training and education of early career scientists (e.g., to attend scientific conferences and training courses, awards) mainly focused on methodologies, monitoring, modeling and data management. In addition, PICES also supports strengthening of infrastructure and network development within its member nations and in developing nations of the Pacific.
• Nansen Program: The R/V Dr. Fridtjof Nansen Program, which later became the EAF-Nansen Project, was initiated in 1975 by the Government of Norway. The goal of the program was to assess fish populations in coastal developing countries to support fisheries management and sustainable use (Bianchi et al., 2016). Capacity development was always at the core of the program, even in the first two phases, with regional scientists and students getting on board the vessel to participate in the cruise’s activities and learn about cruise planning, execution, local fish biodiversity, and use of the data. In this way, the program developed significant capacity and established a broad international network of collaboration. The data is administered by FAO and the fish collections are stored in the local museums and institutions.
• North Pacific acoustic vessels of opportunity: This program demonstrates that acoustic data collected from commercial fishing vessels can be collected at a very high resolution (seconds and meters) over an entire fishing season (Barbeaux et al., 2013). This provides an opportunity to investigate fish distributions and behaviors over a range of spatial and temporal resolutions in a broad range of research applications worldwide (Karp, 2007).
• IMOS and the NESP Marine Biodiversity Hub of Australia: The Integrated Marine Observing System of Australia is currently developing a platform for online teaching with learning modules aimed to explain how to use and interpret the observational data collected by IMOS. One of these modules will be focused on marine productivity using IMOS datasets from continuous plankton recorder on ships of opportunity, fluorescence and satellite ocean color data. The major contribution of IMOS to capacity development is however a collection of best practices, some of which are available on the website17 or published (e.g., Brodie et al., 2018; Hoenner et al., 2018 on acoustic telemetry). The NESP Marine Biodiversity Hub has also contributed to the Oceans Best Practices Platform with manuals. One of these particularly relevant for fisheries is the Marine Sampling Field Manual for Pelagic Stereo BRUVS (Baited Remote Underwater Videos) (Bouchet et al., 2018) which guides on digital video imagery techniques as a means to survey pelagic macro-organisms.
• The Nippon Foundation (NF) and the Partnership for Observation of the Global Oceans (POGO) support a 10-month training program in multidisciplinary observational oceanography for ten early career scholars on an annual basis since 2008. After the training, these students continue to network through the NF-POGO Alumni Network for Ocean (NANO). NANO was established as a means to keep track of the professional development and maximize the benefits of this training investment18.
• Capacity development should use the “Ocean Best Practices” platform19, a growing repository of accepted ocean best practice methods (Pearlman et al., 2017).
Other capacity development initiatives along with their benefits, challenges and recommendations from lessons learnt are summarized in Miloslavich et al. (2018b).
Without capacity development, data will not realize their best value. The programs described here demonstrate that there has been a huge amount of investment in the area of capacity development for ocean observations globally. Yet many countries still lack the budget and have not been able to develop proper capacity. With the exception of the POGO-NANO program and the IOC’s OTGA, there is often no follow-up on how the capacity is then passed on to the countries from which the participants came from. We suggest an increase in formal follow up on these programs as an improvement going forward.
Our Vision for Observing in Support of an Ecosystem Based Fisheries Management
Our vision for future observation for EBFM requires a framework to allow the identification and prioritization of objectives, and observational platforms that are dynamic (e.g., protection of essential habitats, fishing quotas), proactive (anticipation of fishing operation, climate change adaptation), and integrated (balance between the socio-economic benefits of all marine sectors considering dimension of the cultural heritage).
There is mutual benefit to link the GOOS and ocean observing for fisheries advice. A possible communication loop needs to include the elements of defining objectives and the relevant phenomena to be observed, identification of means of observation, the collection of data, the integration and use of the data, and most importantly an evaluation of all steps to refine and give feedback (Figure 5).
Recommendation Summary
• Link ocean observing in the context of GOOS, fisheries observations, and capacity development in a comprehensive ocean observing framework that allows all monitoring actors to work together, maximizing benefits of efforts;
• Coordinate the collection of multidisciplinary environmental information from all sources by linking all relevant organizations and actors, including industry and communities;
• Develop and implement dialogue between data producers, data users, producers of intermediate products and end-users to continually reassess the monitoring objectives in light of policy and user needs;
• Adopt a comprehensive data management framework, including the consistent and sustained use of specific data repositories for specific types of information, implementing linkages of records between these repositories, promoting the use of common data and data exchange formats for interoperability, easy-machine-to-machine exchange and use of open source tools;
• Implement systematic data quality assurance and quality control processes for EOVs;
• Improve and facilitate access of data and metadata for science as well as reliable and well-tailored data products to the public, industry, resource managers and policy and decision makers;
• Improve modeling efforts for dynamical forecasting, including species distributions, and ecosystem models to support management of human activities and conservation efforts;
• Develop and implement coherent capacity development and training programs, including analysis and visualization for users of varying levels of expertise through a joint ocean observing system governance framework.
Author Contributions
JS and SBo developed the idea and concept, and wrote the final manuscript. All authors have contributed to the concept and the writing.
Funding
JS received funding from the Cluster of Excellence Future Ocean and Kiel Marine Science. JK and SS have received funding from the European Union’s Horizon 2020 Research and Innovation Program under grant agreement nos. 633211 (AtlantOS) and 730960 (SeaDataCloud). CS received support from Fundação para a Ciência e Tecnologia (FCT) through the strategic project UID/MAR/04292/2019 granted to MARE and a doctoral grant (SFRH/BD/117890/2016). LM, JA, and PZ were partly funded by the Brazilian Biodiversity Fund (FUNBIO-GEF) and ACTEMSA-Leal Santos Ltda. SK was funded by the UK Department for Environment, Food and Rural Affairs (Marine Advisory Schedule MA016A). MS received support from a Gordon and Betty Moore Foundation award (#3790) and an NSF award (OCE#1829831). MJ, SB, and DT received funding from the NOAA Climate Program Office (grants NA17OAR4310268 and NA17OAR4310108) and the NOAA/NMFS Office of Science and Technology. JM was supported by the Pró-Reitoria de Pesquisa e Pós-graduação, Universidade Federal do Pará (PROPESP/UFPA grant no. 05/2018) and the Conselho Nacional de Desenvolvimento Científico e Tecnológico (CNPq grant no. 438075/2018-8). AF was funded by the Ocean Acidification flagship within the FRAM-High North Centre for Climate and the Environment, Norway. JB received support from the U.S. National Science Foundation and National Oceanic and Atmospheric Administration.
Conflict of Interest Statement
HA was employed by AZTI Tecnalia.
The remaining authors declare that the research was conducted in the absence of any commercial or financial relationships that could be construed as a potential conflict of interest.
Acknowledgments
We want to thank all participants of the workshop at the AGU Ocean Sciences meeting in Portland, OR and the Effects of Climate Change on the Worlds Ocean in Washington, DC in 2018. We are also grateful to the two reviewers and the editor, each of whom gave valuable comments and input. LM, JA, and PZ would like to thank A. Brum, J. Coletto, and M. Pinho (FURGBrazil) and A. Ávila and G. Camboim (UFRGS-Brazil) for their contribution. Some recommendations from this work resulted from discussions within the Global Ocean Observing System program and PEGASuS 2: Ocean Sustainability, a partnership between Future Earth, the National Center for Ecological Analysis and Synthesis (NCEAS), and Global Biodiversity Center at Colorado State University. We particularly wish to thank the larger network of the International Council for the Exploration of the Sea (ICES) for inspiration and contribution of ideas.
Footnotes
- ^ https://www.bco-dmo.org/
- ^ https://www.ncei.noaa.gov
- ^ http://data.ifremer.fr/
- ^ http://www.dfo-mpo.gc.ca/science/data-donnees/biochem/index-eng.html
- ^ https://obis.org/
- ^ www.st.nmfs.noaa.gov/plankton/about/index.html
- ^ http://seabirdtracking.org/
- ^ https://oceanobservatories.org/
- ^ http://marine.copernicus.eu//
- ^ www.emodnet.eu/
- ^ http://www.ices.dk/Pages/default.aspx
- ^ The data has been collected by the Departamento de Oceanografia, Universidade Federal do Rio Grande, Rio Grande, Brazil. Requests to access the dataset should be directed to bGF1cm8uYXNwbUBnbWFpbC5jb20=.
- ^ http://www.ices.dk
- ^ https://classroom.oceanteacher.org/
- ^ http://www.scor-int.org/SCOR_CB_Portal.htm
- ^ http://meetings.pices.int/about
- ^ http://imos.org.au/
- ^ https://nf-pogo-alumni.org/about/
- ^ www.oceanbestpractices.net/
References
Abrahms, B., Scales, K. L., Hazen, E. L., Bograd, S. J., Schick, R. S., Robinson, P., et al. (2018). Physical ocean complexity facilitates energy gain in a top predator. Proc. R. Soc. B 285:20181101. doi: 10.1098/rspb.2018.1101
Badjeck, M. C., Allison, E. H., Halls, A. S., and Dulvy, N. K. (2010). Impacts of climate variability and change on fishery-based livelihoods. Mar. policy 34, 375–383. doi: 10.1016/j.marpol.2009.08.007
Barale, V., Gower, J. F. R., and Alberotanza, L. (2010). Oceanography from Space: Revisited. Berlin: Springer Science & Business Media.
Barbeaux, S., Aydin, K., Holsman, K., Palsson, W., Shotwell, K., Yang, Q., et al. (2017). Chapter 2: Assessment of the Pacific Cod Stock in the Gulf of Alaska. Anchorage, AK: North Pacific Fishery Management Council.
Barbeaux, S. J., Horne, J. K., and Dorn, M. W. (2013). Characterizing walleye pollock (Theragra chalcogramma) winter distribution from opportunistic acoustic data. ICES J. Mar. Sci. 70, 1162–1173. doi: 10.1093/icesjms/fst052
Barnes, B. B., Cannizzaro, J. P., English, D. C., and Hu, C. (2019). Validation of VIIRS and MODIS reflectance data in coastal and oceanic waters: an assessment of methods. Remote Sens. Environ. 220, 110–123. doi: 10.1016/J.RSE.2018.10.034
Benoit-Bird, K. J., Welch, T. P., Waluk, C. M., Barth, J. A., Wangen, I., McGill, P., et al. (2018). An autonomous platform for understanding ocean ecosystems: equipping an underwater glider with a new quantitative echosounder. Limnol. Oceanogr. Methods 16, 734–749. doi: 10.1002/lom3.10278
Bertrand, A., Vogler, R., and Defeo, O. (2018). “Climate change impacts, vulnerabilities and adaptations: Southwest Atlantic and Southeast Pacific marine fisheries,” in FAO - Food and Agriculture Organization of the United Nations. 2018, eds M. Barange, T. Bahri, M. C. M. Beveridge, K. L. Cochrane, S. Funge-Smith, and F. Poulaine (Rome: FAO).
Bianchi, G., Bjordal, Å, Koranteng, K. A., Tandstad, M., Sambe, B., and Stromme, T. (2016). Collaboration between the nansen programme and the large marine ecosystem programmes. Environ. Deve. 17, 340–348. doi: 10.1016/j.envdev.2015.11.003
Blanco Gonzalez, E., Knutsen, H., and Jorde, P. E. (2016). Habitat discontinuities separate genetically divergent populations of a rocky shore marine fish. PLoS One 11:e0163052. doi: 10.1371/journal.pone.0163052
Block, B. A., Jonsen, I. D., Jorgensen, S. J., Winship, A. J., Shaffer, S. A., Bograd, S. J., et al. (2011). Tracking apex marine predator movements in a dynamic ocean. Nature 475, 86–90. doi: 10.1038/nature10082
Bograd, S., Block, B., Costa, D., and Godley, B. (2010). Biologging technologies: new tools for conservation. Introduction. Endanger. Species Res. 10, 1–7. doi: 10.3354/esr00269
Bond, N. A., Cronin, M. F., Freeland, H., and Mantua, N. (2015). Causes and impacts of the 2014 warm anomaly in the NE Pacific. Geophys. Res. Lett. 42, 3414–3420. doi: 10.1002/2015GL063306
Boss, E., Waite, A., Muller-Karger, F., Yamazaki, H., Wanninkhof, R., Uitz, J., et al. (2018). Beyond chlorophyll fluorescence: the time is right to expand biological measurements in ocean observing programs. Limnol. Oceanogr. Bull. 27, 89–90. doi: 10.1002/lob.10243
Bouchet, P., Meeuwig, J., Huveneers, C., Langlois, T., Letessier, T., Lowry, M., et al. (2018). “Marine Sampling Field Manual for Pelagic Stereo BRUVS (Baited Remote Underwater Videos),” in Field Manuals for Marine Sampling to Monitor Australian Waters, Version 1, eds R. Przeslawski, and S. Foster (Canberra, ACT: NESP Marine Biodiversity Hub), 105–132. doi: 10.11636/9781925297669
Boyer, T. P., Baranova, O. K., Coleman, C., García, H. E., Grodsky, A., Locarnini, R. A., et al. (2018). World Ocean Database 2018. Silver Spring, MD: NOAA Printing Officce, 208.
Brodie, S., Hobday, A. J., Smith, J. A., Spillman, C. M., Hartog, J. R., Everett, J. D., et al. (2017). Seasonal forecasting of dolphinfish distribution in eastern Australia to aid recreational fishers and managers. Deep Sea Res. Part 2 Top. Stud. Oceanogr. 140, 222–229. doi: 10.1016/j.dsr2.2017.03.004
Brodie, S., Lédée, E. J. I., Heupel, M. R., Babcock, R. C., Campbell, H. A., Gledhill, D. C., et al. (2018). Continental-scale animal tracking reveals functional movement classes across marine taxa. Sci. Rep. 8:3717. doi: 10.1038/s41598-018-21988-5
Burke, B. J., Peterson, W. T., Beckman, B. R., Morgan, C., Daly, E. A., and Litz, M. (2013). Multivariate models of adult Pacific salmon returns. PLoS One 8:e54134. doi: 10.1371/journal.pone.0054134
Cardénas, J. (1992). Distribución y abundancia de la biomasa íctica, con énfasi especial en la sardina, determinada por medios hidroacústicos. Margarita: Doc. Int. EDIMAR, Fund. La Salle Cien. Nat, 17.
Cárdenas, J. (2003). “Distribución y cuantificación de la biomasa íctica del mar nororiental Venezolano, con énfasis especial en la sardina, determinadas por medios hidroacústicos,” in La sardina (Sardinella aurita), su medio ambiente y explotación en el oriente de Venezuela, eds P. Freón and J. Mendoza (París: IRD Editions), 401–423.
Cárdenas, J., and Achury, A. (2002). Acústica pesquera sobre los recursos marinos del nororiente de Venezuela: evaluación y seguimiento espacio-temporal del stock de sardina, Sardinella aurita Memoria de la Sociedad de Ciencias Naturales La Salle. Available at: http://www.fundacionlasalle.org.ve/userfiles/8-Memoria%20154%2039-54.pdf
Checkley, D. M., Asch, R. G., and Rykaczewski, R. R. (2017). Climate, anchovy, and sardine. Ann. Rev. Mar. Sci. 9, 469–493. doi: 10.1146/annurev-marine-122414-033819
Chierici, M., Signorini, S., Mattsdotter-Björk, M., Fransson, A., and Olsen, A. (2012). Surface water fCO2 algorithms for the high-latitude Pacific sector of the Southern Ocean. Remote Sens. Environ. 119, 184–196. doi: 10.1016/j.rse.2011.12.020
Crossin, G., Cooke, S., Goldbogen, J., and Phillips, R. (2014). Tracking fitness in marine vertebrates: current knowledge and opportunities for future research. Mar. Ecol. Prog. Ser. 496, 1–17. doi: 10.3354/meps10691
Di Lorenzo, E., and Mantua, N. (2016). Multi-year persistence of the 2014/15 North Pacific marine heatwave. Nat. Clim. Chang. 6, 1042–1047. doi: 10.1038/nclimate3082
Dierssen, H. M., Chlus, A., and Russell, B. (2015). Hyperspectral discrimination of floating mats of seagrass wrack and the macroalgae Sargassum in coastal waters of Greater Florida Bay using airborne remote sensing. Remote Sens. Environ. 167, 247–258. doi: 10.1016/J.RSE.2015.01.027
Djurhuus, A., Pitz, K., Sawaya, N. A., Rojas-Márquez, J., Michaud, B., Montes, E. W., et al. (2018). Evaluation of marine zooplankton community structure through environmental DNA metabarcoding. Limnol. Oceanogr. Methods. 16, 209–221. doi: 10.1002/lom3.10237
Dolan, T. E., Patrick, W. S., and Link, J. S. (2016). Delineating the continuum of marine ecosystem-based management: a US fisheries reference point perspective. ICES J. Mar. Sci. 73, 1042–1050. doi: 10.1093/icesjms/fsv242
Dragon, A.-C., Senina, I., Titaud, O., Calmettes, B., Conchon, A., Arrizabalaga, H., et al. (2015). An ecosystem-driven model for spatial dynamics and stock assessment of North Atlantic albacore. Can. J. Fish. Aquat. Sci. 72, 864–878. doi: 10.1139/cjfas-2014-0338
Druon, J.-N., Fiorentino, F., Murenu, M., Knittweis, L., Colloca, F., Osio, C., et al. (2015). Modelling of European hake nurseries in the Mediterranean Sea: an ecological niche approach. Prog. Oceanogr. 130, 188–204. doi: 10.1016/j.pocean.2014.11.005
Druon, J.-N., Fromentin, J. M., Hanke, A., Arrizabalaga, H., Damalas, D., Tičina, V., et al. (2016). Habitat suitability of the Atlantic bluefin tuna by size class: an ecological niche approach. Prog. Oceanogr. 142, 30–46. doi: 10.1016/j.pocean.2016.01.002
Druon, J.-N., Helaouet, P., Beaugrand, G., Fromentin, J.-M., Palialexis, A., and Hoepffner, N. (2019). Satellite-based indicator of zooplankton distribution for global monitoring. Sci. Rep. 9:4732. doi: 10.1038/s41598-019-41212-2
Druon, J.-N., Loyer, S., and Gohin, F. (2005). Scaling of coastal phytoplankton features by optical remote sensors: comparison with a regional ecosystem model. Int. J. Remote Sens. 26, 4421–4444. doi: 10.1080/01431160500227847
Edwards, C. A., Moore, A. M., Hoteit, I., and Cornuelle, B. D. (2015). Regional ocean data assimilation. Annu. Rev. Mar. Sci. 7, 21–42. doi: 10.1146/annurev-marine-010814-015821
Eveson, J. P., Hobday, A. J., Hartog, J. R., Spillman, C. M., and Rough, K. M. (2015). Seasonal forecasting of tuna habitat in the Great Australian Bight. Fish. Res. 170, 39–49. doi: 10.1016/j.fishres.2015.05.008
Fissel, B., Dalton, M., Felthoven, R., Garber-Yonts, B., Haynie, A., Kasperski, S., et al. (2016). Stock Assessment and Fishery Evaluation Report for the Groundfish Fisheries of the Gulf of Alaska and Bering Sea/Aleutian Island Area: Economic Status of the Groundfish Fisheries Off Alaska, 2015. Anchorage, AK: North Pacific Fishery Management Council.
Gasparin, F., Guinehut, S., Mao, C., Mirouze, I., Remy, E., King, R. R., et al. (2019). Requirements for an integrated in situ Atlantic Ocean observing system from coordinated observing system simulation experiments. Front. Mar. Sci. 6:83. doi: 10.3389/fmars.2019.00083
Gassman, J. P. D., López, A., Achury, A., Barrios, J., Cárdenas, A., Díaz, H., et al. (2012). “Seguimiento de la pesca de la sardina (Sardinella aurita) como recurso de interés estratégico: cuantificación de la biomasa mediante prospección acústica (Proyecto INPA 045),” in Proceedings of the III Congreso Venezolano de Diversidad Biológica, Libro de resúmenes,. May 22, 2012, San Carlos, 148.
Gerlotto, F. (1993). Methodologie d’Observation et d’Évaluation par Hydroacoustique des Stocks Tropicaux de Poissons Pélagiques Cotiers: Impact du Comportement et de la Distribution Spatiale. Brest: Université de Bretagne Occidentale.
Gerlotto, F. Y., Ginés Hno (1988). Ecointegración y pesca sardinera: diez años de investigación en EDIMAR. Mem. Soc. Cienc. Natur. Salle Sup. 3:48.
Ginés, H. (1972). Cart∗a Pesquera de Venezuela. 1. Áreas del Nororiente y Guayana Monografía N ° 16. Caracas: Fundación La Salle de Ciencias Naturales, 328.
Gollnow, F., and Lakes, T. (2014). Policy change, land use, and agriculture: the case of soy production and cattle ranching in Brazil, 2001-2012. Appl. Geogr. 55, 203–211. doi: 10.1016/j.apgeog.2014.09.003
Gower, J. F. R., Young, E., and King, S. A. (2013). Satellite images suggest a new Sargassum source region in 2011. Remote Sens. Lett. 4, 764–773. doi: 10.1080/2150704x.2013.796433
Hall, J., Harrison, D. E., and Stammer, D. (eds). (2010). Proceedings of OceanObs’09: Sustained Ocean Observations and Information for Society. Venice: ESA Publication WPP-306, doi: 10.5270/OceanObs09
Hazen, E. L., Palacios, D. M., Forney, K. A., Howell, E. A., Becker, E., Hoover, A. L., et al. (2016). WhaleWatch: a dynamic management tool for predicting blue whale density in the California current. J. Appl. Ecol. 54, 1415–1428. doi: 10.1111/1365-2664.12820
Hazen, E. L., Scales, K. L., Maxwell, S. M., Briscoe, D. K., Welch, H., Bograd, S. J., et al. (2018). A dynamic ocean management tool to reduce bycatch and support sustainable fisheries. Sci. Adv. 4:eaar3001. doi: 10.1126/sciadv.aar3001
Hilborn, R., and Walters, C. J. (2013). Quantitative Fisheries Stock Assessment: Choice, Dynamics and Uncertainty. Berlin: Springer.
Hinds, C., Oxenford, H., Cumberbatch, J., Fardin, F., Doyle, E., and Cashman, A. (2016). Golden Tides: Management Best Practices for Influxes of Sargassum in the Caribbean with a Focus on Clean-Up. Wanstead: University of the West Indies at Cave Hill, 17.
Hobday, A. J., and Hartog, J. R. (2014). Derived ocean features for dynamic ocean management. Oceanography 27, 134–145. doi: 10.5670/oceanog.2014.92
Hobday, A. J., Spillman, C. M., Eveson, J. P., Hartog, J. R., Zhang, X., and Brodie, S. (2018). A framework for combining seasonal forecasts and climate projections to aid risk management for fisheries and aquaculture. Front. Mar. Sci. 5:137. doi: 10.3389/fmars.2018.00137
Hobday, A. J., Spillman, C. M., Hartog, J. R., and Eveson, J. P. (2016). Seasonal forecasting for decision support in marine fisheries and aquaculture. Fish. Oceanogr. 25, 45–56. doi: 10.1111/fog.12083
Hoenner, X., Huveneers, C., Steckenreuter, A., Simpfendorfer, C., Tattersall, K., Jaine, F., et al. (2018). Australia’s continental-scale acoustic tracking database and its automated quality control process. Sci. Data 5:170206.
Hollowed, A. B., Barange, M., Beamish, R. J., Brander, K., Cochrane, K., Drinkwater, K., et al. (2013). Projected impacts of climate change on marine fish and fisheries. ICES J. Mar. Sci. 70, 1023–1037.
Howell, E., Kobayashi, D., Parker, D., Balazs, G., and Polovina, J. (2008). TurtleWatch: a tool to aid in the bycatch reduction of loggerhead turtles Caretta caretta in the Hawaii-based pelagic longline fishery. Endanger. Species Res. 5, 267–278. doi: 10.3354/esr00096
Hu, C., Murch, B., Barnes, B. B., Wang, M., Maréchal, J.-P., Franks, J., et al. (2016). Sargassum Watch Warns of Incoming Seaweed. Washington, DC: EOS, 97. doi: 10.1029/2016EO058355
Jayne, S. R., Roemmich, D., Zilberman, N., Riser, S. C., Johnson, K. S., Johnson, G. C., et al. (2017). The argo program present and future. Oceanography 30, 18–28. doi: 10.5670/oceanog.2017.213
Johnson, D. R., Ko, D. S., Franks, J. S., Moreno, P., and Sanchez-Rubio, G. (2013). “The Sargassum Invasion of the Eastern Caribbean and Dynamics of the Equatorial North Atlantic,” in Proceedings of the 65th Gulf and Caribbean Fisheries Institute, Santa Marta, 102–103.
Kaplan, I. C., and Marshall, K. N. (2016). A guinea pig’s tale: learning to review end-to-end marine ecosystem models for management applications. ICES J. Mar. Sci. 73, 1715–1724. doi: 10.1093/icesjms/fsw047
Kaplan, I. C., Williams, G. D., Bond, N. A., Hermann, A. J., and Siedlecki, S. A. (2016). Cloudy with a chance of sardines: forecasting sardine distributions using regional climate models. Fish. Oceanogr. 25, 15–27. doi: 10.1111/fog.12131
Karp, B. (2007). Collection of Acoustic Data from Fishing Vessels. ICES Cooperative Research Report No 287. Copenhagen: ICES, 83.
Keller, A., Simon, V., Chan, F., Wakefield, W. W., Clarke, M. E., Kamikawa, D., et al. (2010). Demersal fish and invertebrate biomass in relation to an offshore hypoxic zone along the U.S. West Coast. Fish. Oceanogr. 19, 76–87. doi: 10.1111/j.1365-2419.2009.00529.x
Kwiatkowska, E. J., Ruddick, K., Ramon, D., Vanhellemont, Q., Brockmann, C., Lebreton, C., et al. (2016). Ocean colour opportunities from meteosat second and third generation geostationary platforms. Ocean Sci. 12, 703–713. doi: 10.5194/os-12-703-2016
Lapointe, B. E. (1995). A comparison of nutrient-limited productivity in Sargassum natans from neritic vs. oceanic waters of the western North Atlantic Ocean. Limnol. Oceanogr. 40, 625–633. doi: 10.4319/lo.1995.40.3.0625
Lauvset, S. K., Key, R. M., Olsen, A., van Heuven, S., Velo, A., Lin, X., et al. (2016). A new global interior ocean mapped climatology: the 1°x1° GLODAP version 2. Earth Syst. Sci. Data 8, 325–340. doi: 10.5194/essd-8-325-2016
Lembke, C., Silverman, A., Mann, D., Gray, J., Taylor, C., and Hughes, E. (2018). “Utilizing Gliders and Acoustics to Identify Fish Habitat Hotspots. A Case Study 2018,” in Proceedings of the OCEANS 2018 Marine Technology Society/IEEE Oceanic Engineering Society Conference, Charleston, SC, 1–8. doi: 10.1109/OCEANS.2018.8604916
Lewison, R., Hobday, A. J., Maxwell, S., Hazen, E., Hartog, J. R., Dunn, D. C., et al. (2015). Dynamic ocean management: identifying the critical ingredients of dynamic approaches to ocean resource management. Bioscience 65, 486–498. doi: 10.1093/biosci/biv018
Lewison, R. L., Johnson, A. F., and Verutes, G. M. (2018). Embracing complexity and complexity-awareness in marine megafauna conservation and research. Front. Mar. Sci. 5:207. doi: 10.3389/fmars.2018.00207
Lindstrom, E., Gunn, J., Fischer, A., McCurdy, A., Glover, L. K., and Task Team members. (2012). A Framework for Ocean Observing. By the Task Team for an Integrated Framework for Sustained Ocean Observing, UNESCO 2012, IOC/INF-1284. Paris: UNESCO, doi: 10.5270/OceanObs09-FOO
Lopez, J., Moreno, G., Sancristobal, I., and Murua, J. (2014). Evolution and current state of the technology of echo-sounder buoys used by Spanish tropical tuna purse seiners in the Atlantic. Indian and Pacific Oceans. Fish. Res. 155, 127–137. doi: 10.1016/J.FISHRES.2014.02.033
Macaya, E. C., López, B., Tala, F., Tellier, F., and Thiel, M. (2016). “Float and raft: role of buoyant seaweeds in the pylogeography and genetic structure of non-buoyant associated flora,” in Seaweed Phylogeography, eds Z.-M. Hu and C. Fraser (Berlin: Springer), doi: 10.1007/978-94-017-7534-2_4
Maxwell, S. M., Hazen, E. L., Lewison, R. L., Dunn, D. C., Bailey, H., Bograd, S. J., et al. (2015). Dynamic ocean management: defining and conceptualizing real-time management of the ocean. Mar. Policy 58, 42–50. doi: 10.1016/j.marpol.2015.03.014
Mendoza, J. (2015). “Rise and fall of Venezuelan industrial and artisanal marine fisheries: 1950-2010,” in Working Paper Series, ed. J. Mendoza (Vancouver, BC: The University of British Columbia Fisheries Centre).
Mills, K. E., Pershing, A. J., and Hernández, C. M. (2017). Forecasting the seasonal timing of maine’s lobster fishery. Front. Mar. Sci. 4:337. doi: 10.3389/fmars.2017.00337
Miloslavich, P., Bax, N. J., Simmons, S. E., Klein, E., Appeltans, W., Aburto-Oropeza, O., et al. (2018a). Essential ocean variables for global sustained observations of biodiversity and ecosystem changes. Glob. Change Biol. 24, 2416–2433. doi: 10.1111/gcb.14108
Miloslavich, P., Seeyave, S., Ali, E., Bax, N., Delgado, C., Evers-King, H., et al. (2018b). Challenges for global ocean observation: the need for increased human capacity. J. Operat. Oceanogr. Available at: https://www.tandfonline.com/doi/pdf/10.1080/1755876X.2018.1526463?needAccess=true
Möllmann, C., Lindegren, M., Blenckner, T., Bergstrom, L., Casini, M., Diekmann, R., et al. (2013). Implementing ecosystem-based fisheries management: from single-species to integrated ecosystem assessment and advice for Baltic Sea fish stocks. ICES J. Mar. Sci. 71, 1187–1197. doi: 10.1093/icesjms/fst123
Moreno, G., Dagorn, L., Capello, M., Lopez, J., Filmalter, J., Forget, F., et al. (2016). Fish aggregating devices (FADs) as scientific platforms. Fish. Res. 178, 122–129. doi: 10.1016/J.FISHRES.2015.09.021
Muller-Karger, F., Roffer, M., Walker, N., Oliver, M., Schofield, O., Abbott, M., et al. (2013). Satellite remote sensing in support of an integrated ocean observing system. IEEE Geosci. Remote Sens. Mag. 1, 8–18. doi: 10.1109/MGRS.2013.2289656
Muller-Karger, F. E., Hestir, E., Ade, C., Turpie, K., Roberts, D. A., Siegel, D., et al. (2018a). Satellite sensor requirements for monitoring essential biodiversity variables of coastal ecosystems. Ecol. Appl. 28, 749–760. doi: 10.1002/eap.1682
Muller-Karger, F. E., Miloslavich, P., Bax, N. J., Simmons, S., Costello, M. J., Sousa Pinto, I., et al. (2018b). Advancing marine biological observations and data requirements of the complementary essential ocean variables (EOVs) and essential biodiversity variables (EBVs) frameworks. Front. Mar. Sci. 5:211. doi: 10.3389/fmars.2018.00211
Patrick, W. S., and Link, J. S. (2015). Myths that continue to impede progress in ecosystem-based fisheries management. Fisheries 40, 155–160. doi: 10.1080/03632415.2015.1024308
Payne, M. R., Hobday, A. J., MacKenzie, B. R., Tommasi, D., Dempsey, D. P., Fässler, S. M. M., et al. (2017). Lessons from the first generation of marine ecological forecast products. Front. Mar. Sci. 4:289. doi: 10.3389/fmars.2017.00289
Pearlman, J., Bushnell, M., Coppola, L., Karstensen, J., Buttigieg, P. L., Pearlman, F., et al. (2019). Evolving and sustaining ocean best practices and standards for the next decade. Front. Mar. Sci. 6:277. doi: 10.3389/fmars.2019.00277
Pearlman, J. S., Buttigieg, P. L., Simpson, P., Mas, C. M., Heslop, E., and Hermes, J. (2017). Accessing Existing and Emerging Best Practices for Ocean Observation a New Approach for End-to-End Management of Best Practices. Piscataway, NJ: IEEE, 1–7.
Pecoraro, C., Babbucci, M., Franch, R., Rico, C., Papetti, C., Chassot, E., et al. (2018). The population genomics of Yellowfin tuna (Thunnus albacares) at global geographic scale challenges current stock delineation. Sci. Rep. 8:13890. doi: 10.1038/s41598-018-32331-3
Rodriguez-Ezpeleta, N., Álvarez, P., and Irigoien, X. (2017). Genetic diversity and connectivity in Maurolicus muelleri in the bay of biscay inferred from thousands of SNP markers. Front. Genet. 8:195. doi: 10.3389/fgene.2017.00195
Rooker, J., Arrizabalaga, H., Fraile, I., Secor, D., Dettman, D., Abid, N., et al. (2014). Crossing the line: migratory and homing behaviors of Atlantic bluefin tuna. Mar. Ecol. Prog. Ser. 504, 265–276. doi: 10.3354/meps10781
Rueda-Roa, D., Mendoza, J., Muller-Karger, F., Cárdenas, J. J., Achury, A., and Astor, Y. (2017). Spatial variability of Spanish sardine (Sardinella aurita) abundance as related to the upwelling cycle of the southeastern Caribbean Sea. PLoS One 12:e0179984. doi: 10.1371/journal.pone.0179984
Scales, K. L., Hazen, E. L., Jacox, M. G., Castruciio, F., Maxwell, S. M., Lewison, R. L., et al. (2018). Fisheries bycatch risk to marine megafauna is intensified in Lagrangian coherent structures. Proc. Natl. Acad. Sci. U.S.A. 115, 7362–7367. doi: 10.1073/pnas.1801270115
Scott, G. P., and Lopez, J. (2014). The Use of FADs in Tuna Fisheries. Available at: http://www.europarl.europa.eu/RegData/etudes/note/join/2014/514002/IPOL-PECH_NT(2014)514002_EN.pdf (accessed January 2014).
Selkoe, K., D’Aloia, C., Crandall, E., Iacchei, M., Liggins, L., Puritz, J., et al. (2016). A decade of seascape genetics: contributions to basic and applied marine connectivity. Mar. Ecol. Prog. Ser. 554, 1–19. doi: 10.3354/meps11792
Sissini, M. N., Széchy, M. T. M., Barreto, B. B., Gower, J., Liu, G., Lucena, M. B., et al. (2017). The floating Sargasso Sea in South Atlantic Ocean – likely scenarios and management priorities. Phycologia 56, 321–328.
Smetacek, V., and Zingone, A. (2013). Green and golden seaweed tides on the rise. Nature 504, 84–88. doi: 10.1038/nature12860
Song, H., Edwards, C. A., Moore, A. M., and Fiechter, J. (2016). Data assimilation in a coupled physical-biogeochemical model of the California current system using an incremental lognormal 4-dimensional variational approach: Part 3—Assimilation in a realistic context using satellite and in situ observations. Ocean Model. 106, 159–172. doi: 10.1016/j.ocemod.2016.06.005
Spillman, C. M., and Hobday, A. J. (2014). Dynamical seasonal ocean forecasts to aid salmon farm management in a climate hotspot. Clim. Risk Manag. 1, 25–38. doi: 10.1016/j.crm.2013.12.001
Stroemme, T., and Saetersdal, G. (1989). Surveys of the Fish Resources in the Shelf Areas between Suriname and Colombia 1988. Reports on surveys with RV ‘Dr. F. Nansen’, Vol. 1. Bergen: Institute of Marine Research, 142.
Stumpf, R. P., Tomlinson, M. C., Calkins, J. A., Kirkpatrick, B., Fisher, K., Nierenberg, K., et al. (2009). Skill assessment for an operational algal bloom forecast system. J. Mar. Syst. 76, 151–161. doi: 10.1016/j.jmarsys.2008.05.016
Széchy, M. T. M., Guedes, P. M., Baeta-Neves, M. H., and Oliveira, E. N. (2012). Verification of Sargassum natans (Linnaeus) Gaillon (Heterokontophyta: Phaeophyceae) from the Sargasso Sea off the coast of Brazil, western Atlantic Ocean. Check List 8, 638–641.
Taylor, G. T., Muller-Karger, F. E., Thunell, R. C., Scranton, M. I., Astor, Y., Varela, R., et al. (2012). Ecosystem responses in the southern Caribbean Sea to global climate change. Proc. Natl. Acad. Sci.U.S.A. 109, 19315–19320. doi: 10.1073/pnas.1207514109
Tommasi, D., Stock, C. A., Hobday, A. J., Methot, R., Kaplan, I. C., Eveson, J. P., et al. (2017). Managing living marine resources in a dynamic environment: the role of seasonal to decadal climate forecasts. Prog. Oceanogr. 152, 15–49.
Treasure, A., Roquet, F., Ansorge, I., Bester, M., Boehme, L., Bornemann, H., et al. (2017). Marine mammals exploring the oceans pole to pole: a review of the MEOP consortium. Oceanography 30, 132–138. doi: 10.5670/oceanog.2017.234
Turrell, W. R. (2018). Improving the implementation of marine monitoring in the northeast Atlantic. Mar. Pollut. Bull. 128, 527–538. doi: 10.1016/J.MARPOLBUL.2018.01.067
van Tussenbroek, B. I., Hernández-Arana, H. E., Rodríguez-Martínez, R., Espinoza-Avalos, J., Canizales-Flores, H. M., González-Godoya, C. E., et al. (2018). Severe impacts of brown tides caused by Sargassum spp. on near-shore Caribbean seagrass communities. Mar. Pollut. Bull. 122, 272–281. doi: 10.1016/j.marpolbul.2017.06.057
von Schuckmann, K., Le Traon, P.-Y., Smith, N., Pascual, A., Brasseur, P., Fennel, K., et al. (2018). Copernicus marine service ocean state report. J. Oper. Oceanogr. 11, S1–S142. doi: 10.1080/1755876X.2018.1489208
Wang, M., and Hu, C. (2017). Predicting Sargassum blooms in the Caribbean Sea from MODIS observations. Geophys. Res. Lett. 44, 3265–3273. doi: 10.1002/2017gl072932
Webster, R. K., and Linton, T. (2013). Development and implementation of sargassum early advisory system (SEAS). Shore Beach 81, 1–6.
Weijerman, M., Fulton, E. A., and Brainard, R. E. (2016). Management strategy evaluation applied to coral reef ecosystems in support of ecosystem-based management. PLoS One 11:e0152577. doi: 10.1371/journal.pone.0152577
Welch, H., Hazen, E., Bograd, S., Jacox, M., Brodie, S., Robinson, D., et al. (2018). Practical considerations for operationalizing dynamic management tools. J. Appl. Ecol. 56, 459–469. doi: 10.1111/1365-2664.13281
Wilkinson, M. D., Dumontier, M., Aalbersberg, I. J., Appleton, G., Axton, M., Baak, A., et al. (2016). The FAIR guiding principles for scientific data management and stewardship. Sci. Data 3:160018. doi: 10.1038/sdata.2016.18
Zador, S. (2014). Ecosystem Considerations 2014 Status of Alaska’s Marine Ecosystems. Anchorage, AK: North Pacific Fishery Management Council.
Zador, S. (2015). Ecosystem Considerations 2015 Status of Alaska’s Marine Ecosystems. Anchorage, AK: North Pacific Fishery Management Council.
Zador, S., and Yasumiishi, E. (2016). Ecosystem Considerations 2016: Status of the Gulf of Alaska Marine Ecosystem, Stock Assessment and Fishery Evaluation Report. Anchorage, AK: North Pacific Fishery Management Council.
Zador, S., and Yasumiishi, E. (2017). Ecosystem Considerations 2017: Status of the Gulf of Alaska Marine Ecosystem, Stock Assessment and Fishery Evaluation Report. Anchorage, AK: North Pacific Fishery Management Council.
Keywords: Ecosystem Based Management, technology, data needs, capacity development, Integration of Ocean Observing Systems
Citation: Schmidt JO, Bograd SJ, Arrizabalaga H, Azevedo JL, Barbeaux SJ, Barth JA, Boyer T, Brodie S, Cárdenas JJ, Cross S, Druon J-N, Fransson A, Hartog J, Hazen EL, Hobday A, Jacox M, Karstensen J, Kupschus S, Lopez J, Madureira LAS-P, Martinelli Filho JE, Miloslavich P, Santos CP, Scales K, Speich S, Sullivan MB, Szoboszlai A, Tommasi D, Wallace D, Zador S and Zawislak PA (2019) Future Ocean Observations to Connect Climate, Fisheries and Marine Ecosystems. Front. Mar. Sci. 6:550. doi: 10.3389/fmars.2019.00550
Received: 15 November 2018; Accepted: 20 August 2019;
Published: 13 September 2019.
Edited by:
Frank Edgar Muller-Karger, University of South Florida, United StatesReviewed by:
Brian P. V. Hunt, University of British Columbia, CanadaMitchell Alan Roffer, Roffer’s Ocean Fishing Forecasting Service, United States
Copyright © 2019 Schmidt, Bograd, Arrizabalaga, Azevedo, Barbeaux, Barth, Boyer, Brodie, Cárdenas, Cross, Druon, Fransson, Hartog, Hazen, Hobday, Jacox, Karstensen, Kupschus, Lopez, Madureira, Martinelli Filho, Miloslavich, Santos, Scales, Speich, Sullivan, Szoboszlai, Tommasi, Wallace, Zador and Zawislak. This is an open-access article distributed under the terms of the Creative Commons Attribution License (CC BY). The use, distribution or reproduction in other forums is permitted, provided the original author(s) and the copyright owner(s) are credited and that the original publication in this journal is cited, in accordance with accepted academic practice. No use, distribution or reproduction is permitted which does not comply with these terms.
*Correspondence: Jörn O. Schmidt, anNjaG1pZHRAZWNvbm9taWNzLnVuaS1raWVsLmRl