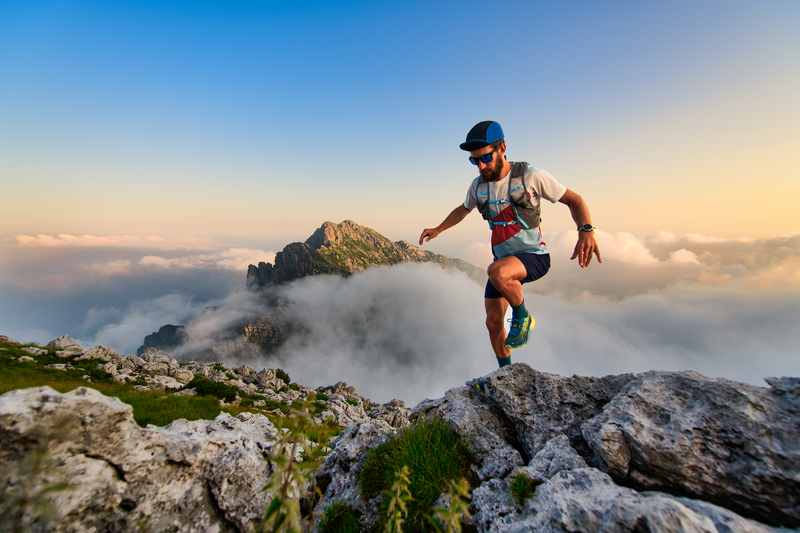
94% of researchers rate our articles as excellent or good
Learn more about the work of our research integrity team to safeguard the quality of each article we publish.
Find out more
ORIGINAL RESEARCH article
Front. Mar. Sci. , 10 September 2019
Sec. Marine Biogeochemistry
Volume 6 - 2019 | https://doi.org/10.3389/fmars.2019.00522
Many calcifying organisms exert significant biological control over the construction and composition of biominerals which are thus generally depleted in oxygen-18 and carbon-13 relative to the isotopic ratios of abiogenic aragonite. The skeletal δ18O and δ13C values of specimens of Mediterranean zooxanthellate (Balanophyllia europaea and Cladocora caespitosa) and non-zooxanthellate corals (Leptopsammia pruvoti and Caryophyllia inornata) were assessed along an 8° latitudinal gradient along Western Italian coasts, spanning ∼2°C and ∼37 W m–2 of annual average sea surface temperature and solar radiation (surface values), respectively. Seawater δ18O and δ13CDIC were surprisingly constant along the ∼850 km latitudinal gradient while a ∼2 and ∼4% variation in skeletal δ18O and a ∼4 and ∼9% variation in skeletal δ13C was found in the zooxanthellate and non-zooxanthellate species, respectively. Albeit Mediterranean corals considered in this study are slow growing, only a limited number of non-zooxanthellate specimens exhibited skeletal δ18O equilibrium values while all δ13C values in the four species were depleted in comparison to the estimated isotopic equilibrium with ambient seawater, suggesting that these temperate corals cannot be used for thermometry-based seawater reconstruction. Calcification rate, linear extension rate, and skeletal density were unrelated to isotopic compositions. The fact that skeletal δ18O and δ13C of zooxanthellate corals were confined to a narrower range at the most isotopically depleted end compared to non-zooxanthellate corals, suggests that the photosynthetic activity may restrict corals to a limited range of isotopic composition, away from isotopic equilibrium for both isotopes. Our data show that individual corals within the same species express the full range of isotope fractionation. These results suggest that metabolic and/or kinetic effects may act as controlling factors of isotope variability of skeleton composition along the transect, and that precipitation of coral skeletal aragonite occurs under controlling kinetic biological processes, rather than thermodynamic control, by yet unidentified mechanisms.
Scleractinian corals retain records of the chemical and physical conditions of the local surrounding seawater at the time of skeletal calcium carbonate accretion (McConnaughey, 2003; Allemand et al., 2004; Meibom et al., 2007), thus serving as oceanic recorders with monthly to seasonal resolution (McCulloch et al., 1999; Cohen et al., 2001; Felis et al., 2003). The skeletal carbon and oxygen isotopic ratios reflect temperatures, evaporation, precipitation, light intensity and primary production activity in the surrounding seawater but in a complex, and non-linear way (Levy et al., 2006; Rodrigues and Grottoli, 2006). In particular, skeletal δ18O is the most commonly used proxy for seawater temperature and salinity (Al-Rousan et al., 2003; Asami et al., 2004; Linsley et al., 2006). In general, the isotopic composition of oceanic water masses changes as a result of water loss by evaporation, that enriches the heavy isotope species in the surface waters, and water inflow by precipitation and/or river discharge, which cause a decrease of δ18O values of the oceanic water (Craig, 1961), thus revealing a direct relationship between salinity and δ18O values. Moreover, at constant δ18O, an composition of seawater increase of 1°C in SST is coupled to a decrease in coral δ18O of 0.18 to 0.22% (CorreÌge, 2006). Coral skeletal δ18O and δ13C are generally shifted toward lower isotope values compared to aragonite in equilibrium with seawater (Weber and Woodhead, 1970, 1972; Weber, 1974; McConnaughey, 1989a). These so-called vital effects (Urey et al., 1951; Weber and Woodhead, 1972) or physiological effects (e.g., Epstein et al., 1951), may override environmental signals (e.g., Meibom et al., 2003, 2004; Rollion-Bard et al., 2003). However, in some cases, the environmentally controlled fraction that reflects the ambient temperature and isotopic composition of the water can be extracted and used for climatic reconstructions, as performed by Smith et al. (2000) in cold-water corals collected from the Norwegian Sea to the Antarctic.
Vital effects are the result of a biologically controlled calcification process (Wilbur and Simkiss, 1979; Allemand et al., 2004). In scleractinian corals, calcification is thought to occur within a physiologically controlled calcifying fluid (cf) in a semi-confined environment between the coral skeleton and its calicoblastic cell layer8,9,10. Carbonate chemistry of the calcifying fluid is exquisitely regulated through Ca2+-ATPase proton pumps acting as Ca2+/H+ exchangers (or antiporters), which remove two H+ ions from the calcifying fluid in exchange for every Ca2+ ion transported from the calicoblastic epithelial cells into the calcifying space (Al-Horani et al., 2003; Zoccola et al., 2004). By this process, calcifying fluid pH can be elevated significantly above ambient. Sources of dissolved inorganic carbon (DIC) can be either from seawater or from coral respiration and can be in the form of: (1) CO2 freely diffusible across lipid membranes (Sueltemeyer and Rinast, 1996); and (2) bicarbonate (HCO3–) requiring specific carrier proteins (Furla et al., 2000; Vidal-Dupiol et al., 2013).
Physiological/vital effects can be attributed to “metabolic” or “kinetic” isotope fractionations. While metabolic effects are caused primarily by respiration and photosynthesis (Weber and Woodhead, 1970; Weber, 1974; Weber et al., 1976; Goreau, 1977a,b; Swart, 1983), kinetic effects associated with the hydration and hydroxylation of carbon dioxide during coral skeletogenesis result in simultaneous isotopic depletion of carbon and oxygen (relative to aragonite isotopic equilibrium; McConnaughey, 1989a,b). The prevailing hypothesis is that photosynthesis preferentially removes the light isotope of carbon from the internal pool of DIC while respiration enriches the pool in the light isotope (Weber and Woodhead, 1970; Weber, 1974; Weber et al., 1976; Goreau, 1977a,b; Swart, 1983). In general, since calcification in symbiotic corals should be on average three times higher during the day (Gattuso et al., 1999), when photosynthetic CO2 uptake is several times faster than respiratory CO2 release (McConnaughey, 1989a; McConnaughey et al., 1997), photosynthesis affects δ13C up to 11% more strongly than respiration (about 1.5%). Thus, corals in which zooxanthellae are photosynthesizing rapidly will tend to show isotopically enriched skeletons compared to those in which photosynthesis is occurring much slower or does not occur at all (i.e., non-zooxanthellate species; Heikoop et al., 2000). Rollion-Bard et al. (2003) suggested that the observed δ18O values reflect partial isotopic re-equilibration with water following kinetic CO2 hydration/hydroxylation reactions while Adkins et al. (2003) proposed a quantitative (carbonate based) model to explain both carbon and oxygen fractionations. Both models allowed a “leak” of seawater to the calcifying sites. Recently, Chen et al. (2018) expanded this model and incorporated carbonic anhydrase activity to better explain the mechanism and the depletion relation between δ18O and δ13C. It is widely recognized that vital effects are not always constant within a genus or species, or even within individual corals, with faster growing individuals or faster growing parts of coral skeletons being generally more depleted in both δ18O and δ13C (e.g., Land et al., 1975; McConnaughey, 1989a; Cohen and McConnaughey, 2003). In fact, it has been observed that biomineralization in corals does not follow classical thermodynamic rules but rather responds to biological kinetic effects, following a mechanism that has not yet been unraveled (Juillet-Leclerc et al., 2018). McConnaughey (1989a) investigated zooxanthellate and non-zooxanthellate coral species from the in an attempt to same growth environment unravel the chemical mechanisms behind vital effects. His results showed that the photosynthetic Pavona had higher δ13C than the non-photosynthetic Tubastrea, suggesting that algal preferential uptake of 12C leaves the residual inorganic calcification “pool” enriched in 13C, thereby increasing the δ13C of the skeleton. Moreover, McConnaughey (1989b) concluded that skeleton carbonate precipitation must be fast enough to allow absorption of HCO3– and/or CO32–, formed by CO2 hydration and hydroxylation, before they isotopically re-equilibrate with seawater.
In the Mediterranean Sea, coral-based paleoclimatic studies are limited compared to tropical regions. Temperate seas like the Mediterranean are characterized by pronounced seasonal irradiance, temperature, and nutrient cycles, while high temperature and irradiance, and low nutrients are far less variable in tropical seas (Ferrier-Pagès et al., 2012). The Mediterranean basin is considered an oligotrophic basin (Sournia, 1973), with generally low chlorophyll concentration, with the exception of a large bloom observed in the Liguro-Provencal Region (D’Ortenzio and Ribera d’Alcalà, 2009). Along the western Italian coast, the wind effect, coastal upwelling, and winter layers mixing, determine a decrease in the biomass of phyto- and zooplankton from north to south (D’Ortenzio and Ribera d’Alcalà, 2009). Local currents and rock composition at the Genova site considered in this study generate particular local conditions leading to higher SSTs than expected at that latitude [annual SST of Ligurian Sea = 18°C and Genova–Portofino (GN) = 19°C]. Moreover, the Strait of Messina, where the Scilla site is located, lies at the center of the Mediterranean Sea and is characterized by strong currents, including upwelling of deeper water of Levantine intermediate water origin (Cortese and De Domenico, 1990), which are colder, more salty and nutrient-rich with respect to the Tyrrhenian Surface Waters (Atlantic Water origin). Such environmental differences must be cautiously taken into account when investigating and interpreting the skeletal isotope signal of temperate corals. Stable isotope and trace element composition, in combination with U-series and radiocarbon dating of the zooxanthellate coral Cladocora caespitosa, and of the cold-water corals Desmophyllum dianthus, Lophelia pertusa, and Madrepora oculata have been validated as high-resolution paleoenvironmental proxies (Peirano et al., 2004; Silenzi et al., 2005; Cohen et al., 2006; Montagna et al., 2008; López Correa et al., 2010; Trotter et al., 2011).
The aim of the present study was to investigate the species-specific variation of skeletal δ18O and δ13C and “vital effects” in four Mediterranean scleractinian species along an 8° latitudinal gradient along the Italian coast. We collected and analyzed the colonial zooxanthellate C. caespitosa (Linnaeus, 1767), the solitary zooxanthellate Balanophyllia europaea (Risso, 1826), and the two solitary non-zooxanthellates Leptopsammia pruvoti Lacaze-Duthiers, 1897 and Caryophyllia inornata (Duncan, 1878). We measured the surrounding seawater δ18O and δ13C of DIC to determine the fractionation between coral skeletons and seawater during calcification.
Specimens of four coral species and seawater samples were collected between July 2010 and June 2013 from six sites along a wide latitudinal gradient spanning ∼850 km and ∼2°C of average sea surface temperature along the Western Italian coast (44°20′N to 36°45′N; Figure 1 and Table 1). B. europaea and C. caespitosa specimens were randomly collected along a reef with southerly exposure at a depth of 5–7 m. All four coral species occur at all six sites, except for C. caespitosa which was not sampled at Scilla. Leptopsammia pruvoti and C. inornata specimens were randomly collected on the vault of crevices at a depth of 15–18 m, except for the Elba site where C. inornata was collected under the wings of a sunken plane wreck, at a depth of 12–15 m. Coral tissue was removed by immersing the samples in a solution of 10% commercial bleach for 3 days. Corals were dried for 4 days at a maximum temperature of 50°C to avoid phase transitions in the skeletal carbonate (Vongsavat et al., 2006). Samples were inspected under a binocular microscope to remove fragments of substratum and calcareous deposits produced by other organisms. Polyp length (L: maximum axis of the oral disc), width (W: minimum axis of the oral disc), and height (h: oral–aboral axis) were measured using a pair of calipers (cf. Goffredo et al., 2007) and dry skeletal mass was weighed using an analytical balance (±0.1 mg).
Figure 1. Map of the Italian coastline indicating the sites where the corals and seawater samples were collected, with pattern of the main currents for the Ligurian Sea and Tyrrhenian Sea (modified from Silenzi et al., 2005; LC: Ligurian–Provencal Current; TC: Tyrrhenian Current). Abbreviations and coordinates of the site in decreasing order of latitude: GN Genova, 44°20′N, 9°08′E; CL Calafuria, 43°27′N, 10°21′E; LB Elba Isle, 42°45′N, 10°24′E; PL Palinuro, 40°02′N, 15°16′E; SC Scilla, 38°01′N, 15°38′E; PN Pantelleria Isle, 36°45′N, 11°57′E.
Table 1. Solar radiation (SR) values at sea surface and sampling depth temperature (DT) at 6 and 16 m at each site.
Seawater samples for δ18O (N = 5–8 per site at 6 and 16 m depth; Table 2) were collected by SCUBA in 50 ml plastic bottles, sealed, and kept in a refrigerator at 4°C for 3–6 months prior to analysis. Seawater samples for δ13C of dissolved inorganic carbon (DIC; N = 2–5 per site at 6 and 16 m depth; Table 2) were collected by SCUBA in borosilicate-glass bottles, and 1 ml of saturated mercuric chloride (HgCl2) was introduced to 100 ml of seawater in order to stop all biological activity. The bottles were kept in a refrigerator at 4°C for 3–6 months prior to analysis. Water samples were collected in duplicate.
Table 2. Linear regression and correlation analysis between skeletal δ13C and δ18O in the four individual species and for each group, zooxanthellate and non-zooxanthellate species.
For skeletal δ13C and δ18O analysis, the whole corallite, which represents approximately the past 5–20 years of growth, was ground in a mortar to obtain a fine and homogeneous powder. Skeletal CaCO3 samples of 220–250 μg were reacted with 100% orthophosphoric acid and CO2 gas was analyzed using a Finnigan GasBench II connected in line to a Finnigan MAT 252 isotope ratio mass spectrometer. Calibration was maintained by routine analyses of internal and international standards. The long-term precision of our internal laboratory standard is 0.06 and 0.10% for carbon and oxygen, respectively. Oxygen isotopes analysis of sea water (δ18Osw) were carried out by equilibrating 0.5 ml of samples with a mixture of 0.5% CO2 in He at 25°C for 24 h. The samples were analyzed on a Gas Bench II connected in line to a Finnigan MAT 252 mass spectrometer. The results are reported relative to the Vienna Standard Mean Ocean Water (VSMOW) with 0.05% (±1σ) long-term precision of laboratory working standard.
For seawater δ13C of DIC (δ13CDIC) analysis, 1 ml of seawater was injected into gas vials pre-flushed with He, then acidified with 0.15 ml H3PO4 and left to react for 24 h in 25°C. The samples were analyzed on a Gas Bench II and Finigan MAT 252. The results are reported relative to the international Vienna-PeeDee Belemnite (VPDB) standard with 0.08% long-term precision (±1σ) of NaHCO3 laboratory standard (chemically pure). The coral δ13Cskeleton and δ18Oskeleton data are reported against VPDB-standard.
The mean annual calcification rate (mass of CaCO3 deposited per year per area unit) was calculated for each specimen by applying the following formula: calcification (mg mm–2 yr–1) = skeletal density (mg mm–3) × linear extension (mm yr–1) (Lough and Barnes, 2000; Carricart-Ganivet, 2004). Corallite length (L, maximum axis of the oral disc), width (W, minimum axis of the oral disc), and height (h, oral-aboral axis) were measured with calipers and the dry skeletal mass (M) was measured with a precision balance. Corallite volume was calculated using the formula: V = L2 × W2 × hπ (Goffredo et al., 2007) The skeletal density for each corallite was calculated as M/V and the linear extension as L/age.
The age of each specimen was estimated by applying the Von Bertalanffy growth function, using the asymptotic length and growth constants for each population following Goffredo et al., 2008 for B. europaea, Caroselli et al., 2012b for L. pruvoti, and Caroselli et al., 2016b for C. inornata.
Average monthly values of salinity were obtained using the Copernicus Marine Service Product MEDSEA_REANALYSIS_PHYS_006_0041, and visualized through the Panoply software version 4.10.42. Monthly at-depth temperatures (DT) at 6 m were obtained from Airi et al. (2014) and at 16 m were obtained from Caroselli et al. (2016a). Monthly values of solar radiation (SR; in W m–2) were obtained from Caroselli et al. (2016a). Temperature data (°C) were recorded by digital thermometers (i-Button, DS1921G-F5#, Maxim Integrated Products, Dallas Semiconductors) placed at the sampling location (∼ 6 or 16 m depth, depending on the species) for each population. Sea Surface Temperature historical data (SST;°C) were obtained for each site from the National Mareographic Network of the Superior Institute for the Environmental Protection and Research (ISPRA3). Site-by-site, historical at-depth temperatures were estimated by linear regression produced between the temperature data recorded by the digital thermometers and SST. Monthly values of solar radiation (SR; W m–2) were obtained from the databank of the Satellite Application Facility on Climate Monitoring (CM-SAF/EUMETSAT4) and represent values at sea surface. In this study, the average DT and SR of the 6 years preceding sampling (n = 72 monthly temperatures) was considered based on the mean turnover time of populations (calculated as the reciprocal of the instantaneous rate of mortality, Z; Caroselli et al., 2016a,b).
One-way analysis of variance (ANOVA) was used to compare environmental parameters, seawater and coral isotope data among sites. Data were checked for normality using a Kolmogorov-Smirnov’s test and for variance homoskedasticity using a Levene’s test. When assumptions for parametric statistics were not fulfilled, the non-parametric Kruskal-Wallis equality-of-populations rank test was used, including the Monte Carlo correction for small sample size (Gabriel and Lachenbruch, 1969). The Monte Carlo correction solves problems in non-parametric tests for small samples, because it estimates the p-value by taking a random sample from the reference set and studies its permutations (Senchaudhuri et al., 1995). Pearson’s correlation coefficients were calculated to test the relationship between environmental parameters and latitude, between skeletal δ13C and δ18O in the four individual species and for each group, zooxanthellate (B. europaea and C. caespitosa) and non-zooxanthellate (C. inornata and L. pruvoti) species, and between skeletal δ13C and δ18O and skeletal parameters (i.e., net calcification rate, linear extension rate, and skeletal density) in three species (B. europaea, C. inornata, and L. pruvoti). Spearman’s rank correlation coefficient was used to calculate the significance of the correlations between coral δ13C and δ18O and latitude and seawater temperature. Spearman’s rank correlation coefficient is an alternative to Pearson’s correlation coefficient (Altman, 1991). It is useful for data that are non-normally distributed and do not meet the assumptions of Pearson’s correlation coefficient (Potvin and Roff, 1993). Analysis of covariance (ANCOVA) was used to examine differences in δ13C and δ18O regression slopes between species. All analyses were computed using SPSS 20.0.
Average DT and solar radiation (SR) both varied among sites (DT at 6 m, Kruskal Wallis test, χ = 16.9, n = 6, p < 0.010; DT at 16 m, Kruskal Wallis test, χ = 21.7, n = 6, p < 0.010; SR, Kruskal Wallis test, χ = 18.4, n = 6, p < 0.010). While SR was negatively correlated with latitude (Pearson’s correlation, n = 6, p < 0.010; Figure 2A), DT was not correlated with latitude (Pearson’s correlation, n = 6, p > 0.050; Figure 2B). The 6 years average monthly values of SR ranged from 162.0 W m–2 at GN to 212.3 W m–2 at PN (Table 1 and Supplementary Table S1). The 6 years average monthly seawater temperature exhibited an annual cycle in all sites, reaching summer maxima of 21.7–23.7°C and 20.3–22.9°C at 6 and 16 m depth, respectively, and winter minima of 13.4–16.7°C and 13.1–15.6°C at 6 and 16 m depth, respectively (Table 1 and Supplementary Tables S2, S3). The temperature difference between 6 and 16 m depth was not significantly different (average temperature, t-Test, t = 1.208, n = 12, p > 0.050; average minimum temperature, t-Test, t = 0.604, n = 12, p > 0.050; average maximum temperature, t-Test, t = 1.489, n = 12, p > 0.050; Supplementary Tables S2, S3), in the order of 0.4–0.8°C for annual average and both, summer and winter months. The annual amplitude was maximal in Genova (∼8–9°C), and minimal in Scilla (∼6°C: Supplementary Tables S2, S3).
Figure 2. The relation between average solar radiation values at sea surface (A), temperature (B), δ18Oseawater (C), and δ13CDIC (D) with latitude at 6 and 16 m depth in 6 sites along the west coast of Italy (∼850 km transect).
Average annual δ18Osw along the Italian western coast was 0.65 ± 0.23% (mean ± SD) at 6 m and 0.71 ± 0.24% at 16 m and ranged between 0.60 and 0.72% at 6 m and between 0.58 and 0.81% at 16 m depth. The average annual δ13CDIC along the transect was 0.99 ± 0.17% at 6 m and 0.99 ± 0.12% at 16 m relative to VPDB and ranged between 0.67 and 1.07% at 6 m and between 0.72 and 1.08% at 16 m depth. No significant differences in average annual δ18Osw were found among sites (Kruskal-Wallis test, χ = 1.611, χ = 3.853, n = 40 and n = 38 at 6 and 16 m depth, respectively, p > 0.050; Figure 2C and Table 2) which was remarkably constant along the 850 km North-South transect. Also, average annual δ13CDIC did not exhibit significant differences among sites either (Kruskal-Wallis test, χ = 9.232, χ = 9.357, n = 22 and n = 20 at 6 and 16 m depth, respectively, p > 0.050; Figure 2D and Table 2).
The isotopic value for a biological aragonite precipitated in quasi-equilibrium with ambient seawater along the latitudinal gradient, calculated from Grossman and Ku (1986) for oxygen [T = 20.6 – 4.34(δ18Oarag – δ18Oseawater] and Romanek et al. (1992) for carbon (δ13Carag = δ13CDIC + 2.7), using the annual average temperature from all stations at both depths, resulted in 0.51% for δ18O and 2.7% for δ13C (Figure 3). Average δ18Oseawater and δ13CDIC were not included in the equation as the graph axis is respectively, δ18Oarag – δ18Oseawater and δ13Carag – δ13CDIC.
Figure 3. Isotopic comparison between Balanophyllia europaea (zooxanthellate), Cladocora caespitosa (zooxanthellate), Leptopsammia pruvoti (non-zooxanthellate), and Caryophyllia inornata (non-zooxanthellate) at 6 sites along the west coast of Italy (∼850 km transect). Regression lines are the best fit to the zooxanthellate and non-zooxanthellate data sets. The black square indicates the estimated aragonite equilibrium value for average annual temperature for the 6 sites along the gradient using Grossman and Ku (1986) for oxygen and Romanek et al. (1992) for carbon. Vertical bar indicates equilibrium values corresponding to maximum and minimum average temperature. r2, Pearson’s coefficient of determination; r, Pearson’s correlation coefficient; n, number of individuals.
Average skeletal δ18O and δ13C along the gradient was −4.32 ± 0.71% (mean ± SD) and −1.66 ± 0.36%, respectively, in B. europaea, −4.28 ± 0.28% and −1.59 ± 0.19%, respectively, in C. caespitosa, −2.50 ± 1.21% and 0.88 ± 0.50%, respectively, in L. pruvoti, and −3.83 ± 1.14% and −0.09 ± 0.47%, respectively, in C. inornata (Table 3) relative to VPDB. Both skeletal δ13C and δ18O differed among sites in all species (B. europaea, ANOVA, n = 30, p < 0.01, F = 4.904 and F = 5.240, respectively; C. caespitosa, ANOVA, n = 25, p < 0.001, F = 39.601 and F = 24.489, respectively; L. pruvoti, ANOVA, n = 30, p < 0.001, F = 13.212 and F = 8.049, respectively; C. inornata, ANOVA, n = 30, p < 0.001, F = 17.735 and F = 11.225, respectively; Table 3). Skeletal isotope data are presented as δ13Cskeleton – δ13CDIC or δ18Oskeleton – δ18Osw in order to account for the isotopic composition of the local seawater in each site. A strong positive correlation between skeletal δ13C and δ18O was observed in all species (Table 4). The slopes within each group, zooxanthellate and non-zooxanthellate, did not differ (B. europaea vs C. caespitosa, ANCOVA, F = 0.382, n = 30 and 25, respectively; L. pruvoti vs C. inornata, ANCOVA, F = 0.492, n = 30 both species; p > 0.050; Figure 3). The slopes did not differ also between the two groups (zooxanthellate vs non-zooxanthellate, ANCOVA, F = 0.994, n = 55 and 60, respectively; p > 0.050; Figure 3). Latitude showed a significant positive correlation with both skeletal δ13C and δ18O in B. europaea (Spearman’s correlation, r2 = 0.361 and 0.438, respectively, n = 30, p < 0.001; Figure 4), and C. caespitosa (Spearman’s correlation, r2 = 0.360, n = 25, p < 0.010 and r2 = 0.214, n = 25, p < 0.050, respectively; Figure 4). Solar radiation showed a symmetrical trend with a negative correlation with both skeletal δ13C and δ18O in B. europaea and C. caespitosa (Supplementary Figure S1). Generally, no significant correlation was found between skeletal δ18O or δ13C and average annual temperature, except for δ18O of C. inornata which correlated positively (Spearman’s correlation, r2 = 0.287, n = 30, p < 0.010; Supplementary Figure S2). Skeletal δ13C and δ18O values of B. europaea were negatively correlated with average minimum temperature (Spearman’s correlation, r2 = 0.301, n = 30, p < 0.001 and r2 = 0.179, n = 30, p < 0.050, respectively; Figures 5A,B), while skeletal δ18O of C. inornata was positively correlated with average minimum temperature (Spearman’s correlation, r2 = 0.201; n = 30, p < 0.050; Figure 5H). Skeletal δ13C and δ18O values were positively correlated with average maximum temperature in C. caespitosa (Spearman’s correlation, r2 = 0.232, n = 25, p < 0.050 and r2 = 0.487, n = 25, p < 0.001, respectively; Figures 6C,D) and C. inornata (Spearman’s correlation, r2 = 0.182, n = 30, p < 0.050 and r2 = 0.397, n = 30, p < 0.001, respectively; Figures 6G,H).
Table 3. Seawater stable isotope data at 6 and 16 m depth in 6 sites along the west coast of Italy (∼850 km transect).
Table 4. Coral aragonite stable isotope data and net calcification rate (mg mm–2 yr–1), linear extension rate (mm yr–1) and bulk skeletal density (mg mm–3) in Balanophyllia europaea, Leptopsammia pruvoti, and Caryophyllia inornata at 6 sites along the west coast of Italy (∼850 km transect).
Figure 4. The relation between skeletal δ13C and δ18O with latitude in B. europaea (A,B), C. caespitosa (C,D), L. pruvoti (E,F), and C. inornata (G,H). r2, Spearman’s determination coefficient; r, Spearman’s correlation coefficient; n, number of individuals.
Figure 5. The relation between skeletal δ13C and δ18O with average minimum temperature in B. europaea (temperature at 6 m; A,B), C. caespitosa (temperature at 6 m; C,D), L. pruvoti (temperature at 16 m; E,F), and C. inornata (temperature at 16 m; G,H). r2, Spearman’s determination coefficient; r, Spearman’s correlation coefficient; n, number of individuals.
Figure 6. The relation between skeletal δ13C and δ18O with average maximum temperature in B. europaea (temperature at 6 m; A,B), C. caespitosa (temperature at 6 m; C,D), L. pruvoti (temperature at 16 m; E,F), and C. inornata (temperature at 16 m; G,H). r2, Spearman’s determination coefficient; r, Spearman’s correlation coefficient; n, number of individuals.
Generally, no correlation was found between skeletal δ13C and δ18O and net calcification rate, linear extension rate, and skeletal density, in neither of the species, except for linear extension rate in L. pruvoti that showed a positive correlation with both δ13C and δ18O (Pearson’s correlation, r2 = 0.288, n = 30, p < 0.010 and r2 = 0.146, n = 30, p < 0.050, respectively; Figure 7 and Table 3).
Figure 7. The relation between skeletal δ13C and δ18O and net calcification rate (mg mm−2 yr−1; A,D), linear extension rate (mm yr−1; B,E) and skeletal bulk density (mg mm−3; C,F) in B. europaea, L. pruvoti, and C. inornata at 6 sites along the west coast of Italy (∼850 km transect). Net calcification rate, linear extension rate and skeletal density data were taken from Goffredo et al. (2009) and Caroselli et al. (2012a, 2016b). r2, Pearson’s coefficient of determination; r, Pearson’s correlation coefficient; n, number of individuals.
This study investigated for the first time the skeletal δ18O and δ13C of individual specimens of zooxanthellate and non-zooxanthellate Mediterranean coral species and the stable isotope signature of the surrounding seawater collected along a ∼850 km latitudinal gradient. Previous studies performed on the solitary zooxanthellate B. europaea along the same latitudinal gradient considered in this study showed a reduction of the net calcification rate, which results in a progressive decrease of skeletal bulk density and an increase in skeletal porosity, especially of larger sized pores with increasing seawater temperature (Caroselli et al., 2011; Fantazzini et al., 2013). This determines a decrease of the resistance of the skeleton to mechanical stress (Goffredo et al., 2015). Furthermore, its population stability and abundance decrease with increasing temperature, as evidenced by a progressive lack of juveniles (Goffredo et al., 2007, 2008). On the other hand, the solitary non-zooxanthellate (i.e., asymbiotic) corals L. pruvoti and C. inornata appear insensitive to temperature along the same gradient (Goffredo et al., 2007, 2008, 2009; Caroselli et al., 2011, 2012a,b; Fantazzini et al., 2013). A study conducted along a natural temperature gradient in the Eastern Adriatic Sea on the colonial zooxanthellate coral C. caespitosa showed increases in net calcification rate with SST (Kružić et al., 2012), in contrast with laboratory observations showing that long-term exposure to high temperature led to a decrease in net calcification (Rodolfo-Metalpa et al., 2006). However, none of these studies investigated the species-specific variation of skeletal δ18O and δ13C under different temperature conditions.
In this study, a wide variation in both skeletal δ18O and δ13C (∼2 to ∼4% and ∼4 to ∼9%, respectively) was found among the investigated species which could be attributed to metabolic/kinetic effects, given the relatively limited seawater temperature difference (∼2°C annual average SST, which however, was not correlated with latitude) and the homogeneity of the seawater δ18O and δ13CDIC measured along the gradient. Seawater δ18O was remarkably constant along the 850 km transect with a restricted variation in salinity from 37.7 psu in Pantelleria to 38.1 psu in Genova, indicating that evaporation and precipitation and/or river discharges do not vary substantially along the gradient. Average δ18Osw values along our gradient (0.65 ± 0.23%) were lower than those measured for surface waters in the Eastern Mediterranean Sea by Pierre et al. (1986) (1.68%) and by Gat et al. (1996) (∼1.38%), probably as a result of higher temperature and salinity values compared to the Western Mediterranean Sea (Sakalli, 2017). Pantelleria seems to show slightly lower δ18Osw values along the latitudinal gradient, perhaps as a remnant of Atlantic water surface inflow, prior to increased evaporation, when moving north as Tyrrhenian Current (Paul et al., 2001). We also observed the lower δ18Osw values for the shallower sampling depths of 6 m in Elba, Calafuria and Genova, which could be linked to river inflow and salinity, however, we exclude this possibility as the average salinity would have to be lower than in the other locations, which is not the case. Further seawater isotope measurements are needed to verify whether this observation is maintained. The average annual δ13C of the DIC also did not vary along the transect, suggesting that local primary production does not control the absolute value and is kept at the same level in coastal waters. Apparently, gas exchange and water mass characteristics override the primary production signal. The well mixed water column and the lack of stratified primary production is evident from the average annual δ13CDIC identity between 6 and 16 m.
At thermodynamic equilibrium, skeletal δ18O is a function of temperature and δ18Oseawater from which the coral deposited its CaCO3. Hence, along the 850 km transect, where δ18Oseawater shows small variations, changes in coral skeletal δ18O should reflect changes in seawater temperature (Weber and Woodhead, 1972; Gagan et al., 2000; Kuhnert et al., 2000). The measured yearly average temperature change along the transect is about 2°C, yielding an expected skeletal δ18O change of about 0.5% according to the coral aragonite temperature dependency (0.22% per 1°C; Epstein et al., 1953; Grossman and Ku, 1986; Böhm et al., 2000). Instead, a ∼2 and ∼4% variation in skeletal δ18O was observed in the zooxanthellate and non-zooxanthellate species, respectively (Figure 3). The skeletal δ13C, which is strongly influenced by photosynthesis (light) and feeding (which directly affects the respired δ13C; McConnaughey, 2003), exhibits also a wide range, ∼4% for zooxanthellate corals and ∼9% for the non-zooxanthellate corals. These ranges, as well as the significant correlations between δ18O and δ13C in all species, point either to the metabolic and/or kinetic effects as possible controlling factors of isotope variability of skeleton composition along the transect (McConnaughey, 1989a; Smith et al., 2000; Schoepf et al., 2014). Given the difficulty in distinguish between kinetic and metabolic isotope effects, we applied the correction for kinetic isotope effects for δ13C proposed by Heikoop et al., 2000, which however, did not improve the statistics or the interpretation of the data (Supplementary Figure S3) so we assume this correction does not work for our dataset. The full range of the isotope effect is presented in the δ18Oskeleton – δ18Osw vs δ13Cskeleton – δ13CDIC plot (Figure 3). The key observations from this plot are:
(1) The isotope range of non-zooxanthellate corals is much larger than that of the zooxanthellate corals in both carbon and oxygen.
(2) Each species encompasses the full range of its group, non-zooxanthellate or zooxanthellate.
(3) The regression lines are almost parallel within each group and did not differ between the two groups (Table 4).
(4) No corals exhibited equilibrium values as calculated for biogenic aragonite.
(5) The isotope range of different specimens on the 850 km transect is almost the same as that observed on a high resolution 2500 μm scale of the cold-water coral D. cristagalli from the Chilean Fjord (Adkins et al., 2003) and on a 4000 μm scale of the cold-water coral L. pertusa from Santa Maria di Leuca (Ionian Sea, Southern Italy; by López Correa et al., 2010). It is also comparable to the SIMS microanalysis δ18Oskeleton range (Juillet-Leclerc et al., 2018) and the high-density bands of C. caespitosa from a hundred year record in north-western Mediterranean (Silenzi et al., 2005). Bulk analyses on mm scale resolution also exhibit a similar range for Tubastrea and Pavona clavus from the Galapagos (McConnaughey, 1989a).
Calcification rate, linear extension rate and skeletal density were suggested as factors which impact the isotopic composition of calcareous organisms such as corals (Land et al., 1975; Erez, 1978; McConnaughey, 1989a). Our results show that there is no correlation between any of these parameters and the measured isotope composition (Figure 7). This conclusion holds at the species level, regardless of their trophic strategy (i.e., non-zooxanthellate or zooxanthellate), all species being slow growing corals (∼0.7 to ∼3 mm yr–1; Peirano et al., 1999). This finding contrasts McConnaughey’s (1989a) assumption that rapid skeletogenesis favors strong kinetic effects. However, we cannot exclude the possibility that this result could also be due to the uncertainty associated with the estimated calcification data (see section “Materials and Methods”). The two zooxanthellate corals, B. europaea and C. caespitosa show significant correlations of δ18O and δ13C with latitude (Figure 4) being depleted with decreasing latitude and thus increasing solar radiation (Figure 2 and Supplementary Figure S2). Given that algal photosynthesis can be inhibited by excessive irradiance (Cullen and Neale, 1994; Caroselli et al., 2015; Iluz and Dubinsky, 2015), the depleted skeletal δ13C could result from inhibited photosynthesis of the symbiotic zooxanthellae at certain times in summer. This is in agreement with previous studies performed on B. europaea along this gradient, which highlighted negative effects on growth, population dynamics, and reproductive parameters (Goffredo et al., 2007, 2008, 2009, 2015; Caroselli et al., 2011; Fantazzini et al., 2013). Moreover, photosynthesis of zooxanthellae has temperature optima (Al-Horani, 2005), thus the decrease in δ13C with increasing minimum temperature in B. europaea could be linked to an inhibition of the photosynthetic process at higher temperatures. C. caespitosa and C. inornata show an increase in δ13C and δ18O with increasing maximum temperature (Figure 6). A possible explanation for the pattern observed in C. caespitosa could be a reduction of the calcification with increasing temperature. Rodolfo-Metalpa et al. (2006) showed that long-term exposure to high temperature led to a decrease in net calcification of C. caespitosa (Rodolfo-Metalpa et al., 2006). However, further studies are needed on the growth of this species along the gradient to validate this hypothesis. Regarding the observed increase in δ13C and δ18O with increasing temperature we were not able to find any suitable explanation.
The discrimination between the “metabolic effects” and “kinetic isotope effects” is difficult. We adopt for this discussion McConnaughey’s (1989a) definition that kinetic isotope effects are characterized by strong linear correlation between skeletal δ18O and δ13C due to discrimination against the heavy isotopes during CO2 hydration and hydroxylation. Metabolic effects in McConnaughey’s (1989a) involve additional positive or negative modulation of skeletal δ13C, reflecting changes in the δ13C of DIC reservoir, caused mainly by photosynthesis and respiration. Our results show that the metabolic effect is not confined to affect carbon isotopes solely. The non-zooxanthellate L. pruvoti and C. inornata show twice the δ18O disequilibrium range compared to the zooxanthellate corals B. europaea and C. caespitosa (Figure 3). This does not support the hypothesis that zooxanthellate and non-zooxanthellate corals attain about the same equilibrium value (McConnaughey, 1989a). The fact that zooxanthellate corals exhibit a narrower range of skeletal δ18O and δ13C, which are confined to the more depleted values, suggests that the photosynthetic activity restricts the corals to a narrow range of isotopic composition, away from isotopic equilibrium for both isotopes. In Adkins’ model, which considers two major pools [CO2 (aq)] and seawater DIC for the mineralization process, this indicates lower% seawater leak and/or higher pH at the calcifying fluid (Adkins et al., 2003). The wider range and the proximity of the skeletal isotope values to equilibrium values (Figure 3) observed in the non-zooxanthellate compared to the zooxanthellate corals investigated here suggest more seawater leak to the calcifying fluid and lower pH of calcification. This may seem apparently in disagreement with the higher ΔpH (pH of calcifying fluid minus pH of seawater) observed for deep-sea species compared to tropical and temperate aragonitic specie, which however, has been suggested as a mechanism to overcome the severe environmental limitations (low levels of carbonate saturation) of the deep ocean (McCulloch et al., 2012), which certainly do not apply to the shallow water non-zooxanthellate species considered here. Moreover, a larger contribution from ambient seawater to the calcifying fluid in non- zooxanthellate corals is supported by the observed higher porosity in L. pruvoti compared to B. europaea (Caroselli et al., 2011). The higher porosity may allow seawater to reach the calcification sites through vacuole transport through cells, channels between cells and gaps between calcifying tissues, and the skeleton (Furla et al., 1998). The Mediterranean shallow water zooxanthellate and non-zooxanthellate corals investigated in this study fit well the general relation between δ18O and δ13C of deep-sea species, including the observation of the “metabolic carbon offset,” in the order of 1–2% (Adkins et al., 2003). However, the “Maximum membrane carbon flux” inflection point (i.e., at the most depleted carbon values δ13C remains constant while δ18O continues to decrease), observed in deep-sea corals is not found in our data (Adkins et al., 2003).
For a given value of δ18O, the zooxanthellate Mediterranean species have heavier δ13C compared to the non-zooxanthellate corals (Figure 3), in agreement with previous studies suggesting that photosynthesis enriches skeletal δ13C (Swart, 1983; McConnaughey, 1989a). Only a limited number of individual non-zooxanthellate corals exhibit skeletal δ18O equilibrium values while all δ13C values in the four Mediterranean species are depleted in comparison to the estimated isotopic equilibrium with ambient seawater. This suggests that temperate corals cannot be used for thermometry-based seawater reconstruction. The weak temperature dependency of B. europaea with average minimum temperature might be the exception (Figure 5). Also, the strong kinetic effect suggested by the high correlation between δ18O and δ13C observed in the zooxanthellate species likely prevents the use of these species as proxies of cloud cover, seasonality, and nutrient/zooplankton levels (e.g., Grottoli and Wellington, 1999; Heikoop et al., 2000; Grottoli, 2002).
The skeletal stable isotopic signatures of individual specimens of zooxanthellate and non-zooxanthellate Mediterranean coral species collected along an 8° latitudinal gradient on the Western Italian coast were assessed. Considering the ∼2°C (annual average SST) temperature difference and the homogeneity of the seawater δ18O and δ13CDIC measured along the gradient, the wide variation in both skeletal δ18O and δ13C (∼2 to ∼4% and ∼4 to ∼9%, respectively) among species may be attributed to metabolic/kinetic effects. In spite of the extensive effort to differentiate between the “carbonate” model and the “kinetic” model as controlling coral calcification (Adkins et al., 2003; McConnaughey, 2003; Chen et al., 2018), our data show that individual corals within the same species express the full range of isotope fractionation, suggesting strong metabolic contributions in addition to the kinetic isotope effect in these temperate corals. Therefore, we conclude that precipitation of coral skeletal aragonite likely occurs under controlled kinetic biological processes, rather than thermodynamic control alone, following a mechanism that is yet to be unraveled.
The raw data supporting the conclusions of this manuscript will be made available by the authors, without undue reservation, to any qualified researcher.
Being non-cephalopod invertebrates, the animals used in this study (Cnidaria, Scleractinia) are not included in the Directive 2010/63/EU of the European Parliament and of the Council of 22 September 2010 on the protection of animals used for scientific purposes. For this reason, no ethical statement is needed.
ZD, GF, and SG conceived and designed the research. FP, EC, and SG collected the specimens and provided the background data. RY, OL, and AS analyzed the samples. FP performed the statistical analyses. FP, RY, OL, SG, and AS participated in the scientific discussion. All authors contributed to the writing of the manuscript and gave final approval for publication.
The research leading to these results was supported by the European Research Council under the European Union’s Seventh Framework Programme (FP7/2007-2013)/ERC grant agreement number (249930- CoralWarm: Corals and global warming: the Mediterranean versus the Red Sea). EC was supported by the ALMA IDEA grant of the University of Bologna for the project “STRAMICRO.”
The authors declare that the research was conducted in the absence of any commercial or financial relationships that could be construed as a potential conflict of interest.
The authors would like to thank the diving centers Centro Immersioni Pantelleria, Il Pesciolino, Bubble Lounge, and Sub Maldive for their logistic assistance in the field. The Scientific Diving School (www.sdseducational.org) provided the technical and logistical support. The De Botton Center for Marine Science at the Weizmann Institute provided partial support.
The Supplementary Material for this article can be found online at: https://www.frontiersin.org/articles/10.3389/fmars.2019.00522/full#supplementary-material
Adkins, J. F., Boyle, E. A., Curry, W. B., and Lutringer, A. (2003). Stable isotopes in deep-sea corals and a new mechanism for “vital effects”. Geochim. Cosmochim. Acta 67, 1129–1143. doi: 10.1016/S0016-7037(02)01203-6
Airi, V., Gizzi, F., Falini, G., Levy, O., Dubinsky, Z., and Goffredo, S. (2014). Reproductive efficiency of a Mediterranean endemic Zooxanthellate coral decreases with increasing temperature along a wide latitudinal gradient. PLoS One 9:e91792. doi: 10.1371/journal.pone.0091792
Al-Horani, F. A. (2005). Effects of changing seawater temperature on photosynthesis and calcification in the scleractinian coral Galaxea fascicularis, measured with O2, Ca2+ and pH micro-sensors. Sci. Mar. 69, 347–354. doi: 10.3989/scimar.2005.69n3347
Al-Horani, F. A., Al-Moghrabi, S. M., and De Beer, D. (2003). The mechanism of calcification and its relation to photosynthesis and respiration in the scleractinian coral Galaxea fascicularis. Mar. Biol. 142, 419–426. doi: 10.1007/s00227-002-0981-8
Allemand, D., Ferrier-Pagès, C., Furla, P., Houlbrèque, F., Puverel, S., Reynaud, S., et al. (2004). Biomineralisation in reef-building corals: from molecular mechanisms to environmental control. C. R. Palevol 3, 453–467. doi: 10.1016/j.crpv.2004.07.011
Al-Rousan, S., Al-Moghrabi, S., Patzold, J., and Wefer, G. (2003). Stable oxygen isotopes in Porites corals monitor weekly temperature variations in the northern Gulf of Aqaba. Red Sea. Coral Reefs 22, 346–356. doi: 10.1007/s00338-003-0321-6
Altman, D. G. (1991). Practical Statistics for Medical Research. New York, NY: Chapman & Hall, CRC, 624.
Asami, R., Yamada, T., Iryu, Y., Meyer, C. P., Quinn, T. M., and Paulay, G. (2004). Carbon and oxygen isotopic composition of a Guam coral and their relationships to environmental variables in the western Pacific. Paleogeogr. Paleoclimatol. Paleoecol. 212, 1–22. doi: 10.1016/j.palaeo.2004.05.014
Böhm, F., Joachimski, M. M., Dullo, W. C., Eisenhauer, A., Lehnert, H., Reitner, J., et al. (2000). Oxygen isotope fractionation in marine aragonite of coralline sponges. Geochim. Cosmochim. Acta 64, 1695–1703. doi: 10.1016/S0016-7037(99)00408-1
Caroselli, E., Brambilla, V., Ricci, F., Mattioli, G., Levy, O., Falini, G., et al. (2016a). Inferred calcification rate of a temperate aZooxanthellate caryophylliid coral along a wide latitudinal gradient. Coral Reefs 35, 919–928. doi: 10.1007/s00338-016-1422-3
Caroselli, E., Ricci, F., Brambilla, V., Mattioli, G., Levy, O., Falini, G., et al. (2016b). Relationships between growth, population dynamics, and environmental parameters in the solitary non-Zooxanthellate scleractinian coral Caryophyllia inornata along a latitudinal gradient in the Mediterranean Sea. Coral Reefs 35, 507–519. doi: 10.1007/s00338-015-1393-9
Caroselli, E., Falini, G., Goffredo, S., Dubinsky, Z., and Levy, O. (2015). Negative response of photosynthesis to natural and projected high seawater temperatures estimated by pulse amplitude modulation fluorometry in a temperate coral. Front. Physiol. 6:317. doi: 10.3389/fphys.2015.00317
Caroselli, E., Mattioli, G., Levy, O., Falini, G., Dubinsky, Z., and Goffredo, S. (2012a). Inferred calcification rate of a Mediterranean aZooxanthellate coral is uncoupled with sea surface temperature along an 8° latitudinal gradient. Front. Zool. 9:32. doi: 10.1186/1742-9994-9-32
Caroselli, E., Zaccanti, F., Mattioli, G., Falini, G., Levy, O., Dubinsky, Z., et al. (2012b). Growth and demography of the solitary scleractinian coral Leptopsammia pruvoti along a sea surface temperature gradient in the Mediterranean Sea. PLoS One 7:e37848. doi: 10.1371/journal.pone.0037848
Caroselli, E., Prada, F., Pasquini, L., Nonnis Marzano, F., Zaccanti, F., Falini, G., et al. (2011). Environmental implications of skeletal micro-density and porosity variation in two scleractinian corals. Zoology 114, 255–264. doi: 10.1016/j.zool.2011.04.003
Carricart-Ganivet, J. P. (2004). Sea surface temperature and the growth of the west Atlantic reef-building coral Montastraea annularis. J. Exp. Mar. Biol. Ecol. 302, 249–260. doi: 10.1016/j.jembe.2003.10.015
Chen, S., Gagnon, A. C., and Adkins, J. F. (2018). Carbonic anhydrase, coral calcification and a new model of stable isotope vital effects. Geochim. Cosmochim. Acta. 236, 179–197. doi: 10.1016/j.gca.2018.02.032
Cohen, A. L., Gaetani, G. A., Lundälv, T., Corliss, B. H., and George, R. Y. (2006). Compositional variability in a cold-water scleractinian, Lophelia pertusa: new insights into “vital effects”. Geochem. Geophys. Geosyst. 7:Q12004. doi: 10.1029/2006GC001354
Cohen, A. L., Layne, G. D., Hart, S. R., and Lobel, P. S. (2001). Kinetic control of skeletal Sr/Ca in a symbiotic coral: implications for the paleotemperature proxy. Paleoceanography 16, 20–26. doi: 10.1029/1999PA000478
Cohen, A. L., and McConnaughey, T. A. (2003). Geochemical perspectives on coral mineralization. Rev. Mineral Geochem. 54, 151–187. doi: 10.2113/0540151
CorreÌge, T. (2006). Sea surface temperature and salinity reconstruction from coral geochemical tracers. Palaeogeogr. Palaeoclimatol. Palaeoecol. 232, 408–428. doi: 10.1016/j.palaeo.2005.10.014
Cortese, G., and De Domenico, E. (1990). Some considerations on the levantine intermediate water distribution in the Straits of Messina. Boll. Ocean. Teor. Appl. 8, 197–207.
Craig, H. (1961). Isotopic variations in meteoric waters. Science 133, 1702–1703. doi: 10.1126/science.133.3465.1702
Cullen, J. J., and Neale, P. J. (1994). Ultraviolet radiation, ozone depletion, and marine photosynthesis. Photosyn. Res. 39, 303–320. doi: 10.1007/BF00014589
D’Ortenzio, F., and Ribera d’Alcalà, M. (2009). On the trophic regimes of the Mediterranean sea: a satellite analysis. Biogeosciences 6, 139–148. doi: 10.5194/bg-6-139-2009
Epstein, S., Buchsbaum, R., Lowenstam, H. A., and Urey, H. C. (1951). Carbonate-water isotopic temperature scale. Bull. Geol. Soc. Am. 62, 417–426.
Epstein, S., Buchsbaum, R., Lowenstam, H. A., and Urey, H. C. (1953). Revised carbonate water isotopic temperature scale. Bull. Geol. Soc. Am. 64, 1315–1325.
Erez, J. (1978). Vital effect on stable-isotope composition seen in foraminifera and coral skeletons. Nature 273, 199–202. doi: 10.1038/273199a0
Fantazzini, P., Mengoli, S., Evangelisti, S., Pasquini, L., Mariani, M., Brizi, L., et al. (2013). A time-domain nuclear magnetic resonance study of Mediterranean scleractinian corals reveals skeletal-porosity sensitivity to environmental changes. Environ. Sci. Technol. 47, 12679–12686. doi: 10.1021/es402521b
Felis, T., Pätzold, J., and Loya, Y. (2003). Mean oxygen-isotope signatures in Porites spp. corals: inter-colony variability and correction for extension-rate effects. Coral Reefs 22, 328–336. doi: 10.1007/s00338-003-0324-3
Ferrier-Pagès, C., Reynaud, S., and Allemand, D. (2012). “Shallow water scleractinian corals of the Mediterranean Sea,” in Life in the Mediterranean Sea: a Look at Habitat Changes, ed. N. Stambler (Hauppauge, NY: Nova Sciences Publishers, Inc), 387–422.
Furla, P., Bénazet-Tambutté, S., Jaubert, J., and Allemand, D. (1998). Diffusional permeability of dissolved inorganic carbon through the isolated oral epithelial layers of the sea anemone, Anemonia viridis. J. Exp. Mar. Biol. Ecol. 221, 71–88. doi: 10.1016/S0022-0981(97)00116-0
Furla, P., Galgani, I., and Durand, I. (2000). Sources and mechanisms of inorganic carbon transport for coral calcification and photosynthesis. J. Exp. Biol. 203, 3445–3457.
Gabriel, K. R., and Lachenbruch, P. A. (1969). Non-parametric ANOVA in small samples: a monte carlo study of the adequacy of the asymptotic approximation. Biometrics 25, 593–596. doi: 10.2307/2528915
Gagan, M. K., Ayliffe, L. K., Beck, J. W., Cole, J. E., Druffel, E. R. M., Dunbar, R. B., et al. (2000). New views of tropical paleoclimates from corals. Quat. Science Rev. 19, 45–64. doi: 10.1016/S0277-3791(99)00054-2
Gat, J. R., Shemesh, A., Tziperman, E., Hecht, A., Georgopoulos, D., and Basturk, O. (1996). The stable isotope composition of waters of the eastern Mediterranean sea. J. Geophys. Res. 101, 6441–6451. doi: 10.1029/95jc02829
Gattuso, J.-P., Frankignoulle, M., and Smith, S. V. (1999). Measurement of community metabolism and significance in the coral reef CO2 source-sink debate. Proc. Natl. Acad. Sci. U.S.A. 96, 13017–13022. doi: 10.1073/pnas.96.23.13017
Goffredo, S., Caroselli, E., Mattioli, G., Pignotti, E., Dubinsky, Z., and Zaccanti, F. (2009). Inferred level of calcification decreases along an increasing temperature gradient in a Mediterranean endemic coral. Limnol. Oceanogr. 54, 930–937. doi: 10.4319/lo.2009.54.3.0930
Goffredo, S., Caroselli, E., Mattioli, G., Pignotti, E., and Zaccanti, F. (2008). Relationships between growth, population structure and sea surface temperature in the temperate solitary coral Balanophyllia europaea (Scleractinia, Dendrophylliidae). Coral Reefs 27, 623–632. doi: 10.1007/s00338-008-0362-y
Goffredo, S., Caroselli, E., Pignotti, E., Mattioli, G., and Zaccanti, F. (2007). Variation in biometry and population density of solitary corals with environmental factors in the Mediterranean Sea. Mar. Biol. 152, 351–361. doi: 10.1007/s00227-007-0695-z
Goffredo, S., Mancuso, A., Caroselli, E., Prada, F., Dubinsky, Z., Falini, G., et al. (2015). Skeletal mechanical properties of Mediterranean corals along a wide latitudinal gradient. Coral Reefs 34, 121–132. doi: 10.1007/s00338-014-1222-6
Goreau, T. J. (1977a). “Carbon metabolism in calcifying and photosynthetic organisms: theoretical models based on stable isotope data,” in Proceedings of the 3rd International Coral Reef Symposium, Vol. 2, (Miami), 395–401.
Goreau, T. J. (1977b). Coral skeletal chemistry: physiological and environmental regulation of stable isotopes and trace elements in Montastrea annularis. Proc. Roy. Soc. Lond. B 196, 291–315. doi: 10.1098/rspb.1977.0042
Grossman, E. L., and Ku, T.-L. (1986). Oxygen and carbon isotope fractionation in biogenic aragonite: temperature effects. Chem. Geol. 59, 59–74. doi: 10.1016/0168-9622(86)90057-6
Grottoli, A. G. (2002). Effect of light and brine shrimp levels on skeletal d13C values in the Hawaiian coral Porites compressa: a tank experiment. Geochim. Cosmochim. Acta 66, 1955–1967. doi: 10.1016/S0016-7037(01)00901-2
Grottoli, A. G., and Wellington, G. M. (1999). Effect of light and zooplankton on skeletal d13C values in the eastern pacific corals Pavona clavus and Pavona gigantea. Coral Reefs 18, 29–41. doi: 10.1007/s003380050150
Heikoop, J. M., Dunn, J. J., Risk, M. J., Schwarcz, H. P., McConnaughey, T. A., and Sandeman, I. M. (2000). Separation of kinetic and metabolic isotope effects in carbon-13 records preserved in reef coral skeletons. Geochim. et Cosmochim. Acta 64, 975–987. doi: 10.1016/S0016-7037(99)00363-4
Iluz, D., and Dubinsky, Z. (2015). Coral photobiology: new light on old views. Zoology 118, 71–78. doi: 10.1016/j.zool.2014.08.003
Juillet-Leclerc, A., Rollion-Bard, C., Reynaud, S., and Ferrier-Pagès, C. (2018). A new paradigm for δ18O in coral skeleton oxygen isotope fractionation response to biological kinetic effects. Chem. Geol. 483, 131–140. doi: 10.1016/j.chemgeo.2018.02.035
Kružiæ, P., Sršen, P., and Benkoviæ, L. (2012). The impact of seawater temperature on coral growth parameters of the colonial coral Cladocora caespitosa (Anthozoa, Scleractinia) in the eastern Adriatic sea. Facies 58, 477–491. doi: 10.1007/s10347-012-0306-4
Kuhnert, H., Pätzold, J., Wyrwoll, K. H., and Wefer, G. (2000). Monitoring climate variability over the past 116 years in coral oxygen isotopes from Ningaloo reef, Western Australia. Int. J. Earth Sci. 88, 725–732. doi: 10.1007/s005310050300
Land, L. S., Lang, J. C., and Barnes, D. J. (1975). Extension rate: a primary control on the isotopic composition of west Indian (Jamaican) scleractinian reef coral skeletons. Mar. Biol. 33, 221–233. doi: 10.1007/BF00390926
Levy, O., Rosenfeld, M., Yam, R., and Shemesh, A. (2006). Heterogeneity of coral skeletons isotopic compositions during the 1998 bleaching event. Limnol. Oceanogr. 51, 1142–1148. doi: 10.4319/lo.2006.51.2.1142
Linsley, B. K., Kaplan, A., Gouriou, Y., Salinger, J., deMenocal, P. B., Wellington, G. M., et al. (2006). Tracking the extent of the south pacific convergence zone since the early 1600s. Geochem. Geophys. Geosyst. 7:Q05003. doi: 10.1029/2005GC001115
López Correa, M., Montagna, P., Vendrell-Simón, B., McCulloch, M., and Taviani, M. (2010). Stable isotopes (δ18O and δ13C), trace and minor element compositions of recent scleractinians and last glacial bivalves at the santa maria di leuca deep-water coral province, Ionian Sea. Deep Sea Res. Part II Top. Stud. Oceanogr. 57, 471–486. doi: 10.1016/j.dsr2.2009.08.016
Lough, J. M., and Barnes, D. J. (2000). Environmental controls on growth of the massive coral Porites. J. Exp. Mar. Biol. Ecol. 245, 225–243. doi: 10.1016/S0022-0981(99)00168-9
McConnaughey, T. (1989a). 13C and 18O isotopic disequilibrium biological carbonates: I. Patterns. Geochim. Cosmochim. Acta 53, 151–162. doi: 10.1016/0016-7037(89)90282-2
McConnaughey, T. (1989b). 13C and 18O isotopic disequilibrium biological carbonates: II In vitro simulation of kinetic isotope effects. Geochim. Cosmochim. Acta 53, 163–171. doi: 10.1016/0016-7037(89)90283-4
McConnaughey, T. A. (2003). Sub-equilibrium oxygen-18 and carbon-13 levels in biological carbonates: carbonate and kinetic models. Coral Reefs 22, 316–327. doi: 10.1007/s00338-003-0325-2
McConnaughey, T. A., Burdett, J., Whelan, J. F., and Paull, C. K. (1997). Carbon isotopes in biological carbonates: Respiration and photosynthesis. Geochim. Cosmochim. Acta. 61, 611–622. doi: 10.1016/S0016-7037(96)00361-4
McCulloch, M., Trotter, J., Montagna, P., Falter, J., Dunbar, R., Freiwald, A., et al. (2012). Resilience of cold-water scleractinian corals to ocean acidification: boron isotopic systematics of pH and saturation state up-regulation. Geochim. Cosmochim. Acta 87, 21–34. doi: 10.1016/j.gca.2012.03.027
McCulloch, M. T., Tudhope, A. W., Esat, T. M., Mortimer, G. E., Chappell, J., Pillans, B., et al. (1999). Coral record of equatorial sea-surface temperatures during the penultimate deglaciation at Huon Peninsula. Science 283, 202–204. doi: 10.1126/science.283.5399.202
Meibom, A., Cuif, J., Hillion, F., Constantz, B. R., Juillet-Leclerc, A., Dauphin, Y., et al. (2004). Distribution of magnesium in coral skeleton. Geophys. Res. Lett. 31:L23306. doi: 10.1029/2004GL021313
Meibom, A., Mostefaoui, S., Cuif, J. P., Dauphin, Y., Houlbrèque, F., Dunbar, R., et al. (2007). Biological forcing controls the chemistry of reef-building coral skeleton. Geophys. Res. Lett. 34:L02601. doi: 10.1029/2006GL028657
Meibom, A., Stage, M., Wooden, J., Constantz, B. R., Dunbar, R. B., Owen, A., et al. (2003). Monthly Strontium/Calcium oscillations in symbiotic coral aragonite: biological effects limiting the precision of the paleotemperature proxy. Geophys. Res. Lett. 30:1418. doi: 10.1029/2002GL016864
Montagna, P., Silenzi, S., Devoti, S., Mazzoli, C., McCulloch, M., Scicchitano, G., et al. (2008). Climate reconstructions and monitoring in the Mediterranean sea: a review on some recently discovered high-resolution marine archives. Rend. Lincei Sci. Fis. Nat. 19, 121–140. doi: 10.1007/s12210-008-0007-7
Paul, H. A., Bernasconi, S. M., Schmid, D. W., and McKenzie, J. A. (2001). Oxygen isotopic composition of the Mediterranean sea since the last glacial maximum: constraints from pore water analyses. Earth Planet. Sci. Lett. 192, 1–14. doi: 10.1016/s0012-821x(01)00437-x
Peirano, A., Morri, C., and Bianchi, C. N. (1999). Skeleton growth and density pattern of the temperate, zooxanthellate scleractinian Cladocora caespitosa from the Ligurian sea (NW Mediterranean). Mar. Ecol. Prog. Ser. 185, 195–201. doi: 10.3354/meps185195
Peirano, A., Morri, C., Bianchi, C. N., Aguirre, J., Antonioli, F., Calzetta, G., et al. (2004). The Mediterranean coral Cladocora caespitosa: a proxy for past climate fluctuations? Glob. Planet. Change 40, 195–200. doi: 10.1016/S0921-8181(03)00110-3
Pierre, C., Vergnaud-Grazzini, C., Thouron, D., and Saliege, J. F. (1986). Compositions isotopiques de l’oxygène et du carbone des masses d’eau en Méditerranée. Mem. Soc. Geol. Ital 36, 165–174.
Potvin, C., and Roff, D. A. (1993). Distribution-free and robust statistical methods: viable alternatives to parametric statistics? Ecology 74, 1617–1628. doi: 10.2307/1939920
Rodolfo-Metalpa, R., Richard, C., Allemand, D., and Ferrier-Pagès, C. (2006). Growth and photosynthesis of two Mediterranean corals, Cladocora caespitosa and Oculina patagonica, under normal and elevated temperatures. J. Exp. Biol. 209, 4546–4556. doi: 10.1242/jeb.02550
Rodrigues, L. J., and Grottoli, A. G. (2006). Calcification rate and the stable carbon, oxygen, and nitrogen isotopes in the skeleton, host tissue, and Zooxanthellae of bleached and recovering Hawaiian corals. Geochim. Cosmochim. Acta 70, 2781–2789. doi: 10.1016/j.gca.2006.02.014
Rollion-Bard, C., Chaussidon, M., and France-Lanord, C. (2003). pH control on oxygen isotopic composition of symbiotic corals. Earth Planet. Sci. Lett. 215, 275–288. doi: 10.1016/S0012-821X(03)00391-1
Romanek, C. S., Grossman, E. L., and Morse, J. W. (1992). Carbon isotopic fractionation in synthetic aragonite and calcite: effects of temperature and precipitation rate. Geochim. Cosmochim. Acta 56, 419–430. doi: 10.1016/0016-7037(92)90142-6
Sakalli, A. (2017). Sea surface temperature change in the Mediterranean sea under climate change: a linear model for simulation of the sea surface temperature up to 2100. Appl. Ecol. Environ. Res 15, 707–716. doi: 10.15666/aeer/1501_707716
Schoepf, V., Levas, S. J., Rodrigues, L. J., McBride, M. O., Aschaffenburg, M. D., Matsui, Y., et al. (2014). Kinetic and metabolic isotope effects in coral skeletal carbon isotopes: a re-evaluation using experimental coral bleaching as a case study. Geochim. et Cosmochim. Acta 146, 164–178. doi: 10.1016/j.gca.2014.09.033
Senchaudhuri, P., Mehta, C. R., and Patel, N. R. (1995). Estimating exact p-values by the method of control variates, or Monte Carlo rescue. J. Am. Stat. Assoc. 90, 640–648. doi: 10.1080/01621459.1995.10476558
Silenzi, S., Bard, E., Montagna, P., and Antonioli, F. (2005). Isotopic and elemental records in a non-tropical coral (Cladocora caespitosa): discovery of a new high-resolution climate archive for the Mediterranean sea. Glob. Planet. Change 49, 94–120. doi: 10.1016/j.gloplacha.2005.05.005
Smith, J. E., Schwarcz, H. P., Risk, M. J., McConnaughey, T., and Keller, N. (2000). Paleotemperatures from deep-sea corals: overcoming ‘vital effects’. Palaios 15, 25–32. doi: 10.1669/0883-1351(2000)015<0025:pfdsco>2.0.co;2
Sournia, A. (1973). La production primaire planctonique en Mediterranee: essai de mise a jour. Bull. Et. Comm. Medit. Special Issue 5, 128.
Sueltemeyer, D., and Rinast, K.-A. (1996). The CO2 permeability of the plasma membrane of Chlamydomonas reinhardtii: mass-spectrometric 18O-exchange measurements from 13 C18 O2 in suspensions of carbonic anhydrase-loaded plasma-membrane vesicles. Planta 200, 358–368.
Swart, P. K. (1983). Carbon and oxygen isotope fractionation in scleractinian corals: a review. Earth Sci. Rev. 19, 51–80. doi: 10.1016/0012-8252(83)90076-4
Trotter, J. A., Montagna, P., McCulloch, M. T., Silenzi, S., Reynaud, S., Mortimer, G., et al. (2011). Quantifying the pH ‘vital effect’ in the temperate Zooxanthellate coral Cladocora caespitosa: validation of the boron seawater pH proxy. Earth Planet. Sci. Lett. 303, 163–173. doi: 10.1016/j.epsl.2011.01.030
Urey, H. C., Lowenstam, H. A., Epstein, S., and McKinney, C. R. (1951). Measurement of paleotemperatures and temperatures of the upper cretaceous of England, Denmark, and the southeastern United States. Bull. Geol. Soc. Am. 62, 399–416.
Vidal-Dupiol, J., Zoccola, D., Tambutté, E., Grunau, C., Cosseau, C., Smith, K. M., et al. (2013). Genes related to ion-transport and energy production are upregulated in response to CO2-driven pH decrease in corals: new insights from transcriptome analysis. PloS One 8:e58652. doi: 10.1371/journal.pone.0058652
Vongsavat, V., Winotai, P., and Meejoo, S. (2006). Phase transitions of natural corals monitored by ESR spectroscopy. Nucl. Instr. Meth. Phys. Res. B 243, 167–173. doi: 10.1016/j.nimb.2005.07.197
Weber, J. M., Deines, P., Weber, P. H., and Baker, P. A. (1976). Depth-related changes in the 13C/12C ratio of coral carbonate deposited by the Caribbean reef-frame-building coral Montastrea annularis: further implications of a model for stable isotope fractionation by scleractinian corals. Geochim. Cosmochim. Acta 40, 31–39. doi: 10.1016/0016-7037(76)90191-5
Weber, J. N. (1974). 13C/12C ratios as natural isotopic tracers elucidating calcification processes in reef-building and non-reef-building corals. Proc. Second. Intl. Coral Reef Symp. 2, 289–298.
Weber, J. N., and Woodhead, P. M. (1972). Temperature dependence of oxygen-18 concentration in reef coral carbonates. J. Geophys. Res. 77, 463–473. doi: 10.1029/JC077i003p00463
Weber, J. N., and Woodhead, P. M. J. (1970). Carbon and oxygen isotope fractionation in the skeletal carbonate of reef-building corals. Chem. Geol. 6, 93–117. doi: 10.1016/0009-2541(70)90009-4
Wilbur, K. M., and Simkiss, K. (1979). Chapter 2.3 carbonate turnover and deposition by Metazoa. Stud. Environ. Sci. 3, 69–106. doi: 10.1016/S0166-1116(08)71055-0
Keywords: stable isotopes, vital effects, kinetic isotope effects, isotopic discrimination, Mediterranean Sea, temperate corals
Citation: Prada F, Yam R, Levy O, Caroselli E, Falini G, Dubinsky Z, Goffredo S and Shemesh A (2019) Kinetic and Metabolic Isotope Effects in Zooxanthellate and Non-zooxanthellate Mediterranean Corals Along a Wide Latitudinal Gradient. Front. Mar. Sci. 6:522. doi: 10.3389/fmars.2019.00522
Received: 26 March 2019; Accepted: 12 August 2019;
Published: 10 September 2019.
Edited by:
Benoit Thibodeau, The University of Hong Kong, Hong KongReviewed by:
Verena Schoepf, The University of Western Australia, AustraliaCopyright © 2019 Prada, Yam, Levy, Caroselli, Falini, Dubinsky, Goffredo and Shemesh. This is an open-access article distributed under the terms of the Creative Commons Attribution License (CC BY). The use, distribution or reproduction in other forums is permitted, provided the original author(s) and the copyright owner(s) are credited and that the original publication in this journal is cited, in accordance with accepted academic practice. No use, distribution or reproduction is permitted which does not comply with these terms.
*Correspondence: Stefano Goffredo, cy5nb2ZmcmVkb0B1bmliby5pdA==; Aldo Shemesh, QWxkby5TaGVtZXNoQHdlaXptYW5uLmFjLmls
†These authors have contributed equally to this work
Disclaimer: All claims expressed in this article are solely those of the authors and do not necessarily represent those of their affiliated organizations, or those of the publisher, the editors and the reviewers. Any product that may be evaluated in this article or claim that may be made by its manufacturer is not guaranteed or endorsed by the publisher.
Research integrity at Frontiers
Learn more about the work of our research integrity team to safeguard the quality of each article we publish.