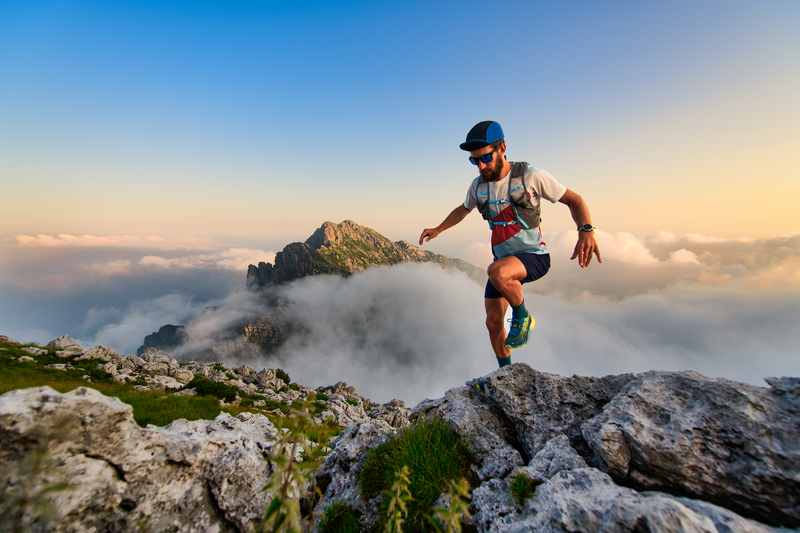
95% of researchers rate our articles as excellent or good
Learn more about the work of our research integrity team to safeguard the quality of each article we publish.
Find out more
ORIGINAL RESEARCH article
Front. Mar. Sci. , 29 March 2019
Sec. Deep-Sea Environments and Ecology
Volume 6 - 2019 | https://doi.org/10.3389/fmars.2019.00159
This article is part of the Research Topic Managing Deep-sea Ecosystems at Ocean Basin Scale, Volume 1 View all 29 articles
A correction has been applied to this article in:
Corrigendum: Hydrodynamic Connectivity of Habitats of Deep-Water Corals in Corsair Canyon, Northwest Atlantic: A Case for Cross-Boundary Conservation
Deep-water corals are significant ecosystem engineers that provide habitat complexity in the deep sea. They are indicator species of vulnerable marine ecosystems because of their slow growth and longevity, characteristics that can prolong recovery from disturbances such as fishing. For populations with discontinuous distributions, such as aggregations of deep-water corals, population connectivity is critical in regulating persistence and recovery and is one of the recommended elements in the design of area-based conservation measures. In this study, we assessed potential pathways of connectivity in the Corsair Canyon Conservation Area, off Nova Scotia, Canada, for populations of the deep-water corals Paragorgia arborea and Primnoa resedaeformis discovered in 2014 and afforded protection in 2016. Corsair Canyon located in the Canadian EEZ, ∼ 20 km from the border between Canada and the United States, is potentially receiving larvae from either the Canadian or US EEZ. In Corsair Canyon, P. arborea was very abundant at depths 484-856 m and some colonies of P. arborea were > 2 m high. These are the locally densest aggregations of P. arborea we have detected on the continental slope off Nova Scotia. We also recorded P. resedaeformis at similar depths. Colonies of both species were most often seen attached perpendicularly to a rock face, and into the current. We assessed hydrodynamic connectivity between Corsair Canyon and other canyons to the northeast and southwest along the continental slope with known occurrences of the two corals, using the ocean model Finite-Volume Community Ocean Model (University of Massachusetts-Dartmouth). Our results indicate that estimated hydrodynamic connectivity originates consistently from canyons to the southwest of Corsair Canyon, particularly Georges, and Heezen Canyons. Of these, only Georges Canyon is within Canada’s EEZ and based on our data has very sparse populations of corals that can supply potential recruits. Predicted connectivity with the Northeast Channel Coral Conservation Area occurs in winter and spring, but the complexity of circulation in those seasons needs to be resolved to confirm the strength of this connection. Our results strongly suggest that cross-boundary coordination is essential in the conservation of aggregations of deep-water corals in the northwest Atlantic, by ensuring larval exchange and connectivity.
Deep-water corals are distributed throughout the world’s oceans and are important habitat engineers that provide structural complexity (Roberts et al., 2006; Buhl-Mortensen et al., 2010) and thus habitat for attachment, nursing and foraging to other invertebrates, such as crustaceans and echinoderms, and fish (Buhl-Mortensen and Mortensen, 2004; Auster, 2005; Du Preez and Tunnicliffe, 2011). Because deep-water corals are long-lived and have slow growth rates, their populations are expected to have slow recovery from natural or anthropogenic perturbations (Clark et al., 2015). For this reason, deep-water corals have been categorized as indicator species of vulnerable marine ecosystems, and their aggregations identified for conservation from fishing activities worldwide (FAO [Food and Agriculture Organization of the United Nations], 2009; ICES [International Council for the Exploration of the Sea], 2016). Some area-based protection has been afforded already to deep-water corals in several countries, including Norway, United Kingdom, Canada, United States, and New Zealand among others, and the level of protection varies from temporary fishing closures and permanent gear bans to Marine Protected Areas.
For area-based conservation actions to be effective, several ecological criteria must be met, including population persistence over space during natural (environmental) and anthropogenic (e.g., fishing) fluctuations (Sale et al., 2006). For spatially fragmented populations or sub-populations with discontinuous distributions, such as patches of dense aggregations of deep-water corals, persistence or recovery from perturbations of individual patches is regulated in part by the degree of population connectivity with other patches, as well as self-recruitment (Kool et al., 2013). For this reason, connectivity, and in particular population connectivity [i.e., the exchange of individuals (demographic) or genes (genetic) between patches or spatially distinct populations] is strongly recommended as one of the elements in the design of networks of Marine Protected Areas in international agreements (Convention on Biological Diversity, 2007) and the scientific literature (Gerber et al., 2003; Crowder and Figueira, 2006; White et al., 2010). For sessile species, such as deep-water corals, the main mechanism to achieve connectivity is through larval dispersal; patterns in connectivity can be used to identify larval sources and sinks, as well as potential stepping stones among patches. However, in the deep sea, measures of larval dispersal and population connectivity are largely lacking (Hilário et al., 2015), making the incorporation of these process in the design of marine reserves challenging. Because of this lack of data in this habitat, as well as in many others, connectivity is often estimated based on patterns of hydrodynamic flow (here termed hydrodynamic connectivity) (e.g., Kool et al., 2015). To our knowledge, population connectivity has been evaluated in the context of conservation in the deep-sea only on a few occasions. Examples include the scleractinian coral Lophelia pertusa in a network of deep-sea MPAs in Scotland (Fox et al., 2017), and deep-sea sponges and deep-water corals in a collection of closed areas by the Northwest Atlantic Fisheries Organization in the high-seas portion of the Grand Banks and Flemish Cap to protect vulnerable marine ecosystems (Kenchington et al., 2019).
The deep-water alcyonacean corals Primnoa resedaeformis and Paragorgia arborea have been a conservation focus for Canada in the northwest Atlantic Ocean in the last 15–20 years. Species distributions models have predicted areas of suitable habitat to occur on steeply sloping regions along the shelf break and shallow continental slope for P. arborea, but wider swaths that extend onto the shelf for P. resedaeformis (Bryan and Metaxas, 2007). In practice, the known locations of these two species along the continental margin off the coast of Nova Scotia and in the Gulf of Maine are fewer than predicted (Bryan and Metaxas, 2006). Within this region, some of the densest aggregations of these two taxa on the Scotian Slope are found in the Northeast Channel Coral Conservation Area (NECCCA) established specifically to protect them. Although abundance of these corals can vary greatly across different sections within the NECCCA, it generally increases with depth from an average of 4.8 and 0.6 colonies 100 m-2 for P. resedaeformis and P. arborea, respectively, at depths < 500 m, to 12.8 and 2.5 colonies 100 m-2, for each species, respectively, at depths > 500 m (Mortensen and Buhl-Mortensen, 2004; Watanabe et al., 2009). Coral abundance within the NECCCA has increased slightly between 2001 and 2014 (Bennecke and Metaxas, 2017). Rates of recruitment vary across the NECCCA and are higher in shallower depths (658 – 671 m) amongst the coral thickets than on the floor of the canyon (860 m depth), where only a handful of colonies are present (Lacharité and Metaxas, 2013). The pattern could be the result of short dispersal distances and localized larval supply, gregarious settlement or hydrodynamics within the broader canyon. Within the coral thickets, recruitment rate was on the order of 300 colonies m-2 yr-1 (Lacharité and Metaxas, 2013), a rate that could result in abundances > 6 orders of magnitude those observed for adult colonies in the NEC.
In addition to NECCCA, four other conservation areas have been designated in the Maritimes region by the Department of Fisheries and Oceans Canada, one Marine Protected Area and three fisheries closures (DFO [Fisheries and Oceans Canada], 2017). The Gully MPA was established in 2004 with one of its conservation objectives being to protect coral diversity. The Lophelia Coral Conservation Area was established in 2004 to protect the only known live reef complex of Lophelia pertusa in Atlantic Canada. The Jordan Basin Conservation Area was established in 2016 to protect high aggregations of P. resedaeformis and other sensitive filter-feeding invertebrate communities. Lastly, also in 2016, the Corsair and Georges Canyons Conservation Area was established to protect high densities of P. arborea. All conservation areas were established based on data on the distribution of corals. However, the potential for connectivity, and thus population persistence or recovery from disturbance, such as fishing, has not been assessed for any of these closures. Because P. arborea and P. resedaeformis are found in canyons and on the continental slope from New Jersey to Newfoundland (Figure 1), potential larval sources for the closures can be located on either side of the Canada-United States border, possibly introducing geopolitical constraints to their conservation.
Figure 1. Distribution of the deep-water corals Paragorgia arborea (A) and Primnoa resedaeformis (B) along the northwest Atlantic continental shelf break from New Jersey, United States, to Newfoundland, Canada. Data are: occurrences obtained from OBIS (http://iobis.org/), NOAA (https://deepseacoraldata.noaa.gov/), Quattrini et al. (2015), and this study; US-Canada boundary: NOAA’s Ocean Service, Office of Coast Survey (OCS); bathymetry: World Ocean Base (Esri, DeLorme, GEBCO, NOAA NGDC, and other contributors; World Ocean Reference (Esri, GEBCO, NOAA, National Geographic, DeLorme, HERE, Geonames.org, and other contributors).
In this study, we assess the potential pathways of hydrodynamic connectivity in the Corsair Canyon Conservation Area (CCCA) for the populations of P. arborea and P. resedaeformis discovered in 2014 and afforded protection in 2016. The reproductive patterns and larval ecology of both species remain mostly unknown and dispersal has not been measured for either species. Primnoa resedaeformis is presumed to be a broadcast spawner (which typically have large dispersal distances) with no evidence of periodicity in gametogenesis and presumed to have non-feeding larvae, which in turn may suggest short planktonic duration (Mercier and Hamel, 2011; Waller et al., 2014). Nothing is known about the reproduction or larval ecology for P. arborea. Based on population structure on the Scotian Shelf and Slope, P. arborea appears to be genetically mixed within individual sites on the western Scotian Shelf (i.e., where CCCA is situated) and thus was proposed to also have wide dispersal (Strychar et al., 2008). Regionally wide dispersal has been proposed as the reason for an absence of regional and bathymetric genetic structure for the species (Herrera et al., 2012). Here, firstly, we describe the distribution and size frequency of P. resedaeformis and P. arborea in Corsair Canyon, as well as in two other neighboring locations that have not been described before. Secondly, we use the Finite-Volume Community Ocean Model (FVCOM; Chen et al., 2003) to simulate broad patterns of hydrodynamic connectivity that can allow us to identify potential locations of allochthonous larval supply into Corsair Canyon. Because of the lack of knowledge on the reproduction and ecology of either species, we did not focus on particular spawning depths and seasons or larval durations for our simulations. We are using the populations in CCCA as a case study because: (1) they encompass local aggregations of some of the highest densities of P. arborea recorded to date in the Canadian northwest Atlantic; and (2) the canyon is located ∼20 km from the border between Canada and the United States and may be receiving larvae from aggregations in either Canadian or US national waters. Identifying the larval origin can assist in the future selection of areas for protection to ensure the persistence of these uniquely dense aggregations and may lead toward cross-boarder collaboration in marine conservation.
We conducted a total of 5 dives in two canyons (3 in Corsair Canyon and 2 in Georges Canyon) and 1 dive at another location on the continental slope (Fiddlers Cove) between Corsair Canyon and the Northeast Channel Coral Conservation Area with the ROV ROPOS (Figure 2). The dive tracks followed exploratory transects ascending the canyon walls and ranged in length between 3.7 and 8.9 km and in depth between ∼300 and ∼2200 m (Table 1). In 2014, the two dive tracks in Corsair Canyon were designed to cover a shallower section with steep topography (R1701) and a deeper section reaching the mouth of the canyon (R1702). The tracks included 6 and 7 transects, for R1701 and R1702, respectively, during which the ROV was flying at ∼1–5 m above the seafloor and at speeds of ∼ 0.3 knots allowing the detection and enumeration of epifauna (except when ascending vertical walls when measured altitude ranged from 1 to 16.1 m). The ROV transited between those transects in water, at altitudes > 8 m above the seafloor and speeds of 0.5–0.75 knots and detection of coral colonies was less reliable. In 2017, the dive track was designed to expand the spatial coverage in Corsair Canyon toward the eastern boundary of the fisheries closure. The 2 dive tracks in Georges Canyon and the 1 dive track at Fiddlers Cove were designed to explore the steepest sections of the most pronounced incisions on the continental shelf that neighbor Corsair Canyon. Except during brief periods of in-water transiting, the transects were generally done 0.5–4 m above the seafloor (7 m at one coral location) and at speeds of ∼0.3 – 0.5 knots.
Figure 2. Location of dive tracks followed by the remotely operated vehicle ROPOS in Georges Canyon in 2017 (R2010, R2011), Corsair Canyon in 2014 (R1701 and R1702) and in 2017 (R2009) and Fiddlers Cove in 2017 (R2012) on the Canadian continental shelf break and slope. Bathymetry data from: World Ocean Base (Esri, DeLorme, GEBCO, NOAA NGDC, and other contributors; World Ocean Reference (Esri, GEBCO, NOAA, National Geographic, DeLorme, HERE, Geonames.org, and other contributors).
Table 1. Transects done by the remotely operated vehicle in submarine canyons and continental slope in Atlantic Canada.
We estimated the distribution and size of colonies of Paragorgia arborea and Primnoa resedaeformis from video by a forward-facing HD camera [Insite Pacific Zeus-Plus HD camera (10x Zoom 5.2–52 mm) mounted on a pan and tilt with extend function]. For each dive, the video footage was split into intervals of 00:10:57:440 hh:mm:ss:msmsms during the initial recording on board ship, resulting in 64 segments for dives R1701, R2009, R2010 and R2011 and 53 segments for dive R2012. We linked video segments with the dive track (ROV heading, geographic coordinates and water depth) using Ocean Floor Observation Protocol (OFOP 3.3.5 by Scientific Abyss Mapping Services) based on synchronized time (Huetten and Greinert, 2008). ROPOS is fitted with a USBL system, allowing precision positioning of the vehicle on the seafloor. Although ROPOS relayed data 4 times per second, only a single datum per second was used for the track file in the OFOP program because of software limitations. Using the Seafloor Observation Window in OFOP, we entered observations of coral colonies and litter manually into the dive protocol along with the time and corresponding coordinates at which the observation was made. For every individual coral colony encountered by ROPOS, an observation identifying the coral species (e.g., Primnoa, Paragorgia), and whether it was alive, damaged or dead was recorded. Observations were georeferenced to produce a distribution of corals along the dive transect in ArcGIS. Observations of lost fishing gear and other debris were also recorded. We measured the size of the corals in ImageJ, using video frame grabs with 2 laser points, 10 cm apart as scale, if the scaling lasers were either on the coral itself or nearby on approximately the same plane as the coral. This requirement resulted in many fewer colonies measured than being encountered. Height was the maximum dimension of the coral protruding outward from the base or the substrate the coral was attached to, and width the maximum dimension approximately parallel to the base or substrate. We calculated median size, 50 and 75% quantiles using R (R Core Team, 2017).
Ocean circulation at the shelf edge of the Gulf of Maine is influenced in part by regional circulation patterns in the Northwest Atlantic, with the most dominant oceanographic feature in the region being the Gulf Stream. The positions of the north wall of the Gulf Stream and the shelf break delineate the Slope Sea, where subsurface flow is composed of different water masses (Gatien, 1976). Warm Slope Water flows northeastward adjacent to the Stream from the surface to ∼ 400 m depth. The Labrador Slope Water flows equatorward deeper (>100 m) and closer to the shelf break, as a continuation of the continental slope component of the Labrador Current originating in the Labrador Sea. At depths > 400–500 m, Labrador Slope Water is typically more common in the northern part of the shelf, progressively shallowing toward the south where North Atlantic Central Water dominates. Given this general pattern, a strong front separating cold fresh water from warm salty water is present along the shelf. North-south shifts are common, but on average, the front is located offshore on the shelf break from the tail of the Grand Banks of Newfoundland to approximately central Georges Bank, from where it follows the 200-m isobath to Cape Hatteras (Gatien, 1976; Townsend et al., 2015; Peterson et al., 2017). Interannual variability of regional oceanographic features, i.e., the location of the shelf-slope front and north wall of the Gulf Stream, and in the strength of the Labrador Current/Slope Water, has been consistently linked to atmospheric conditions, e.g., the North Atlantic Oscillation (Taylor and Stephens, 1998; Chaudhuri et al., 2009; Peterson et al., 2017).
In the vicinity of Georges Bank, shelf-slope water exchange is driven by the influx of Slope water through the Northeast Channel, the relative composition of which varies between the two Slope water masses (Townsend et al., 2006). Shelf-slope exchange is also influenced by warm-core rings (anticyclonic eddies) spawning periodically from the meandering Gulf Stream and inducing entrainment of shelf water onto the slope as they reach the shelf break (Garfield and Evans, 1987). On Georges Bank proper, circulation is dominated by strong M2 tidal currents (semidiurnal) coupled with an anticyclonic residual circulation with summer intensification (Bigelow, 1927; Loder, 1980) and upwelling generally occurring around the edge of the bank (Townsend et al., 2006). Because Corsair Canyon is located in a region of significant seasonal and interannual variability in ocean circulation, we explored potential allochthonous sources of larvae to the canyon over different seasons and years to capture this variability.
We assessed hydrodynamic connectivity in Corsair Canyon offline, using advective velocity fields of the 3rd generation of the ocean model Finite-Volume Community Ocean Model held at the University of Massachusetts-Dartmouth (FVCOM-GOM3 30+ year hindcast; Chen et al., 2003). The model domain is built with an unstructured grid covering the Gulf of Maine, extending offshore of the shelf edge, and western Scotian Shelf. The model consists of 45 sigma layers and varying horizontal resolution between nodes, which increases close to shore and in areas of steep bathymetry. Hourly values of FVCOM from 1978 to 2015 are available online1.
We estimated displacement of water at each time step (10 min) with a backward (implicit) Euler method based on the 3-dimensional advective flow fields. Backward displacement in the x, y, and z dimensions was determined with (opposite) velocity in the u (eastward velocity), v (northward velocity), and w (vertical velocity) flow components, respectively, estimated at the previous time step at this location. We extracted values of velocity components at the given depth of the track, at each position using 2-dimensional spatial (linear) interpolation from the nearest three resolved points (elements for the u, v, and w components) the seabed depth of which exceeded the depth of the track. Fewer than three available points led to early termination of the tracking algorithm. We extracted flow components from the nearest sigma layer at the given depth, with no explicit vertical spatial interpolation. To enhance computing efficiency, variability in sea surface height (height above the geoid) was not considered, although available from the model. Therefore, constant seabed depth (height below the geoid), and consequently vertical depth distribution of sigma layers, was used at all nodes. On and offshore of Georges Bank, the range of sea surface height is ∼1 m and increases to 1–2 m inshore of Georges Bank. If tracks reached within 100 km of the model boundary (coast and offshore), the tracking algorithm was terminated. Tracks were derived in the R computing environment, modified from freely accessible code written in the Python environment2. We examined patterns of connectivity on 5 days per month (11th–15th) for each of 3 months per year (January, May, and September) over 10 years (2004–2013). Each track was assumed to have terminated at 12 pm (UTC) at 500 m depth just offshore from Corsair Canyon (see below) and was temporally extended backward for a maximum of 60 days. Because the planktonic larval duration is not known for P. arborea, we chose this period to reflect a median value for larval duration for bathyal/deep-sea (cnidarian) species, as currently proposed in the literature (Hilário et al., 2015; Young et al., 2018).
Because bathymetric resolution in the ocean model is coarse, the canyon itself is only partially resolved. For this reason, backward displacement was estimated from 15 locations (considered the endpoints of the track) offshore of Corsair Canyon (i.e., deeper than the 500-m depth contour; Figure 3 and Table 2). This resulted in 150 tracks per endpoint based on day-month-year combinations, for a total of 2250 tracks. These locations were selected based on their potential to source Corsair Canyon in ‘forward-tracking’ mode (preliminary data, not shown). Since the algorithm estimates displacement (i.e., based on advective fields), and tracks are estimated in areas of relatively steep bathymetry at the shelf break, the depth of the seabed at its updated position was often shallower than its estimated depth (based on the w displacement), i.e., water ‘hits’ the seabed. To alleviate this, the depth of the track was adjusted to 1 m above the seabed depth at its updated position.
Figure 3. (A) Nine zones of interest along the shelf edge between Northeast Channel (‘NEC’) and southwest Georges Bank. Geographic coordinates of approximate center locations of zones of interest are given in Table 3. Depth contours in meters. ‘EEZ,’ Exclusive Economic Zone. (B) Corsair and Georges Canyons zones of interest bounded by the 200-m and 1000-m depth contours. Endpoints of tracks (n = 15) are indicated. Geographic coordinates of endpoints are given in Table 2.
Table 2. Geographic coordinates of (A) endpoints of hydrodynamic tracks in the vicinity of Corsair Canyon and (B) approximate center points of each zone of interest.
We assessed patterns of connectivity in 9 zones (Figure 3 and Table 2): (1) 6 canyons to the south of Corsair Canyon along the edge of Georges Bank (in order of increasing distance from Corsair): Georges (∼40 km2), Heezen (∼65 km2), Nygren (∼43 km2), Lydonia (∼94 km2), Gilbert (∼105 km2), and Oceanographer (∼144 km2) Canyons (the latter 3 are part of the Northeast Canyons and Seamounts Marine National Monument established in 2016), bounded by the 200-m and 1000-m depth contours; (2) 2 contiguous zones at the mouth of Northeast Channel (NEC; northeast of Corsair Canyon), one in the shallow part of the channel proper (“NEC-Shallow”; ∼576 km2) delimited by a straight cross-channel boundary at the 240-m and the 300-m depth contours, and another (“NEC-Deep”; ∼438 km2) covering the 3 canyons present at the shelf edge seaward “NEC-Shallow” to the 1000-m depth contour; and (3) Corsair Canyon proper, between the 200-m and 500-m depth contours (∼65 km2). Delimiting depth contours were based on the Gulf of Maine Bathymetric Data (MassGIS Data) similar to the bathymetry used in the ocean model.
For all 2250 tracks terminating at the 15 endpoint locations in Corsair Canyon, we determined hydrodynamic connectivity based on occurrence (recorded as binary, 0 or 1) of tracks passing through each zone over the track’s full length. Individual tracks could be connected to multiple zones of interest based on spatial overlap between the track and each particular zone, depending on their temporal evolution; however, only connectivity with Corsair Canyon is described here. Tracks were pooled by month (n = 750 per month) to assess seasonal and interannual variability in hydrodynamic connectivity from zones of interest to Corsair. For each month, patterns in residence time (in hours) and transit time prior to reaching the mouth of Corsair Canyon (minimum, maximum, in days) were derived for each zone of interest. Beyond zones of interest, spatial patterns in tracks over the entire time period were determined using a raster grid with a horizontal cell size of 5 km covering the model domain. For each grid cell, the number of tracks passing through the cell was computed and expressed as a fraction of the total number of tracks (n = 750 for each month).
In Corsair Canyon, coarse sediment covered most of the seabed throughout the dive in the central region of the canyon (R1701). Ripples in the sediment were present in all except one transect, indicating oscillatory water motion, and ranged in size from 10 to 15 cm crest to crest. Low rock reliefs were often covered by consolidated sediment. Rock walls were encountered in all except one segment of the dive track. On the eastern margin of the canyon (R2009), the seafloor appeared relatively flat with very fine sediment and lacking visible megafauna on the southern deeper section of the dive, and more heterogeneous with some boulders without sediment cover, sometimes in clusters, along the northern shallower section, but without any rock wall outcrops. Some burrows were present throughout the dive, and one area also had distinct ripples in the sediment. During the deeper dive in the central region of the canyon (R1702), there were high concentrations of particulate organic matter in the water column. The seafloor was covered with soft sediment, and bioturbation (indicated by burrows and tracks) was evident. We encountered steep walls along the entire dive (20–60 m high), and pillars of bedrock in some of the transects. Many large rock outcrops were covered by thick layers of consolidated sediment. In Georges Canyon, there were only a few rock wall outcrops along both dive tracks, but boulders without sediment cover were scattered throughout the dives. In addition to the common relatively flat areas of fine sediment with burrows and tracks, there were a few steep sloping areas of either fine or consolidated sediment. In Fiddlers Cove, fine sediment covered the relatively smooth sloping walls for most of the dive track (R2012) with occasional boulders, rock walls, and outcrops of consolidated sediment. In some areas, many burrows and tracks were present, likely evidence of bioturbation.
Within Corsair Canyon, water temperature at the sea surface was 11.7°C (2017) to 14.3°C (2014) and decreased to 4.4°C at 1000 m (2017) and 3.5°C at 2000 m (2014). Oxygen concentration was 4.8–5.5 mL L-1 at the surface, decreased to a minimum of 3.5–3.7 mL L-1 at ∼ 200 m and then increased again to 6.1 mL L-1 at 2000 m. In Georges Canyon (2017), water temperature at the surface was 10.9°C and decreased to 4.1°C at 1100 m (R2010). Oxygen concentration was variable and unreliable at the surface but increased to 5.0–5.4 mL L-1 at depths of 600 and 1100 m, for each of R2010 and R2011, respectively. In Fiddlers Cove (2017), water temperature at the surface was 16.3°C and decreased to 4.4°C at 800 m. Oxygen concentration was 3.07 mL L-1 at the surface, decreased to a minimum of 0.05 mL L-1 at ∼240 m, and then increased to 5.1 mL L-1 at 800 m.
In Corsair Canyon, Paragorgia arborea was very abundant during dive R1701 (depths: 484–856 m) with a total of 228 live colonies recorded (Figure 4) and in three different color morphs (pink, white, and purple). We also recorded 97 live colonies of Primnoa resedaeformis at depths of 497–838 m. Colonies of both species were most often seen attached perpendicularly to the rock face, and into the current. Overall, coral colonies were more abundant in areas with rock walls, boulders and cobbles, in Transects 1, 2, and 6 (Figure 4A and Table 1). Some of these colonies were quite large for both species, and for P. arborea, in particular, 4 out of 36 colonies were > 2 m high (Figure 5 and Table 3). The two corals were less abundant in the eastern region of Corsair Canyon where we only recorded 7 colonies of P. arborea and 13 of P. resedaeformis over the entire dive (R2009) (Figure 4B). These colonies were mostly attached to large boulders, and four P. resedaeformis were recruits < 5 cm in height attached to a fishing trap. No P. arborea or P. resedaeformis were recorded in the deeper sections in the eastern and middle regions of the canyon (R1702: 788 – 2190 m, R2009: > 548 m).
Figure 4. Location of colonies of Paragorgia arborea and Primnoa resedaeformis along the dive tracks in Corsair Canyon in 2014 (R1701) (A) and 2017 (R2009) (B). Bathymetric data provided by the Canadian Hydrographic Service, Fisheries and Oceans - Canada.
Figure 5. Boxplots of height and width of measured colonies of Paragorgia arborea in Georges Canyon, Corsair Canyon and Fiddlers Cove. Boxes show 25 and 75% interquantile range, line shows median and whiskers are ± 1.5 IQR.
Table 3. Sizes of colonies of Primnoa resedaeformis measured on dives in Georges Canyon, Corsair Canyon and Fiddlers Cove in 2014 and 2017.
In Georges Canyon, we recorded few P. arborea and P. resedaeformis and most were found around a single location on dive R2010 (13 colonies of each species) with only 2 colonies of P. arborea recorded on R2011 (Figure 6 and Table 1). Similarly, we only recorded 9 P. arborea and 1 P. resedaeformis in Fiddlers Cove, at depths between 620 and 691 m (dive R2012) (Figure 7 and Table 1). The average size of the colonies at both sites was generally smaller than in Corsair Canyon, but still included some large colonies of P. arborea (Figure 5 and Table 3). At both sites and all dives, the corals were attached to larger boulders or rock wall outcrops and were erect in the water column.
Figure 6. Location of colonies of Paragorgia arborea and Primnoa resedaeformis along two dive tracks in Georges Canyon in 2017 (R2010 and R2011). Bathymetric data provided by the Canadian Hydrographic Service, Fisheries and Oceans - Canada.
Figure 7. Location of colonies of Paragorgia arborea and Primnoa resedaeformis along the dive track in Fiddlers Cove in 2017 (R2012). NRCan bathymetric data provided by Dr. Brian Kinlan (NOAA/NCCOS).
We only found damaged or dead colonies in Corsair and Georges Canyons. In Corsair Canyon, we recorded 1 damaged Paragorgia arborea (still attached to the substrate but only partially covered in living tissue) in dive R1701, and 5 colonies of P. arborea and 1 of Primnoa resedaeformis toppled and damaged (detached from their substrate but with some live polyps present). Eight dead colonies (consisting either of skeletons attached to their substrate with no live tissue or toppled skeletons with no live tissue present) of P. arborea were present in dive R1701, all of which were toppled. We also found one unrecognizable coral skeleton during dive R2009. In Georges Canyon, we found 10 coral skeletons which could not be identified to species.
Anthropogenic debris was present at all locations. The least amount was recorded in Corsair Canyon with 2 items in each of dives R1701 and R1702 (1 fishing line in each dive), and 4 pieces in R2009 (3 fishing lines and 1 lobster trap). At Georges Canyon, litter was abundant on both dives with 10 (3 fishing nets, 1 fishing line and 1 lobster trap) and 17 (1 fishing net and 10 fishing lines) items in R2010 (scattered throughout the dive track) and R2011 (concentrated in the northern section), respectively. At Fiddlers Cove, there were a total of 10 items of litter, clustered into a few locations near the middle section of the dive track, with 7 being fishing line.
Seasonal and inter-annual patterns of occurrence of predicted track origins that ended near the mouth of Corsair Canyon were most persistent from immediately adjacent areas: Corsair Canyon proper, Georges Canyon, and Heezen Canyon (Figure 8 and Table 4). Occurrence in these areas among all assessed months and years was observed in 34.6, 65.8, and 29.0% of all predicted tracks, respectively. Estimated connectivity from sites in the Northeast Channel (northeast of Corsair Canyon) occurred in 18.4 and 11.2% of total tracks for the deep and shallow areas, respectively. When pooled, the Northeast Channel could thus be a potentially important source with a probability of occurrence of ~30% within 60 days. Predicted connectivity from the deep area in Northeast Channel was clearly stronger in winter; for example 244 tracks occurred in January in 8 years, compared to 31 tracks in September in 6 years. Predicted connectivity from more distant canyons southwest of Corsair Canyon was more sporadic. Occurrence in these areas ranged from 5.3% of tracks with Lydonia Canyon to 9.6% of tracks with Nygren Canyon in 4–10 years. Despite its distance from Corsair Canyon, occurrence in Oceanographer Canyon was predicted to occur in 5.9% of all tracks. Predicted connectivity with these canyons was most evident in May and September, with tracks occurring in September in 7–8 years.
Figure 8. Hydrodynamic connectivity based on occurrence (out of 10 years) observed between endpoints at the mouth of Corsair Canyon and zones of interest in January, May, and September between 2004 and 2013.
Table 4. Number of tracks originating from each of the 9 zones of interest and ending at 15 endpoints offshore of Corsair Canyon (northwest Atlantic).
Mean residence time in each zone (calculated as the total time a track was located in that zone) was estimated at 16 h over all months and zones of interest (Figure 9). Short residence times were observed in areas southwest of Corsair Canyon, in Georges Canyon and Heezen Canyon, and particularly in Nygren Canyon (mean: 5.4, 7.9, and 9.0 h in May, January, and September, respectively). In contrast, mean residence times were longer in Lydonia Canyon, Gilbert Canyon and Oceanographer Canyon in September (19.1, 14.8, and 20.7 h respectively). Mean residence time was estimated to be consistently above the grand mean in January in the deep area of Northeast Channel (52.4 h), and in the shallow area (31.3 h). We attribute this pattern to the effect of tides in Northeast Channel. The longest residence times (> 200 h) were observed in January in the deep area of Northeast Channel and Oceanographer Canyon (single occurrence of 237 h).
Figure 9. Boxplots of residence time (h) for all tracks overlapping zones of interest segregated by month (sample sizes for the zone-month combinations are given in Table 4). Mean residence times for each month-zone combination are shown (red diamonds), as well as overall average (black dashed line).
Mean minimum transit time between the mouth of Corsair Canyon and zones of interest was estimated at 15.1 days prior to track end time across all years and months (Figure 10). As expected, areas nearer the mouth of Corsair Canyon (Corsair Canyon proper and Georges Canyon) showed short mean transit time, particularly in January for Corsair Canyon proper (1.8 days) and in May for Georges Canyon (0.9 days). Minimum transit time increased with increasing southwestward distance from Corsair Canyon at Heezen Canyon (3.7–8.0 days), Nygren Canyon (15.6–19.2), and Oceanographer Canyon (20.4–25.7). Estimated minimum transit times from Northeast Channel varied between 10.2 and 38.4 days and were generally faster for the deep than shallow area. Similar patterns were predicted for the maximum transit time from the zones of interest (data not shown). Maximum transit times reached ~30 days in Gilbert and Oceanographer Canyons and 41 days in the shallow area of Northeast Channel.
Figure 10. Boxplots of mean minimum transit time calculated from all tracks overlapping each zone of interest, segregated by month (sample sizes for the zone-month combinations are given in Table 4). Mean transit times for each month-zone combination (red diamonds) and overall average (black dashed line) are also shown.
The estimated position of tracks varied among months during the surveyed period (Figure 11). In January, most tracks straddled the upper continental slope at depths < 2000 m. To the southwest, the tracks extended at the farthest to Nygren Canyon, then diverting offshore into deeper waters. To the northeast, tracks were predicted to occur along the slope at the mouth of Northeast Channel, but rarely found northeastward of the Channel. In May, tracks originating from the northeast along the slope close to the shelf break appeared restricted by the 1000-m isobath. Estimated tracks reached further south than in January, most immediately offshore of Oceanographer Canyon, Gilbert Canyon and Lydonia Canyon, without penetrating into the canyons. Around the middle of Georges Bank, tracks extend further offshore down to the 3000-m isobath. In September, consistent with previous observations, no connectivity was predicted with areas northeast of Corsair Canyon. Most tracks originated from the southwest of Corsair Canyon, beyond Oceanographer Canyon. Most of these tracks, however, were confined to the 3000-m isobath, in the upper slope area extending immediately north of Corsair Canyon to Oceanographer Canyon. Overall, high interannual variability in spatial patterns was detected. The areas where > 50% of tracks were predicted to occur were restricted to the immediate adjacent region surrounding Corsair Canyon.
Figure 11. Fraction of the total number of tracks (0–1) of all tracks in the region surrounding the mouth of Corsair Canyon over the maximum temporal length of release (60 d) segregated by month: January, May and September (total of 750 tracks for each month). Densities were computed on a 5 km × 5 km grid. Fractions below 0.05 are not shown.
Overall, these patterns suggest that regional hydrodynamic connectivity from other zones of interest to Corsair Canyon is predicted to be strongest with nearby Georges Canyon and Heezen Canyon. Connectivity with canyons further to the southwest of Corsair Canyon is predicted to occur sporadically, and mostly in summer. At that time, currents flow predominantly northeastward, connecting Corsair Canyon to distant canyons close to the shelf edge, particularly Gilbert and Oceanographer Canyons in late summer (September). In contrast, predicted connectivity with the Northeast Channel (northeast of Corsair Canyon) is mostly observed in winter and spring, making it a potential source for Corsair at this time, with low potential for connectivity in summer. Tracks originating directly from the canyon proper were also observed at times, suggesting Corsair acts as a source, particularly in January.
The two deep-water corals Paragorgia arborea and Primnoa resedaeformis are widely distributed across the Atlantic continental margin of United States and Canada and into the Labrador Sea and spanning ocean basins. However, their distributions are fragmented, and corals are mostly found in canyons or regions of relative steep or heterogeneous topography that may include large boulders or vertical walls. This was the case in our study as well, where a single canyon, Corsair Canyon harbored dense aggregations of large colonies of P. arborea. Even within Corsair Canyon, these aggregations were found only at two locations with steep topography and large boulders. These locations, in addition to providing hard substrate for attachment, likely experience relatively higher current flow than the surrounding flat seafloor and thus higher delivery of food particles. The densities of these coral aggregations were three times to an order of magnitude greater than at equivalent depths in the NECCCA and some of the colonies were very large (i.e., old) with a maximum height of > 3 m (100 s of years old; Bennecke et al., 2016). Corsair Canyon also had the largest number of damaged colonies (mostly from fishing activity) and most litter of all 3 canyons, underscoring the vulnerability of these dense coral patches to human activity. Because of their high abundance and their vulnerability, the coral aggregations in Corsair Canyon were afforded protection through a DFO fisheries closure.
Although the populations are protected, their viability and ability to recover from previous damage by human activity is not necessarily ensured. For spatially fragmented populations, population persistence (or recovery from fishing) depends in part on recruitment and colonization. Recruitment to a population can be either through self-seeding, or through dispersal from an allochthonous source and adequate connectivity with the source population. For coral populations in Corsair Canyon, allochthonous supply, as predicted solely by hydrodynamic connectivity, that originated from Georges and Heezen Canyons to the southwest of Corsair Canyon, was the most consistent during all seasons and most years. Of these, only Georges Canyon is within Canada’s EEZ and based on our data has very sparse populations of corals that can supply recruits. The NECCCA, the only location with significant coral aggregations of P. arborea on the continental shelf break and slope within the Canadian EEZ, exhibited some predicted hydrodynamic connectivity with Corsair Canyon, particularly in winter and spring. Thus, NECCCA may be a source of recruits if winter and spring encompass the spawning season of P. arborea, which is currently unknown. However, based on our results in winter, downwelling and seaward offshore advection through Corsair Canyon may also be more frequent, arguably preventing flow into it and limiting its hydrodynamic connection with NECCCA. Our results suggest that Heezen Canyon, and to a lesser extent Nygren Canyon, which harbor high densities of P. arborea (Metaxas, personal observation), likely are the most consistent larval sources for the populations in Corsair Canyon. Predicted tracks ending in Corsair Canyon originate from regions in close proximity on the continental slope within 1–2 days, but more broadly over the shelf over periods of 30–35 days. These results suggest that the geographic range of potential larval sources will depend on larval duration, although likely restricted to the continental shelf and slope (and even the Gulf of Maine) because deep water corals are not found on the sedimentary habitats beyond the continental slope.
The predicted short transit times in reaching the end points in Corsair Canyon from the canyon proper may be indicative of low self-recruitment within the canyon. However self-recruitment may be higher in September, and less so in May, when longer transit times of up to 60 days were observed. Although these transit times may be indicative of currents bouncing in and out of the canyon, larvae trapped within those currents would be returned to the canyon on multiple occasions. Assuming a larval duration of < 60 days and passive dispersal (also currently unknown), these larvae would be positioned within the canyon at some point during their competency period. Both larval retention and larval return to their release points were demonstrated in simulations using ophiuroids in canyons in southwestern Australia (Kool et al., 2015). Corsair Canyon may act as a larval source to other locations along the slope; experiments using forward tracking can help elucidate this possibility, as well as explore the level of connectivity with NECCCA.
Population persistence of the coral aggregations in Corsair Canyon may require the preservation of coral aggregations in Heezen and Nygren Canyons, i.e., within the EEZ of USA, as the most consistently emerging larval sources. The Northeast Canyons and Seamounts Marine National Monument, established in the USA in 2016, protects some canyons further south that do serve as minor source populations (particularly Oceanographer Canyon in autumn), but the boundary of the Monument does not extend as far north as Heezen and Nygren Canyons. In turn, given that Corsair Canyon may serve as a significant larval source to the southeast, its protection augments the probability of larval supply to canyons in the US EEZ. The broad geographic range over which tracks were predicted to occur over periods of 60 days indicate potential connectivity (however, of unknown magnitude) across the entire continental slope in the region and possibly the Gulf of Maine. Our results strongly suggest that cross-boundary coordination is essential in the conservation of aggregations of deep-water corals in the northwest Atlantic, for ensuring larval exchange and connectivity. The importance in transboundary considerations and cooperation in spatial planning and conservation is being advocated particularly for the marine realm, where few physical barriers to the movement of individuals and populations exist (Arafeh-Dalmau et al., 2017; Aburto-Oropeza et al., 2018). For example, Arafeh-Dalmau et al. (2017) illustrated the feasibility of a transboundary networks of MPAs in the Ensenadian ecoregion, linking the more poorly sampled marine habitats of Baja California, Mexico with the well-established network of marine reserves in California, USA. It is recognized that transnational efforts in marine conservation planning entail decisions complicated by multiple stakeholders, conservation targets and political climates. Including costs of conservation can facilitate the process as illustrated by a study on the crowded Mediterranean Sea (Mazor et al., 2014). Approaches, such as developing access to homogeneous data, promoting transboundary collaborations, developing joint management units, and improving monitoring and surveillance, have been proposed to overcome some of the considerable challenges in cross-boundary conservation (Katsanevakis et al., 2015).
Studies on ecological connectivity of canyons have addressed linkages of individual canyons with the continental shelf (e.g., marine litter originating in coastal zones, Ramirez-Llodra et al., 2013), and as conduits to the deep ocean beyond continental margins (Huthnance, 1995; Canals et al., 2006). Here, we estimated connectivity between canyon systems, a key area where research is currently lacking (Fernandez-Arcaya et al., 2017). To fully describe hydrodynamic connectivity between canyons, regional ocean models that accurately capture bathymetric features on continental margins are needed. In southwestern Australia, connectivity among a series of canyons was strongly influenced by the dominant circulation in the region and was correlated to the distance of the canyon from shore and the shape of the canyon (Kool et al., 2015), both of which affect local hydrography. In our study, the coarse resolution of the bathymetry in the ocean model prevented us from assessing hydrodynamics in the canyon proper because its bathymetry is poorly resolved. It is thus important that high-resolution bathymetric datasets be made readily available through online portals, in particular those held by national hydrographic agencies (see Mayer et al., 2018), to facilitate more realistic ocean modeling efforts. Further, these efforts can assist in the identification of patterns of connectivity which particularly for the offshore, remote areas are presently difficult to quantify accurately.
While we focused on hydrodynamic connectivity, estimates of larval dispersal and ecological connectivity may differ. Biophysical models are one way to estimate larval dispersal, which encompasses larval transport (in turn determined by advection and larval behavior), timing and location of spawning, planktonic larval duration, and larval settlement (Pineda et al., 2007). However, most of these factors remain unknown for most shallow-water species and for nearly all deep-sea species (Metaxas and Saunders, 2009; Hilário et al., 2015). Here, we have attempted to incorporate elements of the location and timing of spawning and larval settlement (by including canyons with known populations of deep-water corals and three different seasons) and planktonic larval duration (see Materials and Methods). We did not incorporate larval behaviors, such as diel or ontogenetic vertical migrations or responses to features in the water column, such as density structure or food layers, because there is absolutely no relevant data for larvae of our focal species. Other studies, using hypothetical but not quantified behaviors, have suggested that the vertical position of larvae of deep-sea species in the water column affects dispersal distance (e.g., Young et al., 2012; McVeigh et al., 2017). However, studies from shallow water species that examined the contributions of different factors in larval dispersal have suggested that site of larval release explains a much greater proportion of the variance than larval vertical migration (Daigle et al., 2016). The relative importance of these factors in modifying our observed patterns of hydrodynamic connectivity remains unknown.
Area-based conservation of deep-water corals is gaining momentum globally. On the northwest Atlantic, in the EEZs of Canada and United States, corals are abundant and diverse in canyons along the continental shelf break. However, their distribution is fragmented and maintaining population connectivity is paramount for sustaining the aggregations in the canyons. The hydrodynamics are complex and seasonally and annually variable, further complicating the identification of sources and sinks of potential coral recruits. We have shown that larval sources may occur across national boundaries and political jurisdictions. Ensuring the long-term preservation of vulnerable marine ecosystems, such as those formed by deep-water corals, requires transboundary collaboration in data collection and cooperation in management actions.
AM conceptualized the study and designed and led the ROV dives that collected the video. ML developed and conducted the analysis on hydrodynamic connectivity. SdM conducted the analysis of the video data on coral distributions. All authors contributed to the writing of the manuscript.
Funding was provided by NSERC grants (RGPIN/222932-2011, RGPIN-2016-04878, RGPST/436808-2013, RGPST/501171-2017) to AM, DFO-Maritimes, Ocean Management Division, NOAA/NMFS Northeast Fisheries Science Center, NOAA Office of Marine and Aviation Operations, NOAA Deep-Sea Coral Research and Technology program and the Canadian Scientific Submersible Facility.
The authors declare that the research was conducted in the absence of any commercial or financial relationships that could be construed as a potential conflict of interest.
We would like to thank Martha Nizinski (Chief Scientist on both expeditions) for her continuous support of this Canada/US collaboration. Brian Kinlan and Matthew Poti (both with NOAA) provided mapping support (often on the fly), without which we would have been flying blind. The crews of the NOAA ship “Henry Bigelow” and the remotely operated vehicle ROPOS worked untiringly to ensure success. Drs. S. Dufour (MUN), P. Lawton (DFO-Maritimes, Science), and P. Snelgrove (MUN) have partnered in our research for more than a decade. We would also like to thank Arieanna Balbar and Swaantje Bennecke for assistance at sea, and Kelsey Desilets for video and data processing. We used hindcasts of the ocean model FVCOM GOM3 made available by Dr. Changsheng Chen and the Marine Ecosystem Dynamics Modeling Laboratory at the University of Massachussetts-Dartmouth (United States).
Aburto-Oropeza, O., Johnson, A. F., Agha, M., Allen, E. B., Allen, M. F., González, J. A., et al. (2018). Harnessing cross-border resources to confront climate change. Environ. Sci. Policy 7, 128–132. doi: 10.1016/j.envsci.2018.01.001
Arafeh-Dalmau, N., Torres-Moye, G., Seingier, G., Montaño-Moctezuma, G., and Micheli, F. (2017). Marine spatial planning in a transboundary context: linking Baja California with California’s network of marine protected areas. Front. Mar. Sci. 4:150. doi: 10.3389/fmars.2017.00150
Auster, P. J. (2005). “Are deep-water corals important habitats for fishes?” in Cold-Water Corals and Ecosystems, eds A. Freiwald and J. M. Roberts (Heidelberg: Springer-Verlag), 747–760. doi: 10.1007/3-540-27673-4_39
Bennecke, S., Kwasnischka, T., Metaxas, A., and Dullo, W.-C. (2016). In situ growth rate of deep-water octocorals determined from 3D photogrammetric reconstructions. Coral Reefs 35, 1227–1239. doi: 10.1007/s00338-016-1471-7
Bennecke, S., and Metaxas, A. (2017). Effectiveness of a deep-water coral conservation area: evaluation of its boundaries and changes in octocoral communities over 13 years. Deep Sea Res. Part II Top. Stud. Oceanogr. 137, 420–435. doi: 10.1016/j.dsr2.2016.06.005
Bryan, T., and Metaxas, A. (2006). Distributional patterns of deep-water corals along the North American continental margins: relationships with environmental factors. Deep Sea Res. Part I Top. Stud. Oceanogr. 53, 1865–1879. doi: 10.1016/j.dsr.2006.09.006
Bryan, T., and Metaxas, A. (2007). Predicting suitable habitat for Paragorgiidae and Primnoidae on the Atlantic and Pacific continental margins of North America. Mar. Ecol. Prog. Ser. 330, 113–126. doi: 10.3354/meps330113
Buhl-Mortensen, L., Vanreusel, A., Gooday, A. J., Levin, L. A., Priede, I. G., Buhl-Mortensen, P., et al. (2010). Biological structures as a source of habitat heterogeneity and biodiversity on the deep ocean margins. Mar. Ecol. 31, 21–50. doi: 10.1111/j.1439-0485.2010.00359.x
Canals, M., Puig, P., de Madron, X. D., Heussner, S., Palanques, A., and Fabres, J. (2006). Flushing submarine canyons. Nature 444:354. doi: 10.1038/nature05271
Chaudhuri, A. H., Bisagni, J. J., and Gangopadhyay, A. (2009). Shelf water entrainment by gulf stream warm-core rings between 75°W and 50°W during 1978-1999. Cont. Shelf Res. 29, 393–406. doi: 10.1016/j.csr.2008.10.001
Chen, C., Liu, H., and Beardsley, R. C. (2003). An unstructured grid, finite-volume, three-dimensional, primitive equations ocean model: application to coastal ocean and estuaries. J. Atmos. Ocean. Technol. 20, 159–186. doi: 10.1175/1520-0426(2003)020<0159:AUGFVT>2.0.CO;2
Clark, M. R., Althaus, F., Schlacher, T. A., Williams, A., Bowden, D. A., and Rowden, A. A. (2015). The impacts of deep-sea fisheries on benthic communities: a review. ICES J. Mar. Sci. 73, i51–i69. doi: 10.1093/icesjms/fsv123
Convention on Biological Diversity (2007). Report of the Expert Workshop on Ecological Criteria and Biogeographic Classification Systems for Marine Areas in need of Protection. UNEP/CBD/EWS.MPA/1/2. Montreal, Canada: Secretariat of the Convention on Biological Diversity.
Crowder, L. B., and Figueira, W. F. (2006). “Metapopulation ecology marine conservation,” in Marine Metapopulations, eds J. P. Kritzer and P. F. Sale (Burlington, MA: Academic Press), 491–516.
Daigle, R. M., Metaxas, A., and Chassé, J. (2016). The relative importance of behavior in larval dispersal in a low energy embayment. Prog. Ocean. 144, 93–117. doi: 10.1016/j.pocean.2016.04.001
DFO [Fisheries and Oceans Canada] (2017). Coral and Sponge Conservation Measures in the Maritimes. Available at: http://www.dfo-mpo.gc.ca/oceans/ceccsr-cerceef/measures-mesures-eng.html
Du Preez, C., and Tunnicliffe, V. (2011). Shortspine thornyhead and rockfish (Scorpaenidae) distribution in response to substratum, biogenic structures and trawling. Mar. Ecol. Prog. Ser. 425, 217–231. doi: 10.3354/meps09005
FAO [Food and Agriculture Organization of the United Nations] (2009). International Guidelines for the Management of Deep-sea Fisheries in the High Seas. Rome: Food and Agriculture Organization of the United Nations.
Fernandez-Arcaya, U., Ramirez-Llodra, E., Aguzzi, J., Allcock, A. L., Davies, J. S., Dissanayake, A., et al. (2017). Ecological role of submarine canyons and need for canyon conservation: a review. Front. Mar. Sci. 4:5. doi: 10.3389/fmars.2017.00005
Fox, A., Henry, L.-A., Corne, D. W., and Roberts, J. M. (2017). Sensitivity of marine protected area connectivity to atmospheric variability. R. Soc. Open Sci. 3:160494. doi: 10.1098/rsos.160494
Garfield, N. III, and Evans, D. L. (1987). Shelf water entrainment by gulf stream warm-core rings. J. Geophys. Res. 92, 13003–13012. doi: 10.1029/JC092iC12p13003
Gatien, M. G. (1976). A study in the slope water region south of halifax. J. Fish. Res. Board Canada 33, 2213–2217. doi: 10.1139/f76-270
Gerber, L. R., Botsford, L. W., Hastings, A., Possingham, H. P., Gaines, S. D., Palumbi, S. R., et al. (2003). Population models for marine reserve design: a retrospective and prospective synthesis. Ecol. Appl. 13, S47–S64. doi: 10.1890/1051-0761(2003)013[0047:PMFMRD]2.0.CO;2
Herrera, S., Shank, T. M., and Sánchez, J. M. (2012). Spatial and temporal patterns of genetic variation in the widespread antitropical deep-sea coral Paragorgia arborea. Mol. Ecol. 21, 6053–6067. doi: 10.1111/mec.12074
Hilário, A., Metaxas, A., Gaudron, S. M., Howell, K. L., Mercier, A., Mestre, N., et al. (2015). Estimating dispersal distance in the deep sea: challenges and applications to marine reserves. Front. Mar. Sci. 2:6. doi: 10.3389/fmars.2015.00006
Huetten, E., and Greinert, J. (2008). Software controlled guidance, recording and post-processing of seafloor observations by ROV and other towed devices: the software package OFOP. Geophys. Res. Abstr. 10:EGU2008–A–03088.
Huthnance, J. M. (1995). Circulation, exchange and water masses at the ocean margin: the role of physical processes at the shelf edge. Prog. Oceanogr. 35, 353–431. doi: 10.1016/0079-6611(95)80003-C
ICES [International Council for the Exploration of the Sea] (2016). Report of the Workshop on Vulnerable Marine Ecosystem Database (WKVME). Peterborough: ICES.
Katsanevakis, S., Levin, N., Coll, M., Giakoumi, S., Shkedi, D., Mackelworth, P., et al. (2015). Marine conservation challenges in an era of economic crisis and geopolitical instability: the case of the Mediterranean Sea. Mar. Policy 51, 31–39. doi: 10.1016/j.marpol.2014.07.013
Kenchington, E., Wang, Z., Lirette, C., Murillo, J. F., Guijarro, J., Yashayaev, I., et al. (2019). Connectivity modelling of areas closed to protect vulnerable marine ecosystems in the northwest Atlantic. Deep Sea Res. Part II Top. Stud. Oceanogr. 143, 85–103. doi: 10.1016/j.dsr.2018.11.007
Kool, J. T., Huang, Z., and Nichol, S. L. (2015). Simulated larval connectivity among Australia’s southwest submarine canyons. Mar. Ecol. Prog. Ser. 539, 77–91. doi: 10.3354/meps11477
Kool, J. T., Moilanen, A., and Treml, E. A. (2013). Population connectivity: recent advances and new perspectives. Landsc. Ecol. 28, 165–185. doi: 10.1007/s10980-012-9819-z
Lacharité, M., and Metaxas, A. (2013). Early life history of deep-water gorgonian corals may limit their abundance. PLoS One 8:e65394. doi: 10.1371/journal.pone.0065394
Loder, J. W. (1980). Topographic rectification of tidal currents on the sides of Georges Bank. J. Phys. Oceanogr. 10, 1399–1416. doi: 10.1175/1520-0485(1980)010<1399:TROTCO>2.0.CO;2
Mayer, L., Jakobsson, M., Allen, G., Dorschel, B., Falconer, R., Ferrini, V., et al. (2018). The nippon foundation – GEBCO Seabed 2030 Project: the quest to see the world’s oceans completely mapped by 2030. Geoscience 8:63. doi: 10.3390/geosciences8020063
Mazor, T., Giakoumi, S., Kirk, S., and Possingham, H. (2014). Large-scale conservation planning in a multinational marine environment: cost matters. Ecol. Appl. 24, 1115–1130. doi: 10.1890/13-1249.1
McVeigh, D. M., Eggleston, D. B., Todd, A. C., Young, M. C., and He, R. (2017). The influence of larval migration and dispersal depth on potential larval trajectories of a deep-sea bivalve. Deep Sea Res. Part I Top. Stud. Oceanogr. 127, 57–64. doi: 10.1016/j.dsr.2017.08.002
Mercier, A., and Hamel, J. F. (2011). Contrasting reproductive strategies in three deep-sea octocorals from eastern Canada: Primnoa resedaeformis, Keratoisis ornata, and Anthomastus grandiflorus. Coral Reefs 30, 337–350. doi: 10.1007/s00338-011-0724-8
Metaxas, A., and Saunders, M. (2009). Quantifying the “bio-” components in biophysical models of larval transport: advances and pitfalls. Biol. Bull. 216, 257–272. doi: 10.1086/BBLv216n3p257
Mortensen, P. B., and Buhl-Mortensen, L. (2004). Distribution of deep-water gorgonian corals in relation to benthic habitat features in the Northeast Channel (Atlantic Canada). Mar. Biol. 144, 1223–1238. doi: 10.1007/s00227-003-1280-8
Peterson, I., Greenan, B., Gilbert, D., and Hebert, D. (2017). Variability and wind forcing of ocean temperature and thermal fronts in the Slope Water region of the Northwest Atlantic. J. Geophys. Res. Oceans 122, 7325–7343. doi: 10.1002/2017JC012788
Pineda, J., Hare, J. A., and Sponaugle, S. (2007). Larval transport and dispersal in the coastal ocean and consequences for population connectivity. Oceanography 20, 22–39. doi: 10.5670/oceanog.2007.27
Quattrini, A. M., Nizinski, M. S., Chaytor, J. D., Demopoulos, A. W. J., Roark, E. B., France, S. C., et al. (2015). Exploration of the canyon-incised continental margin of the northeastern United States reveals dynamic habitats and diverse communities. PLoS One 10:e0139904. doi: 10.1371/journal.pone.0139904
R Core Team. (2017). R: A Language and Environment for Statistical Computing. Vienna: R Foundation for Statistical Computing.
Ramirez-Llodra, E., De Mol, B., Company, J. B., Coll, M., and Sardà, F. (2013). Effects of natural and anthropogenic processes in the distribution of marine litter in the deep Mediterranean Sea. Prog. Oceanogr. 118, 273–287. doi: 10.1016/j.pocean.2013.07.027
Roberts, J. M., Wheeler, A. J., and Freiwald, A. (2006). Reefs of the deep: the biology and geology of cold-water coral ecosystems. Science 312, 543–547. doi: 10.1126/science.1119861
Sale, P. F., Hanski, I., and Kritzer, J. P. (2006). “The merging of metapopulation theory and marine ecology: establishing the historical context,” in Marine Metapopulations, eds J. P. Kritzer and P. F. Sale (Burlington, MA: Elsevier Academic Press), 3–30. doi: 10.1016/B978-012088781-1/50004-2
Strychar, K. B., Kenchington, E. L., Hamilton, L. C., and Scott, D. B. (2008). Phylogenetic diversity of the cold water octocoral Paragorgia arborea (Linnaeus, 1758) off the east coast of Canada. Int. J. Biol. 3, 3–22.
Taylor, A. H., and Stephens, J. A. (1998). The north atlantic oscillation and the latitude of the gulf stream. Tellus A 50, 134–142. doi: 10.1034/j.1600-0870.1998.00010.x
Townsend, D. W., Pettigrew, N. R., Thomas, M. A., Neary, M. G., McGillicuddy, D. J. Jr., and O’Donnell, J. (2015). Water masses and nutrient sources to the Gulf of Maine. J. Mar. Res. 73, 93–122. doi: 10.1357/002224015815848811
Townsend, D. W., Thomas, A. C., Mayer, L. M., Thomas, M. A., and Quinlan, J. A. (2006). “Oceanography of the northwest Atlantic continental shelf,” in The Sea The Global Coastal Ocean, Vol. 14A, eds A. R. Robinson and K. Brink (Cambridge, MA: Harvard University Press), 119–168.
Waller, R., Stone, R. P., Johnstone, J., and Mondragon, J. (2014). Sexual reproduction and seasonality of the Alaskan red tree coral, Primnoa pacifica. PLoS One 9:e90893. doi: 10.1371/journal.pone.0090893
Watanabe, S., Metaxas, A., Sameoto, J. A., and Lawton, P. (2009). Patterns in abundance and size of two deep-water gorgonian octocorals, in relation to depth and substrate features off Nova Scotia. Deep Sea Res. Part I Top. Stud. Oceanogr. 56, 2235–2248. doi: 10.1016/j.dsr.2009.09.003
White, J. W., Botsford, L. W., Hastings, A., and Largier, J. L. (2010). Population persistence in marine reserve networks: incorporating spatial heterogeneities in larval dispersal. Mar. Ecol. Prog. Ser. 398, 49–67. doi: 10.3354/meps08327
Young, C. M., Arellano, S. M., Hamel, J. F., and Mercier, A. (2018). “Ecology and evolution of larval dispersal in the deep sea,” in Evolutionary Ecology of Marine Invertebrate Larvae, eds T. J. Carrier, A. M. Reitzel, and A. Heyland (England: Oxford University Press), doi: 10.1093/oso/9780198786962.003.0016
Keywords: deep-water corals, connectivity, area-based conservation, cross-boundary collaboration, submarine canyons, hydrodynamics, continental slope, fishery closures
Citation: Metaxas A, Lacharité M and de Mendonça SN (2019) Hydrodynamic Connectivity of Habitats of Deep-Water Corals in Corsair Canyon, Northwest Atlantic: A Case for Cross-Boundary Conservation. Front. Mar. Sci. 6:159. doi: 10.3389/fmars.2019.00159
Received: 06 September 2018; Accepted: 12 March 2019;
Published: 29 March 2019.
Edited by:
Murray Roberts, The University of Edinburgh, United KingdomReviewed by:
Alan David Fox, The University of Edinburgh, United KingdomCopyright © 2019 Metaxas, Lacharité and de Mendonça. This is an open-access article distributed under the terms of the Creative Commons Attribution License (CC BY). The use, distribution or reproduction in other forums is permitted, provided the original author(s) and the copyright owner(s) are credited and that the original publication in this journal is cited, in accordance with accepted academic practice. No use, distribution or reproduction is permitted which does not comply with these terms.
*Correspondence: Anna Metaxas, bWV0YXhhc0BkYWwuY2E=
Disclaimer: All claims expressed in this article are solely those of the authors and do not necessarily represent those of their affiliated organizations, or those of the publisher, the editors and the reviewers. Any product that may be evaluated in this article or claim that may be made by its manufacturer is not guaranteed or endorsed by the publisher.
Research integrity at Frontiers
Learn more about the work of our research integrity team to safeguard the quality of each article we publish.