- 1Marine and Freshwater Research Institute, Reykjavik, Iceland
- 2Institute of Earth Sciences, University of Iceland, Reykjavik, Iceland
- 3Department of Oceanography, Dalhousie University, Halifax, NS, Canada
- 4Icelandic Institute of Natural History, Garðabær, Iceland
The need to understand species distribution- and biodiversity patterns in high-latitude marine regions is immediate as these marine environments are undergoing rapid environmental changes, including ocean warming and ocean acidification. By the year 2100, the seas north of the Greenland-Iceland-Faroe (GIF) topographic ridge are predicted to become largely corrosive to aragonite, a form of calcium carbonate commonly formed by calcifying molluscs. We examine depth-diversity relationships in bivalves and gastropods north and south of the GIF ridge, between 200 and 2000 m depth. We also identify bivalve and gastropod species that could be monitored to identify early signs of changes in benthic communities north of the GIF ridge, due to ocean acidification. Patterns of α-diversity were estimated through rarefaction, as E(S20). Regional and depth related β-diversity was analyzed and the additive contribution of species replacement (turnover) and species loss/gain (nestedness) to β-diversity calculated. Despite sharing a significant number of species, diversity patterns differed between the study regions. The diversity patterns also differed between bivalves and gastropods. North of the GIF ridge, the relationship between α-diversity and depth was unimodal with a predominant decrease in bivalve and gastropod α-diversity between 300 and 2000 m depth. Species assemblages in the deep bathyal zone were partly nested subsets of the assemblages in the shallow bathyal zone. South of the GIF ridge, patterns in α-diversity were more ambiguous. Alpha diversity decreased between 300 and 2000 m depth in bivalves, with no clear trend observed in gastropods. This finding contradicts the recognized increase in α-diversity in the bathyal zone in the North Atlantic basin, perhaps due to the oceanographic conditions directly south of the GIF ridge. In contrast to that observed north of the GIF ridge, nestedness did not contribute significantly to β-diversity south of the GIF ridge. This comparative study sheds new light on deep-sea diversity patterns of molluscs in the high-latitude North Atlantic and provides baseline data on species occurrences. This information can inform future assessment of the impact of environmental changes in these regions and management efforts.
Introduction
The benthic realm harbors a remarkable proportion of the ocean’s biodiversity (Snelgrove, 1999). Marine invertebrates that reside on (epifaunal) or in (infaunal) benthic sediments make up a large proportion of this diversity and provide key ecosystem services, for example through provision of structural habitat and through bioturbation (Snelgrove, 1999; Queirós et al., 2013). The vast majority (91%) of marine benthic habitat lies below 200 m depth where darkness prevails and autotrophic activity is negligible (Kennish, 2000). Accessing the deep ocean is a very costly and logistically challenging endeavor, explaining why ecosystems in the deep sea remain insufficiently studied compared with shallow water or terrestrial systems (May and Godfrey, 1994; Higgs and Attrill, 2015). It is more urgent than ever to document and understand biodiversity patterns and ecological processes in deep-sea benthic ecosystems, as these systems, typified by environmental stability (Seibel and Walsh, 2003; Hofmann et al., 2011), limited food availability (Lutz et al., 2007) and low temperatures which limit rate processes (Childress, 1995), may be particularly sensitive to natural and human-induced environmental changes that are occurring at unprecedented rates (Crain et al., 2008; Gehlen et al., 2014; Rogers, 2015).
Since the early work of Hessler and Sanders (1967), much has been learned about general patterns of benthic species biodiversity in the deep ocean and how it changes along bathymetric gradients (Rex and Etter, 2010). Alpha (α) diversity describes local diversity, for example within a single sample or site. Regional and depth related changes in α-diversity were the focus of the majority of early studies so that spatial trends are now considered reasonably well understood for several parts of the North Atlantic (Rex and Etter, 2010). In general, the α-diversity of benthic macrofauna in the North Atlantic exhibit a unimodal diversity pattern: increasing toward the lower bathyal or upper abyssal depths and decreasing toward the deeper abyss (Rex, 1973; Andrew and Scott, 2000). Patterns of benthic diversity north of the Greenland-Iceland-Faroe (GIF) ridge, in the Nordic Seas and Arctic Ocean, are notably different from those generally described for the North Atlantic. In high-latitude regions the relationship between α-diversity and depth is unimodal as generally observed south of the GIF ridge but α-diversity peaks at a much shallower depth (higher bathyal or shallower) with remarkably low diversity at lower bathyal and abyssal depths (Svavarsson, 1997; Bett, 2001). However, the diversity on the shelfs is not considered particularly low (Piepenburg et al., 2011).
Beta (β) diversity describes changes in community composition within a region or along a gradient (Whittaker, 1960, 1972). Increased sampling effort and data availability has facilitated research on β-diversity in the ocean, with results interpreted almost exclusively as spatial replacement of species along depth or horizontal gradients (McClain and Rex, 2015). However, β-diversity can also be affected by species loss (or gain) resulting in smaller communities forming ordered subsets of the species composition of larger communities, a pattern also referred to as “nestedness” (Ulrich and Gotelli, 2007; Almeida-Neto et al., 2008). The specific ecological significance of either species replacement or nestedness has driven multiple efforts intended to mathematically quantify the contribution of these components to β-diversity (McClain and Rex, 2015). In recent years, studies of deep-sea diversity have used a method presented by Baselga (2010, 2012) to partition β-diversity into two additive components: nestedness resultant dissimilarity and dissimilarity resulting from species turnover. This partitioning has allowed for new insight into deep-sea diversity patterns (Wagstaff et al., 2014; Stuart et al., 2017). For example, Brault et al. (2013b) concluded that abyssal neogastropoda assemblies are likely supported through source-sink dynamics, partly based on a significant increase in nestedness with depth along with a decrease in α-diversity. Although not a commonly used method in research of deep-sea diversity, due to the lack of comparable sampling strategies, a regional comparison approach for α- and β-diversity patterns can also help identify the processes that drive deep-sea diversity patterns (e.g., Brault et al., 2013a).
The Mollusca is a species rich and diverse phylum whose species are among the more conspicuous and biodiverse invertebrate macrofauna in the marine environment (Alongi, 1990; Linse et al., 2006). Within the Mollusca phylum, gastropods and bivalves are the most species rich classes (Horton et al., 2019). Species belonging to both groups can play crucial roles as keystone species (Paine, 1969) or as ecosystem engineers, as a result of their feeding mechanisms or calcification traits (e.g., Hall-Spencer and Moore, 2000; Gutiérrez et al., 2003). Calcifying molluscs are considered particularly susceptible to ocean acidification and the concurrent decrease in the saturation state for calcium carbonate (Ω) (Gazeau et al., 2013; Gattuso et al., 2015). As a result of increased pressure, Ω decreases with depth (Millero, 2007), but disentangling the relative ecological importance of Ω and other environmental parameters that relate to depth, such as food availability and sediment characteristics, is challenging. Collecting information on species occurrences and biodiversity of calcifying molluscs is an important first step toward understanding the implication of changes in Ω, temperature and other large scale environmental changes in deep benthic habitats (Urban et al., 2016). The need for baseline data is especially urgent in the Nordic Seas, due to the naturally low Ω of seawater and the fact that Ω is decreasing at an alarming rate in both surface and deep waters (Olafsson et al., 2009;Skogen et al., 2014).
The GIF ridge acts as a topographic barrier that separates two ocean basins: the Nordic Sea basin to the north and the North Atlantic basin to the south. While the maximum sill depth of the Greenland-Iceland ridge and the Iceland-Faroe Island ridge is 620 and 480 m respectively, the seafloor reaches depths exceeding 3000 m south and north of the GIF ridge (Jakobsson, 2002). The biogeographic boundaries at the GIF ridge coincide with a transition between colder and warmer water masses, with Iceland coinciding with the Arctic Front (Hansen and Meincke, 1979). Thus, the island is considered to occupy a key position in the North Atlantic in terms of biogeographical species ranges (Briggs, 1970; Dahlgren et al., 2000; Jöst et al., 2018). Comparisons of biodiversity has previously been conducted between benthic habitats directly north and south of the GIF ridge for hyper-benthic amphipods (Weisshappel and Svavarsson, 1998; Weisshappel, 2000), benthic isopods (Svavarsson et al., 1993; Svavarsson, 1997; Brix et al., 2018; Schnurr et al., 2018), ostracods (Jöst et al., 2018), and foraminifera (Gudmundsson, 1998). However, a comparison of mollusc species diversity patterns between the regions has not been conducted to date.
The main objective of this study was to increase understanding of biodiversity patterns in the high-latitude North Atlantic by investigating α- and β-diversity patterns in molluscs along the bathymetric gradient north and south of the GIF ridge. A secondary objective of this study was to identify bivalve and gastropod species which could be used as indicator species to monitor ecological integrity north of the GIF ridge (Carignan and Villard, 2002). Future monitoring efforts should preferably include a variety of taxa but as bivalves and gastropods are known to be sensitive to Ω, they could provide early warning of natural responses in areas undergoing rapid ocean acidification, such as is observed north of the GIF ridge (Gattuso et al., 2015). To achieve these goals, we use data on bivalves and gastropods collected during the sampling program BIOICE (Benthic Invertebrates of Icelandic waters).
Materials and Methods
Study Regions
The study regions encompass the Icelandic shelf and slope within the Iceland Sea to the north of the GIF ridge and the Icelandic shelf and slope to the south of the GIF ridge (Figure 1). These regions share topographic similarities as they both include the Icelandic shelf and slope and parts of the Mid-Atlantic Ridge but the broad separation by the GIF ridge results in regional hydrographic differences (Stefánsson, 1962). The Iceland Sea is a part of the Nordic Seas along with the Norwegian Sea and Greenland Sea (Jakobsson, 2002). The hydrographic properties of the Iceland Sea are generally described as Arctic Intermediate Water overlying Arctic Deep Water (Stefánsson, 1962; Swift et al., 1980). These waters are characterized by sub-zero temperatures throughout the water column in winter and a shallow (∼200 m) mixed layer in the summer, in which temperatures exceed 0°C (Olafsson, 2003; Olafsson et al., 2009). The oceanographic environment south of the ridge reflects a dynamic interaction between water masses with different physical properties, with temperatures ranging from 2 to >10°C (Hansen and Østerhus, 2000; Malmberg and Valdimarsson, 2003). Ocean warming and ocean acidification have been observed at the surface in both the Iceland and the Irminger Sea (southwest of Iceland) with warming and acidification observed down to at least 1800 m in the Iceland Sea over the last two decades (Olafsson et al., 2009, 2010).
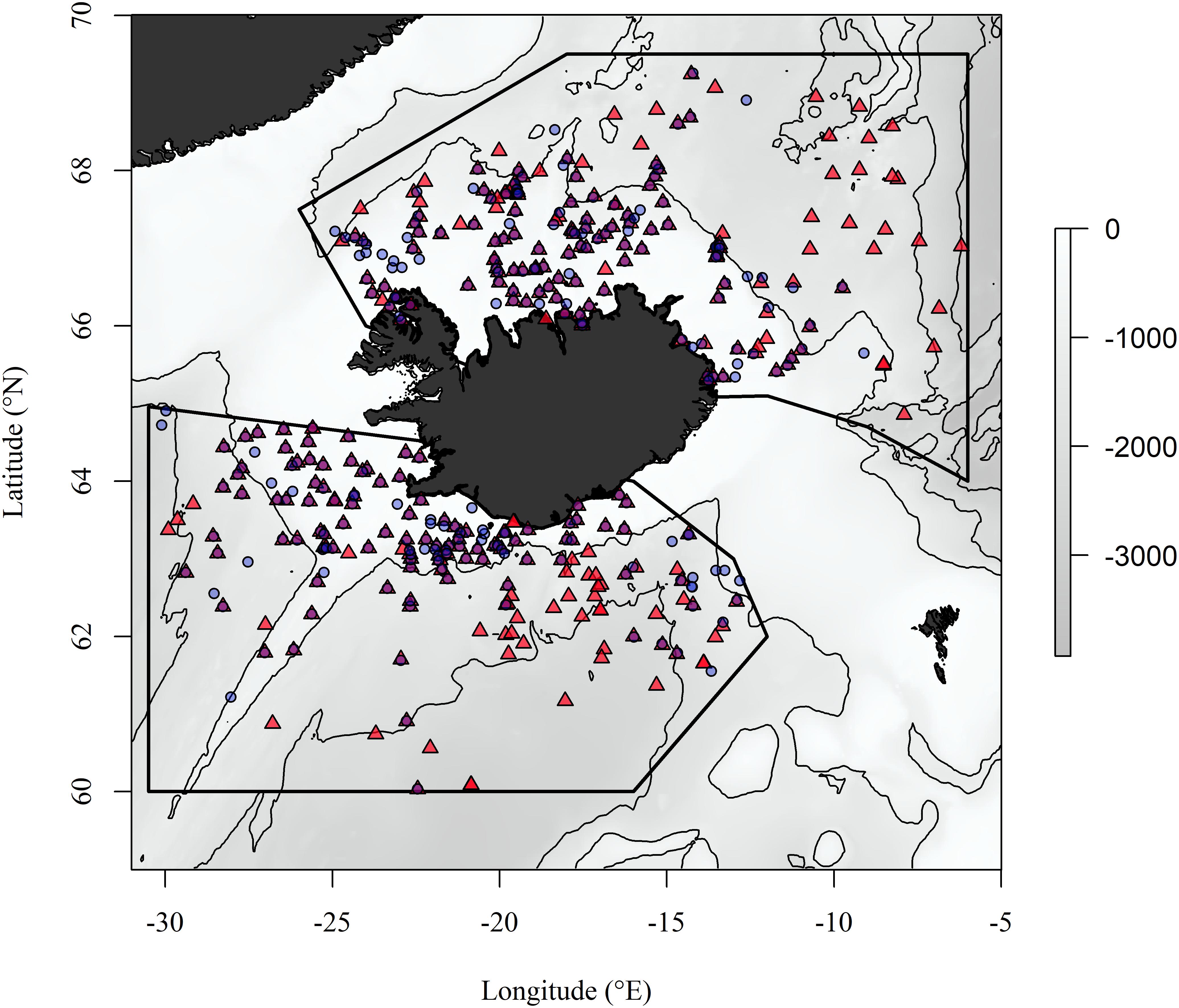
Figure 1. The study regions north and south of the Greenland-Iceland-Faroe ridge and geographic location of BIOICE samples included in the study. Red triangles and blue circles are localities of benthic samples collected through towing a modified RP sled and Sneli sled, respectively. Contour lines are placed at 1000 m depth intervals.
Biological Data
Benthic samples were collected between 20 and 3020 m depth during the years 1991–2004 of the BIOICE program (Gudmundsson, 1998). Four types of towed sampling gears were used in the program, depending on bottom type, but this study only includes data collected using a modified RP sled (Rothlisberg and Pearcy, 1977; Brattegard and Fosså, 1991) and a Sneli sled (Sneli, 1998) since these gears were most frequently deployed and sampled similar species assemblages (Supplementary Material 1). Species records and their taxonomic classification were manually examined and edited where necessary to correspond to entries in the database “World Register of Marine Species” and synonyms standardized to a single name. Information on the sample data considered for the study, a list of all species recorded, number of samples they were recorded in and their observed depth range in each study region is provided (Supplementary Material 2).
Analytical Approach
Sample data collected using a modified RP sled and Sneli sled were pooled for analysis of biodiversity as the sampled species assemblages were similar between the gear types. The total number of samples collected within the study regions was 366 and 383, north and south of the GIF ridge, respectively. Samples collected using a RP sled and Sneli sled contributed near equally to the total number of samples in both regions.
The number of unobserved species, in addition to the observed species richness, was estimated based on the singletons and doubletons in species matrices (Chao, 1987) along with a standard error (Chiu et al., 2014). Estimations were carried out for each region and separately for four 500 m depth ranges, between surface and 2000 m depth, below which sample data were considered insufficient for estimating species richness.
Alpha diversity was estimated using the Sanders-Hurlbert expected number of species (Sanders, 1968; Hurlbert, 1971) in a sample normalized to 20 individuals [E(S20)], excluding from the analysis samples where fewer than 20 individuals of either gastropods or bivalves were collected. Accordingly, the number of samples available for the analysis was reduced to a total of 252 and 250 for bivalves north and south of the GIF ridge respectively, and a total of 146 and 197 samples for gastropods in the same regions respectively. To investigate how E(S20) varied with depth in each region, samples were averaged into 100 m bins and a linear least squares regression used to identify trends in E(S20) as a function of depth within the bathyal zone, or between 300 to 2000 m.
To explore the relationship of depth and region with species assemblages of bivalves and gastropods, a 2-dimensional NMDS analysis on presences-absence (binary) species matrices was conducted, excluding species occurring in less than 5% of samples in each region. The NMDS analysis was based on Sørensen (Bray-Curtis) sample dissimilarity (Sørensen, 1948). The contribution of depth and region to MDS1 (primary NMDS axis) and MDS2 (secondary NMDS axis) were analyzed using a least squares linear regression and a Student t-test respectively.
Optimal methods for analysis of β-diversity along environmental gradients require data collected using standardized sampling methods but like the majority of research on deep-sea diversity patterns of macro- and/or meiofauna (Rex and Etter, 2010), this study relies on methods that are not standardized. The sampling sleds within the BIOICE were typically towed for 20 min but the average tow time was 19 ± 6 min (Supplementary Material 2) and the bottom substrate towed was unknown. We recognize the issues associated with the lack of standardized sampling and issues resulting from the non-random and unsystematic spatial distribution of sample localities in the BIOICE program, especially the limited sampling effort at depths below 1500 m. To address these issues, sample data were aggregated into 100 m depth bins with bins containing fewer than 100 and 50 individual bivalves and gastropods respectively being discarded from the β-diversity analysis. Data were converted to presence-absence (binary) species matrices. The analysis was restricted to an upper depth limit of 300 m to exclude the shallow water communities on the Iceland shelf in the analysis of β-diversity. As a result, the analysis was applied to the depth range of 300 to 1600 m, with 14 depth bins for bivalves but 13 depth bins for gastropods, due to the exclusion of the 1200 m depth bin. To investigate β-diversity dynamics, including the contribution of nestedness to β-diversity, we use two independent methods.
Baselga (2010, 2012) described how β-diversity could be mathematically separated into two components: (A) β-diversity resulting from species replacement between sites which is commonly referred to as species turnover, and (B) the part of dissimilarity that can be attributed to the effect of species loss (or gain) or species nestedness. The calculations of β-diversity and the relative contribution of turnover and nestedness are based on Sørensen (Bray-Curtis) dissimilarity (Sørensen, 1948). Multiple site dissimilarity measures (indicated by capital letters) were generated and gave a single score for β-diversity (βSØR) and the relative contribution of species turnover (βSIM) and nestedness (βSNE) to dissimilarity in each region. A comparison between multiple site dissimilarity scores can only be made between regions with equal sample sizes (Baselga, 2012). However, a repeated sampling of the dissimilarity scores for a subset of the original data frame yields average metrics with standard errors that can be compared between regions with different sample sizes (Baselga, 2012). Although the depth bins were standardized between regions, we report multiple site dissimilarity scores based on 100 sampling repetitions to increase the comparability of the results. Through pairwise comparisons of samples (indicated by lower case letters) we also assessed if β-diversity metrics, referred to as βsør, βsim, and βsne, varied depending on sample depth separations. The relationship between pairwise dissimilarity scores and sample depth separation was explored through least squares linear regression but recognizing that pairwise comparisons inflate the degrees of freedom, a regression was only seen as significant where the resulting p-value for the slope was equal to, or below 0.001.
The direction of nestedness along the bathymetric gradient was explored using the software BINMATNEST (Rodríguez-Gironés and Santamaría, 2006, 2010). The “temperature” of the species presence-absence matrix, which is defined as the sum of “surprises” in an arranged matrix (ranging from 0 to 100°), was determined reflecting a fully nested matrix to nested pattern respectively. The analysis also ranks the depth bins on the basis of nestedness which can be used to inform of the direction of nestedness along an environmental gradient (Stuart et al., 2017). To test if significant nestedness occurred in the species matrices and to rank depth bins on the basis of nestedness, a computation of 1000 null matrices was performed and the species matrices tested for randomness using the recommended choice of null model in BINMATNEST (model 3) (Rodríguez-Gironés and Santamaría, 2006).
Selecting Indicator Species
Bivalve and gastropod species were identified, that could be considered suitable to be monitored to identify early changes in benthic communities due to environmental changes north of the GIF ridge, with special focus on ocean acidification and the decrease in Ω. With the purpose of excluding rare or ubiquitous species, species were listed based on their sampling frequency and abundance in sled samples in the BIOICE dataset, which included 366 samples north of the GIF ridge. In the BIOICE program, species were counted in samples up to at least 100 individuals, so although the dataset does not include complete abundance measurements for highly abundant species, it is possible to identify bivalve and gastropod species as abundant, or not, based on a pre-defined criterion. Species were listed if they met the following criteria: (A) Detected in 40 or more samples; (B) At least 25% of samples included five or more individuals; (C) Detected in 25% or more samples collected within its observed depth range; (D) not observed beyond 1500 m depth in the BIOICE dataset.
With the exception of the BINMATNEST software, all data analysis was performed using R (R-Core-Team, 2018), and the vegan (Oksanen et al., 2015) and betapart packages for R (Baselga and Orme, 2012).
Results
Species Richness
Bivalves were generally more abundant in samples than gastropods, but gastropods were roughly twice as species rich as bivalves in both study regions. The total observed bivalve species richness was 85 north of the GIF ridge and 114 in south of the GIF ridge, with 71 species shared between both regions. Based on the analysis of undetected species richness, the estimated bivalve species richness (±standard error) was 95 ± 8 and 137 ± 15 north and south of the GIF ridge respectively. A bivalve species richness of 7 ± 1 was estimated for the depth range 1501–2000 m north of the GIF ridge compared to a total of 34 ± 6 south of the GIF ridge (Figure 2). The observed gastropod species richness was 173 and 189 north and south of the GIF ridge respectively, with 133 species shared between regions. Estimated total gastropod species richness (±standard error) was 201 ± 12 and 266 ± 33 north and south of the GIF ridge. Gastropod species richness estimated within the depth range 1501–2000 m was 19 ± 11 and 73 ± 9 north and south of the GIF ridge, respectively (Figure 2).
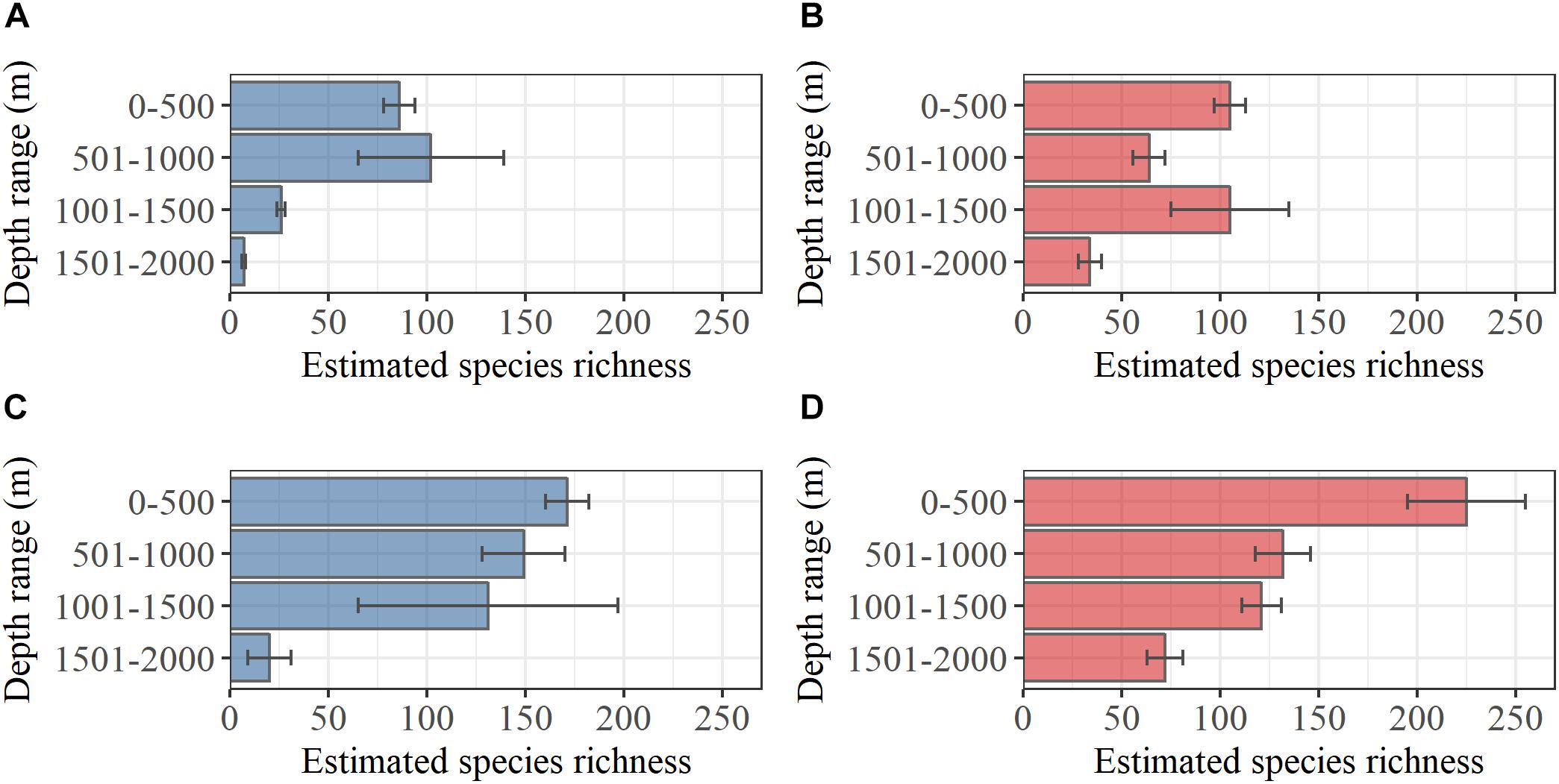
Figure 2. Estimated species richness within 500 m depth intervals based on (Chao, 1987). Error bars represent standard errors of the estimates based on Chiu et al. (2014) for (A) bivalves north of the GIF ridge and (B) bivalves south of the GIF ridge, (C) gastropods north of the GIF ridge and (D) gastropods south of the GIF ridge.
Alpha Diversity
Analysis of E(S20) for bivalves and gastropods north of the GIF ridge indicates a unimodal relationship with depth, reaching a maximum of ∼5 species between 100 and 600 m (Figure 3). A linear regression model of E(S20) fitted within the 300 to 2000 m depth range north of the GIF ridge presents a steep decrease in both bivalve and gastropod α-diversity along the bathymetric gradient with high R2 values found for bivalves (R2 = 0.92) and gastropods (R2 = 0.77) respectively (Table 1). South of the GIF ridge a similar unimodal relationship between α-diversity and depth was observed in bivalves with a modest decrease from 300 to 2000 m depth. In contrast with the north, R2 values were much lower for bivalves in the south (R2 = 0.22) while no clear trend was detected in gastropod E(S20) south of the GIF ridge (Figure 3).
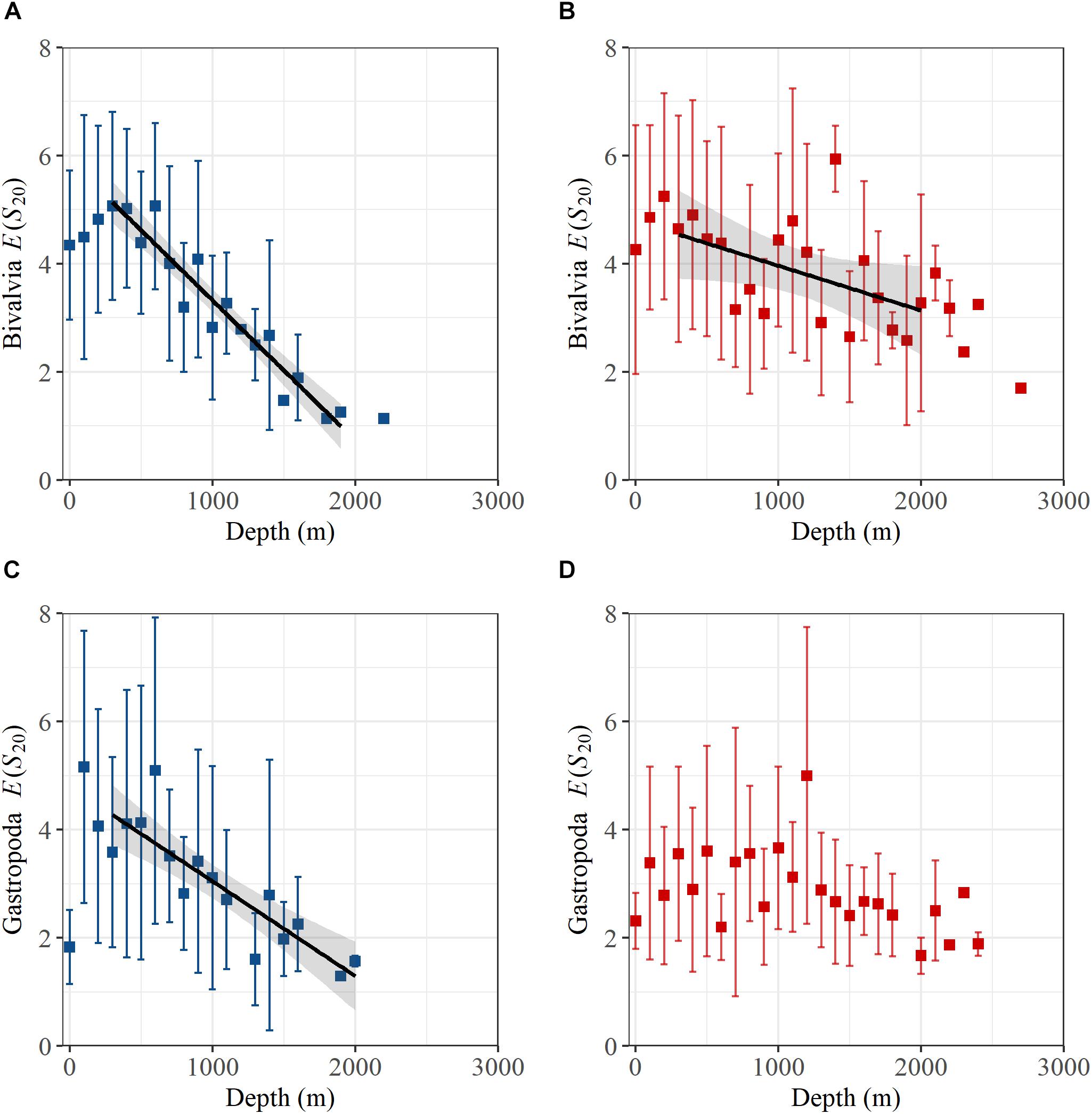
Figure 3. Hurlbert-Sanders expected number of species in a sample of 20 individuals, E(S20), for (A) bivalves north of the GIF ridge, (B) bivalves south of the GIF ridge, (C) gastropods north of the GIF ridge, and (D) gastropods south of the GIF ridge. Rarefied samples were averaged into 100 m depth bins with error bars representing standard deviations. Where only one sample was available for rarefaction there is no error bar shown. Black lines and shaded region are fitted linear regression models and standard error respectively.
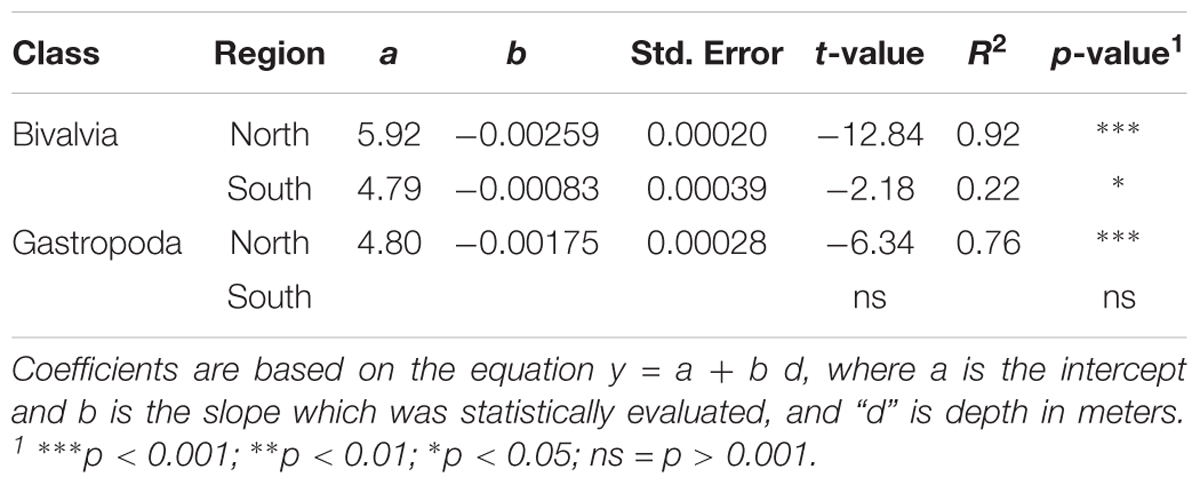
Table 1. Results from least square linear regression models of E(S20) as a function of depth between 300 and 2000 m.
Species Assemblages
Depth influenced community composition more strongly than region in both bivalves and gastropod, but the relationships between these factors and species composition was stronger for bivalves compared to gastropods (Figure 4). The MDS1 score for bivalve communities was strongly correlated with the logarithm of depth (Pearson’s product-moment correlation: r = 0.84, t = 33, df = 469, p < 0.001) and the MDS2 scores differed between the study regions (t-test: t = -26, df = 458, p < 0.001). The MDS1 score for gastropod communities was also significantly correlated with the logarithm of depth although not as strongly as in bivalves (Pearson’s product-moment correlation: r = 0.50, t = -12, df = 406, p < 0.001) and the MDS2 also differed by region (t-test: t = 11, df = 405, p < 0.001).
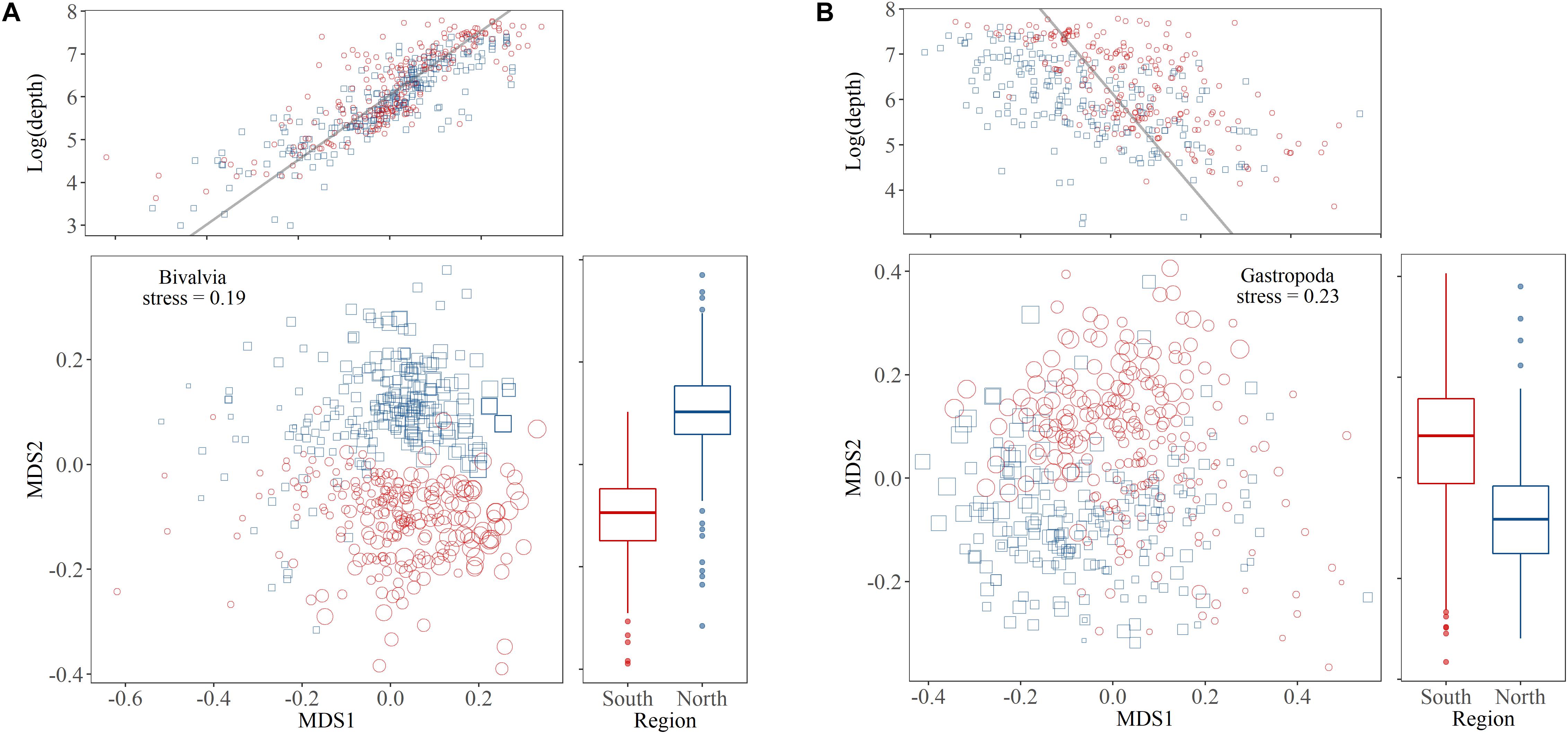
Figure 4. Two-dimensional non-metric multidimensional scaling (NMDS) plots for (A) bivalve and (B) gastropod assemblages. For each class a central plot shows the results of the analysis with sample data representing the Iceland Sea basin north of the GIF ridge (blue squares) and the region south of the GIF ridge (red circles). The point size relates to the logarithm of sample depth. On top is a correlation of the MDS1 scores and the logarithm of depth which was significant in bivalves and gastropods and to the right a boxplot shows the significant regional difference for MDS2 scores in both groups.
Beta Diversity
Analysis of the β-diversity dynamics in bivalve and gastropod species assemblages between 300–1600 m suggests that although the overall β-diversity is comparable between the study regions, there are differences in the relative contribution of nestedness and turnover to β-diversity.
In bivalves, β-diversity was higher north of the GIF ridge compared to that south of the GIF ridge (t-test on pairwise scores for βsør: t = 40.5, df = 189, p < 0.001; Figure 5). Multiple site metrics (±standard deviation) for the region north of the GIF ridge indicate a similar contribution of nestedness (βSNE) and turnover (βSIM) to the overall β-diversity (βSØR) in the region with βSØR = 0.76 ± 0.02, βSIM = 0.39 ± 0.02 and βSNE = 0.37 ± 0.03. Nestedness contributed to a lesser degree to the regional dissimilarity south of the GIF ridge where βSØR = 0.74 ± 0.01, βSIM = 0.58 ± 0.03, and βSNE = 0.17 ± 0.03. Pairwise scores suggest that species community differences increase with increasing depth separation in both regions, i.e., βsør was positively related to depth separation (Table 2). The increase in βsør reflect higher βsne scores with increasing depth separation north of the GIF ridge, whereas species turnover contributes to a greater extent to the change in species communities south of the GIF ridge (Figure 5).
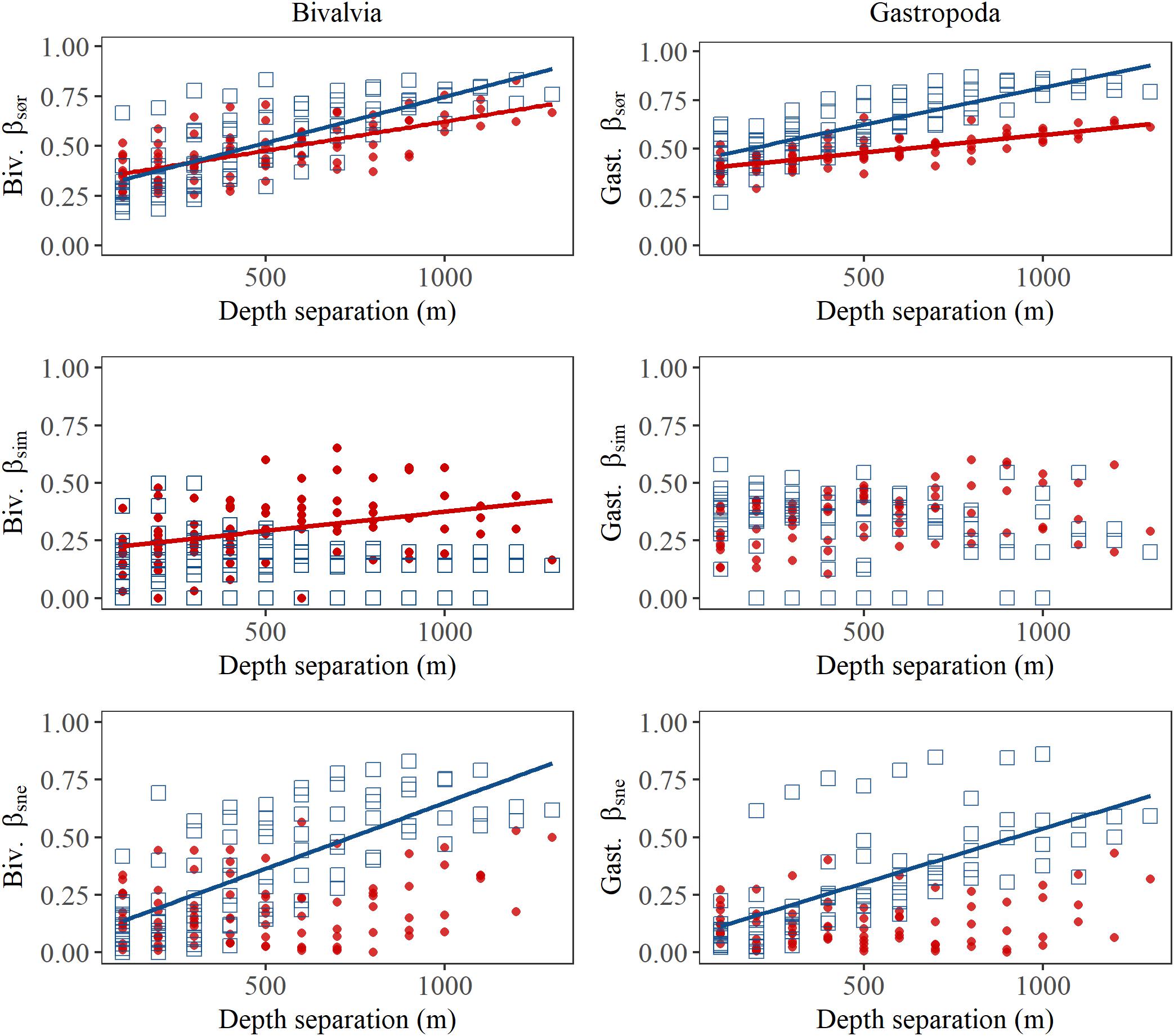
Figure 5. Pairwise dissimilarity of bivalve (left) and gastropod (right) assemblages as a function of depth separation among depth bins in the Iceland Sea north of the GIF ridge (blue square) and south of the GIF ridge (red points). Pairwise overall β-diversity, or βsør is partitioned into βsim or dissimilarity resulting from species replacement and βsne or dissimilarity resulting from nestedness or species loss (or gain). Regression lines indicate where least square linear regression resulted in a p < 0.001 for the slope.
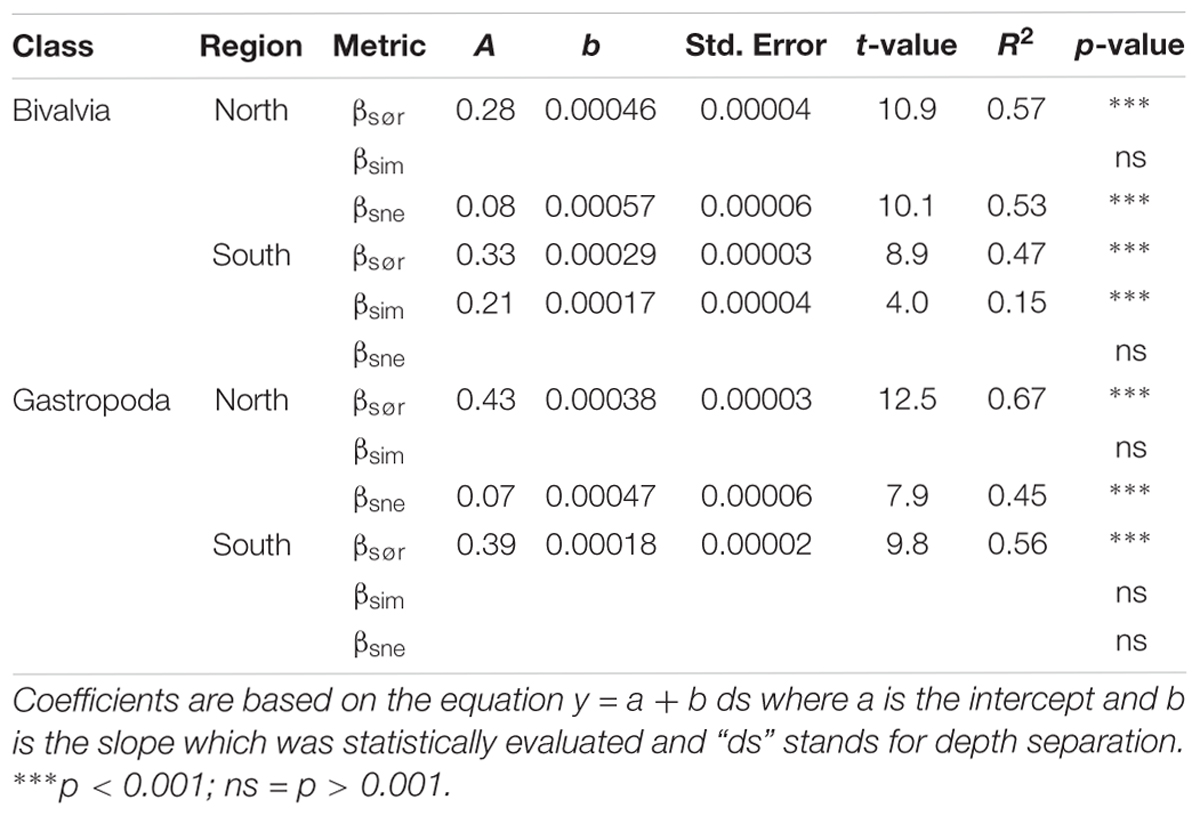
Table 2. Results from linear least squares regression models of Baselga (2010, 2012) pairwise β-diversity metrics as a function of depth separation between 300 and 1600 m.
In gastropods, β-diversity was higher north of the GIF ridge compared to that south of the GIF ridge (t-test on pairwise scores for βsør: t = 47.8, df = 163, p < 0.001; Figure 5). Multiple site metrics (±standard deviation) for the region north of the GIF ridge, βSØR = 0.81 ± 0.01, βSIM = 0.60 ± 0.02, and βSNE = 0.21 ± 0.02, suggest a lesser contribution of nestedness to β-diversity in gastropods compared to bivalves. Multiple site metrics for β-diversity of gastropods south of the GIF ridge were βSØR = 0.75 ± 0.01, βSIM = 0.64 ± 0.02, and βSNE = 0.11 ± 0.02. Results of pairwise comparisons for gastropods indicate an increase in β-diversity (βsør) with increasing depth separation in both regions (Table 2). An increase in βsne with increasing depth separation was observed north of the GIF ridge in gastropods, similar to the trend observed in bivalves (Figure 5). Regression models for gastropods did not detect a trend in βsim with increasing depth separation for either region.
The BINMATNEST test rejected the null hypothesis of no nestedness in bivalve and gastropod species matrices in both regions. A correlation of the rank order of nestedness for each depth bin also indicated a significant linear increase in nestedness with increasing depth (Figure 6). The relationship was relatively strong north of the GIF ridge but relatively weak south of the GIF ridge in both bivalves and gastropods.
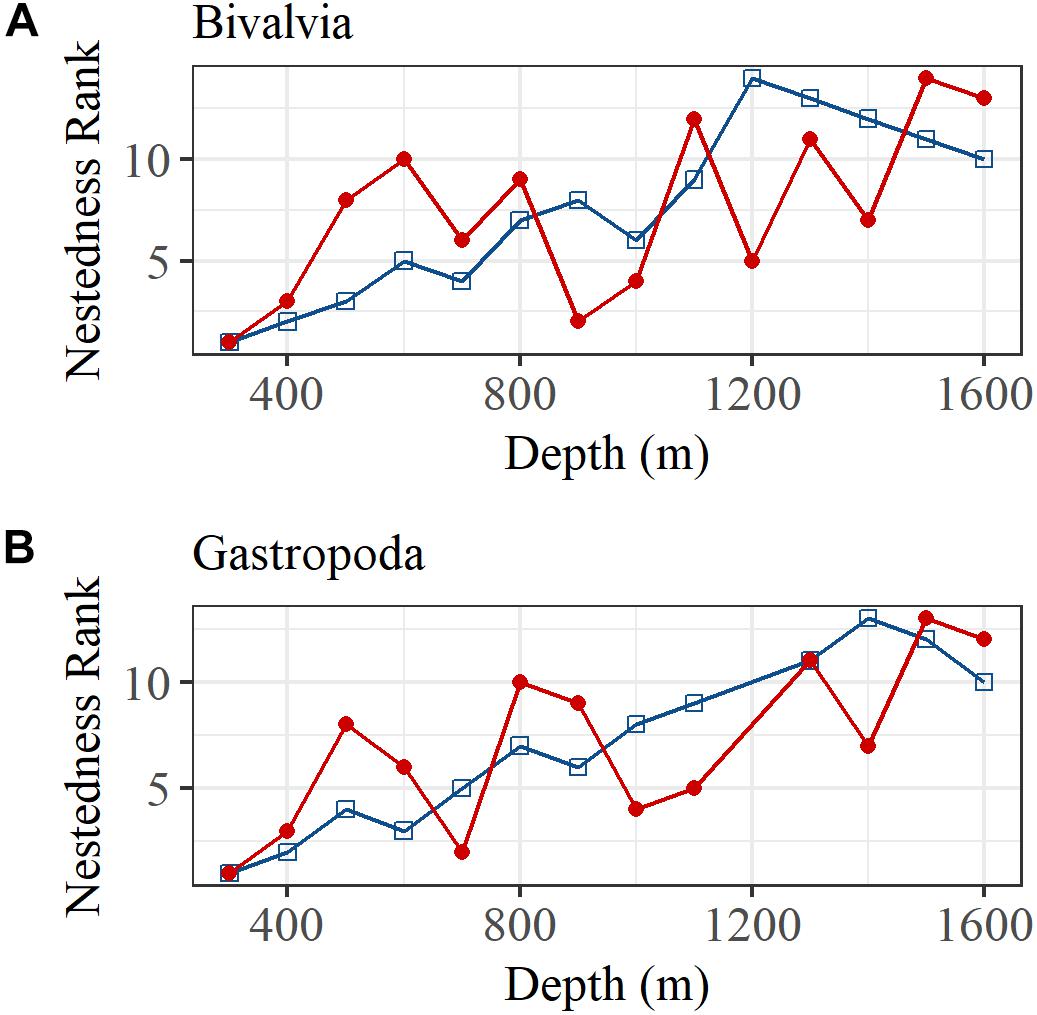
Figure 6. The rank order of nestedness calculated using the BINMATNEST software for (A) bivalves and (B) gastropods, north (blue squares and line) and south (red dots and line) of the GIF ridge.
Identifying Indicator Species
Ten bivalve and five gastropod species met the criteria for monitoring ecological integrity of the benthic ecosystem north of the GIF ridge (Table 3).
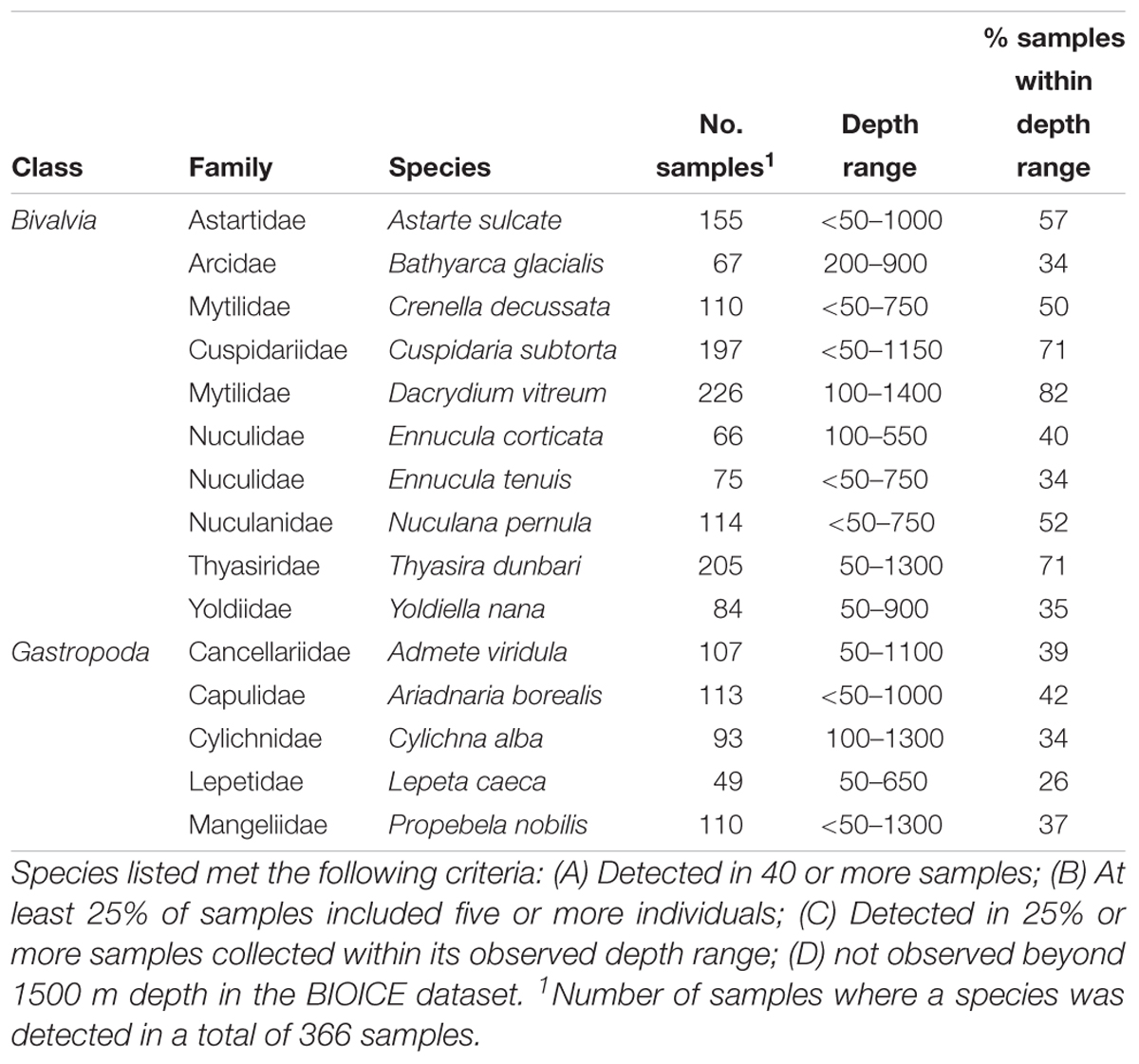
Table 3. Bivalve and gastropod species that are suitable for monitoring ecological integrity of the benthic ecosystem north of the GIF ridge.
Discussion
Species richness on the Icelandic shelf north and south of the GIF ridge was similar. This finding is in agreement with the findings of Piepenburg et al. (2011) who showed that the benthic species richness on the shelfs (above ∼200 m) within the Arctic Seas is not particularly low compared to species richness at lower latitudes, as had been previously suggested based on limited sample data.
Alpha Diversity
We observed a pronounced decrease in α-diversity with increasing depth between 300 and 2000 m depth in both bivalves and gastropods north of the GIF ridge. Notably, species richness estimates indicate an order of a magnitude decrease from the 501–1000 m depth range to the 1501–2000 m depth range in bivalves. These findings are in agreement with a decrease in α-diversity with increasing depth previously described in other benthic taxa in the Nordic Seas, including isopods (Svavarsson, 1997), amphipods (Stephensen, 1940; Weisshappel and Svavarsson, 1998), polychaetes (Narayanaswamy et al., 2005; Oug et al., 2017), foraminifera (Mackensen et al., 1985; Gudmundsson, 2002), and the overall macrofauna (Bett, 2001). In fact, studies on benthic diversity in the deep Nordic Sea basin have consistently described low species richness compared to deep zones in other seas and ocean basins, including the North Atlantic south of the GIF ridge (Bouchet and Warén, 1979; Dahl, 1979; Rex et al., 2000). The cause of the low diversity in the deep Nordic Sea basin is likely linked to low temperatures and limited energy availability as discussed further in the following chapter on beta diversity. The α-diversity trends presented here are also similar to that described for the Arctic Ocean as a whole (Bodil et al., 2011).
The α-diversity patterns observed south of the GIF ridge, i.e., a decrease in E(S20) in bivalves along the bathymetric gradient and a lack of trend in E(S20) in gastropods, contrasts with previously described diversity patterns directly south of the GIF ridge and in the broader North Atlantic (Rex and Etter, 2010). For example, Svavarsson (1997) report an significant increase in isopod E(S200) directly south of the GIF ridge from 200 to 1500 m, but isopod diversity trends are known to deviate from general trends in other macrofauna as the order includes many species specifically adapted to the deep-sea (Hessler et al., 1979; Rex and Etter, 2010). In the broader North Atlantic, Bett (2001) and Narayanaswamy et al. (2005) also reported an increase in overall macrofauna and polychaete E(Sn) between 200 and 2000 m depth in the Rockall Trough, south of the Wyville-Thomson ridge. Also, Brault et al. (2013a) described an increase in E(S20) from the shelf toward depths exceeding 2000 m in bivalves in the western and eastern North Atlantic. The explanation for the observed deviation from the general trend directly south of the GIF ridge is unknown and requires comprehensive data on the environmental setting of the region. A likely explanation is that the oceanographic conditions directly south of the GIF ridge might affect biodiversity patterns (Gudmundsson, 1998), in particular, the relatively brisk current below ∼1500 m depth, carrying North Atlantic Deep Water (NADW) mixed with cool overflow water from the Nordic Seas westward at along the Icelandic continental slope (Dickson et al., 1990; Logemann et al., 2013). Current regimes can also impact upon sediment properties through resuspension of fine sediments, with sediment particle size recognized as an important driver of benthic diversity (Etter and Grassle, 1992; Gray, 2002). A numerical modeling study of sediments indicated that fine grained sediments or clay did not increase with increasing depth south of the GIF ridge as was observed north of the GIF ridge (Ostmann et al., 2014). However, due to the likely patchy sediment environments along the bathymetric gradient (Gray, 1981) and few benthic sampling localities (11) included in the study (Ostmann et al., 2014), the importance of sediment composition in driving diversity trends south of the GIF ridge remains uncertain.
Comparing Bivalves and Gastropods
General trends in bivalve and gastropod α- and β-diversity were similar north and south of the GIF ridge, but there were some marked differences between the groups. A difference is not unexpected as bivalves and gastropods have evolved independently for nearly 500 million years and have different habitat requirements and feeding mechanisms that should be considered (Ponder and Lindberg, 2008). Most bivalves have low mobility, include many infaunal species and are generally suspension or deposit feeders (Roy et al., 2000). Gastropods, on the other hand, are more species rich than bivalves, are functionally more diverse, operating as predators, grazers, omnivores, deposit feeders or carnivores, and are largely epifaunal (Hughes, 1986).
This considered, it is possible that the greater phylogenetic- and functional diversity of gastropods, could account for the larger unexplained variance in overall gastropod assemblages as compared to bivalve assemblages. Despite these differences, species assemblages of both taxonomic groups varied more as a function of water depth than region, indicating that water depth, and specifically the environmental changes associated with water depth, are a significant driver of β-diversity in both regions. This is in agreement with McClain et al. (2012), who described depth to be a better predictor of deep-sea bivalve β-diversity than geographical separation. Our exploration of the BIOICE data also found that a significant number of species were shared between regions to the north and south of the GIF ridge, despite environmental differences.
Beta Diversity
It is possible that the observed patterns in β-diversity could, in conjunction with the rapid decrease in α-diversity along the bathymetric gradient, reflect the geological history of the North Atlantic to some extent (Barry et al., 2013). During the last glacial maximum, ice sheets covered the Nordic Seas, limiting surface primary production and the subsequent downward flux of organic matter to the seafloor. The benthic environment changed dramatically when the ice retreated ∼14 thousand years ago resulting in a significant increase in primary production at the surface and changes in water circulation (Smith et al., 1997; Müller et al., 2009). While Pleistocene glaciations likely eradicated much of the shelf and deep-sea fauna in the Arctic, other shelf fauna found refuge in the deep-sea and are considered the ancestral fauna of some of today’s Arctic deep-sea fauna (Nesis, 1984). Thus, a large proportion of the fauna on the shelfs of the Nordic Seas is likely to have been introduced from the North Atlantic within the Holocene time-period.
Although the geological history of the North Atlantic is likely to play a significant role in shaping the species composition in the Nordic Seas, recent studies provide evidence supporting previous hypothesis indicating that energy dynamics, including chemical energy (i.e., food availability) and kinetic energy (i.e., temperature) are the most important drivers of both α- and β-diversity patterns in the benthic marine environment (Tittensor et al., 2010; Brault et al., 2013a; Woolley et al., 2016; Yasuhara and Danovaro, 2016). Temperature is a particularly important driver at temperatures below 5°C (Yasuhara and Danovaro, 2016) and thus the transition from ∼2 to 4°C south of the GIF ridge to a ∼-1°C north of the ridge undoubtably present many species with a physiological barrier. The physiological effect of hydrostatic pressure in conjunction with low temperatures could also explain the especially low diversity observed in the deep Nordic Sea basin (Brown and Thatje, 2014). Food availability, temperature and hydrostatic pressure are not mutually exclusive drivers but can influence species performance synergistically (e.g., Brockington and Clarke, 2001; Sebert, 2002). The ocean surface north and south of the GIF ridge exhibit significant seasonality in surface primary production, but there are notably shorter periods of phytoplankton blooms in the Iceland Sea, north of the GIF ridge (Pálsson et al., 2012; McGinty et al., 2016). Therefore, the benthic community in the deep Nordic Seas may be mostly composed of species who have successfully adapted to the extreme energy constraints in the region, i.e., sub-zero temperatures and highly pulsed availability of chemical energy.
Based on Baselga (2010) partitioning and the BINMATNEST rank order for bivalve and gastropod assemblages north of the GIF ridge, increasing community dissimilarity is largely due to the increase in nestedness, or species loss with depth, and relates to the decrease in α-diversity. Brault et al. (2013b) also reported lowered α-diversity, coupled with increasing nestedness from bathyal to abyssal depths in neogastropods. They argued that this pattern provides evidence of source-sink dynamics, i.e., that the abyssal populations are not self-sustaining due to the poor habitat quality at greater depths and require introduction of individuals from shallower habitats (Rex et al., 2005; Brault et al., 2013b). Similarly, the increase in nestedness north of the GIF ridge, in concert with the decrease in α-diversity, could indicate that at least some populations of bivalves and gastropods are not self-sustainable in the deeper part of their observed depth ranges, but instead require introduction from a “source”-population at shallower depths where food is more abundant and calcium carbonate saturation states (Ω) higher. For example, bivalve molluscs have been shown to require species specific Ω for successful larval development (Waldbusser et al., 2015). However, as pointed out by Brault et al. (2013b) such diversity patterns do not provide sufficient evidence to establish the existence of source-sink dynamics in a meta-population. Information regarding the genetic structure of “source” and “sink” populations is also required (e.g., Manier and Arnold, 2005).
The contribution of species turnover to β-diversity was considerably greater south of the GIF ridge where nestedness resultant dissimilarity was less significant. Nevertheless, the BINMATNEST analysis of nestedness indicated an increase in nestedness with depth south of the GIF ridge, albeit the correlation was relatively weak compared to that north of the GIF ridge. This was an unexpected finding as species turnover was expected to dominate β-diversity to a greater extent in this region. However, this finding likely relates to the unexpected decrease in α-diversity with increasing depth observed south of the GIF ridge, which again, possibly results from the special oceanographic regime directly south of the GIF ridge.
It is clearly of importance to increase understanding of the relative contribution of various environmental drivers associated with depth (e.g., hydrostatic pressure, food availability, temperature, Ω, and pH) to biodiversity patterns in the deep-sea. Research aimed to increase this understanding remains a challenge due to the significant correlation of multiple environmental parameters along the depth gradient. Recent studies have, however, recognized energy availability as a major driver of diversity patterns in the deep-sea (Brault et al., 2013a; Woolley et al., 2016). Nevertheless, it is likely that other environmental parameters also play a role in driving diversity patterns in distinct groups. For example, the decrease in Ω associated with depth could be a significant driver of biodiversity in the taxonomic groups investigated in this study and other calcifying taxa.
Identifying Indicator Species
Most of the bivalve and gastropod species sampled north of the GIF ridge were recorded at depths shallower than 500 m, with a few species showing extended distribution throughout the bathyal range. Interestingly, the epibenthic pectinid bivalve Hyalopecten frigidus was common in samples collected below 2000 m, suggesting that it is well adapted to the energy constrains of the deep Nordic Sea basin and low Ω. No gastropod species were observed in the few samples collected below 2000 m north of the GIF ridge.
The species considered suitable for monitoring early signs of changes in benthic communities under ocean acidification north of the GIF ridge were 15 in total. Samples collected for the BIOICE program were collected 10–27 years before this study, so it is important to realize that changes in the marine environment north of the GIF ridge are on-going and that environmental changes could already have induced changes in benthic communities in this region. Preferably, an establishment of a monitoring project would require a collection of preliminary data on benthic communities and information on the natural (e.g., annual and seasonal) variability of benthic communities in the region.
Summary and Conclusion
Biodiversity patterns of bivalves and gastropods differed considerably between the bathymetric gradients north and south of the GIF ridge. A clear decrease in α-diversity and an increase in nestedness with depth were observed north of the GIF ridge, whereas a moderate decrease, and no decrease in α-diversity, was observed in bivalves and gastropods, respectively, south of the GIF ridge where species turnover is the dominant β-diversity component. Methods applied to the BIOICE dataset in this study were designed to address issues regarding the non-randomized sampling strategy and the lack of standardized sampling methods. Nevertheless, it is important to acknowledge these issues and the potential effects on the results of this study. As the limited amount of sample data from depths below 1600 m were largely excluded from analysis, this study does not address biodiversity patterns at these greater depths. It is recommended for future research to apply sampling strategies that are better designed to answer questions on biodiversity patterns to validate the findings of this study. Data presented here contribute significantly to the knowledge of molluscan biodiversity and species composition along bathymetric gradients in the high-latitude North Atlantic. This information is important in guiding future attempts to evaluate the degree of vulnerability in bivalve and gastropods communities in the Nordic Seas where environmental change related to ocean warming and acidification is observed and predicted (Olafsson et al., 2009; Bopp et al., 2013) and for efforts to predict the implications of these changes for deep-sea benthic communities more generally (Widdicombe and Spicer, 2008; Urban et al., 2016).
By adding to the general understanding of biodiversity patterns in the North Atlantic, including the Nordic Seas, our results provide an important baseline for future work aimed at quantifying ecosystem responses to rapid environmental changes taking place in the region – information vital for shaping conservation strategies that minimize the detrimental consequences of such changes in the future (Cavanagh et al., 2016).
Data Availability
The raw data supporting the conclusions of this manuscript will be made available by the authors, without undue reservation, to any qualified researcher.
Author Contributions
HE wrote the manuscript and analyzed the data. NM took part in writing the manuscript and data analysis. GG edited the manuscript and provided advise about the species-data.
Funding
This work was supported by the “European Project on Ocean Acidification” EPOCA, which received funding from the European Community’s Seventh Framework Programme (FP7/2007-2013) under grant agreement no. 211384. HE was also supported by a grant from Fisheries Iceland.
Conflict of Interest Statement
The authors declare that the research was conducted in the absence of any commercial or financial relationships that could be construed as a potential conflict of interest.
Acknowledgments
The authors would like to recognize the valuable work of all those who took part in sampling and identifying bivalves and gastropods collected in the BIOICE program, especially Henk H. Djikstra and Jon-Arne Sneli. We also wish to thank Jörundur Svavarsson and Jed McDonald for providing thorough comments on the manuscript.
Supplementary Material
The Supplementary Material for this article can be found online at: https://www.frontiersin.org/articles/10.3389/fmars.2019.00129/full#supplementary-material
References
Almeida-Neto, M., Guimarães, P., Guimarães, P. R., Loyola, R. D., and Ulrich, W. (2008). A consistent metric for nestedness analysis in ecological systems: reconciling concept and measurement. Oikos 117, 1227–1239. doi: 10.1111/j.0030-1299.2008.16644.x
Alongi, D. M. (1990). The ecology of tropical soft-bottom benthic ecosystems. Oceanogr. Mar. Biol. Annu. Rev. 28, 381–496.
Andrew, C., and Scott, L. (2000). Spatial patterns of diversity in the sea: bryozoan species richness in the North Atlantic. J. Anim. Ecol. 69, 799–814. doi: 10.1046/j.1365-2656.2000.00440.x
Barry, T., Berteaux, D., Bültmann, H., Christiansen, J. S., Cook, J. A., Dahlberg, A., et al. (2013). Arctic Biodiversity Assessment 2013. Available at: https://www.caff.is/assessment-series/233-arctic-biodiversity-assessment-2013
Baselga, A. (2010). Partitioning the turnover and nestedness components of beta diversity. Glob. Ecol. Biogeogr. 19, 134–143. doi: 10.1111/j.1466-8238.2009.00490.x
Baselga, A. (2012). The relationship between species replacement, dissimilarity derived from nestedness, and nestedness. Glob. Ecol. Biogeogr. 21, 1223–1232. doi: 10.1111/j.1466-8238.2011.00756.x
Baselga, A., and Orme, C. D. L. (2012). betapart: an R package for the study of beta diversity. Methods Ecol. Evol. 3, 808–812. doi: 10.1111/j.2041-210X.2012.00224.x
Bett, B. J. (2001). UK Atlantic margin environmental survey: introduction and overview of bathyal benthic ecology. Cont. Shelf Res. 21, 917–956. doi: 10.1016/S0278-4343(00)00119-9
Bodil, B. A., Ambrose, W. G. Jr., Bergmann, M., Clough, L. M., Gebruk, A. V., Hasemann, C., et al. (2011). Diversity of the Arctic deep-sea benthos. Mar. Biodivers. 41, 87–107. doi: 10.1007/s12526-010-0078-4
Bopp, L., Resplandy, L., Orr, J. C., Doney, S. C., Dunne, J. P., Gehlen, M., et al. (2013). Multiple stressors of ocean ecosystems in the 21st century: projections with CMIP5 models. Biogeosciences 10, 6225–6245. doi: 10.5194/bg-10-6225-2013
Bouchet, P., and Warén, A. (1979). The abyssal molluscan fauna of the Norwegian Sea and its relation to other faunas. Sarsia 64, 211–243. doi: 10.1080/00364827.1979.10411383
Brattegard, T., and Fosså, J. H. (1991). Replicability of an epibenthic sampler. J. Mar. Biol. Assoc. U.K. 71, 153–166. doi: 10.1017/S0025315400037462
Brault, S., Stuart, C. T., Wagstaff, M. C., McClain, C. R., Allen, J. A., and Rex, M. A. (2013a). Contrasting patterns of α- and β-diversity in deep-sea bivalves of the eastern and western North Atlantic. Deep Sea Res. Part II Top. Stud. Oceanogr. 92, 157–164. doi: 10.1016/j.dsr2.2013.01.018
Brault, S., Stuart, C. T., Wagstaff, M. C., and Rex, M. A. (2013b). Geographic evidence for source–sink dynamics in deep-sea neogastropods of the eastern North Atlantic: an approach using nested analysis. Glob. Ecol. Biogeogr. 22, 433–439. doi: 10.1111/geb.12005
Briggs, J. C. (1970). A faunal history of the North Atlantic Ocean. Syst. Biol. 19, 19–34. doi: 10.1093/sysbio/19.1.19
Brix, S., Stransky, B., Malyutina, M., Pabis, K., Svavarsson, J., and Riehl, T. (2018). Distributional patterns of isopods (Crustacea) in Icelandic and adjacent waters. Mar. Biodivers. 48, 783–811. doi: 10.1007/s12526-018-0871-z
Brockington, S., and Clarke, A. (2001). The relative influence of temperature and food on the metabolism of a marine invertebrate. J. Exp. Mar. Biol. Ecol. 258, 87–99. doi: 10.1016/S0022-0981(00)00347-6
Brown, A., and Thatje, S. (2014). Explaining bathymetric diversity patterns in marine benthic invertebrates and demersal fishes: physiological contributions to adaptation of life at depth. Biol. Rev. 89, 406–426. doi: 10.1111/brv.12061
Carignan, V., and Villard, M.-A. (2002). Selecting indicator species to monitor ecological integrity: a review. Environ. Monit. Assess. 78, 45–61. doi: 10.1023/a:1016136723584
Cavanagh, R. D., Broszeit, S., Pilling, G. M., Grant, S. M., Murphy, E. J., and Austen, M. C. (2016). Valuing biodiversity and ecosystem services: a useful way to manage and conserve marine resources? Proc. R. Soc. B Biol. Sci. 283:20161635. doi: 10.1098/rspb.2016.1635
Chao, A. (1987). Estimating the population size for capture-recapture data with unequal catchability. Biometrics 43, 783–791. doi: 10.2307/2531532
Childress, J. J. (1995). Are there physiological and biochemical adaptations of metabolism in deep-sea animals? Trends Ecol. Evol. 10, 30–36. doi: 10.1016/S0169-5347(00)88957-0
Chiu, C.-H., Wang, Y.-T., Walther, B. A., and Chao, A. (2014). An improved nonparametric lower bound of species richness via a modified good–turing frequency formula. Biometrics 70, 671–682. doi: 10.1111/biom.12200
Crain, C. M., Kroeker, K., and Halpern, B. S. (2008). Interactive and cumulative effects of multiple human stressors in marine systems. Ecol. Lett. 11, 1304–1315. doi: 10.1111/j.1461-0248.2008.01253.x
Dahl, E. (1979). Amphipoda Gammaridea from the deep Norwegian Sea. A preliminary report. Sarsia 64, 57–59. doi: 10.1080/00364827.1979.10411363
Dahlgren, T. G., Weinberg, J. R., and Halanych, K. M. (2000). Phylogeography of the ocean quahog (Arctica islandica): influences of paleoclimate on genetic diversity and species range. Mar. Biol. 137, 487–495. doi: 10.1007/s002270000342
Dickson, R. R., Gmitrowicz, E. M., and Watson, A. J. (1990). Deep-water renewal in the northern North Atlantic. Nature 344, 848–850. doi: 10.1038/344848a0
Etter, R. J., and Grassle, J. F. (1992). Patterns of species diversity in the deep sea as a function of sediment particle size diversity. Nature 360, 576–578. doi: 10.1038/360576a0
Gattuso, J. P., Magnan, A., Billé, R., Cheung, W. W. L., Howes, E. L., Joos, F., et al. (2015). Contrasting futures for ocean and society from different anthropogenic CO2 emissions scenarios. Science 349:aac4722. doi: 10.1126/science.aac4722
Gazeau, F., Parker, L. M., Comeau, S., Gattuso, J.-P., O’Connor, W. A., Martin, S., et al. (2013). Impacts of ocean acidification on marine shelled molluscs. Mar. Biol. 160, 2207–2245. doi: 10.1007/s00227-013-2219-3
Gehlen, M., Séférian, R., Jones, D. O. B., Roy, T., Roth, R., Barry, J., et al. (2014). Projected pH reductions by 2100 might put deep North Atlantic biodiversity at risk. Biogeosciences 11, 6955–6967. doi: 10.5194/bg-11-6955-2014
Gray, J. S. (2002). Species richness of marine soft sediments. Mar. Ecol. Prog. Ser. 244, 285–297. doi: 10.3354/meps244285
Gudmundsson, G. (1998). Distributional limits of Pyrgo species at the biogeographic boundaries of the Arctic and the North-Atlantic Boreal regions. J. Foram. Res. 28, 240–256.
Gudmundsson, G. (2002). Evolution and bipolar distribution of Cornuspiroides (foraminifera): phylogenetic inference using morphological characters and parsimony. J. Foram. Res. 32, 308–318. doi: 10.2113/32.3.308
Gutiérrez, J. L., Jones, C. G., Strayer, D. L., and Iribarne, O. O. (2003). Mollusks as ecosystem engineers: the role of shell production in aquatic habitats. Oikos 101, 79–90. doi: 10.1034/j.1600-0706.2003.12322.x
Hall-Spencer, J., and Moore, P. (2000). Limaria hians (Mollusca: Limacea): a neglected reef-forming keystone species. Aquat. Conserv. 10, 267–277. doi: 10.1002/1099-0755(200007/08)10:4<267::AID-AQC407>3.0.CO;2-B
Hansen, B., and Meincke, J. (1979). Eddies and meanders in the Iceland-Faroe Ridge area. Deep Sea Res. Part A Oceanogr. Res. Pap. 26, 1067–1082. doi: 10.1016/0198-0149(79)90048-7
Hansen, B., and Østerhus, S. (2000). North Atlantic–Nordic Seas exchanges. Prog. Oceanogr. 45, 109–208. doi: 10.1016/S0079-6611(99)00052-X
Hessler, R. R., and Sanders, H. L. (1967). Faunal diversity in the deep-sea. Deep Sea Res. Oceanogr. Abstr. 14, 65–70. doi: 10.1016/0011-7471(67)90029-0
Hessler, R. R., Wilson, G. D., and Thistle, D. (1979). The deep-sea isopods: a biogeographic and phylogenetic overview. Sarsia 64, 67–75. doi: 10.1080/00364827.1979.10411365
Higgs, N., and Attrill, M. (2015). Biases in biodiversity: wide-ranging species are discovered first in the deep sea. Front. Mar. Sci. 2:61. doi: 10.3389/fmars.2015.00061
Hofmann, G. E., Smith, J. E., Johnson, K. S., Send, U., Levin, L. A., Micheli, F., et al. (2011). High-frequency dynamics of ocean pH: a multi-ecosystem comparison. PLoS One 6:e28983. doi: 10.1371/journal.pone.0028983
Horton, T., Kroh, A., Ahyong, S., Bailly, N., Boyko, C. B., Brandão, S. N., et al. (2019). World Register of Marine Species WoRMS. Available at: http://www.marinespecies.org at VLIZ
Hurlbert, S. H. (1971). The nonconcept of species diversity: a critique and alternative parameters. Ecology 52, 577–586. doi: 10.2307/1934145
Jakobsson, M. (2002). Hypsometry and volume of the Arctic Ocean and its constituent seas. Geochem. Geophys. Geosyst. 3, 1–18. doi: 10.1029/2001gc000302
Jöst, A. B., Yasuhara, M., Okahashi, H., Brix, S., Martínez Arbizu, P., and Ostmann, A. (2018). Biogeographic distributions of Cytheropteron species (Ostracoda) in Icelandic waters (sub-polar North Atlantic). Mar. Biodivers. 48, 763–782. doi: 10.1007/s12526-018-0867-8
Linse, K., Griffiths, H. J., Barnes, D. K. A., and Clarke, A. (2006). Biodiversity and biogeography of Antarctic and sub-Antarctic mollusca. Deep Sea Res. Part II Top. Stud. Oceanogr. 53, 985–1008. doi: 10.1016/j.dsr2.2006.05.003
Logemann, K., Ólafsson, J., Snorrason,Á., Valdimarsson, H., and Marteinsdóttir, G. (2013). The circulation of Icelandic waters–a modelling study. Ocean Sci. 9, 931–955. doi: 10.5194/osd-10-763-2013
Lutz, M. J., Caldeira, K., Dunbar, R. B., and Behrenfeld, M. J. (2007). Seasonal rhythms of net primary production and particulate organic carbon flux to depth describe the efficiency of biological pump in the global ocean. J. Geophys. Res. Oceans 112, 1–26. doi: 10.1029/2006JC003706
Mackensen, A., Sejrup, H., and Jansen, E. (1985). The distribution of living benthic foraminifera on the continental slope and rise off southwest Norway. Mar. Micropaleontol. 9, 275–306. doi: 10.1016/0377-8398(85)90001-5
Malmberg, S.-A., and Valdimarsson, H. (2003). Hydrographic conditions in Icelandic waters, 1990–1999. ICES Mar. Sci. Symp. 219, 50–60.
Manier, M. K., and Arnold, S. J. (2005). Population genetic analysis identifies source–sink dynamics for two sympatric garter snake species (Thamnophis elegans and Thamnophis sirtalis). Mol. Ecol. 14, 3965–3976. doi: 10.1111/j.1365-294X.2005.02734.x
May, R. M., and Godfrey, J. (1994). Biological diversity: Differences between land and sea. Philos. Trans. R. Soc. Lond. B Biol. Sci. 343, 105–111. doi: 10.1098/rstb.1994.0014
McClain, C. R., and Rex, M. A. (2015). Toward a conceptual understanding of β-diversity in the deep-sea benthos. Annu. Rev. Ecol. Evol. Syst. 46, 623–642. doi: 10.1146/annurev-ecolsys-120213-091640
McClain, C. R., Stegen, J. C., and Hurlbert, A. H. (2012). Dispersal, environmental niches and oceanic-scale turnover in deep-sea bivalves. Proc. R. Soc. Lond. B Biol. Sci. 279, 1993–2002. doi: 10.1098/rspb.2011.2166
McGinty, N., Guðmundsson, K., Ágústsdóttir, K., and Marteinsdóttir, G. (2016). Environmental and climactic effects of chlorophyll-a variability around Iceland using reconstructed satellite data fields. J. Mar. Syst. 163, 31–42. doi: 10.1016/j.jmarsys.2016.06.005
Millero, F. J. (2007). The marine inorganic carbon cycle. Chem. Rev. 107, 308–341. doi: 10.1021/cr0503557
Müller, J., Masse, G., Stein, R., and Belt, S. T. (2009). Variability of sea-ice conditions in the Fram Strait over the past 30,000 years. Nat. Geosci. 2, 772–776. doi: 10.1038/ngeo665
Narayanaswamy, B. E., Bett, B. J., and Gage, J. D. (2005). Ecology of bathyal polychaete fauna at an Arctic–Atlantic boundary (Faroe–Shetland Channel, North-east Atlantic). Mar. Biol. Res. 1, 20–32. doi: 10.1080/17451000510018971
Nesis, K. (1984). A hypothesis on the origin of western and eastern Arctic distribution areas of marine bottom animals. Sov. J. Mar. Biol. 9, 235–243.
Oksanen, J., Blanchet, F. G., Kindt, R., Legendre, P., Minchin, P. R., O’Hara, R. B., et al. (2015). vegan: Community Ecology Package. R Package Version 2.3-0. Available at: http://CRAN.R-project.org/package=(vegan
Olafsson, J. (2003). Winter mixed layer nutrients in the Irminger and Iceland Seas, 1990-2000. ICES Mar. Sci. Symp. 219, 329–332.
Olafsson, J., Olafsdottir, S. R., Benoit-Cattin, A., Danielsen, M., Arnarson, T. S., and Takahashi, T. (2009). Rate of Iceland Sea acidification from time series measurements. Biogeosciences 6, 2661–2668. doi: 10.5194/bg-6-2661-2009
Olafsson, J., Ólafsdóttir, S. R., Benoit-Cattin, A., and Takahashi, T. (2010). Hydrochemistry in the Irminger and Iceland Seas. PANGAEA. Available at: http://doi.pangaea.de/10.1594/PANGAEA.737186
Ostmann, A., Schnurr, S., and Martínez Arbizu, P. (2014). Marine environment around Iceland: hydrography, sediments and first predictive models of Icelandic deep-sea sediment characteristics. Pol. Polar Res. 35, 151–176. doi: 10.2478/popore-2014-0021
Oug, E., Bakken, T., Kongsrud, J. A., and Alvestad, T. (2017). Polychaetous annelids in the deep Nordic Seas: Strong bathymetric gradients, low diversity and underdeveloped taxonomy. Deep Sea Res. Part II Top. Stud. Oceanogr. 137, 102–112. doi: 10.1016/j.dsr2.2016.06.016
Paine, R. T. (1969). A note on trophic complexity and community stability. Am. Nat. 103, 91–93. doi: 10.1086/282586
Pálsson,ÓK., Gislason, A., Guðfinnsson, H. G., Gunnarsson, B., Ólafsdóttir, S. R., Pétursdóttir, H., et al. (2012). Ecosystem structure in the Iceland Sea and recent changes to the capelin (Mallotus villosus) population. ICES J. Mar. Sci. 69, 1242–1254. doi: 10.1093/icesjms/fss071
Piepenburg, D., Archambault, P., Ambrose, W. G., Blanchard, A. L., Bluhm, B. A., Carroll, M. L., et al. (2011). Towards a pan-Arctic inventory of the species diversity of the macro- and megabenthic fauna of the Arctic shelf seas. Mar. Biodivers. 41, 51–70. doi: 10.1007/s12526-010-0059-7
Ponder, W. F., and Lindberg, D. R. (2008). Phylogeny and Evolution of the Mollusca. Berkeley, CA: University of California Press. doi: 10.1525/california/9780520250925.001.0001
Queirós, A. M., Birchenough, S. N. R., Bremner, J., Godbold, J. A., Parker, R. E., Romero-Ramirez, A., et al. (2013). A bioturbation classification of European marine infaunal invertebrates. Ecol. Evol. 3, 3958–3985. doi: 10.1002/ece3.769
R-Core-Team (2018). R: A Language and Environment for Statistical Computing. Vienna: R Foundation for Statistical Computing.
Rex, M. A. (1973). Deep-sea species diversity: decreased gastropod diversity at abyssal depths. Science 181, 1051–1053. doi: 10.1126/science.181.4104.1051
Rex, M. A., and Etter, R. J. (2010). Deep-Sea Biodiversity: Pattern and Scale. Cambridge, MA: Harvard University Press.
Rex, M. A., McClain, C. R., Johnson, N. A., Etter, R. J., Allen, J. A., Bouchet, P., et al. (2005). A source-sink hypothesis for abyssal biodiversity. Am. Nat. 165, 163–178. doi: 10.1086/427226
Rex, M. A., Stuart, C. T., and Coyne, G. (2000). Latitudinal gradients of species richness in the deep-sea benthos of the North Atlantic. Proc. Natl. Acad. Sci. U.S.A. 97, 4082–4085. doi: 10.1073/pnas.050589497
Rodríguez-Gironés, A. M., and Santamaría, L. (2010). How foraging behaviour and resource partitioning can drive the evolution of flowers and the structure of pollination networks. Open Ecol. J. 3, 1–11. doi: 10.2174/1874213001003040001
Rodríguez-Gironés, M. A., and Santamaría, L. (2006). A new algorithm to calculate the nestedness temperature of presence–absence matrices. J. Biogeogr. 33, 924–935. doi: 10.1111/j.1365-2699.2006.01444.x
Rogers, A. D. (2015). Environmental change in the deep ocean. Annu. Rev. Environ. Resour. 40, 1–38. doi: 10.1146/annurev-environ-102014-021415
Rothlisberg, P., and Pearcy, W. (1977). An epibenthic sampler used to study the ontogeny of vertical migration of Pandalus jordani (Decapoda, Caridea). Fish. Bull. 74, 994–997.
Roy, K., Jablonski, D., and Valentine, J. W. (2000). Dissecting latitudinal diversity gradients: functional groups and clades of marine bivalves. Proc. R. Soc. Lond. B Biol. Sci. 267, 293–299. doi: 10.1098/rspb.2000.0999
Sanders, H. L. (1968). Marine benthic diversity: a comparative study. Am. Nat. 102, 243–282. doi: 10.1086/282541
Schnurr, S., Osborn, K. J., Malyutina, M., Jennings, R., Brix, S., Driskell, A., et al. (2018). Hidden diversity in two species complexes of munnopsid isopods (Crustacea) at the transition between the northernmost North Atlantic and the Nordic Seas. Mar. Biodivers. 48, 813–843. doi: 10.1007/s12526-018-0877-6
Sebert, P. (2002). Fish at high pressure: a hundred year history. Comp. Biochem. Physiol. A Mol. Integr. Physiol. 131, 575–585. doi: 10.1016/S1095-6433(01)00509-8
Seibel, B. A., and Walsh, P. J. (2003). Biological impacts of deep-sea carbon dioxide injection inferred from indices of physiological performance. J. Exp. Biol. 206, 641–650. doi: 10.1242/jeb.00141
Skogen, M. D., Olsen, A., Børsheim, K. Y., Sandø, A. B., and Skjelvan, I. (2014). Modelling ocean acidification in the Nordic and Barents Seas in present and future climate. J. Mar. Syst. 131, 10–20. doi: 10.1016/j.jmarsys.2013.10.005
Smith, J. E., Risk, M. J., Schwarcz, H. P., and McConnaughey, T. A. (1997). Rapid climate change in the North Atlantic during the Younger Dryas recorded by deep-sea corals. Nature 386, 818–820. doi: 10.1038/386818a0
Snelgrove, P. V. R. (1999). Getting to the Bottom of Marine Biodiversity: Sedimentary Habitats: Ocean bottoms are the most widespread habitat on Earth and support high biodiversity and key ecosystem services. Bioscience 49, 129–138. doi: 10.2307/1313538
Sneli, J.-A. (1998). A simple benthic sledge for shallow and deep-sea sampling. Sarsia 83, 69–72. doi: 10.1080/00364827.1998.10413670
Sørensen, T. (1948). A method of establishing groups of equal amplitude in plant sociology based on similarity of species and its application to analyses of the vegetation on Danish commons. Biol. Skr. 5, 1–34.
Stuart, C. T., Brault, S., Rowe, G. T., Wei, C.-L., Wagstaff, M., McClain, C. R., et al. (2017). Nestedness and species replacement along bathymetric gradients in the deep sea reflect productivity: a test with polychaete assemblages in the oligotrophic north-west Gulf of Mexico. J. Biogeogr. 44, 548–555. doi: 10.1111/jbi.12810
Svavarsson, J. (1997). Diversity of isopods (Crustacea): new data from the Arctic and Atlantic Oceans. Biodivers. Conserv. 6, 1571–1579. doi: 10.1023/a:1018322704940
Svavarsson, J., Stromberg, J.-O., and Brattegard, T. (1993). The deep-sea asellote (Isopoda, Crustacea) fauna of the Northern Seas: species composition, distributional patterns and origin. J. Biogeogr. 20, 537–555. doi: 10.2307/2845725
Swift, J. H., Aagaard, K., and Malmberg, S.-A. (1980). The contribution of the Denmark strait overflow to the deep North Atlantic. Deep Sea Res. Part A Oceanogr. Res. Pap. 27, 29–42. doi: 10.1016/0198-0149(80)90070-9
Tittensor, D. P., Mora, C., Jetz, W., Lotze, H. K., Ricard, D., Berghe, E. V., et al. (2010). Global patterns and predictors of marine biodiversity across taxa. Nature 466, 1098–1101. doi: 10.1038/nature09329
Ulrich, W., and Gotelli, N. J. (2007). Null model analysis of species nestedness patterns. Ecology 88, 1824–1831. doi: 10.1890/06-1208.1
Urban, M. C., Bocedi, G., Hendry, A. P., Mihoub, J.-B., Pe’er, G., Singer, A., et al. (2016). Improving the forecast for biodiversity under climate change. Science 353:aad8466. doi: 10.1126/science.aad8466
Wagstaff, M. C., Howell, K. L., Bett, B. J., Billett, D. S., Brault, S., Stuart, C. T., et al. (2014). β-diversity of deep-sea holothurians and asteroids along a bathymetric gradient (NE Atlantic). Mar. Ecol. Prog. Ser. 508, 177–185. doi: 10.3354/meps10877
Waldbusser, G. G., Hales, B., Langdon, C. J., Haley, B. A., Schrader, P., Brunner, E. L., et al. (2015). Saturation-state sensitivity of marine bivalve larvae to ocean acidification. Nat. Clim. Change 5, 273–280. doi: 10.1038/nclimate2479
Weisshappel, J., and Svavarsson, J. (1998). Benthic amphipods (Crustacea: Malacostraca) in Icelandic waters: diversity in relation to faunal patterns from shallow to intermediate deep Arctic and North Atlantic Oceans. Mar. Biol. 131, 133–143. doi: 10.1007/s002270050304
Weisshappel, J. B. (2000). Distribution and diversity of the hyperbenthic amphipod family Eusiridae in the different seas around the Greenland-Iceland-Faeroe-Ridge. Sarsia 85, 227–236. doi: 10.1080/00364827.2000.10414575
Whittaker, R. H. (1960). Vegetation of the Siskiyou Mountains, Oregon and California. Ecol. Monogr. 30, 279–338. doi: 10.2307/1943563
Whittaker, R. H. (1972). Evolution and measurement of species diversity. Taxon 21, 213–251. doi: 10.2307/1218190
Widdicombe, S., and Spicer, J. I. (2008). Predicting the impact of ocean acidification on benthic biodiversity: What can animal physiology tell us? J. Exp. Mar. Biol. Ecol. 366, 187–197. doi: 10.1016/j.jembe.2008.07.024
Woolley, S. N. C., Tittensor, D. P., Dunstan, P. K., Guillera-Arroita, G., Lahoz-Monfort, J. J., Wintle, B. A., et al. (2016). Deep-sea diversity patterns are shaped by energy availability. Nature 533, 393–396. doi: 10.1038/nature17937
Keywords: diversity, alpha diversity, beta diversity, nestedness, bivalvia, gastropoda, Arctic, Iceland
Citation: Egilsdottir H, McGinty N and Gudmundsson G (2019) Relating Depth and Diversity of Bivalvia and Gastropoda in Two Contrasting Sub-Arctic Marine Regions. Front. Mar. Sci. 6:129. doi: 10.3389/fmars.2019.00129
Received: 19 December 2018; Accepted: 04 March 2019;
Published: 22 March 2019.
Edited by:
Telmo Morato, Universidade dos Açores, PortugalReviewed by:
Alastair Brown, University of Southampton, United KingdomPedro A. Ribeiro, University of Bergen, Norway
Copyright © 2019 Egilsdottir, McGinty and Gudmundsson. This is an open-access article distributed under the terms of the Creative Commons Attribution License (CC BY). The use, distribution or reproduction in other forums is permitted, provided the original author(s) and the copyright owner(s) are credited and that the original publication in this journal is cited, in accordance with accepted academic practice. No use, distribution or reproduction is permitted which does not comply with these terms.
*Correspondence: Hronn Egilsdottir, aHJvbm4uZWdpbHNkb3R0aXJAaGFmb2d2YXRuLmlz