- 1Department of Biological Sciences, William & Mary’s Virginia Institute of Marine Science, Gloucester Point, VA, United States
- 2Bodega Marine Laboratory, University of California, Davis, Davis, CA, United States
- 3Department of Environmental Science and Policy, University of California, Davis, Davis, CA, United States
- 4Department of Biology, James Madison University, Harrisonburg, VA, United States
- 5Department of Evolution and Ecology, University of California, Davis, Davis, CA, United States
Human-caused environmental change will have significant non-lethal and indirect impacts on organisms due to altered sensory pathways, with consequences for ecological interactions. While a growing body of work addresses how global ocean change can impair the way organisms obtain and use information to direct their behavior, these efforts have typically focused on one step of the pathway (e.g., reception of a cue/signal), one sensory modality (e.g., visual), or one environmental factor (e.g., temperature). An integrated view of how aspects of environmental change will impact multiple sensory pathways and related ecological processes is needed to better anticipate broader consequences for marine ecosystems. Here, we present a conceptual synthesis of effects of global change on marine sensory ecology, based on a literature review. Our review supports several predictions for how particular sensory pathway steps – production, transmission, and reception/processing of cues/signals – are affected by environmental change. First, the production and reception/processing of multiple modalities of cues/signals are vulnerable to multiple global change stressors, indicating that there are generalizable mechanisms by which environmental change impairs these pathways steps, leading to altered sensory pathway outcomes. Factors that enhance organismal stress as a whole may amplify impacts to these sensory pathways. Second, global change factors tend to affect specific modalities of cue/signal transmission. Consequently, local impacts on ecological processes linked with cue/signal transmission will vary depending on environmental stressor(s) present and the corresponding sensory modality. Finally, because many ecological and evolutionary interactions rely on sensory processing, impairment of sensory pathways may frequently underpin impacts of global ocean change on marine ecosystems. Effects on individual sensory processes will integrate to shape processes like mating, predation, and habitat selection, and we highlight new insights on impacts to ecological interactions by employing our mechanistic conceptual framework.
Introduction
Marine organisms access and react to diverse types of information about biotic and abiotic attributes of their surroundings (Dall et al., 2005; Schmidt et al., 2010). Different modalities, including visual, chemical, mechanical, and auditory modalities, are avenues of sensory information (see review by Nagelkerken et al., 2019). Such information is partitioned into cues and signals. Cues include both passively occurring abiotic information (e.g., temperature) as well as information released unintentionally by organisms. In contrast, signals are deliberately produced by organisms and have evolved to elicit a response from the receiver, defined as a recipient organism (Schmidt et al., 2010). The presence, strength, and variation of cues and signals can aid organisms in decision-making (Kingsford et al., 2002) and can therefore critically mediate many ecological processes in the marine realm, such as predator-prey interactions, mate choice, habitat selection, aggregation activities, long distance navigation, and larval settlement (Zimmer and Butman, 2000; Connaughton et al., 2002; Kingsford et al., 2002; Lohmann et al., 2008; Strod et al., 2008; Hay, 2009; Munday et al., 2009). Consequently, changes in availability and use of cues/signals will have ramifications that could manifest at scales from individuals to ecosystems (Schmidt et al., 2010; Wong and Candolin, 2015; Nagelkerken and Munday, 2016). Marine sensory ecology – the study of how marine organisms obtain and use information to direct their behavior and how this information influences the interactions and distributions of species – can provide a lens through which to examine the many underexplored non-lethal and indirect impacts of global ocean change (Gilman et al., 2010; Schmidt et al., 2010).
Organisms disseminate and gather information from their environment using sensory pathways composed of four steps: production, transmission, reception/processing, and response (Figure 1), and these steps serve as the framework for our literature review and discussion. We use the term pathway to refer to these four steps, which is a distinct and separate definition from a physiological pathway composed of various biomolecules and contained within an organism. Sensory pathways involve multiple organisms as well as the environment as a medium for information transfer. Each sensory pathway begins with production, the process by which cues and signals are generated. Next, transmission occurs, where cues/signals propagate through the environment to the recipient organism (reviewed by Nagelkerken et al., 2019). During reception/processing, the sensory systems of the recipient organism detect the cues/signals, which then undergo neurobiological processing reliant on molecular and electrochemical mechanisms. The sensory pathway concludes with a response, a physiological, morphological, or behavioral reaction (see excellent books: Atema et al., 1988; Bradbury and Vehrencamp, 1998; Collin and Marshall, 2003). Consider the interaction between grazing snails (Tegula funebralis) and predatory sea stars (Pisaster ochraceus) in the marine intertidal zone as a model example of how a sensory pathway operates. Sea star predators produce waterborne chemical cues when they are immersed in tidepools, likely an unintentional consequence of feeding, metabolism, and/or excretion. As these cues mix through the water, they are transmitted to the recipient organism (the snail), where they are detected by sensory receptors and then processed neurologically. An anti-predatory response then ensues, whereby snails crawl out of the tidepools to enter a refuge from predation (Bullock, 1953; Jellison et al., 2016). Sensory pathways can also be more complex if they incorporate information from multiple modalities (Figure 1). The generalist strategy of cue switching or compensation allows organisms to sustain appropriate decision-making when sensory function for one modality is impaired or one type of cue is degraded or less available. In lieu of the impaired sensory modality, the organism can employ an alternative sensory modality to inform the same decision-making process (i.e., predator avoidance; Hartman and Abrahams, 2000; Leahy et al., 2011).
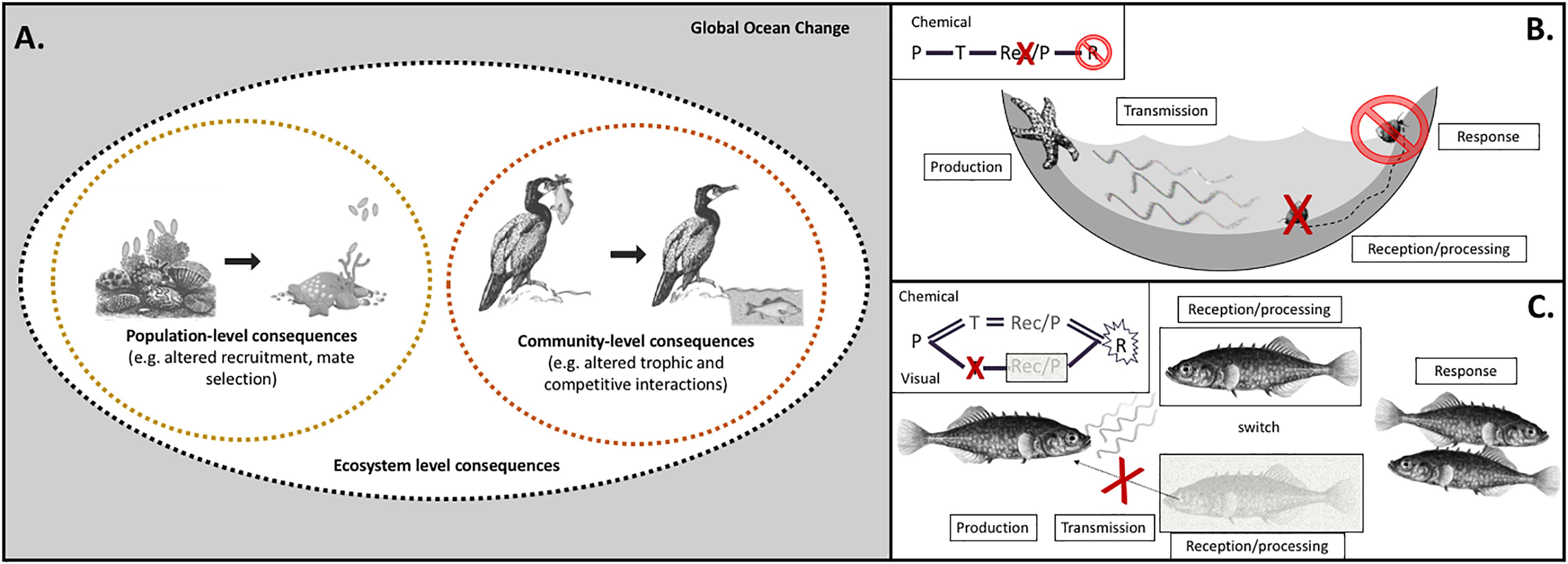
Figure 1. Climate change factors affect individual steps of sensory pathways, with cascading impacts on ecological processes at scales of populations, communities, and ecosystems. (A) The sensitivity of individual pathway steps, as well as how effects on multiple steps combine to shape behavioral outcomes, mediates impacts of climate change on sensory ecology, with both population- and community-level consequences. Global climate change is composed of a suite of environmental factors in marine habitats that are shifting (gray box): temperature, carbonate chemistry, oxygen, salinity, ultraviolet radiation, turbidity, hydrodynamics, stratification, and nutrients. Ecological impacts of global change, both at the population and community levels, are mediated by sensory pathways of specific modalities (e.g., olfaction, vision). For example, disruption of production of olfactory cues may cause recruitment dynamics of coral larvae to change, resulting in population-level consequences. Disruption of visual cues alters predation success of cormorants, with consequences for dynamics of ecological communities. (B) Climate change factors impact sensory pathways at individual step(s) – production (P), transmission (T), reception/processing (Rec/P), which leads to an impaired response (R). For turban snails, ocean acidification disrupts the reception/processing of chemical cues produced by sea star predators. Instead of crawling out of the tidepool to escape predation, the snails cannot respond appropriately to the cue and experience higher mortality rates (Jellison et al., 2016). (C) Species that can compensate by switching to a different modality may be more resilient under climate change. When the transmission of visual cues is impaired, stickleback can switch to rely on olfactory cues for mate selection (Heuschele et al., 2009). Our framework addresses the existing need for a mechanistic articulation of the interaction between the environment, the cue/signal producer, and the recipient organism to better predict ecological consequences of climate change.
Human impacts on the environment may challenge the function of sensory pathways. Anthropogenic greenhouse gas emissions are altering the Earth’s climate, leading to rapid, sustained global ocean change. Sea surface temperature has warmed by ∼0.1°C per decade since 1971 (IPCC, 2013). Consequently, major ocean circulation systems and climatic oscillations have shifted in strength and location, with associated changes in hydrodynamics, upwelling events (e.g., Sydeman et al., 2014), and stratification (e.g., Capotondi et al., 2012). As the upper ocean stratifies, subsurface oxygen concentrations are decreasing, and oxygen minimum zones are expanding (Bograd et al., 2008; Keeling et al., 2010). The global ocean is also absorbing ∼30% of anthropogenic CO2 (Sabine et al., 2004), causing fundamental changes in seawater carbonate chemistry (IPCC, 2013). For example, surface ocean pH has decreased by 0.1 units since the Industrial Revolution (Doney et al., 2008). Emissions of other greenhouse gases are depleting stratospheric ozone, which increases the flux of biologically damaging ultraviolet radiation (UVB) to Earth’s surface and to ecologically relevant depths of the ocean (Smyth, 2011; IPCC, 2013). Additionally, the Earth’s water cycle is shifting as climate changes, resulting in long-term perturbations to cloud cover, precipitation patterns, and sea ice extent (IPCC, 2013). Altered precipitation patterns will affect coastal salinity, turbidity, and inputs of terrestrial-derived nutrients.
Anthropogenic environmental changes described above occur at the global ocean scale, but it is ultimately their effects on individuals, populations, and species across a variety of spatial and temporal scales that will influence ecological processes (Figure 1). In general, these consequences of climate change fall into four categories: migration, acclimatization, evolution, and extinction (O’Connor et al., 2012). Anticipating ecological impacts is not straightforward because different environmental factors may work in different ways to affect sensory pathways. For example, two co-occurring environmental stressors may have antagonistic effects on the same sensory pathway, as has been shown for temperature and ocean acidification (Nagelkerken and Munday, 2016). Alternatively, multiple environmental stressors may act synergistically on sensory pathways. Furthermore, as noted above, organisms may be able to compensate for an impaired sensory pathway by switching cue modalities, allowing them to still gain relevant environmental information and respond appropriately (Figure 1). Therefore in order to anticipate the impacts of global change on marine ecosystems, it is crucial to understand the effects of multiple, co-occurring environmental stressors on the sensory pathways of marine organisms.
Research in marine sensory ecology has expanded rapidly since it was first discussed in the literature (from 426 papers in the 1970s to 493 additional papers in 2017 alone, Web of Science, search term: marine sensory ecology). Likewise, the role of environmental conditions, including anthropogenic changes to them, in modulating the outcome of species interactions has been discussed with increasing frequency (e.g., Sih et al., 2011; Stevens, 2013; Gaylord et al., 2015; Nagelkerken and Munday, 2016; Kelley et al., 2018). Recent reviews include qualitative coverage of particular anthropogenic stressors (e.g., temperature and ocean acidification; Nagelkerken and Munday, 2016) or impacts on individual steps of the sensory pathway (i.e., production and transmission; Nagelkerken et al., 2019). Nagelkerken et al. (2019) focus on how temperature, electromagnetism, and salinity, as well as auditory, chemical, and visual cues, are changing at various spatial and temporal scales in the marine environment and provide some examples of impacts on marine species. Integrating and adding to the growing body of work, we present here a comprehensive, inclusive, and quantitative consideration of responses of marine sensory systems to human-induced perturbations along with accompanying consequences. We employ our conceptual framework (Figure 1) to specify a mechanistic interaction between the environment, the cue/signal producer, and the recipient organism and, in doing so, provide a new perspective concerning effects of global ocean change on regional and local ecological processes.
Effects of Global Ocean Change on Marine Sensory Pathways
In structuring our synthesis of available literature, we asked the multi-factorial question: how do environmental factors (e.g., ocean temperature) influence individual steps of sensory pathways (e.g., production) for various sensory modalities (e.g., visual)? We focused on environmental factors that are at the forefront of global attention (e.g., IPCC, 2013): temperature, carbonate chemistry, oxygen, salinity, UVB, turbidity, hydrodynamics, stratification, and nutrients. We identify emergent patterns across the wide breadth of our survey, specific to individual pathway steps and anthropogenic stressors. In an effort to maintain the large scope of our synthesis, we have chosen to highlight illustrative examples in which global ocean change may alter sensing in the marine environment and consequently, outcomes of sensory pathways. Finally, we interpret and apply the emergent patterns in a discussion of how altered sensory pathways may shape ecological and evolutionary processes. To our knowledge, this is the first quantitative literature review on impacts of global change on marine sensory pathways, and the emergent patterns we identify provide unique insight and research directions for anticipating future impacts on ecological processes.
For our literature search, we first assembled a list of abiotic ocean conditions affected by climate change, as observed and predicted by the Intergovernmental Panel on Climate Change (IPCC, 2013). Then, we searched the literature to identify studies that reported effects of these abiotic conditions on sensory pathways (publications through September 1, 2017; Web of Science, all databases, Clarivate Analytics). Search terms (N = 693; Appendix S1) included the names of the environmental factor, the pathway step, and the sensory modality. For this review, we targeted the first three steps shared by sensory pathways as described above (Figures 1B,C): (1) production, (2) transmission, and (3) reception/processing. The response step was not included because we are not aware of any global change studies that evaluate decision-making in isolation of effects on other steps, i.e., the act of choosing an action from a set of alternative options based on interpretation of available sensory information. Additionally, we focused on visual, chemical, and auditory sensory modalities, as they have been targeted by a majority of relevant studies. We acknowledge that mechanoreception, electroreception, and magnetoreception are modalities of sensory ecology that are presently understudied and represent an interesting area of future research. Furthermore, we acknowledge that it is likely that we did not identify all of the studies that fit our criteria because of the variety of synonymous terminology employed to describe these studies. Next, we tabulated the number of relevant studies based on the sensory pathway step(s) and sensory modality affected, and we employed the following secondary criteria:
• We included any results from freshwater studies when evidence from marine systems was absent from the literature. Many factors that disrupt sensory pathways in freshwater systems can also be present in marine systems due to a similar aqueous environmental medium and some overlap among taxonomic groups.
• We focused on organismal-based cues and thus excluded results based on environmental cues (e.g., temperature as a phenological cue for spawning). While we acknowledge that global ocean change will directly affect species that rely on environmental conditions as cues, these scenarios are outside the scope of this study.
• We excluded effects tied to removal of the cue/signal producer or recipient organism. Global ocean change can cause species distributions to shift and therefore can prevent species from interacting, but in this case, effects on sensory pathways are likely not the primary underlying mechanism.
• Additional filtration of results was necessary when more than 500 results were produced from a search term. We used the “Refine by Research Area” function in Web of Science to exclude topics unrelated to our desired focus of marine and freshwater studies (Appendix S2).
Our quantitative literature search yielded 120 studies that document an effect of a climate change factor on a step of the marine sensory pathway (Figure 2). This modest number of relevant studies, which we have partitioned across different steps of the pathway and different modalities and which includes five freshwater studies, precludes a quantitative analysis of search results. It also indicates that further investigation into the mechanism of impact of climate change on marine sensory ecology is needed for identifying more detailed patterns. We found that more studies have documented impacts of climate change on reception/processing in sensory pathways than for other steps (Figure 2A). Sensory pathways that employ a chemical cue/signal modality are more often affected than pathways that use other modalities (Figure 2B). More impacts on sensory pathways have been documented with respect to carbonate chemistry, temperature, and turbidity than for other climate change stressors, for the studies we examined (Figure 2C). Finally, there is low taxonomic diversity among studies that document impaired sensory pathways by climate change stressors: ray-finned fishes and crustaceans dominate taxonomic groups under consideration (Figure 2D). These summary results of our literature review may be reflective of generalizable impacts associated with particular climate change factors, sensory pathway steps, and sensory modalities. In addition, due to the small number of relevant studies available in the literature, these results are likely shaped by disproportional research effort in particular study systems.
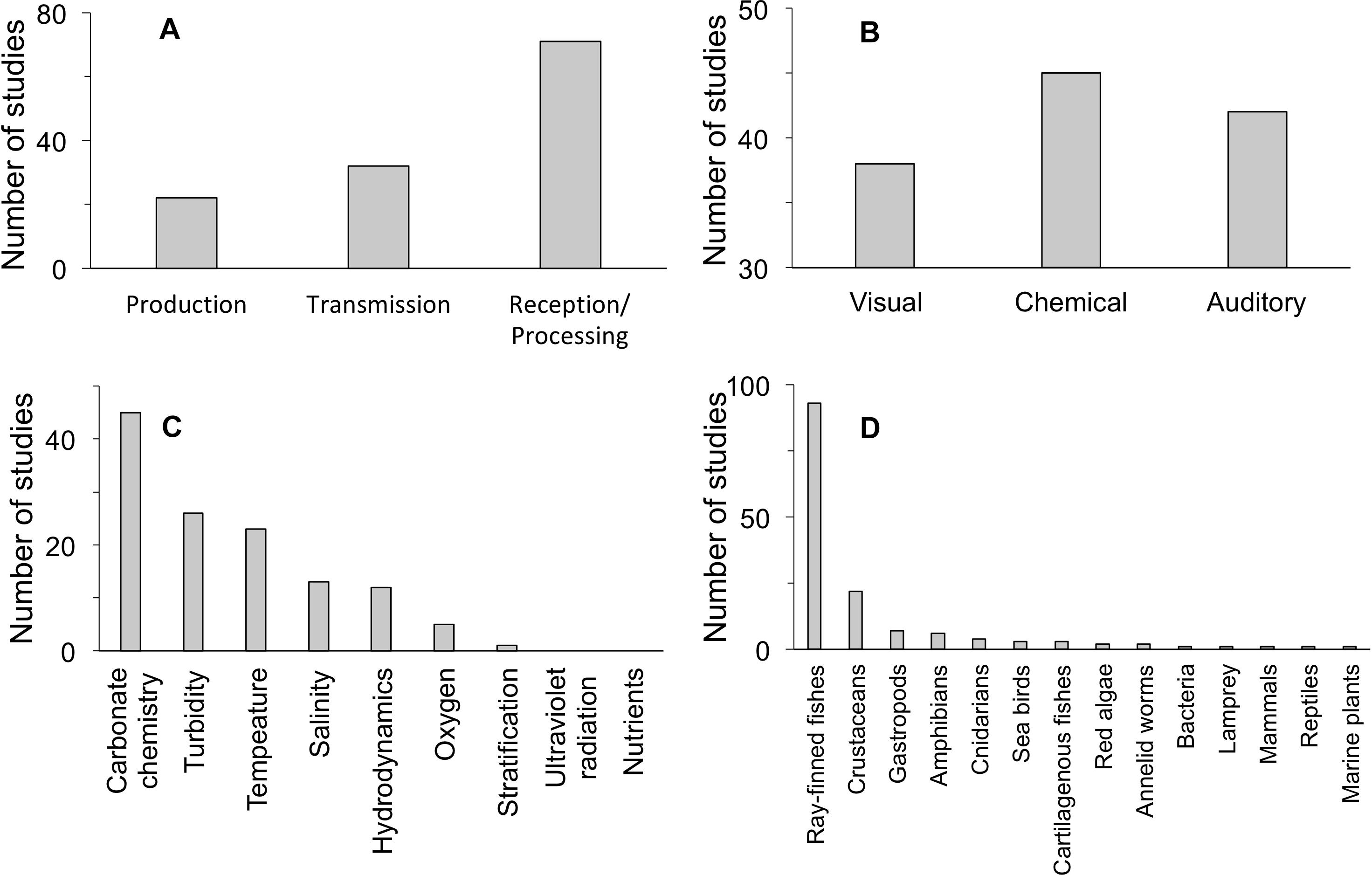
Figure 2. Evidence for effects of global change on sensory pathways, divided by (A) pathway step, (B) sensory modality, (C) environmental factor, and (D) taxonomic group. Relevant freshwater examples are included in the tallies where no marine studies were found: 2 in production, 3 in reception/processing, 2 in visual, 1 in chemical, 1 in auditory, 4 in oxygen, 1 in turbidity, 3 in ray-finned fishes, and 1 in amphibians. See Table 1 for more detailed results of the literature review.
We employed our conceptual framework to identify and interpret emergent patterns in the results of our literature review. We interpret these patterns to highlight the potential consequences of environmental change on sensing in the marine environment and ecological interactions.
Production
Sensory information is produced passively in the form of cues (e.g., metabolic waste) and intentionally in the form of signals (e.g., mating vocalizations). Our review suggests that if an environmental factor affects production of a cue/signal for a particular sensory modality (chemical, visual, auditory), then the factor is likely to affect production for more than one modality. Indeed, four of the five environmental factors that affected production did so for multiple modalities (Table 1). For example, carbonate chemistry affects the production of visual, chemical, and auditory cues/signals (Table 1). This pattern – that an environmental factor affects production of cues/signals generally for multiple sensory modalities or none at all – indicates that there are generalizable mechanisms by which environmental change impairs cue/signal production, beyond direct impacts that are specific to individual modalities or environmental factors. First, changes in environmental conditions can directly impact organismal physiology, thereby affecting cue/signal production regardless of modality. Secondly, changes in environmental factors can trigger changes in timing of cue/signal production.
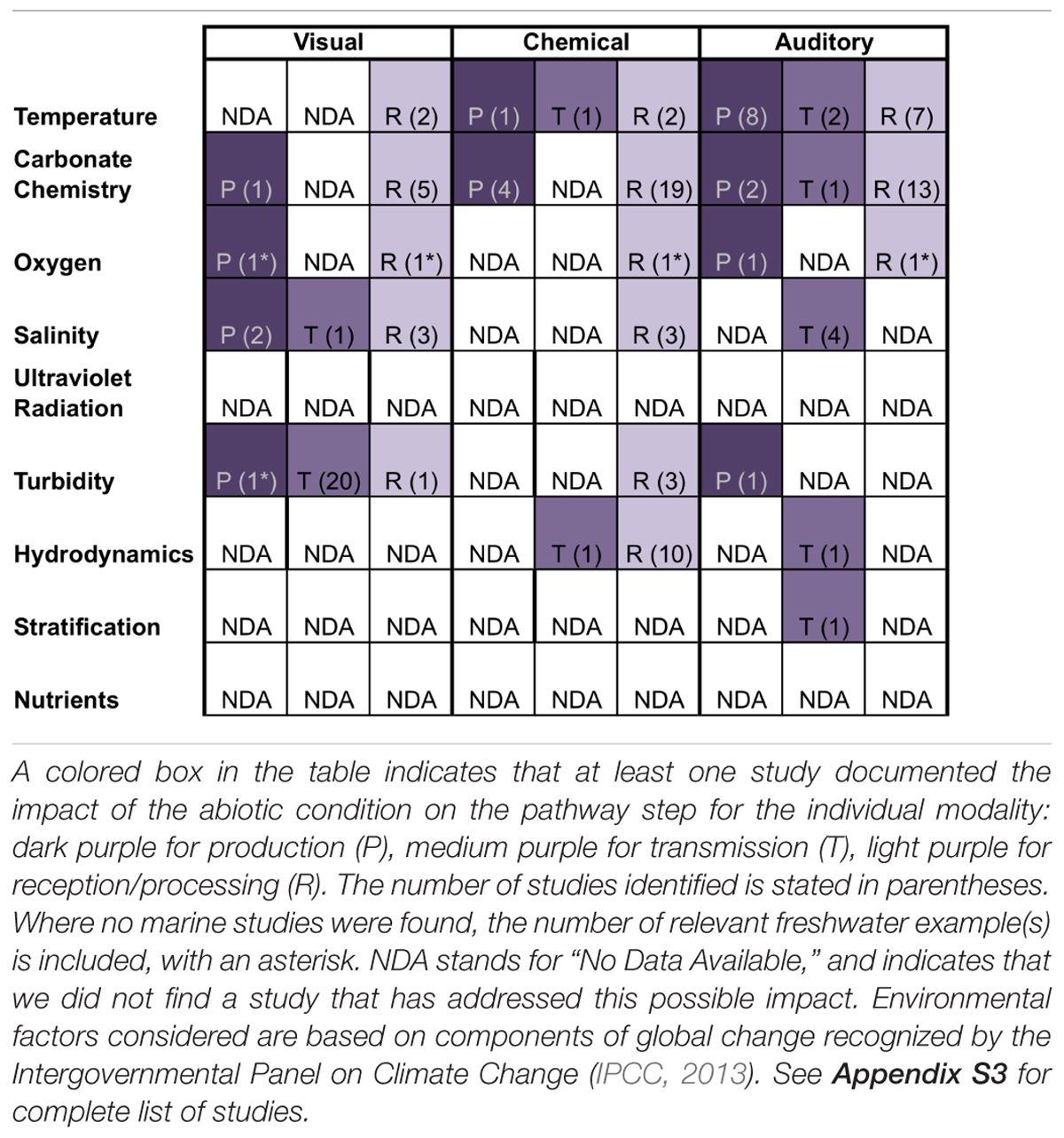
Table 1. Steps of sensory pathways and associated sensory modalities that are affected by aspects of human-caused ocean changes to specific physical attributes of the marine environment.
Global Ocean Change Can Directly Impact Organismal Physiology, Which Affects Cue/Signal Production
Many marine organisms live in highly dynamic environments characterized by rapid fluctuations in abiotic conditions (Helmuth and Hofmann, 2001; Eckman et al., 2003; Hofmann et al., 2011; Cheng et al., 2015). Although their organismal physiology is sufficient for tolerating naturally variable environments, global ocean change may shift the mean and range of natural environmental regimes, creating novel, extreme habitat conditions and leading to physiological disturbance, such as through stress (Sokolova, 2013), and a decrease in fitness. Upregulation of or shifts in physiological processes to combat environmental stress are energetically costly to support and require trade-offs between maintaining physiology necessary for survival and performing other biological functions, like signal production. Consequently, during periods of physiological stress, less energy may be allocated to production, with implications for respective sensory pathways and ecological interactions. In some cases, production of signals, particularly those involved in sexual selection, can be prioritized at the expense of other fitness-related characters, such as growth rate, immunity, and survival (Johansson and Jones, 2007).
A specific example of how global ocean change can cause physiological stress and thus affect cue/signal production is the tradeoff between mating success and physiological stress associated with extreme environments, such as those that naturally occur in the high intertidal zone and that will also occur under climate change. Adult male European green crabs, Carcinus maenas, are found with a range of carapace colors from green to red. Females are more likely to mate with red males than green males, controlling for size (Reid et al., 1997). The desirable red carapace color develops between molts; crabs are green post-molt and turn red as the inter-molt period increases. Thus, green and red males of equivalent size likely have different life history strategies, with green crabs investing in growth and molting more often and red crabs investing in reproduction (Himes et al., 2017). A trade-off occurs because the physiological capacities of green and red male crabs differ. Red crabs are less able to compensate for hypoxia, exhibiting lower rates of respiration and altered behavior (Aldrich and Reid, 1989; Reid and Aldrich, 1989). Red crabs are also more vulnerable to low salinity stress, showing lower capacity for maintaining their hemolymph osmolarity and lower survival (Reid et al., 1997). Therefore, under changing environmental conditions associated with climate change (i.e., increased frequency and intensity of hypoxic or low-salinity events), male crabs may need to molt more often in order to maintain physiological tolerance, but a shorter inter-molt period means their carapaces will be, on average, less red. In this way, maintenance of physiological tolerance comes at the cost of reduced production of the visual signal used for mating.
Impacts of environmental change on sensory pathways are not limited to physiological stress. Even non-stressful changes in environmental conditions can affect cue/signal production. For example, temperature affects metabolic rate and movement. In ectothermic fish, increased temperature can cause an increase in mating display behaviors (Bischoff et al., 1985; Hess, 2010). Male guppies (Poecilia reticulata) raised at higher temperatures produce mating displays at faster rates (i.e., an enlarged and quivering tail), and these males with faster display rates were chosen more often by competent females (Bischoff et al., 1985). Similarly, an increase in vocal activity as well as changes in frequency composition of vocalizations with increased temperature has been documented for several fish species (reviewed by Ladich and Schleinzer, 2015).
Changes to Environmental Factors Can Directly Alter Cue/Signal Production
Cue/signal production may be affected by aspects of the physical environment, such as habitat temperature (Genner et al., 2010). For example, many marine fish produce sounds for communication during mating. In Atlantic croaker (Micropogonias undulatus) and weakfish (Cynoscion regalis), a range of environmental factors, such as temperature, turbidity, and salinity, directly influence sound production (Connaughton et al., 2002; Krahforst et al., 2012). Global ocean change may shift these environmental conditions, such that biological readiness for the production of cues/signals will be triggered at different times or the timing of the trigger for cue/signal production may become mismatched with the subsequent steps of the sensory pathway for mating. Global ocean change may also indirectly influence sound production in these fish through the phenology of reproductive readiness. A mismatch between peak reproductive readiness, when maximal sound production is possible (Connaughton et al., 2002), and the environmental conditions that trigger sound production could develop. Consequently, the timing and success of reproduction could be affected by changing environmental conditions, such as ocean temperature.
Transmission
For most sensory modalities, cues/signals travel through a medium before reaching the recipient organism, and the physical and chemical properties of the medium can have profound effects on the strength and reliability of the information (Stevens, 2013). An emergent pattern is that a majority of aspects of global ocean change affect the transmission of cues/signals (∼60%, N = 6; Table 1). Global ocean change is predicted to alter the physical and chemical properties of seawater (IPCC, 2013), and there are several ways in which environmental change might play a substantial role in modifying the transmission of cues/signals. Firstly, the transport of the cue/signal to the recipient organism can be physically disrupted in an altered medium. Secondly, changes in the medium can degrade the cue/signal before reaching the recipient organism. Lastly, the information received by an organism is determined not just by the absolute strength of the cue/signal, but also by its strength relative to background noise (signal-to-noise ratio; Stevens, 2013). Increased background noise can swamp an organism’s receptors, preventing it from responding to the original cue/signal even if the original cue/signal is unaffected.
Global Ocean Change May Interfere With the Transport of the Cue/Signal Through a Medium
The transport of cues/signals is highly dependent on the nature of the medium, which can dictate how far a cue/signal is transmitted, the speed of the transmission, and whether a cue/signal that reaches a recipient organism is sufficiently strong to elicit a response (see review by Nagelkerken et al., 2019). For example, the density of seawater, which is influenced by both temperature and salinity, influences the transmission of light and sound (Dushaw et al., 1993; Matt et al., 2014).
One of the relatively well-studied cases of transmission interference is the effect of hydrodynamics on the transmission of waterborne chemical cues/signals. Hydrodynamics plays a large role in the transport of molecules and is essential in the transfer of chemical cues/signals over long distances (Webster and Weissburg, 2009). Storm intensity is expect to increase under climate change (IPCC, 2013), which could impact turbulence and wave-generated flows in both open ocean and shallow coastal environments (e.g., Denny, 1987; Sullivan et al., 2012), thereby disrupting chemical gradients and altering the concentrations of cues/signals that organisms use for orienting directional movement and for kinetic responses (Webster and Weissburg, 2009). A good example of the consequences of the disruption of cue/signal transmission through shifts in hydrodynamics is predator-prey interactions where both organisms use information to detect each other (e.g., crabs and clams/snails). For instance, intertidal whelk, Nucella lapillus, respond to chemical cues released from predatory crabs by reducing their activity and movement. This anti-predator behavior is enhanced at intermediate flow velocities, likely because turbulence increases the spatial extent of the cues, mixing them into the boundary layer where snails are able to sense them (Large et al., 2011). However at high levels of turbulence, mixing likely creates intermittent areas of low concentrations of chemical cues and thereby dilutes the signal (Koehl, 2006), rendering the cue undetectable and diminishing the anti-predator behavior of Nucella (Large et al., 2011). Altered structure of chemical cue plumes, such as this, have been shown to modify the behavior of several other prey species (the quahog clam, Smee and Weissburg, 2006; mud crabs, Pruett and Weissburg, 2018). Moreover, turbulent mixing and dilution of chemical cues can influence the ability of some predators to track their prey, such as blue crabs (Callinectes sapidus) that have reduced foraging success in high-flow conditions (Weissburg and Zimmer-Faust, 1993). However, even within a single predator-prey pair, turbulence has been shown to differentially influence behavior (Smee et al., 2008). Although turbulence is likely altering the transmission of cues/signals through increased mixing, the sensitivity of the receiving organism’s sensory receptors could still influence the severity of the outcome (Weissburg et al., 2014).
Changes in the Environment Can Affect Properties of the Cue/Signal
As cues/signals are transmitted to the recipient organism, the characteristics of the medium could degrade the information. Rising temperatures, fluctuations in UVB light levels (due to cloud cover), and changes in pH can denature organic molecules used as cues/signals in sensory pathways (Brown et al., 2002; Chivers et al., 2013; Roggatz et al., 2016; Nagelkerken et al., 2019). The consequences may depend on the specifics of degradation, which may be unique to the combination of environmental factor and the cue/signal modality. For example, under lower seawater pH expected with ocean acidification, functional groups of peptides and proteins, common chemical cue/signal molecules in marine environments (Decho et al., 1998), become protonated. Consequently, the structure and function of these important information molecules may be altered under ocean acidification, as protonation affects binding to cue/signal receptors (Roggatz et al., 2016). If the cue/signal is consequently unrecognizable, the organism might not respond to it appropriately. Descriptions of other types of cue/signal transmission and how they may be affected by global changed are reviewed by Nagelkerken et al. (2019).
Cormorants, Phalacrocorax spp., and their mode of visual predation present an example of sensory pathways interrupted by degradation of cue/signal transmission (Figure 1A). Highly efficient piscivores, cormorants use vision to find their underwater prey (Gremillet et al., 2012). Increased precipitation, an aspect of global change (IPCC, 2013), will lead to increased freshwater runoff as well as nutrient and sediment loads in the nearshore environment. Particulate and dissolved matter alter the chromatic characteristics of light by scattering and absorbing irradiation, causing a decrease in cue/signal light intensity reaching the recipient organism (Gazey, 1970; Loew and McFarland, 1990). Therefore, turbidity deteriorates the properties of visual cues/signals by reducing the intensity of light reflected off target objects, and the resulting loss of contrast and spatial information limits the visual range of organisms (Gazey, 1970; Loew and McFarland, 1990; Meager et al., 2005; Strod et al., 2008; Lu et al., 2013). Indeed, under increasingly turbid water, the distance at which a great cormorant, Phalacrocorax carbo sinensis, reacts to the presence of its prey declines exponentially (Strod et al., 2008). Although it is possible that P. carbo sinensis will switch to a tactile-based foraging method as visual cues/signals are degraded by environmental conditions (White et al., 2007; Gremillet et al., 2012), deterioration of visual cues will likely have significant implications for predator-prey interactions.
Global Ocean Change Can Increase Background Noise and Reduce the Information-to-Noise Ratio
The information-to-noise ratio of any given modality partly dictates how an organism will respond to cues/signals. Regardless of how strong the information of a cue/signal is, if the background informational noise is also substantial, the recipient organism might not be able to isolate the cue/signal. For example, under future ocean acidification, sound is expected to travel farther due to decreased absorption, particularly for sound waves of frequencies below 10 kHz comprising auditory communication by marine mammals and fish as well as background noise from wind-driven waves and anthropogenic activities like shipping (Hester et al., 2008; Ilyina et al., 2010). The underlying mechanism is thought to be a boron species relaxation effect; under lower pH, there are relatively more un-ionized forms of boron, molecules that are smaller than their ionized counterparts and therefore absorb less energy as sound waves pass by Brewer and Hester (2009). Since the Industrial Revolution, sound absorptivity has decreased by ∼10% in the upper 400 m of the ocean (ΔpH = -0.1; Orr et al., 2005), with an additional projected loss of 20–60% by year 2100 (ΔpH = -0.15 to -0.6; Hester et al., 2008; Ilyina et al., 2010). Similarly, sound will propagate more quickly as oceans warm (Munk et al., 1989). Consequently, low-frequency sound transmission in a future ocean for the purposes of sensory ecology will be more difficult, because background noise at a given location will be greater, due to ambient sound coming from a wider regional diameter. Additionally, other anthropogenic impacts on marine environments that are independent of climate change, such as light and sound pollution, increase background environmental noise (Davies et al., 2014; Neenan et al., 2016; and see Nagelkerken et al., 2019). For example, anthropogenic-generated acoustic noise can mask long-distance communications between cetaceans (Weilgart, 2007). A decrease in the information-to-noise ratio as a result of anthropogenic disturbance in the marine environment, its effects on marine sensory pathways, and its ecological implications may be fruitful foci of future research. Although our literature search did not include light and sound pollution, these factors present good examples of factors that decrease signal-to-noise ratios in marine environments.
Reception/Processing
In reception and processing of cues/signals, sensory receptors on the organism convert cue/signal information into electrochemical signals, which undergo processing by neurobiological systems (Rosenthal and Stuart-Fox, 2012). In order to detect effects of global ocean change on reception and processing of cues/signals, it is necessary to experimentally isolate this step of the sensory pathway. We identified more studies in the reception/processing category than in production and transmission combined (Figure 2B). However, many studies we assigned to the reception/processing category were not designed to target reception/processing alone, and therefore effects of experimental treatments on transmission and/or production could have affected the outcomes observed. We recommend that future marine sensory ecology research utilize experimental designs that effectively isolate individual sensory pathway steps, which will test the generalizability of the themes emergent from the existing body of work discussed here.
While the mechanistic underpinnings of disruption of reception/processing have yet to be understood in most systems, one identifiable pattern from our literature review is that if an environmental factor affects reception/processing, then the factor affects reception/processing for multiple sensory modalities (chemical, visual, auditory). Indeed, five of the six environmental factors that affected reception/processing did so for multiple modalities (Table 1). In support, recent evidence suggests that climate stressors, such as decreasing pH and increasing temperature, have a more systemic effect on organisms, independent of specific sensory modality or receptor modifications (Ferrari et al., 2012). Like with cue/signal production, this pattern supports the hypothesis that there are generalizable mechanisms by which environmental change impairs cue/signal production, beyond direct impacts that are specific to individual modalities or environmental factors. First, changes in environmental conditions can directly impact organismal physiology, with consequences for sensory receptor synthesis/maintenance or neural processing networks. Secondly, changes in environmental factors can directly alter the function of neurotransmitters or ion transport processes across neurons during the processing of any cue/signal regardless of modality. Thirdly, global ocean change stressors may directly modify and impair sensory receptors or structures.
Global Ocean Change Directly Impacts Organismal Physiology, Affecting Cue/Signal Reception and Processing
Similar to potential alterations in cue/signal production, global ocean change might induce physiological processes involved in combating environmental stress that are energetically costly to support, leading organisms to prioritize maintaining basal physiology at the expense of other biological functions, like sensory receptor production/maintenance and neurological processing (Johansson and Jones, 2007; Sokolova, 2013). Additionally, even non-stressful changes in environmental conditions may affect cue/signal reception/processing. For example, when larvae of cobia (Rachycentron canadum) are raised under high pCO2, the size, density, and surface area of their otoliths (calcified sensory structures) increases, which likely alters the ability of the larvae to detect sound (Bignami et al., 2013). Interestingly, this effect of ocean acidification comes from direct impacts on organismal physiology, likely caused by increased bicarbonate ion concentrations in the surrounding fluid – a biproduct of physiological ion regulatory processes at the organismal level – that create more energetically favorable calcification conditions (Claiborne and Heisler, 1986; Checkley et al., 2009).
Another example of how global ocean change can affect cue/signal processing through perturbations of organismal physiology is the influence of reduced seawater pH on predator cue recognition in tropical fish. Many groups of fish use chemical cues inadvertently released by predators to detect predation risk and avoid predators (Chivers et al., 2007). This defensive mechanism is impaired when fish are exposed to seawater with elevated pCO2. Under high-pCO2 conditions, fish increase bicarbonate levels to buffer changes in blood plasma pCO2, coupled with a decrease of chloride ions to maintain charge balance (Nilsson et al., 2012; Hamilton et al., 2013; Heuer and Grosell, 2014). Although this physiological process can be a first line of defense against low-pH stress and compromised respiratory function, the resulting changes in ion gradients across cell membranes can interfere with the function of neurotransmitter receptors, like GABA (Nilsson et al., 2012; Hamilton et al., 2013; Heuer and Grosell, 2014). When GABA is present, its receptor, an ion channel, only allows certain anions into the cell. Under normal conditions, the inflow of these anions hyperpolarize the cell and reduce neuronal activity. However, under reduced seawater pH conditions that accompany ocean acidification, the new ion gradients generated across cell membranes can reverse the function of GABA and impair antipredator behavior (Nilsson et al., 2012; Hamilton et al., 2013; Heuer and Grosell, 2014). The presence of self-amplifying physiological processes, like those in the GABA system, may mean that although small near-future changes in seawater chemistry only cause small changes in concentrations of relevant acid-base ions, large neurological impacts can occur (Schunter et al., 2018). Thus, global ocean change could affect the neural processing of cues/signals through its effect on internal physiology of recipient organisms. The trade-off between underlying systemic physiological mechanism(s), like upregulation of acid-base regulation as environmental conditions change, and sensory function may also explain the pattern that not only biochemical homeostasis, but also visual and auditory systems for reception and processing are impaired in reef fish at high pCO2 (Simpson et al., 2011; Ferrari et al., 2012; Forsgren et al., 2013). Because much of this work has focused on fish, determining if these mechanisms are generalizable among taxonomic groups is a valuable future research direction (Clements and Hunt, 2015; Ashur et al., 2017).
Global Ocean Change Can Directly Alter Organismal Neurophysiology Involved in Processing of Cues/Signals
Certain abiotic factors, such as ocean temperature, directly affect marine organisms. The body temperatures of marine ectotherms, and therefore rates of their neurophysiological processes, depend on the water temperature in which they live (Portner, 2002). For instance, many marine fish use auditory cues/signals for social communication, detection of predators and prey, and mate selection. Both temperature and hypoxia can influence the conduction and transduction speed of nerve fibers and channels in sensory hair cells as well as the degradation, release, and replenishment of neurotransmitters (Macdonald et al., 1988; Wysocki et al., 2009; Maiditsch and Ladich, 2014). It is important to note, however, that the referenced studies employed large acute temperature changes (≥10°C), and changes in neurophysiological function caused by sustained, moderate changes in environmental conditions are still unknown. Still, global ocean change will likely increase the frequency and magnitude of extreme environmental conditions (e.g., daily thermal maxima, daily oxygen minima), and existing studies suggest that even these short periods of environmental stress may alter the sensitivity of hearing in fish (Macdonald et al., 1988; Wysocki et al., 2009; Maiditsch and Ladich, 2014).
Changes to Environmental Factors Can Directly Alter Sensory Receptors
Many marine organisms have an assortment of sensory receptors that transduce stimuli into electrochemical impulses that are perceived and translated into a response. These receptors and the accessory structures on which they are located are exposed to the external environment of the organism and therefore changes in the surrounding abiotic environment. For instance, chemoreception is accomplished through receptors often located on external structures, such as antennae barbels and tentacles, or in olfactory organs directly exposed to seawater. Sensory receptors may be vulnerable to alterations in charge distribution at the reception site under ocean acidification conditions due to increased concentrations of hydrogen ions (Tierney and Atema, 1988). For example, hermit crabs (Pagarus bernhardus) detect and locate food over long distances by flicking their antennae, allowing chemicals to bind to receptor sites. Under ocean acidification conditions, hermit crabs were unable to detect food stimuli (de la Haye et al., 2011). Direct changes to chemoreceptor sites could contribute to this sensory impairment because altered physiological conditions of the crabs do not fully explain differences among treatments. The successful interaction and binding of cue/signal molecules to receptors can be disrupted by small changes in receptor structure, for example through protonation (Roggatz et al., 2016), which is known to affect the presence, abundance, and location of intermolecular forces important for intermolecular interactions (e.g., hydrophobic regions, hydrogen bonding, Hardege et al., 2011; Wyatt, 2014). However, while the majority of studies in our review document effects on cue/signal reception/processing (Table 1), few identify the mechanism of impairment, and we currently lack evidence to conclusively establish which sensory structures might be affected, by which stressors, and how often. Our synthesis suggests that direct modification or degradation of sensory structures may be generalizable across modalities for impacts of changing environmental conditions on cue/signal reception/processing, and we expect that individual mechanisms may differ by environmental factor and modality.
Response
Sensory response, i.e., the act of choosing an action from a set of alternative options based on interpretation of available sensory information, was not included in our literature analysis and review because very few studies have directly tested the effects of global ocean change on the response step. We hypothesize that the response of a marine organism could be altered by different environmental conditions, though this conjecture remains untested in the context of global change. If a preceding step of the sensory pathway is impacted by environmental conditions, the information the organism uses to make decisions will be different, changing the behavioral outcome. Additionally, the physiological state of the organism or other information about its surroundings may influence the type of response (Luttbeg et al., 2003; Matassa and Trussell, 2014; Gravem and Morgan, 2016). As we have previously described, changes in the marine environment can increase the energetic cost of physiological maintenance, and organisms may face tradeoffs in the energy they allocate to any particular response. Even if the information is detected and processed properly, decision-making and thus the behavioral response of the organism may depend on the energy available to respond appropriately. For example, if food is scarce or if nutritional demands are high, an organism may perceive predation risk but instead of initiating predator avoidance behavior, it may choose to forage (Gravem and Morgan, 2016).
Consequences of Global Ocean Change Mediated by Sensory Pathways: Ecological and Evolutionary Implications
As the physical conditions of ocean habitats continue to change, shifts in sensory pathways, from production to response, will modulate ecological and evolutionary processes. Here, we consider how altered sensory ecology under global change will impact a sampling of several crucial processes underpinning ecological interactions. These include processes that influence population structure and dynamics (e.g., dispersal, mate selection), processes that influence communities (e.g., predator-prey interactions), and natural selection. We highlight how global ocean change and sensory ecology may shape the predicted outcomes of these ecological and evolutionary processes and why it is vital to understand the mechanisms (i.e., impacted pathway steps or cue modalities) underpinning the outcomes. These considerations have the potential to improve predictions of future ecosystem-level consequences of human-caused ocean change.
Ecological Consequences of Global Change
Habitat Selection and Recruitment
The dispersal of marine larvae, their selection of benthic habitat, and the recruitment of individuals to adult populations contribute to the dynamics of species across spatially structured landscapes. Marine larval dispersal is affected by the interaction of oceanographic processes with larval sensing and behavior (Pineda, 1991) and plays an important role in connecting otherwise separate habitat patches and populations (Hanski and Gilpin, 1991). Numerous sensory cues from chemical, auditory, and visual modalities direct larvae to suitable settlement sites (e.g., Montgomery et al., 2001). For example, larvae of many marine taxa, such as corals, crabs, and mussels, use conspecific chemical cues to locate optimal settlement habitat (e.g., Rodriguez et al., 1993).
Changes in environmental conditions can influence sensory pathways used by larvae during dispersal and during the selection of suitable settlement sites, which could result in maladaptive habitat selection. The most profound effects have been found at the reception/processing step of sensory pathways (Figure 2; e.g., crustaceans: Forward et al., 2009; lamprey: Di Rocco et al., 2014; fay-finned fishes: Allan et al., 2013; amphibians: Telfer and Laberge, 2013; cnidarians: Frommel et al., 2016; gastropods: Manríquez et al., 2013; cartilaginous fishes: Pistevos et al., 2017). For example, conditions of ocean acidification significantly impair sensory behavior of reef fish larvae and cause larvae to settle in poor-quality habitats (Munday et al., 2009; Gerber et al., 2014). Changes in environmental conditions can also affect the transmission of cues/signals, which could alter the perceptual range of larvae (e.g., Porteus et al., 2018), affecting the probability that larvae encounter poor-quality habitat (Hale and Swearer, 2016), though little research has been conducted on this topic. Less is known about effects of climate change on production of cues/signals by habitat-forming foundation species.
Habitat patches often vary in quality, which influences the persistence of local populations following dispersal and recruitment. Sometimes, poor-quality habitats (i.e., sinks) are unable to support local populations without replenishment of individuals from other patches (Dias, 1996). When recruiting individuals prefer a sink over higher-quality patches, due to impaired sensing under global change, they fall into an “ecological trap,” and their habitat selection becomes maladaptive (Hale et al., 2015). Consequently, their fitness is diminished, affecting the fecundity of the population and lowering both birth rates and survivorship. Similarly, the rate of immigration and emigration of populations will be affected, such that larvae will move from source populations to more preferred, but lower-quality habitats. If populations are not properly replenished due to ecological traps, then they may severely decline or go extinct (Battin, 2004; Hale and Swearer, 2016).
Understanding the place(s) in the sensory pathway where changing ocean conditions affect dispersal, habitat selection, and recruitment is essential for predicting changes in population dynamics that may influence the persistence of marine species. Trajectories of ecosystem health under future climate change may vary depending on the affected pathway step involved in habitat selection and interactions with other ecosystem attributes. For example, settlement dynamics of coral are mediated through the production of settlement cues by crustose coralline algae (CCA), which are transmitted through the water, then received and processed by coral planula larvae for effective habitat selection (Tebben et al., 2015). Larval-algal settlement interactions are disrupted under conditions of ocean acidification (Doropoulos et al., 2012; Figure 1A). Tiles with a variety of settlement substrates, including the preferred CCA type, Titanoderma spp., were incubated under different pCO2 treatments and then placed under ambient conditions with competent planulae (Acropora millepora), thereby isolating effects on cue production. When larvae were provided tiles from elevated pCO2 conditions, settlement rates were lower, but larval behavior also changed – larvae no longer preferred to settle on Titanoderma spp., suggesting that cue production was affected by ocean acidification conditions (Doropoulos et al., 2012). If disruption of habitat selection and settlement processes has been identified as a primary driver of decreasing coral settlement and persistence for a particular reef, then factors that ameliorate coral settlement will be different depending on which stressors affect which parts of the sensory pathway. For example, if the production of settlement cues by CCA are altered (e.g., Doropoulos et al., 2012; Webster et al., 2013), then factors that decrease or offset organismal stress may ameliorate effects of the stressor on the CCA. Instead, if transmission of settlement cues released by CCA is being affected, factors that reduce degradation or loss of the cues may help restore settlement success. In the case of A. millepora, because settlement preferences are altered under ocean acidification conditions, recruitment dynamics under future ocean conditions can now be better predicted based on the in situ conditions to which various CCA species are exposed across their biogeographic range. Thus, understanding the exact mechanism for the breakdown in sensory processes related to habitat selection and recruitment will likely improve predictions of impacts of climate change on population dynamics.
Mate Selection
Many species use multiple modalities of cues/signals to make decisions regarding mate choice. Each cue/signal type can provide unique information about different mate qualities or can allow for a more accurate assessment because each type reflects the same aspect of mate quality (reviewed by Candolin, 2003; for marine examples, see Acquistapace et al., 2002; Shine, 2005; Schwab and Brockmann, 2007). After assessing mate quality, two important parameters influence mate choice: preference based on the rank of a prospective mate with respect to other potential partners and choosiness based on the effort invested in mate assessment (Jennions and Petrie, 1997). When choosing a mate, the traits that females consider should be honest indicators of male fitness, thus communicating the male’s ability to pass on genes that will enhance the survival and reproductive success of the female’s offspring (termed the good gene hypothesis).
The physiological effects of changing environmental conditions may manifest as reduced production or reception/processing of cues/signals associated with mate selection. Stressors operating on shorter time scales (i.e., nutritional availability) influence mate choice and sensory processing (Lailvaux and Kasumovic, 2011). Over longer time scales, stress experienced during development can alter an individual’s mate selection process throughout its adult lifetime through effects of reception/processing of cues/signals (shown in terrestrial systems; Nowicki et al., 2002; Holveck and Riebel, 2010). Under global change scenarios, preference and choosiness of mates will likely be affected (Jennions and Petrie, 1997). For example, female sticklebacks (Gasterosteus aculeatus) preferentially lay eggs in nests where the males have a deeper coloration (Bakker and Mundwiler, 1994). Since coloration is linked to the physiological state of the individuals (Milinski and Bakker, 1990), global ocean change may impact the production of mating signals and therefore mating outcomes. Females may engage fewer males due to lack of stimulation and may become choosier (i.e., spend more time evaluating potential mates; Jennions and Petrie, 1997). Similarly, consider the weakfish, C. regalis, which uses sonic muscles to produce sounds for attracting mates. Females evaluate sound frequency of male vocalizations, which varies with male size and distance to the potential mate. Water temperature affects the frequency of the acoustic signal produced, because the twitch rate of the sonic muscle is impacted (Connaughton et al., 2002). If females prefer a specific sound frequency, the altered acoustic signal (i.e., a different frequency) could impair female choice by disguising distance to the male or male size. However, if mate choice relies more on relative frequency (i.e., perception of a lower frequency than background noise or non-mating signals), mate choice may not be impaired. If cue reception is impaired, females may not be able to distinguish between high- and low-quality mates, such that they may rank prospective mates more equally, despite differences in mate quality, and they may invest less effort in mate evaluation. These hypotheses merit investigation, as currently no studies have examined these scenarios.
Global ocean change can also impair the transmission of signals important for mate selection. For species that use visual signals, the increase in turbidity can make it harder for individuals to select mates (Heuschele et al., 2009; Sundin et al., 2010). And though we explicitly did not include anthropogenic noise in our synthesis, sound from human activities poses a significant issue by masking auditory mating signals of certain fish (Amorim et al., 2015; Nagelkerken et al., 2019). For modalities where cue/signal transmission is affected, cues/signals may no longer indicate mate viability or beneficial traits, so they become unreliable, and preference for them will be arbitrary.
The mechanistic foundation of sensory impairment, which we have laid out, could be used to explain or predict differences among species in vulnerability of mate selection to ocean change. Visual, chemical, and auditory modalities have all been used by aquatic animals (marine and freshwater) as a means for mate selection, and some species use multiple modalities to choose mates (Heuschele et al., 2009), while others appear to only use one (Sundin et al., 2010; Fine and Waybright, 2015). Within species, individuals may vary in their ability to use multiple modalities, creating fodder for natural selection, though this concept has yet to be explored. Species that are able to use multiple modalities when choosing mates should be better able to compensate for a breakdown in one modality. Sticklebacks, for instance, are able to use both olfactory and visual cues when choosing mates (Heuschele et al., 2009), suggesting that if conditions become turbid, they are able to switch to olfactory cues. Table 1 shows that visual cues are most likely to be impacted by turbidity, which has minimal impact on chemical cues. Similarly, chemical cues are affected by hydrodynamics but visual cues are not. This pattern where one stressor impacts a particular modality but not another could be a means by which we identify areas of compensation. For species that only use one modality, mate selection may be particularly vulnerable to sensory disruption. For instance, under turbid conditions, the broad-nosed pipefish, Syngnathus typhle, which uses visual cues to select mates, is unable to compensate with olfactory cues (Sundin et al., 2010). Further, changes in the reliability of one modality over another may have consequences for the spatial scales of ecological processes, based on the spatial range of cue/signal transmission for the respective modalities. As the sensory pathway degrades, shorter-range modalities, such as olfaction, may be favored over longer-range modalities. The inability to select fitter mates will likely have implications for the adaptation of a species to changing environments.
Predator-Prey Interactions
Predator-prey relationships are principal species interactions that structure ecological communities through phenomena ranging from trophic cascades to the prevention of competitive exclusion (Hairston et al., 1960; Paine, 1966). Many predators and prey use cues/signals to obtain reliable information about the environment and each other (Weissburg et al., 2014). In doing so, prey can optimize the costs and benefits of anti-predator behavior versus other behaviors like foraging. Consequently, in predator-prey models, a key parameter that influences prey behavior and the outcome for prey fitness is the quality of the information the prey or predator obtains (Luttbeg and Trussell, 2013). For this reason, factors affecting the process by which predators and prey sense one another have the potential to influence the outcome of predator-prey interactions. A substantial amount of work in recent decades has demonstrated that human-induced changes to the environment can have pervasive effects on trophic interactions with consequences for community dynamics (Harley et al., 2006; Gilman et al., 2010; Gaylord et al., 2015; Kroeker et al., 2016). Although altered sensory responses are likely to shape the outcome of trophic interactions, much of this work does not specifically focus on sensory stress as a mechanism for altered interactions (Nagelkerken and Munday, 2016).
Yet, predator-prey interactions can be affected by changes to sensory ecology from environmental parameters expected to shift with global ocean change, including turbidity, hydrodynamics, and water chemistry (IPCC, 2013). Turbidity can alter predation success by reducing the distance and angle at which visual predators like cormorants can detect prey (Figure 1A; Strod et al., 2008). Hydrodynamics can influence the availability of predator cues and thus information about predation risk (Weissburg and Zimmer-Faust, 1994; Smee and Weissburg, 2006; Large et al., 2011). Altered water chemistry associated with future ocean acidification can impair the ability of snails and larval fish to respond to predator cues, which makes them exhibit riskier behavior and, in turn, increases mortality rates (Munday et al., 2010; Jellison et al., 2016).
How global ocean change affects the outcome of predator-prey interactions and the larger food web may depend on the differential sensory impairment between predator and prey (Weissburg et al., 2014). If the sensory pathway of the predator is more sensitive to global ocean change relative to the prey’s pathway, the prey’s ability to avoid predation will likely increase. In contrast, if the prey’s sensory pathway is more impaired by global ocean change, their fear response will likely decrease, leading to increased predation (Weissburg et al., 2014). Furthermore, a predator has an ecological influence on its community not only through consumption of prey, but also through shifts in the prey’s feeding behavior in the presence of the predator (Schmitz et al., 1997). Consider a simple food web with three members: predator, prey, and basal resource. When sensory impairment is significant for both predator and prey, they are unable to detect each other. This scenario predicts maximal consumption of the basal resource by the prey since neither predation nor fear will limit prey foraging. Alternatively, if only the predator is impaired, predation will decrease; however, the prey should still respond to predation risk and decrease their consumption of lower trophic levels. Thirdly, if only the prey is impaired, prey foraging behavior will not be affected in the presence of predators and their likelihood of being consumed by a predator will increase, reducing prey population density and amount of basal resource consumed. This spectrum of scenarios where the loss of fear can be compensated by the increased mortality in the prey species produces a multitude of potential outcomes for the basal resource.
Future research efforts to characterize impacts on sensory pathway steps, as well as to characterize environmental conditions on relevant spatial scales, will improve the application of our conceptual framework toward predicting consequences of global ocean change on predator-prey interactions and marine food webs. For instance, knowledge of how global ocean change will alter the production, transmission, and reception/processing of sensory cues/signals as well as an understanding of how differential sensory impairment varies among functional groups (e.g., predators versus prey) could provide baseline predictions of effects on lower trophic levels. For example, consider if transmission of visual predator cues is impaired by turbidity, there still remains room for compensation via olfaction, which is less likely to be influenced by turbid waters. However, in a case where a climate change stressor alters reception/processing, there is an increased chance that the neural processing of all sensory modalities will be impaired (see section Global Ocean Change Can Directly Alter Organismal Neurophysiology Involved in Processing of Cues/Signals). Consequently, without acclimatization or evolutionary processes, shifts in community structure would be more likely. In addition, if we anticipate that the severity of impairment in the sensory pathways of species at higher trophic levels will be greater than for their prey (e.g., Menge and Sutherland, 1987), then we might also be able to anticipate shifts in strength of trophic cascades and reductions in biodiversity of respective food webs. While the results from this paper do not necessarily provide a framework for management, they do provide a foundation on what we can expect as sensory pathways change.
Evolutionary Consequences of Global Change
The effects of global ocean change on marine sensory pathways not only will have ecological consequences, but also may operate over longer timescales to have evolutionary consequences (for a broader perspective, see Nagelkerken et al., 2019). Our synthesis of how sensory pathways are altered by aspects of global ocean change highlights a potential mechanism underpinning ecological and evolutionary traps: an evolved sensory pathway that produced adaptive behaviors under previous environments is now maladaptive under novel environments (Schlaepfer et al., 2002, 2005, 2010; Robertson and Hutto, 2006). In the context of our framework, it is not that the cue/signal contains poor information in the novel environment. The cue/signal may be absent or diminished due to altered production/transmission, the information from the cue/signal may not be processed correctly, or the cue/signal may now produce a dysfunctional response. Consequently, the cue/signal itself is rendered unreliable or inaccurate, and resulting behaviors are maladaptive in the new environment. Climate change certainly may not render all behaviors maladaptive – the behaviors induced by some impaired sensory pathways may still be more beneficial than a lack of response. Furthermore, our framework points to the need for future research to identify the role of existing local adaptation and regimes of natural selection in shaping responses of population and species to global ocean change. Implications of global change will be best anticipated by identifying the step of the sensory pathway that is impacted and the type of impact, e.g., whether the organisms have lost sensitivity to the cue/signal, there is greater masking of the cue/signal by the environment, or the cue/signal itself has been modified by the environment. Different types of impacts to sensory pathways will require different sorts of adaptive changes that may have varying constraints.
Understanding the mechanism by which climate change affects particular sensory pathways provides insight for anticipating where and when phenotypic plasticity is important for shaping local adaptation of ecological interactions. For example, marine sensory ecology can provide a novel interpretation of the theory of signal detection (Macmillan and Creelman, 2005; Sih et al., 2011). According to this theory, an organism’s response to a cue/signal stimulus is determined by intensity thresholds for that particular cue/signal and background intensities of other cues/signals. In the case of predator-prey relationships, the prey’s response threshold to predator cue/signal intensity will be optimally balanced where the benefit of increasing the likelihood of detecting a predator matches the cost of incorrectly identifying a predator. If cue/signal reliability is high, prey will easily distinguish between intensities of relevant cues/signals and background cues/signals and therefore between safe and dangerous situations. When cue/signal reliability is reduced, as we expect will occur under future ocean change conditions, the prey’s response threshold to predator cue/signal intensity will likely increase to maintain the cost/benefit balance of detecting the predator. Phenotypic plasticity – for example the ability of an organism to change its intensity thresholds for a behavioral response as signal-to-noise ratios change – may influence the ongoing adaptation of the sensory pathway (e.g., Kelly et al., 2017).
Additionally, understanding the impacted step of the pathway is important for predicting how phenotypic plasticity may constrain ecological outcomes. When production or detection of a cue/signal is affected, then the absolute value of the intensity of the cue (produced or detected, respectively) will be different with respect to the producer’s state (the relationship between cue and its information changes). Assuming the cue continues to be reliable, some organisms will have the plasticity to adjust their response norm appropriately (Sol et al., 2002, 2005; Wright et al., 2010). Some species may also be able to use secondary or alternative cues to gather the necessary information via compensation. When the transmission of the cue/signal is affected, the relationship between cue/signal and its information may remain the same, but if cue/signal properties (i.e., strength, frequency composition) become decoupled from the information (e.g., distance to mate, size of prey), cue accuracy will decrease. Consequently, organisms may switch which cues are predominantly used, moving away from cues that are vulnerable to damage during transmission (e.g., Webster et al., 2007). Consequently, ecological processes of generalist species that are able to utilize multiple cue types will likely be less affected by global ocean change due to flexible phenotypes (Sih, 2013). In addition, selection pressure for phenotypic plasticity may increase. If more than one pathway step is affected, use of the cue/signal for information gathering may become impractical. Being a specialist without the ability to use other cues/signals to obtain the underlying information may become maladaptive, and negative selection pressure may increase on these traits. However, in cases where cues/signals provide low quality information regardless of the modality (signal to noise ratio is low), specialists may be more discriminate, leading to an appropriate response (Sih, 2013). Clearly, a mechanistic understanding of the impacts on sensory pathways steps for particular modalities supports the critical need for future exploration of the evolutionary consequences of marine sensory ecology under global change.
Conclusion and Future Directions
Clearly, global ocean change will disrupt marine sensory pathways. Our conceptual framework and synthesis support the value of understanding impacts on individual pathway steps as an approach for predicting broader consequences. By evaluating the body of work on effects of global ocean change on marine sensory pathways, our synthesis yields several novel contributions. First, the production and reception/processing of multiple modalities of cues/signals are vulnerable to global change stressors, indicating that there are generalizable mechanisms by which environmental change impairs these pathways steps, leading to altered sensory pathway outcomes. Factors that enhance organismal stress as a whole may amplify impacts to these sensory pathways. Second, global change factors tend to affect specific modalities of cue/signal transmission. While perhaps not surprising due to the physical drivers of this pathway step, the consequence of this outcome is that local impacts of global change on ecological processes linked with cue/signal transmission will vary depending on environmental stressor(s) present and the affected sensory modality. Due to the plethora of ways in which environmental conditions can affect sensory pathways, employing a framework of sensory ecology will be important for accurately predicting consequences of global environmental change for marine ecological and evolutionary interactions.
In closing, there is broad recognition of the importance of sensory ecology to the function of marine ecosystems. As the global ocean change biology community builds on the relatively small number of studies conducted to date at the interface of global environmental change and marine sensory ecology, several key knowledge gaps merit further investigation:
• Future research should build on the interspecific diversity found among past studies to support testing of hypotheses on whether our emergent patterns are generalizable among taxonomic groups, functional groups, classes of body size, and life history strategies.
• Relatively little is known about how global ocean change will affect signal/cue production. Future research efforts should employ experimental designs that isolate this pathway step to investigate its sensitivity to global ocean change.
• While the physics underlying the relationship between the properties of a medium and the transmission of a cue/signal is relatively well studied, whether projected environmental conditions under global ocean change scenarios cause ecologically relevant changes in cue/signal transmission remains largely unknown. Future research on cue/signal transmission should employ ecologically relevant scenarios of future environmental conditions and should link effects to ecological outcomes.
• A better understanding of neural processing of cues/signals in marine organisms will enhance our ability to detect the incidence and consequences of effects of global ocean change on cue/signal reception/processing.
• Global ocean change may disproportionately affect marine sensory pathways through impacts on physiological maintenance. While much is known about the physiological consequences of environmental stress, future research efforts should fully describe how physiological maintenance processes underpin mechanisms of cue/signal production and reception/processing.
• Local environmental conditions will have a strong effect on the production, transmission, and reception/processing of cues/signals. Efforts to characterize and monitor local environmental conditions will improve predictions of impacts on local populations and communities.
• While our results indicate a generalizable mechanism(s) underlying the presence/absence of impacts of climate change on sensory pathways and corresponding ecological interactions, it is worth noting that the nature of the impacts on the ecological interactions will likely depend on the specifics of the modality and how the properties of the cue/signal are altered. For example, ecological outcomes of sensory impairment will likely depend on how perceptual distance is changed, what specific channels of information (e.g., frequencies) are affected, and whether there is a change in intensity of the cue/signal. The generalizable mechanisms we describe can be used for formulating broad predictions for impacts of global change in dynamic multi-stressor coastal ecosystems, but we acknowledge that precise predictions about impacts on specific ecological interactions will likely require direct examination on a case-by-case basis.
• A critical yet unknown part of predicting sensory ecology in a changing ocean is how impacts of environmental change on individual steps will integrate across entire sensory pathways to alter the responses of marine organisms. The observed behavioral response produced by sensory pathways may be related to the step of the pathway that is most dramatically affected by global ocean change, regardless of whether or how other pathway steps are affected. For example, if production is the most vulnerable pathway step, then the outcome of global ocean change on sensory response is similar when only production is affected and when both production and reception/processing are affected. Alternatively, effects on individual sensory pathway steps could integrate to generate a novel response through additive, synergistic, or antagonistic interactions.
Author Contributions
ER, BJ, GN, ES, SW, and BG conceived and designed the review. ER, BJ, GN, ES, and HB performed the literature search and organized the database. ER, BJ, GN, and ES wrote major sections of the manuscript with essential contributions from all authors.
Funding
This work was supported by NSF award OCE-1636191.
Conflict of Interest Statement
The authors declare that the research was conducted in the absence of any commercial or financial relationships that could be construed as a potential conflict of interest.
Acknowledgments
We are grateful to Grace Ha and Dr. Rochelle Seitz for helpful feedback on early versions of the project.
Supplementary Material
The Supplementary Material for this article can be found online at: https://www.frontiersin.org/articles/10.3389/fmars.2019.00346/full#supplementary-material
References
Acquistapace, P., Aquiloni, L., Hazlett, B. A., and Gherardi, F. (2002). Multimodal communication in crayfish: sex recognition during mate search by male Austropotamobius pallipes. Can. J. Zool. 80, 2041–2045. doi: 10.1139/z02-171
Aldrich, J. C., and Reid, D. G. (1989). “Individual consistencies in relative organiz sizes and oxygen consumption in Carcinus maenas (L.),” in Phenotypic Responses in Aquatic Ectotherms, ed. J. C. Aldrich (Dublin: Japaga), 107–114.
Allan, B. J. M., Domenici, P., McCormick, M. I., Watson, S.-A., and Munday, P. L. (2013). Elevated CO2 affects predator-prey interactions through altered performance. PLoS One 8:e58520. doi: 10.1371/journal.pone.0058520
Amorim, M. C. P., Vasconcelos, R. O., and Fonseca, P. J. (2015). “Fish sounds and mate choice,” in Sound Communication in Fishes. Animal Signals and Communication, Vol. 4, ed. F. Ladich (Vienna: Springer), 1–33. doi: 10.1007/978-3-7091-1846-7_1
Ashur, M. M., Johnston, N. K., and Dixson, D. L. (2017). Impacts of ocean acidification on sensory function in marine organisms. Integr. Comp. Biol. 57, 63–80. doi: 10.1093/icb/icx010
Atema, J., Fay, R. R., Popper, A. N., and Tavolga, W. N. (eds). (1988). Sensory Biology of Aquatic Animals. New York, NY: Springer-Verlag.
Bakker, T. C. M., and Mundwiler, B. (1994). Female mate choice and male red coloration in a natural three-spined stickleback (Gasterosteus aculeatus) population. Behav. Ecol. 5, 74–80. doi: 10.1093/beheco/5.1.74
Battin, J. (2004). When good animals love bad habitats: ecological traps and the conservation of animal populations. Conserv. Biol. 18, 1482–1491. doi: 10.1111/j.1523-1739.2004.00417.x
Bignami, S., Enochs, I. C., Manzello, D. P., Sponaugle, S., and Cowen, R. K. (2013). Ocean acidification alters the otoliths of a pantropical fish species with implications for sensory function. Proc. Natl. Acad. Sci. U.S.A. 18, 7366–7370. doi: 10.1073/pnas.1301365110
Bischoff, R. J., Gould, J. L., and Rubenstein, D. I. (1985). Tail size and female choice in the guppy (Poecilia reticulata). Behav. Ecol. Sociobiol. 17, 253–255. doi: 10.1007/BF00300143
Bograd, S. J., Castro, C. G., Di Lorenzo, E., Palacios, D. M., Bailey, H., Gilly, W., et al. (2008). Oxygen declines and the shoaling of the hypoxic boundary in the California current. Geophys. Res. Lett. 35:L12607.
Bradbury, J., and Vehrencamp, S. (1998). Principles of Animal Communication, 1st Edn. Sunderland, MA: Sinauer Associates, Inc.
Brewer, P. G., and Hester, K. (2009). Ocean acidification and the increasing transparency of the ocean to low-frequency sound. Oceanography 22, 86–93. doi: 10.2307/24861026
Brown, G. E., Adrian, J. C. Jr., Lewis, M. G., and Tower, J. M. (2002). The effects of reduced pH on chemical alarm signalling in ostariophysan fishes. Can. J. Fish. Aquat. Sci. 59, 1331–1338. doi: 10.1139/f02-104
Bullock, T. (1953). Predator recognition and escape responses of some intertidal gastropods in presence of starfish. Behavior 5, 130–140. doi: 10.1163/156853953x00078
Candolin, U. (2003). The use of multiple cues in mate choice. Biol. Rev. 78, 575–595. doi: 10.1017/s1464793103006158
Capotondi, A., Alexander, M. A., Bond, N. A., Curchitser, E. N., and Scott, J. D. (2012). Enhanced upper ocean stratification with climate change in the CMIP3 models. J. Geophys. Res. Oceans 117:C04031.
Checkley, D. M., Dickson, A. G., Takahashi, M., Radich, J. A., Eisenkolb, N., and Asch, R. (2009). Elevated CO2 enhances otolith growth in young fish. Science 324:1683. doi: 10.1126/science.1169806
Cheng, B. S., Bible, J. M., Chang, A. L., Ferner, M. C., Wasson, K., Zabin, C. J., et al. (2015). Testing local and global stressor impacts on a coastal foundation species using an ecologically realistic framework. Glob. Change Biol. 21, 2488–2499. doi: 10.1111/gcb.12895
Chivers, D. P., Dixson, D. L., White, J. R., McCormick, M. I., and Ferrari, M. C. O. (2013). Degradation of chemical alarm cues and assessment of risk throughout the day. Ecol. Evol. 3, 3925–3934. doi: 10.1002/ece3.760
Chivers, D. P., Wisenden, B. D., Hindman, C. J., Michalak, T. A., Kusch, R. C., Kaminskyj, S. G. W., et al. (2007). Epidermal “alarm substance” cells of fishes maintained by non-alarm functions: possible defence against pathogens, parasites and UVB radiation. Proc. R. Soc. B Biol. Sci. 274, 2611–2619. doi: 10.1098/rspb.2007.0709
Claiborne, J. B., and Heisler, N. (1986). Acid-base regulation and ion transfers in the carp (Cyprinus carpio): pH compensation during graded long- and short-term environmental hypercapnia, and the effect of bicarbonate infusion. J. Exp. Biol. 126, 41–61.
Clements, J., and Hunt, H. (2015). Marine animal behaviour in a high CO2 ocean. Mar. Ecol. Prog. Ser. 536, 259–279. doi: 10.3354/meps11426
Collin, S. P., and Marshall, N. J. (eds). (2003). Sensory Processing in Aquatic Environments. New York, NY: Springer-Verlag.
Connaughton, M., Fine, M., and Taylor, M. H. (2002). Weakfish sonic muscle: influence of size, temperature and season. J. Exp. Biol. 205, 2183–2188.
Dall, S., GiraldeauI, L., Olsson, O., McNamara, J., and Stephens, D. (2005). Information and its use by animals in evolutionary ecology. Trends Ecol. Evol. 20, 187–193. doi: 10.1016/j.tree.2005.01.010
Davies, T. W., Duffy, J. P., Bennie, J., and Gaston, K. J. (2014). The nature, extent, and ecological implications of marine light pollution. Front. Ecol. Environ. 12, 347–355. doi: 10.1890/130281
Decho, A. W., Browne, K. A., and Zimmer-Faust, R. K. (1998). Chemical cues: why basic peptides are signal molecules in marine environments. Limnol. Oceanogr. 43, 1410–1417. doi: 10.4319/lo.1998.43.7.1410
de la Haye, K. L., Spicer, J. I., Widdicombe, S., and Briffa, M. (2011). Reduced sea water pH disrupts resource assessment and decision making in the hermit crab Pagurus bernhardus. Anim. Behav. 82, 495–501. doi: 10.1016/j.anbehav.2011.05.030
Denny, M. W. (1987). Life in the maelstrom: the biomechanics of wave-swept rocky shores. Trends Ecol. Evol. 2, 61–66. doi: 10.1016/0169-5347(87)90150-9
Di Rocco, R. T., Belanger, C. F., Imre, I., Brown, G. E., and Johnson, N. S. (2014). Daytime avoidance of chemosensory alarm cues by adult sea lamprey (Petromyzon marinus). Can. J. Fish. Aquat. Sci. 71, 824–830. doi: 10.1139/cjfas-2013-0381
Dias, P. C. (1996). Sources and sinks in population biology. Trends Ecol. Evol. 11, 326–330. doi: 10.1016/0169-5347(96)10037-9
Doney, S. C., Fabry, V. J., Feely, R. A., and Kleypas, J. A. (2008). Ocean acidification: the other CO2 problem. Ann. Rev. Mar. Sci. 1, 169–192.
Doropoulos, C., Ward, S., Diaz-Pulido, G., Hoegh-Guldberg, O., and Mumby, P. J. (2012). Ocean acidification reduces coral recruitment by disrupting intimate larval-algal settlement interactions. Ecol. Lett. 15, 338–346. doi: 10.1111/j.1461-0248.2012.01743.x
Dushaw, B. D., Worcester, P. F., Cornuelle, B. D., and Howe, B. M. (1993). On equations for the speed of sound in seawater. J. Acoust. Soc. Am. 93, 255–275.
Eckman, J., Duggins, D., and Siddon, C. (2003). Current and wave dynamics in the shallow subtidal: implications to the ecology of understory and surface-canopy kelps. Mar. Ecol. Prog. Ser. 265, 45–56. doi: 10.3354/meps265045
Ferrari, M. C. O., McCormick, M. I., Munday, P. L., Meekan, M. G., Dixson, D. L., Lönnstedt, O., et al. (2012). Effects of ocean acidification on visual risk assessment in coral reef fishes. Funct. Ecol. 26, 553–558. doi: 10.1111/j.1365-2435.2011.01951.x
Fine, M. L., and Waybright, T. D. (2015). Grunt variation in the oyster toadfish Opsanus tau: effect of size and sex. PeerJ 3:e1330. doi: 10.7717/peerj.1330
Forsgren, E., Dupont, S., Jutfelt, F., and Amundsen, T. (2013). Elevated CO2 affects embryonic development and larval phototaxis in a temperate marine fish. Ecol. Evol. 3, 3637–3646. doi: 10.1002/ece3.709
Forward, R. B., Bourla, M. H., Lessios, N. N., and Cohen, J. H. (2009). Orientation to shorelines by the supratidal amphipod Talorchestia longicornis: wavelength specific behavior during sun compass orientation. J. Exp. Mar. Biol. Ecol. 376, 102–109. doi: 10.1016/j.jembe.2009.06.016
Frommel, A. Y., Margulies, D., Wexler, J. B., Stein, M. S., Scholey, V. P., Williamson, J. E., et al. (2016). Ocean acidification has lethal and sub-lethal effects on larval development of yellowfin tuna, Thunnus albacares. J. Exp. Mar. Biol. Ecol. 482, 18–24. doi: 10.1016/j.jembe.2016.04.008
Gaylord, B., Kroeker, K. J., Sunday, J. M., Anderson, K. M., Barry, J. P., Brown, N. E., et al. (2015). Ocean acidification through the lens of ecological theory. Ecology 96, 3–15. doi: 10.1890/14-0802.1
Genner, M. J., Halliday, N. C., Simpson, S. D., Southward, A. J., Hawkins, S. J., and Sims, D. W. (2010). Temperature-driven phenological changes within a marine larval fish assemblage. J. Plankton Res. 32, 699–708. doi: 10.1093/plankt/fbp082
Gerber, L. R., Mancha-Cisneros, M. D. M., O’Connor, M. I., and Selig, E. R. (2014). Climate change impacts on connectivity in the ocean: implications for conservation. Ecosphere 5, 1–18.
Gilman, S. E., Urban, M. C., Tewksbury, J., Gilchrist, G. W., and Holt, R. D. (2010). A framework for community interactions under climate change. Trends Ecol. Evol. 25, 325–331. doi: 10.1016/j.tree.2010.03.002
Gravem, S. A., and Morgan, S. G. (2016). Prey state alters trait-mediated indirect interactions in rocky tide pools. Funct. Ecol. 30, 1574–1582. doi: 10.1111/1365-2435.12628
Gremillet, D., Nazirides, T., Nikolaou, H., and Crivelli, A. J. (2012). Fish are not safe from great cormorants in turbid water. Aquat. Biol. 15, 187–194. doi: 10.3354/ab00430
Hairston, N. G., Smith, F. E., and Slobodkin, L. B. (1960). Community Structure, population control, and competition. Am. Nat. 94, 421–425. doi: 10.1086/282146
Hale, R., and Swearer, S. E. (2016). Ecological traps: current evidence and future directions. Proc. R. Soc. B Biol. Sci. 283:20152647. doi: 10.1098/rspb.2015.2647
Hale, R., Treml, E. A., and Swearer, S. E. (2015). Evaluating the metapopulation consequences of ecological traps. Proc. R. Soc. B Biol. Sci. 282:20142930. doi: 10.1098/rspb.2014.2930
Hamilton, T. J., Holcombe, A., and Tresguerres, M. (2013). CO2-induced ocean acidification increases anxiety in rockfish via alteration of GABAA receptor functioning. Proc. R. Soc. B Biol. Sci. 281:20132509. doi: 10.1098/rspb.2013.2509
Hanski, I., and Gilpin, M. (1991). Metapopulation dynamics: brief history and conceptual domain. Biol. J. Linn. Soc. 42, 3–16. doi: 10.1016/b978-0-12-284120-0.50004-8
Hardege, J. D., Rotchell, J. M., Terschak, J., and Greenway, G. M. (2011). Analytical challenges and the development of biomarkers to measure and to monitor the effects of ocean acidification. TrAC Trends Anal. Chem. 30, 1320–1326. doi: 10.1016/j.trac.2011.07.004
Harley, C. D. G., Randall Hughes, A., Hultgren, K. M., Miner, B. G., Sorte, C. J. B., Thornber, C. S., et al. (2006). The impacts of climate change in coastal marine systems. Ecol. Lett. 9, 228–241. doi: 10.1111/j.1461-0248.2005.00871.x
Hartman, E. J., and Abrahams, M. V. (2000). Sensory compensation and the detection of predators: the interaction between chemical and visual information. Proc. R. Soc. Lond. Ser. B Biol. Sci. 267, 571–575. doi: 10.1098/rspb.2000.1039
Hay, M. E. (2009). Marine Chemical Ecology: chemical signals and cues structure marine populations, communities, and ecosystems. Annu. Rev. Mar. Sci. 1, 193–212. doi: 10.1146/annurev.marine.010908.163708
Helmuth, B. S., and Hofmann, G. E. (2001). Microhabitats, thermal heterogeneity, and patterns of physiological stress in the rocky intertidal zone. Biol. Bull. 201, 374–384. doi: 10.2307/1543615
Hess, E. H. (2010). Temperature as a regulator of the attack-response of Betta splendens. Z. Tierpsychol. 9, 379–382. doi: 10.1111/j.1439-0310.1952.tb01657.x
Hester, K. C., Peltzer, E. T., Kirkwood, W. J., and Brewer, P. G. (2008). Unanticipated consequences of ocean acidification: a noisier ocean at lower pH. Geophys. Res. Lett. 35:L19601.
Heuer, R. M., and Grosell, M. (2014). Physiological impacts of elevated carbon dioxide and ocean acidification on fish. Am. J. Physiol. Regul. Integr. Comp. Physiol. 307, R1061–R1084. doi: 10.1152/ajpregu.00064.2014
Heuschele, J., Mannerla, M., Gienapp, P., and Candolin, U. (2009). Environment-dependent use of mate choice cues in sticklebacks. Behav. Ecol. 20, 1223–1227. doi: 10.1093/beheco/arp123
Himes, A. R., Balschi, W. S., Pelletier, G., and Frederich, M. (2017). Color phase—specific ion regulation of the European green crab Carcinus maenas in an oscillating salinity environment. J. Shellfish Res. 36, 465–479. doi: 10.2983/035.036.0218
Hofmann, G. E., Smith, J. E., Johnson, K. S., Send, U., Levin, L. A., Micheli, F., et al. (2011). High-frequency dynamics of ocean pH: a multi-ecosystem comparison. PLoS One 6:e28983. doi: 10.1371/journal.pone.0028983
Holveck, M.-J., and Riebel, K. (2010). Low-quality females prefer low-quality males when choosing a mate. Proc. R. Soc. B Biol. Sci. 277, 153–160. doi: 10.1098/rspb.2009.1222
Ilyina, T., Zeebe, R. E., and Brewer, P. G. (2010). Future ocean increasingly transparent to low-frequency sound owing to carbon dioxide emissions. Nat. Geosci. 3, 18–22. doi: 10.1038/ngeo719
IPCC (2013). “Climate change 2013: the physical science basis,” in Proceedings of the Contribution of the Working Group I to the Fifth Assessment Report of the Intergovernmental Panel on Climate Change, eds T. F. Stocker, D. Qin, G. K. Plattner, M. Tignor, S. K. Allen, and J. Boschung (Cambridge: Cambridge University Press).
Jellison, B. M., Ninokawa, A. T., Hill, T. M., Sanford, E., and Gaylord, B. (2016). Ocean acidification alters the response of intertidal snails to a key sea star predator. Proc. R. Soc. Lond. B Biol. Sci. 283:20160890. doi: 10.1098/rspb.2016.0890
Jennions, M. D., and Petrie, M. (1997). Variation in mate choice and mating preferences: a review of causes and consequences. Biol. Rev. 72, 283–327. doi: 10.1111/j.1469-185x.1997.tb00015.x
Johansson, B. G., and Jones, T. M. (2007). The role of chemical communication in mate choice. Biol. Rev. 82, 265–289. doi: 10.1111/j.1469-185x.2007.00009.x
Keeling, R. F., Körtzinger, A., and Gruber, N. (2010). Ocean deoxygenation in a warming world. Annu. Rev. Mar. Sci. 2, 199–229. doi: 10.1146/annurev.marine.010908.163855
Kelley, J. L., Chapuis, L., Davies, W. I. L., and Collin, S. P. (2018). Sensory system responses to human-induced environmental change. Front. Ecol. Evol. 6:95. doi: 10.3389/fevo.2018.00095
Kelly, M. W., Pankey, M. S., DeBiasse, M. B., and Plachetzki, D. C. (2017). Adaptation to heat stress reduces phenotypic and transcriptional plasticity in a marine copepod. Funct. Ecol. 31, 398–406. doi: 10.1111/1365-2435.12725
Kingsford, M. J., Leis, J. M., Shanks, A., Lindeman, K. C., Morgan, S. G., and Pineda, J. (2002). Sensory environments, larval abilities and local self-recruitment. Bull. Mar. Sci. 70, 309–340.
Koehl, M. A. R. (2006). The fluid mechanics of arthropod sniffing in turbulent odor plumes. Chem. Senses 31, 93–105. doi: 10.1093/chemse/bjj009
Krahforst, C. S., Walsh, J. P., Sprague, M. W., Eulie, D. O., Corbett, D. R., and Luczkovich, J. J. (2012). “Influence of turbidity on the incidence of sound production in Atlantic Croaker (Micropogonias undulatus) in Pamlico Sound, North Carolina,” in The Effects of Noise on Aquatic Life, eds A. N. Popper and A. Hawkins (New York, NY: Springer), 169–171. doi: 10.1007/978-1-4419-7311-5_38
Kroeker, K. J., Sanford, E., Rose, J. M., Blanchette, C. A., Chan, F., Chavez, F. P., et al. (2016). Interacting environmental mosaics drive geographic variation in mussel performance and predation vulnerability. Ecol. Lett. 19, 771–779. doi: 10.1111/ele.12613
Ladich, F., and Schleinzer, G. (2015). Effect of temperature on acoustic communication: sound production in the croaking gourami (labyrinth fishes). Comp. Biochem. Physiol. Part A Mol. Integr. Physiol. 182, 8–13. doi: 10.1016/j.cbpa.2014.11.013
Lailvaux, S. P., and Kasumovic, M. M. (2011). Defining individual quality over lifetimes and selective contexts. Proc. R. Soc. B Biol. Sci. 278, 321–328. doi: 10.1098/rspb.2010.1591
Large, S., Smee, D., and Trussell, G. (2011). Environmental conditions influence the frequency of prey responses to predation risk. Mar. Ecol. Prog. Ser. 422, 41–49. doi: 10.3354/meps08930
Leahy, S. M., McCormick, M. I., Mitchell, M. D., and Ferrari, M. C. O. (2011). To fear or to feed: the effects of turbidity on perception of risk by a marine fish. Biol. Lett. 7, 811–813. doi: 10.1098/rsbl.2011.0645
Loew, E. W., and McFarland, W. N. (1990). “The underwater visual environment,” in The Visual System of Fish, eds R. H. Douglas and M. B. A. Djamgoz (London: Chapman & Hall), 1–44.
Lohmann, K., Lohmann, C., and Endres, C. (2008). The sensory ecology of ocean navigation. J. Exp. Biol. 211, 1719–1728. doi: 10.1242/jeb.015792
Lu, X. X., Ran, L. S., Liu, S., Jiang, T., Zhang, S. R., and Wang, J. J. (2013). Sediment loads response to climate change: a preliminary study of eight large Chinese rivers. Int. J. Sediment. Res. 28, 1–14. doi: 10.1016/s1001-6279(13)60013-x
Luttbeg, B., Rowe, L., and Mangel, M. (2003). Prey state and experimental design affect relative size of trait- and density-mediated indirect effects. Ecology 84, 1140–1150. doi: 10.1890/0012-9658(2003)084
Luttbeg, B., and Trussell, G. C. (2013). How the informational environment shapes how prey estimate predation risk and the resulting indirect effects of predators. Am. Nat. 181, 182–194. doi: 10.1086/668823
Macdonald, J. A., Montgomery, J. C., and Wells, R. M. G. (1988). The physiology of McMurdo Sound fishes: current New Zealand research. Comp. Biochem. Physiol. Part B Comp. Biochem. 90, 567–578. doi: 10.1016/0305-0491(88)90297-0
Macmillan, N. A., and Creelman, C. D. (2005). Detection Theory: A User’s Guide, 2nd Edn. Mahwah, NJ: Lawrence Erlbaum Associates.
Maiditsch, I. P., and Ladich, F. (2014). Effects of temperature on auditory sensitivity in eurythermal fishes: common carp Cyprinus carpio (Family Cyprinidae) versus Wels Catfish Silurus glanis (Family Siluridae). PLoS One 9:e108583. doi: 10.1371/journal.pone.0108583
Manríquez, P. H., Jara, M. E., Mardones, M. L., Navarro, J. M., Torres, R., Lardies, M. A., et al. (2013). Ocean acidification disrupts prey responses to predator cues but not net prey shell growth in Concholepas concholepas (loco). PLoS One 8:e68643. doi: 10.1371/journal.pone.0068643
Matassa, C. M., and Trussell, G. C. (2014). Prey state shapes the effects of temporal variation in predation risk. Proc. R. Soc. B Biol. Sci. 281:20141952. doi: 10.1098/rspb.2014.1952
Matt, S., Hou, W., Woods, S., Goode, W., Jarosz, E., and Weidemann, A. (2014). A novel platform to study the effect of small-scale turbulent density fluctuations on underwater imaging in the ocean. Methods Oceanogr. 11, 39–58. doi: 10.1016/j.mio.2015.01.001
Meager, J. J., Solbakken, T., Utne-Palm, A. C., and Oen, T. (2005). Effects of turbidity on the reactive distance, search time, and foraging success of juvenile Atlantic cod (Gadus morhua). Can. J. Fish. Aquat. Sci. 62, 1978–1984. doi: 10.1139/f05-104
Menge, B. A., and Sutherland, J. P. (1987). Community regulation: variation in disturbance, competition, and predation in relation to environmental stress and recruitment. Am. Nat. 130, 730–757. doi: 10.1086/284741
Milinski, M., and Bakker, T. C. M. (1990). Female sticklebacks use male coloration in mate choice and hence avoid parasitized males. Nature 344, 330–333. doi: 10.1038/344330a0
Montgomery, J. C., Tolimieri, N., and Haine, O. S. (2001). Active habitat selection by pre-settlement reef fishes. Fish Fish. 2, 261–277. doi: 10.1046/j.1467-2960.2001.00053.x
Munday, P. L., Dixson, D. L., Donelson, J. L., Jones, G. P., Pratchett, M. S., Devitsina, G. V., et al. (2009). Ocean acidification impairs olfactory discrimination and homing ability of a marine fish. Proc. Natl. Acad. Sci. U.S.A. 106, 1848–1852. doi: 10.1073/pnas.0809996106
Munday, P. L., Dixson, D. L., McCormick, M. I., Meekan, M., Ferrari, M. C. O., and Chivers, D. P. (2010). Replenishment of fish populations is threatened by ocean acidification. Proc. Natl. Acad. Sci. U.S.A. 107, 12930–12934. doi: 10.1073/pnas.1004519107
Munk, W. H., Forbes, A. M. G., Munk, W. H., and Forbes, A. M. G. (1989). Global ocean warming: an acoustic measure? J. Phys. Oceanogr. 19, 1765–1778. doi: 10.1175/1520-0485(1989)019<1765:gowaam>2.0.co;2
Nagelkerken, I., Doney, S. C., and Munday, P. L. (2019). “Consequences of anthropogenic changes in the sensory landscape of marine animals,” in Oceanography and Marine Biology: An Annual Review, Vol. 57, eds S. T. Hawkins, A. L. Allcock, A. E. Bates, L. B. Firth, I. P. Smith, S. E. Swearer, and P. A. Todd (Boca Raton: CRC Press, Taylor & Francis Group), 229–264.
Nagelkerken, I., and Munday, P. L. (2016). Animal behaviour shapes the ecological effects of ocean acidification and warming: moving from individual to community-level responses. Glob. Change Biol. 22, 974–989. doi: 10.1111/gcb.13167
Neenan, S. T. V., Piper, R., White, P. R., Kemp, P., Leighton, T. G., and Shaw, P. J. (2016). “Does masking matter? Shipping noise and fish vocalizations,” in The Effects of Noise on Aquatic Life II. Advances in Experimental Medicine and Biology, Vol. 875, eds A. Popper and A. Hawkins (New York, NY: Springer), 747–753. doi: 10.1007/978-1-4939-2981-8_91
Nilsson, G. E., Dixson, D. L., Domenici, P., McCormick, M. I., Sørensen, C., Watson, S.-A., et al. (2012). Near-future carbon dioxide levels alter fish behaviour by interfering with neurotransmitter function. Nat. Clim. Change 2, 201–204. doi: 10.1038/nclimate1352
Nowicki, S., Searcy, W., and Peters, S. (2002). Brain development, song learning and mate choice in birds: a review and experimental test of the “nutritional stress hypothesis”. J. Comp. Physiol. A Neuroethol. Sens. Neural Behav. Physiol. 188, 1003–1014. doi: 10.1007/s00359-002-0361-3
O’Connor, M. I., Selig, E. R., Pinsky, M. L., and Altermatt, F. (2012). Toward a conceptual synthesis for climate change responses. Glob. Ecol. Biogeogr. 21, 693–703. doi: 10.1111/j.1466-8238.2011.00713.x
Orr, J. C., Fabry, V. J., Aumont, O., Bopp, L., Doney, S. C., Feely, R. A., et al. (2005). Anthropogenic ocean acidification over the twenty-first century and its impact on calcifying organisms. Nature 437, 681–686. doi: 10.1038/nature04095
Paine, R. T. (1966). Food web complexity and species diversity. Am. Nat. 100, 65–75. doi: 10.1086/282400
Pineda, J. (1991). Predictable upwelling and the shoreward transport of planktonic larvae by internal tidal bores. Science 253, 548–549. doi: 10.1126/science.253.5019.548
Pistevos, J. C. A., Nagelkerken, I., Rossi, T., and Connell, S. D. (2017). Antagonistic effects of ocean acidification and warming on hunting sharks. Oikos 126, 241–247.
Porteus, C. S., Hubbard, P. C., Uren Webster, T. M., van Aerle, R., Canário, A. V. M., Santos, E. M., et al. (2018). Near-future CO2 levels impair the olfactory system of a marine fish. Nat. Clim. Change 8, 737–743. doi: 10.1038/s41558-018-0224-8
Portner, H. O. (2002). Climate variations and the physiological basis of temperature dependent biogeography: systemic to molecular hierarchy of thermal tolerance in animals. Comp. Biochem. Physiol. A Mol. Integr. Physiol. 132, 739–761. doi: 10.1016/s1095-6433(02)00045-4
Pruett, J. L., and Weissburg, M. J. (2018). Hydrodynamics affect predator controls through physical and sensory stressors. Oecologia 186, 1079–1089. doi: 10.1007/s00442-018-4092-8
Reid, D., and Aldrich, J. (1989). Variations in response to environmental hypoxia of different colour forms of the shore crab, Carcinus maenas. Comp. Biochem. Physiol. Part A Physiol. 92, 535–539. doi: 10.1016/0300-9629(89)90361-7
Reid, D. G., Abelló, P., Kaiser, M. J., and Warman, C. G. (1997). Carapace colour, inter-moult duration and the behavioural and physiological ecology of the shore crab Carcinus maenas. Estuar. Coast. Shelf Sci. 44, 203–211. doi: 10.1006/ecss.1996.0212
Robertson, B. A., and Hutto, R. L. (2006). A framework for understanding ecological traps and an evaluation of existing evidence. Ecology 87, 1075–1085. doi: 10.1890/0012-9658(2006)87
Rodriguez, S. R., Ojeda, F. P., and Inestrosa, N. C. (1993). Settlement of benthic marine invertebrates. Mar. Ecol. Prog. Ser. 97, 193–207. doi: 10.3354/meps097193
Roggatz, C. C., Lorch, M., Hardege, J. D., and Benoit, D. M. (2016). Ocean acidification affects marine chemical communication by changing structure and function of peptide signalling molecules. Glob. Change Biol. 22, 3914–3926. doi: 10.1111/gcb.13354
Rosenthal, G. G., and Stuart-Fox, D. (2012). “Environmental disturbance and animal communication,” in Behavioural Responses to a Changing World, eds U. Candolin and B. B. M. Wong (Oxford: Oxford University Press), 280.
Sabine, C. L., Feely, R. A., Gruber, N., Key, R. M., Lee, K., Bullister, J. L., et al. (2004). The oceanic sink for anthropogenic CO2. Science 305, 367–371. doi: 10.1126/science.1097403
Schlaepfer, M. A., Runge, M. C., and Sherman, P. W. (2002). Ecological and evolutionary traps. Trends Ecol. Evol. 17, 474–480. doi: 10.1016/s0169-5347(02)02580-6
Schlaepfer, M. A., Sherman, P. W., Blossey, B., and Runge, M. C. (2005). Introduced species as evolutionary traps. Ecol. Lett. 8, 241–246. doi: 10.1111/j.1461-0248.2005.00730.x
Schlaepfer, M. A., Sherman, P. W., and Runge, M. C. (2010). “Decision making, environmental change, and population persistence,” in Evolutionary Behavioral Ecology, eds D. E. Westneat and C. W. Fox (Oxford: Oxford University Press), 506–515.
Schmidt, K. A., Dall, S. R. X., and van Gils, J. A. (2010). The ecology of information: an overview on the ecological significance of making informed decisions. Oikos 119, 304–316. doi: 10.1111/j.1600-0706.2009.17573.x
Schmitz, O. J., Beckerman, A. P., and O’Brien, K. M. (1997). Behaviorally mediated trophic cascades: effects of predation risk on food web interactions. Ecology 78, 1388–1399. doi: 10.1890/0012-9658(1997)078
Schunter, C., Welch, M. J., Nilsson, G. E., Rummer, J. L., Munday, P. L., and Ravasi, T. (2018). An interplay between plasticity and parental phenotype determines impacts of ocean acidification on a reef fish. Nat. Ecol. Evol. 2, 334–342. doi: 10.1038/s41559-017-0428-8
Schwab, R. L., and Brockmann, H. J. (2007). The role of visual and chemical cues in the mating decisions of satellite male horseshoe crabs, Limulus polyphemus. Anim. Behav. 74, 837–846. doi: 10.1016/j.anbehav.2007.01.012
Shine, R. (2005). All at sea: aquatic life modifies mate-recognition modalities in sea snakes (Emydocephalus annulatus, Hydrophiidae). Behav. Ecol. Sociobiol. 57, 591–598. doi: 10.1007/s00265-004-0897-z
Sih, A. (2013). Understanding variation in behavioural responses to human-induced rapid environmental change: a conceptual overview. Anim. Behav. 85, 1077–1088. doi: 10.1016/j.anbehav.2013.02.017
Sih, A., Ferrari, M. C. O., and Harris, D. J. (2011). Evolution and behavioural responses to human-induced rapid environmental change. Evol. Appl. 4, 367–387. doi: 10.1111/j.1752-4571.2010.00166.x
Simpson, S. D., Munday, P. L., Wittenrich, M. L., Manassa, R., Dixson, D. L., Gagliano, M., et al. (2011). Ocean acidification erodes crucial auditory behaviour in a marine fish. Biol. Lett. 7, 917–920. doi: 10.1098/rsbl.2011.0293
Smee, D. L., Ferner, M. C., and Weissburg, M. J. (2008). Alteration of sensory abilities regulates the spatial scale of nonlethal predator effects. Oecologia 156, 399–409. doi: 10.1007/s00442-008-0995-0
Smee, D. L., and Weissburg, M. J. (2006). Clamming up: environmental forces diminish the perceptive ability of bivalve prey. Ecology 87, 1587–1598. doi: 10.1890/0012-9658(2006)87
Smyth, T. J. (2011). Penetration of UV irradiance into the global ocean. J. Geophys. Res. 116:C11020.
Sokolova, I. M. (2013). Energy-limited tolerance to stress as a conceptual framework to integrate the effects of multiple stressors. Integr. Comp. Biol. 53, 597–608. doi: 10.1093/icb/ict028
Sol, D., Duncan, R. P., Blackburn, T. M., Cassey, P., and Lefebvre, L. (2005). Big brains, enhanced cognition, and response of birds to novel environments. Proc. Natl. Acad. Sci. U.S.A. 102, 5460–5465. doi: 10.1073/pnas.0408145102
Sol, D., Timmermans, S., and Lefebvre, L. (2002). Behavioural flexibility and invasion success in birds. Anim. Behav. 63, 495–502. doi: 10.1006/anbe.2001.1953
Strod, T., Izhaki, I., Arad, Z., and Katzir, G. (2008). Prey detection by great cormorant (Phalacrocorax carbo sinensis) in clear and in turbid water. J. Exp. Biol. 211, 866–872. doi: 10.1242/jeb.014324
Sullivan, P. P., Romero, L., McWilliams, J. C., and Melville, W. K. (2012). Transient evolution of Langmuir turbulence in ocean boundary layers driven by hurricane winds and waves. J. Phys. Oceanogr. 42, 1959–1980. doi: 10.1175/jpo-d-12-025.1
Sundin, J., Berglund, A., and Rosenqvist, G. (2010). Turbidity hampers mate choice in a pipefish. Ethology 116, 713–721.
Sydeman, W. J., Garcia-Reyes, M., Schoeman, D., Rykaczewski, R., Thompson, S., Black, B., et al. (2014). Climate change and wind intensification in coastal upwelling ecosystems. Science 345, 77–80. doi: 10.1126/science.1251635
Tebben, J., Motti, C. A., Siboni, N., Tapiolas, D. M., Negri, A. P., Schupp, P. J., et al. (2015). Chemical mediation of coral larval settlement by crustose coralline algae. Sci. Rep. 5:10803. doi: 10.1038/srep10803
Telfer, A. C., and Laberge, F. (2013). Responses of Eastern red-backed salamanders (Plethodon cinereus) to chemical cues of prey presented in soluble and volatile forms. Physiol. Behav. 114–115, 6–13. doi: 10.1016/j.physbeh.2013.03.001
Tierney, A. J., and Atema, J. (1988). Behavioral responses of crayfish (Orconectes virilis and Orconectes rusticus) to chemical feeding stimulants. J. Chem. Ecol. 14, 123–133. doi: 10.1007/BF01022536
Webster, D. R., and Weissburg, M. J. (2009). The hydrodynamics of chemical cues among aquatic organisms. Annu. Rev. Fluid Mech. 41, 73–90. doi: 10.1146/annurev.fluid.010908.165240
Webster, M. M., Atton, N., Ward, A. J. W., and Hart, P. J. B. (2007). Turbidity and foraging rate in threespine sticklebacks: the importance of visual and chemical prey cues. Behaviour 144, 1347–1360. doi: 10.1163/156853907782418222
Webster, N. S., Uthicke, S., Botté, E. S., Flores, F., and Negri, A. P. (2013). Ocean acidification reduces induction of coral settlement by crustose coralline algae. Glob. Change Biol. 19, 303–315. doi: 10.1111/gcb.12008
Weilgart, L. S. (2007). The impacts of anthropogenic ocean noise on cetaceans and implications for management. Can. J. Zool. 85, 1091–1116. doi: 10.1016/j.marpolbul.2018.01.014
Weissburg, M., Smee, D. L., and Ferner, M. C. (2014). The sensory ecology of nonconsumptive predator effects. Am. Nat. 184, 141–157. doi: 10.1086/676644
Weissburg, M. J., and Zimmer-Faust, R. K. (1993). Life and death in moving fluids: hydrodynamic effects on chemosensory-mediated predation. Ecology 74, 1428–1443. doi: 10.2307/1940072
Weissburg, M. J., and Zimmer-Faust, R. K. (1994). Odor plumes and how blue crabs use them in finding prey. J. Exp. Biol. 197, 349–375.
White, C. R., Day, N., Butler, P. J., Martin, G. R., and Martin, G. (2007). Vision and foraging in cormorants: more like herons than hawks? PLoS One 2:e639. doi: 10.1371/journal.pone.0000639
Wong, B. B. M., and Candolin, U. (2015). Behavioral responses to changing environments. Behav. Ecol. 26, 665–673. doi: 10.1093/beheco/aru183
Wright, T. F., Eberhard, J. R., Hobson, E. A., Avery, M. L., and Russello, M. A. (2010). Behavioral flexibility and species invasions: the adaptive flexibility hypothesis. Ethol. Ecol. Evol. 22, 393–404. doi: 10.1080/03949370.2010.505580
Wyatt, T. D. (2014). Pheromones and Animal Behavior: Chemical Signals and Signatures, 2nd Edn. Cambridge: Cambridge University Press.
Wysocki, L. E., Montey, K., and Popper, A. N. (2009). The influence of ambient temperature and thermal acclimation on hearing in a eurythermal and a stenothermal otophysan fish. J. Exp. Biol. 212, 3091–3099. doi: 10.1242/jeb.033274
Keywords: sensory pathway, global ocean change, production, transmission, reception, cue, signal
Citation: Rivest EB, Jellison B, Ng G, Satterthwaite EV, Bradley HL, Williams SL and Gaylord B (2019) Mechanisms Involving Sensory Pathway Steps Inform Impacts of Global Climate Change on Ecological Processes. Front. Mar. Sci. 6:346. doi: 10.3389/fmars.2019.00346
Received: 04 March 2019; Accepted: 06 June 2019;
Published: 03 July 2019.
Edited by:
Scott Large, National Oceanic and Atmospheric Administration (NOAA), United StatesReviewed by:
Brian Helmuth, Northeastern University, United StatesJohan Sven Eklöf, Stockholm University, Sweden
Copyright © 2019 Rivest, Jellison, Ng, Satterthwaite, Bradley, Williams and Gaylord. This is an open-access article distributed under the terms of the Creative Commons Attribution License (CC BY). The use, distribution or reproduction in other forums is permitted, provided the original author(s) and the copyright owner(s) are credited and that the original publication in this journal is cited, in accordance with accepted academic practice. No use, distribution or reproduction is permitted which does not comply with these terms.
*Correspondence: Emily B. Rivest, ZWJyaXZlc3RAdmltcy5lZHU=
†Deceased