- 1CSIRO Data61, Hobart, TAS, Australia
- 2National Institute of Water and Atmospheric Research Ltd., Riccarton, New Zealand
- 3CSIRO Data61, Canberra, ACT, Australia
Ships have been translocating species around the world for hundreds of years but attempts to understand and manage this issue date back only three decades. Here we review the assessment and management of risks from vessel biofouling and ballast water over this time period from an Australian and New Zealand perspective. We detail a history of successes and failures at the science-policy interface that include international guidelines for biofouling management and the recent ratification of a ballast water convention. We summarize the efficacy and costs of current treatment options, and highlight the practical challenges and policy implications of managing the diffuse and succinct bio-invasion risks that shipping creates pre- and post-border. We then use the lessons learnt over the last 30 years to recommend a future empirical strategy.
Introduction
The role of ballast and biofouling as vectors for the translocation of species globally has been known for over 60 years (Elton, 1958; Medcof, 1975). The magnitude of the consequences for human health, the economy and environment, however, was not widely publicized until the mid-1980s (Carlton, 1985; William et al., 1988). At this time, and in the decade that followed, the gravity of the problem was underlined by the introduction of Cholera to Peru (McCarthy and Khambaty, 1994), the global increase in the frequency, intensity and distribution of paralytic shellfish poisoning (Hallegraeff and Bolch, 1992), the role of the Atlantic comb jelly Mnemiopsis leidyi in the collapse of the Black Sea ecosystem (Shiganova, 1998), the economic and environmental impacts of the zebra mussel Dreissena polymorpha (Holland, 1993), and a growing awareness that the number of species being translocated around the globe was much larger than previously realized (Carlton, 1992).
The international community was initially quick to respond to the marine pest threat. In 1991 the Marine Environment Protection Committee (MEPC), a subcommittee of the International Maritime Organization (IMO), adopted the International Guidelines for Preventing the Introduction of Unwanted Aquatic Organisms and Pathogens from Ships Ballast Water and Sediment Discharges (Figure 1). Progress after that was significantly slower. The MEPC guidelines began a series of international efforts to manage ballast water. It took 13 years, however, before the International Convention for the Control and Management of Ships’ Ballast Water and Sediments was adopted by the IMO in 2004, and another 13 years before the convention was ratified in September 20171.
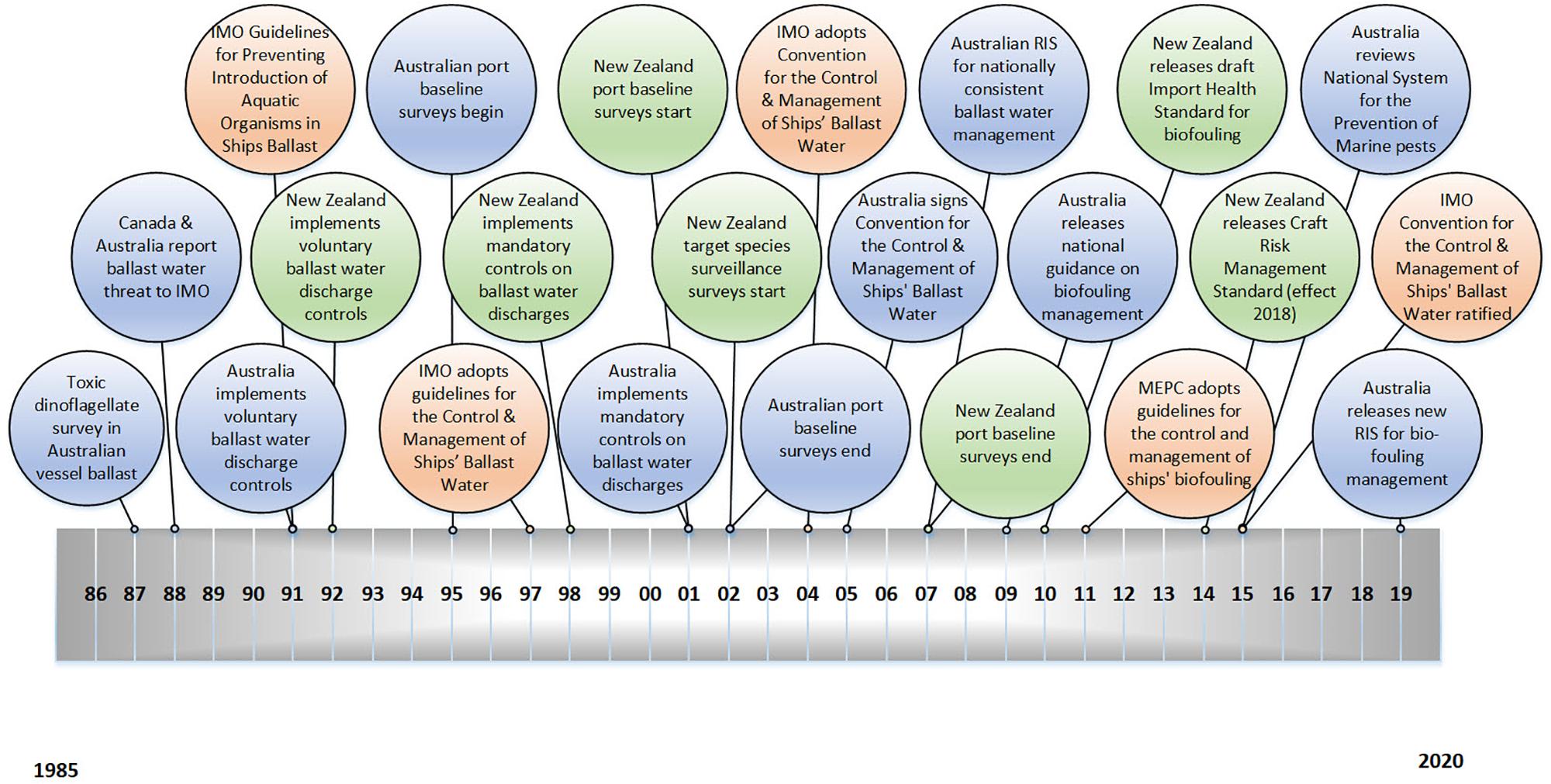
Figure 1. Timeline of major international (orange), Australian (blue) and New Zealand (green) management actions associated with marine pest introductions by ballast water and hull fouling, from 1985 to the present day.
Policies that seek reasonable assurance of a net benefit must balance the often readily quantifiable costs of management against the more uncertain economic, environmental and health benefits. Scientific risk assessment is often used to help find this balance. Whilst some cost-benefit calculations for ballast water management suggested clear net benefits (World Wildlife Fund, 2009), uncertainty around this process contributed to the delay between recognizing the ballast water threat and managing it.
The principle of risk versus return is implicit in the ballast water convention. A key component is regulation A-4 which lays out the nature of exemptions that can be given to vessels. These require the application of defined risk analysis methods which are described in Guideline G7, significant elements of which reflect thinking and approaches that were originally developed in Australia and New Zealand. Both countries were leaders in introducing controls to manage the risks posed by introduced marine species. This was supported by their well-developed terrestrial biosecurity systems and island status which reduces certain complications faced by other jurisdictions.
The recent ratification, and entry into force, of the convention provides an impetus to review current knowledge around the risk-based management of marine pests. In the 15 years since the convention was adopted, Australian and New Zealand authorities have gained significant practical experience in applying different risk assessment techniques, and theoretical developments in estimating risk have taken place. This paper performs that review. It considers risk management for threats posed by both hull fouling and ballast water and for completeness considers the risk-based management of both domestic and international ballast water. We first review the Australian and New Zealand experience of using risk-based approaches, and then propose a conceptualization of the problem that is consistent with the convention but respects the relevant uncertainties and knowledge. We close by discussing the implications of this conceptualization and recommend future research directions.
Risk Assessment and Management
Ballast Water
At the outset of the marine pest issue, scientists and regulators naturally looked to existing analogs for guidance on how risk assessment for marine pest introductions might be conducted. Many analysts (including ourselves) turned to three situations that, at least initially, looked analogous to the problem of risk assessment for marine pest incursions via ballast water and biofouling: (i) assessing the pre-border risk posed by accidental introductions of pests of agricultural production systems, including aquaculture; (ii) the risk of spread and impacts following post-border outbreaks of pests in these systems; and (iii) pre-border assessments of the incidental risks posed by deliberate introductions of biocontrol agents.
With exception of biocontrol agents, where assessment requirements and guidelines were developed relatively late (Barratt et al., 2010), risk guidelines and procedures were well established for each of the other situations, and this material often carried the imprimatur of respected national and international organizations (Kohler and Stanley, 1984; Kellar, 1993; Morley, 1993; Office International des Epizooties, 1996; Food and Agricultural Organization, 2006).
A common feature of these guidelines and procedures was that each adopted a species-specific perspective to the risk assessment problem, advocating that each assessment be treated on a case-by-case basis, using the characteristics of the species and receiving environment to guide the assessment process. Unsurprisingly, species-specific approaches for risk assessment of pests associated with biofouling and ballast water were identified as a possible approach to vector management, in-keeping with international expectations for trade.
There were, nevertheless, some misgivings about this approach at the time. Public submissions to a 1996 New Zealand government discussion paper on proposed regulatory approaches for the management of ballast water and biofouling highlighted the lack of detailed information available to underpin species-specific assessments, including on the species likely to be transported into New Zealand, the prospects for their establishment and likely consequences (New Zealand Government, 1998).
In the absence of this information, the Government supported a precautionary approach which assumed that all ballast discharges from vessels entering New Zealand could contain unwanted species, and in May 1998, New Zealand was among the first countries to implement mandatory requirements for ballast water management that reflected the three management actions – mid-ocean exchange preferably 200 nautical miles from land and in water over 200 m deep, onboard treatment, or discharge to an on-shore facility – identified in the 1991 IMO guidelines, without any provisions for exemption based on risk assessment (McConnell, 2002). Australia implemented similar mandatory requirements for international ballast water in July 2001 (Figure 1), but also sought to offer exemptions based on a species-specific, risk-based, Decision Support System, supported by a target-species list and port baseline surveys (McConnell, 2002; Hayes and Sliwa, 2003; Campbell et al., 2007) due to concerns about the feasibility and cost of mid-ocean exchange, particularly for domestic voyages between Australian ports.
Species-specific methods were not the only approach advocated for biofouling and ballast water risk assessment. Carlton et al. (1995) raised the notion that environmental distance could act as a proxy for probability of survival, suggesting that translocations of ballast water between saline and fresh water, and between polar and tropical, environments would be safe because of the severe environmental dissimilarity between source and recipient regions. Hilliard and Raaymakers (1997) extended this idea and proposed a method of assessing ballast water risks based almost entirely on the environmental distance between the source and recipient ports measured with 37 variables, rather than just temperature and salinity.
Considerations and recommendations for how to conduct risk assessments using both species-specific approaches and environmental matching, for journeys within and between bioregions, were eventually published by Barry et al. (2008). Early drafts of this document were shared with members of some of the national delegations to the MEPC, and the overall approach and recommendations were taken up in the IMO G7 Guidelines for ballast water risk assessment (Marine Environment Protection Committee [MEPC], 2007). The guidelines provided for three types of assessments – environmental matching, species-specific and species’ biogeography – and were subsequently revised in 2017 to include the concept of “Same Risk Area” to recognize the possibility of species-specific, low risk scenarios where target species are already present in all ports within an area or had a high probability of establishing in all locations via natural dispersal (Marine Environment Protection Committee [MEPC], 2017).
Importantly, when the IMO guidelines were released only one (mid-ocean exchange) of the three management options identified by the guidelines was practically available because no shipboard treatments were available globally and no shore-based reception facilities were approved in either Australia or New Zealand. There was therefore some trepidation among Australian and New Zealand officials at this time that each country’s trading partners might not accept blanket regulation on ballast water discharges because of the possible ramifications for trade (New Zealand Government, 1998). Many other countries, however, adopted similar regulations (Bailey, 2015) and mid-ocean exchange quickly became standard practice, routinely performed by international vessels whenever journey conditions permitted. According to information provided to the Australian Decision Support System, for example, virtually all international vessels arriving in Australia were able to complete mid-ocean exchange in accordance with the guidelines.
A strong rationale for risk-based exemptions from the blanket-management described in the guidelines remained, however, for vessels undertaking short coastal journeys between ports within the same nation, or in other regions such as the North Sea and Baltic Sea, where it was not possible to meet the D-1 performance standard because there was not enough time, depth or distance to perform 95% volumetric exchange of ballast in water 200 m deep and/or more than 200 nautical miles from shore (Behrens et al., 2005; David et al., 2013). In Australia, target species risk-based management shifted focus to domestic ballast water transfers, culminating in the Australian Domestic Ballast Water Risk Assessment tool2.
Biofouling
Biofouling threats were originally conceived as arising primarily from fouling organisms that accumulated over time on a vessel’s hull. Biofouling increases frictional drag which adversely impacts the vessel’s performance (Schultz et al., 2011), hence it is standard practice for marine coatings to be applied to vessel hulls at intervals of between 18 months to 5 years to prevent corrosion and retard biofouling growth (Almeida et al., 2007). Coatings are tailored to the operational profiles of different vessel types to maximize performance and to extend the time the vessel can remain in the water.
Empirical analysis of vessel biofouling confirms that the amount of fouling material on submerged surfaces is correlated to the number of days since the vessel was last cleaned and anti-fouled and the pattern of use of the vessel, including how often it is used and any significant periods of lay-up, but the effect of the last two factors may vary according to vessel type, the type of anti-fouling paint used, and how good the coating application was (Floerl et al., 2005; Davidson et al., 2009; Inglis et al., 2010; Lacoursière-Roussel et al., 2012; Lane et al., 2019).
Biofouling on the hulls of commercial and recreational vessels can be managed if owners apply anti-fouling coatings that suit their vessel’s operational profile, and perform regular maintenance in accordance with the manufacturers specifications (Davidson et al., 2016). Biofouling, however, is not evenly distributed over the hull, but tends to be most abundant in areas where the anti-fouling coatings are damaged or degraded and in recesses that are protected from the drag created by the vessel moving through the water (“niche areas”) (Hayes, 2002). On modern merchant vessels, these niche areas can comprise up to 27% of the wetted surface area of a vessel (Moser et al., 2017) and contain >80% of the biofouling on a vessel (Coutts and Dodgshun, 2007; Inglis et al., 2010).
Concerns about vessel biofouling risks and the problem of niche area fouling were raised by Australia, New Zealand and Friends of the Earth International in 2005 at the 54th session of the IMO MEPC. In 2007, the MEPC approved the development of international measures for minimizing the transfer of invasive aquatic species through biofouling as a new high priority item in the work program of the Bulk Liquids and Gases Sub-Committee. The resulting Guidelines for the Control and Management of Ships’ Biofouling to Minimize the Transfer of Invasive Aquatic Species (Marine Environment Protection Committee [MEPC], 2011) were subsequently adopted by MEPC for commercial vessels in 2011 [MEPC.207(62)] and recreational craft in 2012 (MEPC.1/Circ.792).
The MEPC biofouling guidelines present best practice for choosing, applying and maintaining anti-fouling systems for vessels and include recommendations for regular in-water inspection and cleaning of problem areas, training and record keeping. Member States were requested to take urgent action to apply the guidelines and to report back to MEPC on experience gained through their implementation (MEPC.1/Circ.811). Although voluntary, the guidelines do not preclude individual States from applying other mandatory measures to provide additional protection from invasive biofouling species within their jurisdiction.
In 2004, the New Zealand government commissioned a multi-year research survey of over 500 international merchant, recreational, passenger, fishing, and slow-moving vessels to characterize the biofouling risks associated with arriving vessels (Inglis et al., 2010; Piola and Conwell, 2010). The survey showed that most vessels (>70%) from all the major types examined conveyed some biofouling into New Zealand on arrival. Over 65% of the 187 biofouling species identified in the study were non-indigenous to New Zealand and >70% of them had not yet established in New Zealand (Inglis et al., 2010).
This research was used, with other sources of information, to inform a qualitative import risk analysis of vessel biofouling (Bell et al., 2011), which eschewed a species-specific approach in favor of analysis of risks from 20 broad taxonomic groupings of biofouling organisms. This was due to: (i) the large number of species that had been recorded from studies of biofouling worldwide (>2000); (ii) the relatively poor quality of information available on their global distributions, ecology and potential impacts; and, (iii) the difficulty in predicting from this large species pool which species may be problematic. Twelve of the 20 taxonomic groups that were assessed contained species adjudged to present non-negligible risks to New Zealand’s marine ecosystems and for which risk management measures could be justified. This finding, and the need for a simple, streamlined clearance procedure for vessels at the border, meant that a precautionary approach was again proposed, with any macro-organisms found on the hull of an arriving vessel considered to be risk organisms (Bell et al., 2011).
Following the risk assessment recommendations, the New Zealand government initially proposed an Import Health Standard that required vessels arriving into New Zealand to meet a clean hull standard that was defined as having “no visible aquatic organisms on the hull, including niche areas, except as a slime layer”. The practicality of the clean hull standard, however, was subsequently questioned during consultation because even well-maintained vessels will accumulate some biofouling while operational (Inglis et al., 2010).
The Import Health Standard was revised and released in May 2014 as a Craft Risk Management Standard with an initial 4-year lead-in period before becoming mandatory in 2018 (Ministry for Primary Industries, 2018). The “Clean hull” requirement was altered to allow some macro-fouling with the amount depending on the intended length of stay within New Zealand waters. These allowances were explicitly described as biofouling thresholds within the standard. Vessels intending to remain in New Zealand for more than 21 days or which intended to visit areas not designated as “Places of First Arrival” are permitted to have no more than a slime layer and goose barnacles present on any area of the hull and niches. Vessels visiting Places of First Arrival and intending to remain in New Zealand for less than 21 days are permitted an additional allowance of some early-stage macro-fouling, specifically macroalgae, barnacles, tubeworms and bryozoans. The standard specifies thresholds for the maximum allowable size (in the case of macroalgae), cover and richness of these four taxonomic groups on the wind/water line, general hull area and niches, with justification for these thresholds described by Georgiades and Kluza (2017).
Subsequent debate about the Craft Risk Management Standard has been mostly directed at the biofouling thresholds and their practicality. This debate, however, overlooks the sections of the standard that outline a range of acceptable measures for meeting the “Clean hull” standard (section 2.3). These include continual maintenance of the vessel in accordance with the MEPC 2011 biofouling guidelines, cleaning within 30 days of arrival in New Zealand, the application of approved treatments or submission of a Craft Risk Management plan that outlines steps taken to reduce risk sufficiently to meet the standard. In practice most vessels resort to these other forms of acceptable evidence to demonstrate compliance with the standard rather than the biofouling thresholds.
In Australia progress on managing biofouling lagged behind ballast water because: (i) it was considered, at least initially, to be adequately managed in commercial vessels by extant management practices; (ii) at least partially managed in the recreational sector by extant practice, and; (iii) because the stakeholder groups in the recreational sector are more diffuse and therefore more difficult to represent within the biosecurity governance arrangements of the time.
In 2011, Hewitt et al. (2011) completed a qualitative assessment of the likelihood of entry, establishment and impacts of more than 1781 individual biofouling species. They then identified 56 Species of Concern that were not currently known to be present in Australia, had a high probability of arriving in Australian waters as biofouling on international vessels and had the potential to cause unacceptable impacts to environmental, economic, social/cultural or human health values. Western Australia and the Northern Territory had corresponding schedules listing Species of Concern that currently include 82 and 44 species, respectively, (Northern Territory of Australia, 2009).
The Commonwealth Government of Australia subsequently released a Regulatory Impact Statement for consultation (Price Waterhouse Coopers, 2011) that proposed new regulations, whereby commercial and recreational vessels would be assessed using an online tool to assess biofouling risk. Vessels assessed as “high” or “extreme” risk would be subject to restrictions on the time they could operate at any one port (48 h), at a series of ports (8 days total) or within Australian waters (14 days). If the vessel was unable to conduct its business within these restrictions it would be required either to leave Australian waters or be subject to a hull inspection to determine if any quarantine pests (Species of Concern) were present.
In 2015, a national review of arrangements for marine biosecurity highlighted significant concerns among stakeholders about the species-based risk assessment and approach to biofouling regulation. High among these concerns were the costs of developing and maintaining lists of Species of Concern, the evidential basis for assessing risk and the administrative burden to vessel operators of implementing this regime. The review recommended biofouling requirements for international vessels that were more closely aligned to the (Marine Environment Protection Committee [MEPC], 2011) guidelines and to the approaches taken by New Zealand and California. A new Regulatory Impact Statement, issued in April 2019, reflects this outcome. It proposes three regulatory options for consideration, with the preferred option being the requirement for vessels to implement vessel-specific biofouling management practices consistent with the Marine Environment Protection Committee 2011 guidelines (Australian Government Department of Agriculture and Water Resources, 2019). These practices require vessels to develop and maintain a Biofouling Management Plan and BioFouling Record Book, without which vessels will be targeted for inspection on arrival and required to provide evidence that their biofouling risks had been “appropriately managed.” The regulations also provide for a 5-year soft-start period, starting in September 2020, during which vessels without a BioFouling Management Plan may use other options, including treating or cleaning the hull and niche areas less than 30 days prior to arrival, to demonstrate effective biofouling management.
At a state level currently, only Western Australia and the Northern Territory have formal policies on biofouling. Vessels arriving to Western Australia ports from outside the state are required to ensure that marine pests and diseases are not being carried in biofouling and inspectors accredited by the West Australian Department of Fisheries routinely inspect vessels. This Department also maintains a voluntary, online risk assessment tool3 that allows operators of commercial vessels, non-trading, petroleum and commercial fishing vessels to assess the biofouling risk associated with any planned international or interstate movements, and thereby assist in the endorsement of any planned activities with the relevant state agencies.
Efficacy and Costs
Ballast Water Exchange
Shipboard studies of ballast water exchange show that the efficiency of volumetric exchange can vary from 66% to more than 99%, depending on ship type and method of exchange (Ruiz and Reid, 2007; Molina and Drake, 2016). Using the empty-refill method, exchange efficiencies typically exceed 98%, but if not managed correctly this method can create hazards for the vessel including instability and excessive shear forces on the hull (Endresen et al., 2004). Although safer, the flow-through method can result in much lower exchange efficiencies because of mixing between the influent and effluent water (Noble et al., 2016). Meta-analysis of empirical studies shows that concentrations of zooplankton are reduced by both methods of ballast water exchange by between 34 and 100%, with higher variability in outcomes for protists, bacteria and virus-like particles (Molina and Drake, 2016).
Ballast water exchange involves two types of costs: (i) the cost of operating the pumps, including fuel, energy, labor, and maintenance; and, (ii) the opportunity costs associated with slowing ship speed or diversion to areas that meet the D1 Standard of the Ballast Water Management Convention. The pumping costs depend on the type and size of the vessel, the ballast tank configuration and the method of exchange. Arthur et al. (2015b) estimate the average operational cost of exchange for vessels entering Australia at between USD $0.017 and $0.029 per tonne, with an average cost per vessel of USD $2790 for bulk carriers and USD $2020 for other vessel types. The total annual cost of ballast water exchange by vessels entering Australia was estimated at USD $29.3 million (Arthur et al., 2015b).
Costs associated with delay or diversion will vary according to the length of voyage and ship’s ballast water capacity. For most vessels traveling to New Zealand from other overseas ports these are not likely to be significant because most routes allow sufficient time to perform exchange in suitable areas. For shorter, coast-wise voyages, however, they can be significant. For example, mandatory diversion of ships traveling between Australian ports to areas that are at least 50 nautical miles from the coast and 200 meters deep (i.e., less stringent than the D-1 standard) has been estimated to cost more than USD $46 million per year (CIE, 2007).
Ballast Water Management Systems
To comply with Regulation D-3 of the Ballast Water Convention, ballast water management systems must have Type-approval from the Flag State administration. Type approval requires both land-based and shipboard testing of performance relative to the D-2 discharge standard (IMO, 2018a). As at January 2019, 14 administrations had advised Type approval of 76 ballast water management systems, 29 of which used active substances involving chemical or biological treatment (IMO, 2019). Although manufacturers continue to refine their systems to improve performance, a 2010 review of available technologies showed variable efficiency in removing dinoflagellates, phytoplankton and zooplankton from ballast water (Tsolaki and Diamadopoulos, 2010). Physical separation techniques (filtration and cyclonic separation) reduced concentrations by between 8 and 95% of original values. Mechanical treatments (ultraviolet, heat treatment and electric pulse applications) were generally more effective at reducing phytoplankton and zooplankton (reductions of 40% to more than 95% relative to controls) but less effective at treating dinoflagellate cysts (6–40% reductions). Treatments with active substances had the greatest efficacy, generally achieving reductions in excess of 80% across a range of organisms (Tsolaki and Diamadopoulos, 2010). Vessels entering the coastal waters of the United States are increasingly reporting the use of ballast water management systems but as of December 2015, 20 months before the Convention entered into force, they accounted for less than 2% of all ballast water discharged in the United States per month, (Davidson et al., 2017) whereas all ships must now meet the D-2 standard by the 8th September 2024.
The costs associated with ballast water management systems vary according to the type of system, vessel type and size, and whether it is to be installed on a new build or retrofitted to an existing vessel. King et al. (2012) reviewed information provided by vendors for a range of system types and ship types. Purchase prices of the units ranged between USD $640,000 and $947,000. Estimated costs of installation varied from USD $27,000 to $70,000 for new builds, depending on vessel type, and from USD $48,000 to $173,000 for retrofits. For most systems, the annual operating costs for maintenance were typically between USD $9,000 and $17,000, depending on vessel type and size, but technologies that used active substances had a much wider range (USD $31,000–$296,000) because the use of chemicals varied widely between different ship types and sizes.
Biofouling
In contrast to ballast water, there does not appear to be any comprehensive analysis of the compliance levels or efficacy of the Marine Environment Protection Committee 2011 biofouling guidelines. Guidance released in 2013 specified a range of performance measures for evaluation and a questionnaire pro forma to aid in the review (IMO, 2013). The MEPC has recently committed to review the guidelines in 2020–2021 based on these measures (IMO, 2018b). Data is emerging, however, in New Zealand. In 2015, the Ministry of Primary Industries initiated surveys of bio fouling on 40 arriving vessels to assess compliance with the (then voluntary) Craft Risk Management Standard. Thirty-nine vessels (greater than 95% of all arrivals) had some biofouling in niche areas and 16 (40%) were non-compliant with the short-stay biofouling thresholds (Kluza, 2018). Since the standard came into force in 2018, New Zealand authorities have taken action against 14 high-risk vessels, representing less than 1% of all arrivals. Six of these vessels were ordered to leave New Zealand waters within 24 h, while the others faced restrictions on the number of ports they could visit.
Section 7 of the MEPC biofouling guidelines recommends cleaning, maintenance and periodic inspection of ships to remove biofouling, which implies costs to vessel owners, operators and relevant authorities. Although technologies exist for in-water cleaning of vessels, most do not contain and capture material removed during the cleaning process so that biological material and contaminants from the anti-fouling coatings are released into the surrounding environment (Morrisey and Woods, 2015). As a result, in-water cleaning is banned or tightly regulated in many jurisdictions. The cost of removing the vessel to dry dock and applying anti-fouling coatings ranges from around USD $100,000 per vessel weighing up to 5,000 tonnes to over USD $464,000 per vessel (for vessels >200 meters in length) and may take 5–7 days with average opportunity costs per vessel per day of USD $4,400 for bulk vessels, USD $9,600 for general cargo vessels and USD $11,200 for container vessels (Branson, 2012; Inglis et al., 2012).
Cost estimates for inspection regimes are currently more difficult to come by in part because there are no internationally agreed protocols for inspection and documentation of biofouling. The Australian 2019 Regulatory Impact Statement for biofouling management estimates that the additional dive time needed to add biofouling considerations to the scheduled class survey, in-water inspections (that occur on average once every 2.5 years) will cost AUD $667, whereas specific in-water biofouling inspections are estimated to cost AUD $7,000 per vessel (Australian Government Department of Agriculture and Water Resources, 2019). The statement also estimates that the regulatory burden of developing and maintaining a vessel’s biofouling Management Plan and biofouling Record Book will be approximately AUD $15 per vessel per year.
Practical Challenges and their Implications
Risk assessments for ballast water and biofouling face a number of similar challenges, with biofouling presenting additional difficulties because unlike ballast water, whose source can be determined relatively precisely, biofouling can be acquired from multiple locations during a vessel’s in-service period, making its source pools harder to identify. Some of these challenges where foreshadowed by Simberloff (2006), and several others identified by Barry et al. (2008).
Environmental Variables, Distance and Invasion Risk
Two primary problems occur with environmental matching: the choice of environmental variables, and the distance metric itself. Firstly, the analysis by Hilliard and Raaymakers (1997), and the GloBallast risk assessments that subsequently adopted this approach (for example Clarke et al., 2004) include a large number of variables in the distance calculation. Barry et al. (2008), however, demonstrate that introducing any environmental variables into the calculation that are not truly predictive of introduction or establishment success creates noise which diminishes the signal of the true predictive distance.
Secondly, environmental distance is not currently well calibrated with any of the potential ballast water risk assessment endpoints of survival, establishment or impact. Several studies use environmental distance to identify donor regions that may act as sources of successful invaders, or incorporate environmental distance into parameters of invasion models (see for example Keller et al., 2011; Seebens et al., 2013), but the empirical relationship between distance and survival or establishment of non-native species has not, in our opinion, been adequately determined. Furthermore, as Ruiz et al. (2013) demonstrate the relationship between these variables and historical introductions may no longer be discernible, and without systematic surveys the relationship into the future will remain obscured by uncertainty about the date of location, the source of inoculation and the responsible vector.
Data Needs and Saturation of Species-Specific Assessment
The primary problems associated with species-specific risk assessment are: (i) the potential for the risk assessment to identify all vessels as high risk (i.e., offer no potential for low risk outcomes) as more and more species potentially transported by the vector are added to the assessment - a process that we refer to as “saturation,” (ii) the target species data needs and potential complexity of the modeling task; and, (iii) the availability and currency of information on the distribution of non-native species.
An analysis of an early proposal to develop a risk-based Decision Support System for international ballast water discharges in Australia showed that saturation can occur quite rapidly: 97% of international arrivals were deemed to be high risk with a target species list that was at that time restricted to only 12 species. This was largely due to the lack of information on the presence or absence of the target species in the international donor ports. In the absence of this information the risk assessment took a conservative approach and assumed target species were present, and risk reductions were not forthcoming in other parts of the assessment process.
Saturation did not occur, however, in the analysis of domestic ballast water translocations because the target list was smaller (reduced from 12 to 9), the Australian port baseline surveys (Campbell et al., 2007) and literature (for example Sliwa et al., 2008) provided better data on the distribution of non-native species in Australian ports, and risk reductions were forthcoming in the survival probability models that the assessment used.
The collation of data on the distribution and ecology of non-native species around the world (Katsanevakis and Roy, 2015) provides a growing information platform that can be used to populate parameters in species-specific risk assessment, such as the probability that the donor port contains a target pest. The number of different regional databases, only some of which are actively maintained, presents challenges, however, and the cost of acquiring information on the infection status of port, and maintaining the currency of this information, could become an important impediment to the long-term maintenance of species-specific risk assessments because: (i) as data ages the status of donor ports becomes increasingly uncertain; and, (ii) defensible (conservative) approaches to uncertainty lead to saturation.
Simberloff (2006) highlights a number of difficulties with species-specific approaches to invasion risk, in particular the data requirements, suggesting “Knowledge on most species is simply in-sufficient to enable more than educated guesses about the likelihood that a species will establish and impacts it might cause.” Assessing the invasion risk of the Pacific oyster (Crassostrea gigas) through ballast water or biofouling highlights the data and modeling complexities that can arise: The risk assessment supporting the Australian ballast water Decision Support System minimizes the complexity of the modeling task by choosing a survival endpoint over the alternatives of establishment or impact (Hayes, 2003), on the grounds that the latter endpoints required more complex models, and for species deemed a priori to be environmental pests the probability of survival was sufficiently close to stakeholders’ concerns to allow decision makers to make management decisions.
The probability of survival was assessed by simulating a species completing its life-cycle, and comparing the temperature tolerance at life-stage against simulated time series of daily temperature extremes in ports across Australia (Hayes et al., 2007, 2008). For sub-tidal species, the data and calculations proved tractable and have since been improved following an independent review (Arthur et al., 2015c). For inter-tidal species, such C. gigas, however, the analysis hit significant impediments. Firstly, the daily temperature regime experienced by inter-tidal organisms is substantially different to that of sub-tidal organisms because of their periodic exposure to the interacting elements of sunlight, wind speed and air temperate. Secondly, the body temperature of inter-tidal organisms is also influenced by body size and shape (Helmuth, 2002). These factors substantially raise the number of uncertain parameters in what was otherwise a relatively simple survival model. Whilst interest in these types of biophysical models is increasing in order to predict the effects of climate change (Levy et al., 2015), the data required to develop these types of models for target species is likely to be prohibitive.
Port Surveys
Species-specific risk assessments also require good information on the distribution of target species. Section 6.4.8 of the IMO G7 guidelines, for example, stipulates “if a target species is already present in the recipient port, it may be reasonable to exclude that species from the overall risk assessment for that port unless that species is under active control.” In effect, if all of the species that a vessel is assessed as being high risk against are already present (and not being actively controlled) in the recipient port, then the vessel defaults to low risk. Obviously, this approach makes no provision for species other than the target species, but it is based on the reasonable expectation that treatment costs should not be imposed on vessels if they are translocating species to locations where they already exist.
Section 6.4.8 can be problematic because of the high degree of power it imposes on the validity and currency of port survey information. In 1995 Australia embarked on a series of port baseline surveys following the “CRIMP protocols” (Hewitt and Martin, 1996, 2001). By 2005, 39 Australian ports had been surveyed to an accredited standard with these protocols, and the results entered into a National Port Survey Database (McEnnulty et al., 2005). The protocols were used extensively in New Zealand and elsewhere in the world, and have been the most widely implemented method of baseline survey for invasive species (Campbell et al., 2007).
The Australian baseline surveys helped define the distribution of non-native species in Australia. The surveys, however, are relatively expensive, and require a very high level of taxonomic expertise to successfully post-process the samples that are collected (Bishop and Hutchings, 2011). For example, only about 27% of the 15,412 taxonomic records in the Australian National Port Survey Database include a complete species name. The remaining records represent identifications to the level of Genus only (McEnnulty et al., 2005).
Australia responded to the requirement to maintain up-to-date records of the distribution on non-native species in its ports by developing a set of port monitoring guidelines that stipulated not only which ports should be regularly monitored but also to what standard (Australian Government Department of Agriculture Fisheries and Forestry, 2010a,b). The guidelines identified 18 ports around Australia as the minimum required to form an effective National Monitoring System.
Many of these ports, however, are large, complex regions that are difficult to sample for reasons such as low visibility and/or strong currents. Furthermore, the sample sizes and hence costs of sampling these locations to a standard with high statistical power proved to be too high (reportedly between AUD $175,000 and AUD $355,000), to repeat on a regular basis, and only 5 of the 18 locations, together with seven other locations, were surveyed to the recommended standard.
A lack of clearly understood objectives among stakeholders, together with the high cost of the surveys, coinciding with a significant reduction in resources allocated to marine pest research and development in Australia at this time, stalled the implementation of the National Monitoring System, and as part of a wider review of Australia’s marine biosecurity systems (Australian Government Department of Agriculture and Water Resources, 2015), it was recommended that the system be abandoned and replaced with a surveillance system based on cheaper methods designed with clearer objectives (Arthur et al., 2015a).
One of the cheaper methods recommended by Arthur et al. (2015a) – the use of eDNA probes based on species-specific primers – holds promise but requires extensive testing to determine the relationship between presence and abundance of pests in the environment and the availability of eDNA to sample. Currently these probes may suffer high rates of false negatives (at the survey level), because eDNA is not sampled even though the species is present (Wood et al., 2018). These types of probes are also, by construction, species-specific which again raises the prospect of an inevitably restricted target list. eDNA probes based on meta-barcoding may offer the opportunity for “screening level” assessments of multi-species assemblages, but these probes are currently beset with challenges in the supporting infrastructure (i.e., incomplete sequence databases) and analytical pipelines that render them prone to high rates of false positives and false negatives (Ammon et al., 2018). The other method suggested by Arthur et al. (2015a) – sampling only in areas where ballast water is discharged or loaded – are unproven, and in large ports with significant circulation due to strong currents or tidal ranges may not be beneficial.
In 2001 New Zealand embarked on a national series of port baseline surveys based on the CRIMP protocols, and by 2007 had completed 43 surveys, including repeat surveys of 13 ports and 3 international marinas. The baselines surveys ended in 2007 and were considered at the time to provide a good indication of the then current distribution of non-indigenous species. In 2002 New Zealand also commenced with a series of targeted (species-specific) surveys initially designed to provide early detection of 7 high risk species. Surveys are now implemented in 11 harbors nationally every 6 months at an annual cost of approximately NZ $2 million (Arthur et al., 2015a). A risk-based stratification of environments within each harbor is used to prioritize allocation of sample effort based on the likely distribution of founding populations of the primary target species (Inglis et al., 2006). These surveys adopt a more pragmatic approach to the problem of achieving a high statistical power (sensitivity) and use survey methods that are generic, quick and efficient to implement, with minimal processing of samples post-collection. Provisional identification of target species occurs in the field with only specimens of target and unrecognized taxa being retained for verification by taxonomic experts. In this way, relatively large sample sizes and coverage can be achieved in each survey at low sample cost. Because the surveys are principally focused on specific taxa, other groups of organisms (e.g., pelagic species, fish and benthic invertebrates) are not well sampled. The sensitivity of an individual survey varies among target species and is optimized across them using a risk-based Stochastic Scenario Tree model (Martin et al., 2007; Morrisey et al., 2012). For some species the per-survey sensitivity is less than the standards typically used to provide Proof of Freedom in veterinary and disease surveillance systems and, in these cases, the New Zealand surveys use Bayesian temporal discounting to provide Proof of Freedom based on non-detection in repeat, ongoing surveys.
Future Approaches to Marine Pest Risk Assessment and Management
Many of the current approaches to marine pest risk assessment are based on the conceptualization that risk can be resolved at the species level, with detailed and sufficiently accurate assessments of individual risks. This view is realistic in certain circumstances. In aquaculture systems, for example, the number of identified pests is typically limited and therefore the knowledge base required to implement species-specific assessment is potentially manageable. This approach is also assisted by the similarity of aquaculture production systems internationally, so that knowledge developed in one location will readily translate to other locations. Moreover, aquaculture systems will have a commercial imperative and financial base to fund the required research and to balance the costs and benefits.
The utility of species-specific approaches is also clear in managing post-border incursions. A key example would be attempting to eradicate an invasive species. In this case the threat is identified and knowledge can be developed from direct observations of the species’ behavior in its native and/or previously introduced range. In the case of post-border management, previous experience with arthropods suggests that taxon-specific information can be used to develop specific control plans and thereby improve the probability of successful eradication (Tobin et al., 2014). In developing response plans for specific target species, it is useful to cover a range of functional groups so that a response plan can be adapted easily for an incursion by an unexpected, but functionally similar species.
Pre-border, species-specific approaches to manage invasive species that pose a potential threat to environmental values are more problematic for several reasons. Firstly, the suite of potential invasive species is large but their distribution and ability to invade is poorly known. In addition, our ability to predict impact is limited, relying either on the species behavior in other non-native locales, or theoretical predictions as to the outcomes of the (potentially very) complex interactions between the species and the receiving environment. The Australian and New Zealand experience is that the inherent uncertainty and magnitude of this task can lead to policy impasses: there is potentially some level of risk in all vessels movements but its magnitude is uncertain. Hence any stakeholder can interpret it in a way that is consistent with their position. Moreover, currently there is no agreed way of determining a threshold of acceptable risk.
If we accept that species-specific approaches will not provide a practical basis for managing pre-border environmental pests we need to consider alternatives. Our starting point is to accept that all translocation pathways potentially carry some risk of environmental impact. If mitigation of this risk via a treatment was effectively cost free we would mandate it and the risk would be managed. In reality the costs of mitigation can be high. Hence there is a need to trade off the costs of mitigation versus the potential impact on the environment in a transparent and rational way.
Theory and experimental trials indicate that removing biological material, via ballast water exchange, ballast water treatment or increasingly stringent hull and niche area cleaning, will reduce inoculation pressure and therefore invasion risk (Bailey, 2015; Molina and Drake, 2016). In the limit, the elimination of all biological material on or contained in a vessel will achieve zero invasion risk. Thus we can conceptually rank treatments in terms of the amount of biological removed. It is important, however, that any such procedure accounts for operational conditions and distinguishes between application of a treatment - i.e., the management endpoint - and the actual amount of total biological inputs that are removed - i.e., the biological endpoint. Empirical analysis of the efficacy of ballast water exchange in Chesapeake Bay, for example, illustrates that operational parameters, such as changing trade patterns, can lead to an overall increase in inoculation pressure despite implementation of the management endpoint (Carney et al., 2017).
The key question is how stringent management activities should be in order to mitigate the risk to an acceptable level. Intuitively we believe that a biological threshold exists, we just are uncertain about where it is. Adaptive policy settings and the use of expert panels to define the settings and monitor performance against them are increasingly important means for dealing with complex systems with uncertain management outcomes (Walker et al., 2001). Our key observation is that if we impose a management action, based on expert opinion for example, then we must monitor its biological efficacy, observe the number of incursions that occur and adjust the action if this rate is unacceptable. In this way, we analyze the system outcomes across all species rather than a bottom up assessment of individual species. Evaluation and monitoring of performance at the system level, measured in a way that is meaningful to decision makers, provides the key feedback to assess how well risk has been mitigated (Amendola, 2002).
In essence we propose that a treatment standard is defined that is underpinned and enforced by a rigorous compliance monitoring system. Adherence to this standard potentially results in ecological, economic and social impacts on the jurisdiction due to the establishment of new marine pests. These changes are detected by the system monitoring and that is used by management to assess the acceptability of the rate and nature of these changes. If these changes are unacceptable (because the standard is not stringent enough) the standard is modified.
In practice we recognize several practical challenges. Firstly, setting an expert based threshold involves pragmatism and can appear ad hoc. The alternative, however, may be that no action is taken at all while more information is sought to reduce uncertainty. Unfortunately, some of these uncertainties may be irreducible. The proposal converts the problem into one that can collect explicit data (e.g., incursions) to assess performance. This provides a more transparent basis to explore costs and benefits of a system. The threshold can also be tailored to different sectors and jurisdictions where needed. To break any impasse about the practicality of the approach, the threshold could be aligned to outcomes that are achievable with current best practice and technology in the first instance, and then modified as further information became available.
Secondly, the proposed approach require effective compliance and compliance monitoring is therefore a key component of the system. As noted, compliance monitoring should consider both management and biological endpoints, and could be targeted at the highest risk vessels based on (for example) environmental-matching risk assessment (Barry et al., 2015). We stress that the estimate of risk referred to here relates to the relative risk the vessel may pose, compared to all other vessels. This is a simpler task than assessing absolute risk.
Thirdly, the proposed approach relies on monitoring ecosystem outcomes. This is arguably the most significant challenge. The appropriate level of system monitoring is not solely a science issue but should also reflect the information needs of decision makers. At one extreme a jurisdiction could rely on passive monitoring to provide information about the impacts of new incursion on marine systems. Passive monitoring would rely on members of the public or industry to identify significant impacts as and when they become significant enough to be detected, and also relies on the media and/or government agencies to champion the issue and raise public awareness. Such a strategy, however, exposes jurisdictions to a number of threats such as creeping baselines (Papworth et al., 2009) and potentially an inability to identify incursions until they occupy large areas and are thereby costlier or impractical to eradicate (Tobin et al., 2014). Nonetheless, we believe this is the default approach in many jurisdictions.
At the other extreme, a jurisdiction could use detailed monitoring at a large number of sites to provide an information base for decision making. As discussed previously, detailed surveys in complex port environments are currently expensive and have been hard to justify to funders so careful consideration of the purpose and design of monitoring is needed. This is an area that we believe requires additional attention. The New Zealand experience is that this challenge can be met with a pragmatic approach to survey sensitivity that relies on repeat surveys conducted by a core team who become familiar with the system and are thereby able to identify unusual occurrences. This approach, however, still costs approximately NZ $180,000 per port per year. It remains to be seen if the New Zealand experience could be scaled up to Australia but we believe this is worth further examination.
Finally, an adaptive, standard-based approach implies that the standard could change, and this could result in cost increases (or decreases) as industry moves to a more (or less) stringent standard. Such an approach also requires a standard that ideally: (i) can be assessed, at least in principle, by non-specialists to assist compliance and minimize associated costs; and (ii) describes what an acceptable level of biological contamination is - i.e., what is an acceptable level of fouling or acceptable concentration of organisms of different size classes in ballast water.
This type of standard is prescribed by the IMO D2 Ballast Water Treatment standards and is a feature of the New Zealand Craft Risk Management Standard for hull fouling (Georgiades and Kluza, 2017). The development of such a standard has the advantage of providing an unambiguous definition of what is and is not acceptable that can be directly related to observed environmental outcomes. The proposed biofouling management regime in Australia, which follows the MEPC guidelines, however, has moved away from this approach toward a standard based on a management endpoint not a biological endpoint. This could make it more difficult for an adaptive regime to respond to new information on incursion rates.
In a globalized world it is obviously helpful if standards are consistent world-wide. The ballast water convention is a clear example that, given time, a biological standard, and the technology needed to implement it, can be adopted around the world. The long lead times for international consensus mean that it is important that a similar approach for biofouling commence as soon as possible. In the interim countries can still apply their own standards consistent with their country-specific acceptable level of protection as defined by the World Trade Organization. Different countries are free to apply their own standards as long as they apply equally to all vessels and are, therefore, not trade restrictive.
The adaptive approach outlined above is consistent with the risk management measures adopted by countries for terrestrial biosecurity threats. For example, the importation of soil is tightly controlled in Australia, not on the basis of species-specific assessment but instead by a recognition that soil is a potential vector for a large number of organisms and is therefore unacceptable. Similarly, there are standards applied to the required cleanliness of shipping containers rather than relying on species-specific assessments.
In marine systems where the pathways are capable of sampling from a large species pool and where there is large uncertainty about which species will be transported and prosper in any given region, a species based approach to pre-border risk assessment will not scale well because it becomes impractical as the number of species to be assessed rises. This problem is compounded by the potentially large range of values that are held in marine environments (environmental, social and cultural) and uncertainty in how introduced species may affect them.
Species-specific approaches can, however, help sharpen thinking and preparedness and should not therefore be discarded altogether. Species with a well-established track record of invasion and impact should be identified as unwanted, and they provide an opportunity to refine post-border preparedness - i.e., develop species-specific tactics for detection, eradication and control. If these tactics can be also be developed with a view to how they might be adapted to incursions by unknown/unanticipated species, by example considering the functional group that high profile invaders belong to, then this will improve a jurisdictions ability to respond to new incursions quickly as they are detected.
Author Contributions
All authors listed have made a substantial, direct and intellectual contribution to the work, and approved it for publication.
Conflict of Interest Statement
GI is employed by NIWA. NIWA undertakes the Marine High Risk Site Surveillance program under contract to biosecurity New Zealand.
The remaining authors declare that the research was conducted in the absence of any commercial or financial relationships that could be construed as a potential conflict of interest.
Footnotes
- ^ http://www.imo.org/en/About/Conventions/ListOfConventions/Pages/International-Convention-for-the-Control-and-Management-of-Ships’-Ballast-Water-and-Sediments-(BWM).aspx
- ^ http://www.agriculture.gov.au/biosecurity/avm/vessels/ballast#quick-domestic-ballast-water-risk-assessment-tool
- ^ https://vesselcheck.fish.wa.gov.au/
References
Almeida, E., Diamantino, T. C., and de Sousa, O. (2007). Marine paints: the particular case of antifouling paints. Prog. Organ. Coat. 59, 2–20. doi: 10.1016/j.porgcoat.2007.01.017
Amendola, A. (2002). Recent paradigms for risk informed decision making. Saf. Sci. 40, 17–30. doi: 10.1016/S0925-7535(01)00039-X
Ammon, U. V., Wood, S. A., Laroche, O., Zaiko, A., Tait, L., Lavery, S., et al. (2018). Combining morpho-taxonomy and metabarcoding enhances the detection of non-indigenous marine pests in biofouling communities. Sci. Rep. 8:16290. doi: 10.1038/s41598-018-34541-34541
Arthur, T., Arrowsmith, L., Parsons, S., and Summerson, R. (2015a). Monitoring for Marine Pests: A Review of the Design and Use of Australia’s National Monitoring Strategy and Identification of Possible Improvements. Canberra, ACT: Australian Government Department of Agriculture, Water Resources.
Arthur, T., Summerson, R., and Mazur, K. (2015b). A Comparison of the Costs and Effectiveness of Prevention, Eradication, Containment and Asset Protection of Invasive Marine Species Incursions. Canberra, ACY: Australian Government Department of Agriculture, Water Resources.
Arthur, T., Zhao, S., Caley, P., Robinson, A., Gregg, M., and McCrudden, R. (2015c). Updating the Methods for Ballast Water Risk Table Construction. CEBRA project 1301C. Melbourne, VIC: Centre of Excellence for Biosecurity Risk Analysis, Melbourne University.
Australian Government Department of Agriculture Fisheries and Forestry (2010a). Australian Marine Pest Monitoring Guidelines. Canberra, ACT: Australian Government Department of Agriculture Fisheries & Forestry.
Australian Government Department of Agriculture Fisheries and Forestry (2010b). Australian Marine Pest Monitoring Manual. Canberra, ACT: Australian Government Department of Agriculture Fisheries & Forestry.
Australian Government Department of Agriculture and Water Resources (2015). Review of National Marine Pest Biosecurity. Canberra, ACT: Australian Government Department of Agriculture, Water Resources.
Australian Government Department of Agriculture and Water Resources, (2019). Australian biofouling management requirements for international vessel arrivals. Consultation Regulation Impact Statement. Canberra, ACT: Australian Government Department of Agriculture, Water Resources, Biosecurity Animal Division.
Bailey, S. A. (2015). An overview of thirty years of research on ballast water as a vector for aquatic invasive species to freshwater and marine environments. Aquat. Ecosyst. Health Manag. 18, 261–268. doi: 10.1080/14634988.2015.1027129
Barratt, B. I. P., Howarth, F. G., Withers, T. M., Kean, M., and Ridley, G. S. (2010). Progress in risk assessment for classical biological control. Biol. Control 52, 245–254. doi: 10.1016/j.biocontrol.2009.02.012
Barry, S. C., Caley, P., Liu, S., Paini, D. R., Carey, G., and Clark, J. (2015). Development of an Expert Based Model for Improved Biofouling Risk Assessment. CEBRA project 1402A. Melbourne, VIC: Centre of Excellence for Biosecurity Risk Analysis, Melbourne University.
Barry, S. C., Hayes, K. R., Hewitt, C. L., Behrens, H. L., Dragsund, E., and Bakke, S. M. (2008). Ballast water risk assessment: principles, processes, and methods. ICES J. Mar. Sci. 65, 121–31. doi: 10.1093/icesjms/fsn004
Behrens, H. L., Leppäkoski, E., and Olenin, S. (2005). Ballast Water Risk Assessment Guidelines for the North Sea and Baltic Sea. Stensberggata: Nordic Innovation Centre Project Number 04051.
Bell, A. S., Phillips, S., Georgiades, E., Kluza, D., and Denny, C. (2011). Risk Analysis: Vessel Biofouling. Report Prepared by the Policy; Risk Directorate, MAF Biosecurity New Zealand. Wellington: Ministry for Agriculture, Forestry.
Bishop, M. J., and Hutchings, P. A. (2011). How useful are port surveys focused on target pest identification for exotic species management? Mar. Pollut. Bull. 62, 36–42. doi: 10.1016/j.marpolbul.2010.09.014
Branson, J. (2012). Cost-Benefit Analysis of Proposed Import Health Standard for Vessel Biofouling. Technical Paper No: 2018/68. Wellington: Ministry for Primary Industries.
Campbell, M. L., Gould, B., and Hewitt, C. L. (2007). Survey evaluations to assess marine bioinvasions. Mar. Pollut. Bull. 55, 360–78. doi: 10.1016/j.marpolbul.2007.01.015
Carlton, J. T. (1985). Transoceanic and interoceanic dispersal of coastal marine organisms: the biology of ballast water. Oceanogr. Mar. Biol. 23, 313–371.
Carlton, J. T. (1992). Introduced marine and estuarine mollusks of north america: an end-of-the-20th-century perspective. J. Shellfish Res. 11, 489–505.
Carlton, J. T., Reid, D. M., and van Leeuwen, H. (1995). The Role of Shipping in the Introduction of Nonindigenous Aquatic Organisms to the Coastal Waters of the United States (Other Than the Great Lakes) and an Analysis of Control Options. Report No. CG-D-11-95. Springfield, VA: National Technical Information Service.
Carney, K. J., Minton, M. S., Holzer, K. K., Miller, A. W., McCann, L. D., and Ruiz, G. M. (2017). Evaluating the Combined Effects of Ballast Water Management and Trade Dynamics on Transfers of Marine Organisms by Ships. PLoS One 12:e0172468. doi: 10.1371/journal.pone.0172468
CIE, (2007). Ballast Water Management - A Regulation Impact Statement. Canberra, ACT: Centre for International Economics.
Clarke, C., Hilliard, R., Liuy, Y., Polglaze, J., Zhao, D., Xu, X., et al. (2004). Ballast Water Risk Assessment, Port of Dalian, People’s Republic of China, November 2003: Final Report. GloBallast Monograph Series No. 12. London: International Maritime Organisation.
Coutts, A. D., and Dodgshun, T. J. (2007). The nature and extent of organisms in vessel sea-chests: a protected mechanism for marine bioinvasions. Mar. Pollut. Bull. 54, 875–86. doi: 10.1016/j.marpolbul.2007.03.011
David, M., Gollasch, S., and Leppäkoski, E. (2013). Risk assessment for exemptions from ballast water management – the baltic sea case study. Mar. Pollut. Bull. 75, 205–217. doi: 10.1016/j.marpolbul.2013.07.031
Davidson, I. C., Brown, C. W., Sytsma, M. D., and Ruiz, G. M. (2009). The role of container ships as transfer mechanisms of marine biofouling species. Biofouling 25, 645–655. doi: 10.1080/08927010903046268
Davidson, I. C., Minton, M. S., Carney, K. J., Miller, A. W., and Ruiz, G. M. (2017). Pioneering patterns of ballast treatment in the emerging era of marine vector management. Mar. Policy 78, 158–62. doi: 10.1016/j.marpol.2017.01.021
Davidson, I. C., Scianni, C., Hewitt, C. L., Everett, R., Holm, E. R., Tamburri, M. N., et al. (2016). Mini-Review: assessing the drivers of ship biofouling management – aligning industry and biosecurity goals. Biofouling 32, 411–28. doi: 10.1080/08927014.2016.1149572
Endresen, Ø., Lee Behrens, H., Brynestad, S., Bjørn Andersen, A., and Skjong, R. (2004). Challenges in global ballast water management. Mar. Pollut. Bull. 48, 615–23. doi: 10.1016/j.marpolbul.2004.01.016
Floerl, O., Inglis, G. J., and Hayden, B. J. (2005). A risk-based predictive tool to prevent accidental introductions of nonindigenous marine species. Environ. Manag. 35, 765–78. doi: 10.1007/s00267-004-0193-198
Food and Agricultural Organisation (2006). International Standards for Phytosanitary Measures. Secretariat of the International Plant Protection Convention. Rome: FAO.
Georgiades, E., and Kluza, D. (2017). Evidence-Based decision making to underpin the thresholds in New Zealand’s craft risk management standard: biofouling on vessels arriving to New Zealand. Mar. Technol. Soc. J. 51, 76–88. doi: 10.4031/MTSJ.51.2.5
Hallegraeff, G. M., and Bolch, C. J. (1992). Transport of diatom and dinoflagellate resting spores in Ships’ ballast water: implications for plankton biogeography and aquaculture. J. Plankton Res. 14, 1067–84. doi: 10.1093/plankt/14.8.1067
Hayes, K. R. (2002). Identifying hazards in complex ecological systems. Part 2: infection modes and effects analysis for biological invasions. Biol. Invasions 4, 251–261. doi: 10.1023/A:1020943231291
Hayes, K. R., McEnnulty, F., Sutton, C., and Cooper, S. (2008). Ballast Water Decision Support System (DSS) Service Level Agreement (Sla) Iii. Final Report for the Australian Government Department of Agriculture, Fisheries and Forestry. Hobart, TAS: CSIRO Division of Mathematics, Information Sciences.
Hayes, K. R., McEnnulty, F., Gunasekera, R., Patil, J., Green, M., Lawrence, E., et al. (2007). Ballast Water Decision Support System (DSS) Service Level Agreement (Sla) – Part Ii. Final Report for the Australian Government Department of Agriculture, Fisheries and Forestry. Hobart, TAS: CSIRO Division of Marine, Atmospheric Research.
Hayes, K. R., and Sliwa, C. (2003). Identifying potential marine pests—a deductive approach applied to Australia. Mar. Pollut. Bull. 46, 91–98. doi: 10.1016/S0025-326X(02)00321-321
Hayes, (2003). Biosecurity and the Role of Risk-Assessment. in Bioinvasions: Pathways, Vectors, and Management Strategies, eds G. M. Ruizcpesnm, and J. T. Carlton, 382–414. (Washington, DC: Island Press).
Helmuth, B. (2002). How do we measure the environment? Linking intertidal thermal physiology and ecology through biophysics. Integr. Comp. Biol. 42, 837–845. doi: 10.1093/icb/42.4.837
Hewitt, C. L., and Martin, R. B. (1996). Port Surveys for Introduced Marine Species – Background Considerations and Sampling Protocols. CRIMP Technical Report 4. Hobart, TAS: Division of Fisheries, CSIRO.
Hewitt, C. L., and Martin, R. B. (2001). Revised Protocols for Baseline Port Surveys for Introduced Marine Species – Design Considerations, Sampling Protocols and Taxonomic Sufficiency. CRIMP Technical Report Number 22. Hobart,TAS: CSIRO Marine Research.
Hewitt, C. L., Campbell, M. L., Coutts, A. D., Dahlstrom, A., Shields, D. J., and Valentine, J. P. (2011). Species Biofouling Risk Assessment. A report commissioned by the Australian Government Department of Agriculture, Fisheries & Forestry, Canberra, Australia. Available at: http://www.agriculture.gov.au/SiteCollectionDocuments/animal-plant/pests-diseases/marine-pests/biofouling-consult/species-biofouling-risk-assessment.pdf (accessed July 26, 2019).
Hilliard, R. W., and Raaymakers, S. (1997). Ballast Water Risk Assessment -12 Queensland Ports: Stage 5 Report-Executive Summary & Synthesis of Stages 1-4. EcoPorts Monograph Series No. 14. Brisbane,QLD: Ports Corporation of Queensland.
Holland, R. E. (1993). Changes in planktonic diatoms and water transparency in Hatchery Bay, Bass Island Area, Western Lake Erie Since the establishment of the Zebra Mussel. J. Great Lakes Res. 19, 617–624.
IMO. (2018a). Ballast Water Management Convention and BWMS Code. London: International Maritime Organisation.
IMO, (2018b). Report of the Marine Environment Protection Committee on Its Seventy-Second Session. MEPC 72/17. London: International Maritime Organisation.
IMO, (2013). Guidance for Evaluating the 2011 Guidelines for the Control and Management of Ships’ Biofouling to Minimize the Transfer of Invasive Aquatic Species. MEPC.1/Circ 811. London: International Maritime Organisation.
Inglis, G. J., Floerl, O., and Woods, C. (2012). Scenarios of Vessel Biofouling risk and Their Management: An Evaluation of Options. Technical Paper No. 2012/07. Wellington: Ministry of Agriculture & Forestry.
Inglis, G. J., Floerl, O., Ahyong, S., Cox, S., Unwin, M., Ponder-Sutton, A., Seaward, K., et al. (2010). The Biosecurity Risks Associated with Biofouling on International Vessels Arriving in New Zealand: Summary of the Patterns and Predictors of Fouling. Biosecurity New Zealand Technical Paper No: 2008/XX. Wellington: MAF Biosecurity New Zealand.
Inglis, G. J., Hurren, H., Gust, N., Oldman, J., Fitridge, I., Floerl, O., and Hayden, B. (2006). Surveillance Design for Early Detection of Unwanted Exotic Marine Organisms in New Zealand. Biosecurity New Zealand Technical Paper No. 2005-17. Wellington: MAF Biosecurity New Zealand.
Katsanevakis, S., and Roy, H. E. (2015). Alien species related information systems and information management. Manag. Biol. Invasions 6, 115–117. doi: 10.3391/mbi.2015.6.2.01
Kellar, J. A. (1993). The application of risk analysis to international trade in animal and animal products. Rev. Sci. Tech. 12, 1023–1044 doi: 10.20506/rst.12.4.745
Keller, R. P., Drake, J. M., Drew, M. B., and Lodge, D. M. (2011). Linking environmental conditions and ship movements to estimate invasive species transport across the global shipping network. Divers. Distrib. 17, 1366–9516. doi: 10.1111/j.1472-4642.2010.00696
King, D., Hagan, P., Riggio, M., and Wright, D. (2012). Preview of global ballast water treatment markets. J. Mar. Eng. Technol. 11, 3–15.
Kluza, D. (2018). “Does This Look Risky to You? Profiling Vessel Biofouling in a Biosecurity Context,” in Proceedings of the ICMB-X - Tenth International Conference on Marine Bioinvasions, Puerto Madryn.
Kohler, C. C., and Stanley, J. G. (1984). Implementation of a review and decision model for evaluating proposed exotic fish introductions in Europe and North America. Eur. Inland Fish. Advis. Comm. Tech. Pap. 42, 541–549.
Lacoursière-Roussel, A., Forrest, B. M., Guichard, F., Piola, R. F., and McKindsey, C. W. (2012). Modeling biofouling from boat and source characteristics: a comparative study between Canada and New Zealand. Biol. Invasions 14, 2301–2314. doi: 10.1007/s10530-012-0230-230
Lane, S. E., Hollings, T., Hayes, K. R., McEnnulty, F. R., Green, M., Georgiades, E., et al. (2019). Risk factors for fouling biomass: evidence from small vessels in Australia. Biofouling 34, 1032–1045. doi: 10.1080/08927014.2018.1536202
Levy, O., Buckley, L. B., Keitt, T. H., Smith, C. D., Boateng, K. O., Kumar, D. S., et al. (2015). Resolving the life cycle alters expected impacts of climate change. Proc. Biol. Sci. 282:20150837. doi: 10.1098/rspb.2015.0837
Marine Environment Protection Committee [MEPC] (2017). 2017 Guidelines for Risk Assessment Under Regulation a-4 of the Bwm Convention 2017. London: International Maritime Organisation.
Marine Environment Protection Committee [MEPC] (2007). Guidelines for Risk Assessment Under Regulation a-4 of the Bwm Convention (G7). London: International Maritime Organisation.
Marine Environment Protection Committee [MEPC] (2011). 2011 Guidelines for the Control and Management of Ships’ Biofouling to Minimize the Transfer of Invasive Aquatic Species. London: International Maritime Organisation.
Martin, P. A. J., Cameron, A. R., and Greiner, M. (2007). Demonstrating freedom from disease using multiple complex data sources. 1: a new methodology based on scenario trees. Preven. Vet. Med. 79, 71–97. doi: 10.1016/j.prevetmed.2006.09.008
McCarthy, S. A., and Khambaty, F. M. (1994). International dissemination of epidemic vibrio cholerae by cargo ship ballast and other nonpotable waters. Appl. Environ. Microbiol. 60, 2597–2601.
McConnell, M. L. (2002). Globallast Legislative Review - Final Report. GloBallast Monograph Series No. 1. London: International Maritime Organisation.
McEnnulty, F. R., Hayes, K. R., Cooper, S., Sliwa, C., Dunstan, P., Migus, S., et al. (2005). National Port Survey Collection: Consolidation, Analysis and Integration. Hobart, TAS: Australian Government Department of Agriculture, Fisheries, Forestry, CSIRO Marine Research.
Medcof, J. C. (1975). Living marine animals in a Ship’s ballast water. Proc. Natl. Shellfisheries Assoc. 65, 11–12.
Ministry for Primary Industries, (2018). Craft Risk Management Standard: Biofouling on Vessels Arriving to New Zealand. Wellington: Ministry for Primary Industries.
Molina, V., and Drake, L. A. (2016). Efficacy of open-ocean ballast water exchange: a review. Manag. Biol. Invasions 7, 375–88. doi: 10.3391/mbi.2016.7.4.07
Morley, R. S. (1993). A model for the assessment of the animal disease risks associated with the importation of animals and animal products. Rev. Sci. Tech. 12, 1055–1092. doi: 10.20506/rst.12.4.743
Morrisey, D., Inglis, G. J., Peacock, L., and Seaward, K. (2012). Stochastic Scenario Tree Modelling for the Marine High Risk Surveillance. NIWA Report No. NEL2013-003 prepared for the Ministry for Primary Industries contract SOW12099. Nelson: National Institute of Weather, Atmospheric.
Morrisey, D., and Woods, C. (2015). In-water cleaning technologies: Review of Information. Technical Paper No: 2015/38. Wellington: Ministry for Primary Industries.
Moser, C. S., Wier, T. P., First, M. R., Grant, J. F., Riley, S. C., Robbins-Wamsley, S. H., et al. (2017). Quantifying the extent of niche areas in the global fleet of commercial ships: the potential for ‘Super-Hot Spots’ of Biofouling. Biol. Invasions 19, 1745–1759. doi: 10.1007/s10530-017-1386-4
New Zealand Government, (1998). Ballast Water and Ships’ Hull Defouling - a Government Strategy. Wellington: Ministry of Fisheries.
Noble, M., Ruiz, G. M., and Murphy, K. R. (2016). Chemical assessment of ballast water exchange compliance: implementation in North America and New Zealand. Front. Mar. Sci. 3:66. doi: 10.3389/fmars.2016.00066
Northern Territory of Australia, (2009). Schedule I - Aquatic Pests. Fisheries Amendment (Noxious Fish and Aquatic Pests) Regulations 2009. Subordinate Legislation No. 6 of 2009. Available at: https://legislation.nt.gov.au/api/sitecore/SubordinateLegislation/PDF?id=4242 (accessed July 26, 2019).
Office International des Epizooties, (1996). International Animal Health Code (Mammals, Birds and Bees). Paris: Office International des Epizooties.
Papworth, S. K., Rist, J., Coad, L., and Milner-Gulland, E. J. (2009). Evidence for shifting baseline syndrome in conservation. Conserv. Lett. 2, 93–100. doi: 10.1111/j.1755-263X.2009.00049.x
Piola, R. F., and Conwell, C. (2010). Vessel Biofouling as a Vector for the Introduction of Nonindigenous Marine Species to New Zealand: Fishing Vessels. MAF Biosecurity New Zealand Technical Paper No: 2010/11. Wellington: MAF Biosecurity New Zealand.
Price Waterhouse Coopers, (2011). Proposed Australian Biofouling Management Requirements - Consultation Regulation Impact Statement. Canberra, ACT: Australian Government Department of Agriculture, Fisheries & Forestry.
Ruiz, G. M., Fofonoff, P. W., Ashton, G., Minton, M. S., and Miller, A. W. (2013). Geographic variation in marine invasions among large estuaries: effects of ships and time. Ecol. Appl. 23, 311–20. doi: 10.1890/11-1660.1
Ruiz, G. M., and Reid, D. F. (2007). Current State of Understanding about the Effectiveness of Ballast Water Exchange (BWE) in Reducing Aquatic Nonindigenous Species (ANS) Introductions to the Great Lakes Basin and Chesapeake Bay, USA: Synthesis and Analysis of Existing Information. NOAA Technical Memorandum GLERL–142, Ann Arbor, M. I., USA. Available at: https://www.oregon.gov/deq/Rulemaking%20Docs/ballast2016currentstateBWE.pdf (accessed July 26, 2019).
Schultz, M. P., Bendick, J. A., Holm, E. R., and Hertel, W. M. (2011). Economic impact of biofouling on a naval surface ship. Biofouling 27, 87–98. doi: 10.1080/08927014.2010.542809
Seebens, H., Gastner, M. T., and Blasius, B. (2013). The risk of marine bioinvasion caused by global shipping. Ecol. Lett. 16, 782–90. doi: 10.1111/ele.12111
Shiganova, T. A. (1998). Invasion of the black sea by the ctenophore Mnemiopsis Leidyi and recent changes in pelagic community structure. Fish. Oceanogr. 7, 305–310. doi: 10.1046/j.1365-2419.1998.00080.x
Simberloff, D. (2006). Risk assessment, blacklists, and white lists for introduced species: are predictions good enough to be useful? Agric. Resour. Econ. Rev. 35, 1–10 doi: 10.1017/s1068280500010005
Sliwa, C., Migus, S., Hayes, K. R., and McEnnulty, F. (2008). “Marine Bioinvasions in Australia,” in Marine Bioinvasions, Ecology, Conservation and Management Perspectives, eds J. Crookscpesnm, and G. Rilov. (Amsterdam: Springer-Verlag).
Tobin, P. C., Kean, J. M., Suckling, D. M., McCullough, D. G., Herms, D. A., and Stringer, L. D. (2014). Determinants of successful arthropod eradication programs. Biol. Invasions 16, 401–414. doi: 10.1007/s10530-013-0529-525
Tsolaki, E, and Diamadopoulos, E. (2010). Technologies for ballast water treatment: a review. J. Chem. Technol. Biotechnol. 85, 19–32. doi: 10.1002/jctb.2276
Walker, W. E., Rahman, S. A., and Cave, J. (2001). Adaptive policies, policy analysis, and policy-making. Eur. J. Operat. Res. 128, 282–89. doi: 10.1016/S0377-2217(00)00071-70
William, R. J., Griffiths, F. B., Van der Wal, E. J., and Kelly, J. (1988). Cargo vessel ballast water as a vector for the transport of non-indigenous marine species. Estuarine Coast. Shelf Sci. 26, 409–420. doi: 10.1016/0272-7714(88)90021-2
Wood, S. A., and Pochon, X. Ming, W. von Ammon, U. Woods, C. Carter, M. et al. (2018). Considerations for incorporating real-time pcr assays into routine marine biosecurity surveillance programmes: a case study targeting the mediterranean fanworm (Sabella spallanzanii) and club tunicate (Styela clava). Genome 62, 137–146. doi: 10.1139/gen-2018-0021
Keywords: marine pest, risk assessment, monitoring, ballast water, biofouling
Citation: Hayes KR, Inglis GJ and Barry SC (2019) The Assessment and Management of Marine Pest Risks Posed by Shipping: The Australian and New Zealand Experience. Front. Mar. Sci. 6:489. doi: 10.3389/fmars.2019.00489
Received: 23 April 2019; Accepted: 19 July 2019;
Published: 06 August 2019.
Edited by:
Jessica Redfern, Southwest Fisheries Science Center (NOAA), United StatesReviewed by:
Hanno Seebens, Senckenberg Biodiversity and Climate Research Centre, GermanyKevin Alexander Hovel, San Diego State University, United States
Copyright © 2019 Hayes, Inglis and Barry. This is an open-access article distributed under the terms of the Creative Commons Attribution License (CC BY). The use, distribution or reproduction in other forums is permitted, provided the original author(s) and the copyright owner(s) are credited and that the original publication in this journal is cited, in accordance with accepted academic practice. No use, distribution or reproduction is permitted which does not comply with these terms.
*Correspondence: Keith R. Hayes, keith.hayes@csiro.au