- 1CSA Ocean Sciences Inc., Stuart, FL, United States
- 2Department of Biological Sciences, Center for Coastal Oceans Research, Florida International University, Miami, FL, United States
- 3Department of Integrative Biology, University of California, Berkeley, Berkeley, CA, United States
Water flow through seagrass beds transports nutrients, affects sediment stability and chemistry, and imposes hydrodynamic forces on shoots that alter canopy configuration. Past studies done under diverse conditions yielded conflicting results about the effects of shoot density on flow through seagrass bed canopies. We used eelgrass, Zostera marina, to study how the density of flexible shoots affect the hydrodynamics of seagrass beds in unidirectional water flow. By exposing randomly-arranged shoots of uniform length to current velocities controlled in a flume, the effects of shoot density and distance downstream from the bed edge could be determined without confounding factors. Comparison of velocity profiles within beds to those upstream of beds showed that flow was slower in the beds. However, shoot density, downstream distance, and current velocity did not affect the percent reduction in flow velocity in a bed. Turbulence enhances mixing of substances carried in the water. Here, turbulence intensity (index of the importance of turbulent velocity fluctuations relative to average current velocity) was lower when ambient flow was faster, but was not affected by shoot density or downstream position, Drag (hydrodynamic force on a shoot that bends it over in the flow direction) provides another measure of how the canopy affects flow experienced by a shoot. Drag was not affected by current velocity, shoot density, or downstream position in the bed. Gaps between shoots can enhance light and flow penetration into the canopy, but when shoots are bent over by flow, they can cover gaps. Faster ambient currents caused greater gap closure, which at each current speed was greater for high shoot densities. Thus, canopy gap closure did not correlate with percent flow reduction in grass beds or with drag on individual shoots, both of which were independent of shoot density and ambient current velocity. Since changing shoot density does not affect the flow in a grass bed exposed to a given ambient current, our results are inconsistent with the hypothesis that the high shoot densities observed in grass beds in habitats exposed to rapid flow are due to a direct, adaptive response of the grass to the flow environment.
Introduction
Studies of water flow through seagrass beds have been carried out under different conditions (e.g., unidirectional currents vs. waves, field sites vs. laboratory flumes, various species vs. physical mimics or mathematical models), and thus have yielded conflicting results about the effects of shoot density on flow within and above seagrass beds. Even though focusing on the numerous studies that have examined the behavior of unidirectional water flow in and around seagrass canopies (e.g., Fonseca et al., 1982, 1983, 2007; Fonseca and Fisher, 1986; Fonseca and Kenworthy, 1987; Gambi et al., 1990; Ackerman and Okubo, 1993; Worcester, 1995; Koch and Gust, 1999; Verduin and Backhaus, 2000; Abdelrhman, 2003; Newell and Koch, 2004; Peterson et al., 2004; Fonseca and Koehl, 2006; Lacy and Wyllie-Echeverria, 2011; Lei and Nepf, 2016) consistently reveals that seagrass beds reduce flow velocity within the canopy, the effect of (seagrass1) shoot density on this velocity reduction is not clear.
Effects of Shoot Density on Water Flow in Seagrass Beds
The discrepancies between various empirical studies of shoot density effects on flow may be due to differences between studies in the range of densities compared, the flow conditions, the position within a seagrass bed that the flow was measured, and the morphology of the seagrass. For example, Lacy and Wyllie-Echeverria (2011) found for eelgrass (Zostera marina) that flow speed was attenuated more by beds with higher shoot densities. However, their shoot densities were low (44–63 shoots m−2) and the plants they studied were from water depths of 3–4 m and thus had longer leaves (∼1.5 m leaf length) than do Z. marina from shallower sites (e.g., plants used in this study taken from seagrass beds of <2 m water depth; Fonseca et al., 2002). Likewise, Worcester (1995) considered the lower end of the shoot density range in an open, natural system and under very low ambient current speeds. She found that in a natural setting with current speeds of only 0.05 m s−1 and shoot density (with very large plants) of <200 shoots m−2, found there was still significant reduction in currents within as opposed to above the canopy, whereas the turbulence of the flow was unaffected. In contrast Adhitya et al. (2014) studied seagrass beds with higher shoot densities (400 and 1100 shoots m−2) and found that shoot density affected the flow dynamics at a very low ambient current speed (0.10 m s−1), which is so slow that it does not fully deflect a seagrass canopy (Fonseca and Kenworthy, 1987). However, Adhitya et al. (2014) also found the spatial organization of seagrass shoots at the meadow scale was more important than shoot density within a patch in explaining hydrodynamic effects of seagrass beds. Paul and Gillis (2015) also found an effect of shoot density on flow reduction by beds of Z. noltii. They visually compared velocity profiles and concluded that canopy bending, and waving did not affect the flow-reduction capacity of their grass beds. In contrast, Fonseca et al. (1982, 2007), Fonseca and Fisher (1986), and Gambi et al. (1990) found little influence of shoot density on the degree of current speed reduction within beds of Z. marina. Interestingly, while Gambi et al. (1990) found no statistically significant difference in shear velocity or turbulence intensity in seagrass beds as a function of shoot density, they concluded “More replicates in each position probably would reveal statistically significant differences between densities.” Their assumption that shoot density affects flow was compelling, and their paper has been interpreted by others as having demonstrated that shoot density affects flow (e.g., Koch et al., 2006).
Various mathematical models have explored whether shoot density affects water flow within grass beds. Dijkstra and Uittenbogaard (2010) simulated the effect of shoot density and flexibility and found that turbulence intensity was decreased as shoot density increased (up to 10,000 shoots per m−2), and that the drag force on stiff shoots was greater than on more flexible shoots. Their model suggests that both high shoot density and increased flexibility could be mechanisms that enable seagrass to withstand rapid ambient currents. Abdelrhman (2003) used shoot density as a factor influencing in-canopy flow in a model and found that high shoot density reduced transport through the canopy by increasing the tortuosity of the paths of water moving around seagrass shoots. However, Abdelrhman’s model did not clarify the hydrodynamic conditions under which these different shoot densities may occur in the field. The model of Newell and Koch (2004) assumed critical shoot-density thresholds for wave attenuation by seagrass beds, and the model of Koch et al. (2006) also assumed that higher shoot density produced lower in-bed flow velocities. Thus, shoot density effects have become codified in models and perception, in spite of the conflicting empirical results. One way to resolve this issue would be to measure the hydrodynamic effects of shoot density at known positions in beds of actual plants of one species (rather than physical mimics) when they are exposed to the same unidirectional flow conditions, and to compare the effects of shoot densities using conventional statistics that directly consider the variability of each effect.
Effects of Seagrass Flexibility on Canopy Structure and Water Flow
Seagrass shoots, particularly those of strap-leaved species, are extremely flexible (Fonseca and Koehl, 2006; Fonseca et al., 2007), as anyone who has handled these plants can attest. Flexible seagrasses are bent over by the drag force imposed by flowing water (Bouma et al., 2005), while the buoyancy of grass blades resists their downward bending (Luhar and Nepf, 2011), as has also been demonstrated for macroalgae (e.g., Stewart, 2004). The magnitude of drag on a sessile organism depends on the projected area of the organism normal to the flow direction, thus bending over in flowing water is a mechanism of reducing drag (e.g., Charters et al., 1969; Koehl, 1976, 1977, 1986; Denny et al., 1985; Denny, 1988; Koehl and Alberte, 1988; Carrington, 1990; Abdelrhman, 2003). Increasing the ambient flow velocity encountered by a sessile organism causes higher drag. Therefore, the drag on a shoot within a grass bed is a measure of how the other plants in the canopy affect the flow experienced by that shoot.
When a group of flexible seagrass shoots bend in response to flowing water, they can form a compact, interwoven structure that intensifies the reduction of water velocity within the compressed canopy and simultaneously redirects the flow over the canopy (Fonseca et al., 1982; Gambi et al., 1990), thereby shifting high shear stresses from the sediment surface to the top of the canopy (Fonseca et al., 1982). Such a reduction in flow-induced shear on the substratum should enhance both rhizosphere stability and carbon accumulation on the sediment (sensu Kenworthy et al., 1982).
Flexible and compressible grass canopies pose a challenge to understanding the effects of shoot density on flow. The relationship between shoot density and water-speed reduction in canopies composed of stiff macrophytes (e.g., marsh grass communities composed of upright shoots with relatively high flexural stiffness) has been determined via experiments using arrays of rigid rods in a flume (e.g., Nepf, 1999). In contrast, the degree of bending and canopy compression of flexible plants like seagrasses can depend on ambient current velocity and presumably on shoot density as well. Therefore, the effects of shoot density on flow through flexible canopies should be determined for the same sets of grass arrays at a range of different ambient flow speeds.
Fonseca et al. (2007) suggested that the arrangement of shoots in a seagrass bed might affect both water flow and light transmission into the bed. Seagrass shoots sometimes are arranged in rows (Fonseca et al., 2007) that can create anisotropic gaps in the canopy, and random arrangements of shoots can also create gaps in grass beds. Canopy gaps in terrestrial systems have long been recognized as local regions of higher light intensity and as initiation points of disturbance propagation (e.g., Sprugel, 1976; Veblen, 1985; Iwasa et al., 1991), and similar effects have been postulated for seagrasses (Fonseca et al., 1983; Valentine et al., 1994). Folkard (2011) studied how gaps within an array of flexible vegetation introduce flow into the canopy and described the likely role of gaps on various depositional process. The effects of shoot density on the formation or closure of gaps in seagrass canopies as these flexible plants are bent over by ambient water flow should be determined for different current speeds, and the effects of these gaps on flow velocities and turbulence should be measured. This would provide useful information to assess various mechanisms that have been proposed for how shoot density in a seagrass bed might be changed in response to ambient water flow.
Does Ambient Current Speed Affect Shoot Density in Seagrass Beds?
It has long been suggested that faster ambient water flow might lead to an increase in shoot density in seagrass beds (e.g., Conover, 1964; Short, 1975; Peterson et al., 2004). Subsequent work showing that shoot density of Z. marina was positively correlated with current speed (Kenworthy et al., 1982) was consistent with this prediction. However, a number of other factors are known to affect shoot density. For example, in light-limited environments, shoot density varies positively with light intensity (Short et al., 1995; Ruiz and Romero, 2001; Krause-Jensen et al., 2003). Furthermore, increasing sediment organic content or nutrient availability can lead to increases in shoot density (e.g., Koch, 2001; Holmer et al., 2008; Howard et al., 2016; Ceccherelli et al., 2018). These factors are in turn affected by the deformation of shoots by hydrodynamic drag, the formation or closure of gaps in the canopy in flowing water, and the transport of dissolved nutrients and sediment by water moving around and through a grass canopy. Therefore, determining whether current speed drives a shoot density response in seagrasses is complicated by the dynamic canopy architecture of most seagrasses in response to water motion.
Objectives of This Study
The goal of this study was to determine whether shoot density in a seagrass bed exposed to unidirectional ambient water flow affects the hydrodynamics and canopy architecture of the bed. We avoided the contradictory results of earlier studies conducted under diverse conditions by focusing on one species of seagrass (Z. marina) with very flexible shoots exposed to unidirectional currents of different velocities that we controlled in a laboratory flume. For all shoot density and flow treatments, we held shoot size constant and measured water flow, forces on shoots, and gaps in the canopy at the same defined positions in the grass bed. The specific questions that we addressed using this system were:
1. How does shoot density affect the reduction in water speed and the change in turbulence intensity within a seagrass bed as a function of distance from the upstream edge of the canopy when seagrass beds are exposed to ambient unidirectional currents of different speeds?
2. How do shoot density and ambient current velocity affect the hydrodynamic forces on shoots in a seagrass bed at different distances downstream from the upstream edge of the bed?
3. Does reduction in seagrass shoot density by random removal of plants affect the formation of gaps in the canopy, and how are those gaps affected by the speed of the ambient current that bends the shoots?
Answers to these questions can be used to address the issue of whether differences in seagrass shoot density observed in the field represent a direct adaptive response to ambient flow regime. For example, if seagrass shoots in a bed of a given density experience the same hydrodynamic forces and gap structure irrespective of downstream distance in the bed, and if those forces and gaps are not affected by shoot density, then the speed of the ambient unidirectional current flow should not be considered a direct driver of shoot density. Consequently, other factors (e.g., nutrients, light) could alone explain the large range in seagrass shoot densities often seen for Z. marina over small geographic distances.
Materials and Methods
Seagrass Used in the Flume
Healthy (i.e., intact, minimally epiphytized) shoots of Zostera marina were collected near Beaufort, NC, in July from two sites described by Fonseca and Bell (1998): Middle Marsh [site mmnl1], and southern Core Sound [site hih2]). Shoots were kept in flowing seawater in tanks (40 l) at ambient temperature (∼24°C) and salinity (∼34) under flood lamps (Philips 250 W model: K250PARFL) that exposed them to ∼250 μE m−2 s−1 for 24 h per day to stimulate photosynthesis and stabilize flexural stiffness.
A seagrass shoot attached to the substratum and exposed to unidirectional water flow parallel to the substratum is bent by the hydrodynamic drag like a cantilevered beam is bent by an applied force. The flexural stiffness (EI) of a beam, its resistance to being bent by a force, depends on the stiffness (E, elastic modulus) of the material from which it is made, and on the distribution of material around its axis of bending (I, second moment of area of a beam cross-section) (e.g., Wainwright et al., 1976). Because one focus of our study was the role that seagrass flexibility plays in affecting gaps in seagrass canopies and thus flow through the canopies, we measured the flexural stiffness of grass from both field collection sites. Using methods described in Fonseca and Koehl (2006), the EI of both leaf and sheath samples from randomly selected seagrass shoots from each site were measured by being bent like a cantilevered beam by a point load, where:
where, F is the force (N) applied at a point along the cantilever at distance L (m) from the attached end of the cantilever, and δ is the linear deflection distance (m) in the direction of force application of the point on the beam where the force was applied. Deflection was always δ < 0.10 L so that small-deflection beam equation (Equation 1) would apply. Differences among the plants from the two field sites were tested by one-way analysis of variance (ANOVA) after natural log +1 transformation to determine if there were differences in flexural stiffness among plant sources that could influence subsequent trials.
Flume and Experimental Seagrass Beds
The seawater flume (8 m long × 1 m wide × 0.75 m deep) used for this study is described in Fonseca and Koehl (2006) and Fonseca et al. (2007). Consistent with past studies in this flume, the constructed seagrass bed was 0.25 m wide by 1.0 m long, and the water depth was 0.3 m. Shoots were held in place in holes in a clear acrylic plate (0.25 × 1.0 m) that was fit into a recessed portion of flume floor to be flush with the main floor of the flume. Random shoot arrangements were used to avoid the flow artifacts measured in beds of shoots arranged in unnatural, evenly-spaced rows (see Fonseca et al., 2007). Random arrangements of shoots were created in the plate by overlaying it with a clear plastic grid (25 × 100 cm) on which each point where lines intersected was numbered (1–2500). A random-number generator (produced by a HP-11c calculator) was used to select the points where shoots should be placed for the highest shoot density desired (1246 shoots m−2). Holes (6.25 mm diameter) were drilled through the plate at these points. The shoot of a Z. marina was wedged through each hole so that the upper surface of the plate intersected the shoot at the same position as the sediment surface intersected the shoot in the field. The shoots were held in natural upright positions and the orientation of the shoots with respect to the direction of water flow in the flume was arbitrary. Shoots were kept moist when in air and were not exposed to air for more than 3 min between the time collected and when installed in the flume.
Seagrass-flow interactions were tested for seven different shoot densities (1246, 1046, 846, 646, 448, and 246 shoots m−2) that were chosen to approximate the normal range of densities of monotypic stands of Z. marina in the Beaufort, NC, area (natural observed range of 185–1133 shoots m−2; Fonseca and Bell, 1998). As described above, shoots were randomly arranged at the highest shoot density. After the flow trials were run at the highest density, some shoots were selected using the random-number generator to be removed to achieve the next lower density, and so on.
Drag Measurements
The hydrodynamic force pushing a shoot downstream (drag) was measured for shoots in grass beds of different densities. For each shoot density tested, a seagrass shoot was arbitrarily selected from those collected in the field and this single shoot was attached to a force transducer as described in Fonseca and Koehl (2006) and Fonseca et al. (2007). The force transducer and attached shoot were placed in the grass bed at the flume midline, first at the position 0.50 m downstream from the leading edge of the grass bed, and then at the position 1.00 m downstream from the leading edge. At each position, drag force was measured to the nearest 0.01 N at a sampling rate of 5 Hz for a period of 5 min, and the mean force was calculated. Such drag measurements were repeated 9 times at each position for each shoot density and freestream current velocity.
Flow Measurements
Three freestream water speeds representative of currents measured just upstream of beds of Z. marina at sites near Beaufort, NC (Fonseca and Bell, 1998), were tested in the flume: 21, 32, and 63 cm s−1. Following protocols developed in Fonseca and Koehl (2006) and Fonseca et al. (2007), a Marsh-McBirney Model 523 electromagnetic current flow meter was used to record horizontal water velocities at the midline of the flume at heights of 2, 5, 8, 11, 14, 17, 20, and 23 cm above the substratum. Water velocities were recorded for 30 s at 5 Hz at each height. Examples of the velocity profiles measured in this way are shown in Figure 1. Velocity profiles were measured at three sampling locations along the midline of the flume: over bare substratum at a position 25 cm upstream of the leading edge of the constructed grass bed, and in the grass bed at distances of 50 and 100 cm downstream from the leading-edge.
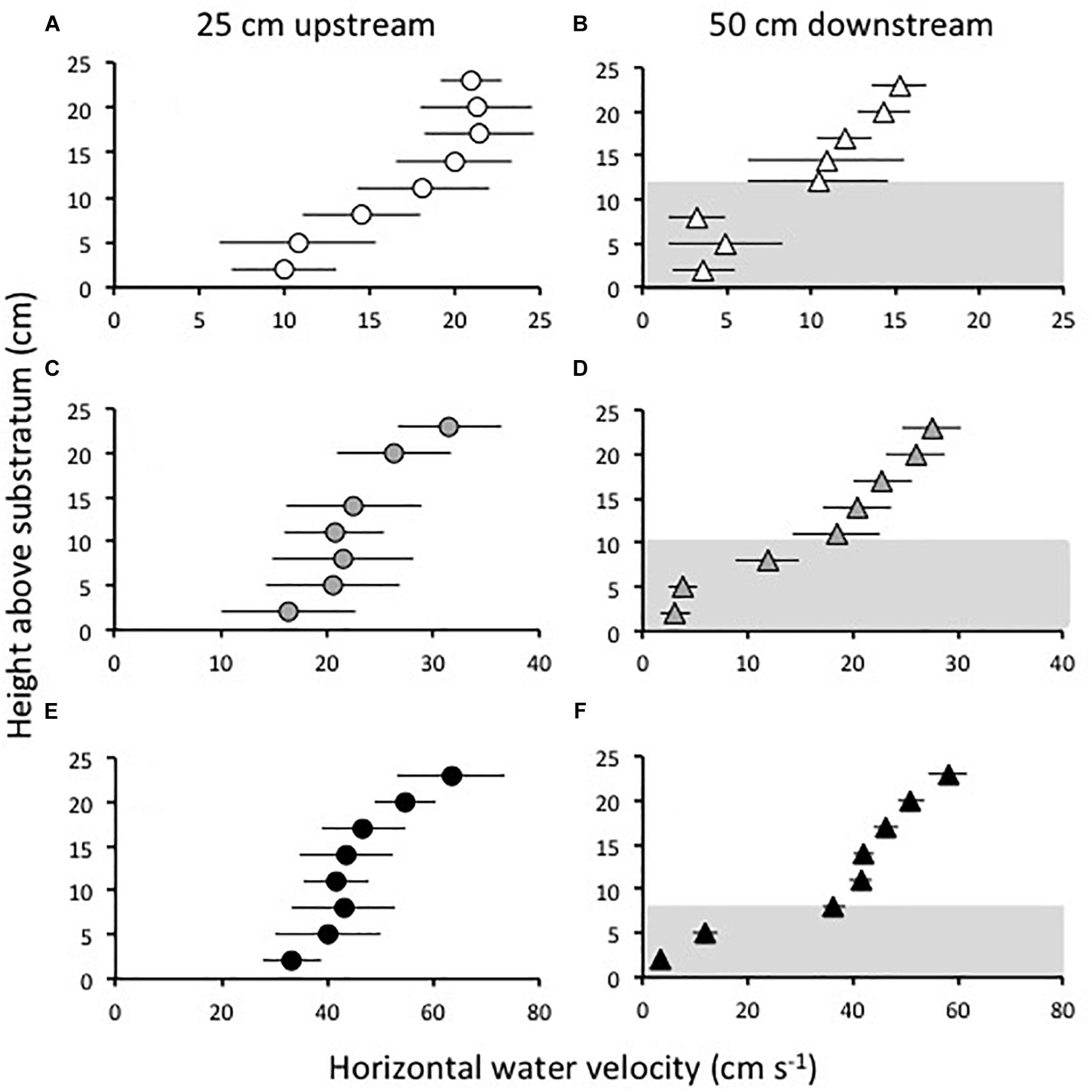
Figure 1. Examples of velocity profiles measured in the flume at a position 25 cm upstream from the leading edge of a grass bed (A,C,E) with a density of 646 shoots m−2, and at a position 50 cm downstream of the leading edge of the bed (B,D,F), when the current velocity in the flume was “slow” (21 cm s−1) (A,B), “intermediate” (32 cm s−1) (C,D), and “fast” (63 cm s−1) (E,F). Symbols show mean horizontal velocities measured at different heights above the substratum and error bars show one standard deviation. The velocities measured above the substratum within the grass canopy are indicated in gray at the position 50 cm downstream from the leading edge of the bed. Shoots are bent over farther in faster flow, compressing the canopy. Evidence of this canopy compression is the reduction of canopy height at higher ambient current velocities (compare B,D,F).
The height of the seagrass canopy above the substratum was measured through a glass panel in the side of the flume. Canopy heights were measured at both the 50 and 100 cm positions downstream of the leading edge of the grass bed for every shoot density and water speed tested. A ruler was set against the glass panel and the time averaged height of the deflected canopy visually estimated to the nearest cm. The canopy heights were used to determine which positions above the substratum in each velocity profile were within or above the canopy. In every case, the heights at which flow was measured that were within the canopy were 2, 5, 8, and in some lower velocities, 11–13 cm above the substratum, while all the other heights at which flow was measured were above the canopy.
For each shoot density and freestream current speed tested, the reduction in flow velocity within the grass bed canopy was determined at the positions 50 and 100 cm downstream from the leading edge of the bed. The mean flow velocity at each height within the canopy was determined for each treatment, as was the mean velocity at the same height at the position 25 cm upstream from the canopy. The percent reduction in flow within the canopy was then calculated for each height at each downstream position as described by Fonseca and Koehl (2006) and Fonseca et al. (2007). The mean % reduction in velocity for all the heights within the canopy was then determined for each downstream position (50 and 100 cm) in the canopy.
Turbulent eddies stir the water, thereby enhancing mixing of substances carried by the water and reducing their local depletion or accumulation in the water around organisms. Turbulence intensity is a dimensionless number that is a measure of the importance of velocity fluctuations (due to turbulence) relative to the average current velocity. Arrays of plants can decrease turbulence intensity of ambient flow by damping out fluid motion, or can increase turbulence intensity by shedding vortices or fluttering as the water flows past them. Comparison of the turbulence intensity of the ambient water flow outside of arrays of macrophytes with that of the flow inside the canopies has long been used to assess the effects of those canopies on the level of turbulence in the water (e.g., Anderson and Charters, 1982; Koehl and Alberte, 1988; Gambi et al., 1990; Worcester, 1995). So that we could compare our results to those of such earlier studies, we used our measurements of flow velocity as a function of time at each height (h) within the canopy to calculate the turbulence intensity at that height (Ih), as defined in Gambi et al. (1990):
where rmsUh is the root mean square of the velocity at height h (in our study velocities were measured at 0.02 s intervals for a duration of 30 s, and rmsUh was computed using the RMSE function in Sas Institute Inc, 2002), and ûh is the mean of the velocities measured over that 30 s period at height h. We calculated the Ih ’s for each of the heights at the positions in a grass bed that were 0.50 and 1.00 m downstream from the leading edge of the bed.
Canopy Deflection and Gap Area
For each experimental grass bed tested, the lengths of 10 arbitrarily selected shoots were measured to the nearest millimeter to determine mean grass length. For each current speed tested, the ratio of canopy height to mean shoot length was used as a measure of canopy deflection.
When shoots are randomly arranged in a seagrass bed, there can be gaps in the canopy. However, when flexible grass canopies are bent over in flowing water, the blades can cover gaps in the canopy and separate the slower flow within the canopy from the faster flow above it. We evaluated the gaps in the test grass beds at each shoot density and freestream current velocity by taking digital images of the entire test bed from above the flume. A downward-facing digital camera was positioned at a fixed point above the middle of the grass bed while the current was flowing and the entire bed was captured in a single digital image. Point Count software (Kohler and Gill, 2006) was utilized to randomly select 100 points in each image. The number of points on seagrass (as opposed to gaps, i.e., flume floor visible between regions of grass) grass were tallied for each photograph and used to calculate the percent canopy gap closure (PCG) as the percent of points falling on seagrass.
Data Analysis
At each ambient freestream flow velocity (21, 32, or 63 cm s−1) and position in the grass bed (0.50 or 1.00 m downstream from the leading edge of the bed), we tested whether shoot density or percent canopy closure affected three dependent variables: (1) percent reduction in current velocity within the canopy, (2) turbulence intensity within the canopy, and (3) drag force exerted on a shoot. For each flow velocity and shoot density, we also tested whether downstream position in the grass bed affected these three dependent variables.
One-way ANOVAs were performed after log transformation to satisfy assumptions of normality in order to test the effects of current speed, shoot density, PCG, and distance into the test canopy on percent flow reduction, turbulence intensity and force on individual shoots. Because of flow continuity, there can be no expectation of independence among measurements in the flume, thus freeing us to use this statistic as a tool to determine the change in velocities specifically as the result of non-independent factors (Fonseca and Koehl, 2006). Because we were interested in flow conditions within the entire canopy, our statistical tests of percent flow reduction and turbulence intensity utilized means of these parameters at each discrete elevation within the canopy as replicates. Percentages were arcsine transformed prior to application of ANOVA. Differences among means were determined by Duncan’s multiple range test [DMRT] to guard conservatively against Type I error.
Results
Shoot Characteristics
Average leaf length from five randomly selected Z. marina shoots was 103.8 mm (s.d. 40.7), sheath length was 25.6 mm (s.d. 6.2), and leaf and sheath width were 3.2 mm (s.d. 0.45). There was no significant difference in shoot total length (sheath + leaf) between shoots collected from the two field sites (ANOVA, df = 1, F = 0.03, p = 0.861).
The flexural stiffness (EI) of sheaths of Z. marina shoots (mean = 1.89 × 10−6 N m−2, s.d. = 1.13 × 10−6, n = 12 shoots) was approximately seven times higher than the EI of individual leaves (mean = 2.84 × 10−7 N m−2, s.d. = 1.99 × 10−7, n = 24 leaves, each from a different shoot) This difference in stiffness was significant (ANOVA, df = 1, F = 47.51, p < 0.0001). Flexural stiffnesses of both leaves and sheaths were not significantly different between the two field sites (Table 1), indicating that all plants used in this study should have responded similarly to water flow.
Force on Shoots
There were no significant difference in the force exerted on shoots across current speeds as a function of shoot density (1246, 1046, 846, 646, 448, and 246 shoots m−2), nor was there a suggestion of a trend (Table 2). There was no significant difference in drag force exerted on shoots as a function either of distance into the canopy or of current speed (Table 2).
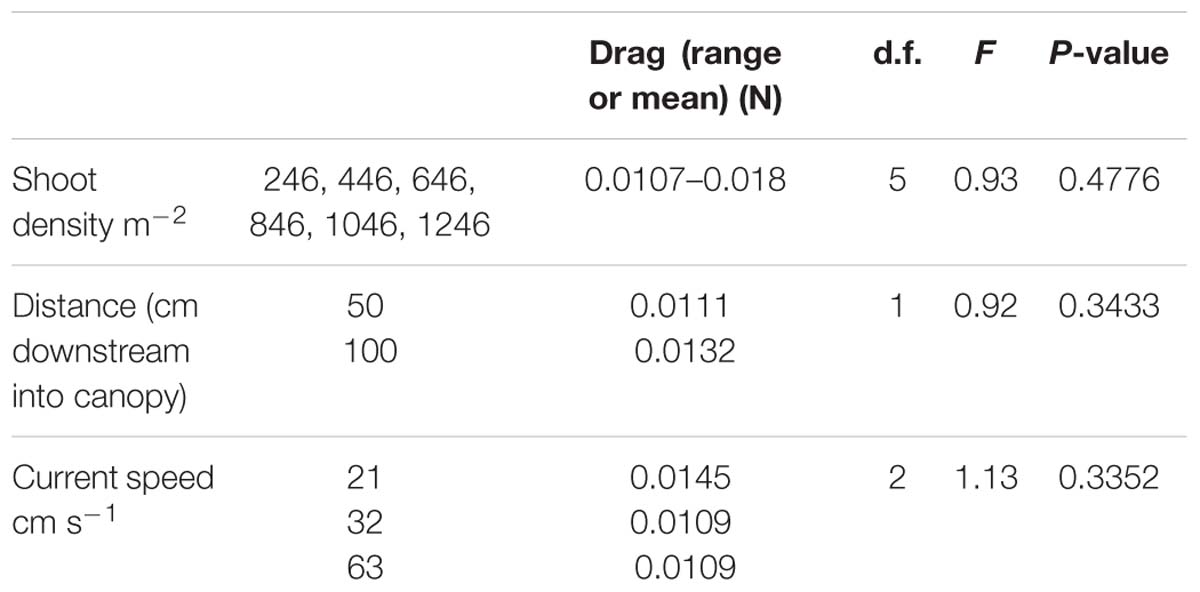
Table 2. Drag force on shoots as a function of shoot density, distance downstream into the canopy, and freestream current speed.
Percent Reduction in Current Speed
There were no significant differences in the effect of shoot density on percent current speed reduction among distances downstream into the canopy if all current speeds were pooled (p > 0.05) (Figures 2A,B). Similarly, there was no effect of shoot density on percent current speed reduction for any of the three current velocities tested (Figures 3A–C). There was some indication that the lowest shoot density (246 shoots m−2) was tending toward a diminished capacity to reduce current velocity at the 32 and 63 cm s−1 speeds, but the variability of percent current velocity reduction within the canopy environment resulted in a non-significant difference.
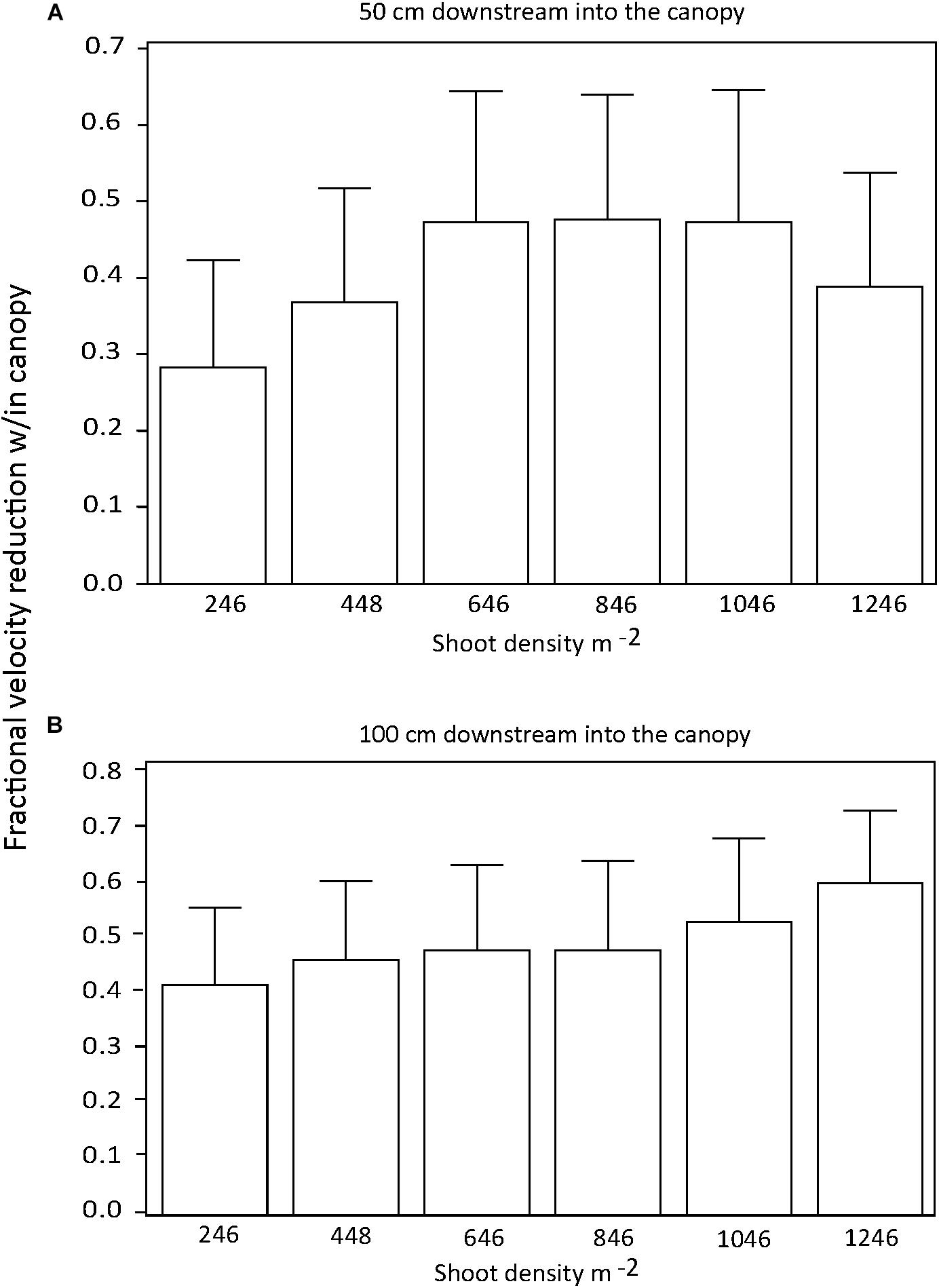
Figure 2. Percent (fractional) reduction in current speed within the seagrass canopy as compared to an upstream location (−25 cm) for 50 (A) and 100 cm (B) downstream into test canopies.
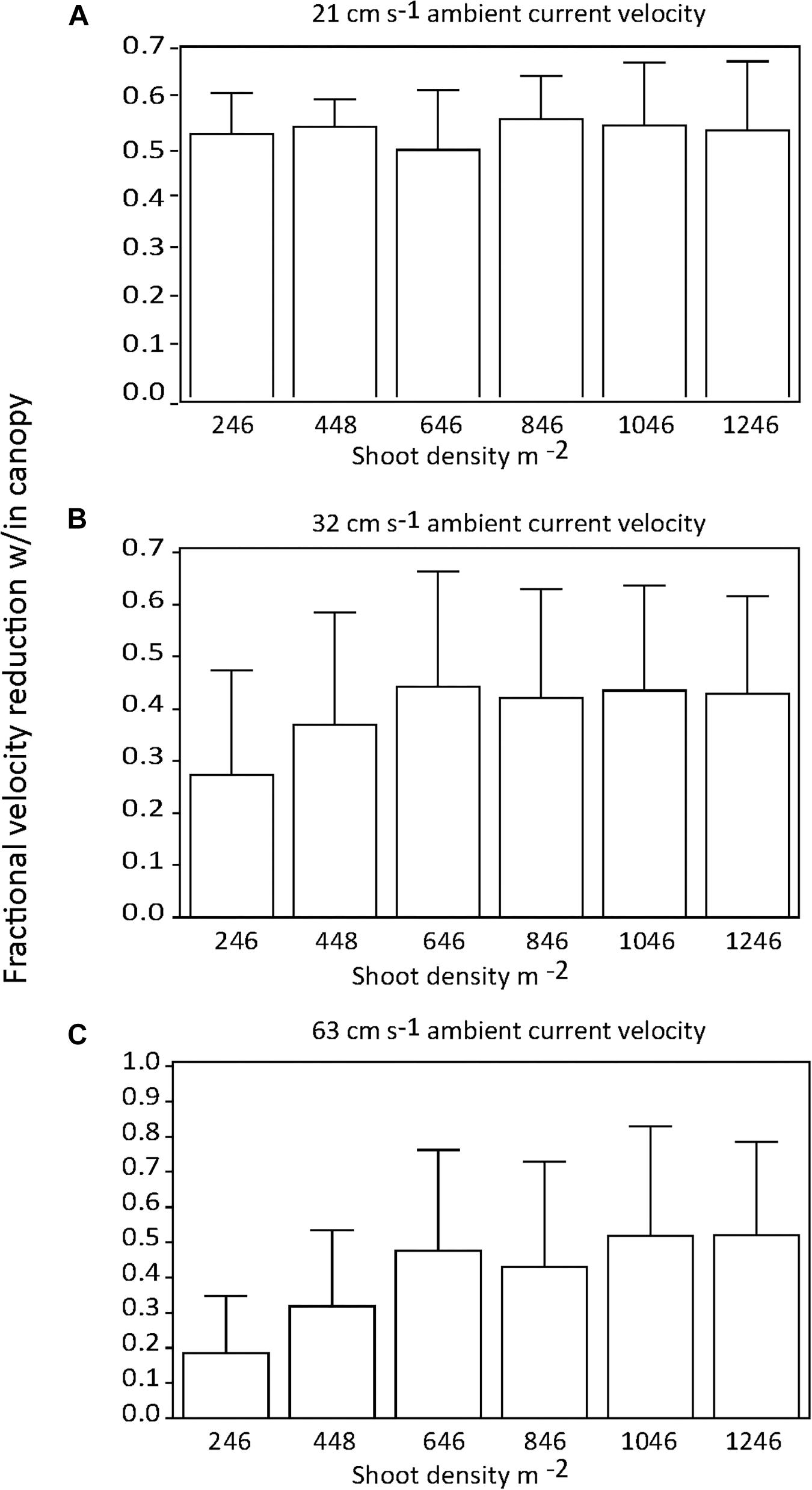
Figure 3. Percent (fractional) reduction in current speed within the seagrass canopy as compared to an upstream location (−0.25 m) for each shoot density by current regime tested; (A) = 21, (B) = 32, and (C) = 63 cm s−1.
Turbulence Intensity
There were no significant differences in the effect of shoot density on turbulence intensity among distances downstream into the canopy (p > 0.05; Figures 4A,B). Similarly, there was no consistent effect of shoot density on turbulence intensity for any of the three current velocities tested (Figures 5A–C). At the mid-range current speed (32 cm s−1), some shoot densities were significantly different from others, but there was no systematic (with shoot density) pattern to these differences (Figure 5B). The faster the current speed, the lower the turbulence intensity within the canopy (Figures 5A–C).
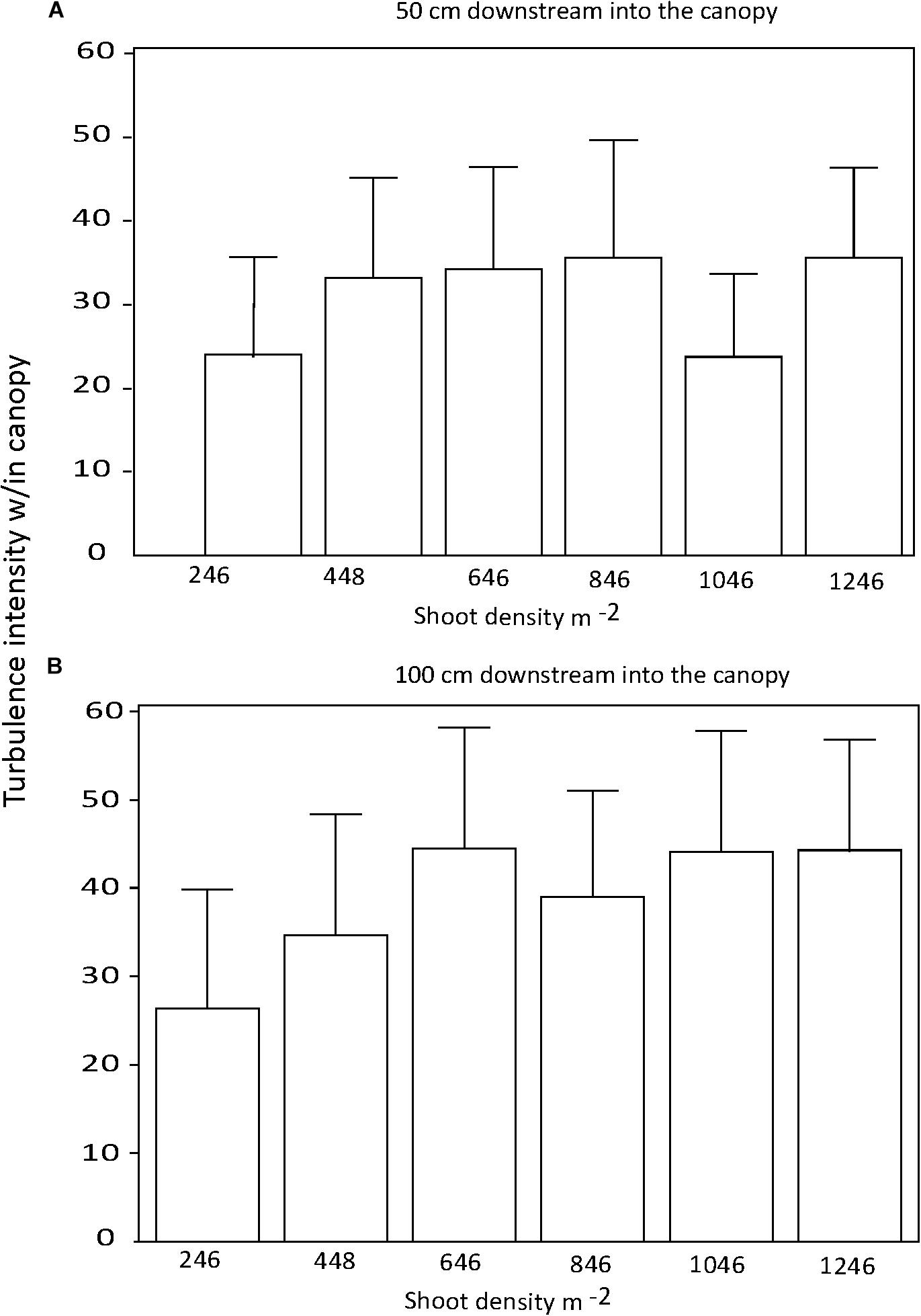
Figure 4. Turbulence intensity within the seagrass canopy for 0.5 (A) and 1.0 m (B) downstream into test canopies.
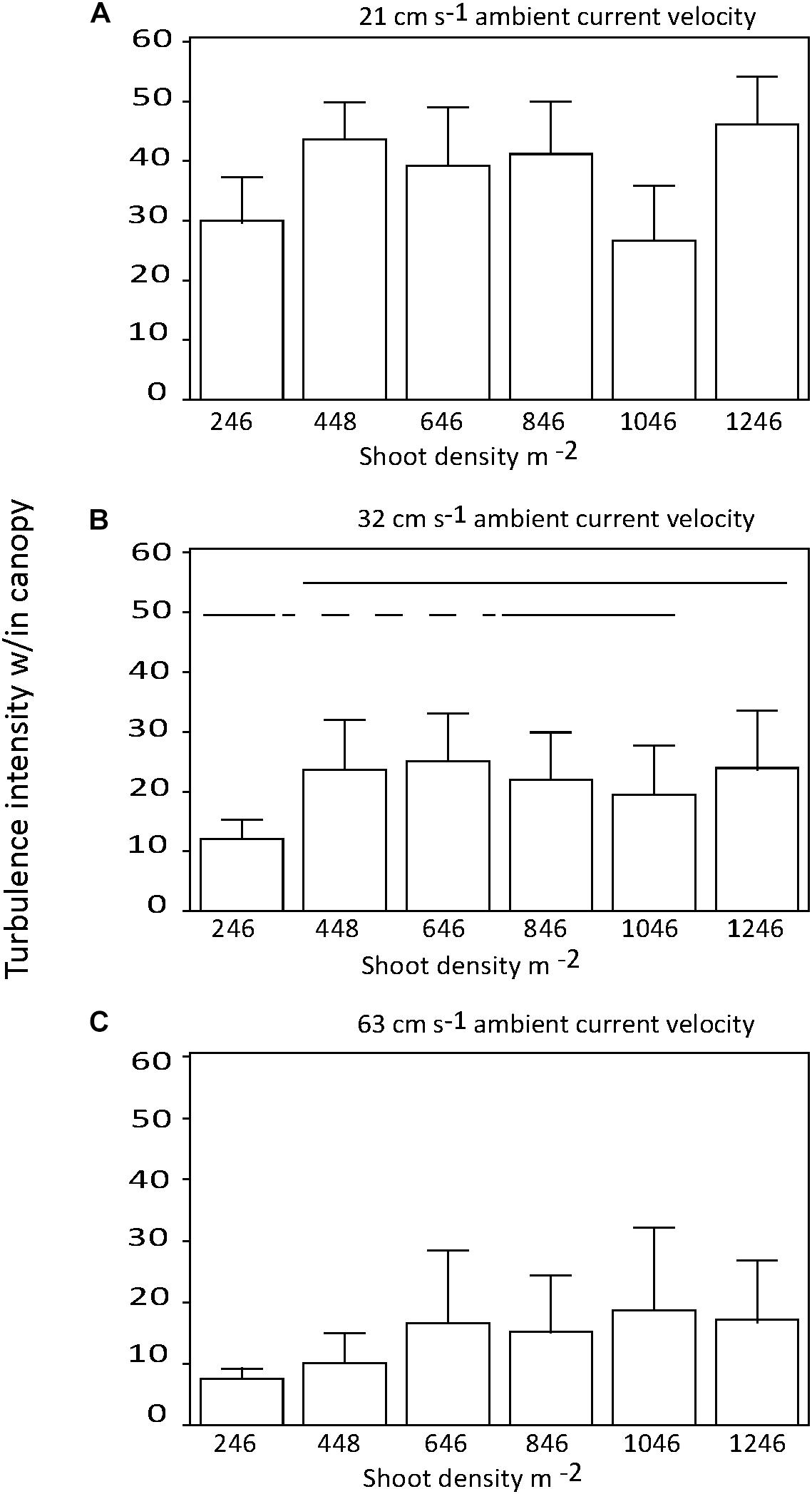
Figure 5. Turbulence intensity within the seagrass canopy for each shoot density by current regime tested; (A) = 21, (B) = 32, and (C) = 63 cm s−1. Horizontal solid lines show treatments that are not significantly different; dashed lines cross significantly different treatments to join equivalent treatments.
Canopy Gap Closure
Percent canopy gap closure (PCG; shown as a fractional change) increased with shoot density irrespective of current regime (Figure 6A); however, there was no significant difference in PCG among the five lowest shoot densities and limited overlap in non-significant differences among the highest shoot densities, despite increased shoot bending and canopy compression (Figure 1, compare Figures 1B,D,F). There was a stepwise increase in canopy closure with both shoot density and increased current speed, with similar proportions of PCG occurring among current speeds (Figure 6B). Current speed resulted in significant (p < 0.05) differences in PCG only among the highest and lowest speeds tested (mean PCG at 21 cm s−1 was 20.3% [n = 6; s.d. 10.4], at 32 cm s−1 was 32% [n = 6; s.d. 15.9], and at 63 cm s−1 was 41% [n = 6; s.d. 23.9]).
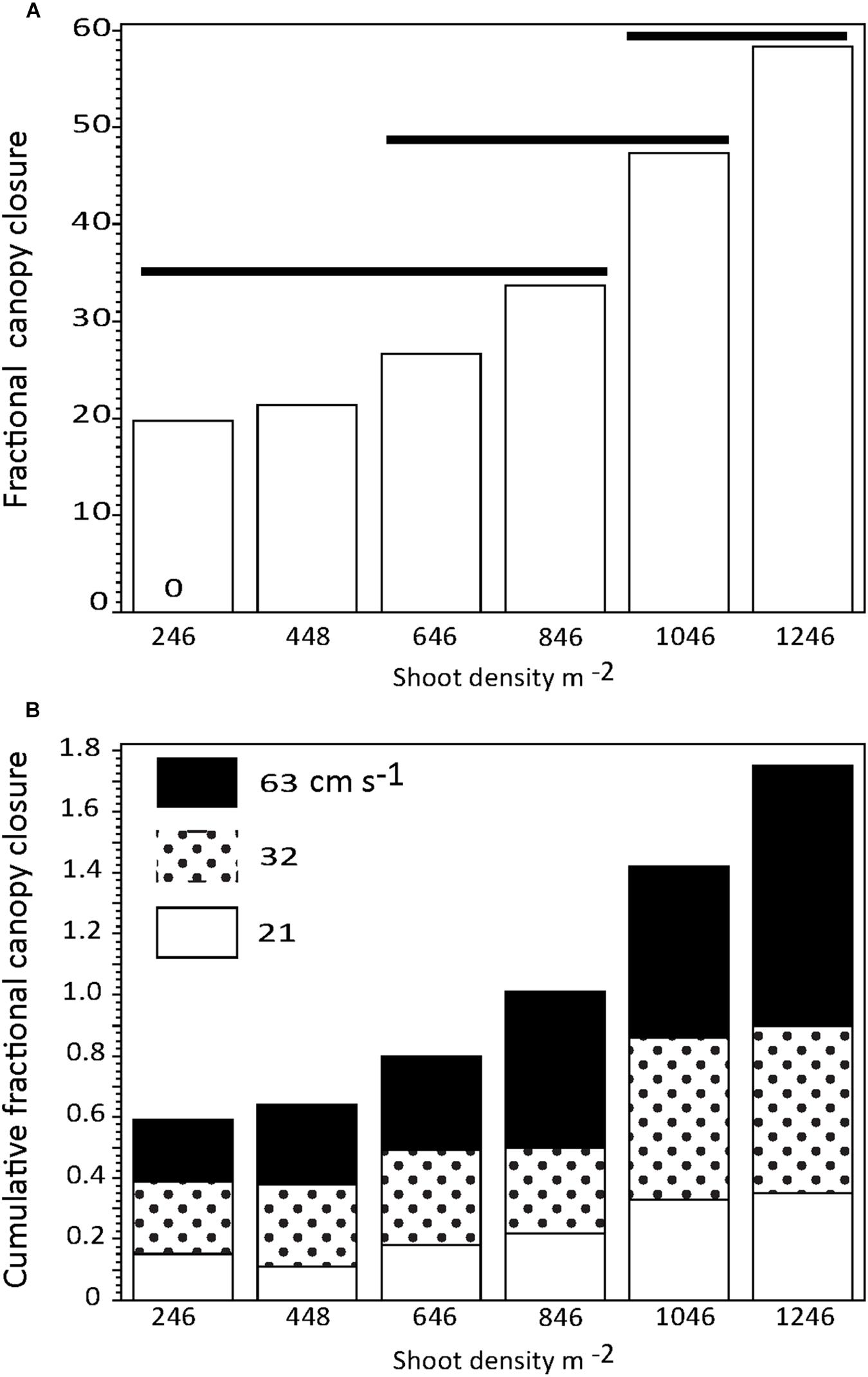
Figure 6. (A) Percent canopy closure as a function of Z. marina shoot density irrespective of current speed. (B) Cumulative percent canopy closure by Z. marina shoot density showing the relative effects ofcurrent speed. Horizontal solid lines show treatments that are not significantly different.
Discussion
Canopies of aquatic plants play important roles in marine and freshwater ecosystems, such as providing food and habitat for animals, and stabilizing and altering the chemistry of sediments. The performance of these functions by canopies of seagrasses or macroalgae depends on their interaction with the ambient water flow. We have focused on seagrass beds exposed to unidirectional water currents to examine the roles of shoot density and flexibility in affecting the hydrodynamics of submerged aquatic plant canopies.
Shoot Density
Many studies have revealed that the vertical velocity profile and turbulence structure of a unidirectional water current are altered upon encounter with a seagrass bed, where a zone of rapid flow above the canopy and a zone of slower flow within the bed develop (Fonseca et al., 1982, 1983, 2007; Fonseca and Kenworthy, 1987; Gambi et al., 1990; Ackerman and Okubo, 1993; Worcester, 1995; Koch and Gust, 1999; Verduin and Backhaus, 2000; Abdelrhman, 2003; Peterson et al., 2004; Chen et al., 2013; Figure 1). If a seagrass bed is composed of flexible shoots, they are bent over by flowing water and overlap, thereby forming a compressed canopy. The roles of shoot density and of canopy deflection in determining the degree to which water flow is slowed within and re-directed above a bed of flexible seagrasses has been unclear. Our study of flow through beds of very flexible Zostera marina plants showed that shoot density had little influence on flow reduction and turbulence intensity at each downstream position in the bed and at each current velocity that we tested. Although our data hinted that the capacity of a grass bed to reduce flow velocity might be lower for the sparsest shoot densities we tested when exposed to the most rapid ambient currents we imposed, this result was not significant. Our data do not show the dramatic reductions in flow within a canopy as shoot density increases that were predicted by Abdelrhman (2003), who assumed that the simple displacement of water by shoots would limit water movement within the canopy. Instead, our data suggest that the effect of shoot density on velocity reduction was largely eclipsed by other changes in the canopy architecture due to flow-induced bending of flexible shoots and the resulting canopy compression. Furthermore, our data suggests that the sedimentation that occurs in the slowed flow in a grass bed, and thus carbon deposition and sediment organic content, would be facilitated across much of the range of Z. marina shoot densities observed in nature.
Shoot Flexibility
The inconsistent results of different studies of the effects of shoot density on the hydrodynamics of seagrass beds exposed to unidirectional currents can be explained in part by differences in the flexibility of the shoots or physical models composing the canopy. For example, Chen et al. (2007) adopted Nepf’s (1999) approach of using arrays of rigid cylinders to evaluate the effects of shoot density on flow through and around submerged plant canopies and found that increasing shoot density decreased water velocities in the canopy. In contrast, we found that shoot density had little effect on flow reduction in canopies of flexible seagrass. Dijkstra and Uittenbogaard (2010), who used both mathematical modeling and flume experiments with plastic mimics of shoots of different stiffness to determine water flow in canopies, discovered that shoot stiffness had a bigger effect on flow reduction in the canopy than did shoot density.
When a flexible organism attached to the substratum is bent over by ambient water flow, its projected area normal to the flow direction is reduced and the drag it experiences is lower than drag on a stiff organism of the same size and shape. This drag-reducing function of flexibility has been measured for macroalgae and sessile aquatic animals (e.g., Koehl and Wainwright, 1977; Koehl, 1984, 1986; Koehl et al., 2001), and for seagrass shoots and physical models (e.g., Bouma et al., 2005; Fonseca et al., 2007; Dijkstra and Uittenbogaard, 2010). Such drag reduction enhances the ability of a flexible organism to avoid being ripped off the substratum by ambient water motion. Another advantage of flexibility occurs when groups of attached organisms form canopies. Fonseca and Fisher (1986) found that canopies composed of species of seagrass that had flexible shoots compressed more in flowing water, and thereby provided greater protection from sediment erosion, than did canopies of species with stiffer shoots. Because faster flow causes greater deflection and streamlining of flexible shoots, and thus more canopy compression, it is not surprising that the drag we measured on flexible Z. marina shoots within grass beds was independent of ambient current velocity.
Canopy Configuration and Gap Closure
Gaps between shoots in a canopy can enhance light and flow penetration into the canopy, but when flexible shoots are bent over by flowing water, they can cover gaps. Not unexpectedly, we found that gap closure was greater when canopies of flexible Z. marina were exposed to fast ambient currents than when they experienced slower flow. Furthermore, at each current speed, gap closure was more pronounced in canopies with high shoot densities than in those with low densities. However, canopy gap closure did not correlate with the percent velocity reduction in grass beds or with drag on individual shoots, both of which were independent of ambient current velocity and shoot density. A possible explanation is that the effects of canopy closure on the flow above and through a flexible seagrass canopy were manifested at very low levels of gap closure (∼20% PCG) and at low ambient current speeds (e.g., Fonseca and Kenworthy, 1987). Our result that downstream position had no effect on flow reduction, turbulence intensity, drag on a shoot, and gap closure suggests that the flow structure in a flexible canopy develops quickly with distance as water moves into the canopy, as has been seen in other studies (Fonseca et al., 1982, 2007; Fonseca and Fisher, 1986; Gambi et al., 1990; Abdelrhman, 2003).
The lack of an effect of shoot density on velocity reduction and turbulence intensity in seagrass canopies could help explain why experiments in which shoot density was manipulated on a local patch scale in one hydrodynamic environment did not alter sediment characteristics in seagrass beds (Armitage and Fourqurean, 2016; Howard et al., 2016). This could explain the lack of a relationship between sediment stores of organic carbon and seagrass density that has been observed on a regional scale (Campbell et al., 2015).
Does Ambient Current Speed Affect Shoot Density in Seagrass Beds?
High shoot densities have been observed in grass beds in habitats exposed to rapid water flow (e.g., Conover, 1964; Kenworthy et al., 1982; Fonseca and Bell, 1998). We found that changing the density of flexible shoots does not affect the flow in a grass bed exposed to a given ambient current. Therefore, our results are inconsistent with the hypothesis that high shoot density is due to a direct, adaptive response of the grass to rapid water flow.
Rather than current speed providing some signal that stimulates an increase in shoot density, water flow might affect shoot spacing indirectly via a variety of mechanisms. For example, the accumulation of sediment and its content of nutrients and organic matter all are functions of the depositional environment, and all are drivers of seagrass shoot density (Holmer et al., 2008; Howard et al., 2016; Ceccherelli et al., 2018). In very oligotrophic environments, high nutrient supply increases shoot density (e.g., Fourqurean et al., 1995; Agawin et al., 1996). Similarly, in such resource-limited habitats where nutrients are taken up through seagrass leaves rather than the roots, high flow velocities increase nutrient delivery by making the diffusion boundary layer around the leaves thinner (e.g., Koch, 2001). In contrast, at eutrophic sites where sediment anoxia induces plant stress, slow flow regimes can result in reduced shoot density (e.g., Borum et al., 2005; Holmer et al., 2008; Brodersen et al., 2015). Feedback between a number of interacting environmental factors can also affect the light reaching seagrass, and thus their growth patterns, as described by van der Heide et al. (2011). For example, in habitats exposed to rapid water flow, seagrass canopies trap suspended particles, thereby improving water clarity and enhancing light. In contrast, in estuaries with slow water flow, eutrophication reduces the light reaching the seagrass.
Our data are consistent with the view that the high shoot densities of seagrass canopies in habitats exposed to rapid water flow can be due to the effects of water motion on other factors, such as light and nutrients, that affect seagrass growth.
Author Contributions
MF led the research and oversaw the experimental process and drafted the original manuscript. MK reviewed and revised the manuscript with regard to flow dynamics and interaction with the flexible seagrass shoots. JF reviewed and revised the manuscript with regard to environmental feedbacks and alternative mechanisms influencing seagrass shoot density.
Funding
Support was provided early and in part by the NOAA, Beaufort Laboratory (to MF) and later by CSA Ocean Sciences Inc. (to MF) and by National Science Foundation Grant IOS-1655318 (to MK). This is contribution #123 from the Center for Coastal Oceans Research in the Institute of Water and Environment at Florida International University.
Conflict of Interest Statement
MF was employed by NOAA during the collection of the data and by CSA Ocean Sciences Inc. during the analysis and writing of the manuscript.
The remaining authors declare that the research was conducted in the absence of any commercial or financial relationships that could be construed as a potential conflict of interest.
Acknowledgments
Thanks to L. Rose for providing technical support of the study at the NOAA, Beaufort Laboratory. Early review of this work by the late E. M. Koch and subsequently, G. Piniak, A. Uhrin and three reviewers greatly improved the manuscript.
Footnotes
- ^ Hereafter “shoot density” is in reference to seagrass unless otherwise stated.
References
Abdelrhman, M. A. (2003). Effect of eelgrass Zostera marina canopies on flow and transport. Mar. Ecol. Prog. Ser. 248, 67–83. doi: 10.3354/meps248067
Ackerman, J. D., and Okubo, A. (1993). Reduced mixing in a marine macrophyte canopy. Funct. Ecol. 7, 305–309. doi: 10.3732/ajb.89.7.1119
Adhitya, A. T., Bouma, J., Folkard, A. M., van Katwijk, M. M., Callaghan, D., de Iongh, H. H., et al. (2014). Comparison of the influence of patch-scale and meadow-scale characteristics on flow within seagrass meadows: a flume study. Mar. Ecol. Prog. Ser. 516, 49–59. doi: 10.3354/meps10873
Agawin, N. S. R., Duarte, C. M., and Fortes, M. D. (1996). Nutrient limitation of Philippine seagrasses (Cape Bolinao, NW Philippines): in situ experimental evidence. Mar. Ecol. Progr. Ser. 138, 233–243. doi: 10.3354/meps138233
Anderson, S. M., and Charters, A. C. (1982). A fluid dynamic study of seawater flow through Gelidium nudifrons. Limnol. Oceanogr. 27, 399–412. doi: 10.4319/lo.1982.27.3.0399
Armitage, A. R., and Fourqurean, J. W. (2016). Carbon storage in seagrass soils: long-term nutrient history exceeds the effects of near-term nutrient enrichment. Biogeosciences 13, 313–321. doi: 10.5194/bg-13-313-2016
Borum, J., Pedersen, O., Greve, T. M., Frankovich, T. A., Zieman, J. C., Fourqurean, J. W., et al. (2005). The potential role of plant oxygen and sulphide dynamics in die-off events of the tropical seagrass, Thalassia testudinum. J. Ecol. 93, 148–158. doi: 10.1111/j.1365-2745.2004.00943.x
Bouma, T. J., De Vries, M. B., Low, E., Peralta, G., Tanczos, I. C., van de Koppel, J., et al. (2005). Trade-offs related to ecosystem engineering: a case study on stiffness of emerging macrophytes. Ecology 86, 2187–2199. doi: 10.1890/04-1588
Brodersen, K. E., Nielsen, D. A., Ralph, P. J., and Kühl, M. (2015). Oxic microshield and local pH enhancement protects Zostera muelleri from sediment derived hydrogen sulphide. New Phytol. 205, 1264–1276. doi: 10.1111/nph.13124
Campbell, J. E., Lacey, E. A., Decker, R. A., Crooks, S., and Fourqurean, J. W. (2015). Carbon storage in seagrass beds of Abu Dhabi, United Arab Emirates. Est. Coasts 38, 242–251. doi: 10.1007/s12237-014-9802-9
Carrington, E. (1990). Drag and dislodgment of an intertidal macroalga, consequences of morphological variation in Mastocarpus papillatus. Kutzing. J. Exp. Mar. Biol. Ecol. 139, 185–200. doi: 10.1016/0022-0981(90)90146-4
Ceccherelli, G., Oliva, S., Pinna, S., Piazzi, L., Procaccini, G., Marin-Guirao, L., et al. (2018). Seagrass collapse due to synergistic stressors is not anticipated by phenological changes. Oecologia 186, 1137–1152. doi: 10.1007/s00442-018-4075-9
Charters, A. C., Neushul, M., and Barilotti, C. (1969). The functional morphology of Eisenia arborea. Proc. Intl. Seaweed Symposium 6, 89–105.
Chen, S., Sanford, L., Koch, E. W., Shi, F., and North, W. W. (2007). A nearshore model to investigate the effects of seagrass bed geometry on wave attenuation and suspended sediment transport. Est. Coasts 30, 296–310. doi: 10.1007/bf02700172
Chen, Z., Jiang, C., and Nepf, H. (2013). Flow adjustment at the leading edge of a submerged aquatic canopy. Water Resour. Res. 49, 5537–5551. doi: 10.1002/wrcr.20403
Conover, J. T. (1964). Environmental Relationships of Benthos in Salt Ponds (plant Relationships). Tech. Rep. No. 3, Univ. R.I. Grad. School Oceanogr. Kingston, RI: University of Rhode Island.
Denny, M. W. (1988). Biology and the Mechanics of the Wave-Swept Environment. Princeton, NJ: Princeton University Press.
Denny, M. W., Daniel, T., and Koehl, M. A. R. (1985). Mechanical limits to the size of wave-swept organisms. Ecol. Monogr. 55, 69–102. doi: 10.2307/1942526
Dijkstra, J. T., and Uittenbogaard, R. E. (2010). Modeling the interaction between flow and highly flexible aquatic vegetation. Water Resour. Res. 46:W12547.
Folkard, A. M. (2011). Flow regimes in gaps within stands of flexible vegetation: laboratory flume simulations. Environ. Fluid. Mech. 11, 289–306. doi: 10.1007/s10652-010-9197-5
Fonseca, M. S., and Bell, S. S. (1998). The influence of physical setting on seagrass landscapes near Beaufort North Carolina, USA. Mar. Ecol. Prog. Ser. 171, 109–121. doi: 10.3354/meps171109
Fonseca, M. S., and Fisher, J. S. (1986). A comparison of canopy friction and sediment movement between four species of seagrass with reference to their ecology and restoration. Mar. Ecol. Prog. Ser. 29, 15–22. doi: 10.3354/meps029015
Fonseca, M. S., Fisher, J. S., Zieman, J. C., and Thayer, G. W. (1982). Influence of the seagrass Zostera marina (L.) on current flow. Est. Coast. Shelf Sci. 15, 351–364. doi: 10.1016/0272-7714(82)90046-4
Fonseca, M. S., and Kenworthy, W. J. (1987). Effects of current on photosynthesis and distribution of seagrasses. Aquat. Bot. 27, 59–78. doi: 10.1016/0304-3770(87)90086-6
Fonseca, M. S., and Koehl, M. A. R. (2006). Modeling flow in seagrass canopies: the influence of patch width. Est. Coastal Shelf Sci. 67, 1–9. doi: 10.1016/j.ecss.2005.09.018
Fonseca, M. S., Koehl, M. A. R., and Kopp, B. S. (2007). Biomechanical factors contributing to self-organization in seagrass landscapes. J. Exp. Mar. Biol. Ecol. 340, 227–246. doi: 10.1016/j.jembe.2006.09.015
Fonseca, M. S., Whitfield, P. E., Kelly, N. M., and Bell, S. S. (2002). Modeling seagrass landscape pattern and associated ecological attributes. Ecol. Appl. 12, 218–237. doi: 10.1890/1051-0761(2002)012
Fonseca, M. S., Zieman, J. C., Thayer, G. W., and Fisher, J. S. (1983). The role of current velocity in structuring seagrass meadows. Est. Coast. Shelf Sci. 17, 367–380. doi: 10.1016/0272-7714(83)90123-3
Fourqurean, J. W., Powell, G. V. N., Kenworthy, W. J., and Zieman, J. C. (1995). The effects of long-term manipulation of nutrient supply on competition between the seagrasses Thalassia testudinum and Halodule wrightii in Florida Bay. Oikos 72, 349–358.
Gambi, M. C., Nowell, A. R. M., and Jumars, P. A. (1990). Flume observations on flow dynamics in Zostera marina (eelgrass) beds. Mar. Ecol. Prog. Ser. 61, 159–169. doi: 10.3354/meps061159
Holmer, M., Argyrou, M., Dalsgaard, T., Danovaro, R., Diaz-Almela, E., Carlos, M. D. E., et al. (2008). Effects of fish farm waste on Posidonia oceanica meadows: synthesis and provision of monitoring and management tools. Mar. Pollut. Bull. 56, 1618–1629. doi: 10.1016/j.marpolbul.2008.05.020
Howard, J. L., Perez, A., Lopes, C. C., and Fourqurean, J. W. (2016). Fertilization changes seagrass community structure but not blue carbon storage: results from a 30-year field experiment. Est. Coasts 39, 1422–1434. doi: 10.1007/s12237-016-0085-1
Iwasa, Y., Kazunori, S., and Nakashima, S. (1991). Dynamic modeling of wave regeneration (Shimagare) in subalpine Abies forests. J. Theor. Ecol. 152, 143–158. doi: 10.1016/s0022-5193(05)80448-5
Kenworthy, W. J., Zieman, J. C., and Thayer, G. W. (1982). Evidence for the influence of seagrasses on the benthic nitrogen cycle in a coastal plains estuary near Beaufort, North Carolina. Oecologia 54, 152–158. doi: 10.1007/BF00378387
Koch, E. W. (2001). Beyond light: physical, geological and geochemical parameters as possible submersed aquatic vegetation habitat requirements. Estuaries 24, 1–17.
Koch, E. W., Ackerman, J., Verduin, J., van Keulen, M., Larkum, A., Orth, R., et al. (2006). “Fluid dynamics in seagrass ecology—from molecules to ecosystems,” in Seagrasses: Biology, Ecology and Conservation, ed. R. Joseph Orth (Dordrecht: Springer).
Koch, E. W., and Gust, G. (1999). Water flow in tide- and wave-dominated beds of the seagrass Thalassia testudinum. Mar. Ecol. Prog. Ser. 184, 63–72. doi: 10.3354/meps184063
Koehl, M. A. R. (1976). “Mechanical design in sea anemones,” in Coelenterate Ecology and Behavior, ed. G. O. Mackie (Boston, MA: Springer), 22–31.
Koehl, M. A. R. (1977). Mechanical organization of cantilever-like sessile organisms, sea anemones. J. Exp. Biol. 69, 127–142.
Koehl, M. A. R. (1984). How do benthic organisms withstand moving water? Am. Zool. 24, 57–70. doi: 10.1093/icb/24.1.57
Koehl, M. A. R. (1986). “Seaweeds in moving water: form and mechanical function,” in On the Economy of Plant Form and Function, ed. T. J. Givnish (London: Cambridge University Press), 603–634.
Koehl, M. A. R., and Alberte, R. S. (1988). Flow, flapping and photosynthesis of Nereocystis luetkeana: a functional comparison of undulate and flat blade morphologies. Mar. Biol. 99, 435–444. doi: 10.1007/bf02112137
Koehl, M. A. R., Helmuth, B., and Carpenter, R. (2001). “Growing and flowing,” in The Algorithmic Beauty of Seaweeds, Sponges and Corals, eds J. A. Kaandorp and J. E. Kubler (Heidelberg: Springer-Verlag).
Koehl, M. A. R., and Wainwright, S. A. (1977). Mechanical adaptations of a giant kelp. Limnol. Oceanogr. 22, 1067–1071. doi: 10.4319/lo.1977.22.6.1067
Kohler, K. E., and Gill, S. M. (2006). Coral point count with excel extensions (CPCe): a visual basic program for the determination of coral and substrate coverage using random point count methodology. Comp. Geosci. 32, 1259–1269. doi: 10.1016/j.cageo.2005.11.009
Krause-Jensen, D., Pedersen, M. F., and Jensen, C. (2003). Regulation of eelgrass (Zostera marina) cover along depth gradients in Danish coastal waters. Estuaries 26, 866–877. doi: 10.1007/bf02803345
Lacy, J. R., and Wyllie-Echeverria, S. (2011). The influence of current speed and vegetation density on flow structure in two macrotidal eelgrass canopies. Limnol. Oceanogr. Fluids Environ. 1, 38–55. doi: 10.1215/21573698-1152489
Lei, J., and Nepf, H. (2016). Impact of current speed on mass flux to a model flexible seagrass blade.” J. Geophys. Res. 121, 4763–4776. doi: 10.1002/2016jc011826
Luhar, M., and Nepf, H. M. (2011). Flow-induced reconfiguration of buoyant and flexible aquatic vegetation. Limnol. Oceanogr. 56, 2003–2017. doi: 10.4319/lo.2011.56.6.2003
Nepf, H. M. (1999). Drag, turbulence and diffusion in flow through emergent vegetation. Wat. Res. Res. 35, 470–489.1999. doi: 10.1016/j.watres.2008.10.027
Newell, R. I. E., and Koch, E. W. (2004). Modeling seagrass density and distribution in Response to changes in turbidity stemming from bivalve filtration and seagrass sediment stabilization. Estuaries 27, 793–806. doi: 10.1007/bf02912041
Paul, M., and Gillis, L. G. (2015). Let if flow: how does an underlying current affect wave propagation over a natural seagrass meadow? Mar. Ecol. Prog. Ser. 523, 57–70. doi: 10.3354/meps11162
Peterson, C. H., Luettich, R. A., Micheli, F., and Skilleter, G. A. (2004). Attenuation of water flow inside seagrass canopies of differing structure. Mar. Ecol. Prog. Ser. 268, 81–92. doi: 10.3354/meps268081
Ruiz, J. M., and Romero, J. (2001). Effects of in situ experimental shading on the Mediterranean seagrass Posidonia oceanica (l.) Delile. Mar. Ecol. Prog. Ser. 215, 107–120. doi: 10.3354/meps215107
Short, F. T. (1975). Eelgrass Production in Charlestown Pond: An Ecological Analysis and Simulation Model. M.S. Thesis, University of Rhode Island, Kinston, RI, 180.
Short, F. T., Burdick, D. M., and Kaldy, J. E. (1995). Mesocosm experiments quantify the effects of eutrophication on eelgrass, Zostera marina. Limnol. Oceanogr. 40, 740–749. doi: 10.4319/lo.1995.40.4.0740
Sprugel, D. G. (1976). Dynamic structure of wave-regenerated Abies balsamea forests in the north-eastern United States. J. Ecol. 64, 889–911.
Stewart, H. L. (2004). Hydrodynamic consequences of maintaining an upright posture by different magnitudes of stiffness in buoyancy in the tropical alga Turbinaria ornata. J. Mar. Syst. 49, 157–167. doi: 10.1016/j.jmarsys.2003.05.007
Valentine, J. F., Heck, K. L., Harper, P., and Beck, M. (1994). Effects of bioturbation in controlling turtlegrass Thalassia testudinum (Banks ex Konig) abundance: evidence from field enclosures and observations in the Northern Gulf of Mexico. J. Exp. Mar. Biol. Ecol. 178, 181–192. doi: 10.1016/0022-0981(94)90035-3
van der Heide, T., van Nes, E. H., van Katwijk, M. M., Olff, H., and Smolders, A. J. P. (2011). Positive feedbacks in seagrass ecosystems – evidence from large-scale empirical data. PLoS One 6:e16504. doi: 10.1371/journal.pone.0016504
Veblen, T. T. (1985). “Stand dynamics in Chilean Nothofagus forests,” in The Ecology of Natural Disturbance and Patch Dynamics, eds P. S. White and S. T. A. Pickett (Cambridge, MA: Academic Press), 35–51. doi: 10.1016/b978-0-08-050495-7.50008-9
Verduin, J. J., and Backhaus, J. O. (2000). Dynamics of plant-flow interactions for the seagrass Amphibolis antarctica: field observations and model simulations. Est. Coast. Shelf Sci. 50, 185–204. doi: 10.1006/ecss.1999.0567
Wainwright, S. A., Biggs, W. D., Currey, J. D., and Gosline, J. M. (1976). Mechanical Design in Organisms. New York: John Wiley and Sons.
Keywords: seagrass, current speed, shoot density, flexibility, canopy, gaps
Citation: Fonseca MS, Fourqurean JW and Koehl MAR (2019) Effect of Seagrass on Current Speed: Importance of Flexibility vs. Shoot Density. Front. Mar. Sci. 6:376. doi: 10.3389/fmars.2019.00376
Received: 06 September 2018; Accepted: 17 June 2019;
Published: 09 July 2019.
Edited by:
Virginia B. Pasour, Army Research Office, United StatesReviewed by:
Lucy Gwen Gillis, Leibniz Centre for Tropical Marine Research (LG), GermanyJennifer Joan Verduin, Murdoch University, Australia
Free Espinosa Torre, University of Seville, Spain
Copyright © 2019 Fonseca, Fourqurean and Koehl. This is an open-access article distributed under the terms of the Creative Commons Attribution License (CC BY). The use, distribution or reproduction in other forums is permitted, provided the original author(s) and the copyright owner(s) are credited and that the original publication in this journal is cited, in accordance with accepted academic practice. No use, distribution or reproduction is permitted which does not comply with these terms.
*Correspondence: Mark S. Fonseca, bWZvbnNlY2FAY29uc2hlbGYuY29t