- 1CIBIO/InBIO, Centro de Investigação em Biodiversidade e Recursos Genéticos, Universidade do Porto, Vairão, Portugal
- 2EPHYSLAB, Environmental Physics Laboratory, Facultad de Ciencias, Universidad de Vigo, Ourense, Spain
- 3Departamento de Biologia, Faculdade de Ciências da Universidade do Porto, Porto, Portugal
- 4Department of Biological Sciences, University of South Carolina, Columbia, SC, United States
Coastal marine biodiversity within eastern boundary upwelling systems (EBUS) is closely linked to the cooler sea temperatures associated with them. It has been suggested that global warming could lead to enhanced sea surface cooling in EBUS via the intensification of upwelling-favorable winds. Conversely, increased stratification and the widespread warming of the world’s oceans could drive these systems in the opposite direction. These competing mechanisms hold the potential for driving the thermal envelopes of EBUS toward – or away from – the thermal envelopes found outside EBUS, with likely contrasting implications for biodiversity conservation in each scenario. Here we characterize the patterns of net sea surface warming rates over more than three decades throughout the global ocean to evaluate if waters inside EBUS are changing differently from those outside EBUS. Results point to a trend of reduced warming inside EBUS, especially along the nearshore. We found that reduced net warming was prevalent in Pacific EBUS but restricted in Atlantic EBUS. In contrast, net warming in the coastal ocean outside EBUS was pervasive and generally associated with proximity to land. Our results suggest that EBUS have been responding to climate change differently from the rest of the global ocean, potentially buffering coastal biomes from decades of global warming.
Introduction
Eastern boundary upwelling systems (EBUS) cover a small area of the world’s oceans, yet they contribute disproportionately to global ocean productivity (IPCC, 2014). While the influence of upwelling over productivity has been widely investigated (Mackas et al., 2006), few studies address the thermal fingerprint of upwelling as a major ecosystem modulator per se (Emerson, 1956; Bosman et al., 1987; Fenberg et al., 2015), despite extensive evidence of temperature as a strong driver of the geographical distributions of coastal species (Breeman, 1988). During transient periods of upwelling relaxation, sea surface temperature (SST) raises dramatically (Send et al., 1987; Barth et al., 2007), a reminder that without upwelling these regions would be markedly warmer. Hence, uplifted cold bottom water shapes the thermal environment within upwelling regions (Kämpf and Chapman, 2016), providing key thermal refugia to cold-affinity species. Climate change impacts SST inside EBUS in two main ways: cooling down coastal waters via the intensification of upwelling-favorable winds (Bakun, 1990; Sydeman et al., 2014), or warming them through increased stratification and the widespread warming of the world’s oceans (IPCC, 2013). The net outcome from the interplay between these two opposing forces is complex and varies spatially and temporally (Bakun et al., 2015; Rykaczewski et al., 2015), which may explain why, inside EBUS, evidence on past SST trends and confidence in future predictions remains fragmented and conflicting (García-Reyes et al., 2015; Varela et al., 2015). Even less clear is the extent to which these and other competing mechanisms could be forcing the thermal envelopes of EBUS to become more similar to the thermal envelopes found in waters outside EBUS. Here we present estimates of sea surface warming rates over more than three decades at a 500-km band along the world’s coastlines and investigate if EBUS are warming faster or slower than the rest of the world’s ocean.
Materials and Methods
We used 37 years of daily SSTs (1982–2018) from NOAA 1/4 arc-degree Daily Optimum Interpolation SST version 2 [dOISST.v2; (Banzon et al., 2016)] to investigate if EBUS are warming at the same rate as the rest of the world’s oceans. We followed a conservative approach, using a broad definition of EBUS boundaries (Kämpf and Chapman, 2016) and avoiding spatial or temporal data sub-setting as over-tailoring is known to hinder cross-study comparisons (García-Reyes et al., 2015). We also used the entire dataset available because, by definition, it is not possible to extract data during the upwelling season in non-upwelling locations. Shorter-term fluctuations (e.g., inter-annual variability or decadal trends) were not considered in this analysis for the sake of clarity. Thus, trends reported reveal net warming over the entire study period, irrespective of driving mechanisms or trend reversals over shorter periods of time. We used AVHRR-only data due to its long temporal span and because it has been shown to out-perform other datasets in coastal areas (Lima and Wethey, 2012). By using only remotely sensed SST we bypass the difficulty in obtaining global, consistent and continuous in situ records, but caution is warranted since nearshore satellite-derived data involve greater interpolation, more so within upwelling regions due to their particularly unfavorable atmospheric conditions. Despite the cautionary advice, it is worth noting that the majority of the additional interpolation occurs in upwelling locations and under peak upwelling conditions, with the implication that any bias introduced will tend to be toward the overestimation of SST values. Hence, the methodology used here yields conservative estimates of the magnitude of the differences between the warming rates inside and outside EBUS.
The analysis was restricted to a 500-km band along the world’s coastlines in order to include most portions of coastal ocean under the influence of upwelling (Kämpf and Chapman, 2016). Other methods to set a boundary that could be considered more geographically relevant – such as isobaths or wind stress curl – were avoided because in some locations or periods they result in very narrow bands of usable data – most notably off the Humboldt system – rendering comparisons between systems and the rest of the global ocean impractical. Distance to land was computed using the dOISST.v2 land mask. For each individual pixel, seasonal variability was removed by subtracting from each day its corresponding climatology [a 7-day running mean of the average temperature for each day of the year, computed over the entire period under analysis; (Foster and Rahmstorf, 2011; Chatfield, 2016)]. Average warming rates were then calculated as the slope of the linear regression of seasonally detrended SST versus time (Lima and Wethey, 2012). To compensate for temporal autocorrelation that could be causing an underestimation of the standard errors of the warming rates, we reduced the effective degrees of freedom using a conservative approach (Foster and Rahmstorf, 2011) where autocorrelation structure was treated as an autoregressive moving average process – ARMA(1,1). Spatial averages of warming rates were based on area-weighted grid point estimates. The full code used in this analysis can be found at https://github.com/ruiseabra/oisst_warming_trends.git.
Results
Overall, the mean rate of warming inside EBUS (0.07 ± 0.14°C/decade [s.d.]; Figure 1) was less than half the warming rate found in the coastal ocean outside EBUS (0.19 ± 0.11°C/decade [s.d.]; hereafter referred as non-EBUS), and close to half the rate for the global ocean surface between 60°N and 60°S (0.15 ± 0.11°C/decade [s.d.]). Warming in EBUS was slowest closer to the shore, whereas in non-EBUS the reverse occurred (Figures 2A–D). In addition, nearly two thirds of the area of EBUS warmed slower than the global ocean (one third in non-EBUS) and a third of it has even cooled (3.5% in non-EBUS), suggesting a buffering effect on warming is present along EBUS.
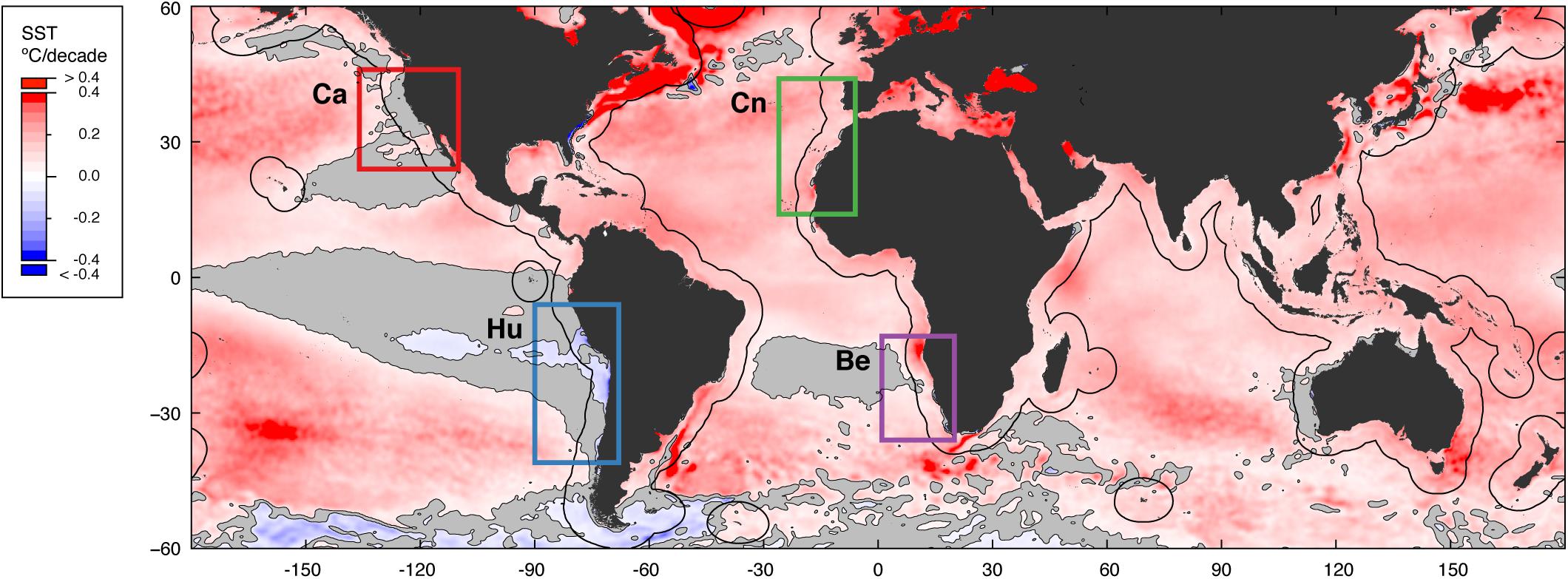
Figure 1. Sea surface warming rates (1982–2018) throughout the global ocean. Colored squares delimit EBUS (Ca: California, Hu: Humboldt, Cn: Canary, Be: Benguela). Black lines offshore from major landmasses and large islands indicate the 500 km distance from land. Gray areas correspond to statistically non-significant rates of SST change at the α = 0.05 level.
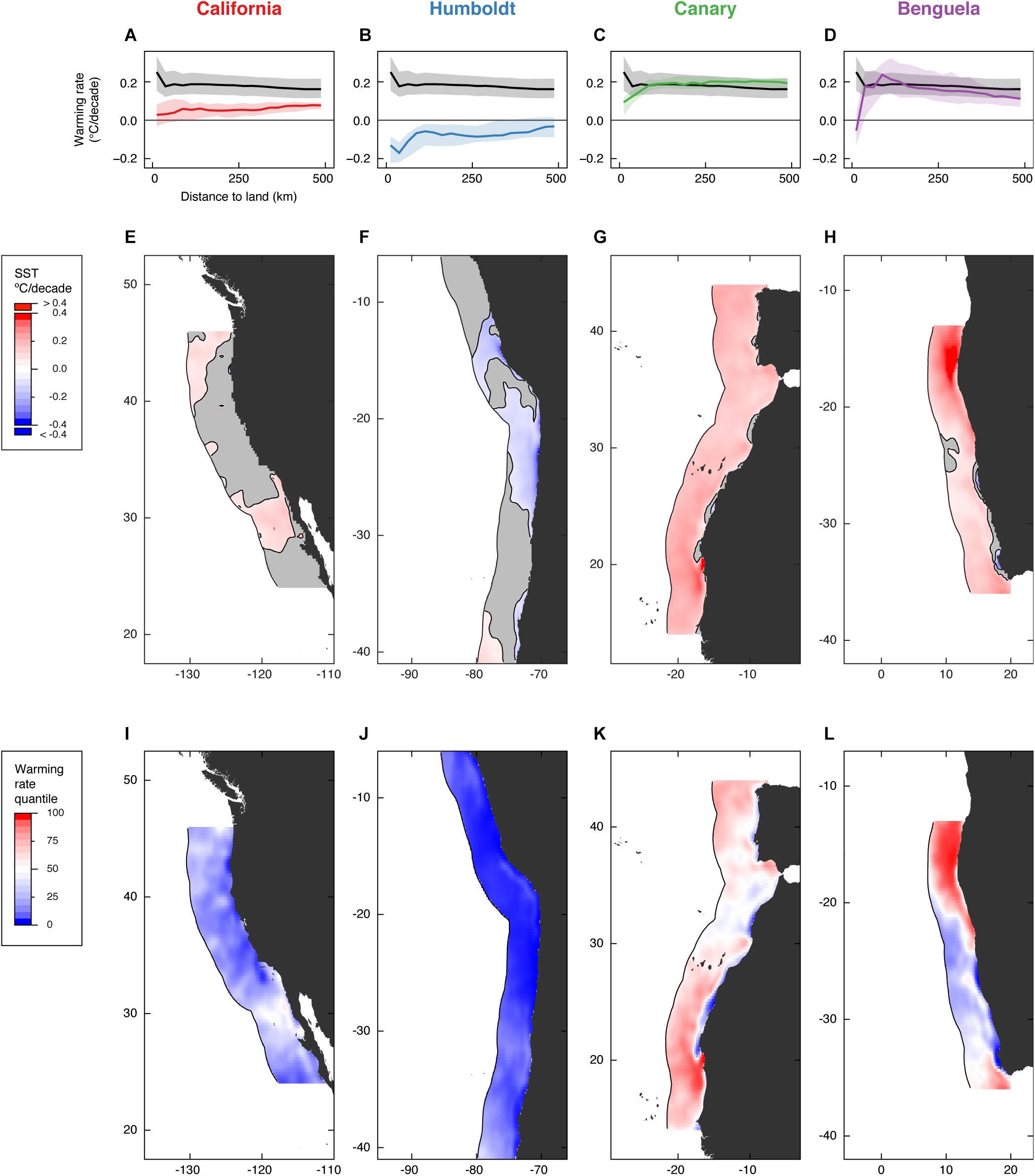
Figure 2. Patterns of SST warming along the coastal ocean (up to 500 km from major landmasses) inside EBUS. Area-weighted warming rate averages for each 25 km distance bin show that while warming has been fastest toward the nearshore in non-EBUS (black line over gray shade), the opposite occurred in all EBUS (A–D; lines correspond to the median and shaded areas represent the interquartile range). The reduced warming along the nearshore is also evident in the detailed views of the SST warming rates inside EBUS (E–H) and their ranking relative to the warming rates found in the entire global ocean between 60°N and 60°S (I–L). Gray areas in panels E–H correspond to statistically non-significant rates of SST change at the α = 0.05 level.
Patterns in warming trends also varied considerably between ocean basins, between EBUS, and inside some of the EBUS. Warming was attenuated to a greater extent in Pacific EBUS than in Atlantic EBUS, at all distances (average warming rates 0.06 ± 0.05 and –0.07 ± 0.08°C/decade [s.d.] in California and Humboldt, 0.19 ± 0.06 and 0.17 ± 0.11°C/decade [s.d.] in Canary and Benguela; Figures 2E–H). Rates of SST change were relatively homogenous over the entire span of the California and Humboldt upwelling systems (Figures 2E,F), and large portions have even experienced a net significant cooling (p < 0.05) over the past 37 years. Waters in Pacific EBUS ranked among those with the lowest warming rates in the entire global ocean (average warming rate quantile 20.3 ± 11.6 in California and 4.3 ± 5.3 in Humboldt [s.d.], with quantiles ranging from 0.0 to 60.0; Figures 2I,J). In contrast, warming rates were highly heterogeneous in the Canary and Benguela upwelling systems (Figures 2G,H), with very high warming rates recorded for vast swaths of coastal ocean, and reduced warming restricted to small pockets along the nearshore. The strong divergence between the warming trend in some nearshore locations and their oceanic counterparts has been noted before (Santos et al., 2012a,b) and is a distinctive characteristic of these two systems. Rates of SST change in Atlantic EBUS ranked markedly higher (average warming rate quantile 61.5 ± 17.6 in Canary and 52.4 ± 28.9 in Benguela [s.d.]; Figures 2K,L), and both include areas among those that warmed the least and warmed the most in the entire global ocean (warming rate quantiles ranging from 0.1 to 99.8 in Canary and 0.0 to 99.7 in Benguela). Despite widespread warming in Atlantic EBUS, the majority of the nearshore still warmed slower than the median warming for the nearshore in the entire global ocean (90.3% of the nearshore in Canary and 95.2% in Benguela; 97.7% in California and 100% in Humboldt).
Discussion
Our results point to a pattern of reduced net warming inside EBUS, especially toward the nearshore. There is, however, an important distinction between less-than-average warming and cooling. In this respect, the Humboldt system is the only EBUS where SST consistently showed a negative linear trend over the last 37 years. This is partially in contrast with previous indications that both the California and Humboldt systems had been mostly cooling along the nearshore (Lima and Wethey, 2012). This attenuation of the negative linear trend observed for the California system between 1982 and 2012 is likely related with the strong 2014–2016 Northeast Pacific marine heat wave and its associated persistent positive anomalies along the nearshore (Chao et al., 2017; Gentemann et al., 2017). Such short-term, sharp changes in SST warming trends are naturally not evidenced in multi-decadal SST warming trends, and this is a clear limitation of this study. Still, despite this recent warming of the California system, the magnitude and spatial heterogeneity of warming trends are lower in Pacific EBUS than in Atlantic EBUS, suggesting that basin-wide processes, rather than hemisphere-wide [e.g., the pronounced warming of the northern hemisphere (Ji et al., 2014)], are likely to be more important drivers of the warming patterns in EBUS (Mendelssohn and Schwing, 2002).
While the present analysis is mostly focused toward the description of spatial patterns of net SST warming rates, insights can still be drawn regarding how the underlying driving mechanisms might be changing over time. In particular, it has been suggested that climate change may lead to the poleward migration of upwelling-favorable winds (Rykaczewski et al., 2015). While it is unclear if and how changes in upwelling intensity modulate warming rates, the presence of upwelling seems to be sufficient to affect SST trends (Lima and Wethey, 2012; Varela et al., 2015). Thus, it follows that locations at the equatorial edge of EBUS should be at greater risk of upwelling shutdown, and consequently of drastic changes in their thermal envelope (deCastro et al., 2014). The remarkably high warming rates here reported for the equatorial portions of Atlantic EBUS (0.60°C/decade off Mauritania, southern Canary, and 0.54°C/decade off Angola, northern Benguela) are consistent with this mechanism. This rapid rise of temperature has occurred in the vicinity of other regions that have experienced no net warming (e.g., –0.05 to 0.60°C/decade 140 km apart at the southern edge of Canary EBUS; Figure 2G), which might be a suggestion that upwelling as a whole could be shifting geographically rather than monotonically expanding or intensifying (Cropper et al., 2014). Alternatively, the same pattern could be an expression of increasing incursions – or even migration – of regional oceanic currents. This is particularly evident in the Benguela system where the equatorial warming could be due shifts in the Angola Current and the warming signal at the polar edge could be the result of fluctuations in the intensity of the Agulhas Current, rather than a consequence of changes to the local upwelling regime (Rouault et al., 2010). We found no evidence of a similar pattern of rapid warming at the equatorial edge in any of the Pacific EBUS (Figures 2E,F). The combined effects of the warming of deep ocean layers (Roemmich et al., 2015), which may lead to an increase in the baseline temperature of upwelled waters, and the projected unabated global temperature rise (Raftery et al., 2017) will likely lead to further increased pressure on the pockets of reduced warming in Atlantic EBUS.
The complex patterns of warming in EBUS reported here could have important biological ramifications. While peak bottom water uplift is spatially congruent with the thermal signal, peak productivity is temporally and spatially offset (Kämpf and Chapman, 2016). This means that in Atlantic EBUS nutrient rich bottom water is being increasingly upwelled to warmer and more stratified areas, especially toward their equatorial edge, with yet debatable consequences for marine productivity and fisheries (Demarcq, 2009; Rykaczewski and Dunne, 2010; Gruber, 2011; Renault et al., 2016). In contrast, Pacific EBUS – and most notably the Humboldt system – appear to be more thermally stable, potentially offering some respite to coastal pelagic and benthic communities from the negative pressures imposed by human activity (e.g., pollution and overfishing). The warming trends identified are perhaps even more significant for the interpretation of nearshore biogeography. Sessile and low-mobility organisms inhabiting rocky shores and nearshore benthic ecosystems of EBUS typically rely on the unique thermal envelope brought about by the upwelling of cold water (Bosman et al., 1987; Fenberg et al., 2015; Seabra et al., 2016) and have limited ability to escape impending changes. Regions with a strong upwelling-driven thermal signal have been described as important refugia and are associated with unique community compositions and genetic pools (van den Hoek and Donze, 1967; Lourenço et al., 2016). Our results clearly affirm the role of EBUS in providing nearshore communities with long-term environmental stability, effectively buffering them from the strong warming experienced along the nearshore elsewhere in the global coastal ocean over the past 37 years. If the nearshore of EBUS had warmed at the same rate as the nearshore along non-EBUS, it would currently be on average 0.9 ± 0.8°C warmer (from 0.6 ± 0.7°C in Canary to 1.4 ± 0.8°C in Humboldt [s.d.]). This stability, however, may lead to delayed biogeographic responses, and risks leaving species with reduced dispersal capabilities outpaced by climate change should upwelling catastrophically collapse. As reported, such regime shifts are more likely toward the equatorial edge of EBUS and appear to already be taking place in Atlantic EBUS. This issue is compounded by the shallow spatial gradients of SST naturally found along equatorial regions (Burrows et al., 2011), which already expose equatorial species to very high velocities of habitat change. Furthermore, the progressive reduction of areas with low warming rates into restricted nearshore pockets can potentially trap species, turning these areas into net sinks of species richness and genetic diversity (Burrows et al., 2014; Lourenço et al., 2016). Non-linear biodiversity responses to changes in upwelling (Iles et al., 2011) further complicate our understanding of climate change impacts in these systems. Importantly, the coastal regions along the two equatorial edges of Atlantic EBUS, where the thermal signature of upwelling appears to be changing the most, are severely understudied, impairing our ability to accurately assess the biological significance of the important shift that has already taken place. This mounting pressure must be taken into consideration when designing research and conservation policies.
Overall, our results suggest that moderate ecosystem-wide changes driven by shifts in the thermal envelope may be afoot in the California system, should not be significant in the Humboldt system, and can be expected to be extensive in both Atlantic EBUS. In particular, the provision of cool habitat along the Canary upwelling system appears to be highly compromised.
Data Availability
The datasets generated for this study are available on request to the corresponding author.
Author Contributions
FL and MG-G designed the study. RS and AS developed the software. RS and FL performed the analyses. RS wrote the initial version of the manuscript. All authors discussed and interpreted the results and edited the manuscript.
Funding
This research was supported by FEDER funds through the Operational Program for Competitiveness Factors – COMPETE and by National Funds through FCT – Foundation for Science and Technology under the references NORTE-01-0145-FEDER-031053 and POCI-01-0145-FEDER-031088, and by Xunta de Galicia under the project ED431C 2017/64-GRC “Programa de Consolidación e Estruturación de Unidades de Investigación Competitivas (Grupos de Referencia Competitiva).” RS was supported by MARINFO – NORTE-01-0145-FEDER-000031, funded by Norte Portugal Regional Operational Program (NORTE2020), under the PORTUGAL 2020 Partnership Agreement, through the European Regional Development Fund (ERDF). FL and CM were supported by FCT (IF/00043/2012 and PD/BD/114038/2015, respectively). DW was supported by NSF (1129401) and NASA (NNX11AP77G).
Conflict of Interest Statement
The authors declare that the research was conducted in the absence of any commercial or financial relationships that could be construed as a potential conflict of interest.
References
Bakun, A. (1990). Global climate change and intensification of coastal ocean upwelling. Science 247, 198–201. doi: 10.1126/science.247.4939.198
Bakun, A., Black, B. A., Bograd, S. J., García-Reyes, M., Miller, A. J., Rykaczewski, R. R., et al. (2015). Anticipated effects of climate change on coastal upwelling ecosystems. Curr. Clim. Change Rep. 1, 85–93. doi: 10.1007/s40641-015-0008-4
Banzon, V., Smith, T. M., Chin, T. M., Liu, C., and Hankins, W. (2016). A long-term record of blended satellite and in situ sea-surface temperature for climate monitoring, modeling and environmental studies. Earth Syst. Sci. Data 8, 165–176. doi: 10.5194/essd-8-165-2016
Barth, J. A., Menge, B. A., Lubchenco, J., Chan, F., Bane, J. M., Kirincich, A. R., et al. (2007). Delayed upwelling alters nearshore coastal ocean ecosystems in the northern California current. Proc. Natl. Acad. Sci. U.S.A. 104, 3719–3724. doi: 10.1073/pnas.0700462104
Bosman, A. L., Hockey, P. A. R., and Siegfried, W. R. (1987). The influence of coastal upwelling on the functional structure of rocky intertidal communities. Oecologia 72, 226–232. doi: 10.1007/BF00379273
Breeman, A. M. (1988). Relative importance of temperature and other factors in determining geographic boundaries of seaweeds: experimental and phenological evidence. Helgoland. Mar. Res. 42, 199–241. doi: 10.1007/BF02366043
Burrows, M. T., Schoeman, D. S., Buckley, L. B., Moore, P. J., Poloczanska, E. S., Brander, K., et al. (2011). The pace of shifting climate in marine and terrestrial ecosystems. Science 334, 652–655. doi: 10.1126/science.1210288
Burrows, M. T., Schoeman, D. S., Richardson, A. J., Molinos, J. G., Hoffmann, A., Buckley, L. B., et al. (2014). Geographical limits to species-range shifts are suggested by climate velocity. Nature 507, 492–495. doi: 10.1038/nature12976
Chao, Y., Farrara, J. D., Bjorkstedt, E., Chai, F., Chavez, F., Rudnick, D. L., et al. (2017). The origins of the anomalous warming in the California coastal ocean and San Francisco Bay during 2014-2016. J. Geophys. Res. Oceans 122, 7537–7557. doi: 10.1002/2017JC013120
Cropper, T. E., Hanna, E., and Bigg, G. R. (2014). Spatial and temporal seasonal trends in coastal upwelling off Northwest Africa, 1981-2012. Deep Sea Res. Part II 86, 94–111. doi: 10.1016/j.dsr.2014.01.007
deCastro, M., Gómez-Gesteira, M., Costoya, X., and Santos, F. (2014). Upwelling influence on the number of extreme hot SST days along the Canary upwelling ecosystem. J. Geophys. Res. Oceans 119, 3029–3040. doi: 10.1002/2013JC009745
Demarcq, H. (2009). Trends in primary production, sea surface temperature and wind in upwelling systems (1998-2007). Prog. Oceanogr. 83, 376–385. doi: 10.1016/j.pocean.2009.07.022
Emerson, W. K. (1956). Upwelling and associated marine life along Pacific Baja California, Mexico. J. Paleontol. 30, 393–397. doi: 10.2307/1300275
Fenberg, P. B., Menge, B. A., Raimondi, P. T., and Rivadeneira, M. M. (2015). Biogeographic structure of the northeastern Pacific rocky intertidal: the role of upwelling and dispersal to drive patterns. Ecography 38, 83–95. doi: 10.1111/ecog.00880
Foster, G., and Rahmstorf, S. (2011). Global temperature evolution 1979–2010. Environ. Res. Lett. 6:044022. doi: 10.1088/1748-9326/6/4/044022
García-Reyes, M., Sydeman, W. J., Schoeman, D. S., Rykaczewski, R. R., Black, B. A., Smit, A. J., et al. (2015). Under pressure: climate change, upwelling, and eastern boundary upwelling ecosystems. Front. Mar. Sci. 2:109. doi: 10.3389/fmars.2015.00109
Gentemann, C. L., Fewings, M. R., and Reyes, M. G. (2017). Satellite sea surface temperatures along the West Coast of the United States during the 2014–2016 northeast Pacific marine heat wave. Geophys. Res. Lett. 44, 312–319. doi: 10.1002/2016GL071039
Gruber, N. (2011). Warming up, turning sour, losing breath: ocean biogeochemistry under global change. Philos. Trans. A Math. Phys. Eng. Sci. 369, 1980–1996. doi: 10.1098/rsta.2011.0003
Iles, A. C., Gouhier, T. C., Menge, B. A., Stewart, J. S., Haupt, A. J., and Lynch, M. C. (2011). Climate-driven trends and ecological implications of event-scale upwelling in the California Current System. Glob. Change Biol. 18, 783–796. doi: 10.1111/j.1365-2486.2011.02567.x
IPCC (2013). Climate Change 2013: The Physical Science Basis. Contribution of Working Group I to the Fifth Assessment Report of the Intergovernmental Panel on Climate Change, eds T. F. Stocker, D. Qin, G. K. Plattner, M. Tignor, S. K. Allen, J. Boschung, et al. Cambridge: Cambridge University Press.
IPCC (2014). Climate Change 2014: Impacts, Adaptation, and Vulnerability. Part B: Regional Aspects. Contribution of Working Group II to the Fifth Assessment Report of the Intergovernmental Panel of Climate, eds V. R. Barros, C. B. Field, D. J. Dokken, M. D. Mastrandrea, K. J. Mach, T. E. Bilir, et al. Cambridge: Cambridge University Press.
Ji, F., Wu, Z., Huang, J., and Chassignet, E. P. (2014). Evolution of land surface air temperature trend. Nat. Clim. Change 4, 462–466. doi: 10.1038/nclimate2223
Kämpf, J., and Chapman, P. (2016). Upwelling Systems of the World. Cham: Springer International Publishing, doi: 10.1007/978-3-319-42524-5
Lima, F. P., and Wethey, D. S. (2012). Three decades of high-resolution coastal sea surface temperatures reveal more than warming. Nat. Commun. 3:704. doi: 10.1038/ncomms1713
Lourenço, C. R., Zardi, G. I., McQuaid, C. D., Serrão, E. A., Pearson, G. A., Jacinto, R., et al. (2016). Upwelling areas as climate change refugia for the distribution and genetic diversity of a marine macroalga. J. Biogeogr. 43, 1595–1607. doi: 10.1111/jbi.12744
Mackas, D. L., Strub, P. T., Thomas, A. C., and Montecino, V. (2006). “Eastern regional ocean boundaries pan-regional overview,” in The Sea, eds A. R. Robinson and R. Brink (Cambridge, MA: Harvard University Press), 21–60.
Mendelssohn, R., and Schwing, F. B. (2002). Common and uncommon trends in SST and wind stress in the California and Peru-Chile current systems. Prog. Oceanogr. 53, 141–162. doi: 10.1016/S0079-6611(02)00028-9
Raftery, A. E., Zimmer, A., Frierson, D. M. W., Startz, R., and Liu, P. (2017). Less than 2 °C warming by 2100 unlikely. Nat. Clim. Change 7, 637–641. doi: 10.1038/nclimate3352
Renault, L., Deutsch, C., McWilliams, J. C., Frenzel, H., Liang, J.-H., and Colas, F. (2016). Partial decoupling of primary productivity from upwelling in the California Current system. Nat. Geosci. 9, 505–508. doi: 10.1038/NGEO2722
Roemmich, D., Church, J., Gilson, J., Monselesan, D., Sutton, P., and Wijffels, S. (2015). Unabated planetary warming and its ocean structure since 2006. Nat. Clim. Change 5:240. doi: 10.1038/nclimate2513
Rouault, M., Pohl, B., and Penven, P. (2010). Coastal oceanic climate change and variability from 1982 to 2009 around South Africa. Afr. J. Mar. Sci. 32, 237–246. doi: 10.2989/1814232X.2010.501563
Rykaczewski, R. R., and Dunne, J. P. (2010). Enhanced nutrient supply to the California Current Ecosystem with global warming and increased stratification in an earth system model. Geophys. Res. Lett. 37:L21606. doi: 10.1029/2010GL045019
Rykaczewski, R. R., Dunne, J. P., Sydeman, W. J., García-Reyes, M., Black, B. A., and Bograd, S. J. (2015). Poleward displacement of coastal upwelling-favorable winds in the ocean’s eastern boundary currents through the 21st century. Geophys. Res. Lett. 42, 6424–6431. doi: 10.1002/2015GL064694
Santos, F., deCastro, M., Gómez-Gesteira, M., and Álvarez, I. (2012a). Differences in coastal and oceanic SST warming rates along the Canary upwelling ecosystem from 1982 to 2010. Cont. Shelf Res. 47, 1–6. doi: 10.1016/j.csr.2012.07.023
Santos, F., Gómez-Gesteira, M., deCastro, M., and Álvarez, I. (2012b). Differences in coastal and oceanic SST trends due to the strengthening of coastal upwelling along the Benguela current system. Cont. Shelf Res. 34, 79–86. doi: 10.1016/j.csr.2011.12.004
Seabra, R., Wethey, D. S., Santos, A. M., Gomes, F., and Lima, F. P. (2016). Equatorial range limits of an intertidal ectotherm are more linked to water than air temperature. Glob. Change Biol. 22, 3320–3331. doi: 10.1111/gcb.13321
Send, U., Beardsley, R. C., and Winant, C. D. (1987). Relaxation from upwelling in the coastal ocean dynamics experiment. J. Geophys. Res. 92, 1683–1698. doi: 10.1029/JC092iC02p01683
Sydeman, W. J., García-Reyes, M., Schoeman, D. S., Rykaczewski, R. R., Thompson, S. A., Black, B. A., et al. (2014). Climate change and wind intensification in coastal upwelling ecosystems. Science 345, 77–80. doi: 10.1126/science.1251635
van den Hoek, C., and Donze, M. (1967). Algal phytogeography of the European Atlantic coasts. Blumea 15, 63–85.
Keywords: climate change, biogeography, refugia, coastal, intertidal, thermal landscape, thermal stress
Citation: Seabra R, Varela R, Santos AM, Gómez-Gesteira M, Meneghesso C, Wethey DS and Lima FP (2019) Reduced Nearshore Warming Associated With Eastern Boundary Upwelling Systems. Front. Mar. Sci. 6:104. doi: 10.3389/fmars.2019.00104
Received: 23 December 2018; Accepted: 20 February 2019;
Published: 08 March 2019.
Edited by:
Thomas Wernberg, The University of Western Australia, AustraliaReviewed by:
Robert William Schlegel, Dalhousie University, CanadaJoachim Ribbe, University of Southern Queensland, Australia
Copyright © 2019 Seabra, Varela, Santos, Gómez-Gesteira, Meneghesso, Wethey and Lima. This is an open-access article distributed under the terms of the Creative Commons Attribution License (CC BY). The use, distribution or reproduction in other forums is permitted, provided the original author(s) and the copyright owner(s) are credited and that the original publication in this journal is cited, in accordance with accepted academic practice. No use, distribution or reproduction is permitted which does not comply with these terms.
*Correspondence: Fernando P. Lima, ZnBsaW1hQGdtYWlsLmNvbQ==