- 1Department of Biology, Norwegian University of Science and Technology (NTNU), Trondheim, Norway
- 2Department of Biotechnology and Food Science, Norwegian University of Science and Technology (NTNU), Trondheim, Norway
- 3SINTEF Ocean, Trondheim, Norway
Microalgae are regarded as a promising alternative that can replace fishmeal and fish oil in aquaculture. Under N-limitation, many microalgae species change their carbon storage patterns in favor of neutral lipids (NLs) mainly in the form of triacylglycerol (TAG), but fatty acids in polar lipids (PL) are nutritionally more available for fish than those esterified into NLs. In the present study, the effect of N-limitation on the lipid content and fatty acid profiles in different lipid classes of Phaeodactylum tricornutum, Isochrysis aff. galbana clone T-Iso, Rhodomonas baltica, and Nannochloropsis oceanica were investigated. The microalgae cells were cultivated by two different methods, batch and semi-continuous culture, to create strong and moderate N-limitation, and this in turn will significantly affect the biomass and lipid productivity. All four species accumulated lipids mainly in the form of TAG, in response to strong nitrogen limitation. N. oceanica, however, accumulated 51% of the dry weight as lipid in moderate nitrogen limitation and up to 87% of the fatty acid was in TAG. Isochrysis aff. galbana clone T-Iso was the only species where the fraction of polyunsaturated fatty acid (PUFA), especially the fraction of docosahexaenoic acid (DHA), increased with increasing nitrogen limitation. Total lipid productivity showed no increase in batch culture although stronger nitrogen limitation led to lipid accumulation. P. tricornutum had the highest eicosapentaenoic acid (EPA) content, while N. oceanica showed the highest EPA productivity due to the high content of lipid. The highest DHA productivity was found in Isochrysis aff. galbana clone T-Iso from moderate N-limitation, mainly due to the high biomass productivity. Based on the results from the current study, N. oceanica and T-Iso are two promising microalgae strains ass long-term sustainable sources of n-3 long chain -PUFAs under moderate N-limitation. As shown in the present study, increased lipid content in microalgal cells due to strong N-limitation induction may not increase the lipid productivity because biomass production is usually reduced. Therefore, a combination of approaches such as metabolic engineering, conditioning and selection may be needed to further increase the n-3 LC-PUFA productivity without substantial loss of biomass.
Introduction
Fish oils are used as a lipid source fish feed due to the high content of long-chain polyunsaturated fatty acids (LC-PUFA). However, the global supply of fish oils is limited and it cannot meet the future demands for aquaculture and human consumption (Salem and Eggersdorfer, 2015). As a result, alternative raw materials are increasingly being evaluated for use in aquafeed formulation (Chauton et al., 2014).
Many efforts have been made to replace fish oils with vegetable oils in formulated fish feed, but the major limit of using vegetable oils is their lack of n-3 LC-PUFA. Microalgae, on the other hand, are natural primary producers of n-3 LC-PUFA (Shah et al., 2018). The typical EPA-producing microalgae species include Nannochloropsis spp, Monodus subterraneus, Nitzschia spp., and the model diatom P. tricornutum (Wen and Chen, 2000; Lu et al., 2001; Hu and Gao, 2003; Yang et al., 2013). Whereas, the typical DHA-producing species include Isochrysis galbana (Reitan et al., 1994; Fidalgo et al., 1998) and the thraustochytrids Aurantochytrium spp. (Taoka et al., 2009) Thraustochytrium spp. and Schizochytrium spp. (Wang et al., 2018). Moreover, microalgae with high nutritional values are widely used in aquaculture, either for direct consumption for molluscs and shrimp, or indirectly through live feed for fish larvae (Reitan et al., 1997; Brown and Robert, 2002). Previous studies demonstrated that microalgae contain on average 30–40% protein, 10–20% lipids, and 5–15% carbohydrates (Brown et al., 1997; Reitan et al., 1997). Some species, for instance Nannochloropsis spp., contain as much as 37–60% (by DW) lipids and are identified as strong candidates for lipid production (Doan et al., 2011).
Phylogenetic affiliation to a large extent determines the potential lipid content and fatty acid profile (Cañavate et al., 2016), but biochemical composition of the algal biomass can be modulated by varying growth conditions (Reitan et al., 1994; Chen et al., 2011; Fakhry and El Maghraby, 2015; Vu et al., 2016). Research on nitrogen limitation has been conducted for decades, as it is one of the most important strategies to increase triacylglycerol (TAG) content (Chen et al., 2015). Under N-limitation, many microalgae species change their carbon storage patterns in favor of neutral lipids (NLs) mainly in the form of TAG (Reitan et al., 1994; Illman et al., 2000; Rodolfi et al., 2009). In N. oceanica, the lipid content doubled under N-depletion compared to N-repletion, and glycerolipids (mainly TAG) increased from 8.3 to 44.2% (Jia et al., 2015). The EPA content in TAG increased from 3.6 μmol g−1 DW under N-repletion to 21 μmol g−1 DW under N-limitation, and the accumulation of EPA was correlated with the degradation of EPA-containing membrane glycerolipids (Jia et al., 2015). The accumulation of TAG and changes in fatty acid profiles under N-limitation have also been observed in several diatoms, green algae and red algae (Rodolfi et al., 2009; Sharma et al., 2012; Valenzuela et al., 2012; Yang et al., 2013). However, these types of studies mainly focus on comparing nitrogen-deficient to nitrogen-sufficient conditions in one cultivation method (Breuer et al., 2012; Griffiths et al., 2012; Chen et al., 2015). Some studies investigated the lipid content in response to different nitrogen concentrations and temperature in batch culture (Converti et al., 2009; Fakhry and El Maghraby, 2015). The fatty acid profiles of NLs and polar lipids (PLs) have a great impact on the selection of cultivation and processing strategies (Meireles et al., 2003; Huang et al., 2013). For example, when algae are cultured for the supply of PUFAs, FAs in PLs are nutritionally more available for fish than those esterified into NLs (Guedes et al., 2010). There are few studies that focus on lipid accumulation under different degrees of N-limitation and the localization of n-3 LC-PUFA content in different lipid class.
In the present study, the effect of N-limitation on the lipid content and fatty acid profiles in different lipid class of P. tricornutum, Isochrysis aff. galbana clone T-Iso, R. baltica, and N. oceanica were investigated. The microalgae cells were cultivated by two different methods, batch and semi-continuous culture, to create strong and moderate N-limitation, and this in turn will significantly affect the biomass and lipid productivity. Because EPA and DHA are the two fatty acids with the highest value when considering microalgae as an alternative source to fish oil, the production and the localization of these fatty acids were also studied.
Materials and Methods
Microalgae and Culture Medium
Four microalgae species (1) Phaeodactylum tricornutum CCMP 2561, (2) Isochrysis aff. galbana clone T-Iso CCAP 927/14, (3) Rhodomonas baltica NIVA-5/91, and (4) Nannochloropsis oceanica CCMP1779 were investigated in the present study. Stock cultures of Phaeodactylum tricornutum CCMP 2561 and Rhodomonas baltica NIVA-5/91 were from SINTEF Ocean, Trondheim, while Isochrysis aff. galbana clone T-Iso CCAP 927/14 and Nannochloropsis oceanica CCMP1779 were from NTNU, Center of Fisheries and Aquaculture. Prior to each experiment microalgae were grown in cell culture flasks with f/2 medium (Guillard, 1975) at 20°C for about 3 days. Seawater was filtered (0.22 μm) and autoclaved before use.
Cultivation Methods
To obtain micraolgae cells with strong and moderate N-limitation, we analyzed cells from late stationary phase (μ < 0.05 μmax) in batch culture (BT) and from steady state (μ = 0.5 μmax) in semi-continuous culture (SC).
In BT, preacclimated aliquots of P. tricornutum, T-Iso, and R. baltica-cultures were inoculated into 2.4 L modified f/2 medium (10% of the original amount of sodium nitrate and 25% more sodium phosphate than the original receipt) and divided into two 1.2 L glass cylinders (LWS 05, Inst. Getreideverarbeitung GmbH, Germany). In order to have enough samples for further analysis, N. oceanica were inoculated into 8 L of modified f/2 medium and divided into 8 cylinders. Cultures were constantly illuminated by artificial light, with an average light intensity of about 150 μmol photons m−2s−1 at the culture surface. The temperature was kept constant at 22°C. In the first 2 days of cultivation, filtered air was supplied to the cultures. From day 3, the filtered air was mixed with 650 ppm CO2, and pH was thereafter maintained around 8.5. The supply of filtered air, together with magnetic stirring ensured proper mixing in the cultures. Cultures were grown for 7 or 8 days, depending on species, until reaching the stationary phase.
In SC, about 100 mL stock cultures from BT were inoculated into the same cultivation system, with parallel cultures for P. tricornutum, T-Iso and R. baltica, and 8 replicates for N. oceanica. Algae in the SCs were grown at a specific growth rate equal to 0.5μ max by controlling the dilution rate. Daily dilution with harvesting at the same time each day started from day 4 or 5. Algal cells were collected as described for BT when the cultures reached a steady state phase at day 7 or 8. This was continued for about 20 days to collect a sufficient biomass for later analysis. The biomasses from all samplings were pooled together.
Harvesting
Algal cells were harvested by centrifugation at 10,000 g for 10 min at 4°C. The supernatant was discarded and cell pellets were transferred into 5 mL centrifuge tubes, rinsed with distilled water and centrifuged at 10,000 g (Jouan KR 22i centrifuge) for 10 min at 4°C. N2 gas was added to cell pellets and these were then stored at −80°C for further analysis. Parallel cultures of each microalgae were pooled together during the centrifugation to obtain enough material for analyses.
Determination of Maximum Growth Rate and Dilution Rate for SC
About 5 mL cultures from each cylinder were sampled daily to follow the growth. Optical density (OD) was measured in a spectrophotometer (UviLine 9100, Schott® Instruments, Germany) at 750 nm. Cell numbers of P. tricornutum, T-Iso, and R. baltica were counted by coulter counter (Multisizer™ 3 Coulter Counter®, Beckman Coulter Inc., Miami, FL, USA) and N. oceanica was counted in Hemocytometer under microscope.
Maximum growth rates of the algae (μmax) in early exponential phase were calculated (Reitan et al., 1994) and the dilution rate of SC was set at 50% of μmax. In steady state dilution cultures, the growth rate was controlled by the dilution rate (Reitan et al., 1994).
Carbon and Nitrogen Analysis
For C and N analysis, exact volumes (10–15 mL) of the cultures were filtered through pre-combusted (480°C, 2 h) Whatman GF/C glass fiber filters and stored at −20°C. Before C and N analysis, filters were exposed to vapor of concentrated hydrochloric acid for 15 min to remove inorganic carbon. The filters were packed into tin capsules and dried at 60°C for 48 h. The analysis of C and N were done in an Elemental Combustion System CHNS-O (Costech ECS, model 4010, Costech International, Firenze, Italy).
Total Lipids and Fatty Acids Analysis
Total lipids were extracted by homogenizing cells in chloroform/methanol (2:1, v/v), filtering through a glass fiber filter before adding 0.88% potassium chloride to the mixture. After centrifugation at 1,640 g for 10 min, the hypophase was collected and evaporated under N2. The total lipid content was then determined gravimetrically (Folch et al., 1957). Fatty acid methyl esters (FAME) were prepared from total lipid by acid-catalyzed transesterification at 50°C for 16 h (Christie, 2003), and FAME was extracted and quantified with C19:0 as an internal standard by a gas chromatograph (AutoSystem XL, Perkin Elmer, Waltham, MA) with Total Chrom Version 6.3.1 software. The system was equipped with an auto-injector (1 μl, inlet temperature 250°C) and a flame ionization detector (FID, 280°C). The temperature program for the oven was 90°C for 1 min, then raised to 150°C at 30°C min−1 and finally raised to 225°C at 3°C min−1 and held for 7 min. Helium was used as the carrier gas, and the fused silica capillary column was coated with chemically bound polyethylene glycol (CP-Wax 52CB, 25 m × 0.25 mm i.d; Varian, Palo Alto, CA).
Triacylglycerols and Polar Lipids Analysis
Triacylglycerols (TAG) and polar lipids (PL) were separated on HPTLC silica gel 60 plates (10 × 10 cm, Merck KGaA Damstadt, Germany). Each plate was activated at 110°C for 1 h and 20 μl of the extracted lipid (10 mg mL−1 in chloroform/methanol 2:1, v/v) was applied as a 1 cm streak by a LINOMAT IV sprayer (CAMAG, Mutlenz, Switzerland). The plate with lipids was developed in hexane: diethylether: acetic acid (70:30:1, v/v) and visualized under UV light, after spraying with 1 mg mL−1 2,7-dichlorofluorescein in methanol/water (95:5, v/v) containing 0.1 mg mL−1 BHT. Triacylglycerols and polar lipids were scraped into glass tubes and acid-catalyzed transesterification was performed as described above. The FAMEs were washed with 2% potassium bicarbonate, concentrated under N2, and quantified by gas chromatograph as described above.
Calculation of Biomass and Lipid Productivity
The dry weight (DW) of the microalgae were calculated as:
where 2.45 is a constant number (Huo et al., 2015).
The biomass productivity per day (P) of SC cultures were calculated as:
where ΔV is the daily diluted volume and culture volume is the total volume of culture. Productivity in the BT cultures were calculated as the biomass at harvest divided by the number of cultivation days. The productivity value was further used to calculate lipid, EPA, and DHA productivity.
Statistical Analyses
Mean ± standard error of the mean (SEM) is presented, and differences in μmax between different species were tested with one-way ANOVA and Tukey's multiple comparison test. Differences in means of N:C ratio, C and N cell−1, total lipid content and productivity were tested for each species between two cultivation methods with a t-test in SPSS (IBM SPSS statistics 24). Means of fatty acid in the same species between two cultivation methods were tested with a t-test, and % fatty acid was transformed to arcsin square root prior to t-test. Means of maximum specific growth rate (μmax d−1) between species were compared with one-way ANOVA followed by Tukey's multiple comparison tests. All tables were made in Excel 2016 and figures in SIGMA PLOT 14.0 (Systat Software Inc).
Result
Growth Parameters
Batch cultivation was conducted over 7 days for P. tricornutum, T-Iso, and R. baltica, and over 8 days for N.oceanica (Figure 1). All four microalgae species grew exponentially and entered the stationary phase before harvesting. Optical density was measured during the cultivation, and the measurements were linearly correlated with the growth in cell numbers of the cultures in all four microalgae species (R2 = 0.93–0.99). For R. baltica, T-Iso, and N.oceanica the μmax were found between day two and three, and for P. tricornutum between day three and four (Table 1). P. tricornutum had the highest μmax and N. oceanica had the lowest (p < 0.05).
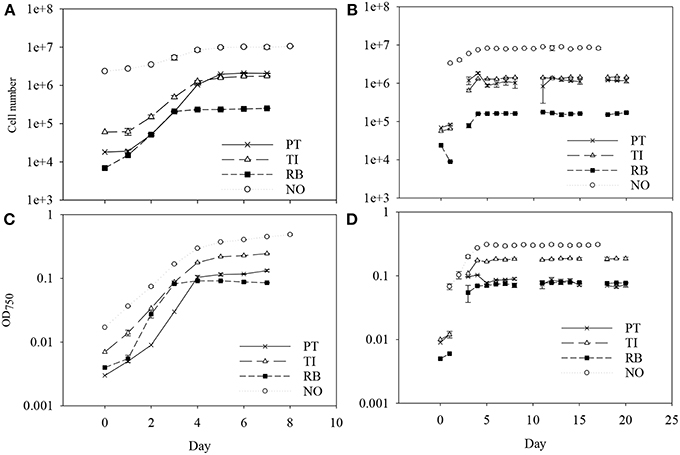
Figure 1. The cell number and the optical density for batch culture (A,C) and for semicontinuous culture (B,D) of four marine microalgae species cultured under nitrogen limitation. Mean with SE bars. PT, P. tricornutum; TI, Isochrysis aff. galbana clone T-Iso; RB, Rhodomonas baltica; NO, N. oceanica.
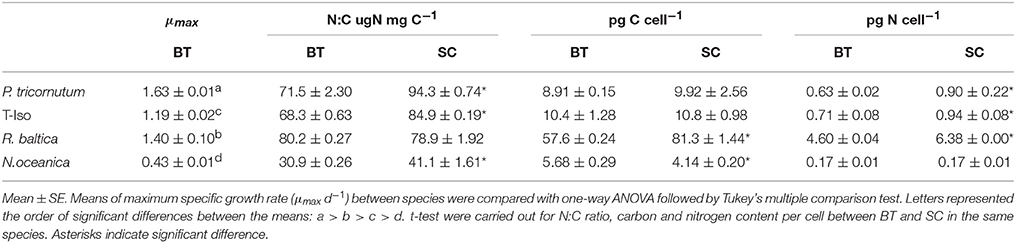
Table 1. Maximum specific growth rate (μmax d−1), N:C ratio, carbon, and nitrogen content per cell for four microalgae species from batch and semi-continuous culture.
From day five or six, daily harvesting (according to 50% of μmax) in the SC cultures was initiated, and the cell density was nearly the same from day to day (Figure 1). This shows a steady state growth, where the growth rate is determined by the dilution rate (D) and the cells are chemically equal from day to day.
Total Content of Carbon, Nitrogen, and Lipid
The N:C ratio of P. tricornutum, T-Iso and N. oceanica was 24–33% higher in SC than in late stationary phase in BT (p < 0.05). No significant difference was found for R. baltica (p > 0.05) (Table 1). The C content per cell was significantly higher in SC compared to BT for R. baltica, whereas it was 42% higher in BT for N.oceanica (p < 0.05). The N content per cell was 32–42% higher in SC than in BT for P. tricornutum, T-Iso and R. baltica (p < 0.05), while it was the same for N.oceanica (p > 0.05).
The highest lipid content was found in N. oceanica in SC, whereas P. tricornutum in SC had the lowest content of lipids (Figure 2). The content of total lipid increased by 58–96% in P. tricornutum, T-Iso, and R. baltica with increased N-limitation (p < 0.05). This was the opposite for N. oceanica, where the lipid content was 16% lower in BT compared to SC (p < 0.05).
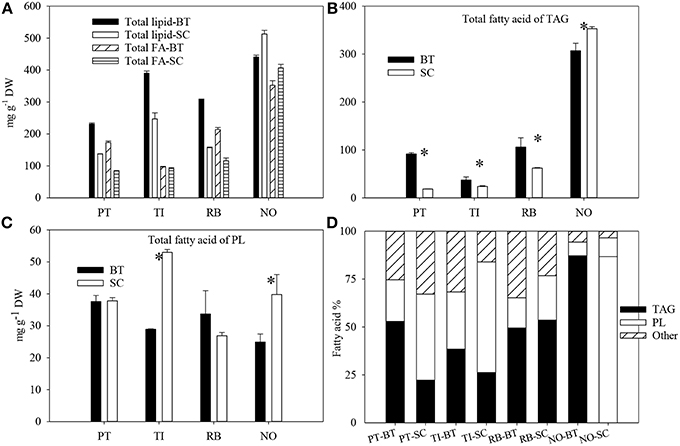
Figure 2. The total lipid content and total fatty acid content (A), total fatty acid content in triacylglycerol (B), total fatty acid content in polar lipids (C), and fatty acid distribution (%) in triacylglycerol, polar lipids and others (D) of four marine microalgae species cultured under nitrogen limitation, by batch (BT) and semi-continuous culture (SC). Mean with SE bars. Asterisk shows significant difference (p < 0.05). PT, P. tricornutum; TI, Isochrysis aff. galbana clone T-ISO; RB, R. baltica; NO, N. oceanica.
Fatty Acid Composition
The FA composition varied between species (Table 2), but all four species in our study showed a high content of 16:0 (palmitic acid). P. tricornutum and N. oceanica had similar FA profiles, dominated by 14:0, 16:0, 16:1n-7, and 20:5n-3 (EPA). Together these FAs contributed with approximately 80 and 86% of total fatty acids in P. tricornutum and N. oceanica, respectively. In addition, N. oceanica had a high percentage of 18:1n-9 (oleic acid) and P. tricornutum had highest percentage of 20:5n-3 (EPA) among the four species. T-Iso and R. baltica had similar FAs profiles, with 14:0, 16:0, 18:1n-9, 18:2n-6, and 18:4n-3 as dominating fatty acids. They represented 63 and 74% of the total FAs, respectively. For R. baltica, the fatty acid 18:3n-3 was found at a high percentage, whereas T-Iso had a high percentage of 22:6 n-3.
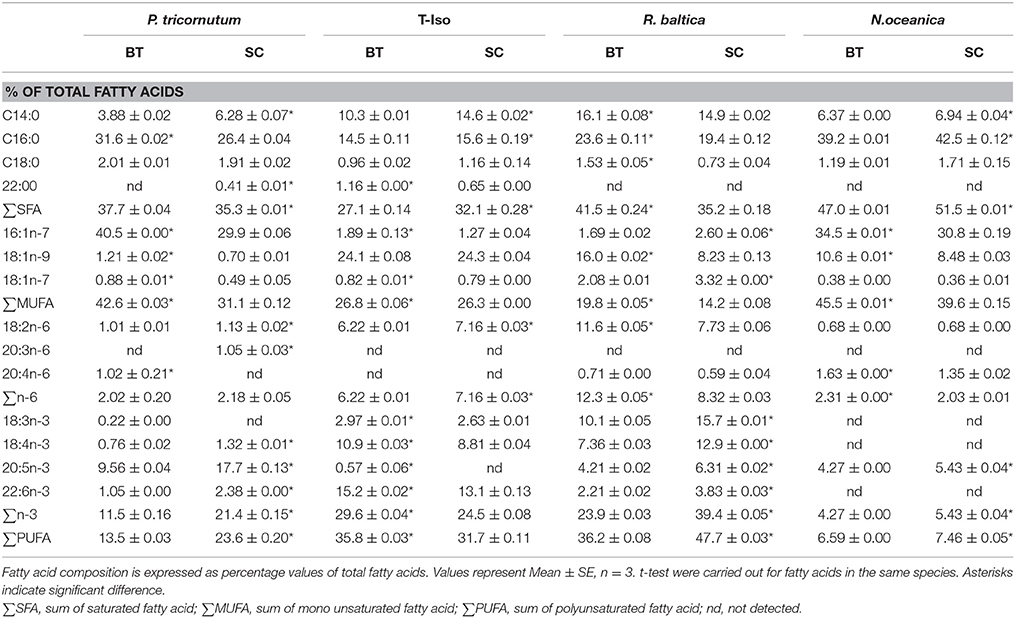
Table 2. Fatty acid profiles (% of total fatty acids) of four microalgae species from batch and semi-continuous culture.
For P. tricornutum, the percentage of monounsaturated fatty acid (MUFA) increased by 36%, whereas PUFA decreased by 43% in BT compared to SC (p < 0.05). In T-Iso the response in distribution of fatty acids to strong N-limitation was: 16% decrease in SFA, 13% increase in PUFA, and no change in MUFA (p < 0.05). N. oceanica also showed a decrease in SFA and PUFA, of 8 and 12%, respectively, in BT compared to SC (p < 0.05). Opposite to T-Iso there was a 15% increase in MUFA (p < 0.05).
In R. baltica, SFA and MUFA increased by 18 and 39%, respectively, whereas PUFA decreased by 24% with increasing N-limitation (p < 0.05). The percentage of EPA decreased by 21–45% in P. tricornutum, R. baltica, and N. oceanica, whereas DHA increased by 16% in T-Iso with increasing N-limitation (p < 0.05).
Fatty Acid Composition in Triacylglycerols and Polar Lipids
The FA content of TAG in the four species were similar to that of total lipids, and the FA content was higher in BT compared to SC except for N. oceanica (p < 0.05) (Figure 2). The total fatty acids of polar lipids (PL) in T-Iso and N. oceanica decreased by 45 and 37% respectively (p < 0.05), with increased N-limitation, whereas it showed no changes in R. baltica and P. tricornutum (p > 0.05). The fatty acids were localized mainly in TAG in P. tricornutum and T-Iso in BT, and mainly in PL in SC. For N. oceanica, 86–87% of the fatty acids were localized in TAG both in BT and in SC.
The dominating fatty acids in TAG in P. tricornutum were 16:0 and 16:1n-7, and EPA was found at a low percentage (Table 3). The fatty acids 16:0, 18:1n-9, 18:2n-6, 18:4n-3, and 22:6n-3 were the dominating fatty acids of TAG in T-Iso, and only 18:4n-3 increased with increasing N-limitation (p < 0.05). The fatty acids 14:0, 16:0, 18:1n-9, 18:2n-6, 18:3n-3, and 18:4n-3 predominated the fatty acid profiles of TAG in R. baltica, and EPA was found in a low amount, similar to P. tricornutum. All n-3 PUFAs in R. baltica increased in BT (p < 0.05). N. oceanica had the highest fatty acid content in TAG among the four species, with 16:0, 16:1n-7, and 18:1n-9 as dominating fatty acids.
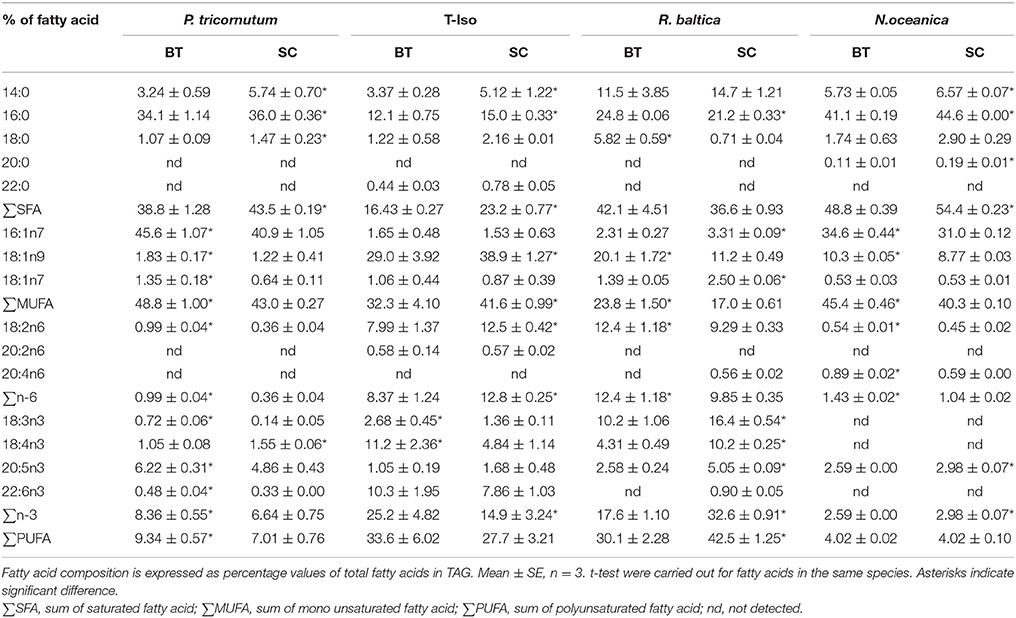
Table 3. Fatty acid profiles in triacylglycerol (TAG) of four microalgae species cultured under nitrogen limitation, by batch (BT), and semi-continuous culture (SC).
The dominating fatty acids in PL in P. tricornutum were 16:0, 16:1n-7, and 20:5n-3, and they accounted for 62–68% of the total fatty acids in PL (Table 4). The fatty acid 16:0 remained the same, whereas 16:1n-7 and 20:5n-3 decreased by 11 and 16% (p < 0.05), respectively in BT. 14:0, 16:0, 18:1n-9, 18:4n-3, and 22:6n-3 fatty acids were the dominating fatty acids of PL in T-Iso, and they showed a slight decrease or remained the same in BT, except for the 14% increase in 18:1n-9 (p < 0.05). For R. baltica, a 51–77% decrease in all n-3-PUFAs was observed in BT (p < 0.05). 16:0, 18:0, 16:1n-7, 18:1n-9, 20:4n-6, and 20:5n-3 were the dominating fatty acids of PL in N. oceanica, and all fatty acids had no significant differences in BT compared to SC (p > 0.05).
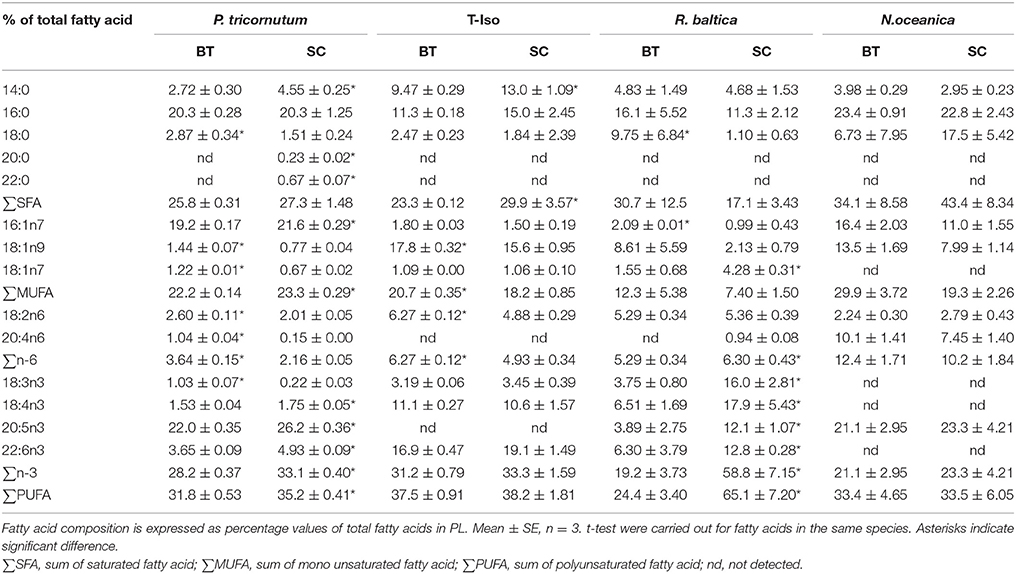
Table 4. Fatty acid profiles in polar lipid (PL) of four microalgae species cultured under nitrogen limitation, by batch (BT) and semi-continuous culture (SC).
Biomass and Lipid Productivity
The microalgae species that had the highest biomass productivity was N. oceanica at the late stationary phase with strong N-limitation (Figure 3). The major difference between the two cultivation methods was found in R. baltica. The biomass productivity decreased by 60–67% (p < 0.05) in BT in all microalgae species except for N. oceanica.
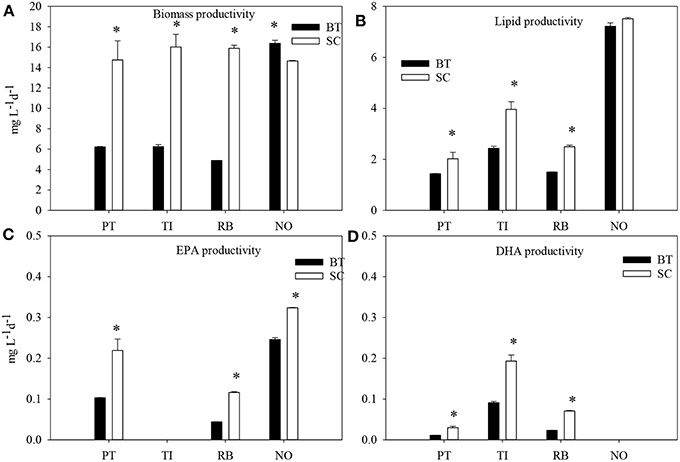
Figure 3. The biomass productivity (A) lipid productivity (B), EPA productivity (C) and DHA productivity (D) of four marine microalgae species cultured under nitrogen limitation, by batch (BT) and semi-continuous culture (SC). PT, P. tricornutum; TI, Isochrysis aff. galbana clone T-Iso; RB, R. baltica; NO, N. oceanica. Mean values with SE bars. t-test were carried out for productivity between BT and SC in the same species. Asterisks indicate significant difference.
The highest lipid production was obtained in N. oceanica in SC and lowest in P. tricornutum and R. baltica in SC, 7.51 and 1.43 and 1.50 mg L−1d−1, respectively. The lipid productivity decreased in BT, compared with SC in all microalgae species except N. oceanica. The production of EPA doubled in P. tricornutum and R. baltica and increased by 31% in N. oceanica in SC (p < 0.05). The productivity of DHA doubled in P. tricornutum and T-Iso, and increased by three times in R. baltica in SC (p < 0.05). N. oceanica had the highest EPA productivity and T-Iso had the highest DHA productivity, both were in SC.
Discussion
Microalgae may be a valuable feed raw material because of their natural content of PUFAs, but phylogeny and cultivation conditions determine the cellular lipid content. Lipid accumulation correlates with some limiting factors (often N), which in turn may result in a lower biomass yield (and overall lower lipid yield). Production of essential fatty acids from microalgae is therefore a compromise between maximizing the lipid content by modulation of growth conditions without lowering biomass production, or a matter of harvesting cells at the right moment.
The total lipid content varied between the four marine microalgae species tested here, and between the two cultivation methods. Among the four species, N. oceanica had the highest lipid content under moderate N-limitation, representing the high interest in lipid production. P. tricornutum, R. baltica, and T-Iso had higher lipid content in batch culture, indicating that these three species accumulate lipids mainly when strong N-limitation stops cell division (Breuer et al., 2012; Griffiths et al., 2012). However, this trend was the opposite for N. oceanica. The lipid content of N. oceanica in our study was higher than previously found under N-repleted conditions by (Fakhry and El Maghraby, 2015). This suggests that N. oceanica accumulated lipid when growing under N-limitation. The lower lipid content under stronger N-limitation may be due to the improper harvesting time. Lipid per cell reached a maximum at day six and then started to decrease (Sandmann et al., 2018). This indicates that the harvesting of N. oceanica should be done at a time when lipid content is highest. R. baltica showed the greatest difference of lipid content between the two cultivation methods, with nearly a doubling in total lipid from moderate to strong N-limitation. This indicates that R. baltica responds strongest to stronger N-limitation among the four microalgae species, partially because of the phycobilin content (da Silva et al., 2009).
Next to content or yield of lipids, the localization of essential fatty acids is of great interest because of digestibility or processing challenges, and fatty acids are mainly localized in either storage lipids in neutral TAGs or in polar membranes. The fatty acid content of TAG increased dramatically under strong N-limitation in all species except for N. oceanica. This may be attributed to biosynthesis or conversion of existing polar membrane lipids into TAG (Xiao et al., 2013). The fatty acid content of PL decreased with stronger N-limitation in T-Iso and N. oceanica, whereas it increased in R. baltica and was the same in P. tricornutum. When algae are cultured for supply of PUFAs such as EPA and DHA in fish and animal feed, PL are nutritionally more available than those esterified into NL (Guedes et al., 2010). In our study, the percentage of EPA was found in a higher fraction in PL than in TAG in all species, and it decreased with stronger N-limitation. This indicates that EPA was mainly located in PL which decreased with strong N-limitation. T-Iso was found to have high fraction of DHA in PL but the percentage decreased in strong N-limitation. Therefore, microalgae should be cultured under N-repletion conditions to obtain higher EPA and DHA in PL when supplying for n-3 LC-PUFAs in fish feed.
In P. tricornutum, a higher MUFA content (dominated by 16:1n7), was found in strong N-limitation, due to TAG accumulation (Vu et al., 2015). The percentage of PUFA, including EPA which is among the most desired components for aquaculture, decreased almost 50% with stronger N-limitation, but the absolute content of EPA (mg g−1) increased due to increased fatty acid content. This was also found in P. cruentum where N-limitation reduced the synthesis of n-3 PUFA while it induced accumulation of TAG (Breuer et al., 2012; Qiao et al., 2016).
Among the four species, T-Iso was the only species where PUFA content increased in strong N-limitation due to the increase in 18:4n-3 and 20:4n-6. We also found that T-Iso had the highest DHA content of the four species of microalgae (Harrison et al., 1990; Reitan et al., 1994; Breuer et al., 2012). The percentage of DHA in TAG increased by 30% with stronger N-limitation, which resulted in an increase of DHA in total lipid. Our study suggested that stronger N-limitation gave a desired increase in PUFA, especially the content of DHA in T-Iso.
In R. baltica, the percentage of MUFA increased with the increase N-limitation, and 18:1n9 was doubled: an effect that was found only in R. baltica. The two fatty acids that dominate the PUFA content were 18:3n3 and 18:3n4, a pattern also found in previous studies of Rhodomonas sp. (Fernández-Reiriz et al., 1989; Patil et al., 2007). On the other hand, PUFA production including EPA and DHA decreased with strong N-limitation. R. baltica was the only species that contained both EPA and DHA of the four studied species. The increase in PUFA in the algae diet would enhance the egg production and somatic growth of copepods (Rasdi and Qin, 2016) and the high EPA/DHA content explains why R. baltica especially is a preferred live feed for copepods in hatcheries (Zhang et al., 2013; Vu et al., 2015).
In N. oceanica, up to 86–87% of the fatty acids were in TAG, which is good for biodiesel production (Chen et al., 2015; Ma et al., 2016). The PUFA content, including EPA, was very low under N-limitation, and showed a slight decrease with stronger N-limitation. The nutrient limitation probably reduced the synthesis of PUFA (Sukenik, 1991; Reitan et al., 1994; Xiao et al., 2013).
Calculation of biomass productivity in batch systems depended on the size of the inoculum and the period until harvesting, and the comparison between different cultures is only a relative indicator. However, some general trends were observed, and the biomass productivity significantly decreased under strong N-limitation in all species except for N. oceanica. This in turn led to lower lipid productivity, although the strong N-limitation increased the lipid content more than 50%. The EPA and DHA productivities are both of great importance for exploitation of marine microalgae in aquaculture. Among the four species, N. oceanica showed the highest EPA productivity and it decreased with stronger N-limitation. The highest DHA productivity was found in T-Iso under moderate N-limitation. Based on the result, N. oceanica and T-Iso are two promising microalgae strains for producing an alternative source of n-3 LC-PUFAs under moderate N-limitation.
Conclusion
As shown in the present study, N. oceanica and T-Iso are two promising microalgae strains for producing an alternative source of n-3 LC-PUFAs under moderate N-limitation. N-limitation led to TAG accumulation, however, increased lipid content in microalgal cells under strong N-limitation may not increase the lipid productivity because biomass production usually is reduced. Therefore, a combination of approaches such as metabolic engineering, conditioning and selection may be required to further increase both lipid and n-3 LC-PUFA content without substantial loss of biomass.
Author Contributions
XW and HF contributed to the running of the experiment, sample and data analysis. XW wrote the manuscript. KL analyzed lipid and fatty acid composition. MC participated in the experimental design and guided in sampling and data analysis and manuscript preparation. OV is the project leader of MIRA that funded the study and he contributed in the experimental design and manuscript writing. KR is the project leader of Micro-Feed which funded the study as well, he contributed in the experimental design and manuscript writing.
Funding
This study was part of two research projects Microbial raw materials as source for protein and EPA and DHA for use in aquafeed, No.248355 and Microbially produced raw materials for aquafeed, No.239001 which are, founded by ERANET-COFASP and Norwegian research council, respectively.
Conflict of Interest Statement
The authors declare that the research was conducted in the absence of any commercial or financial relationships that could be construed as a potential conflict of interest.
Acknowledgments
We thank Åsmund Johansen and Torfinn Solvang from SINTEF Ocean for excellent technical assistance with the cultivation system and Marte Schei and Merethe Selnes for carbon and nitrogen analysis. We also thank the referees for their valuable comments.
References
Breuer, G., Lamers, P. P., Martens, D. E., Draaisma, R. B., and Wijffels, R. H. (2012). The impact of nitrogen starvation on the dynamics of triacylglycerol accumulation in nine microalgae strains. Bioresour. Technol. 124, 217–226. doi: 10.1016/j.biortech.2012.08.003
Brown, M., and Robert, R. (2002). Preparation and assessment of microalgal concentrates as feeds for larval and juvenile Pacific oyster (Crassostrea gigas). Aquaculture 207, 289–309. doi: 10.1016/S0044-8486(01)00742-6
Brown, M. R., Jeffrey, S. W., Volkman, J. K., and Dunstan, G. A. (1997). Nutritional properties of microalgae for mariculture. Aquaculture 151, 315–331. doi: 10.1016/S0044-8486(96)01501-3
Cañavate, J. P., Armada, I., Ríos, J. L., and Hachero-Cruzado, I. (2016). Exploring occurrence and molecular diversity of betaine lipids across taxonomy of marine microalgae. Phytochemistry 124, 68–78. doi: 10.1016/j.phytochem.2016.02.007
Chauton, M., Reitan, K., Norsker, N.-H., Tveterås, R., and Kleivdal, H. (2014). A techno-economic analysis of industrial production of marine microalgae as a source of EPA and DHA-rich raw material for aquafeed: Research challenges and possibilities. Aquaculture 436, 95–103. doi: 10.1016/j.aquaculture.2014.10.038
Chen, M., Tang, H., Ma, H., Holland, T. C., Ng, K. Y. S., and Salley, S. O. (2011). Effects of nutrients on growth and lipid accumulation in the green algae Dunaliella tertiolecta. Bioresour. Technol. 102, 1649–1655. doi: 10.1016/j.biortech.2010.09.062
Chen, Y., Tang, X., Kapoore, R. V., Xu, C., and Vaidyanathan, S. (2015). Influence of nutrient status on the accumulation of biomass and lipid in Nannochloropsis salina and Dunaliella salina. Energy Convers. Manag. 106, 61–72. doi: 10.1016/j.enconman.2015.09.025
Christie, W. W. (2003). Lipid Analysis : Isolation, Separation, Identification and Structural Analysis of Lipids. 3rd edn. Bridgewater, MA: Oily.
Converti, A., Casazza, A. A., Ortiz, E. Y., Perego, P. D., and Borghi, M. (2009). Effect of temperature and nitrogen concentration on the growth and lipid content of Nannochloropsis oculata and Chlorella vulgaris for biodiesel production. Chem. Eng. Process. 48, 1146–1151. doi: 10.1016/j.cep.2009.03.006
da Silva, A. F., Lourenço, S. O., and Chaloub, R. M. (2009). Effects of nitrogen starvation on the photosynthetic physiology of a tropical marine microalga Rhodomonas sp. (Cryptophyceae). Aquatic Bot. 91, 291–297. doi: 10.1016/j.aquabot.2009.08.001
Doan, T. T. Y., Sivaloganathan, B., and Obbard, J. P. (2011). Screening of marine microalgae for biodiesel feedstock. Biomass Bioenergy 35, 2534–2544. doi: 10.1016/j.biombioe.2011.02.021
Fakhry, E. M., and El Maghraby, D. M. (2015). Lipid accumulation in response to nitrogen limitation and variation of temperature in Nannochloropsis salina. Bot. Stud. 56:6. doi: 10.1186/s40529-015-0085-7
Fernández-Reiriz, M. J., Perez-Camacho, A., Ferreiro, M. J., Blanco, J., Planas, M., Campos, M. J., et al. (1989). Biomass production and variation in the biochemical profile (total protein, carbohydrates, RNA, lipids and fatty acids) of seven species of marine microalgae. Aquaculture 83, 17–37. doi: 10.1016/0044-8486(89)90057-4
Fidalgo, J. P., Cid, A., Torres, E., Sukenik, A., and Herrero, C. (1998). Effects of nitrogen source and growth phase on proximate biochemical composition, lipid classes and fatty acid profile of the marine microalga Isochrysis galbana. Aquaculture 166, 105–116.
Folch, J., Lees, M., and Stanley, G. H. S. (1957). A simple method for the isolation and purification of total lipids from animal tissues. J. Biol. Chem. 226, 497–509.
Griffiths, M. J., van Hille, R. P., and Harrison, S. T. L. (2012). Lipid productivity, settling potential and fatty acid profile of 11 microalgal species grown under nitrogen replete and limited conditions. J. Appl. Phycol. 24, 989–1001. doi: 10.1007/s10811-011-9723-y
Guedes, A. C., Meireles, L. A., Amaro, H. M., and Malcata, F. X. (2010). Changes in lipid class and fatty acid composition of cultures of Pavlova lutheri, in response to light intensity. J. Am. Oil Chem. Soc. 87, 791–801. doi: 10.1007/s11746-010-1559-0
Harrison, P. J., Thompson, P. A., and Calderwood, G. S. (1990). Effects of nutrient and light limitation on the biochemical composition of phytoplankton. J. Appl. Phycol. 2, 45–56. doi: 10.1007/BF02179768
Hu, H., and Gao, K. (2003). Optimization of growth and fatty acid composition of a unicellular marine picoplankton, Nannochloropsis sp., with enriched carbon sources. Biotechnol. Lett. 25, 421–425. doi: 10.1023/A:1022489108980
Huang, X., Huang, Z., Wen, W., and Yan, J. (2013). Effects of nitrogen supplementation of the culture medium on the growth, total lipid content and fatty acid profiles of three microalgae (Tetraselmis subcordiformis, Nannochloropsis oculata and Pavlova viridis). J. Appl. Phycol. 25, 129–137. doi: 10.1007/s10811-012-9846-9
Huo, S., Zhou, W., Wang, Z., Zhu, S., Dong, L., Huang, W., et al. (2015). Biomass measurement of microalgae cultivated under photoautotrophic conditions for biofuels. Energy Sour. Part A. 37, 1447–1454. doi: 10.1080/15567036.2012.742597
Illman, A. M., Scragg, A. H., and Shales, S. W. (2000). Increase in Chlorella strains calorific values when grown in low nitrogen medium. Enzyme Microb. Technol. 27, 631–635. doi: 10.1016/S0141-0229(00)00266-0
Jia, J., Han, D., Gerken, H. G., Li, Y., Sommerfeld, M., Hu, Q., et al. (2015). Molecular mechanisms for photosynthetic carbon partitioning into storage neutral lipids in Nannochloropsis oceanica under nitrogen-depletion conditions. Algal Res. 7, 66–77. doi: 10.1016/j.algal.2014.11.005
Lu, C., Rao, K., Hall, D., and Vonshak, A. (2001). Production of eicosapentaenoic acid (EPA) in Monodus subterraneus grown in a helical tubular photobioreactor as affected by cell density and light intensity. J. Appl. Phycol. 13, 517–522. doi: 10.1023/A:1012515500651
Ma, X. N., Chen, T. P., Yang, B., Liu, J., and Chen, F. (2016). Lipid Production from Nannochloropsis. Mar. Drugs 14:E61. doi: 10.3390/md14040061
Meireles, L. A., Guedes, A. C., and Malcata, F. X. (2003). Lipid class composition of the microalga Pavlova lutheri: eicosapentaenoic and docosahexaenoic acids. J. Agric. Food Chem. 51, 2237–2241. doi: 10.1021/jf025952y
Patil, V., Källqvist, T., Olsen, E., Vogt, G., and Gislerød, H. R. (2007). Fatty acid composition of 12 microalgae for possible use in aquaculture feed. Aquacult. Int. 15, 1–9. doi: 10.1007/s10499-006-9060-3
Qiao, H., Cong, C., Sun, C., Li, B., Wang, J., and Zhang, L. (2016). Effect of culture conditions on growth, fatty acid composition and DHA/EPA ratio of Phaeodactylum tricornutum. Aquaculture 452, 311–317. doi: 10.1016/j.aquaculture.2015.11.011
Rasdi, N. W., and Qin, J. G. (2016). Improvement of copepod nutritional quality as live food for aquaculture: a review. Aquacult. Res. 47, 1–20. doi: 10.1111/are.12471
Reitan, K. I., Rainuzzo, J. R., and Øie, G., and Olsen, Y. (1997). A review of the nutritional effects of algae in marine fish larvae. Aquaculture 155, 207–221. doi: 10.1016/S0044-8486(97)00118-X
Reitan, K. I., Rainuzzo, J. R., and Olsen, Y. (1994). Effect of nutrient limitation on fatty acid and lipid content of marine microalgae. J. Phycol. 30, 972–979. doi: 10.1111/j.0022-3646.1994.00972.x
Rodolfi, L., Chini Zittelli, G., Bassi, N., Padovani, G., Biondi, N., Bonini, G., et al. (2009). Microalgae for oil: strain selection, induction of lipid synthesis and outdoor mass cultivation in a low-cost photobioreactor. Biotechnol. Bioeng. 102, 100–112. doi: 10.1002/bit.22033
Salem, N. J., and Eggersdorfer, M. (2015). Is the world supply of omega-3 fatty acids adequate for optimal human nutrition? Curr. Opin. Clin. Nutr. Metab. Care 18, 147–154. doi: 10.1097/MCO.0000000000000145
Sandmann, M., Schafberg, M., Lippold, M., and Rohn, S. (2018). Analysis of population structures of the microalga Acutodesmus obliquus during lipid production using multi-dimensional single-cell analysis. Sci. Rep. 8:6242. doi: 10.1038/s41598-018-24638-y
Shah, M. R., Lutzu, G. A., Alam, A., Sarker, P., Chowdhury, M. A. K., Parsaeimehr, A., et al. (2018). Microalgae in aquafeeds for a sustainable aquaculture industry. J. Appl. Phycol. 30, 197–213. doi: 10.1007/s10811-017-1234-z
Sharma, K. K., Schuhmann, H., and Schenk, P. M. (2012). High lipid induction in microalgae for biodiesel production. Energies 5, 1532–1553. doi: 10.3390/en5051532
Sukenik, A. (1991). Ecophysiological considerations in the optimization of eicosapentaenoic acid production by Nannochloropsis sp. (Eustigmatophyceae). Bioresour. Technol. 35, 263–269. doi: 10.1016/0960-8524(91)90123-2
Taoka, Y., Nagano, N., Okita, Y., Izumida, H., Sugimoto, S., and Hayashi, M. (2009). Influences of culture temperature on the growth, lipid content and fatty acid composition of Aurantiochytrium sp. Strain mh0186. Mar. Biotechnol. 11, 368–374. doi: 10.1007/s10126-008-9151-4
Valenzuela, J., Mazurie, A., Carlson, R. P., Gerlach, R., Cooksey, K. E., Peyton, B. M., et al. (2012). Potential role of multiple carbon fixation pathways during lipid accumulation in Phaeodactylum tricornutum. Biotechnol. Biofuels 5:40. doi: 10.1186/1754-6834-5-40
Vu, M., Jepsen, P., Jørgensen, N., Hansen, W. B., and Nielsen, S. (2015). Laboratory Scale Photobioreactor for High Production of Microalgae Rhodomonas salina Used as Food for Intensive Copepod Cultures (Rotterdam).
Vu, M. T. T., Douëtte, C., Rayner, T. A., Thoisen, C., Nielsen, S. L., and Hansen, B. W. (2016). Optimization of photosynthesis, growth, and biochemical composition of the microalga Rhodomonas salina—an established diet for live feed copepods in aquaculture. J. Appl. Phycol. 28, 1485–1500. doi: 10.1007/s10811-015-0722-2
Wang, Q., Ye, H., Sen, B., Xie, Y., He, Y., Park, S., et al. (2018). Improved production of docosahexaenoic acid in batch fermentation by newly-isolated strains of Schizochytrium sp. and Thraustochytriidae sp. through bioprocess optimization. Synth. Syst. Biotechnol. 3, 121–129. doi: 10.1016/j.synbio.2018.04.001
Wen, Z. Y., and Chen, F. (2000). Production potential of eicosapentaenoic acid by the diatom Nitzschia laevis. Biotechnol. Lett. 22, 727–733. doi: 10.1023/A:1005666219163
Xiao, Y., Zhang, J., Cui, J., Feng, Y., and Cui, Q. (2013). Metabolic profiles of Nannochloropsis oceanica IMET1 under nitrogen-deficiency stress. Bioresour. Technol. 130, 731–738. doi: 10.1016/j.biortech.2012.11.116
Yang, Z. K., Niu, Y. F., Ma, Y. H., Xue, J., Zhang, M. H., Yang, W. D., et al. (2013). Molecular and cellular mechanisms of neutral lipid accumulation in diatom following nitrogen deprivation. Biotechnol. Biofuels 6:67. doi: 10.1186/1754-6834-6-67
Zhang, J., Wu, C., Pellegrini, D., Romano, G., Esposito, F., Ianora, A., et al., (2013). Effects of different monoalgal diets on egg production, hatching success and apoptosis induction in a Mediterranean population of the calanoid copepod Acartia tonsa (Dana). Aquaculture 400–401, 65–72. doi: 10.1016/j.aquaculture.2013.02.032
Keywords: nitrogen, cultivation conditions, marine microalgae, lipids, PUFAs, aquafeed
Citation: Wang X, Fosse HK, Li K, Chauton MS, Vadstein O and Reitan KI (2019) Influence of Nitrogen Limitation on Lipid Accumulation and EPA and DHA Content in Four Marine Microalgae for Possible Use in Aquafeed. Front. Mar. Sci. 6:95. doi: 10.3389/fmars.2019.00095
Received: 06 November 2018; Accepted: 18 February 2019;
Published: 12 March 2019.
Edited by:
António V. Sykes, Centro de Ciências do Mar (CCMAR), PortugalReviewed by:
Francisco Javier Alarcón, University of Almería, SpainNor Azman Kasan, Universiti Malaysia Terengganu, Malaysia
Copyright © 2019 Wang, Fosse, Li, Chauton, Vadstein and Reitan. This is an open-access article distributed under the terms of the Creative Commons Attribution License (CC BY). The use, distribution or reproduction in other forums is permitted, provided the original author(s) and the copyright owner(s) are credited and that the original publication in this journal is cited, in accordance with accepted academic practice. No use, distribution or reproduction is permitted which does not comply with these terms.
*Correspondence: Xinxin Wang, aWFtd3gxMDhAZ21haWwuY29t
†Present Address: Keshuai Li, BioMar AS, Trondheim, Norway