- 1Sorbonne Université, CNRS, IRD, MNHN, Laboratoire d’Océanographie et du Climat: Expérimentations et Approches Numériques (LOCEAN-IPSL), Paris, France
- 2IMEDEA(CSIC-UIB), Instituto Mediterráneo de Estudios Avanzados, Esporles, Spain
- 3Jet Propulsion Laboratory, California Institute of Technology, Pasadena, CA, United States
- 4Aix-Marseille Univ., Université de Toulon, CNRS, IRD, MIO UM 110, Marseille, France
- 5Ocean University of China/Pilot National Laboratory for Marine Science and Technology, Qingdao, China
- 6Norwegian Polar Institute, Tromsø, Norway
- 7Department of Marine Sciences, University of Gothenburg, Gothenburg, Sweden
- 8Department of Oceanography, University of Cape Town, Rondebosch, South Africa
- 9UMR 8539, Laboratoire de Météorologie Dynamique, École Polytechnique, ENS, CNRS, Paris, France
- 10Fisheries and Oceans Canada, Northwest Atlantic Fisheries Centre, St. John’s, NL, Canada
- 11CSIRO Oceans and Atmosphere, Antarctic Climate and Ecosystems Cooperative Research Centre, Hobart, TAS, Australia
- 12Remote Sensing Solutions, Inc., Monrovia, CA, United States
- 13Laboratoire d’Etudes en Géophysique et Océanographie Spatiale, (CNRS/CNES/IRD), Université Toulouse III, Toulouse, France
Conceived as a major new tool for climate studies, the Surface Water and Ocean Topography (SWOT) satellite mission will launch in late 2021 and will retrieve the dynamics of the oceans upper layer at an unprecedented resolution of a few kilometers. During the calibration and validation (CalVal) phase in 2022, the satellite will be in a 1-day-repeat fast sampling orbit with enhanced temporal resolution, sacrificing the spatial coverage. This is an ideal opportunity – unique for many years to come – to coordinate in situ experiments during the same period for a focused study of fine scale dynamics and their broader roles in the Earth system. Key questions to be addressed include the role of fine scales on the ocean energy budget, the connection between their surface and internal dynamics, their impact on plankton diversity, and their biophysical dynamics at the ice margin.
Introduction
Importance of Fine Scales
The oceanic fine scales (1–100 km) have relatively short time scales but crucially affect ocean physics and ecology up to the climate scale, due to the strong gradients created by energetic dynamics (Ferrari and Wunsch, 2009; Su et al., 2018). These gradients are associated with strong vertical transport, connecting the ocean’s upper layer to its interior (Lévy et al., 2001; Ferrari, 2011). The horizontal and vertical fine-scale dynamics modulate the energy cascade (McWilliams, 2016) as well as ice-sea (Manucharyan and Thompson, 2017) and air-sea (Lehahn et al., 2014; Sasaki et al., 2014; Renault et al., 2018) interactions. The temporal scale associated with these horizontal and vertical fine scales is days to weeks, the same as in many important ecological processes including phytoplankton demography and competition, and the duration of foraging trips for many marine predators. This temporal resonance is one of the reasons behind the fine-scale variability that appears in marine ecosystems and their services, including biogeochemical cycles (Lévy et al., 2012; Olita et al., 2013; McGillicuddy, 2014; d’Ovidio et al., 2015; Mahadevan, 2016; Lehahn et al., 2018), biodiversity (d’Ovidio et al., 2010; Lévy et al., 2015), fish distribution (Godø et al., 2012; Watson et al., 2018), and even foraging strategies of megafauna (Tew Kai et al., 2009; Della Penna et al., 2017).
A Troubling Gap at the Global Scale Between Fine-Scale Modeling and Observing Capacities
On the modeling side, great progress has been made in characterizing this regime over the past few decades. Physical and biophysical configurations for processes of the order of 10 s of km are now considered standard for regional circulation models. Field campaigns like AlborEx (Pascual et al., 2017), LatMix (Shcherbina et al., 2015), LATEX (Petrenko et al., 2017) have also shown that individual fine-scale features may be experimentally targeted. The frontier now stands in the integrated role of the fine scales in the Earth system: what are their net global impacts? How do their properties vary regionally and seasonally? On this topic a troubling gap has formed between models and observations. Field campaigns can target individual features, but they represent a tiny fraction of the possible ocean conditions. Moreover, most in situ studies are biased by the choice of stronger and longer-lived fine scales, which are the only ones that can be reliably tracked today with remote sensing tools. We rely on models extending these observations to the global ocean (e.g., Qiu et al., 2018), but this knowledge gap between models and observations at the global scale may hide key physical or biophysical mechanisms that models do not represent correctly.
The Role of Remote Sensing and the SWOT Mission
In order to address this knowledge gap, the scientific community has been focusing on novel platforms. Among these are satellite missions that will provide global coverage at high spatiotemporal resolutions. Remote sensing does not provide ground truth of all fine-scale physical and biophysical processes, but can provide a critical synoptic context for fine-scale features, helping to separate spatial from temporal variability, supporting strategies for in situ field campaigns, and assessing the representativeness of in situ data. A future fine-scale resolving satellite mission is the NASA/CNES SWOT (Surface Water and Ocean Topography) satellite mission, to be launched at the end of 2021 (see details in the Morrow et al., 2019 SWOT paper in this same issue). SWOT is an altimetric mission. While satellite altimetry today provides one-dimensional, along-track observations, SWOT will provide wide-swath, two-dimensional sea surface height (SSH) fields similar to sea surface temperature (SST) and ocean color fields, but without being affected by clouds, due to its microwave Ka-band radar interferometer. SWOT will directly provide a key dynamic variable of the ocean, SSH, with a 2D view at an unprecedented resolution (15–30 km in wavelength, depending on sea state: see Morrow et al., 2019 for details).
Two Phases, One Unprecedented Opportunity
The SWOT mission is characterized by two temporal phases. The first phase is for mission calibration and validation. It will last 3 months (January–March 2022) with a 1-day-repeat cycle (so-called “fast-sampling phase”). The second phase (so-called “nominal orbit”) will have a 21-day-repeat cycle and last several years (Morrow et al., unpublished). Here we focus on the unprecedented experimental opportunities offered by the fast-sampling phase in early 2022. These cloud-free SSH observations are available across large regions (120 km wide swaths and their crossing point, so-called “crossovers,” see Figure 1). These are distributed all around the globe, with a temporal resolution of 1 day. This 1-day repeat has never been available with the conventional nadir-looking altimetry in the past and is not expected from other planned missions in the future. In the following we present some of the observational opportunities for which SWOT is expected to be especially useful, as well as challenges and recommended practices for interpreting its maps. Examples of in situ campaigns that are planned under SWOT crossovers are then described.
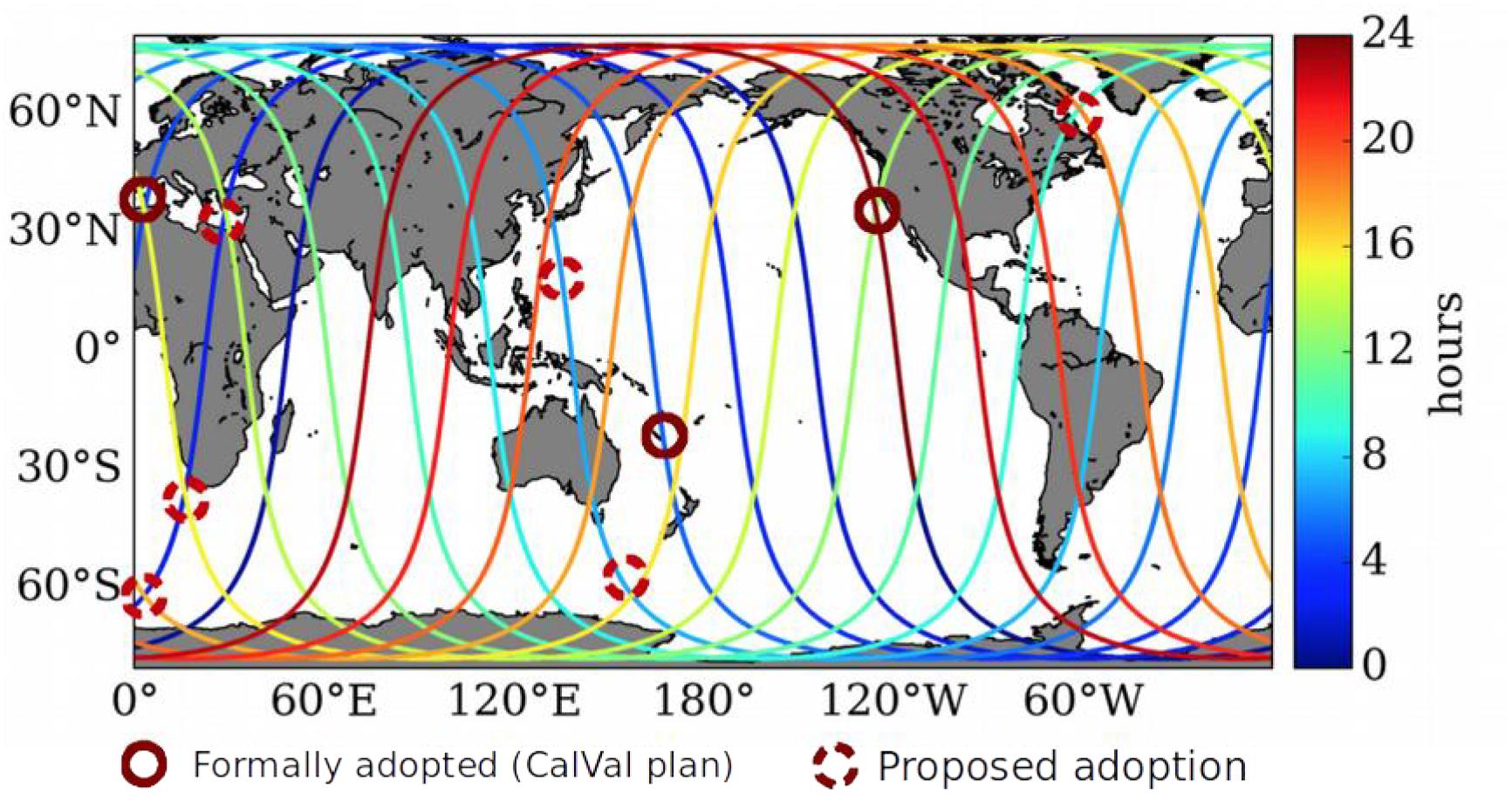
Figure 1. SWOT orbit during the fast-sampling phase [adapted from Wang et al., 2018, ©Copyright (2017) AMS]. During the first months of the mission (expected for January–March 2022), the satellite will be on a special orbit which will overfly a smaller portion of the global ocean with a repeat cycle of 1 day (twice per day on crossovers). This so-called fast-sampling phase will resolve both the spatial and the temporal variability of the ocean fine-scale features along the ∼120 km wide swath. Successively, the nominal orbit of SWOT will cover the entire globe (between 78 N and 78 S) with a repeat cycle of 21 days. The circles represent the study regions discussed in Section “Some Case Studies for the SWOT Fast-Sampling Phase.”
Science Opportunities for Physical and Biophysical Processes
Studying the energy budget in the ocean requires fine-scale observations. The dynamics of fine scales plays a key role in both the direct and inverse energy cascade (McWilliams, 2016) and therefore in the regulation of the ocean energy budget. Fine scales are associated with potential energy to kinetic energy conversion in high wavenumbers (Boccaletti et al., 2007) and eventually kinetic energy dissipation (Nikurashin et al., 2012). A precise spatial and temporal representation of these processes is required for a correct estimation of the ocean energy budget and for designing optimal parameterizations for high resolution, as well as climate resolving numerical models. For example, the intensity of the fine-scale density gradients and vertical velocities in numerical models strongly depends on the rate of kinetic energy accumulation along the direct cascade, before being dissipated by microscale non-linear processes.
Today, there are no direct fine-scale observations of ocean currents from space. Surface geostrophic currents derived from satellite altimetry maps only represent large scales >150–200 km (Chelton et al., 2011) which are mostly in quasi-geostrophic balance. At spatial scales smaller than 200 km, geostrophic or cyclo-geostrophic motions are not always dominant. SWOT SSH will observe both balanced (eddies) and unbalanced (internal tides and internal gravity waves) motions at these scales. This is an opportunity to characterize their 2D spatial structure globally, and to observe their interactions, important for the ocean energy budget, mixing and dissipation.
Biophysical couplings at fine scales present large uncertainties, introducing a notorious main source of error in representing ocean dynamics on a variety of interdisciplinary issues, ranging from the biological carbon pump and its associated export (Lévy et al., 2012; Siegel et al., 2016) to plankton diversity and spatial planning of commercial fisheries (Scales et al., 2018; Watson et al., 2018) and marine protected areas (Della Penna et al., 2017). The observations during the SWOT fast-sampling phase present a novel opportunity for studying the ecology of microbial populations, in particular when paired with high throughput techniques. Characterization of the microbial community structure by morphological techniques [automated flow cytometry Marrec et al. (2018)] and machine learning applied to microscopy as well as “-omics” techniques (e.g., Villar et al., 2015) are underway and now provide the possibility of repeatedly mapping the fine-scale planktonic community structure and dynamics on regions spanning several tens of km in a quasi-synoptic way. When deployed on the regions overflown by SWOT during the fast-sampling phase, these techniques should yield empirical evidence of the ecological effect of impulsive events tied to fine-scale physics (Talmy et al., 2014).
Finally, SWOT data during both the fast-sampling and nominal phases have a high potential for marine ecology applications. Animal telemetry studies have shown to greatly benefit from the comparison with altimetry-derived circulation features (e.g., Della Penna et al., 2017). These biologging data are the cornerstone for the spatial planning of marine protected areas. However, biologging fine-scale resolving precision, which is now routinely available, remains largely unexploited due to resolution limitations in nadir altimetry gridded products.
Challenges for Combining SWOT and in Situ Observations
Entanglement of Space and Time Variability
In most cases, a detailed understanding of the fine-scale processes associated with SSH fine-scale physical features will not be possible on the basis of satellite information alone but will require in situ information as well. In this regard, a fine-scale in situ observing network optimized for SWOT observations should ideally instrument a region ∼100 km × 100 km wide, with a ∼km horizontal spatial resolution and ideally hydrographic profiles extended up to the bottom. The key challenge, however, is the time constraint. The daily or shorter temporal resolution required to disentangle fine-scale spatial and temporal variability, currently renders a regular mapping which respects all these requirements out of the reach. The definition of a fine-scale sampling strategy is therefore a critical task which consists of choosing which aspects to favor and which to sacrifice (Wang et al., 2019). Viable solutions (Shcherbina et al., 2015; Jaffe et al., 2017; Pascual et al., 2017; Petrenko et al., 2017; Wang et al., 2018; see also section “Some Case Studies for the SWOT Fast-Sampling Phase”) include the use of an affordable number of moorings deployed on a smaller area, or along a one-dimensional array; the restriction to ship-based shallow CTD casts and to instruments in use, to minimize the occupation time at sample stations; the coordinated use of autonomous platforms; and the use of non-regular, time-dependent (i.e., adaptive) grids. All these strategies may benefit from near-real time feature detection (e.g., d’Ovidio et al., 2015) from satellite and in situ data, in order to optimize the position and orientation of the sampling grid.
Entanglement of the Balanced and Unbalanced Motion
At spatial scales <200 km, the combined observation of balanced (eddies) and unbalanced motions (internal gravity waves and internal tides) impose great challenges in interpreting and fully utilizing the high-resolution SWOT SSH data (Qiu et al., 2018). In contrast to the majority of the larger SSH features observed with nadir altimetry, not all the SSH fine-scale gradients correspond to quasi-geostrophic currents. Recommended approaches for disentangling the two components exist and need to be implemented in order to avoid errors in the interpretation of SWOT observations. First of all, the regional and seasonal variation should be considered, as the relative strength of balanced and unbalanced motion greatly varies in space and time (Torres et al., 2018). The transition scale from balanced to unbalanced motion, as well the seasonal variations of their relative strength have been documented with good accuracy from both model studies and in situ observations (Qiu et al., 2017). These data can provide valuable a priori information to disentangle balanced and unbalanced motion. Internal tides contribute a significant SSH signature at scales <200 km, and coherent internal tide corrections are being developed to remove tides from future fine-scale altimetry and in situ data (e.g., Zaron and Ray, 2017). In addition, in situ ADCP or glider measurements will also help the eddy-wave separation following the framework provided in Rainville et al. (2013).
Some Case Studies for the SWOT Fast-Sampling Phase
The primary objective of the fast-sampling phase is mission CalVal. During this period, orbit crossovers will open opportunities for challenging in situ data with several processes, which until now, mainly addressed model studies (like the ones described in section “Science Opportunities for Physical and Biophysical Processes”) on a variety of ocean regions. These opportunities are unique in providing synoptic images of the fine-scale ocean circulation twice a day. The SWOT nominal period for the fast-sampling phase is January–March 2022. With an acknowledgment of the risk of the mission being postponed due to unplanned technical delays, the SWOT Science Team encourages the international community to coordinate future fine-scale campaigns around the world so that a large number of SWOT crossovers will be occupied by in situ studies during the fast-sampling phase. Besides the principal CalVal site in the California Current System (Wang et al., 2018; Morrow et al., unpublished), we present here some other possible locations (Figure 1) and the associated planned in situ activities at various levels of maturity.
The Western Pacific: Energetic Mid-Latitude Sites and Tropical Regions With Internal Tides
The Subtropical Countercurrents in the Western Pacific is baroclinically unstable, producing energetic eddies. In this region, the Pilot National Laboratory for Marine Science and Technology (Qingdao) is planning an in situ observing system along the SWOT ground track as part of its Ocean Energy Cascading Observation Study, aiming to validate and complement the SWOT measurements. Three moorings will be deployed in a triangular shape separated by ∼20 km. They will collect hydrographic records over the upper 3000 m to calculate dynamic height and infer SSH and velocity records above the main thermocline (∼500 m). With sufficient funding support, this mooring system could be extended over a larger spatial domain by deploying additional moorings or gliders as substitutes. The collected data should provide a benchmark for validating the SWOT-measured SSH and the inversion of three-dimensional ocean flows based on SSH. Within the triangular mooring system, additional underwater and wave gliders are planned to resolve processes toward the submesoscale (∼3 km).
In the region around New Caledonia in the South-West Tropical Pacific, where strong internal tides are observed, another study is planned in the framework of the SWOT CalVal plan. Deployments of moorings, gliders, and ship-borne studies using underway CTDs and AD will be used to understand and characterize the interactions between high frequency motions and mesoscale activity.
Polar and Subpolar Regions
The Antarctic Circumpolar Current
The Southern Ocean is a major player in the heat (Liu et al., 2018) and carbon (DeVries et al., 2017) uptake and transport, with the Antarctic Circumpolar Current (ACC) being the strongest current in the world, with hotspots occurring where the current interacts with topographic features (e.g., Chapman and Morrow, 2014). In recent years, studies have pointed to fine-scale processes that are able to dominate biogeochemical transfers (e.g., Swart et al., 2015). During the 1-day repeat phase, one SWOT track will cross the ACC upstream from Macquarie Ridge, a highly energetic ocean environment. This region has a high signal-to-noise ratio in SWOT measurements compared to adjacent ACC areas (Wang et al., 2019). It is of great interest, with expected sub-mesoscale signals in the form of internal waves, sub-mesoscale eddies and other potential small-scale features while crossing some sharp fronts of the ACC. The daily-repeat SWOT measurement allows the detection of the coherence of features and their connection to in situ observations (ship-based, floats, moorings, drifters, gliders). A pre-SWOT cruise (October 2018) in the area led by IMAS/CSIRO/ACE-CRC will be the base prototype of an in situ effort to be proposed in 2022 during the SWOT fast sampling phase.
Another Southern Ocean crossover is located in the Cape Basin. Interactions between the Agulhas Current Retroflection, its associated shedding of large (order of 200 km) Agulhas Rings, circulation of the Benguela Upwelling Region/ Benguela Drift, and the northern domains of the ACC, provide a truly complex “cauldron” of oceanic flows (Dencausse et al., 2011) that have climate-relevant impacts at both regional and global scales. Large thermohaline horizontal gradients, strong shear flows and intense atmosphere-ocean exchanges (Rouault and Lutjeharms, 2000) are associated with these interactions and can fuel baroclinic instabilities that evolve rapidly and at a small scale. The protrusion of the edge of the Agulhas Bank and other bathymetric features (Agulhas Ridge, the Protea, Simpson, Wyandot, Schmit-Ott seamounts) allows for an energy exchange between a very active meso- to submesoscale flow field (Dencausse et al., 2011; Kersalé et al., 2018) and underlying topography, causing further instabilities and variability. SWOT information can be contextualized with past or ongoing observational efforts in the region (the eastern SAMBA tall moorings PIES and Argo vertical profiles, glider deployments, and monitoring lines, – Du Plessis et al., 2017; Krug et al., 2017; Kersalé et al., 2018). Logistically, the relative proximity of Cape Town, research ships and existing facilities, provide reasonable access to the site to conduct ship-based surveys and deployments of high-resolution sampling platforms such as profiling gliders and autonomous surface vehicles.
Sea-Ice Dynamics and Subpolar Seas
Other opportunities for both hemispheres come from the potential use of SWOT observations to study the interactions of the cryosphere (the ice shelves, the icebergs and the sea ice) with the open ocean. The structures are commonly observed from satellite images of sea ice but are not, or are poorly observed by available altimetry satellites due to orbit limitations, a coarse resolution or the proximity of coastlines – all issues that will be addressed by the SWOT mission. For example, Manucharyan and Thompson (2017) recently showed with a model study that eddies and filaments form in the Marginal Ice Zone (MIZ) at the time of sea ice melt, due to baroclinic instabilities caused by salinity and temperature gradients. These features persist for several days and induce large oceanic vertical velocities (10 m d-1). In addition, fine-scale physical information ought to be linked to the recent discovery of sea ice fall in algal bloom that can directly be observed from satellites (Lieser et al., 2015). The interactions between ice shelves and the ocean are also prone to form ocean mesoscale eddies at the front of ice shelves (Li et al., 2017). These “shelf-eddies” might play an important role in the transfer of heat toward the ice shelves, and on the biogeochemistry of the coastal Southern Ocean. In the Southern Hemisphere, there are several SWOT crossovers which will fall over the Antarctic ice margin zone and over the Antarctic shelf break, which therefore are good candidates for the aforementioned problems. In the Northern Hemisphere, one of the SWOT ground tracks crosses a series of hydrographic sections located in the Labrador Sea, a biologically rich sub-arctic sea regularly monitored by Fisheries and Oceans Canada (DFO) as part of the Atlantic Zone Monitoring Program. Ship time and monitoring data could be leveraged to perform a dedicated experiment during the CalVal phase.
The Mediterranean as a Lab for Developing Fine-Scale in situ Strategies
Two SWOT crossovers fall within the western and eastern Mediterranean basins, respectively. One on the northern flank of the Algerian current, between Algeria and the Balearic Island; and one in the middle of the Rhodes’ gyre. Due to its small deformation radius compared to the gaps between ground tracks at mid-latitudes, the Mediterranean sea is a region where nadir altimetry is known to capture only a small part of the mesoscale dynamics, and therefore, where SWOT is expected to provide a substantial improvement. The presence of low tides, of relatively weak internal wave dynamics (with peaks of activity mainly confined to specific and known regions), together with low cloud coverage, are further reasons for considering the Mediterranean crossovers in interdisciplinary studies.
Both sites display interesting dynamics. The western site is in a region where meanders of the Algerian current pinch off forming mesoscale eddies that propagate cyclonically from the African coast toward the Balearic abyssal plane. This Algerian current transports fresher water of Atlantic origin possibly enriched by nutrient input along the north African coast. In May 2018, the French-Spanish collaborative campaign PROTEVS-PRESWOT tested the implementation of coupling fine-scale physics with fine-scale biological measurements. The objectives of this joint experiment was to (1) evaluate the interest of the western Mediterranean SWOT crossover, (2) gain experience in multi-platform, multi-institution campaign coordination with the constraint imposed by traces of the SWOT satellite, and (3) explore the dynamics present in this region, that has been scarcely sampled in the past with only some recent high-resolution data collected by underwater gliders (Heslop et al., 2017; Aulicino et al., 2018). This work builds on several previous fine-scale experiments in the Western Mediterranean Sea (Nencioli et al., 2011; Pascual et al., 2017; Petrenko et al., 2017; Marrec et al., 2018).
Concluding Statement
The SWOT fast-sampling phase will provide a three-month window in early 2022, with intensive temporal sampling at the cost of spatial coverage. The opportunity to have in situ deployments for instrumenting under SWOT swaths during this period, particularly at crossovers, should not be missed: the comparison between in situ data and SWOT synoptic maps will provide an unprecedented view of the fine-scale variability in different dynamical regimes in multiple ocean basins. These data will serve as an empirical basis for interpreting future fine-scale observations over the next decade, quantifying the role of fine-scale dynamics in the Earth system.
Author Contributions
Fd’O, AP, JW, AD, ZJ, SM, GG, SSw, SSp, FC, BL, and RM wrote sections of the manuscript. All authors contributed to manuscript revision, read, and approved the submitted version.
Conflict of Interest Statement
YC was employed by company Remote Sensing Solution and is also affiliated with Seatrec, Inc.
The remaining authors declare that the research was conducted in the absence of any commercial or financial relationships that could be construed as a potential conflict of interest.
Acknowledgments
AP acknowledges support from the Spanish Research Agency and the European Regional Development Fund (Award no. CTM2016-78607-P/PRE-SWOT). Part of the research for this paper was carried out at the Jet Propulsion Laboratory, California Institute of Technology, under a contract with the National Aeronautics and Space Administration. JW and L. L. Fu acknowledge the support from SWOT mission. We thank the “Délégation Générale de l’Armement” which funded through the “Programme d’Etudes Amont Protevs II” the 2018 PROTEVSBIOSWOT campaign (PI Franck Dumas from the Service hydrographique et océanographique de la Marine) and makes a fruitful collaboration with the SWOT Science Team possible.
References
Aulicino, G., Cotroneo, Y., Ruiz, S., Sánchez Román, A. J., Pascual, A., Fusco, G., et al. (2018). Monitoring the algerian basin through glider observations, satellite altimetry and numerical simulations along a SARAL/AltiKa track. J. Mar. Syst. 179, 55–71. doi: 10.1016/j.jmarsys.2017.11.006
Boccaletti, G., Ferrari, R., and Fox-Kemper, B. (2007). Mixed layer instabilities and restratification. J. Phys. Oceanogr. 37, 2228–2250. doi: 10.1175/JPO3101.1
Chapman, C. C., and Morrow, R. (2014). Variability of Southern Ocean jets near topography. J. Phys. Oceanogr. 44, 676–693. doi: 10.1175/JPO-D-13-0182.1
Chelton, D. B., Gaube, P., Schlax, M. G., Early, J. J., and Samelson, R. M. (2011). The influence of nonlinear mesoscale eddies on near-surface oceanic chlorophyll. Science 334, 328–332. doi: 10.1126/science.1208897
Della Penna, A., Koubbi, P., Cotté, C., Bon, C., Bost, C. A., and d’Ovidio, F. (2017). Lagrangian analysis of multi-satellite data in support of open ocean Marine Protected Area design. Deep Sea Res. Part II Top. Stud. Oceanogr. 140, 212–221. doi: 10.1016/j.dsr2.2016.12.014
Dencausse, G., Arhan, M., and Speich, S. (2011). Is there a continuous subtropical front south of africa? J. Geophys. Res. 116:14. doi: 10.1029/2010JC006587
DeVries, T., Holzer, M., and Primeau, F. (2017). Recent increase in oceanic carbon uptake driven by weaker upper-ocean overturning. Nature 543, 215–218. doi: 10.1038/nature21068
d’Ovidio, F., De Monte, S., Alvain, S., Dandonneau, Y., and Lévy, M. (2010). Fluid dynamical niches of phytoplankton types. Proc. Natl. Acad. Sci. U.S.A. 107, 18366–18370. doi: 10.1073/pnas.1004620107
d’Ovidio, F., Della Penna, A., Trull, T. W., Nencioli, F., Pujol, M. I., Rio, M. H., et al. (2015). The biogeochemical structuring role of horizontal stirring: lagrangian perspectives on iron delivery downstream of the Kerguelen plateau. Biogeosciences 12, 5567–5581. doi: 10.5194/bg-12-5567-2015
Du Plessis, M., Swart, S., Ansorge, I. J., and Mahadevan, A. (2017). Submesoscale processes accelerate seasonal restratification in the Subantarctic Ocean. J. Geophys. Res. 122, doi: 10.1002/2016JC012494
Ferrari, R. (2011). Ocean science. A frontal challenge for climate models. Science 332, 316–317. doi: 10.1126/science.1203632
Ferrari, R., and Wunsch, C. (2009). Ocean circulation kinetic energy: reservoirs, sources, and sinks. Annu. Rev. Fluid Mech. 41, 253–282. doi: 10.1146/annurev.fluid.40.111406.102139
Godø, O. R., Samuelsen, A., Macaulay, G. J., Patel, R., Hjøllo, S. S., Horne, J., et al. (2012). Mesoscale eddies are oases for higher trophic marine life. PLoS One 7:e30161. doi: 10.1371/journal.pone.0030161
Heslop, E. E., Saìnchez-Romaìn, A., Pascual, A., Rodriìguez, D., Reeve, K. A., FaugeÌre, Y., et al. (2017). Sentinel-3A views ocean variability more accurately at finer resolution. Geophys. Res. Lett. 44, 367–312. doi: 10.1002/2017GL076244
Jaffe, J. S., Franks, P. J. S., Roberts, P. L. D., Mirza, D., Schurgers, C., Kastner, R., et al. (2017). A swarm of autonomous miniature underwater robot drifters for exploring submesoscale ocean dynamics. Nat. Commun. 8:14189. doi: 10.1038/ncomms14189
Kersalé, M., Lamont, T., Speich, S., Terre, T., Laxenaire, R., Roberts, M. J., et al. (2018). Moored observations of mesoscale features in the Cape Basin: characteristics and local impacts on water mass distributions. Ocean Sci. 14, 923–945. doi: 10.5194/os-14-923-2018
Krug, M., Swart, S., and Gula, J. (2017). Submesoscale cyclones in the agulhas current. Geophys. Res. Lett. 44, 346–354. doi: 10.1002/2016GL071006
Lehahn, Y., d’Ovidio, F., and Koren, I. (2018). A satellite-based lagrangian view on phytoplankton dynamics. Annu. Rev. Mar. Sci. 10, 99–119. doi: 10.1146/annurev-marine-121916-063204
Lehahn, Y., Koren, I., Rudich, Y., Bidle, K. D., Trainic, M., Flores, J. M., et al. (2014). Decoupling atmospheric and oceanic factors affecting aerosol loading over a cluster of mesoscale North Atlantic eddies. Geophys. Res. Lett. 41, 4075–4081. doi: 10.1002/2014GL059738
Lévy, M., Ferrari, R., Franks, P. J. P., Martin, A. P., and Riviere, P. (2012). Bringing physics to life at the submesoscale. Geophys. Res. Lett. 39:L14602. doi: 10.1029/2012GL052756
Lévy, M., Jahn, O., Dutkiewicz, S., Follows, M. J., and d’Ovidio, F. (2015). The dynamical landscape of marine phytoplankton diversity. J. R. Soc. Interface 12:20150481. doi: 10.1098/rsif.2015.0481
Lévy, M., Klein, P., and Treguier, A. M. (2001). Impact of sub-mesoscale physics on production and subduction of phytoplankton in an oligotrophic regime. J. Mar. Res. 59, 535–565. doi: 10.1357/002224001762842181
Li, Y., McGillicuddy, D. J., Dinniman, M. S., and Klinck, J. M. (2017). Processes influencing formation of low-salinity high-biomass lenses near the edge of the ross ice shelf. J. Mar. Syst. 166, 108–119. doi: 10.1016/j.jmarsys.2016.07.002
Lieser, J. L., Curran, M. A. J., Andrew, R. B., and Davidson, A. T. (2015). Antarctic slush-ice algal accumulation not quantified through conventional satellite imagery: beware the ice of march. Cryosphere Discuss. 9, 6187–6222. doi: 10.5194/tcd-9-6187-2015
Liu, W., Lu, J., Xie, S. P., and Fedorov, A. (2018). Southern Ocean heat uptake, redistribution, and storage in a warming climate: the role of meridional overturning circulation. J. Clim. 31, 4727–4743. doi: 10.1175/JCLI-D-17-0761.1
Mahadevan, A. (2016). The impact of submesoscale physics on primary productivity of plankton. Annu. Rev. Mar. Sci. 8, 161–184. doi: 10.1146/annurev-marine-010814-015912
Manucharyan, G. E., and Thompson, A. F. (2017). Submesoscale sea ice-ocean interactions in marginal ice zones. J. Geophys. Res. 122, 9455–9475. doi: 10.1002/2017JC012895
Marrec, P., Grégori, G., Doglioli, A. M., Dugenne, M., Della Penna, A., Bhairy, N., et al. (2018). Coupling physics and biogeochemistry thanks to high resolution observations of the phytoplankton community structure in the north-western mediterranean sea. Biogeosciences 15, 1579–1606. doi: 10.5194/bg-2017-343
McGillicuddy, D. J. Jr. (2014). Mechanisms of physical-biological-biogeochemical interaction at the oceanic mesoscale. Ann. Rev. Mar. Sci. 8, 125–159. doi: 10.1146/annurev-marine-010814-015606
McWilliams, J. C. (2016). Submesoscale currents in the ocean. Proc. R. Soc. A 472:20160117. doi: 10.1098/rspa.2016.0117
Morrow, R., Fu, L. L., Ardhuin, F., Benkiran, M., Chapron, B., Cosme, E., et al. (2019). Global observations of fine-scale ocean surface topography with the Surface Water and Ocean Topography (SWOT) mission. Front. Mar. Sci. doi: 10.3389/fmars.2019.00232
Nencioli, F., d’ Ovidio, F., Doglioli, A. M., and Petrenko, A. A. (2011). Surface coastal circulation patterns by in-situ detection of lagrangian coherent structures. Geophys. Res. Lett. 38:L17604. doi: 10.1029/2011GL048815
Nikurashin, M., Vallis, G. K., and Adcroft, A. (2012). Routes to energy dissipation for geostrophic flows in the Southern Ocean. Nat. Geosci. 6, 48–51. doi: 10.1038/ngeo1657
Olita, A., Sparnocchia, S., Cusí, S., Fazioli, L., Sorgente, R., Tintoré, J., et al. (2013). Observations of phytoplankton spring bloom onset triggered by a density front in NW Mediterranean. Ocean Sci. Discuss. 10, 1559–1580. doi: 10.5194/osd-10-1559-2013
Pascual, A., Ruiz, S., Olita, A., Troupin, C., Claret, M., Casas, B., et al. (2017). A multiplatform experiment to unravel meso-and submesoscale processes in an intense front (AlborEx). Front. Mar. Sci. 4:39. doi: 10.3389/fmars.2017.00039
Petrenko, A. A., Doglioli, A. M., Nencioli, F., Kersalé, M., Hu, Z., and d’Ovidio, F. (2017). A review of the LATEX project: mesoscale to submesoscale processes in a coastal environment. Ocean Dyn. 67, 513–533. doi: 10.1007/s10236-017-1040-9
Qiu, B., Chen, S., Klein, P., Wang, J., Torres, H., Fu, L.-L., et al. (2018). Seasonality in transition scale from balanced to unbalanced motions in the world ocean. J. Phys. Oceanogr. 48, 591–605. doi: 10.1175/JPO-D-17-0169.1
Qiu, B., Nakano, T., Chen, S., and Klein, P. (2017). Submesoscale transition from geostrophic flows to internal waves in the northwestern Pacific upper ocean. Nat. Commun. 8:14055. doi: 10.1038/ncomms14055
Rainville, L., Lee, C. M., Rudnick, D. L., and Yang, K. C. (2013). Propagation of internal tides generated near luzon strait: observations from autonomous gliders. J. Geophys. Res. Oceans 118, 4125–4138. doi: 10.1002/jgrc.20293
Renault, L., McWilliams, J. C., and Gula, J. (2018). Dampening of submesoscale currents by air-sea stress coupling in the californian upwelling system. Sci. Rep. 8:13388. doi: 10.1038/s41598-018-31602-3
Rouault, M., and Lutjeharms, J. R. E. (2000). Air-sea exchange over an Agulhas eddy at the Subtropical Convergence. Global Atmos. Ocean Syst. 7, 125–150.
Sasaki, H., Klein, P., Qiu, B., and Sasai, Y. (2014). Impact of oceanic-scale interactions on the seasonal modulation of ocean dynamics by the atmosphere. Nat. Commun. 5, 1–8. doi: 10.1038/ncomms6636
Scales, K. L., Hazen, E. L., Jacox, M. G., Castruccio, F., Maxwell, S. M., Lewison, R. L., et al. (2018). Fisheries bycatch risk to marine megafauna is intensified in lagrangian coherent structures. Proc. Natl. Acad. Sci. U.S.A. 115, 7362–7367. doi: 10.1073/pnas.1801270115
Shcherbina, A. Y., Sundermeyer, M. A., Kunze, E., D’Asaro, E., Badin, G., Birch, D., et al. (2015). The LatMix summer campaign: submesoscale stirring in the upper ocean. Bull. Am. Meteorol. Soc. 96, 1257–1279. doi: 10.1175/BAMS-D-14-00015.1
Siegel, D. A., Buesseler, K. O., Behrenfeld, M. J., Benitez-Nelson, C. R., Boss, E., Brzezinski, M. A., et al. (2016). Prediction of the export and fate of global ocean net primary production: the exports science plan. Front. Mar. Sci. 3:22. doi: 10.3389/fmars.2016.00022
Su, Z., Wang, J., Klein, P., Thompson, A. F., and Menemenlis, D. (2018). Ocean submesoscales as a key component of the global heat budget. Nat. Commun. 9:775. doi: 10.1038/s41467-018-02983-w
Swart, S., Thomalla, S. J., and Monteiro, P. M. S. (2015). The seasonal cycle of mixed layer dynamics and phytoplankton biomass in the Sub-Antarctic Zone: a high-resolution glider experiment. J. Mar. Syst. 147, 103–115. doi: 10.1016/j.jmarsys.2014.06.002
Talmy, D., Blackford, J., Hardman-Mountford, N. J., Polimene, L., Follows, M. J., and Geider, R. J. (2014). Flexible C: N ratio enhances metabolism of large phytoplankton when resource supply is intermittent. Biogeosciences 11, 4881–4895. doi: 10.5194/bg-11-4881-2014
Tew Kai, E., Rossi, V., Sudre, J., Weimerskirch, H., Lopez, C., Hernandez-Garcia, E., et al. (2009). Top marine predators track lagrangian coherent structures. Proc. Natl. Acad. Sci. U.S.A. 106, 8245–8250. doi: 10.1073/pnas.0811034106
Torres, H., Klein, P., Menemenlis, D., Qiu, B., Su, Z., Wang, J., et al. (2018). Partitioning ocean motions into balanced motions and internal gravity waves: a modeling study in anticipation of future space missions. J. Geophys. Res. Oceans 123, 8084–8105. doi: 10.1029/2018JC014438
Villar, E., Farrant, G. K., Follows, M., Garczarek, L., Speich, S., Audic, S., et al. (2015). Ocean plankton. Environmental characteristics of Agulhas rings affect interocean plankton transport. Science 348:1261447. doi: 10.1126/science.1261447
Wang, J., Fu, L., Qiu, B., Menemenlis, D., Farrar, J. T., Chao, Y., et al. (2018). An observing system simulation experiment for the calibration and validation of the surface water ocean topography sea surface height measurement using in situ platforms. J. Atmos. Oceanic Technol. 35, 281–297. doi: 10.1175/JTECH-D-17-0076.1
Wang, J., Fu, L., Torres, H. S., Chen, S., Qiu, B., and Menemenlis, D. (2019). On the spatial scales to be resolved by the surface water and ocean topography KA-band radar interferometer. J. Atmos. Oceanic Technol. 36, 87–99. doi: 10.1175/JTECH-D-18-0119.1
Watson, J. R., Fuller, E. C., Castruccio, F. S., and Samhouri, J. F. (2018). Fishermen follow fine-scale physical ocean features for finance. Front. Mar. Sci. 5:46. doi: 10.3389/fmars.2018.00046
Keywords: remote sensing, ocean dynamics, energy cascade, biogeochemial processes, submesoscale, mesoscale
Citation: d’Ovidio F, Pascual A, Wang J, Doglioli AM, Jing Z, Moreau S, Grégori G, Swart S, Speich S, Cyr F, Legresy B, Chao Y, Fu L and Morrow RA (2019) Frontiers in Fine-Scale in situ Studies: Opportunities During the SWOT Fast Sampling Phase. Front. Mar. Sci. 6:168. doi: 10.3389/fmars.2019.00168
Received: 14 November 2018; Accepted: 15 March 2019;
Published: 30 April 2019.
Edited by:
John Siddorn, Met Office, United KingdomReviewed by:
Yann Drillet, Mercator Ocean, FrancePaolo Oddo, NATO Centre for Maritime Research and Experimentation, Italy
Copyright © 2019 d’Ovidio, Pascual, Wang, Doglioli, Jing, Moreau, Grégori, Swart, Speich, Cyr, Legresy, Chao, Fu and Morrow. This is an open-access article distributed under the terms of the Creative Commons Attribution License (CC BY). The use, distribution or reproduction in other forums is permitted, provided the original author(s) and the copyright owner(s) are credited and that the original publication in this journal is cited, in accordance with accepted academic practice. No use, distribution or reproduction is permitted which does not comply with these terms.
*Correspondence: Francesco d’Ovidio, ZnJhbmNlc2NvLmRvdmlkaW9AbG9jZWFuLWlwc2wudXBtYy5mcg==